- Red Sea Research Center, Division of Biological and Environmental Science and Engineering (BESE), King Abdullah University of Science and Technology, Thuwal, Saudi Arabia
Climate change, and in particular the unprecedented rapid global warming, presents a major threat to corals, with warming rates potentially exceeding the adaptive capacities of most coral species. Assisted gene flow, the human facilitated introduction of temperature resilience alleles from warmer to threatened colder populations via the movement of individuals (assisted migration) or their gametes (selective breeding), has been suggested as a tool to transfer thermal adaptations among populations. Due to its strong latitudinal temperature gradient and extreme temperature conditions, the Red Sea constitutes an ideal location to investigate the potential of this strategy. Here, we relocated Porites lobata colonies from three reefs along the Saudi Arabian Red Sea with different mean sea surface temperature summer maxima (ranging from 30.9 °C in Duba, 32.5 °C in Thuwal, to 33.8 °C in Jazan) to a common garden experiment in the intermediate central location. Five colonies from each location were fragmented and deployed in situ in early summer of 2018 to investigate physiological differences in bleaching, survival, and growth. Results showed significantly higher bleaching in fragments from Duba, followed by 65% mortality. Even though no bleaching was observed in fragments from Jazan, mortality rates of around 20% indicated that other environmental parameters besides temperature might influence coral health and survival. These results suggest that assisted gene flow via translocation alone may be restricted in its success due to a lack of local adaptations to environmental conditions other than temperature. However, strategies like inter-populational breeding may overcome these limitations as they might allow producing offspring with both increased thermal tolerance and local adaptations.
Introduction
Coral reefs are biodiversity hotspots and provide critical ecosystem services (e.g., food, coastal protection, tourism) to hundreds of millions of people around the world (Cinner et al., 2012; Pendleton et al., 2016). However, these important ecosystems are in rapid decline (Hughes et al., 2017a). Rising ocean temperature caused by climate change stands out as the most severe threat to these ecosystems globally, and it is linked to reduced health, coral cover, and diversity (Hoegh-Guldberg, 1999; Hoegh-Guldberg et al., 2007; Hughes et al., 2017b). According to the latest IPCC report, coral reefs are projected to decline by 70–90% till the end of this century if temperatures reach 1.5 °C above pre-industrial levels, and losses as high as >99% are expected with an increase of 2 °C or more (Bindoff et al., 2019). Thus, considering the biological and socio-economical value of coral reefs, the need to restore and preserve these ecosystems remains imperative (Duarte et al., 2020).
Given the fast pace of climate change, more active management approaches are being explored to ensure the survival of reefs under future conditions (Anthony et al., 2017). Indeed, the field is transitioning from conservation management to active and progressive interventions fostering higher resilience in these systems (National Academies of Sciences Engineering and Medicine, 2019). With the planet facing unprecedented rapid climatic changes, assisted evolution has been proposed as a mechanism to speed up natural evolutionary processes via assisted migration, selective breeding, microbiome manipulation, acclimatization to stress conditions and genetic manipulation (Oppen et al., 2015; Putnam and Gates, 2015; van Oppen et al., 2017; Rosado et al., 2019; Buerger et al., 2020; Howells et al., 2021; Humanes et al., 2021; Quigley et al., 2021; Voolstra et al., 2021; Peixoto et al., 2022; Santoro et al., 2022). Although a dramatic reduction of global carbon emissions remains mandatory to ensure a successful outcome of such strategies, estimates suggest that marine ecosystems could be restored by mid-century (Duarte et al., 2020).
In corals, thermal tolerance varies predictably along temperature gradients (Woolsey et al., 2015) and can differ even at small spatial scales, reflecting habitat thermal regime heterogeneities (Bay and Palumbi, 2014; Palumbi et al., 2014; Camp et al., 2018; Safaie et al., 2018; Thomas et al., 2018). Genetic diversity across depth, distance from shore and human influence levels can contribute to these different phenotypic responses to heat stress (Barshis et al., 2010; Bongaerts et al., 2010; Bongaerts et al., 2013; Lundgren et al., 2013; van Oppen et al., 2018; Jurriaans and Hoogenboom, 2019; Tisthammer et al., 2020; Drury et al., 2022). These physiological differences in coral thermotolerance appear to be heritable (Dixon et al., 2015; Kenkel et al., 2015b; Howells et al., 2021). For instance, up–to–10-fold increase in odds of survival of Acropora millepora larvae under heat stress was seen when at least one parent originated from a warmer lower-latitude reef (Dixon et al., 2015). Thus, assisted gene flow (AGF) via the movement of thermally adapted coral colonies (assisted migration) or their gametes (selective breeding) to vulnerable populations in colder regions has been suggested as a management strategy to increase resilience of threatened populations (Hoegh-Guldberg et al., 2008; Aitken and Whitlock, 2013; Anthony et al., 2017; Morikawa and Palumbi, 2019; Chen et al., 2022). This method has become increasingly relevant since a study has shown that natural long-distance spread of warm-adapted corals is either unlikely or requires over 30 generations (Quigley et al., 2019). AGF theoretically permits increasing thermal tolerance of non-adapted populations under current and future climate change scenarios by increasing the frequency of beneficial alleles (Oppen et al., 2015; van Oppen et al., 2017). Indeed, AGF was successfully verified using cryopreserved sperm in Acropora palmata from different Caribbean populations (Hagedorn et al., 2021) and in Acropora tenuis from the Great Barrier Reef (Daly et al., 2022). While selective breeding involves a more active strategy to introduce beneficial alleles into a population through direct crossing, assisted migration rather relies on the passive introduction of beneficial alleles through the translocation of adult colonies. An important difference between these approaches is that translocated colonies need to survive at the new site until the reproductive period and actively reproduce with local colonies for their beneficial alleles to be introduced into the target population. Hence, assisted migration can simultaneously improve resilience in cooler populations and rescue warmer populations, which are also predicted to exceed their thermal threshold under future scenarios (Riegl et al., 2011).
Coles and Riegl (2013) advocated using coral colonies from the thermo-resilient Gulf, one of the hottest regions with coral reefs, as source populations for assisted migration projects outside the Gulf. Similarly, the Red Sea is a hot environment with even more diverse reef ecosystems (DiBattista et al., 2016) that may provide a resource of thermally tolerant genotypes for a vast number of species. The Red Sea presents a natural temperature gradient, with mean maximum annual sea surface temperature of 33 °C in the south, decreasing northwards to 27 °C in the Gulf of Aqaba (data from 1982 to 2015, Chaidez et al., 2017). Therefore, it is an ideal environment to study thermal adaptation in corals. Coral genetic connectivity across the >4000 km of Red Sea coast is still largely unknown. While (Robitzch et al., 2015) found a lack of genetic structure in Pocillopora verrucosa, some authors argue that the environmental gradients could create ideal conditions for genetically distinct populations of benthic invertebrates (Berumen et al., 2019). In fact, Giles et al. (2015) observed a distinct population of the sponge Stylissa carteri in the south, at around the Farasan Islands, which was explained mainly by its unique environment.
To get insights into how local evolutionary adaptation and phenotypic plasticity influence thermal/stress tolerance and to evaluate the potential of applying assisted migration to Red Sea reefs, we performed a common garden experiment. Colonies of the coral Porites lobata were translocated from the Northern and Southern Red Sea to a central intermediate location, where survival, bleaching, and growth were monitored monthly. Since previous research has found that corals locally adapt to high-temperature environments (Dixon et al., 2015; Woolsey et al., 2015; Thomas et al., 2018; Jurriaans and Hoogenboom, 2019), we hypothesized that colonies from the warmer Southern Red Sea would exhibit higher bleaching resilience than colonies from the Northern or Central Red Sea, thus being a potential source population for assisted migration efforts.
Methods
Coral collection
Porites lobata colonies were collected from Duba (N 27.302158, E 35.639559), Jazan (two sites, N16.899118, E 42.122707; N 17.076619, E 41.927458) and Thuwal (Al Fahal reef, N 22.24816 E 38.96401, just offshore King Abdullah University of Science and Technology, KAUST) in January/February 2018. These locations have different thermal regimes and span approximately 1500 km or ten degrees latitude (Figure 1). Five colonies from each site were collected using hammer and chisel while scuba diving. Colonies collected were at least three meters apart and up to five meters deep. Corals were transported to KAUST wrapped in bubble wrap inside plastic boxes to maintain high moisture levels. At KAUST, corals were immediately placed in 260 L acrylic tanks (27 L/min flow) for one month before being transferred to nurseries on the sheltered site of Al Fahal reef, Thuwal. Colonies were brought back to the tanks at KAUST in April 2018. Water temperature in tanks started at 26 °C and was slowly increased up to 31 °C following the natural warming in the local coral reefs to simulate field conditions (Figure 2). The temperature was recorded every 15 minutes for the experiment’s duration using HOBO temperature loggers (Onset Corp) attached either to the coral tree nurseries© (Nedimyer et al., 2011) or the aquarium tanks.
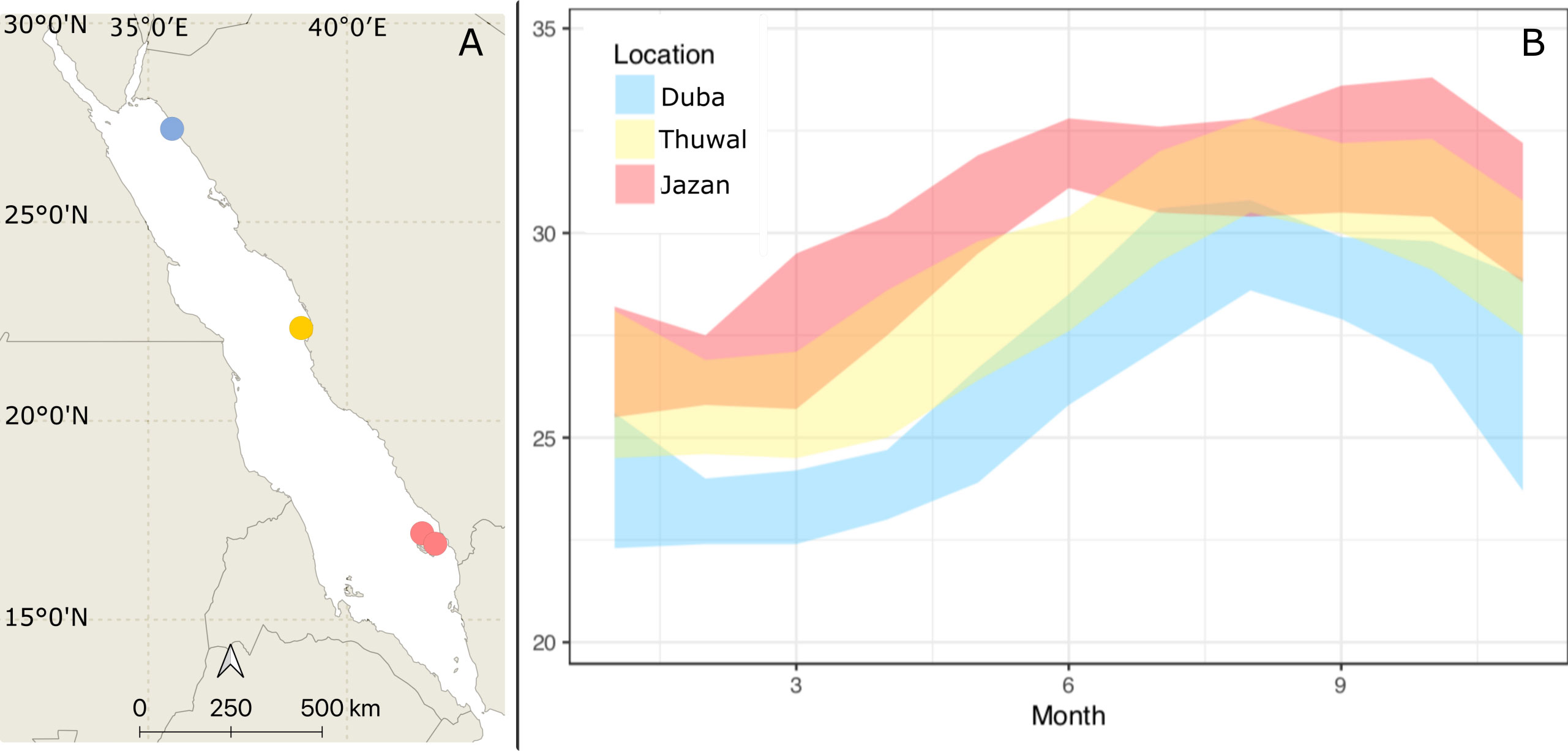
Figure 1 (A) Porites lobata colonies were collected during Winter 2018 at Duba (Northern Red Sea), Thuwal (Central Red Sea), and Jazan (Southern Red Sea). The common garden experiment was deployed at the thermally intermediate location, Thuwal, indicated on the map. (B) Maximum and minimum annual temperatures for the three sampling locations (World sea temperature 2022).
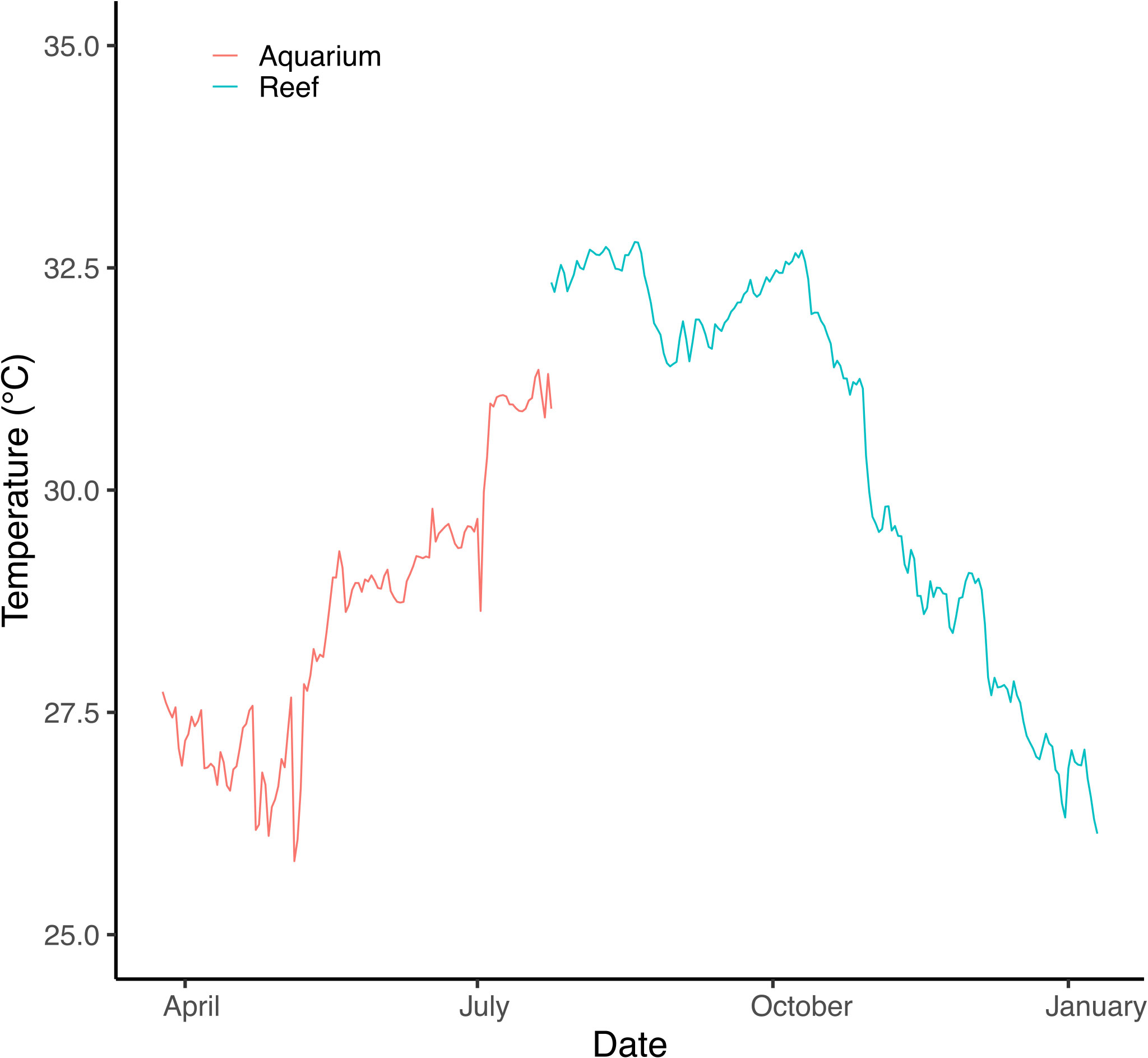
Figure 2 Temperature measured in experimental acclimation tanks and at the common garden field location (Al Fahal, Thuwal). The temperature was slowly increased in experimental tanks to mimic field conditions up to in situ deployment in late July.
Common garden experiment set up and analysis
We split each colony into ten fragments using a rotary tool (Dremel 4000, Dremel, USA). The average area of fragments was 427.39 ± 17.3 mm2 (mean ± SE). Fragments were then attached to custom-made circular glazed ceramic tiles with cyanoacrylate glue and coral epoxy (Aqua Medic) and left in aquaria for three weeks for acclimation. Two coral tree nurseries© were assembled using PVC pipes following the model developed by (Nedimyer et al., 2011). Column length was 70 cm, with alternating PVC arms spaced 20 cm apart. Holes were drilled on the PVC arms where the fragments were attached using cable ties. In total, we distributed 150 fragments onto two nursery trees. Trees were deployed in situ in July 2018, at the thermally intermediate location (Al Fahal reef, Thuwal), at approximately three meters depth. Bleaching and survival were surveyed regularly by scuba diving for eight months. Fragments were considered bleached if they visually appeared completely or partially white (Supplementary Figure 5).
Growth and mortality data was analyzed in the statistical programming language R (R Core Team, 2016) version 4.2.0. Kaplan-Meier survival curves were used to visualize probability of survival for each timepoint, with a right censoring approach. This analysis is used to compare time-to-event, in this case death, of coral fragments. Because it is impossible to know the exact day of death, we assume time of death was the first survey a fragment was seen dead. Survival curves were computed by location and by colony within each location. Pairwise log-rank tests were then performed to test for differences between locations and colonies, with Benjamini-Hochberg correction. Data was analyzed with the package Survminer 0.4.9.
Bleaching data for each colony and location was visualized with ggplot 3.3.5 package (Wickham, 2009). Shapiro-wilk and Levene tests were performed to determine data normality and homoscedasticity. Given the data was not normal and with heterogeneous variance, statistically significant differences in percentage of fragments bleached between locations and colonies were tested monthly using Kruskal-wallis tests with Benjamini-Hochberg correction. Dunn test was then performed for post hoc pairwise comparisons with the FSA 0.9.3 package.
Photos were recorded each month to evaluate surface area growth rates, measured with the software ImageJ. Pictures were taken perpendicular to the coral fragment using a Canon Powershot in a G15 Canon WP-DC48 underwater housing. The daily average growth rate was calculated for each colony and analyzed with Kruskal-wallis test followed by Dunn test with Benjamini-Hochberg correction.
Results
Bleaching
The highest levels of bleaching were observed during August 2018 (p<0.05), after temperatures reached around 33 °C (July-August 2018). Overall, bleaching was significantly higher in fragments from the colder northern location (Duba), affecting up to 75% of the fragments (Figure 3A, p<0.001). On August 14th, bleaching in Duba fragments was significantly higher than Jazan and Thuwal (p<0.05, Table 1). In the subsequent survey, August 23rd, bleaching in Duba fragments was still significantly higher than Jazan (p<0.05), but not Thuwal (Table 1). However, it is important to note that while the majority of Duba fragments were completely bleached, Thuwal fragments were only partially pale and affected areas covered less than 50% of the fragment. Nonetheless, it is interesting to note that bleaching susceptibility was colony specific. While most of the collected colonies from Duba experienced bleaching of 60-100% of their fragments, one particular colony (D13) showed no signs of bleaching at all (Supplementary Figure 4A).
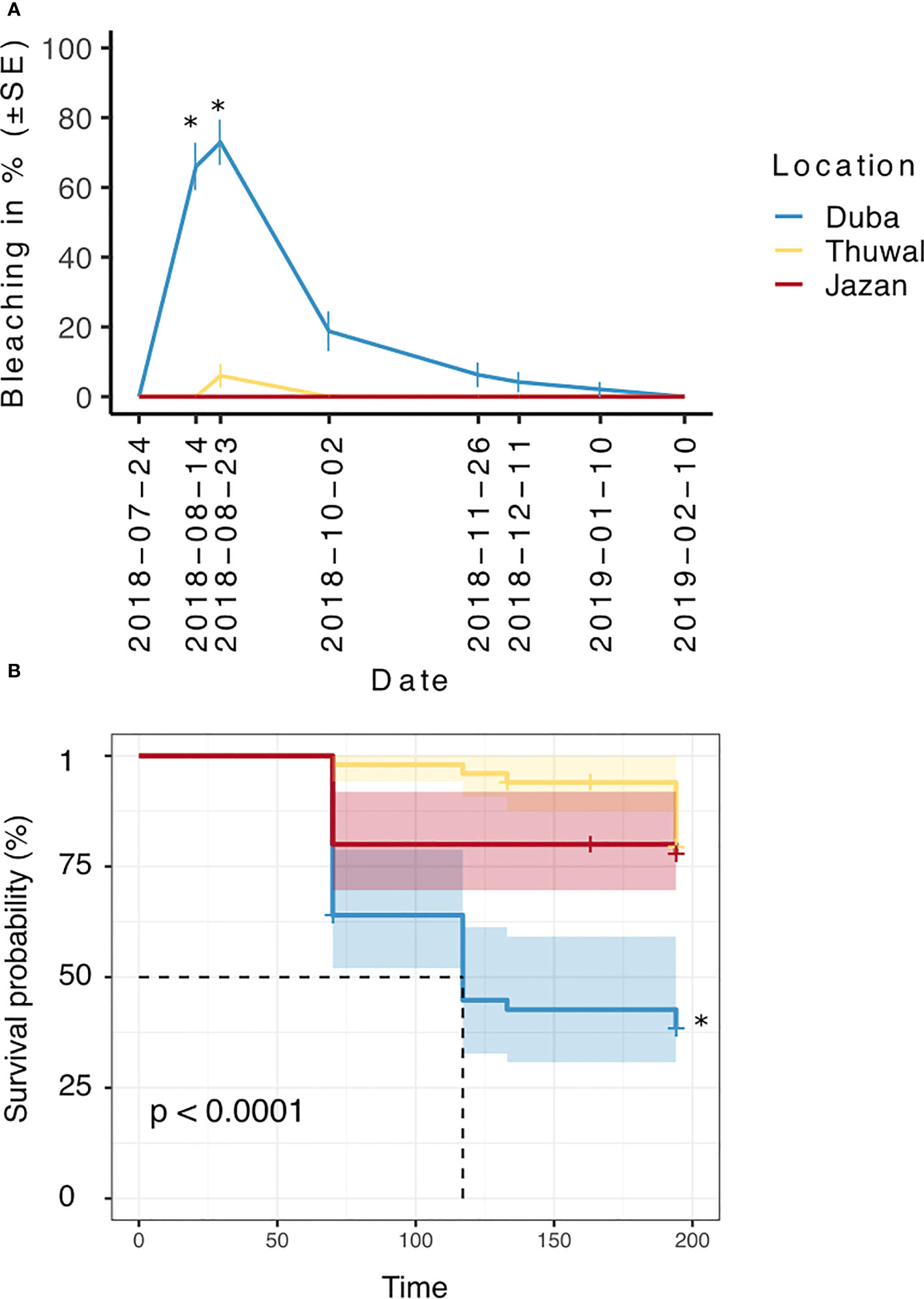
Figure 3 Percentage of bleached (A) and alive (B) fragments of P. lobata colonies collected from three different latitudes (Duba, Thuwal, Jazan) of the Red Sea during a common garden experiment deployed at the intermediate temperature location Thuwal from July 2017 until February 2018. * correspond to significant differences between locations (p < 0.05).
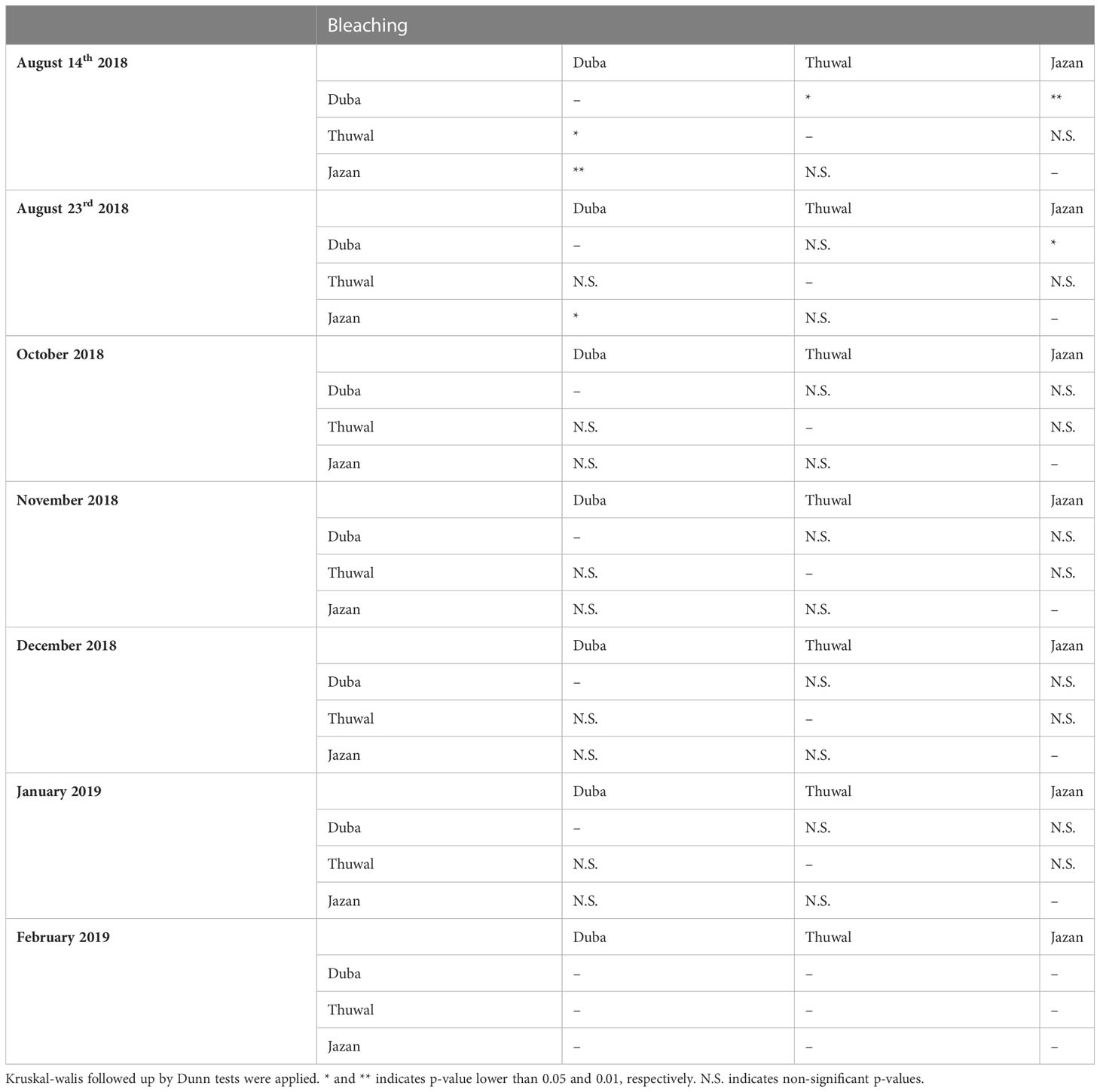
Table 1 Statistically significant differences on bleaching rates of Porites lobata colonies collected from three different latitudes (Duba, Thuwal, Jazan) of the Red Sea during a common garden experiment deployed at the intermediate temperature location Thuwal from July 2017 until February 2018.
In line with our expectations, no bleaching was observed in fragments from the Southern location, Jazan (Figure 3A).
Mortality
Fragments from Duba experienced a steep decrease in survival following bleaching (up to ~35%, Figure 3B). Overall, the survival of Duba fragments was significantly lower than that of fragments from Thuwal and Jazan (p<0.001). Aligned with previous observations on bleaching, survival appeared to be colony-specific (Supplementary Table 2). Colony D13 showed a remarkably high survival rate of 90%, throughout the experiment (Supplementary Figure 4B). Likewise, colony D08 displayed remarkable resilience, reaching 80% fragment survival despite all its fragments having bleached (Supplementary Figure 4B).
Although survival estimates seemed initially higher in Thuwal than Jazan fragments, this was not statistically significant. Despite showing no signs of bleaching, fragments from Jazan experienced a decline in survival of around 20% by October 2018, which remained steady throughout the experiment. Survival rates of fragments from Thuwal remained relatively stable for most of the surveyed months, declining approximately 15% by the end of the experiment, in February 2019 (Figure 3B).
Growth
Overall, average daily growth rates were negative, meaning coral fragments mainly lost tissue throughout the experiment. Growth rates of colonies from Duba, averaged across all fragments, (-2.70 mm2/day ± 0.53, mean ± SE), were significantly lower than those from Jazan (-0.57 mm2/day ± 0.21, mean ± SE, z = -4.064, p<0.001) and Thuwal (-0.45 mm2/day ± 0.43, mean ± SE, z= -3.945, p<0.001, Figure 4A). This difference was more pronounced in the summer months, when averaged growth rates of colony fragments from Duba were lower than those from Thuwal in July-August 2018 (z = -3.195, p=0.004) and lower than those from Thuwal and Jazan in August-October 2018 (z = -3.373, p=0.002 and z = -2.862, p=0.006, respectively). For the following months, there was no difference in growth rates between locations (Supplementary Figure 1).
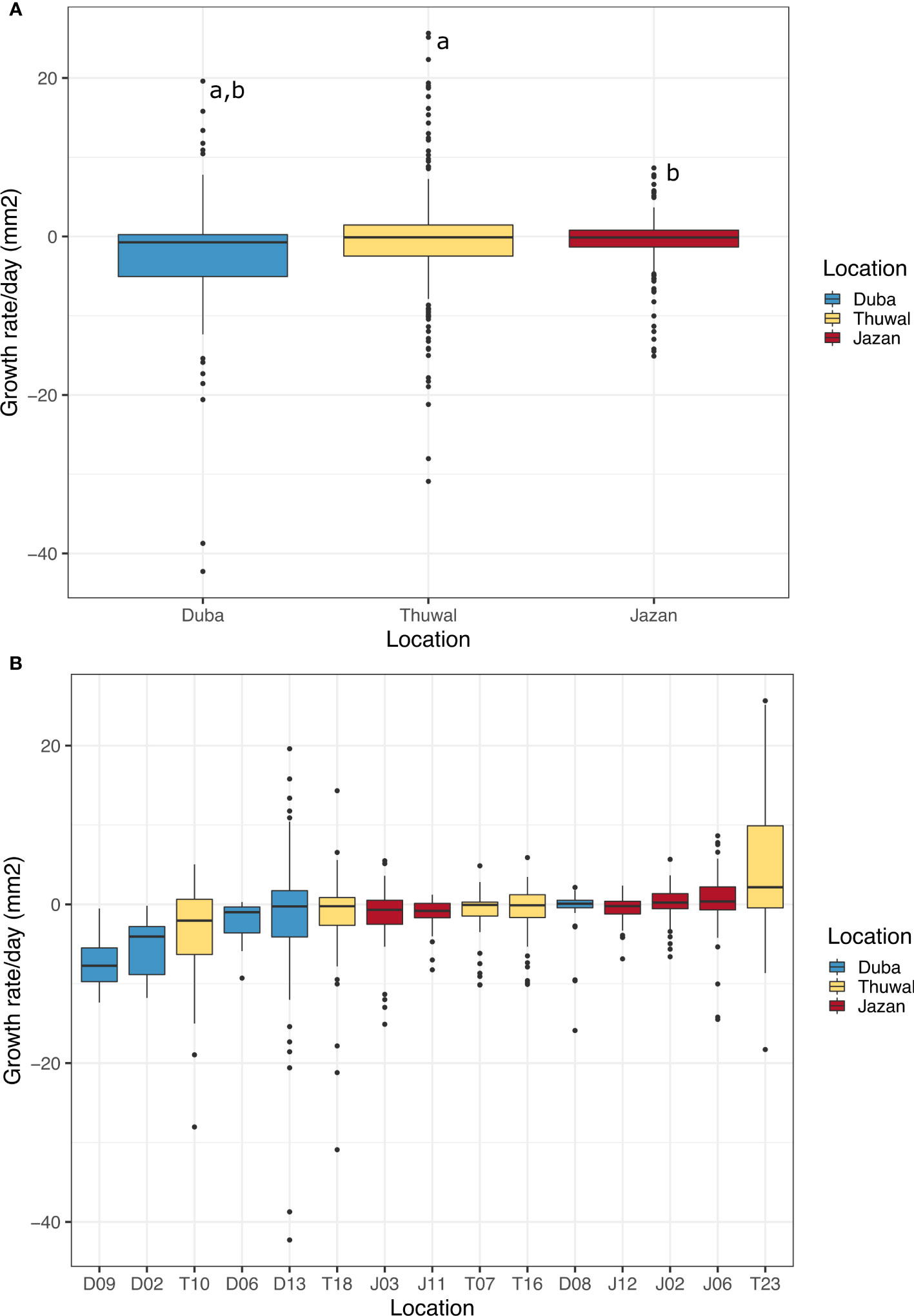
Figure 4 Daily growth rate averages (mm2) for colonies of P. lobata collected in from three different latitudes of the Red Sea during a common garden experiment deployed at Thuwal from July 2017 until February 2018 (A). Average of daily growth rate of all colonies by location; (B) Average of daily growth rate by colony. The numbers on the bottom indicate the number of live fragments by the end of the experiment for each colony. Equal letters represent significant differences.
Average daily growth rates of Duba fragments did not differ significantly between months. Growth rates were mostly negative throughout the experiment, aligning with the high incidence of bleaching and mortality observed for samples from this location (Supplementary Figure 2). Despite a lower average daily growth rate, some Duba colonies presented growth rates comparable to colonies from Thuwal and Jazan, indicating genotypic variability within local populations (Figure 4B). Notably, D08 and D13 showed higher daily growth rates (Table 2), aligned with the increased survival and bleaching recovery/resistance described previously for these specific colonies. Colony D13 was the only one showing a positive growth rate peak during August-October 2018, followed by a decline in November and recovery from December onwards. Colony D08 growth remained stable throughout the experiment (Supplementary Figure 3). The remaining colonies died early in the experiment.
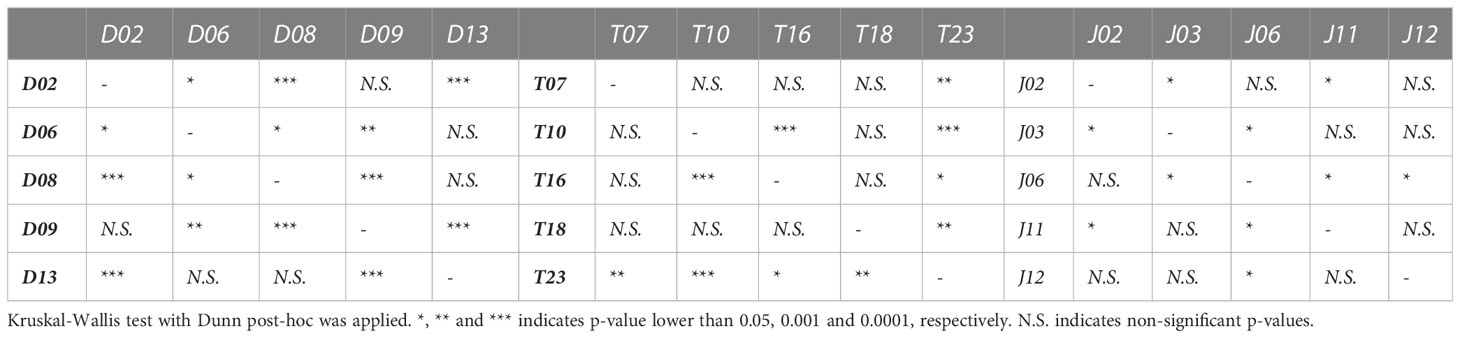
Table 2 Statistically significant differences on average daily growth rate of Porites lobata colonies collected from three different latitudes (Duba, Thuwal, Jazan) of the Red Sea during a common garden experiment deployed at the intermediate temperature location Thuwal from July 2017 until February 2018.
For all Jazan colonies, average daily growth rates were stable throughout the experiment (Supplementary Figure 3). Colony J02 and J06 showed the highest daily growth rates (Figure 4B and Table 2). Coral fragments from Thuwal colonies had an initial positive growth rate in August-October 2018, but declined until December 2018, followed by a recovery throughout the rest of the experiment (Supplementary Figure 2). However, the August-October 2018 growth peak was mainly caused by colony T23, the fastest-growing colony from all locations (Figure 4B, Supplementary Figure 3 and Table 2). Meanwhile, daily growth rate from colony T10 figured amongst the lowest observed (Figure 4B), also indicating genotypic variation within local populations.
Discussion
As expected, our common garden study revealed significantly higher bleaching resistance of colonies from higher-temperature environments (Jazan and Thuwal), showing that thermal tolerance reflects the temperature regimes of natal habitats. However, thermal tolerance alone did not translate to higher survival rates. Although not statistically significant, colonies from Jazan, which come from the warmest location, showed higher mortality rates than local colonies from Thuwal, which had the highest initial survival rates of the three locations.
Not surprising, growth rates from all locations were mainly negative. This could be attributed to the fact that the experiment started in the summer and that the higher temperatures had a negative impact on growth rates (Pratchett et al., 2015; Anderson et al., 2017). We also observed two high temperature peaks at Al Fahal reef during the experiment: one in August 2018 and another in October 2018, which probably impaired colony growth further.
Increased coral thermotolerance in colonies from higher temperature environments
In this study, we observed high temperature resilience for P. lobata colonies from the Southern Red Sea. The region around 16°–20° N is characterized by distinct temperature, salinity, nutrient and turbidity profiles, which could induce local adaptation and population connectivity breaks in the population (Sheppard, 1991; Berumen et al., 2019). In fact, Giles et al. (2015) observed a distinct population of the sponge Stylissa carteri in the further south, around the Farasan Islands, which was mainly explained by its unique environment. A similar population connectivity breakage was observed in the south, at 19° N, for the anemonefish Amphiprion bicinctus, which also correlated with prevalent environmental gradients (Nanninga et al., 2014).
Usually, a significant effect from the site of origin in common garden experiments imply fixed genotypic effects (Sanford and Kelly, 2011). Other common garden and reciprocal transplantation studies showed a significant effect of native habitat (Palumbi et al., 2014; Bay and Palumbi, 2017; Morikawa and Palumbi, 2019; Schoepf et al., 2019; Barott et al., 2021). For instance, in American Samoa colonies from a highly variable temperature reef were more heat tolerant than colonies from a less variable reef. These heat tolerant colonies maintained their resilience after transplantation to a common garden or experimental heat stress (Palumbi et al., 2014; Bay and Palumbi, 2017; Morikawa and Palumbi, 2019) Although colonies from the moderately variable temperature habitats acquired resistance when moved to highly variable temperatures it wasn’t at the same level of resistance displayed by native colonies, emphasizing the role of both acclimatization and adaptation in climate resilience (Palumbi et al., 2014). Schoepf et al. (2019) performed a coral translocation experiment from extreme macrotidal and high temperature reefs in Kimberly Bay, Australia to 4 °C cooler mesocosms. After 9 months, they found that transplanted fragments maintained their health and native heat tolerance, highlighting their value as source reefs for assisted gene flow purposes.
Many factors are known to influence coral thermal adaptation, including latitude (Coles et al., 1976; Berkelmans and van Oppen, 2006; Smith-Keune and van Oppen, 2006; Ulstrup et al., 2006; Dixon et al., 2015), reef microhabitats with different ambient temperature regimes (Oliver and Palumbi; Palumbi et al., 2014; Thomas et al., 2018) and reef location (inshore vs. offshore reefs, (Kenkel et al., 2013; Kenkel et al., 2015b). All these studies indicate some level of adaptation to local thermal regimes that can result in more thermotolerant genotypes. In our study, we observed that colony bleaching susceptibility and mortality aligned with the local thermal regime of their location of origin. Since colonies from all different sites were previously acclimated to the same environment, we can infer that local adaptation, rather than acclimation, drove the main response. However, it is possible that the experimental acclimation period was not long enough to remove any non-genetic adaptations to previous environmental conditions as it is currently unknown how long such effects might last in corals.
Besides possible local adaptations, Symbiodiniaceae composition can also influence adaptive potential to environmental change (Marhoefer et al., 2021). In the Red Sea, Porites spp colonies from the South were observed to have a higher abundance of Symbiodiniaceae from the genus Durisdinium, in particular D. trenchii (Terraneo et al., 2019). This genus, formally known as clade D, is known to confer higher thermo-resilience (Rowan, 2004; Berkelmans and van Oppen, 2006; Stat and Gates, 2011). Thus, higher bleaching tolerance in fragments from Jazan and Thuwal could also be related to the Symbiodiniaceae type these colonies harbored. Conversely, colonies from Thuwal were reported to have mainly symbionts from the genus Cladocopium, with only ~25% of the colonies harboring Durisdinium species (Terraneo et al., 2019). It has been suggested that high Cladocopium abundance is driven by colder water preference (Sawall et al., 2014). In fact, Cladocopium abundance in P. lobata in the Red Sea seems to follow an increasing trend from south to north (Terraneo et al., 2019). Considering that the high thermotolerance conferred by Durisdinium usually comes with a growth trade off (Stat and Gates, 2011), the preference for Cladocopium in corals from Thuwal might be a strategy to endure colder winters without compromising on growth.
This study observed significantly more bleaching of coral colonies originating from the Northern (Duba) than in corals from the Southern and Central Red Sea. The mean sea surface temperature summer maximum in Duba is 30.9 °C, two degrees lower than the summer maximum temperature during the common garden experiment (~33 °C). Studies show that corals are sensitive to temperatures above the mean summer maximum, and increased bleaching probability can be expected with temperatures even 1 °C higher than the MMM for 3-4 weeks (Berkelmans et al., 2004; Hoegh-Guldberg et al., 2017).
Osman et al., 2018 looked at historical temperature data from the latest 2015–2016 El Niño event in combination with heat stress experiments. They determined degree heating weeks experienced by each location, which is a measurement of cumulative effects of heat stress of a given location, calculated by adding temperature anomalies that exceed the maximum monthly mean by at least 1°C over a 12-week time period (Liu et al., 2003). They observed that locations in the Northern Red Sea have never experienced bleaching events, despite reaching more than 8-degree heating weeks, which was more than what was observed in areas in the Central and Southern Red Sea that documented severe bleaching (less than 8-degree heating weeks). Experiments with Stylophora pistillata from the Gulf of Aqaba reported no signs of bleaching even after experimentally subjecting colonies to 1.5 months at 1–2 °C above their long-term summer maximum, corresponding to 11-degree heating weeks (Krueger et al., 2017). A recent study demonstrated S. pistillata can trigger rapid changes in gene expression in response to heat stress, surviving temperatures of 32°C, which corresponds to five degrees above their maximum monthly mean (Savary et al., 2021). Coral fragments in the aforementioned experiments didn’t bleach, confirming they live well beyond their thermal limits. Bleaching in Northern colonies in our experiment can be explained by the higher in situ sea surface temperature of our common garden location, ~1000 km south of the location of the studies by Krueger et al. (2017); Osman et al. (2018) and Savary et al. (2021).
Interestingly, specific colonies showed particularly high bleaching thresholds or high recovery capacity (i.e., colonies D13 and D08, respectively). Variability in average daily growth rate amongst colonies can also be seen in all three locations. This suggests the presence of physiological diversity, with occurrence of thermotolerant and resilient colonies even in populations considered more vulnerable. Previous research has observed similar results along the Florida reef tract, even within smaller spatial scales (Drury et al., 2017). In Hawaii, Drury et al. (2022) found that intra-populational variance in both symbiont type and host genotype in Montipora capitata contributed to thermal tolerance, as indicated by bleached vs. non-bleached phenotypes.
This genetic diversity can be valuable for future reef restoration efforts since it means that particularly resilient local corals could also be used as source for future restoration plans. This becomes especially important in light of this study, as we show that resilience to high temperature is not the only factor determining the success at a given site. Future studies should therefore focus on fast and cost-effective methods to screen local colony temperature resilience (Voolstra et al., 2020; Zoccola et al., 2020; Evensen et al., 2021; Voolstra et al., 2021; Evensen et al., 2022).
Limitations of assisted migration to coral restoration
Previous studies in the Red Sea have identified high genetic connectivity for the broadcast spawning coral Pocillopora verrucosa, with some genetic differentiation only in the extreme South (Robitzch et al., 2015; Buitrago-López et al., 2023). However, given natural long-distance spread of warm-adapted corals is unlikely to happen in a short time frame (Quigley et al., 2019), human assistance can be beneficial to speed up this process to keep up with climate change.
In this study, we observed that even though fragments from Jazan did not experience bleaching, their survival rate was still similar to fragments from the central location, Thuwal. This indicates some level of adaptation to other local environmental variables besides temperature. Similar observations have also been made in reciprocal transplantation experiments between inshore and offshore reefs in the Florida Keys, where selection against Porites astreoides transplants was reported (Kenkel et al., 2015a). They concluded that environmental variables other than temperature contribute to coral population structure. Fine-scale adaptation comes with a price: decreased fitness in foreign environments, since different selection forces act in diverse environments (Hereford, 2009; Howells et al., 2013; Kenkel et al., 2015a; Bay and Palumbi, 2017). This can hamper assisted migration efforts by introducing the potential for maladaptation to non-temperature related environmental factors (Aitken and Whitlock, 2013), thus limiting the recovery of reefs. Additionally, differences in Symbiodiniaceae identity could also lead to maladaptation in a foreign environment. Therefore, more studies are needed to verify genetic identity of symbiotic partners, if transplanted colonies from warm reefs are able to maintain their health in foreign environments, and if this is stable across generations and over the timescales required for such assisted migration projects, i.e. years to decades (Schoepf et al., 2019).
Differences in chl a, inorganic nutrients and salinity along the Red Sea coast (Raitsos et al., 2013; Kürten et al., 2016; Mezger et al., 2016; Wafar et al., 2016) can explain higher initial survival of the local colonies (Thuwal), since they were already adapted to the many local environmental conditions. Jazan transplants, although likely adapted to higher temperature as reflected in their low bleaching incidence, still had to adjust to other local parameters, while Duba colonies had to cope with temperature stress in addition to the other new environmental parameters. Moreover, it is possible that fragments from Jazan were still affected by depleted energy reserves from previous bleaching events, as seen by Klepac and Barshis (2020).
While care should be taken with population level generalizations based on few colonies, this dataset indicates that reef restoration efforts based on the translocation of more resilient coral colonies from farther locations might be limited by local adaptation. As such, they should be continuously monitored to follow the health of native and translocated colonies. Thus, even though some level of acclimatization is possible, this is limited by fixed genetic effects and historical thermal limits (Howells et al., 2013). This also means prioritizing small spatial scale transplantations, to ensure environmental conditions are as similar as possible and maximize transplants survival (Kenkel et al., 2015a). Nonetheless, assisted migration is still valuable as a means to rescue populations threatened by increasing temperatures.
Finally, assisted migration may come with other risks, such as importing pathogens and invasive species (Hoegh-Guldberg et al., 2008; Chen et al., 2022) and the possibility of outbreeding depression. Interpopulation hybrids could have decreased fitness due to the disruption of locally co-adapted gene complexes and generate intermediate genotypes with less fitness than either parent (Edmands, 2006). However, this is unlikely given the high connectivity patterns of broadcast corals in the Red Sea (Robitzch et al., 2015; Buitrago-López et al., 2023).
Therefore, even though assisted migration can benefit coral communities in some scenarios, more studies are needed to assess the risks and benefits of this practice. Given the pitfalls mentioned above, other active conservation tools should also be explored. As such, selective breeding might be a viable alternative. Selecting parental colonies from both warm-adapted source and destination environments can lead to offspring with novel phenotypes, introducing thermotolerant alleles into cooler reef habitats while retaining adaptions to the local environment. This could increase offspring survival by providing them with beneficial alleles from both parental populations, while minimizing potential maladaptation (Oppen et al., 2015). In fact, breeding experiments between Platygyra daedalea from the Arabian Gulf and Indian Ocean showed benefits of selective breeding to the heat naïve Indian Ocean population (Howells et al., 2021). Although more theoretical and applied research is essential, we encourage active measures towards building reef resilience and minimizing anthropogenic disturbances to sustain the vital ecosystem functions provided by coral reefs in the face of global change.
Data availability statement
The raw data supporting the conclusions of this article will be made available by the authors, without undue reservation.
Ethics statement
The manuscript presents research on animals that do not require ethical approval for their study.
Author contributions
MA and SS-R conceptualized the study and SS-R conceived the experimental design. MB and SS-R conducted the sampling expeditions. MB carried out the experiment and analyzed the data. HZ contributed to the statistical analysis. MB wrote the manuscript with critical feedback from all authors. All authors contributed to the article and approved the submitted version.
Acknowledgments
We thank Amr Gusti, Alicia Schmidt-Roach, Maha Cziesielski, Royale Hardenstine, Shannon Brown, Timothy Thomson and Angus Li for all the assistance during fieldwork. We also thank logistical support provided by the KAUST Coastal and Marine Resources Core Lab. This publication is based on work supported by the KAUST Office of Sponsored Research under award no. FCC/1/1973-47-01.
Conflict of interest
The authors declare that the research was conducted in the absence of any commercial or financial relationships that could be construed as a potential conflict of interest.
Publisher’s note
All claims expressed in this article are solely those of the authors and do not necessarily represent those of their affiliated organizations, or those of the publisher, the editors and the reviewers. Any product that may be evaluated in this article, or claim that may be made by its manufacturer, is not guaranteed or endorsed by the publisher.
Supplementary material
The Supplementary Material for this article can be found online at: https://www.frontiersin.org/articles/10.3389/fmars.2023.1181456/full#supplementary-material
References
Aitken S. N., Whitlock M. C. (2013). Assisted gene flow to facilitate local adaptation to climate change. Annu. Rev. Ecol. Evol. Syst. 44, 367–388. doi: 10.1146/annurev-ecolsys-110512-135747
Anderson K. D., Cantin N. E., Heron S. F., Pisapia C., Pratchett M. S. (2017). Variation in growth rates of branching corals along Australia’s Great Barrier Reef. Sci. Rep. 7, 2920. doi: 10.1038/s41598-017-03085-1
Anthony K., Bay L. K., Costanza R., Firn J., Gunn J., Harrison P., et al. (2017). New interventions are needed to save coral reefs. Nat. Ecol. Evol. 1, 1420–1422. doi: 10.1038/s41559-017-0313-5
Barott K. L., Huffmyer A. S., Davidson J. M., Lenz E. A., Matsuda S. B., Hancock J. R., et al. (2021). Coral bleaching response is unaltered following acclimatization to reefs with distinct environmental conditions. PNAS 118, e2025435118. doi: 10.1073/pnas.2025435118
Barshis D. J., Stillman J. H., Gates R. D., Toonen R. J., Smith L. W., Birkeland C. (2010). Protein expression and genetic structure of the coral Porites lobata in an environmentally extreme Samoan back reef: does host genotype limit phenotypic plasticity? Mol. Ecol. 19, 1705–1720. doi: 10.1111/j.1365-294X.2010.04574.x
Bay R. A., Palumbi S. R. (2014). Multilocus adaptation associated with heat resistance in reef-building corals. Curr. Biol. 24, 2952–2956. doi: 10.1016/j.cub.2014.10.044
Bay R. A., Palumbi S. R. (2017). Transcriptome predictors of coral survival and growth in a highly variable environment. Ecol. Evol. 7, 4794. doi: 10.1002/ece3.2685
Berkelmans R., De’ath G., Kininmonth S., Skirving W. J. (2004). A comparison of the 1998 and 2002 coral bleaching events on the Great Barrier Reef: spatial correlation, patterns, and predictions. Coral Reefs 23, 74–83. doi: 10.1007/s00338-003-0353-y
Berkelmans R., van Oppen M. J. (2006). The role of zooxanthellae in the thermal tolerance of corals: a ‘nugget of hope’ for coral reefs in an era of climate change. Proc. R Soc. B Biol. Sci. 273, 2305–2312. doi: 10.1098/rspb.2006.3567
Berumen M. L., Voolstra C. R., Daffonchio D., Agusti S., Aranda M., Irigoien X., et al. (2019). The red sea: environmental gradients shape a natural laboratory in a nascent ocean. Springer Cham pp, 1–10. doi: 10.1007/978-3-030-05802-9_1
Bindoff N. L., Cheung W. W. L., Kairo J. G., Arstegui J., Guinder V. A., Hallberg R., et al. (2019). Changing ocean, marine ecosystems, and dependent communities. In The Ocean and Cryosphere in a Changing Climate: Special Report of the Intergovernmental Panel on Climate Change (Cambridge University Press), 447–588. doi: 10.1017/9781009157964.007
Bongaerts P., Frade P. R., Ogier J. J., Hay K. B., Van Bleijswijk J., Englebert N., et al. (2013). Sharing the slope: Depth partitioning of agariciid corals and associated Symbiodinium across shallow and mesophotic habitats (2-60 m) on a Caribbean reef. BMC Evol. Biol. 13, 1–15. doi: 10.1186/1471-2148-13-205
Bongaerts P., Riginos C., Ridgway T., Sampayo E. M., van Oppen M. J. H., Englebert N., et al. (2010). Genetic Divergence across Habitats in the Widespread Coral Seriatopora hystrix and Its Associated Symbiodinium. PloS One 5 (5). doi: 10.1371/journal.pone.0010871
Buerger P., Alvarez-Roa C., Coppin C. W., Pearce S. L., Chakravarti L. J., Oakeshott J. G., et al. (2020). Heat-evolved microalgal symbionts increase coral bleaching tolerance. Sci. Adv. 6, eaba2498. doi: 10.1126/sciadv.aba2498
Buitrago-López C., Cárdenas A., Hume B. C. C., Gosselin T., Staubach F., Aranda M., et al. (2023). Disparate population and holobiont structure of pocilloporid corals across the Red Sea gradient demonstrate species-specific evolutionary trajectories. Mol. Ecol. 32, 2151–2173. doi: 10.1111/mec.16871
Camp E. F., Schoepf V., Mumby P. J., Hardtke L. A., Rodolfo-Metalpa R., Smith D. J., et al. (2018). The future of coral reefs subject to rapid climate change: lessons from natural extreme environments. Front. Mar. Sci. 5, 4. doi: 10.3389/fmars.2018.00004
Chaidez V., Dreano D., Agusti S., Duarte C. M., Hoteit I. (2017). Decadal trends in Red Sea maximum surface temperature. Sci. Rep. 7, 8144. doi: 10.1038/s41598-017-08146-z
Chen Z., Grossfurthner L., Loxterman J. L., Masingale J., Richardson B. A., Seaborn T., et al. (2022). Applying genomics in assisted migration under climate change: Framework, empirical applications, and case studies. Evol. Appl. 15, 3–21. doi: 10.1111/eva.13335
Cinner J. E., McClanahan T. R., Graham N. A. J., Daw T. M., Maina J., Stead S. M., et al. (2012). Vulnerability of coastal communities to key impacts of climate change on coral reef fisheries. Glob. Environ. Chang. 22, 12–20. doi: 10.1016/j.gloenvcha.2011.09.018
Coles S. L., Okiel P. L. J., Lewis C. R. (1976). Thermal tolerance in tropical versus subtropical pacific reef corals. Pacif. Sci. 30 (2),159–166.
Coles S. L., Riegl B. M. (2013). Thermal tolerances of reef corals in the Gulf: A review of the potential for increasing coral survival and adaptation to climate change through assisted translocation. Mar. pollut. Bull. 72, 323–332. doi: 10.1016/j.marpolbul.2012.09.006
Daly J., Hobbs R. J., Zuchowicz N., O’Brien J. K., Bouwmeester J., Bay L., et al. (2022). Cryopreservation can assist gene flow on the Great Barrier Reef. Coral Reefs 41, 455–462. doi: 10.1007/s00338-021-02202-x
DiBattista J. D., Roberts M. B., Bouwmeester J., Bowen B. W., Coker D. J., Lozano-Cortés D. F., et al. (2016). A review of contemporary patterns of endemism for shallow water reef fauna in the Red Sea. J. Biogeogr. 43, 423–439. doi: 10.1111/jbi.12649
Dixon G. B., Davies S. W., Aglyamova G. A., Meyer E., Bay L. K., Matz M. V. (2015). Genomic determinants of coral heat tolerance across latitudes. Science 348, 1460–1462. doi: 10.1126/science.1261224
Drury C., Manzello D., Lirman D. (2017). Genotype and local environment dynamically influence growth, disturbance response and survivorship in the threatened coral, Acropora cervicornis. PLoS ONE 12 (3), e0174000. oi: 10.1371/journal.pone.0174000
Drury C., Bean N. K., Harris C. I., Hancock J. R., Huckeba J., CM H., et al. (2022). Intrapopulation adaptive variance supports thermal tolerance in a reef-building coral. Commun. Biol. 5, 486. doi: 10.1038/s42003-022-03428-3
Duarte C. M., Agusti S., Barbier E., Britten G. L., Castilla J. C., Gattuso J.-P., et al. (2020). Rebuilding marine life. Nature 580, 39–51. doi: 10.1038/s41586-020-2146-7
Edmands S. (2006). Between a rock and a hard place: evaluating the relative risks of inbreeding and outbreeding for conservation and management. Mol. Ecol. 16, 463–475. doi: 10.1111/j.1365-294X.2006.03148.x
Evensen N. R., Fine M., Perna G., Voolstra C. R., Barshis D. J. (2021). Remarkably high and consistent tolerance of a Red Sea coral to acute and chronic thermal stress exposures. Limnol. Oceanogr. 66, 1718–1729. doi: 10.1002/lno.11715
Evensen N. R., Voolstra C. R., Fine M., Perna G., Buitrago-López C., Cárdenas A., et al. (2022). Empirically derived thermal thresholds of four coral species along the Red Sea using a portable and standardized experimental approach. Coral Reefs 41, 239–252. doi: 10.1007/s00338-022-02233-y
Giles E. C., Saenz-Agudelo P., Hussey N. E., Ravasi T., Berumen M. L. (2015). Exploring seascape genetics and kinship in the reef sponge Stylissa carteri in the Red Sea. Ecol. Evol. 5, 2487–2502. doi: 10.1002/ece3.1511
Hagedorn M., Page C. A., O’Neil K. L., Flores D. M., Tichy L., Conn T., et al. (2021). Assisted gene flow using cryopreserved sperm in critically endangered coral. Proc. Natl. Acad. Sci. 118, e2110559118. doi: 10.1073/pnas.2110559118
Hereford J. (2009). A quantitative survey of local adaptation and fitness trade-offs. Am. Nat. 173, 579–588. doi: 10.1086/597611
Hoegh-Guldberg O. (1999). Climate change, coral bleaching and the future of the world’s coral reefs. Mar. Freshw. Res 50, 839–866. doi: 10.1071/MF99078
Hoegh-Guldberg O., Mumby P. J., Hooten A. J., Steneck R. S., Greenfield P., Gomez E., et al. (2007). Coral reefs under rapid climate change and ocean acidification. Science 318, 1737–42. doi: 10.1126/science.1152509
Hoegh-Guldberg O., Hughes L., McIntyre S., Lindenmayer D. B., Parmesan C., Possingham H. P., et al. (2008). Assisted colonization and rapid climate change. Sci. (80- ) 321, 345 LP–346. doi: 10.1126/science.1157897
Hoegh-Guldberg O., Poloczanska E. S., Skirving W., Dove S. (2017). Coral reef ecosystems under climate change and ocean acidification. Front. Mar. Sci. 4, 158. doi: 10.3389/fmars.2017.00158
Howells E. J., Abrego D., Liew Y. J., Burt J. A., Meyer E., Aranda M. (2021). Enhancing the heat tolerance of reef-building corals to future warming. Sci. Adv. 7, eabg6070. doi: 10.1126/sciadv.abg6070
Howells E. J., Berkelmans R., van Oppen M. J. H., Willis B. L., Bay L. K. (2013). Historical thermal regimes define limits to coral acclimatization. Ecology 94, 1078–1088. doi: 10.1890/12-1257.1
Hughes T. P., Barnes M. L., Bellwood D. R., Cinner J. E., Cumming G. S., Jackson J. B. C. C., et al. (2017a). Coral reefs in the anthropocene. Nature 546, 82. doi: 10.1038/nature22901
Hughes T. P., Kerry J. T., Álvarez-Noriega M., Álvarez-Romero J. G., Anderson K. D., Baird A. H., et al. (2017b). Global warming and recurrent mass bleaching of corals. Nature 543, 373–377. doi: 10.1038/nature21707
Humanes A., Beauchamp E. A., Bythell J. C., Carl M. K., Craggs J. R., Edwards A. J., et al. (2021). An experimental framework for selectively breeding corals for assisted evolution. Front. Mar. Sci. 8. doi: 10.3389/fmars.2021.669995
Jurriaans S., Hoogenboom M. O. (2019). Thermal performance of scleractinian corals along a latitudinal gradient on the Great Barrier Reef. Philos. Trans. R Soc. B Biol. Sci. 374, 20180546. doi: 10.1098/rstb.2018.0546
Kenkel C. D., Almanza A. T., Matz M. V. (2015a). Fine-scale environmental specialization of reef-building corals might be limiting reef recovery in the Florida Keys. Ecology 96, 3197–3212. doi: 10.1890/14-2297.1
Kenkel C. D., Goodbody-Gringley G., Caillaud D., Davies S. W., Bartels E., Matz M. V. (2013). Evidence for a host role in thermotolerance divergence between populations of the mustard hill coral ( Porites astreoides ) from different reef environments. Mol. Ecol. 22, 4335–4348. doi: 10.1111/mec.12391
Kenkel C. D., Setta S. P., Matz M. V. (2015b). Heritable differences in fitness-related traits among populations of the mustard hill coral, Porites astreoides. Heredity (Edinb) 115, 509–516. doi: 10.1038/hdy.2015.52
Klepac C. N., Barshis D. J. (2020). Reduced thermal tolerance of massive coral species in a highly variable environment. Proc. R Soc. B Biol. Sci. 287 (1933). doi: 10.1098/rspb.2020.1379
Krueger T., Horwitz N., Bodin J., Giovani M.-E., Escrig S., Meibom A., et al. (2017). Common reef-building coral in the Northern Red Sea resistant to elevated temperature and acidification. R. Soc. open sci. 4, 170038. doi: 10.1098/rsos.170038
Kürten B., Al-Aidaroos A. M., Kürten S., El-Sherbiny M. M., Devassy R. P., Struck U., et al. (2016). Carbon and nitrogen stable isotope ratios of pelagic zooplankton elucidate ecohydrographic features in the oligotrophic Red Sea. Prog. Oceanogr. 140, 69–90. doi: 10.1016/j.pocean.2015.11.003
Liu G., Strong A. E., Skirving W. (2003). Remote sensing of sea surface temperatures during 2002 Barrier Reef coral bleaching. Eos Trans. Am. Geophys Union 84, 137–141. doi: 10.1029/2003EO150001
Lundgren P., Vera J. C., Peplow L., Manel S., van Oppen M. J. H. (2013). Genotype - environment correlations in corals from the Great Barrier Reef. BMC Genet. 14, 9. doi: 10.1186/1471-2156-14-9.
Marhoefer S. R., Zenger K. R., Strugnell J. M., Logan M., van Oppen M. J. H., Kenkel C. D., et al. (2021). Signatures of adaptation and acclimatization to reef flat and slope habitats in the coral Pocillopora damicornis. Front. Mar. Sci. 8, 1194. doi: 10.3389/fmars.2021.704709
Mezger E. M., Nooijer L. J., Boer W., Brummer G. J. A., Reichart G. J. (2016). Salinity controls on Na incorporation in Red Sea planktonic foraminifera. Paleoceanography 31, 1562–1582. doi: 10.1002/2016PA003052
Morikawa M. K., Palumbi S. R. (2019). Using naturally occurring climate resilient corals to construct bleaching-resistant nurseries. Proc. Natl. Acad. Sci. 116, 10586–10591. doi: 10.1073/pnas.1721415116
Nanninga G. B., Saenz-Agudelo P., Manica A., Berumen M. L. (2014). Environmental gradients predict the genetic population structure of a coral reef fish in the Red Sea. Mol. Ecol. 23, 591–602. doi: 10.1111/mec.12623
National Academies of Sciences Engineering and Medicine (2019). A Research Review of Interventions to Increase the Persistence and Resilience of Coral Reefs (Washington, D.C: National Academies Press).
Nedimyer K., Gaines K., Roach S. (2011). Coral Tree Nursery©: An innovative approach to growing corals in an ocean-based field nursery. AACL Bioflux 4, 442–446.
Oliver T. A., Palumbi S. R. (2011). Do fluctuating temperature environments elevate coral thermal tolerance? Coral Reefs 30, 429–440.
Oppen M. J. H., Oliver J. K., Putnam H. M., Gates R. D., van Oppen M. J. H., Oliver J. K., et al. (2015). Building coral reef resilience through assisted evolution. Proc. Natl. Acad. Sci. 112, 1–7. doi: 10.1073/pnas.1422301112
Osman E. O., Smith D. J., Ziegler M., Kürten B., Conrad C., El-Haddad K. M., et al. (2018). Thermal refugia against coral bleaching throughout the northern Red Sea. Glob. Chang. Biol. 24, e474–e484. doi: 10.1111/gcb.13895
Palumbi S. R., Barshis D. J., Traylor-Knowles N., Bay R. A. (2014). Mechanisms of reef coral resistance to future climate change. Science 80- ) 344, 895–898. doi: 10.1126/science.1251336
Peixoto R. S., Voolstra C. R., Sweet M., Duarte C. M., Carvalho S., Villela H., et al. (2022). Harnessing the microbiome to prevent global biodiversity loss. Nat. Microbiol 7, 1726–1735. doi: 10.1038/s41564-022-01173-1
Pendleton L., Comte A., Langdon C., Ekstrom J. A., Cooley S. R., Suatoni L., et al. (2016). Coral reefs and people in a high-CO2 world: where can science make a difference to people? PloS One 11, e0164699. doi: 10.1371/journal.pone.0164699
Pratchett M. S., Anderson K. D., Hoogenboom M. O., Widman E., Baird A. H., Pandolfi J. M., et al. (2015). Spatial, temporal and taxonomic variation in coral growth—implications for the structure and function of coral reef ecosystems. Oceanogr. Mar. Biol. Annu. Rev. 53, 215–295. doi: 10.1201/B18733-7
Putnam H. M., Gates R. D. (2015). Preconditioning in the reef-building coral Pocillopora damicornis and the potential for trans-generational acclimatization in coral larvae under future climate change conditions. J. Exp. Biol. 218, 2365–2372. doi: 10.1242/jeb.123018
Quigley K. M., Alvarez Roa C., Beltran V. H., Leggat B., Willis B. L. (2021). Experimental evolution of the coral algal endosymbiont, Cladocopium goreaui: lessons learnt across a decade of stress experiments to enhance coral heat tolerance. Restor. Ecol. 29, e13342. doi: 10.1111/rec.13342
Quigley K. M., Bay L. K., Oppen M. J. H. (2019). The active spread of adaptive variation for reef resilience. Ecol. Evol. 9, 11122–11135. doi: 10.1002/ece3.5616
Raitsos D. E., Pradhan Y., Brewin R. J. W., Stenchikov G., Hoteit I. (2013). Remote sensing the phytoplankton seasonal succession of the red sea. PloS One 8, e64909. doi: 10.1371/journal.pone.0064909
R Core Team (2016). R: A language and environment for statistical computing (Vienna: R Foundation for Statistical Computing).
Riegl B. M., Purkis S. J., Al-Cibahy A. S., Abdel-Moati M. A., Hoegh-Guldberg O. (2011). Present limits to heat-adaptability in corals and population-level responses to climate extremes. PloS One 6 (9), e24802. doi: 10.1371/journal.pone.0024802
Robitzch V., Banguera-Hinestroza E., Sawall Y., Al-Sofyani A., Voolstra C. R. (2015). Absence of genetic differentiation in the coral Pocillopora verrucosa along environmental gradients of the Saudi Arabian Red Sea. Front. Mar. Sci. 2, 5. doi: 10.3389/fmars.2015.00005
Rosado P. M., Leite D. C. A., Duarte G. A. S., Chaloub R. M., Jospin G., Nunes da Rocha U., et al. (2019). Marine probiotics: increasing coral resistance to bleaching through microbiome manipulation. ISME J. 13, 921–936. doi: 10.1038/s41396-018-0323-6
Rowan R. (2004). Thermal adaptation in reef coral symbionts. Nature 430, 742–742. doi: 10.1038/430742a
Safaie A., Silbiger N. J., McClanahan T. R., Pawlak G., Barshis D. J., Hench J. L., et al. (2018). High frequency temperature variability reduces the risk of coral bleaching. Nat. Commun. 9, 1671. doi: 10.1038/s41467-018-04074-2
Sanford E., Kelly M. W. (2011). Local adaptation in marine invertebrates. Ann. Rev. Mar. Sci. 3, 509–535. doi: 10.1146/annurev-marine-120709-142756
Santoro E. P., Borges R. M., Espinoza J. L., Freire M., Messias C. S. M. A., Villela H. D. M., et al. (2022). Coral microbiome manipulation elicits metabolic and genetic restructuring to mitigate heat stress and evade mortality. Sci. Adv. 7, eabg3088. doi: 10.1126/sciadv.abg3088
Savary R., Barshis D. J., Voolstra C. R., Cárdenas A., Evensen N. R., Banc-Prandi G., et al. (2021). Fast and pervasive transcriptomic resilience and acclimation of extremely heat-tolerant coral holobionts from the northern Red Sea. Proc. Natl. Acad. Sci. U.S.A. 118 (19). doi: 10.1073/pnas.2023298118
Sawall Y., Al-Sofyani A., Banguera-Hinestroza E., Voolstra C. R. (2014). Spatio-Temporal Analyses of Symbiodinium Physiology of the Coral Pocillopora verrucosa along Large-Scale Nutrient and Temperature Gradients in the Red Sea. PloS One 9, e103179. doi: 10.1371/journal.pone.0103179
Schoepf V., Carrion S. A., Pfeifer S. M., Naugle M., Dugal L., Bruyn J., et al. (2019). Stress-resistant corals may not acclimatize to ocean warming but maintain heat tolerance under cooler temperatures. Nat. Commun. 10, 4031. doi: 10.1038/s41467-019-12065-0
Sheppard C. R. C. (1991). Corals and coral communities of Arabia. In: Buttiker W., Krupp F. Eds. Fauna of Saudi Arabia (Basle: Natural History Museum) 12, 3–170.
Smith-Keune C., van Oppen M. (2006). Genetic structure of a reef-building coral from thermally distinct environments on the Great Barrier Reef. Coral Reefs 25, 493–502. doi: 10.1007/s00338-006-0129-2
Stat M., Gates R. D. (2011). Clade D Symbiodinium in scleractinian corals: A “Nugget” of hope, a selfish opportunist, an ominous sign, or all of the above? J. Mar. Biol. 2011, 1–9. doi: 10.1155/2011/730715
Terraneo T. I., Fusi M., Hume B. C. C., Arrigoni R., Voolstra C. R., Benzoni F., et al. (2019). Environmental latitudinal gradients and host-specificity shape Symbiodiniaceae distribution in Red Sea Porites corals. J. Biogeogr. 46, 2323–2335. doi: 10.1111/jbi.13672
Thomas L., Rose N. H., Bay R. A., López E. H., Morikawa M. K., Ruiz-Jones L., et al. (2018). Mechanisms of thermal tolerance in reef-building corals across a fine-grained environmental mosaic: lessons from Ofu, American Samoa. Front. Mar. Sci. 4, 434. doi: 10.3389/fmars.2017.00434
Tisthammer K. H., Forsman Z. H., Toonen R. J., Richmond R. H. (2020). Genetic structure is stronger across human-impacted habitats than among islands in the coral Porites lobata. PeerJ 2020, e8550. doi: 10.7717/peerj.8550
Ulstrup K., Berkelmans R., Ralph P., van Oppen M. (2006). Variation in bleaching sensitivity of two coral species across a latitudinal gradient on the Great Barrier Reef: the role of zooxanthellae. Mar. Ecol. Prog. Ser. 314, 135–148. doi: 10.3354/meps314135
van Oppen M. J. H., Bongaerts P., Frade P., Peplow L. M., Boyd S. E., Nim H. T., et al. (2018). Adaptation to reef habitats through selection on the coral animal and its associated microbiome. Mol. Ecol. 27, 2956–2971. doi: 10.1111/mec.14763
van Oppen M. J. H., Gates R. D., Blackall L. L., Cantin N., Chakravarti L. J., Chan W. Y., et al. (2017). Shifting paradigms in restoration of the world’s coral reefs. Glob. Chang. Biol. 23, 3437–3448. doi: 10.1111/gcb.13647
Voolstra C. R., Buitrago-López C., Perna G., Cárdenas A., Hume B. C. C., Rädecker N., et al. (2020). Standardized short-term acute heat stress assays resolve historical differences in coral thermotolerance across microhabitat reef sites. Glob. Chang. Biol. 26, 4328–4343. doi: 10.1111/gcb.15148
Voolstra C. R., Suggett D. J., Peixoto R. S., Parkinson J. E., Quigley K. M., Silveira C. B., et al. (2021). Extending the natural adaptive capacity of coral holobionts. Nat. Rev. Earth Environ. 211(2), 747–762. doi: 10.1038/s43017-021-00214-3
Wafar M., Qurban M. A., Ashraf M., Manikandan K. P., Flandez A. V., Balala A. C. (2016). Patterns of distribution of inorganic nutrients in Red Sea and their implications to primary production. J. Mar. Syst. 156, 86–98. doi: 10.1016/j.jmarsys.2015.12.003
Wickham H. (2009). Elegant graphics for data analysis (ggplot2) (Springer-Verlag New York), 177–182. doi: 10.1007/978-0-387-98141-3_6
Woolsey E. S., Keith S. A., Byrne M., Schmidt-Roach S., Baird A. H. (2015). Latitudinal variation in thermal tolerance thresholds of early life stages of corals. Coral Reefs 34, 471–478. doi: 10.1007/s00338-014-1253-z
Keywords: assisted evolution, coral resilience, thermotolerance, Porites lobata, common garden experiment, climate change, coral bleaching
Citation: Barreto MM, Schmidt-Roach S, Zhong H and Aranda M (2023) Assessing the feasibility of assisted migration of corals in the Red Sea. Front. Mar. Sci. 10:1181456. doi: 10.3389/fmars.2023.1181456
Received: 07 March 2023; Accepted: 25 July 2023;
Published: 21 August 2023.
Edited by:
Nina Yasuda, The University of Tokyo, JapanReviewed by:
Margaret Miller, SECORE International, United StatesYuichi Nakajima, National Institute for Environmental Studies (NIES), Japan
Copyright © 2023 Barreto, Schmidt-Roach, Zhong and Aranda. This is an open-access article distributed under the terms of the Creative Commons Attribution License (CC BY). The use, distribution or reproduction in other forums is permitted, provided the original author(s) and the copyright owner(s) are credited and that the original publication in this journal is cited, in accordance with accepted academic practice. No use, distribution or reproduction is permitted which does not comply with these terms.
*Correspondence: Sebastian Schmidt-Roach, c2ViYXN0aWFuLnNjaG1pZHRyb2FjaEBrYXVzdC5lZHUuc2E=; Manuel Aranda, bWFudWVsLmFyYW5kYUBrYXVzdC5lZHUuc2E=; Marcelle Muniz Barreto, bWFyY2VsbGUubXVuaXpiYXJyZXRvQGthdXN0LmVkdS5zYQ==