- 1Environmental Research Institute, UHI North Highland, University of the Highlands and Islands, Thurso, United Kingdom
- 2International Centre for Island Technology, School of Energy, Geoscience, Infrastructure, and Society, Heriot-Watt University, Stromness, United Kingdom
- 3The Lyell Centre, Heriot-Watt University, Edinburgh, United Kingdom
- 4Marine Biogeochemistry Research Division, GEOMAR Helmholtz Centre for Ocean Research Kiel, Kiel, Germany
- 5Department of Civil and Environmental Engineering, Imperial College London, London, United Kingdom
- 6Institute of Oceanology, Polish Academy of Sciences, Sopot, Poland
Climate change and plastic pollution are two of the most pressing environmental challenges caused by human activity, and they are directly and indirectly linked. We focus on the relationship between marine plastic litter and the air-sea flux of greenhouse gases (GHGs). Marine plastic litter has the potential to both enhance and reduce oceanic GHG fluxes, but this depends on many factors that are not well understood. Different kinds of plastic behave quite differently in the sea, affecting air-sea gas exchange in different, largely unknown, ways. The mechanisms of air-sea exchange of GHGs have been extensively studied and if air-sea gas transfer coefficients and concentrations of the gas in water and air are known, calculating the resulting GHG fluxes is reasonably straightforward. However, relatively little is known about the consequences of marine plastic litter for gas transfer coefficients, concentrations, and fluxes. Here we evaluate the most important aspects controlling the exchange of GHGs between the sea and the atmosphere and how marine plastic litter could change these. The aim is to move towards improving air-sea GHG flux calculations in the presence of plastic litter and we have largely limited ourselves to identifying processes, rather than estimating relative importance.
1 Introduction
Climate change and plastic pollution are among the most pressing environmental challenges caused by human activity (Ocean Decade, 2022; United Nations, 2022) and the evidence that in certain oceanic regions and marine environments these problems are related is growing (Cornejo-D’Ottone et al., 2020; Bergmann et al., 2022; Ford et al., 2022; Kvale, 2022; Lincoln et al., 2022). Over 17 million metric tons of plastic entered the ocean in 2021 and this is projected to double or triple by 2040 (United Nations, 2022). Royer et al. (2018) have shown how degradation of plastics in the marine environment can be a minor source of climate-relevant trace gases, but this is not the only way plastic can affect climate gases. Here we focus on the relationship between marine plastic litter (MPL) and the flux between the atmosphere and the ocean (air-sea) of greenhouse gases (GHGs). Carbon dioxide (CO2) is the most well-known GHG, and the ocean absorbs about 25% of the CO2 emitted by human activities (Watson et al., 2020), but there are other relevant and very potent GHGs such as methane (CH4), and nitrous oxide (N2O) that should also be considered.
The mechanisms of air-sea exchange of GHGs have been extensively studied (Garbe et al., 2014). Calculations of air-sea fluxes of a gas using a bulk formula that expresses the flux as the product of a transfer velocity and an effective concentration difference are now enabled by standard and accessible tools (Shutler et al., 2016; Holding et al., 2019). Johnson (2010) provides a method to calculate the transfer velocity of any gas. Formulae for transfer velocity are not exact and are an important source of uncertainty in fluxes (Wrobel and Piskozub, 2016; Woolf et al., 2019), but they are quite robust when derived from an empirical or mechanistic method (Goddijn-Murphy et al., 2016). However, the effect of MPL on these mechanisms may be substantial and the resulting anomaly in flux is unknown. There are 60 publications in the years 2011-2020 that address both plastic pollution and climate change in marine systems (Ford et al., 2022), but these and later studies have not yet described the connection in relation to air-sea gas exchange in a systematic investigation. In this study we identify and evaluate known factors controlling the air-sea GHG flux (Garbe et al., 2014) in relation to MPL to enable parameterization and exploration of the interconnected processes at wider spatial and temporal scales.
2 Plastic litter in the ocean
There are many kinds of plastic litter, consisting of many different polymer compositions, sizes, shapes, and surfaces. Plastic size ranges from microplastics (< 5 mm) to macroplastics covering sizes from 5 mm to large plastic pieces such as lost or discarded fishing nets. Primary microplastics are made on purpose, for example, pellets used in manufacturing and microbeads originating from certain cosmetic products, while secondary microplastics are a result from fragmentation or wear and tear of larger plastic pieces in the environment including ship paint, textile fibers, and vehicle tire wear waste (GESAMP, 2019). As air-sea gas exchange occurs through the air-sea interface, we are concerned with MPL near the surface. Macro- and microplastics behave quite differently in the sea. Microplastics are mostly in suspension below the water surface (Kukulka et al., 2012; Kooi et al., 2016) while macroplastics are present at any depth and can float on top of the sea surface, where they are exposed to air. Our review will examine both micro- and macroplastics.
MPL concentrations may be low on a global scale but can be locally significant in certain areas such as coastal zones and river plumes. In particular, ocean fronts and eddies are turbulent areas where frontal dynamics can accumulate MPL of all sizes (van Sebille et al., 2020). These turbulent areas substantially affect surface boundary layers, enhancing air-sea gas transfer (D’Asaro et al., 2011; Rutgersson et al., 2011; Shin et al., 2022). Convergence zones are also areas known for increased biological productivity affecting air-sea GHG fluxes. Therefore, both direct and indirect effects of MPL on air-sea GHG fluxes could be significant in these regions respectively. Figure 1 identifies important processes, which will be detailed in following sections.
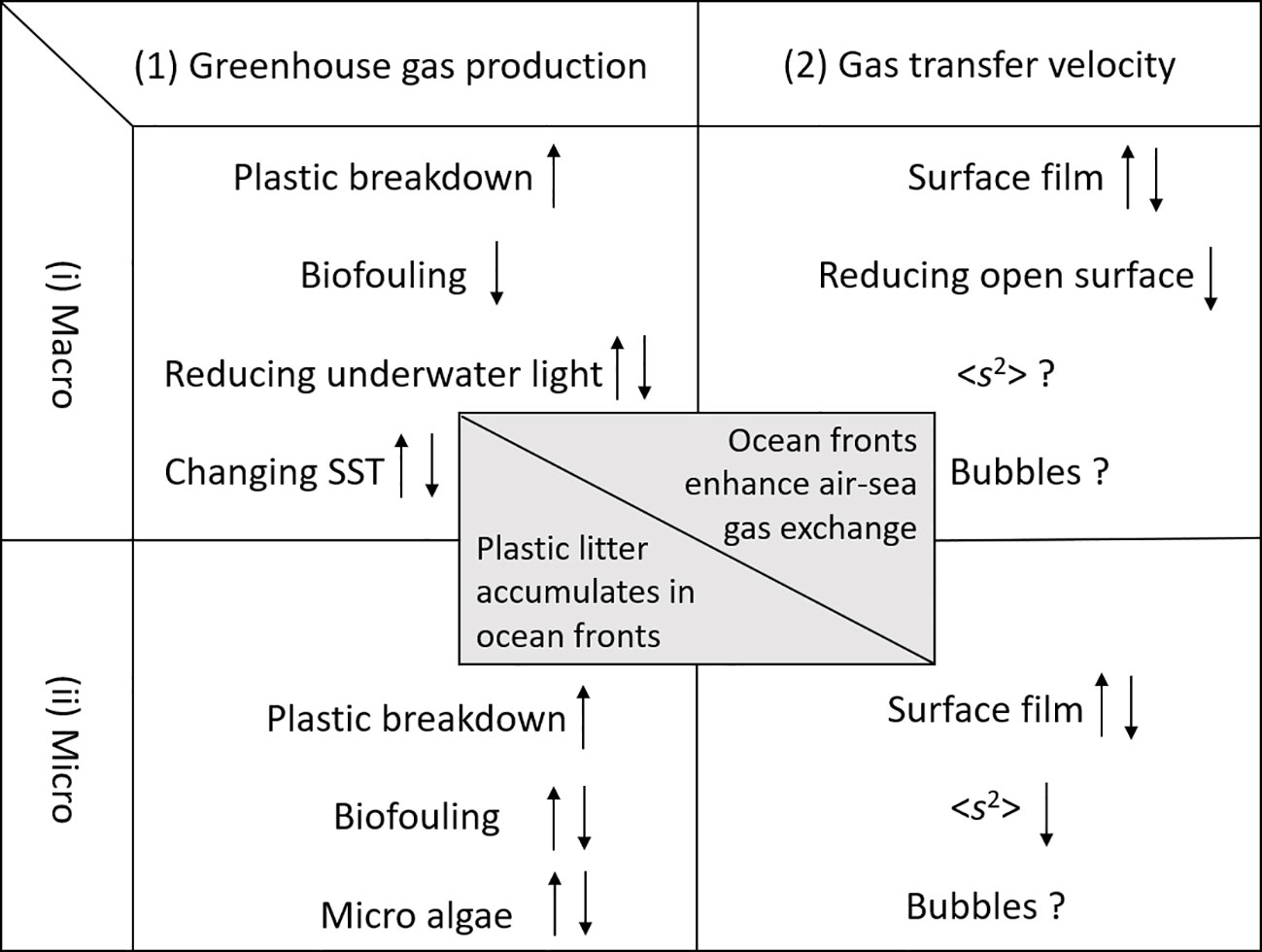
Figure 1 Main processes and factors relating to MPL that control (1) GHG production and consumption (in air as well as in water), and (2) air-sea gas transfer velocity. Arrow up (down) indicates an increase (decrease) of GHG production or decrease (increase) of GHG consumption. For air-sea gas transfer velocity, arrow up (down) indicates enhanced (reduced) values.
3 Factors controlling ocean gas fluxes
At the open water surface, we can calculate the air-sea gas flux, F (mol m-2 s-1) for a chemically unreactive gas as the product of (a) the concentration difference (thermodynamic driving potential), and (b) overall gas transfer velocity across the sea surface, Kw (m s-1) (kinetic forcing function),
In the above equation, Ca and Cw are the respective concentrations (mol m-3) of the gas in the lower atmosphere and upper ocean surface, H is the dimensionless gas-over-liquid form of the Henry’s law constant (a function of temperature and salinity), and F is positive for a gas flux from the atmosphere to the ocean. Kw is dependent on the individual transfer velocities in water, kw, and in air, ka, and for sparingly soluble gases, such as CO2, N2O, and CH4, we can take kw as a practical estimation of Kw (Johnson, 2010). In the following, we assess the links both between MPL and kw, and between MPL and the concentration difference ΔC (= Ca/H-Cw). For a detailed review of gas transfer across the air-sea interface we refer the reader to Garbe et al. (2014).
3.1 Concentration difference, ΔC
The most appropriate concentration difference to consider is that which occurs across a marine microlayer, the mass boundary layer (MBL), at the sea surface (Woolf et al., 2019). The factors that affect this difference include sea surface temperature (SST), transport, biology and chemistry (Garbe et al., 2014) and are considered in the following sections. We identify MPL as an additional factor, notably since breaking down plastic can be a source of GHGs above, within, and below the microlayer (Royer et al., 2018), and since the presence of MPL can affect factors that control respective GHG concentrations (Figure 1).
3.2 Gas transfer velocity, kw
The water-side transfer velocity of a gas has a rather complicated dependence on the properties of the dissolved gas and upon environmental conditions that control turbulence in air and in water, and dynamics at the interface. Associated are microbreaking, turbulence, waves, bubbles and spray, rain and surface films, parameterized by wind, heat flux, fetch, mixing depth, and biological and chemical activity (Garbe et al., 2014). Energy dissipation directly relates air-sea gas exchange to turbulence, but this parameter is challenging to measure and alternatives have been used. Gas transfer is dominated by the small-scale sea surface roughness generated by sea surface wind and is parameterized accordingly. For insoluble gases, we should also account for bubble-mediated gas transfer (Woolf, 1997). In general, transfer can occur directly across the unbroken sea surface, or can be mediated by bubbles or particles in the broken sea surface.
4 The role of marine plastic litter in the air-sea flux of greenhouse gases
4.1 Marine plastic litter and ΔC
4.1.1 Plastic breakdown
The breakdown of MPL, enhanced by exposure to sunlight, can produce CH4, and indirect GHG ethylene (C2H4) and other hydrocarbon gases such as ethane (C2H6) and propylene (C3H6) (Royer et al., 2018). CH4 is less abundant in the atmosphere than CO2 but its global warming potential is 23 times that of CO2 while C2H4, C2H6 and C3H6 are volatile organic compounds that play a role in the atmospheric production of ozone (O3), the third most important GHG after CO2 and CH4 (Ehhalt et al., 2001). Royer et al. (2018) showed that low-density polyethylene (LDPE) produced the most hydrocarbon gases among plastic materials tested and that plastic emissions were higher in air than in water, partly explained in warm climates by plastic in air reaching higher temperatures than in water. ΔC is therefore changed, in the presence of plastic breakdown, through increases in Cw, as well as Ca likely accelerated by a warming climate. Their first results imply that CH4 production by plastics is insignificant on a global scale but for the other hydrocarbon gases with much lower global emissions to the atmosphere, production from plastics might have more significance. Royer et al. (2018) suggest that as macroplastics are broken down over time into microplastics, and microplastics have a greater surface area to volume ratio, hydrocarbon gas production rates will likely accelerate. According to Royer et al. (2018), biofouling reduces the exposure of the plastic surface to sunlight and hence hydrocarbon production. The formation of a biofilm on surfaces exposed to seawater can start within a few hours (Oberbeckmann et al., 2015).
4.1.2 Sea surface temperature
The thermal skin, the top millimeter or so of the upper ocean, is generally slightly cooler, on average 0.14 K (Donlon et al., 1999), than the water below. As solubility of a gas is highly sensitive to SST, this has consequences for calculating ΔC across the MBL, and Woolf et al. (2016) show that the thermal skin increased the net global air to sea flux by 0.34 Pg C yr-1. An opposing effect on calculated fluxes is related to warm layers near the surface that can occur during the day (Woolf et al., 2016). Goddijn-Murphy et al. (2022) report surface temperatures of floating macroplastic litter items higher than SST (up to +10 °C) during a summer day around noon and lower than SST (down to -3 °C) during the night and early morning hours, after deployment at sea for less than an hour as 1×1 m artificial rafts. MPL may therefore introduce temperature anomalies in the surrounding water, varying in a diel cycle, that result in gas flux anomalies.
4.1.3 Transport
In the context of thermodynamic forcing, vertical transport of GHGs can be described by a combination of molecular, turbulent and diffusive transport processes (Garbe et al., 2014). The vertical distribution of plastic debris within the upper water column is driven by the wind (Kukulka et al., 2012). The effect of the microplastic physical properties such as shape, density and size on turbulent transport processes and settling velocity of its particles has been discussed in the review paper by Phan and Luscombe (2023). The formation of biofilms could alter physical properties that control the buoyancy of microplastics (Oberbeckmann et al., 2015). Here we consider how MPL could affect Cw at the base of the MBL and within the upper ocean generally. Directly: changes in CO2 surface concentrations on a regional scale have been attributed to lateral and vertical mixing (Takahashi et al., 2009). Could MPL somehow enhance the mixing of gas concentrations below MBL or change the mixing depth? Indirectly: through materials attached to microplastics that accumulate in the surface microlayer, affecting its geochemistry and hence ΔC (Galgani and Loiselle, 2019; Galgani and Loiselle, 2021). Breaking waves and ocean turbulence mix microplastic particles temporarily down to several meters or even to tens or hundreds of meters after which the particles rise back to the surface, as waves and turbulence decay (van Sebille et al., 2020). Could these microplastics transport materials that change the biogeochemistry from the deep to the MBL, or conversely, remove these from the MBL to the deep? Furthermore, numerical simulations show that microplastic particles can stick to rising bubbles. This mechanism may be effective in increasing vertical transport of microplastic particles to the surface but is expected to be dependent on the hydrophilicity of the plastic particle (Lehmann et al., 2023).
4.1.4 Biology and chemistry
Most published papers linking MPL and the air-sea flux of GHGs have focused on biogeochemistry in the upper ocean and how it is affected by the plastisphere, the aqueous microplastics and the microbial community growing on it (Galgani and Loiselle, 2019; Naik et al., 2019; Prata et al., 2019; Cornejo-D’Ottone et al., 2020; Galgani and Loiselle, 2021). Oberbeckmann et al. (2015) present a review of biofilms attached to marine microplastic. Cornejo-D’Ottone et al. (2020) measured CO2 and N2O production as well as consumption by the plastisphere in the field, depending on the surrounding waters’ biogeochemical characteristics. Photosynthesizing marine algae (macro as well as micro) remove CO2 from the environment and play an important role in the carbon cycle (Arenas and Vaz-Pinto, 2014). Marine microplastics can induce algal blooms but have conversely been associated with inhibiting microalgae growth and reducing their chlorophyll and photosynthesis (Prata et al., 2019; Shen et al., 2020; MacLeod et al., 2021). Macroplastics in a sheltered location in the sea can show the first signs of biofouling, in the form of algal coating after two weeks and a denser biofouling and larger organisms after three months (Fazey and Ryan, 2016; Goddijn-Murphy et al., 2022). Enhancement of marine gel production, enriching the sea-surface microlayer with surfactants, by the presence of microplastic has been recently shown in a mesocosm experiment (Galgani et al., 2023). They found a ~3% reduction in water side CO2 concentration. Conversely, plastic debris around the Antarctic Peninsula was found to host a wide range of bacteria in biofilm, including pathogenic species and some strains with multiple antibiotic resistance (Bargagli and Rota, 2023). Findings in soil communities show that bacteria in biofilms are a significant source of CO2, which is hypothesized to be the case in aqueous communities as well (Nguyen et al., 2021; Rauscher et al., 2023). Interestingly, this was found specifically on forms of MPL made from renewable, bio-based materials, which are thought to reduce the carbon footprint of the industry (Coppola et al., 2021).
Finally, a slightly different biological effect related to MPL and GHGs is the expansion of oxygen minimum zones (OMZs) due the presence of microplastics. A recent modelling study shows that zooplankton consumption of microplastics leads to an increase in primary producers (Kvale et al., 2021). This can lead to increased export, subsequent remineralization, and deoxygenation in some regions. In a follow-on study, Kvale and Oschlies (2023) show that even if there is complete removal of microplastic stocks and sources to the ocean, there may be a lag in recovery from deoxygenation. OMZs are known to be large producers of N2O (e.g., Ward et al., 2009) and certain OMZs have been shown to be large producers of CO2 as well (Paulmier et al., 2011). Kvale et al. (2023) report that microplastics can alter the sinking rates of zooplankton faecal pellets and biological calcification in ways that depend on both ecosystem structure as well as the microplastics themselves; reduction of sinking pellets leads to less carbon sequestration in the deep ocean and calcification reduces surface alkalinity which leads to a decrease in air-sea carbon flux.
4.1.5 Underwater light climate
Macroplastics floating on the water surface change the intensity and spectral shape of the light transmitted across the air-sea interface. Floating plastics reduce photosynthetically active radiation (PAR) from 400–700 nm (and hence reducing subsurface CO2 consumption), depending on the light transparency of the plastic ranging from 0% for opaque plastic to 80% for clear plastic (Goddijn-Murphy and Dufaur, 2018), and abundance. Plastics degrade under the influence of ultraviolet B (UVB; 280-320 nm) light in the sun (Royer et al., 2018), which implies that shading by plastic would reduce GHG production from MPL breakdown. Naidoo and Glassom (2019) measured average sea surface microplastic concentrations from 0.07 to 42 plastic particles/100 m2 at various locations in different parts of the world. Koestner et al. (2023) measured some modification of the underwater light field by microplastics but not enough to significantly affect the underwater light levels in UV-PAR. However, Galgani and Loiselle (2019) observed increased colored dissolved organic matter (CDOM) at the sea surface and immediate underlying water in the presence of microplastic particles that may provide additional photochemical protection in surface waters (Tilstone et al., 2010).
4.2 Marine plastic litter and kw
4.2.1 Small scale sea surface roughness
Direct gas transfer through the unbroken surface is dependent on the short capillary-gravity wave portion of the surface wave (Bock et al., 1999). Small-scale sea surface roughness, represented by the mean square slope, 〈s2〉, of short capillary gravity waves, has therefore been used instead of wind in kw parameterizations as an alternative. From low to moderate wind speed, kw increases linearly with 〈s2〉 in laboratory and field observations (Garbe et al., 2014). Evans and Ruf (2022) associated microplastics with the suppression of roughening of the ocean surface by winds. However Sun et al. (2023) found that the damping effect of plastic particles on<s2> can be only observed for fractions of coverage above O(5~10%), that is much larger than typical for microplastic of O(0.1%). It is possible that this damping is caused by a factor correlated with the presence of microplastics, such as surfactants on the ocean surface (Evans and Ruf, 2022). According to D’Asaro et al. (2018), man-made and natural surfactants accumulate in surface current convergence zones, where debris will also accumulate. It is possible that biofouling of MPL or increased biological activity due to the presence of MPL, generates surfactants (section 4.1.4). It is also possible that surfactants are a by-product of plastic breakdown. Pereira et al. found up to 32% reduction in CO2 exchange compared to surfactant-free sea surface water in the Atlantic Ocean (Pereira et al., 2018) and up to 50% in coastal waters of the North Sea (Pereira et al., 2016). The addition of plastic particles to the MBL could create a conduit that breaks the surface film. It is unknown to what extent floating macroplastics could affect 〈s2〉 and near surface turbulence. Prytherch et al. (2017) did not find proof of increased waterside turbulent forcing by floating sea ice.
4.2.2 Bubbles
For poorly soluble gases such as CO2, N2O, and CH4, and higher wind speeds, bubble-mediated gas transfer through the broken sea surface must be included and is expected to scale with fractional whitecap coverage at the surface, W (Woolf, 1997). Recent work has shown that relationships between W and the energy dissipation rate of breaking waves also need to consider the penetration depth of bubble plumes during active breaking, suggesting the latter may be a better proxy for bubble-mediated gas transfer (Callaghan, 2018). The effect of MPL on bubbles and their depth is not known. Other questions are: does the presence of MPL increase or decrease W and does MPL affect bubble size, void fraction of the bubbles, and cleanliness/mobility of the bubble surface (Woolf et al., 2007). Bubbles and surface debris generally align with the wind in convergence zones in the ocean surface called windrows (van Sebille et al., 2020). Windrows are common and clearly identifiable features of the surface ocean where MPL and biological materials accumulate and air-sea gas transfer velocity may be enhanced due to bubble action
5 Discussion
Many different factors control air-sea gas transfer of GHGs and the presence of MPL can both enhance and reduce ΔC, kw, and resulting GHG fluxes (Figure 1; Table 1). Most relevant research has been about biological and chemical factors, such as plastic breakdown, plastic biofouling, and consequences for microalgae. There is less known about physical factors of MPL that could interfere with air-sea gas exchange, such as SST, transport,<s2>, and W. MPL is a growing concern, especially where concentrations are high. More research is required to determine MPL effects on air-sea gas transfer. For this we will need to study the effect of MPL on many different factors that control air-sea gas exchange parameters and the relation between those factors and parameters under different conditions (Table 1). Production of gases (Royer et al., 2018) may be less important than alteration of the sea surface physics, but we need further research to distinguish which mechanisms could be more important than others, depending on the type of MPL (e.g., size and composition) and the location and scale of the investigation. A combination of laboratory and field experiments is appropriate, since specific mechanisms can be investigated in the laboratory, but effects must be verified in the field. This will enable us to better calculate and map air-sea GHG fluxes in MPL polluted environments at regional and global scales using existing tools tailored to assess the overall impact of MPL on the marine environment.
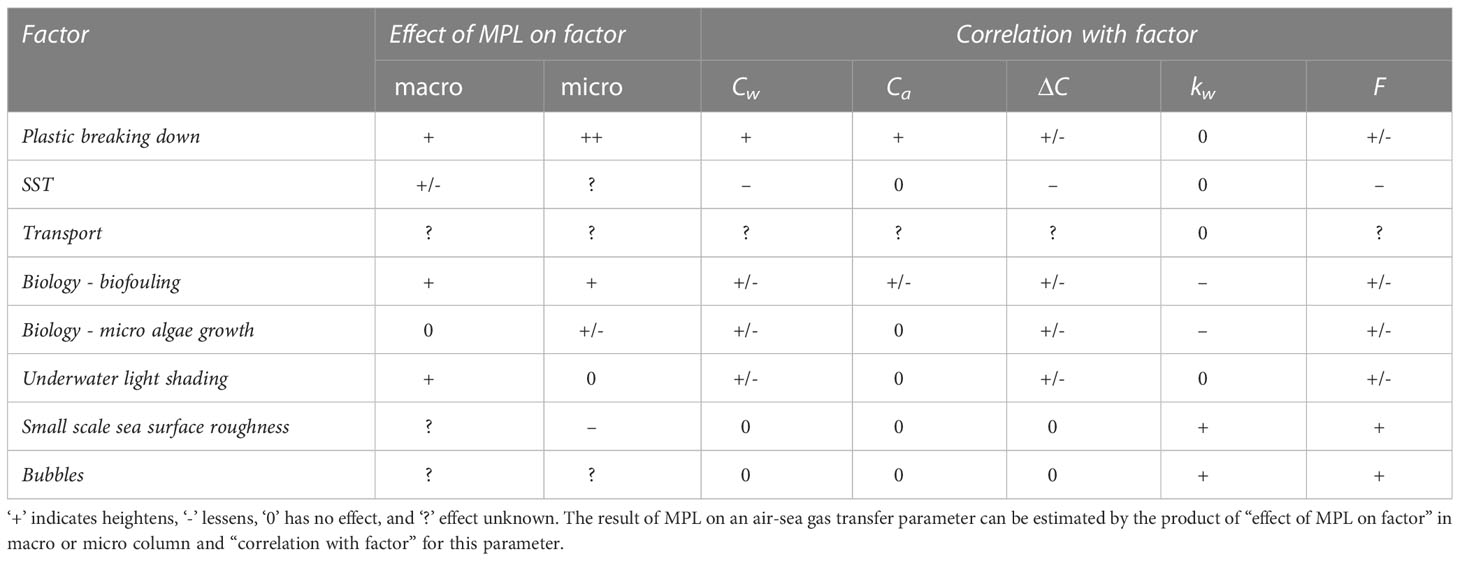
Table 1 Main factors that control air-sea gas exchange of poorly soluble GHG CO2, the effect of MPL on those factors, and how these correlate with air-sea gas transfer parameters.
Author contributions
LG-M: Conceptualization, Investigation, Writing – original draft, revision and editing, Funding Acquisition. DW, RP, AC, CM, and JP: Investigation, Writing – original draft, revision and editing. All authors contributed to the article and approved the submitted version.
Funding
This research was funded by the Discovery Element of the European Space Agency’s Basic Activities, grant number 4000132579/20/NL/GLC. RP acknowledges support to the BOOGIE project from the ERC under the European Union’s Horizon 2020 research and innovation program (grant number 949495). JP acknowledges support to the SURETY project from Polish National Science Centre, NCN (grant number 2021/41/B/ST10/00946). AC acknowledges funding from the Natural Environment Research Council [grant number NE/T000309/1].
Acknowledgments
This is a contribution to the Surface Ocean-Lower Atmosphere Study (SOLAS) integrated topic on Science and Society. This paper contributes to the science plan of the Surface Ocean-Lower Atmosphere Study (SOLAS), which is partially supported by the U.S. National Science Foundation (Grant OCE-1840868) via the Scientific Committee on Oceanic Research (SCOR).The authors would like to acknowledge revision and editing of this paper by Benjamin Williamson.
Conflict of interest
The authors declare that the research was conducted in the absence of any commercial or financial relationships that could be construed as a potential conflict of interest.
Publisher’s note
All claims expressed in this article are solely those of the authors and do not necessarily represent those of their affiliated organizations, or those of the publisher, the editors and the reviewers. Any product that may be evaluated in this article, or claim that may be made by its manufacturer, is not guaranteed or endorsed by the publisher.
References
Arenas F., Vaz-Pinto F. (2014). “Marine algae as carbon sinks and allies to combat global warming. chap 5,” in Marine algae: biodiversity, taxonomy, environmental assessment, and biotechnology. Eds. Pereira L., Magalhaes Neto J. (London: CRC Press), 178–194. doi: 10.1201/b17540-6
Bargagli R., Rota E. (2023). Microplastic interactions and possible combined biological effects in Antarctic marine ecosystems. Animals 13, 162. doi: 10.3390/ani13010162
Bergmann M., Collard F., Fabres J., Gabrielsen G. W., Provencher J. F., Rochman C. M., et al. (2022). Plastic pollution in the Arctic. Nat. Rev. Earth Environ. 3, 323–337. doi: 10.1038/s43017-022-00279-8
Bock E. J., Hara T., Frew N. M., McGillis W. R. (1999). Relationship between air-sea gas transfer and short wind waves. J. Geophys. Res. 104 (C11), 25,821–25,831. doi: 10.1029/1999JC900200
Callaghan A. H. (2018). On the relationship between the energy dissipation rate of surface-breaking waves and oceanic whitecap coverage. J. Phys. Oceanogr. 48, 2609–2626. doi: 10.1175/JPO-D-17-0124.1
Coppola G., Gaudio M. T., Lopresto C. G., Calabro V., Curcio S., Chakraborty S. (2021). Bioplastic from renewable biomass: a facile solution for a greener environment. Earth Syst. Environ. 5, 231–251. doi: 10.1007/s41748-021-00208-7
Cornejo-D’Ottone M., Molina V., Pavez J., Silva N. (2020). Greenhouse gas cycling by the plastisphere: the sleeper issue of plastic pollution. Chemosphere 246, 125709. doi: 10.1016/j.chemosphere.2019.125709
D’Asaro E., Lee C., Rainville L., Harcourt R., Thomas L. (2011). Enhanced turbulence and energy dissipation at ocean fronts. Science 332 (6027), 318–322. doi: 10.1126/science.1201515
D’Asaro E. A., Shcherbinab A. Y., Klymakc J. M., Molemakere J., Novellif G., Guigand C. M., et al. (2018). Ocean convergence and the dispersion of flotsam. Proc. Natl. Acad. Sci. 115, 1162–1167. doi: 10.1073/pnas.1718453115
Donlon C. J., Nightingale T. J., Sheasby T., Turner J., Emery W. J. (1999). Implications of the oceanic thermal skin temperature deviation at high wind speed. Geophys. Res. Lett. 26, 2505–2508. doi: 10.1029/1999GL900547
Ehhalt D., Prather M., Dentener F., Derwent R., Edward J., Dlugokencky E., et al. (2001). “Atmospheric chemistry and greenhouse gases. chap 4,” in IPCC - climate change 2001: the scientific basis (Cambridge: Cambridge University Press). Available at: https://www.grida.no/publications/270.
Evans M. C., Ruf C. S. (2022). Toward the detection and imaging of ocean microplastics with a spaceborne radar. IEEE Trans. Geosci. Remote Sens. 60, 1–9. doi: 10.1109/TGRS.2021.3081691
Fazey F. M., Ryan P. G. (2016). Biofouling on buoyant marine plastics: an experimental study into the effect of size on surface longevity. Environ. Pollut. 210, 354–360. doi: 10.1016/j.envpol.2016.01.026
Ford H. V., Jones N. H., Davies A. J., Godley B. J., Jambeck J. R., Napper I., et al. (2022). The fundamental links between climate change and marine plastic pollution. Sci. Total Environ. 806 (Pt 1), 150392. doi: 10.1016/j.scitotenv.2021.150392
Galgani L., Loiselle S. A. (2019). Plastic accumulation in the Sea surface microlayer: an experiment-based perspective for future studies. Geosci 9, 66. doi: 10.3390/geosciences9020066
Galgani L., Loiselle S. A. (2021). Plastic pollution impacts on marine carbon biogeochemistry. Environ. Pollut. 268 (Pt A), 115598. doi: 10.1016/j.envpol.2020.115598
Galgani L., Tzempelikou E., Kalantzi I., Tsiola A., Tsapakis M., Pitta P., et al. (2023). Marine plastics alter the organic matter composition of the air-sea boundary layer, with influences on CO2 exchange: a large-scale analysis method to explore future ocean scenarios. Sci. Total Environ. 857, 159624. doi: 10.1016/j.scitotenv.2022.159624
Garbe C. S., Rutgersson A., Boutin J., Delille B., Fairall C. W., Gruber N., et al. (2014). “Transfer across the air-sea interface. chap 2,” in Ocean-atmosphere interactions of gases and particles. Eds. Liss P. S., Johnson M. T. (New York: NY: Springer Earth System Sciences), 55–144. doi: 10.1007/978-3-642-25643-12
GESAMP (2019). Guidelines or the monitoring and assessment of plastic litter and microplastics in the ocean. Eds. Kershaw P. J., Turra A., Galgani F. (Nairobi: IMO/FAO/UNESCO-IOC/UNIDO/WMO/IAEA/UN/UNEP/UNDP/ISA Joint Group of Experts on the Scientific Aspects of Marine Environmental Protection). Rep. Stud. GESAMP No. 99, 130p.
Goddijn-Murphy L. M., Dufaur J. (2018). Proof of concept for a model of light reflectance of plastics floating on natural waters. Mar. Pollut. Bull. 135, 1145–1157. doi: 10.1016/j.marpolbul.2018.08.044
Goddijn-Murphy L., Williamson B. J., McIlvenny J., Corradi P. (2022). Using a UAV thermal infrared camera for monitoring floating marine plastic litter. Remote Sens. 14, 3179. doi: 10.3390/rs14133179
Goddijn-Murphy L., Woolf D. K., Callaghan A. H., Nightingale P. D., Shutler J. D. (2016). A reconciliation of empirical and mechanistic models of the air-sea gas transfer velocity. J. Geophys. Res. 121, 818–835. doi: 10.1002/2015JC011096
Holding T., Ashton I. G., Shutler J. D., Land P. E., Nightingale P. D., Rees A. P., et al. (2019). The FluxEngine air–sea gas flux toolbox: simplified interface and extensions for in situ analyses and multiple sparingly soluble gases. Ocean Sci. 15, 1707–1728. doi: 10.5194/os-15-1707-2019
Johnson M. T. (2010). A numerical scheme to calculate temperature and salinity dependent air-water transfer velocities for any gas. Ocean Sci. 6, 913–932. doi: 10.5194/os-6-913-2010
Koestner D., Foster R., El-Habashi A. (2023). “On the potential for optical detection of microplastics in the ocean,” in Frontiers in ocean observing: emerging technologies for understanding and managing a changing ocean. Oceanography. 36 (Supplement 1), 49–51. doi: 10.5670/oceanog.2023.s1.15
Kooi M., Reisser J., Slat B., Ferrari F. F., Schmid M. S., Cunsolo S., et al. (2016). The effect of particle properties on the depth profile of buoyant plastics in the ocean. Sci.Rep 6, 33882. doi: 10.1029/2012GL051116
Kukulka T., Proskurowski G., Morét-Ferguson S. E., Meyer D. W., Law K. L. (2012). The effect of wind mixing on the vertical distribution of buoyant plastic debris. Geophys. Res. Lett. 39, L07601. doi: 10.1029/2012GL051116
Kvale K. (2022). Implications of plastic pollution on global marine carbon cycling and climate. Emerg. Top. Life Sci. 6 (4), ETLS20220013. doi: 10.1042/ETLS20220013
Kvale K., Hunt C., James A., Koeve W. (2023). Regionally disparate ecological responses to microplastic slowing of faecal pellets yields coherent carbon cycle response. Front. Mar. Sci. 10. doi: 10.3389/fmars.2023.1111838
Kvale K., Oschlies A. (2023). Recovery from microplastic-induced marine deoxygenation may take centuries. Nat. Geosci. 16, 10–12. doi: 10.1038/s41561-022-01096-w
Kvale K., Prowe A. E. F., Chien C.-T., Landolfi A., Oschlies A. (2021). Zooplankton grazing of microplastic can accelerate global loss of ocean oxygen. Nat. Commun. 12, 2358. doi: 10.1038/s41467-021-22554-w
Lehmann M., Häusl F. P., Gekle S. (2023). Modeling of vertical microplastic transport by rising bubbles. Micropl. Nanopl. 4 (2023), 3. doi: 10.1186/s43591-023-00053-7
Lincoln S., Andrews B., Birchenough S. N. R., Chowdhury P., Engelhard G. H., Harrod O., et al. (2022). Marine litter and climate change: inextricably connected threats to the world’s oceans. Sci. Total Environ. 837, 155709. doi: 10.1016/j.scitotenv.2022.155709
MacLeod M., Arp H. P. H., Tekman M. B., Jahnke A. (2021). The global threat from plastic pollution. Science 373, 61–65. doi: 10.1126/science.abg5433
Naidoo T., Glassom D. (2019). Sea-Surface microplastic concentrations along the coastal shelf of KwaZulu–natal, south Africa. Mar. Pollut. Bull. 149, 110514. doi: 10.1016/j.marpolbul.2019.110514
Naik R. K., Naik M. M., D’Costa P. M., Shaikh F. (2019). Microplastics in ballast water as an emerging source and vector for harmful chemicals, antibiotics, metals, bacterial pathogens and HAB species: a potential risk to the marine environment and human health. Mar. Pollut. Bull. 149, 110525. doi: 10.1016/j.marpolbul.2019.110525
Nguyen N. H. A., El-Temsah Y. S., Cambier S., Calusinska M., Hrabak P., Pouzar M., et al. (2021). Attached and planktonic bacterial communities on bio-based plastic granules and micro-debris in seawater and freshwater. Sci. Total Environ. 785, 147413. doi: 10.1016/j.scitotenv.2021.147413
Oberbeckmann S., Löder M. G. J., Labrenz M. (2015). Marine microplastic-associated biofilms – a review. Environ. Chem. 12, 551–562. doi: 10.1071/EN15069
Ocean Decade (2022) The ocean decade – the science we need for the ocean we want. Available at: https://www.oceandecade.org (Accessed 20 September 2022).
Paulmier A., Ruiz-Pino D., Garçon V. (2011). CO2 maximum in the oxygen minimum zone (OMZ). Biogeosciences 8, 239–252. doi: 10.5194/bg-8-239-2011
Pereira R., Ashton I., Sabbaghzadeh B., Shutler J. D., Upstill-Goddard R. C. (2018). Reduced air–sea CO2 exchange in the Atlantic ocean due to biological surfactants. Nat. Geosci. 11, 492–496. doi: 10.1038/s41561-018-0136-2
Pereira R., Schneider-Zapp K., Upstill-Goddard R. C. (2016). Surfactant control of gas transfer velocity along an offshore coastal transect: results from a laboratory gas exchange tank. Biogeosciences 13, 3981–3989. doi: 10.5194/bg-13-3981-2016
Phan S., Luscombe C. K. (2023). Recent trends in marine microplastic modeling and machine learning tools: potential for long-term microplastic monitoring. J. Appl. Phys. 133, 020701. doi: 10.1063/5.0126358
Prata J. C., da Costa J. P., Lopes I., Duarte A. C., Rocha-Santos T. (2019). Effects of microplastics on microalgae populations: a critical review. Sci. Total Environ. 665, 400–405. doi: 10.1016/j.scitotenv.2019.02.132
Prytherch J., Brooks I. M., Crill P. M., Thornton B. F., Salisbury D. J., Tjernström M., et al. (2017). Direct determination of the air-sea CO2 gas transfer velocity in Arctic sea ice regions. Geophys. Res. Lett. 44, 3770–3778. doi: 10.1002/2017GL073593
Rauscher A., Meyer N., Jakobs A., Bartnick R., Lueders T., Lehndorff E. (2023). Biodegradable microplastic increases CO2 emission and alters microbial biomass and bacterial community composition in different soil types. Appl. Soil Ecol. 182, 104714. doi: 10.1016/j.apsoil.2022.104714
Royer S.-J., Ferrón S., Wilson S. T., Karl D. M. (2018). Production of methane and ethylene from plastic in the environment. PloS One 13 (8), e0200574. doi: 10.1371/journal.pone.0200574
Rutgersson A., Smedman A., Sahlée E. (2011). Oceanic convective mixing and the impact on air–sea gas transfer velocity. Geophys. Res. Lett. 38 (2), L02602. doi: 10.1029/2010GL045581
Shen M., Ye S., Zeng G., Zhang Y., Xing L., Tang W., et al. (2020). Can microplastics pose a threat to ocean carbon sequestration? Mar. Pollut. Bull. 150, 110712. doi: 10.1016/j.marpolbul.2019.110712
Shin Y., Deike L., Romero L. (2022). Modulation of bubble-mediated CO2 gas transfer due to wave-current interactions. Geophys. Res. Lett. 49, e2022GL100017. doi: 10.1029/2022GL100017
Shutler J. D., Land P. E., Piolle J., Woolf D. K., Goddijn-Murphy L., Paul F., et al. (2016). FluxEngine: a flexible processing system for calculating atmosphere–ocean carbon dioxide gas fluxes and climatologies. J. Atmos. Ocean. Technol. 33, 741–756. doi: 10.1175/JTECH-D-14-00204.1
Sun Y., Bakker T., Ruf C., Pan Y. (2023). Effects of microplastics and surfactants on surface roughness of water waves. Sci. Rep. 13, 1978. doi: 10.1038/s41598-023-29088-9
Takahashi T., Sutherland S. C., Wanninkhof R., Sweeney C., Feely R. A., Chipman D. W., et al. (2009). Climatological mean and decadal change in surface ocean pCO2, and net sea–air CO2 flux over the global oceans. Deep-Sea Res. Pt. II 56, 554–577. doi: 10.1016/j.dsr2.2008.12.009
Tilstone G. H., Airs R. L., Vicente V. M., Widdicombe C. E., Llewellyn C. A. (2010). High concentrations of mycosporine-like amino acids and colored dissolved organic matter in the sea surface microlayer off the Iberian peninsula. Limnol. Oceanogr. 55, 1835–1850. doi: 10.4319/lo.2010.55.5.1835
United Nations (2022) THE 17 GOALS | sustainable development. Available at: https://sdgs.un.org/goals (Accessed 20 September 2022).
van Sebille E., Aliani S., Lavender Law K., Maximenko N., Alsina J. M., Bagaev A., et al. (2020). The physical oceanography of the transport of floating marine debris. Environ. Res. Lett. 15, 023003. doi: 10.1088/1748-9326/ab6d7d
Ward B. B., Devol A. H., Rich J. J., Chang B. X., Bulow S. E., Naik H., et al. (2009). Denitrification as the dominant nitrogen loss process in the Arabian Sea. Nature 461, 78–81. doi: 10.1038/nature08276
Watson A. J., Schuster U., Shutler J. D., Holding T., Ashton I. G. C., Landschützer P., et al. (2020). Revised estimates of ocean-atmosphere CO2 flux are consistent with ocean carbon inventory. Nat. Commun. 11, 4422. doi: 10.1038/s41467-020-18203-3
Woolf D. (1997). “Bubbles and their role in air-sea gas exchange,” in The sea surface and global change. Eds. Liss P., Duce R. (Cambridge: Cambridge University Press), 173–205.
Woolf D. K., Land P. E., Shutler J. D., Goddijn-Murphy L. M., Donlon C. J. (2016). On the calculation of air-sea fluxes of CO2 in the presence of temperature and salinity gradients. J. Geophys. Res. Oceans 121, 1229–1248. doi: 10.1002/2015JC011427
Woolf D. K., Leifer I. S., Nightingale P. D., Rhee T. S., Bowyer P., Caulliez G., et al. (2007). Modelling of bubble-mediated gas transfer: fundamental principles and laboratory test. J. Mar. Syst. 66, 71–91. doi: 10.1016/j.jmarsys.2006.02.011
Woolf D., Shutler J. D., Goddijn-Murphy L., Watson A., Chapron B., Nightingale P. D., et al. (2019). Key uncertainties in the recent air-Sea flux of CO2. Global Biogeochem. Cycles. 33 (12), 1548–1563. doi: 10.1029/2018GB006041
Keywords: marine plastic litter, greenhouse gasses, air-sea gas transfer, air-sea interaction, carbon cycling, climate change
Citation: Goddijn-Murphy L, Woolf DK, Pereira R, Marandino CA, Callaghan AH and Piskozub J (2023) The links between marine plastic litter and the air-sea flux of greenhouse gases. Front. Mar. Sci. 10:1180761. doi: 10.3389/fmars.2023.1180761
Received: 06 March 2023; Accepted: 27 June 2023;
Published: 31 July 2023.
Edited by:
Seung-Kyu Kim, Incheon National University, Republic of KoreaReviewed by:
Karin Kvale, GNS Science, New ZealandCopyright © 2023 Goddijn-Murphy, Woolf, Pereira, Marandino, Callaghan and Piskozub. This is an open-access article distributed under the terms of the Creative Commons Attribution License (CC BY). The use, distribution or reproduction in other forums is permitted, provided the original author(s) and the copyright owner(s) are credited and that the original publication in this journal is cited, in accordance with accepted academic practice. No use, distribution or reproduction is permitted which does not comply with these terms.
*Correspondence: Lonneke Goddijn-Murphy, bG9ubmVrZS5nb2RkaWpuLW11cnBoeUB1aGkuYWMudWs=