- 1Department of Production Biology, Nofima, Ås, Norway
- 2Department of Veterinary Medical Sciences, University of Bologna, Ozzano Emilia, Italy
- 3Centro di ricerca Agricoltura e Ambiente, Consiglio per la ricerca e l’analisi dell’economia agraria-CREA-AA, Rome, Italy
- 4Department of Innovation, Consumer and Sensory Science, Nofima, Ås, Norway
- 5Blue Economy Research Area, Scottish Association for Marine Science, SAMS, Scotland, United Kingdom
- 6School of Economics and Business, Norwegian University of Life Sciences, NMBU, Ås, Norway
- 7Department of Agricultural and Food Sciences, University of Bologna Alma Mater Studiorum, Bologna, Italy
- 8Department of Agriculture Engineering, Universidad Politécnica de Cartagena, Cartagena, Spain
The objective of this perspective paper is to present and discuss how systemic innovations can deliver a step change in the way food is produced in Europe. The production of healthy, safe and affordable food can contribute toward a just transition to net zero carbon (C) for Europe. A systemic and cross sectorial approach can contribute to climate mitigation by transfer of atmospheric CO2 to the terrestrial biosphere using low trophic species (LTS), including plants, seaweed and mussels (i.e. C sequestration) and increasing organic C stocks in soils and vegetation biomass (i.e. C storage). Innovative combinations of technologies applied to LTS, processed animal protein, new crops, and diversified and integrated production systems can link the high primary productivity rates of the marine environment to the C storage capability of the terrestrial food sector. Furthermore, the important roles of both private and public sector actors and better use of systemic approaches to further elucidate the multi-dimensional and multi-level interplays in complex food systems needs consideration. This can pave the way for linking and scaling up C-neutral marine and terrestrial food production systems into a future sustainable and circular bioeconomy. This systems-based approach can address some of the challenges associated with the current farming systems, as interdisciplinary research on aquaculture innovation can support the development of a resilient and sustainable food system. Examples of technologies provided include: a custom configured and digital user-oriented co-creation approach for Responsible Research and Innovation (RRI), a WebGIS tool on soil C storage, innovative composting methods, advanced breeding methods, new machinery for low greenhouse gas diversified orchard farming, AI model systems to improve decision support systems in management of soil, vertical farming, and animal feeding.
1 Introduction
European food production and farming systems still result in significant air, water, and soil pollution, biodiversity loss, climate change, animal welfare challenges and excessive use of natural resources, including water and energy. Agriculture is responsible for 10.3% of the EU’s greenhouse gas (GHG) emissions, of which nearly 70% come from livestock (EEA, 2019). Furthermore, nitrogen (N) and phosphorus (P) cycles exceed their safe operating space in Europe by a factor of 3.3 and 2, respectively, resulting in diffuse terrestrial, aquatic and atmospheric pollution (EEA, 2020).
The Farm to Fork strategy of the European Union (EU) responding to these challenges aims to accelerate a “just transition” for all actors to sustainable food systems, ensuring that the economic, social and environmental foundations of food and nutrition security are not compromised for current and future generations (EC, 2019; EC, 2020). This is also in line with the UN’s Sustainable Development Goals (UN, 2022). The strategy requires and builds on scalable innovative solutions, such as agro-ecological and organic practices, alternative and circular sources of protein for animal feeds (e.g. plant-based, ocean-based, insect-based, single-cell-based and animal by-products), and sustainable food from the oceans and aquaculture. In addition, the application of diversified cropping, low carbon (C) feed ingredients and farming practices of fish and marine low trophic species (LTS e.g. seaweed (SW) and mussels) have been proposed to reduce GHG emissions, mitigate climate changes, and tackle pollution and biodiversity loss (Maia et al., 2016; SAPEA, 2017; Kinley, 2020; Morais et al., 2020; Morugán-Coronado et al., 2020; Winther et al., 2020; Hoegh-Guldberg et al., 2019; Colombo et al., 2022). To innovate we need to consider the important roles of private and public sector actors, and make better use of systemic approaches to further elucidate the multi-dimensional and multi-level interplays in complex aquaculture and agriculture systems in Europe, as emphasized for aquaculture by Joffre et al. (2017). The authors argue that integration of institutional, political, economic, and socio-cultural dimensions can improve the management of the innovation process and support the development of a resilient and sustainable food system. One needs to acknowledge though, that this transformation is in reality a contested, competitive and political process that needs dialogue, compromises and negotiation of common futures and not a matter of rational design (Leeuwis et al., 2021).
Our objective is to present and discuss how such systemic innovations as a circular economy approach (Figure 1) can deliver a step change in the way food is produced in Europe, and that the production of healthy, safe and affordable food can be one solution in a just transition to net zero C for Europe.
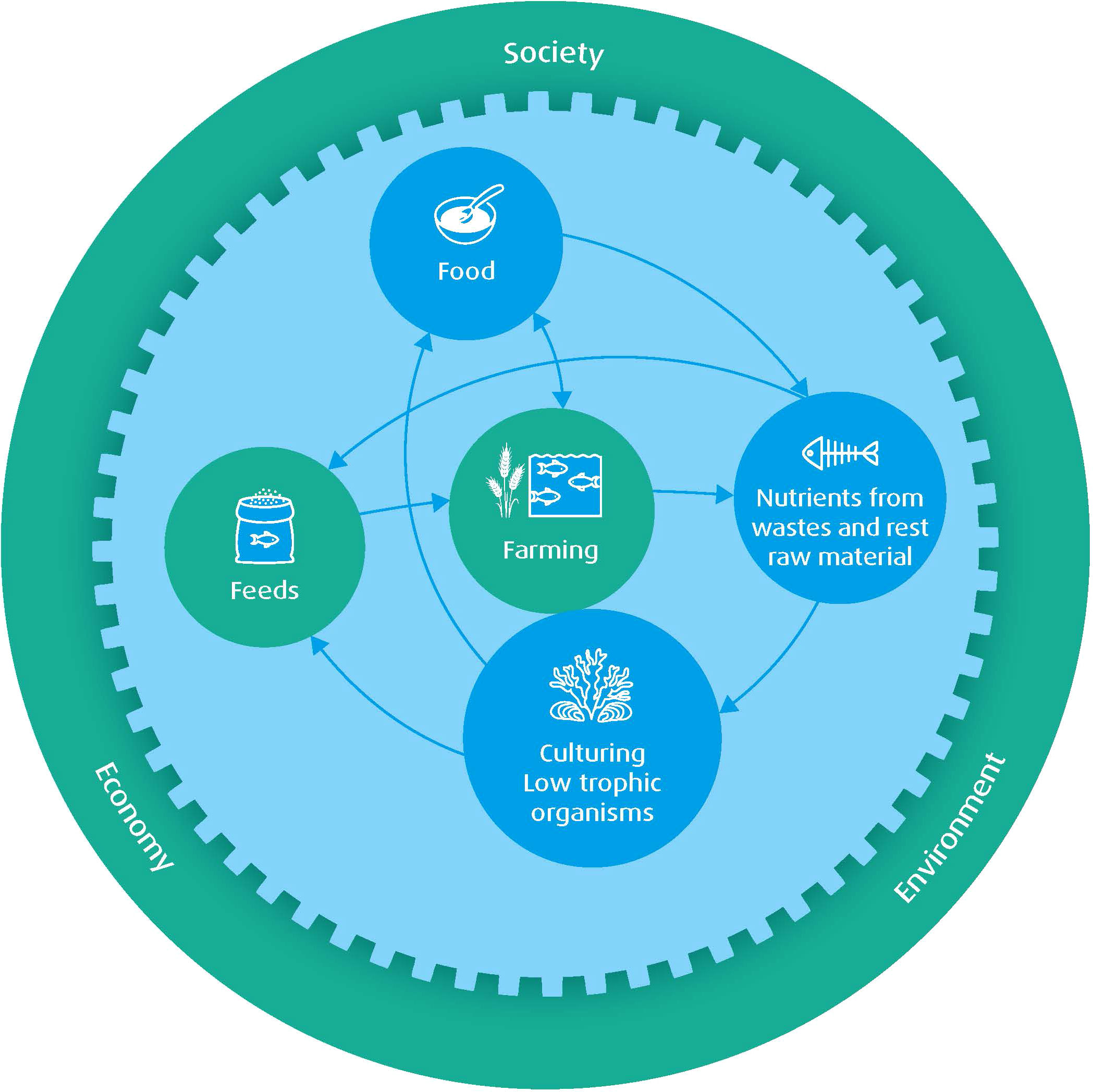
Figure 1 The vision of a climate neutral farming in a green and blue circular bioeconomy. Illustration: Krysspress/Nofima.
2 Opportunities for climate neutral marine and terrestrial farming
Climate neutral food production can be facilitated either by reducing the emissions of current or new production systems or through facilitating carbon sequestration and storage within the food production systems (Bossio et al., 2020).
2.1 Reducing the emissions from the food production system
Total GHG emissions from agriculture in EU27 were estimated to 472 Mt CO2e in 1990, whereas fish farming was estimated to represent a total of 5.1 Mt CO2e in 2010 (MacLeod et al., 2020). In 2020, the total emissions from the agriculture sector still reached 424 Mt CO2e (EEA, 2022), where enteric emissions from livestock made up 43.7% of total agricultural emissions and 80% of total agricultural methane (CH4) emissions. This makes livestock emissions the largest GHG source in agriculture and the largest source of CH4 emissions in EU (40%). Emissions from livestock may be reduced by means of feeding strategies, such as dietary inclusion of SW or microalgae (Maia et al., 2016; Roque et al., 2019; Kinley, 2020; Morais et al., 2020), high forage quality, increased levels of concentrates (Van Gastelen et al., 2019), and feed additives (e.g. probiotics, polyphenols, tannins) (Palangi et al., 2022). We suggest that selected dietary interventions can reduce enteric CH4 gas emissions (by 25% in 2030) while improving cattle production and avoiding negative effects on the animal health and welfare and the quality of dairy products and meat (Table 1).
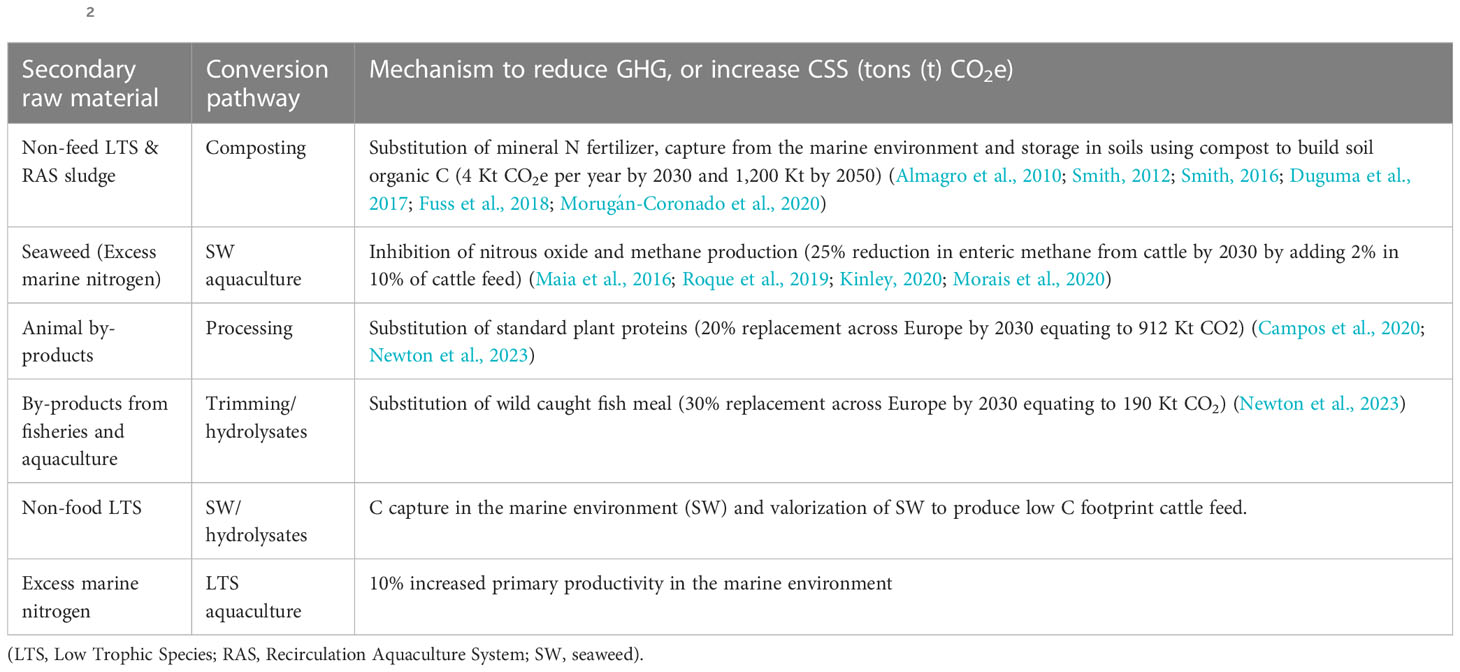
Table 1 Circular pathways linking aquatic and terrestrial food production and assumed mechanisms of reduced GHG and increased CSS (resulting tons CO2e).
Ocean-based climate mitigation options offer significant potential to limit global warming by reducing GHG emissions (e.g. providing low C farm seafood as an alternative to land produced protein) (SAPEA, 2017; Hoegh-Guldberg et al., 2019). Spillias et al. (2023) modeled the global expansion potential of SW farming and explored how increased SW utilization for dietary, livestock feed and fuel production could affect the environmental footprint of agriculture. It was concluded that global production of SW has the potential to reduce the environmental impacts of terrestrial agriculture, but caution is needed to ensure that these challenges are not displaced from the land to the ocean. Further, the aquatic food production sector has shown remarkable growth worldwide over the last decades and is still the fastest growing animal-producing sector (FAO, 2018). However European aquaculture production is stagnating, and finfish farming comprises most of the value of the production (FAO, 2022). Feed for finfish is responsible for 90% of the GHG footprint of farmed fish, mostly through their highly processed vegetable ingredients such as soybean protein concentrate, glutens, rapeseed and palm oil (MacLeod et al., 2020; Winther et al., 2020; Newton et al., 2023) Product diversification strategies encouraging the farming of non-fed finfish and LTS, such as SW and shellfish, would reduce GHG emissions while maintaining the supply of seafood to Europe. For expansion of global food production from the ocean, marine aquaculture is identified to have the largest potential. In particular aquaculture with filter feeding invertebrates (e.g. molluscs) for direct human consumption should be noted, or finfish cultured together with cultivated algae to mitigate environmental impact of finfish production in a more ecological way (Ellis and Tiller, 2019). Stetkiewicz et al. (2022) found that seafood remains under-researched compared to the role of terrestrial animal and plant production in food security. Furthermore, these authors concluded that far more attention is needed to the role of seafood in global food security, and call for the integration of seafood in a wider interdisciplinary approach to global food system research. For example, the social readiness level regarding consumer behavior and solutions to area conflicts need to be increased before these types of innovations can be scaled up in a sustainable way. Aquaculture food products, such as farmed fish from freshwater and marine aquaculture and blue mussels generally show a low C footprint (0.6-5 kg CO2e kg-1 edible product) compared to meat products from poultry (6.5 CO2e kg-1) and livestock (8.6-19 kg CO2e kg-1 meat), assuming no land-use change (Fry, 2011; Poore and Nemecek, 2018). In aquaculture, there is a significant potential to improve the C footprint of farmed fish by using circular feed ingredients (including fish and terrestrial animal by-products) and feeding strategies with low C footprint (Kinley, 2020; Winther et al., 2020; Colombo et al., 2022).
2.2 Carbon sequestration and storage (CSS)
Soil plays a pivotal role by a net transfer of atmospheric CO2 to the terrestrial biosphere. Soil can contribute to the mitigation of climate change: for example respiration from soil and vegetation is responsible for a flux of 118.7 Pg C yr-1, lower than the photosynthesis flux (123 Pg C yr−1), turning land into a C sink. The stock of C in soils is three times higher than in vegetation and twice the C stock in the atmosphere (Smith, 2012). Biomass is the second largest terrestrial compartment of stored C, so diversified cropping systems (mostly agroforestry systems) have been proposed as a sustainable strategy to increase land productivity, biodiversity and C sequestration in soil and biomass to ensure climate neutrality (Duguma et al., 2017; Morugán-Coronado et al., 2020). Diversified cropping systems with adaptation of machinery for sowing, cutting, pruning, weed control and harvesting, conservation agriculture and soil amendments with by-products from aquaculture and animal farming can decrease GHG emissions (by 30% in 2030) and enhance CSS in soil and perennial biomass (483 Mt CO2e yr-1) (Almagro et al., 2010; IPCC, 2019; Morugán-Coronado et al., 2020; Brooker et al., 2021; EEA, 2022). Soil C sequestration represents a significant GHG removal strategy - also called negative emission technology (Smith, 2016). Increasing the soil organic carbon (SOC), essential for soil quality that influence agricultural production, delivery of ecosystem services and food security, can be viewed as a win-win strategy. For example C sequestration in soils from SW and CaCO3 from SW and mussel farming, reused in a renewable cycle that store their C for prolonged time periods, and from crop production on land can therefore have significant impacts on the atmosphere and climate change (Fodrie et al., 2017; Chenu et al., 2019; Morugán-Coronado et al., 2020; Alonso et al., 2021). Global estimates of soil C sequestration potential vary considerably and is altered by soil amendments. Fuss et al. (2018) indicates a potential of up to 5 Gt CO2 yr−1 for soil C sequestration and 0.5–2 Gt CO2 yr−1 for biochar.
This potential can be further increased; the efficiency of both crop rotations with cereals/ley/legumes/catch crops and agroforestry systems in fruit trees with alley cropping of herbs, vegetables and fodder can be improved to decrease inputs (pesticides, fertilizers, water) and increase CSS (Tiefenbacher et al., 2021). Crop diversification, as a nature-based approach, increases biodiversity and soil biological activity including attraction of natural antagonists to pests/diseases, reduces erosion and improves soil quality with increased C and water storage capacity, reducing the need of external input. Despite the large cropland surface occupied by cereals in European agricultural land (48%), tree crops represent 10% of total cropland in Europe, highly relevant for the Mediterranean region, with a potential to CSS in biomass as ~200-500 kg C per tree in the long-term (Almagro et al., 2010; Ma et al., 2020). For more details on assumptions of CO2e emissions and CSS, see Table 1.
In terms of aquatic food production, the culturing of low trophic organisms offers significant opportunity to sequester carbon (Hoegh-Guldberg et al., 2019). SW cultivation can capture 1500T of CO2 yr-1 km-2, that can be increased if the production is managed purposely to increase carbon sequestration (Broch et al., 2019). However, to make a difference to climate change this carbon must be effectively removed from the carbon cycle for meaningful periods of times (generally more than 100 years). In countries where the large-scale cultivation of seaweed for food is already well developed, cultivation is resulting in significant sequestration along with other environmental benefits (Zheng et al., 2019).
3 Overall concept
In line with the Farm to Fork strategy we propose the following strategic priorities:
1. Linking marine and terrestrial systems by closing the global C and N cycle using composted and processed marine and terrestrial waste and by-products in agricultural soils for long-term C storage (see Figure 2). Useful tools based on digital technologies that can contribute to this include: WebGIS tool on predicting soil C storage and AI model systems to improve decision support systems (DSS) in management of soil, vertical farming, and animal feeding.
2. Increase C sequestration by diversifying crop agriculture and aquaculture, including kickstarting a SW bioeconomy in Europe. Examples of relevant innovations here are: New machinery for low GHG diversified orchard farming and advanced breeding methods, including selection for new low trophic aquaculture species.
3. Reduce GHG emissions by developing circular feed systems for farm fish and cattle using cultured SW, by-products from fishery, aquaculture and processed terrestrial farm animal by-products. Innovative urban and vertical farming solutions shorten the distance from food production to urban consumers and to input resources, reducing GHG emissions from transportation while limiting land area required. Vertical farming is the cultivation of plants (mostly fresh vegetables and herbs, to date) in light opaque and thermally insulated chambers, where artificial lighting is used, and full control of environmental parameters is provided. In vertical farms, plants are grown on stacked tiers, using hydroponics, enabling substantial savings of water and mineral nutrients, as well as the use of land (ranging 90 to 99% as compared with greenhouse and open-field cultivation, respectively) (Graamans et al., 2018; Orsini et al., 2020; van Delden et al., 2021).
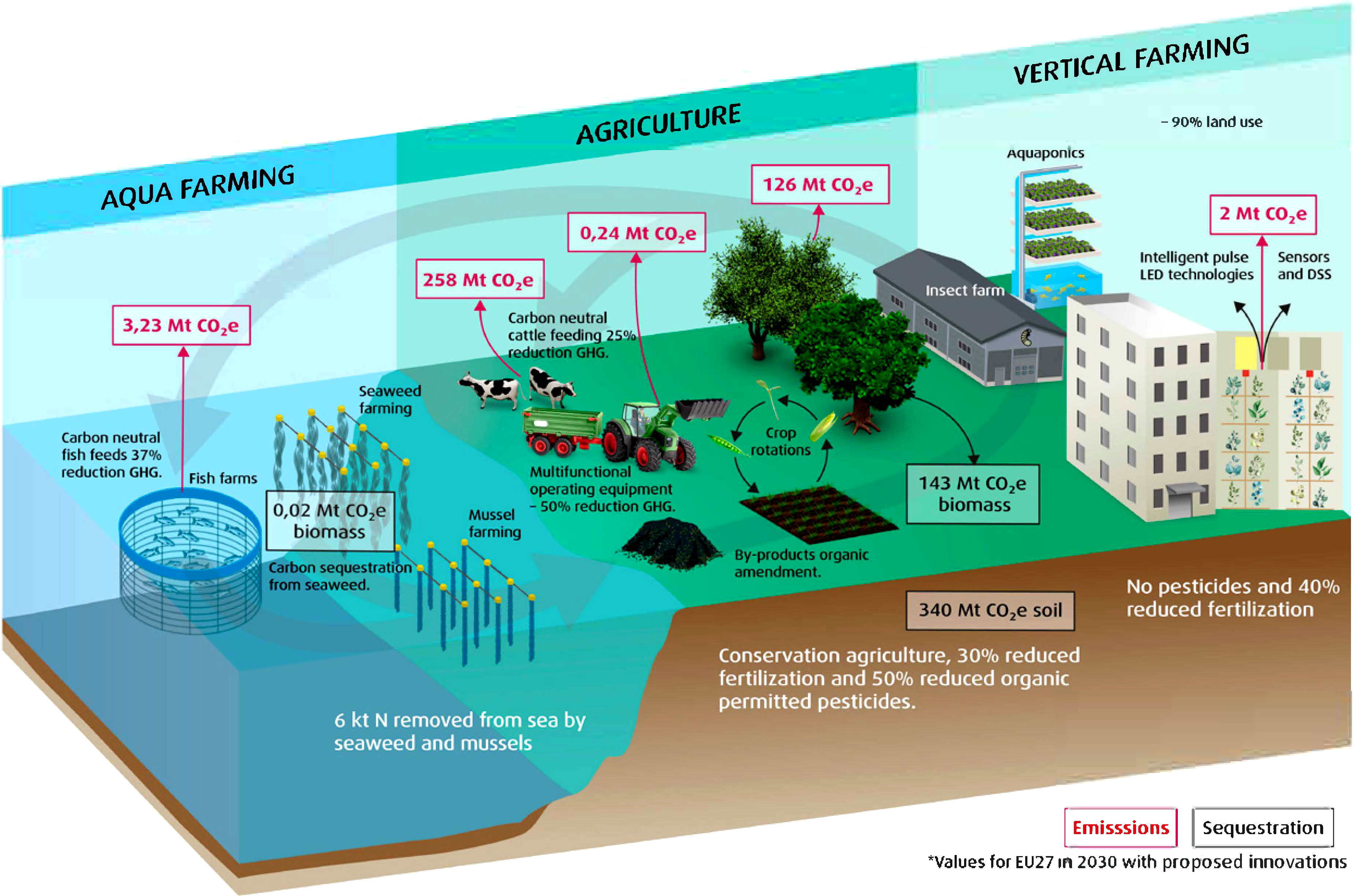
Figure 2 Overall climate neutral farming with suggested innovations in 2030, and resulting annual GHG emissions and CSS in EU27 showing total CSS (483 Mt CO2e) exceeding total GHG emissions (231 Mt CO2e) assuming the same amount of food produced. Annual nitrogen (N) mass (6 kT) removed from sea [assuming 100 kT fresh weight SW and 800 kT mussels produced in 2030 and N composition data reviewed by Thomas et al. (2022)]. This strategy providing nutrient for food and feed and replacing mineral fertilizers, demonstrates how linking aquaculture and agriculture sectors contributes to a circular food system. (DSS, Decision Support system.). Illustration: Krysspress/Nofima.
This trans-sectorial and circular approach will lead the way for leveraging the significant potential to increase climate neutral farming and seafood production in Europe through a systems-based approach to food production that circumvents the siloed approach of agriculture and aquaculture. By this it finally allows for the development of integrated and circular food production across the land sea interface. The proposed innovations could contribute to reduce GHG emissions and increase CSS to achieve overall climate neutral farming by 2030 (Figure 2). The total potential CSS (483 Mt CO2e), with the adoption of proposed innovations on 8% of agriculture land in the EU 27 in 2030, will exceed the total GHG emissions in both agriculture and fish farming in 1990 as well as emissions reduced with innovations here (335 Mt CO2e).
4 Discussion
A holistic approach of Responsible Research and Innovation (RRI) is required to maximize the exploitation of the innovations proposed here and scale them up to climate neutral farming at a regional level. RRI is a dynamic and iterative process, in which all relevant stakeholders participate, respond and are responsible for both the research/innovation process and its outcomes. RRI aligns both the process and its outcomes with the values, needs, expectations and concerns of the society. The RRI framework of EU includes the following key areas: ethics, societal engagement, gender equality, open access, and science education. This implies a process of structured cooperation between a myriad of actors from various parts of society, including researchers, citizens, policy makers, business and third sector organizations and clusters. This multi-actor, transdisciplinary approach is often challenging, but needs to be applied throughout such comprehensive innovation processes (Gonera and Pabst, 2019). In complex global systems such as aquaculture, the development and translation process of technology and innovation platforms is nonlinear and involves a plurality of stakeholders with diverging interests. Inherent and emerging frictions can be addressed by designing good collaboration platforms adapted to local and national conditions and stakeholders. Intermediaries need to be able to facilitate an iterative, reflexive innovation process aligned with the existing expectations and practices of ‘multi-stakeholder’ innovation in each country and thus enable for a wider process of food systems innovation (Bush et al., 2021).
The sustainability of the systemic solutions and their challenges and potential to generate significant positive impacts should be assessed, aiming at climate neutral farming through reduced global warming potential, and feasible transition toward sustainable food systems. Although the potential of replacing part of food-competing feedstuffs with food system by-products and residues, the prospects face various challenges (Sandström et al., 2022). For example, the production of alternative feeds can be limited by the availability of by-products and residues, nutritional aspects (i.e. variability in nutritional composition, high ash content), consumer acceptance, or by existing regulations, such as bans on intra-species feed recycling in farmed animal production in the European Union. An integrated assessment approach to these innovations addressing the environmental, economic and social dimensions of sustainability is needed. This should also involve Life Cycle Costing (Ciroth et al., 2008; De Menna et al., 2018) and the monetization of these impacts (Aanesen et al., 2023) in an amended Cost-benefit analysis in agreements with the ISO Standards 14008: 2019 (Monetary valuation of environmental impacts) and ISO 14007:2019 (Determining Environmental Costs and Benefits), respectively.
The output from this assessment framework can be the basis for, and complemented by, an assessment of the circularity potential of the proposed solutions. For this, a relevant indicator can be deployed like the Material Circularity Indicator developed by the Ellen MacArthur Foundation (2015). The circularity potential can be further enriched by a cost-benefit analysis (CBA) performed on selected solutions to assess whether estimated social benefits outweigh estimated social costs.
Products from climate neutral farms and circular food systems will ultimately need to be supported by the market, with consumers choosing the products (Ortega et al., 2011). Food consumption and culture vary greatly between regions. Furthermore, nutritional properties and safety assessment of feed ingredients as well as food safety and quality of final food products need to be ensured. Hence, dedicated work on consumer awareness, food safety and affordability are also needed to address these concerns in addition to regulatory aspects, meeting consumer demand, income level etc. on food products developed (e.g. triploid mussels, SW, meat and milk from animals fed sustainable feeds). Will for instance European consumers accept, afford and demand chromosome manipulated (triploid) sterile mussels, farmed fish fed feeds including processed animal by products, when climate benefits are documented?
Consumers should be engaged as co-creators. Consumer information should be used to expand the scope of sustainability analysis. This will also help identify the limitations and uncertainties of current business models, while providing qualitative assessment of consumer acceptance and use of food products. This can support producers to grow their consumer segment with products from climate neutral farming. Such insights will also be useful in the further development of regulations on food safety.
5 Conclusion and implications
A systemic and cross sectorial approach can contribute to climate mitigation by transfer of atmospheric CO2 to the terrestrial biosphere using low trophic species, including plants, SW and mussels (i.e. C sequestration) and increasing organic C stocks in soils and vegetation biomass (i.e. C storage). Innovative mixes of technologies applied to LTS, marine and terrestrial animal by-products, new crops, diversified and integrated production systems can link the high primary productivity rates of the marine environment to the C storage capability of the terrestrial food sector. This can pave the way for linking and scaling up C-neutral marine and terrestrial food production systems into a future sustainable and circular bioeconomy.
Data availability statement
The raw data supporting the conclusions of this article will be made available by the authors, without undue reservation.
Author contributions
IO wrote the first version based on earlier cooperation among the authors. All authors contributed to the article and approved the submitted version.
Funding
AH was funded through the AquaVitae project under the European Union’s Research and Innovation Programme, Grant No. 818173. IO was funded through the European Union’s Horizon 2020 research and innovation program (ERA-NET BlueBio cofund Grant No. 817992) and through the SeaSoil project funded by The Research Council of Norway (Grant No. 339232). AG was funded by the Norwegian Fund for Research Fees for Agricultural Products (FFL) supporting the study through the project “FoodForFuture” (Grant No. 314318).
Conflict of interest
The authors declare that the research was conducted in the absence of any commercial or financial relationships that could be construed as a potential conflict of interest.
Publisher’s note
All claims expressed in this article are solely those of the authors and do not necessarily represent those of their affiliated organizations, or those of the publisher, the editors and the reviewers. Any product that may be evaluated in this article, or claim that may be made by its manufacturer, is not guaranteed or endorsed by the publisher.
References
Aanesen M., Czajkowski M., Lindhjem H., Navrud S. (2023). Trade-offs in the transition to a blue economy - Mapping social acceptance of aquaculture expansion in Norway. Sci. Total Environ. 859, 160199. doi: 10.1016/j.scitotenv.2022.160199
Almagro M., López J., Boix-Fayos C., Albaladejo J., Martínez-Mena M. (2010). Belowground carbon allocation patterns in a dry Mediterranean ecosystem: A comparison of two models. Soil Biol. Biochem. 42, 1549–1557. doi: 10.1016/j.soilbio.2010.05.031
Alonso A. A., Álvarez-Salgado X. A., Antelo L. T. (2021). Assessing the impact of bivalve aquaculture on the carbon circular economy. J. Cleaner Prod. 279, 123873. doi: 10.1016/j.jclepro.2020.123873
Bossio D. A., Cook-Patton S. C., Ellis P. W., Fargione J., Sanderman J., Smith P., et al. (2020). The role of soil carbon in natural climate solutions. Nat. Sustain. 3, 391–398. doi: 10.1038/s41893-020-0491-z
Broch O.J., Omholt A.M., Bekkby T., Gundersen H., Forbord S., Handå A., et al. (2019). The Kelp cultivation potential in coastal and offshore regions of Norway. Front. Mar. Sci. 5 (529). doi: 10.3389/fmars.2018.00529
Brooker R. W., George T. S., Homulle Z., Karley A. J., Newton A. C., Pakeman R. J., et al. (2021). Facilitation and biodiversityecosystem function relationship in crop production systems and their role in sustainable farming. J. Ecol. 109, 2054–2020. doi: 10.3929/ethz-b-000485518
Bush S. R., Pauwelussen A., Badia P., Kruk S., Little D., Newton R., et al. (2021). Implementing aquaculture technology and innovation platforms in Asia. Aquaculture 530, 735822. doi: 10.1016/j.aquaculture.2020.735822
Campos I., Valente L. M. P., Matos E., Marques P., Freire F. (2020). Life-cycle assessment of animal feed ingredients: Poultry fat, poultry by-product meal and hydrolyzed feather meal. J. Cleaner Prod. 252 (12), 119845. doi: 10.1016/j.jclepro.2019.119845
Chenu C., Angers D. A., Barré P., Derrien D., Arrouays D., Balesdent J. (2019). Increasing organic stocks in agricultural soils: Knowledge gaps and potential innovations. Soil Tillage Res. 188, 41–52. doi: 10.1016/j.still.2018.04.011
Ciroth A., Huppes G., Klopffer W., Rudenauer I., Steen B., Swarr T. (2008). Environmental Life Cycle Costing. Eds. Hunkeler D., Lichtenvort K., Rebitzer G. (Boca Raton, FL, USA: CRC Press).
Colombo S. M., Roy K., Mraz J., Wan A. H. L., Davies S. J., Tibbetts S. M., et al. (2022). Towards achieving circularity and sustainability in feeds for farmed blue foods. Rev. Aquacult. 1-, 27. doi: 10.1111/raq.12766
De Menna F., Dietershagen J., Loubiere M., Vittuari M. (2018). Life cycle costing of food waste: A review of methodological approaches. Waste Manage. 73, 1–13. doi: 10.1016/j.wasman.2017.12.032
Duguma L. A., Nzyoka J., Minang P.A., Bernard F. (2017). How agroforestry propels achievement of nationally determined contributions. ICRAF Policy Brief no. 34. World Agroforestry Centre, Nairobi, Kenya. 8 pp. Available at: https://apps.worldagroforestry.org/downloads/Publications/PDFS/PB17360.pdf.
EC (2019). The European Green Deal Communication from the Commission to the European Parliament, the European Council, the Council, the European Economic and Social Committee and the Committee of the Regions (Brussels: EC COM). https://eur-lex.europa.eu/legal-content/EN/TXT/PDF/?uri=CELEX:52019DC0640
EC (2020). A Farm to Fork Strategy for a fair, healthy and environmentally-friendly food system (Brussels: Communication from the Commission to the European Parliament, in EC COM. 2020).
EEA (2019) Annual European Union greenhouse gas inventory 1990-2017 and inventory report 2019. 2019. (Copenhagen: UNFCCC Secretariat).
EEA (2020) EEA/FOEN report, (2020). Is Europe living within the limits of our planet? Available at: https://www.eea.europa.eu/publications/is-europe-living-within-the-planets-limits (Accessed 30th June 2023).
EEA (2022) Annual European Union greenhouse gas inventory 1990-2020 and inventory report 2022 (Copenhagen: UNFCCC Secretariat). Available at: https://www.eea.europa.eu//publications/annual-european-union-greenhouse-gas-1 (Accessed 30th June 2023).
Ellen MacArthur Foundation (2015) Circularity Indicators - An approach to measuring circularity. Methodology (Circularity-Indicators-Methodology | Shared by Business (thirdlight.com) (Accessed 30th June 2023).
Ellis J., Tiller R. (2019). Conceptualizing future scenarios of integrated multi-trophic aquaculture (IMTA) in the Norwegian salmon industry. J. Mar. Policy. 201, 198–209. doi: 10.1016/j.marpol.2019.02.049
FAO (2018). The state of World Fisheries and Aquaculture - Meeting the sustainable development goals (Rome, Italy: FAO). Licence: CC BY-NC-SA 3.0 IGO. Available at: https://www.fao.org/3/i9540en/i9540en.pdf (Accessed August 8, 2023).
FAO (2022) The state of World Fisheries and Aquaculture 2022 (Aquaculture production (fao.org) (Accessed 30th June 2023).
Fodrie F. J., Rodriguez A. B., Gittman R. K., Grabowski J. H., Lindquist N. L., Peterson C. H., et al. (2017). Oyster reefs as carbon sources and sinks. Proc. R. Soc. B. 284, 20170891. doi: 10.1098/rspb.2017.0891
Fry J. (2012). Carbon footprint of scottish suspended mussels and intertidal oysters. Scottish Aquaculture Research Forum (SARF). (Scotland, UK: Scottish Aquaculture Research Forum (SARF)). 55 pp. Available at: https://8ab8cd86-3be5-48c3-ae48-2ffaa6c11045.filesusr.com/ugd/1618a8_37899cc5fe7c4e5cab84995a7d6c1980.pdf?index=true (Accessed August 8, 2023).
Fuss S., Lamb W. F., Callaghan M. W., Hilaire J., Felix Creutzig F., Amann T., et al. (2018). Negative emissions—Part 2: Costs, potentials and side effects. Environ. Res. Lett. 13 (6), 063002. doi: 10.1088/1748-9326/aabf9f
Gonera A., Pabst R. (2019). The use of design thinking in transdisciplinary research and innovation consortia: Challenges, enablers and benefits. J. Innovation Manage. 7 (3), 96–122. doi: 10.24840/2183-0606_007.003_0006
Graamans L., Baeza E., van den Dobbelsteen A., Tsafaras I., Stanghellini C. (2018). Plant factories versus greenhouses: comparison of resource use efficiency. Agric. Syst. 160, 31–43. doi: 10.1016/j.agsy.2017.11.003
Hoegh-Guldberg O., Caldeira K., Chopin T., Gaines S., Haugan P., Hemer M., et al. (2019) The Ocean as a Solution to Climate Change: Five Opportunities for Action (Washington DC: World Resources Institute). Available at: http://www.oceanpanel.org/climate (Accessed February 22, 2023).
IPCC (2019). Climate Change and Land: an IPCC special report on climate change, desertification, land degradation, sustainable land management, food security, and greenhouse gas fluxes in terrestrial ecosystems. Eds. Shukla P. R., Skea J., Buendia E.C., Masson-Delmotte V., Pörtner H.-O., Roberts D. C., Zhai P., Slade R., Connors S., Diemen R.v., Ferrat M., Haughey E., Luz S., Neogi S., Pathak M., Petzold J., Pereira J.P., Vyas P., Huntley E., Kissick K., Belkacemi M., Malley J. (Cambridge, UK and New York, NY, USA: Cambridge University Press). 896 pp. doi: 10.1017/9781009157988
Joffre O. M., Klerkx L., Dickson M., Verdegem M. (2017). How is innovation in aquaculture conceptualized and managed? A systematic literature review and reflection framework to inform analysis and action. Aquaculture 470, 129–148. doi: 10.1016/j.aquaculture.2016.12.020
Kinley R. D. (2020). Mitigating the carbon footprint and improving productivity of ruminant livestock agriculture using a red seaweed. J. clean. prod. 259, 2020 v.259. doi: 10.1016/j.jclepro.2020.120836
Leeuwis C., Boogaard B. K., Atta-Krah K. (2021). How food systems change (or not): governance implications for system transformation processes. Food Secur. 13, 761–780. doi: 10.1007/s12571-021-01178-4
Ma Z., Chen H. Y., Bork E. W., Carlyle C. N., Chang S. X. (2020). Carbon accumulation in agroforestry systems is affected by tree species diversity, age and regional climate: A global meta-analysis. Global Ecol. Biogeogr. 29, 1817–1828. doi: 10.1111/geb.13145
MacLeod M. J., Hasan M. R., Robb D. H. F., Mamun-Ur-Rashid M. (2020). Quantifying greenhouse gas emissions from global aquaculture. Sci. Rep. 10 (1), 11679. doi: 10.1038/s41598-020-68231-8
Maia M. R. G., Fonseca A. J. M., Oliveira H. M., Mendonça C., Cabrita A. R. J. (2016). The potential role of seaweeds in the natural manipulation of rumen fermentation and methane production. Sci. Rep. 6 (1), 32321. doi: 10.1038/srep32321
Morais T., Inácio A., Coutinho T., Ministro M., Cotas J., Pereira L., et al. (2020). Seaweed potential in the animal feed: A review. J. Mar. Sci. Eng. 8, 559. doi: 10.3390/jmse8080559
Morugán-Coronado A., Linares C., Gómez-López M. D., Faz Á., Zornoza R. (2020). The impact of intercropping, tillage and fertilizer type on soil and crop yield in fruit orchards under Mediterranean conditions: A meta-analysis of field studies. Agric. Syst. 178, 102736. doi: 10.1016/j.agsy.2019.102736
Newton R. W., Maiolo S., Malcorps W., Little D. C. (2023). Life Cycle Inventories of marine ingredients. Aquaculture 565, 739096. doi: 10.1016/j.aquaculture.2022.739096
Orsini F., Pennisi G., Zulfiqar F., Gianquinto G. (2020). Sustainable use of resources in plant factories with artificial lighting (PFALs). Eur. J. Hortic. Sci. 85, 297–309. doi: 10.17660/eJHS.2020/85.5.1
Ortega D. L., Wang H. H., Wu L., Olynk N. J. (2011). Modeling heterogeneity in consumer preferences for select food safety attributes in China. Food Policy. 36, 318–324. doi: 10.1016/j.foodpol.2010.11.030
Palangi V., Taghizadeh A., Abachi S., Lackner M. (2022). Strategies to mitigate enteric methane emissions in ruminants: A review. Sustain. (Switzerland) 14, 13229. doi: 10.3390/su142013229
Poore J., Nemecek T. (2018). Reducing food’s environmental impacts through producers and consumers. Science 360, 987–992. doi: 10.1126/science.aaq0216
Roque B. M., Salwen J. K., Kinley R., Kebreab E. (2019). Inclusion of Asparagopsis armata in lactating dairy cows’ diet reduces enteric methane emission by over 50 percent. J. Cleaner Prod. 234, 132–138. doi: 10.1016/j.jclepro.2019.06.193
Sandström V., Chrysafi A., Lamminen M., Troell M., Jalava M., Piipponen J., et al (2022). Food system by-products upcycled in livestock and aquaculture feeds can increase global food supply? Nat Food. 3, 729–740. doi: 10.1038/s43016-022-00589-6
High Level Group of Scientific Advisors (2017). Scientific Opinion EC, Brussels 3, 71. doi: 10.2777/66235
Smith P. (2012). Soils and climate change. Curr. Opin. Environ. Sustain. 4 (5), 539–544. doi: 10.1016/j.cosust.2012.06.005
Smith P. (2016). Soil carbon sequestration and biochar as negative emission technologies. Global Change Biol. 22 (3), 1315–1324. doi: 10.1111/gcb.13178
Spillias S., Valin H., Batka M., Sperling F., Havlík P., Leclère D., et al. (2023). Reducing global land-use pressures with seaweed farming. Nat. Sustain 6, 380–390. doi: 10.1038/s41893-022-01043-y
Stetkiewicz S., Norman R. A., Allison E. H., Andrew N. L., Ara G., Banner-Stevens G., et al. (2022). Seafood in food security: A call for bridging the terrestrial-aquatic divide. Front. Sustain. Food Syst. 5. doi: 10.3389/fsufs.2021.703152
Thomas J.-B. E., Sinha R., Strand Å., Söderqvist T., Stadmark J., Franzén F., et al. (2022). Marine biomass for a circular blue-green bioeconomy? A life cycle perspective on closing nitrogen and phosphorus land-marine loops. J. Ind. Ecol. 26, 2136–2153. doi: 10.1111/jiec.13177
Tiefenbacher A., Sandén T., Haslmayr H. P., Miloczki J., Wenzel W., Spiegel H. (2021). Optimizing carbon sequestration in croplands: a synthesis. Agronomy 11, 882. doi: 10.3390/agronomy11050882
van Delden S. H., SharathKumar M., Butturini M., Graamans L. J. A., Heuvelink E., Kacira M., et al. (2021). Current status and future challenges in implementing and upscaling vertical farming systems. Nat. Food 2, 944–956. doi: 10.1038/s43016-021-00402-w
Van Gastelen S., Dijkstra J., Bannink A. (2019). Are dietary strategies to mitigate enteric methane emission equally effective across dairy cattle, beef cattle, and sheep? J. Dairy Sci. 102, 6109–6130. doi: 10.3168/jds.2018-15785
Winther J. G., Dai M., Rist T., Hoel A. H., Li Y., Trice A., et al. (2020). Integrated ocean management for a sustainable ocean economy. Nat. Ecol. Evol. 4 (11), 1451–1458. doi: 10.1038/s41559-020-1259-6
Keywords: blue foods, aquaculture, crop diversification, low trophic organisms, circular feeds, systemic innovations, carbon sequestration, carbon storage
Citation: Olesen I, Bonaldo A, Farina R, Gonera A, Hughes AD, Navrud S, Orsini F, Parma L and Zornoza R (2023) Moving beyond agriculture and aquaculture to integrated sustainable food systems as part of a circular bioeconomy. Front. Mar. Sci. 10:1178014. doi: 10.3389/fmars.2023.1178014
Received: 02 March 2023; Accepted: 31 July 2023;
Published: 17 August 2023.
Edited by:
Lynne Falconer, University of Stirling, United KingdomReviewed by:
Richard Newton, University of Stirling, United KingdomCopyright © 2023 Olesen, Bonaldo, Farina, Gonera, Hughes, Navrud, Orsini, Parma and Zornoza. This is an open-access article distributed under the terms of the Creative Commons Attribution License (CC BY). The use, distribution or reproduction in other forums is permitted, provided the original author(s) and the copyright owner(s) are credited and that the original publication in this journal is cited, in accordance with accepted academic practice. No use, distribution or reproduction is permitted which does not comply with these terms.
*Correspondence: Ingrid Olesen, aW5ncmlkLm9sZXNlbkBub2ZpbWEubm8=