- 1Key Laboratory of Tropical Hydrobiology and Biotechnology of Hainan Province, College of Marine Sciences, Hainan University, Haikou, China
- 2State Key Laboratory of Marine Resource Utilization in South China Sea, Hainan University, Haikou, China
- 3College of Animal Science and Technology, Hainan University, Haikou, China
- 4Dezhou Key Laboratory for Applied Bile Acid Research, Shandong Longchang Animal Health Product Co., Ltd., Dezhou, China
This study aimed to evaluate the effects of dietary bile acids on growth performance, feed utilization, body composition, digestive enzyme activity, and related gene expression in juvenile leopard coral grouper (Plectropomus leopardus). Five diets with varying levels of dietary bile acids (0, 0.15, 0.3, 0.45 and 0.6%) were formulated and each diet was fed to triplicate groups of 15 fish (13.14 ± 0.14 g) for ten weeks. Results showed that the significant enhancement in weight gain (WG) and specific growth rate (SGR) was observed in fish fed 0.3% bile acids (P < 0.05). Additionally, feed conversion ratio (FCR) was significantly improved with bile acids inclusion (P < 0.05). Higher feed intake was also recorded in bile acids inclusion groups, and significantly up-regulated gene expression of hypothalamus neuropeptide Y (NPY) was found in 0.3% group compared with the control group (P < 0.05). Dietary bile acids (0.3%) significantly decreased lipid deposition in the whole-body (P < 0.05), and lower hepatosomatic index (HSI) and viscerosomatic index (VSI) were also found in this group. Moreover, intestinal trypsin and lipase activities were significantly increased in 0.3% group (P < 0.05) to promote feed digestion. In addition, the relative expression levels of intestinal fatty acid binding protein 2 (FABP2) significantly up-regulated with inclusion of dietary bile acids, suggesting an enhancement of fatty acid transport (P < 0.05). In contrast, cholesterol transport related genes NPC1 like intracellular cholesterol transporter 1 (NPC1L1) and CD36 were notably down-regulated as bile acids inclusion (P < 0.05). This study provides valuable information for the formulation of diets for juvenile coral trout and indicates that dietary bile acids could potentially be used to improve growth performance and feed utilization.
Introduction
Bile acids are synthesized from cholesterol in the liver, then conjugated to taurine or glycine to increase their solubility and stored in the gallbladder. Upon feed intake, bile acids are released into the intestine by stimulating the hormone cholecystokinin (CCK) (Di Ciaula et al., 2018). With amphipathic properties, they act as an emulsifier to facilitate the digestion and absorption of dietary lipids and fat-soluble nutrients through micellar dispersion (Macierzanka et al., 2019). Additionally, bile acids can improve digestion and assimilation of dietary proteins by accelerating the hydrolysis ability of pancreatic proteases (Gass et al., 2007). In recent decades, researches have revealed that bile acids are vital signaling molecules involved in modulating cell proliferation, gene expression, and nutrient metabolism through their receptors in different tissues, including farnesoid X receptor (FXR), G-protein-coupled bile acid receptor-1 (TGR5), and the liver X receptors α and β (LXR) (Lefebvre et al., 2009; Chiang, 2013; Shulpekova et al., 2022). Recent studies have also suggested that bile acids may be related to appetite regulation, mainly through the secretion of gastrointestinal hormones (Kuhre et al., 2018).
Based on the multiple physiological functions of bile acids in animals, bile acids have been authorized as a new feed additive in China, garnering increasing attention in the aquaculture industry since 2014 (Yao et al., 2021). The popularity of high-fat diet to improve protein utilization and aquaculture efficiency has been on the rise in recent years; however, long-term feeding of such diets can lead to adverse effects such as abnormal fat deposition and health issues, which can ultimately slow down the growth performance of fish (Tan et al., 2019; Li et al., 2022). Studies conducted on typical carnivorous species such as largemouth bass (Micropterus salmoides L.) (Yin et al., 2021) and large yellow croaker (Pseudosciaena crocea) (Ding et al., 2020) have demonstrated that suitable dietary bile acids can attenuate the negative effects of high-fat diets by improving lipid digestion and absorption, antioxidant capacity, and intestinal health status. Additionally, the increasing use of plant-based ingredients in fish diets has been shown to alter bile acid status in fish, resulting in diminished nutrient digestion and absorption (Romano et al., 2020). Dietary bile acid supplementation has been reported to attenuate the impairment of growth performance and digestive function of fish caused by low-fish meal diets (Yamamoto et al., 2007; Iwashita et al., 2009; Ni et al., 2021). Lastly, studies in grass carp (Ctenopharyngodon idella) have revealed that bile acids can increase intestinal microbiota diversity, induce community succession, and improve immune function (Xiong et al., 2018; Peng et al., 2019).
The leopard coral grouper (Plectropomus leopardus), also known as coral trout, is a highly economically valuable marine fish species due to its desirable flesh taste and attractive colorful skin (Zhou et al., 2020). In recent years, the success of artificial breeding has enabled the rapid development of coral trout aquaculture along the southern coast of China in tropical and subtropical climates. The availability of highly qualified aquafeed is essential to support this growth, yet nutrition and feed studies in this fish species are still quite limited. It has only been reported that juvenile coral trout require a dietary crude protein content of 50% for optimum growth (Xia et al., 2020). Compared with other grouper species, coral trout have a slower feeding process and relatively lower feed utilization (Giri et al., 2021). With this collective background, the present study aims to evaluate the effects of dietary bile acids on growth and feed utilization of coral trout. Additionally, we also further investigated the transcriptional regulation of feeding-related neuropeptides and lipids transporters after bile acids supplementation.
Materials and methods
The animal experiment protocol was reviewed and approved by the Committee of Animal Welfare and Ethics of Hainan University (HNUAUCC-2021-00085).
Experimental diets
Five isonitrogenous (50%) and isolipidic (10%) experimental diets were formulated containing graded bile acids (0%, 0.15%, 0.3%, 0.45%, 0.6%) (Table 1). Bile acids were extracted from porcine bile (Shandong Longchang Animal Health Product Co., Ltd., Jinan, China; Purity 99%; containing 68% hyodeoxycholic acid, 17% chenodeoxycholic acid and 9% hyocholic acid). All ingredients were well ground, weighed, and mixed in a Hobart mixer (A-200T Mixer Bench Model) for 30 minutes. Fish oil and soybean oil were gradually added and mixed constantly. Subsequently, 30-50 mL of water per 100 g of dry matter were slowly blended into the premixed ingredients. The diets were produced to a noodle-like shape at 3 mm diameter in a twin-screw extruder (Institute of Chemical Engineering, South China University of Technology, Guangzhou) and were pelletized. All diets were air dried at 20°C and were stored at -20°C until use.
Fish and feeding trial
Juvenile coral trout were obtained from a local commercial hatchery (Wenchang, Hainan province, China). Fish were acclimated with commercial diets for 2 weeks prior to the experiment. 225 health fish with similar size (average body weight, 13.14 ± 0.14 g) were randomly distributed into 15 floating cages (L 60 cm×W 50 cm×H 50 cm) placed in an indoor cement tank (4×3×1.5 m3). The fish were fed to apparent satiation twice daily (8:00 am, 4:30 pm) over the 10-week trial. Water temperature (31 ± 0.5°C), total ammonia (0-0.10 mg/L) and dissolved oxygen (5.9 ± 0.2 mg/L) were monitored daily, and the fish were exposed to a 12 h: 12 h light: dark cycle.
Samples collection
After the experiment, all fish in each cage were counted and weighed after being subjected to a 24-h fasting period. Seven fish per cage were randomly collected and anesthetized with tricaine methanesulfonate (MS-222, 50 mg/L, Sigma, St. Louis, Missouri). Two of the fish were used to analyze whole-body composition. At the same time, three were individually weighed, measured, and then dissected to obtain viscera and liver for calculated body condition indices, including condition factor (CF) and hepatosomatic index (HSI). Muscle samples were collected from these three fish for chemical composition analysis. The remaining two fish had their liver samples kept in liquid nitrogen. Afterwards they were stored at -80°C for enzyme activity analysis. The mid-intestine samples from these two fish were collected for RNA extraction.
Biochemical analyses
The proximate composition of the fish whole-body, white muscle and experimental diets were analyzed according to the standard methods. Crude protein was determined by the Kjeldahl method after acid digestion using a Kjeltec 1030 Auto Analyzer system (FOSS Tecator, Sweden). Dry matter was determined by heating samples at 105°C. Crude lipid was quantified by ether extraction using a Soxtec System HT6 (Haineng SOX406, Shandong, China). Ash content was determined by heating 2 g samples at 550°C for 3 h.
Digestive enzyme activity assays
Mid-intestine samples were rinsed and homogenized in the commercial buffer (1:9 w/v) on ice. The supernatant of the enzyme extract was gently pipetted after the homogenate was centrifuged. Subsequently, the total protein content, trypsin and lipase activities were determined according to the methods of the commercial kits. Lipase kit was purchased from Shanghai Yifei Biotechnology Co. Ltd. (Shanghai, China). Trypsin and protein content kits were purchased from Nanjing Jiancheng Bioengineering Institute (Nanjing, China).
RNA extraction, reverse transcription, and real-time quantitative PCR analysis
Total RNA was extracted from the mid-intestine using Trizol Reagent (Invitrogen, Waltham, Massachusetts) followed by quality measurement on a 1.0% denaturing agarose gel and yield determination on a NanoDrop ND-1000 spectrophotometer (NanoDrop, Wilmington, Delaware). Subsequently, RNA was treated with RNA-free DNase (Takara, Japan) to remove genome DNA, and reversely transcribed to complementary DNA (cDNA) using the Revert Aid First Strand cDNA Synthesis Kit (Takara) as per the manufacturer’s instructions. The cDNA templates were stored at -20°C for further analysis. Primers were designed according to the related sequences on NCBI and verified before performing the quantitative PCR (Table 2). Real-time PCR was conducted using a quantitative thermal cycler (Roche Light Cycler 480, Switzerland) in a total volume of 20 μL containing 10 μL of Power SYBR Green PCR Master Mix (Takara), 0.8 μL of each primer (10 μmol), 6.4 μL of nuclease-free water, and 2 μL of cDNA mix. The real-time PCR program was as follows: 95°C for 30 s, followed by 40 cycles of 95°C for 5 s, 60°C for 20 s, and 65°C for 15 s for melting curve analysis. β-actin was used as the housekeeping gene to normalize the results. Three replicates were performed for each tested gene, and the expression levels of the target genes were calculated using the 2-ΔΔCt method (Livak and Schmittgen, 2001).
Calculation formula and statistical analysis
The normality and homogeneity of the data were assessed using Hartley’s test. Subsequently, a one-way analysis of variance (ANOVA) and Tukey’s Multiple Range Test (SPSS 22.0 for Windows, Chicago IL American) were conducted to determine if significant differences occurred between different treatments. Statistical significance was set at P < 0.05. All data are presented as mean ± S.E.M.
Results
Growth performance and feed utilization
The results of growth performance and feed utilization of juvenile coral trout are shown in Table 3. FBW and WG were increased as dietary bile acids level increased from 0% to 0.3%, and after that, it slowly decreased when dietary bile acids level continued to rise from 0.45% to 0.6%. Significant difference in growth performance was only found between 0.3% group and the control group (P < 0.05). Significantly higher FCR was found in the control group compared with those bile acids inclusion groups (0.3%, 0.45% and 0.6%), and the lowest value was recorded in 0.3% group. No significant difference in survival was observed among all the experimental groups (P > 0.05).
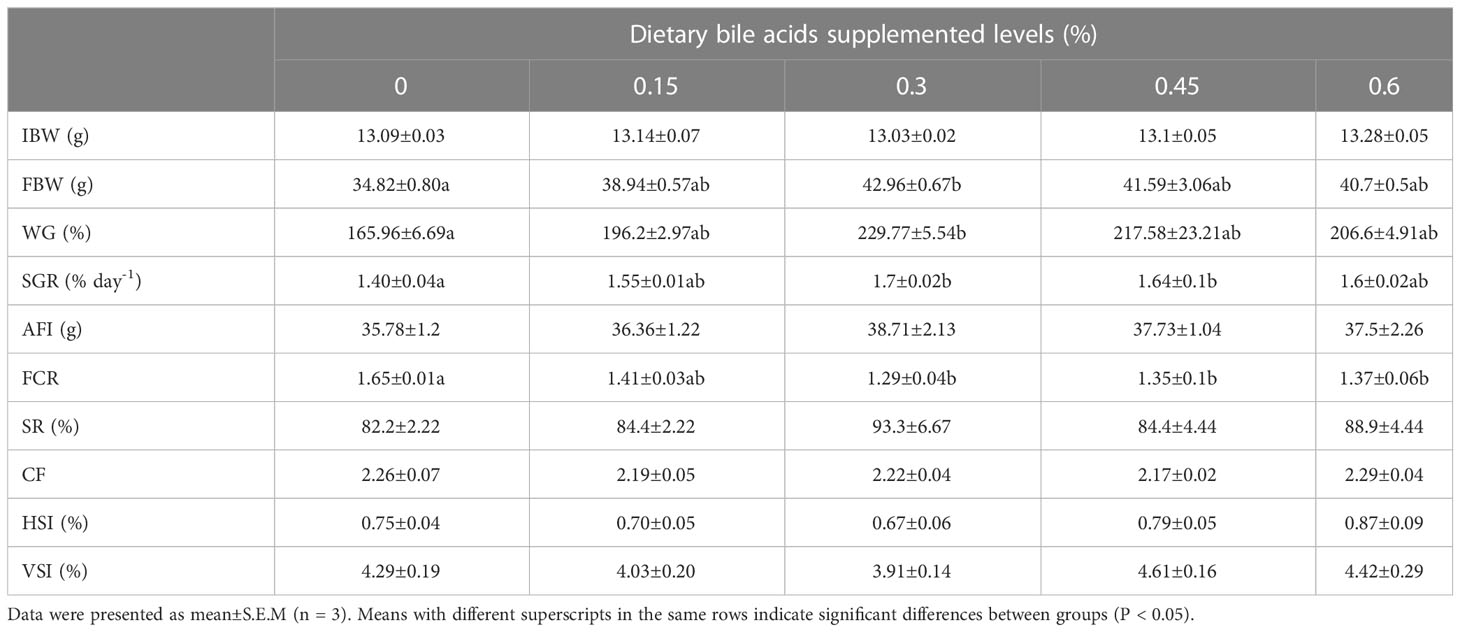
Table 3 Growth performance, feed utilization and morphological indexes of coral trout fed the experimental diets for 10 weeks.
Whole-body composition, muscle composition and morphological indexes
As shown in Table 4, there were no significant differences in whole body moisture, crude protein and muscle composition among the five groups (P > 0.05), while the highest whole body and muscle crude protein were present in bile acids 0.3% group. In addition, fish in 0.3% group had the lowest whole-body crude lipid and significant difference was found between 0.3% group and the control group (P < 0.05), while no remarkable differences were observed between control group and other bile acids supplements groups (0.15%, 0.45% and 0.6%). There were no obvious differences in morphological indexes among all the experimental treatments (P > 0.05), while HSI and VSI were lower in 0.3% group compared with other treatments (Table 3).
Intestinal digestive enzyme activities
Dietary bile acids levels significantly influenced intestinal trypsin and lipase activities (Figure 1). The significantly higher trypsin activity was found in 0.3%, 0.45% and 0.6% groups compared with the control groups (P < 0.05), while no significant difference was found between 0.15% and the control group (P > 0.05). As for lipase activity, significantly higher value was recorded in 0.3% group compared with other treatments (P < 0.05). Meanwhile, significant difference was also found between 0.45% group and control group (P < 0.05).
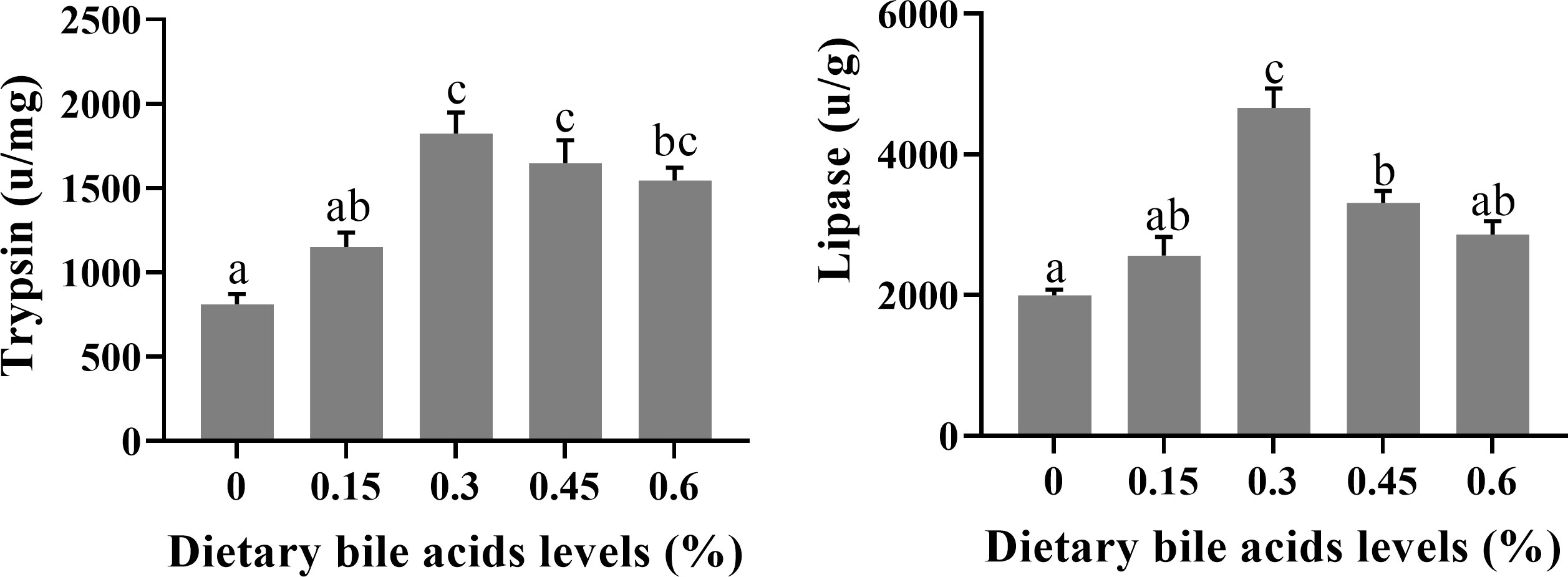
Figure 1 Effects of dietary bile acids levels on intestine digestive enzyme activities of coral trout. Values are expressed as mean ± S.E.M (n=6). Different letters indicate significant differences among groups (P < 0.05).
Expression of feed intake regulation-related genes in the hypothalamus
The gene expression of feed intake regulation related-peptide in the hypothalamus of coral trout is shown in Figure 2. NPY mRNA expression level was apparently higher in the 0.15 and 0.3% groups compared with the control group (P < 0.05), which was nearly 2 times than that in the control group. And there was no significant difference in NPY mRNA expression in the bile acids 0.45, 0.6, and control groups (P > 0.05). Ghrelin gene expression reached the highest level in the 0.3 group, while there was no significant difference among all the treatment groups (P > 0.05). The dietary fatty acid level did not significantly affect CCK and leptin mRNA expression (P > 0.05).
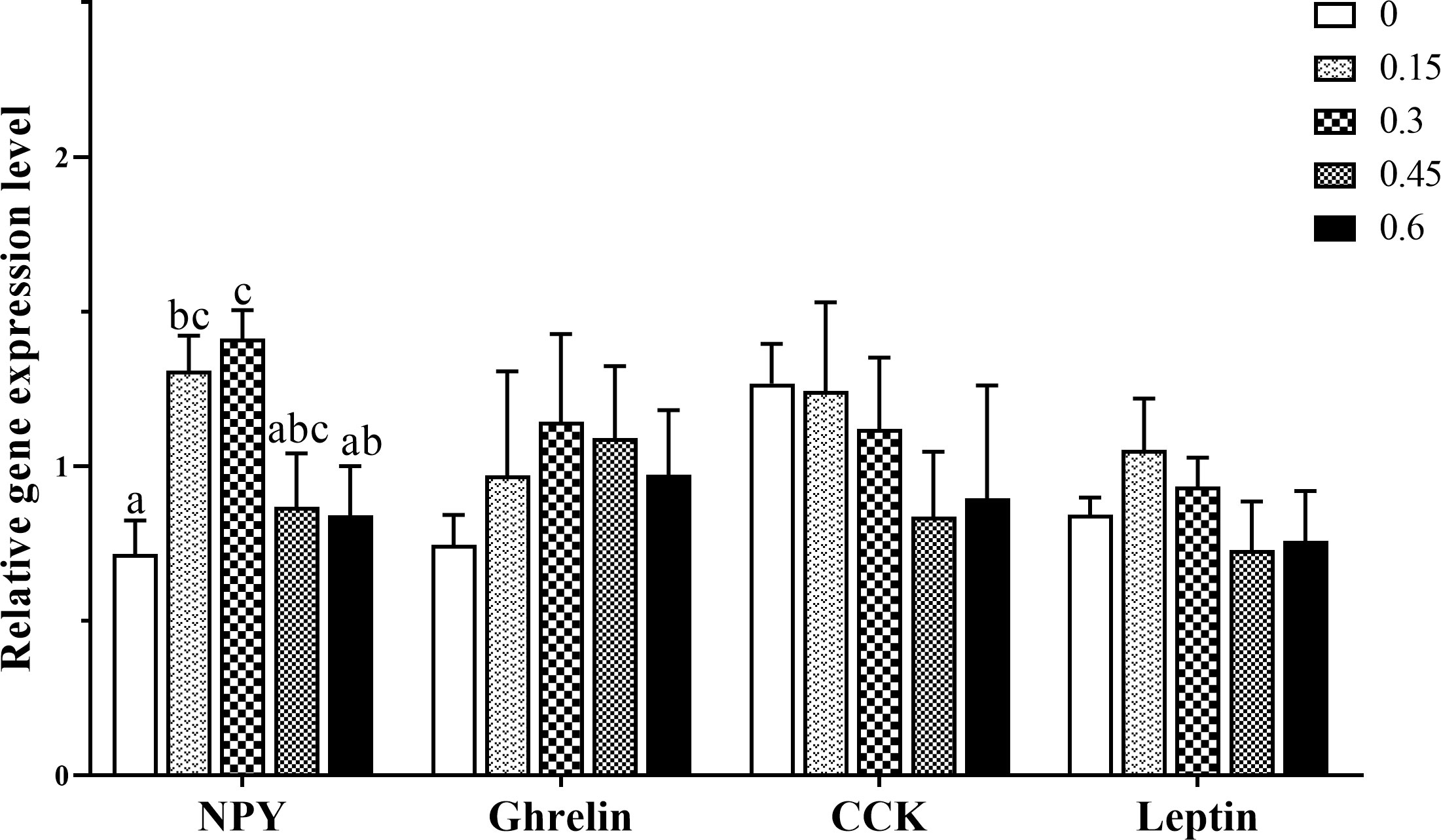
Figure 2 Effects of dietary bile acids levels on feeding regulation-related gene expression in the hypothalamus of coral trout. Values are expressed as mean ± S.E.M (n=6). Different letters indicate significant differences among groups (P < 0.05). NPY, neuropeptide Y CCK, cholecystokinin.
Expression of lipids transport-related genes in the intestine
The effects of dietary bile acids on mRNA expression of lipids transport-related genes are shown in Figure 3. Fatty acid transporter CD36 mRNA expression was down-regulated with the inclusion of dietary bile acids, and significant difference was found between the control group and 0.15, 0.45 and 0.6% groups (P < 0.05). Cholesterol transporter NPC1L1 was also found, resulting in an over 1.5-fold decrease in bile acids inclusion groups compared with the control group (P < 0.05). In contrast, significantly up-regulated gene expression of FABP2 was recorded in group 0.3% compared with the control group (P < 0.05). As for FATP4 mRNA expression, a remarkable decreased was found in 0.6% group (P < 0.05), and no significance difference was found among other treatments (P > 0.05). In addition, the inclusion of bile acids did not affect IAP gene expression (P > 0.05).
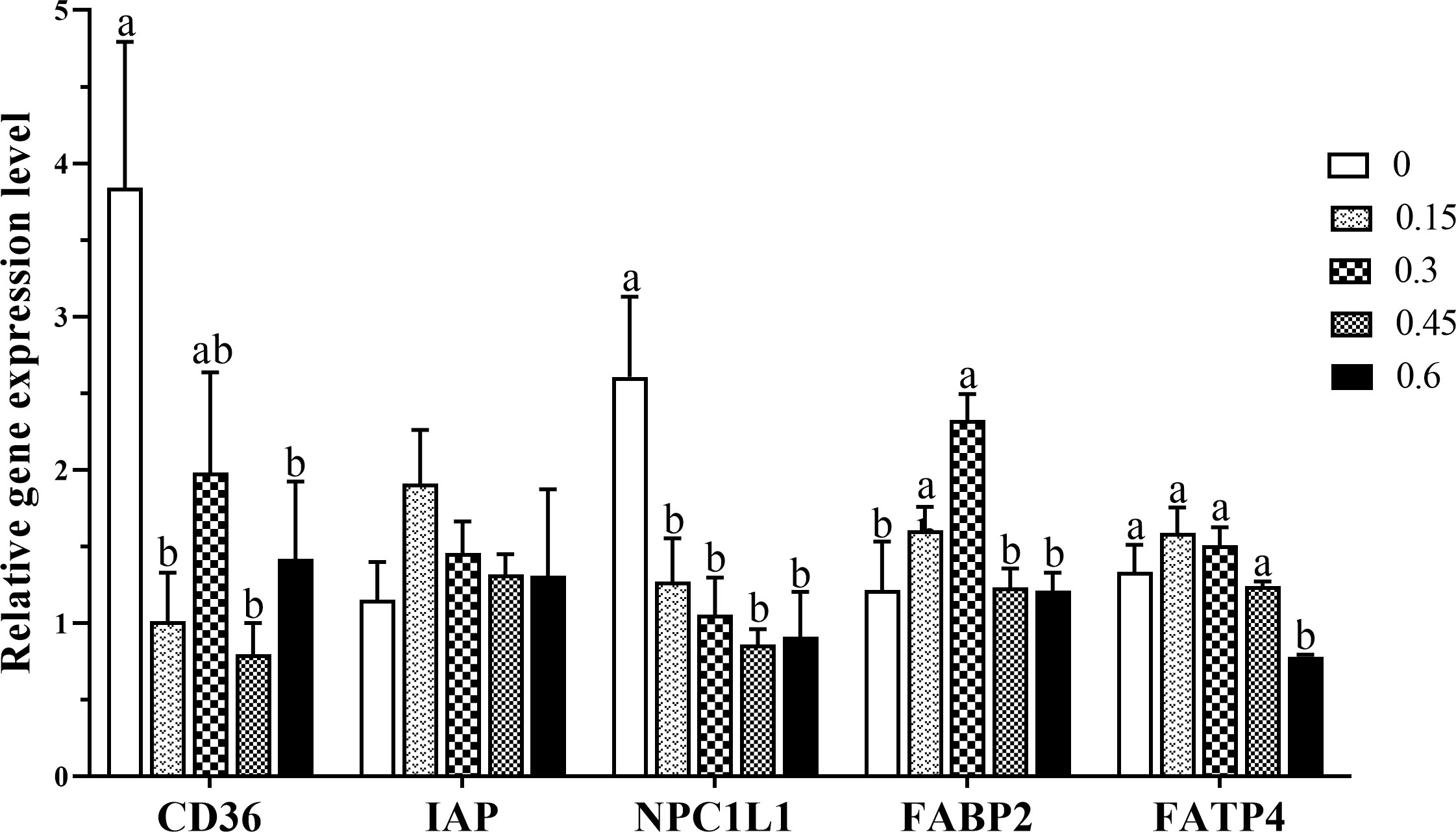
Figure 3 Gene expression levels of fatty acid transporters in the intestine of coral trout responded to the different dietary bile acids levels. Values are expressed as mean ± S.E.M (n=6). Different letters indicate significant differences among groups (P < 0.05). IAP, alkaline phosphatase; NPC1L1, NPC1 like intracellular cholesterol transporter 1; FABP2, fatty acid binding protein 2; FATP4, Fatty acid transport protein 4.
Expression of fatty acid biosynthesis-related genes and bile acids receptors in the intestine
Figure 4 presents the experimental data on the expression of ACACA and FASN genes affected by dietary bile acids levels. The transcripts expression of ACACA was significantly down-regulated when fish fed with moderate or higher levels (0.3%-0.6%) of bile acids (P < 0.05). In addition, the significantly lower gene expression of FASN was observed in higher bile acids inclusion groups compared with the control group (P < 0.05). Meanwhile, FASN mRNA expression in the 0.3% group was also lower than the control group, but no significant difference was found (P < 0.05).
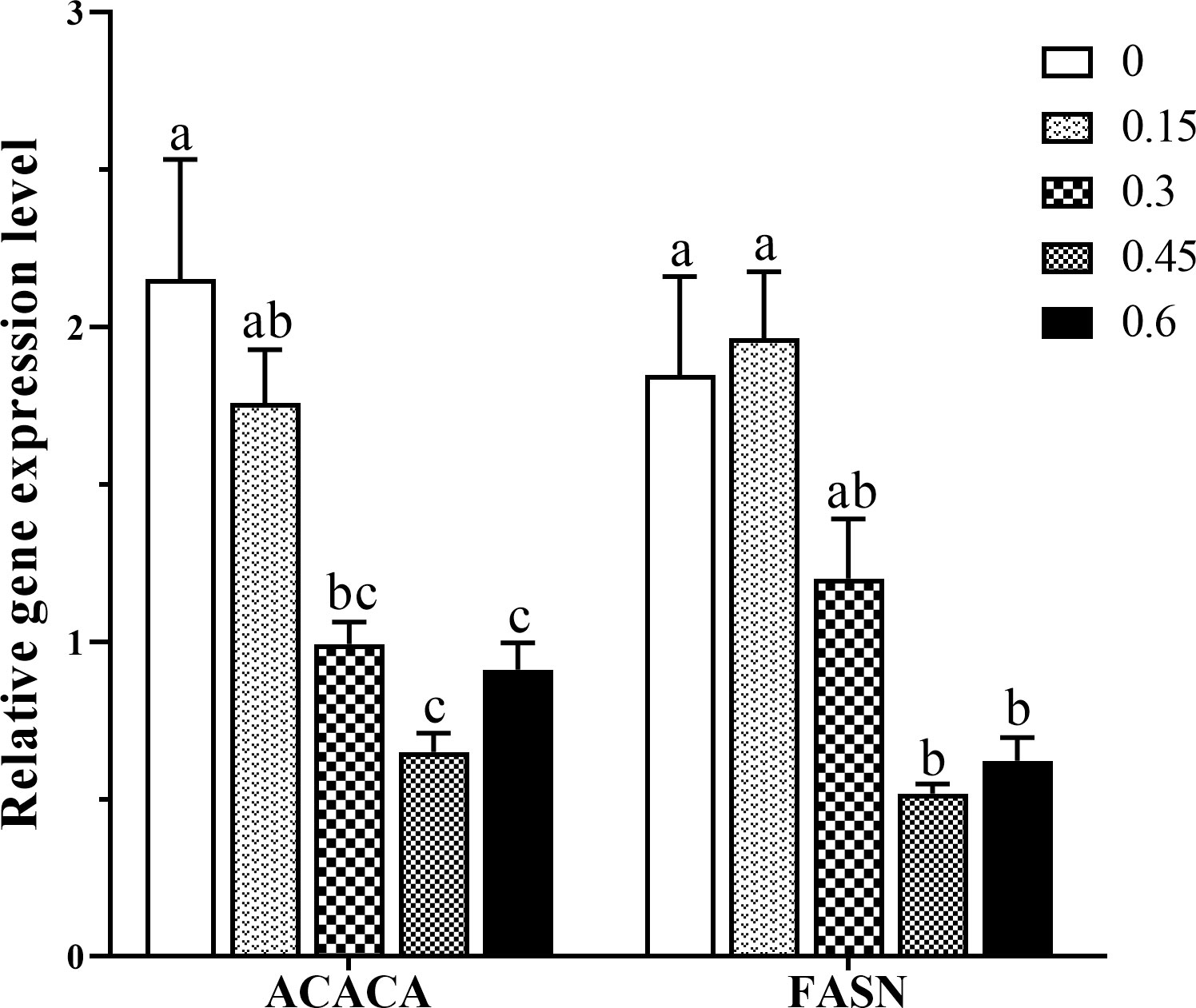
Figure 4 Effects of dietary bile acids levels on ACACA and FASN gene expression in the intestine of coral trout. Values are expressed as mean ± S.E.M (n=6). Different letters indicate significant differences among groups (P < 0.05). ACACA, Acetyl-coenzyme A carboxylase-α; FASN, fatty acid synthase.
As shown in Figure 5, bile acids inclusion profoundly affected the intestine gene expression of bile acids receptors. Generally, moderate bile acids inclusion (0.3%) produced a notable up-regulation of genes encoding receptors FXR and TGR5 (P < 0.05). As for LXR, gene expression was significantly up-regulated in 0.15% and 0.3% groups compared with the control group (P < 0.05).
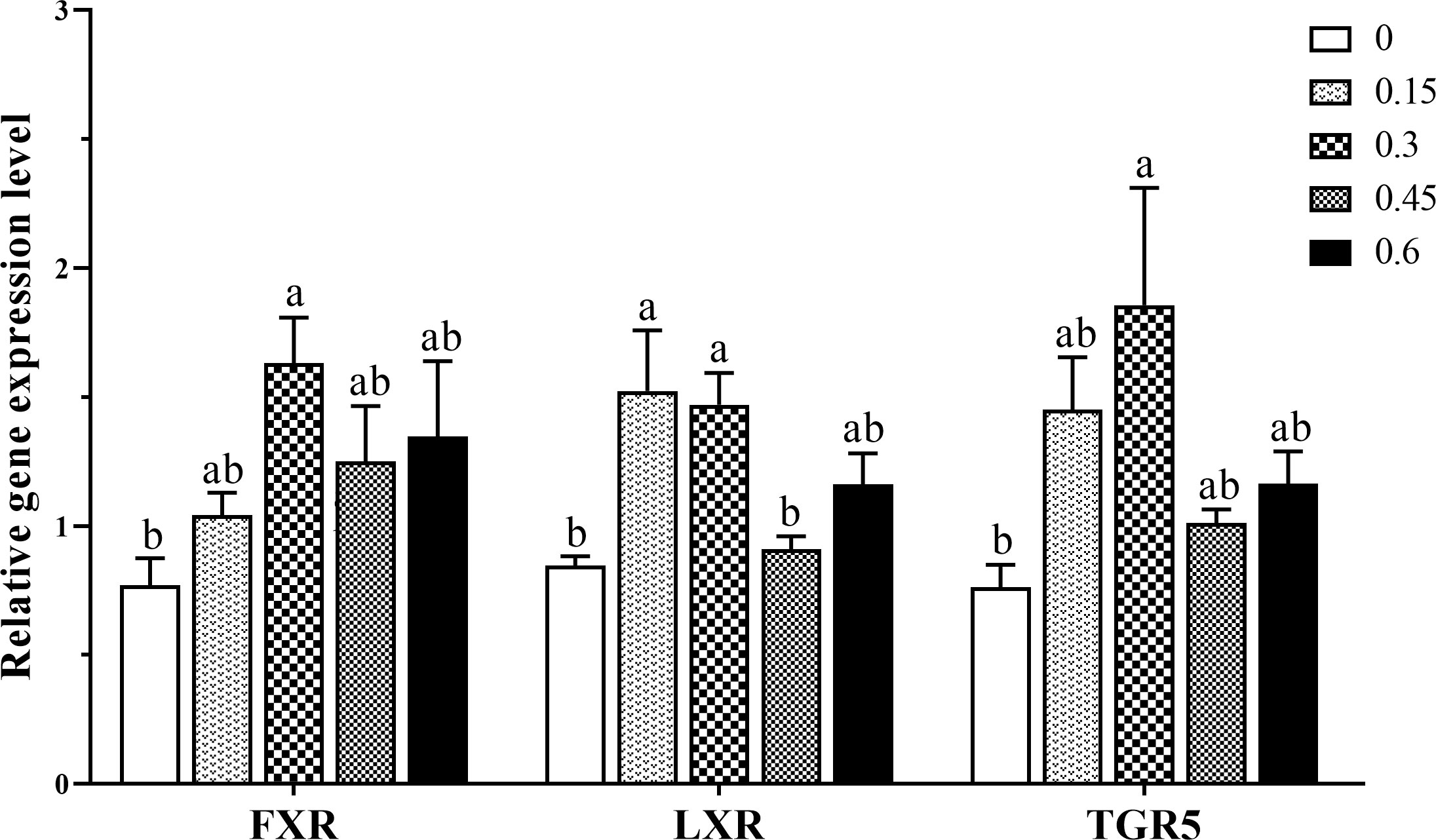
Figure 5 Gene expression levels of bile acids receptors in the intestine of coral trout responded to the different dietary bile acids levels. Values are expressed as mean ± S.E.M (n=6). Different letters indicate significant differences among groups (P < 0.05). FXR, Farnesoid X receptor; LXR, liver X receptor; TGR5, G-protein-coupled bile acid receptor-1.
Discussion
It has been reported that groupers have a low capability to utilize lipids as an energy source (Giri et al., 2021). Consequently, it is essential to formulate a diet that can improve feed efficiency and support the rapid growth of coral trout. Bile acids have been demonstrated to play a pivotal role in the digestion and absorption of dietary lipids in the intestine. Dietary supplements of bile acids have been reported to enhance growth performance in some carnivorous fish species, including Japanese flounder (Paralichthys olivaceus) (0.1%) (Alam et al., 2002), cobia (Rachycentron canadum) (Zhou et al., 2010), turbot (Scophthalmus maximus) (0.15%) (Sun et al., 2014) and hybrid grouper (Epinephelus fuscoguttatus♀ × E. lanceolatus♂) (0.09%) (Xu et al., 2022b). In the present study, WG was significantly improved when coral trout fed a diet containing 0.3% dietary bile acids compared with the control group (0%) after 10 weeks of feeding trail. A study by Yin et al. (2021) found that there were no significant differences in the WG of largemouth bass fed a high-fat diet with 0.03%, 0.06%, and 0.09% dietary bile acids. Similarly, dietary supplementation of lower levels of bile acids did not significantly affect the growth performance of black seabream (Sparus microcephalus) (0.02%) (Jin et al., 2019) and rainbow trout (Oncorhynchus mykiss) (Hang et al., 2022). Most of the studies including current study reported that fish fed with higher levels of dietary bile acids did not exhibit growth retardation. However, it has been observed that excessive dietary bile acids (1.8%) can negatively impact Atlantic salmon (Salmo salar L.) growth performance (Kortner et al., 2016). This suggests that the optimal and excessive dietary bile salt concentrations may be species-specific.
Feed intake is a major factor that influences the growth performance of fish by altering energy and metabolic balance. Measuring feed intake has been suggested as an important criterion for assessing the efficacy of different bile acids in aquafeeds (Romano et al., 2020). Our study showed that dietary bile acids increased feed intake compared with the control group. Similarly, a study conducted on yellowtail (Seriola quinqueradiata) revealed that increasing ursodeoxycholic acid (UDCA) concentration (0%, 0.4%, or 0.8%) resulted in a linear increase in feed intake (Deshimaru et al., 1982). To investigate the signaling pathway of orexigenic regulation of bile acids, we examined the expression of appetite-regulating peptides in the hypothalamus, a crucial area in the brain responsible for controlling feed intake. NPY has been identified as one of the brain’s most abundant neuropeptides, acting as an orexigenic factor (Loh et al., 2015). The present study showed that bile acids supplementation (0.15% and 0.3%) significantly improved hypothalamus NPY mRNA expression. Study has demonstrated that ghrelin is highly expressed in the stomach/gut of fish, with moderate levels detected in the brain, and that it is able to stimulate feed intake (Unniappan et al., 2005). However, the present study showed that dietary bile acids levels did not affect hypothalamus ghrelin mRNA expression. Additionally, this study found that the gene expression levels of CCK and leptin, which act as anorexigenic factors, were not significantly affected by dietary bile acids. These results may be attributed to the limited capability of bile acids to regulate fish feeding in this study, as although a higher feed intake was observed in bile acids supplement groups was observed, the difference was not significant. Previous research indicated that bile salts are highly effective facial taste stimuli, and that neural pathways exist to transmit bile salt information to the primary gustatory nucleus of the medulla (Rolen and Caprio, 2008). Further research is warranted to explore the regulation of bile acids on fish feeding in different species.
Efficient lipid digestion necessitates using bile acids as endogenous emulsifiers to mix lipids and related proteins in the proximal part of the digestive tract. Studies have suggested that bile acids may enhance fish growth performance by optimizing lipid digestion. Evidence has been presented that a deficiency in bile acids can decrease the efficiency of lipid digestion, thus harming growth performance (Deng et al., 2013; Kortner et al., 2013). The current study also showed that FCR was notably improved with the inclusion of 0.3-0.6% of dietary bile acids. In addition, bile acids not only increase the surface area of intraluminal lipids and promote adsorption of lipase, but also enhance lipase and proteolysis activities (Gass et al., 2007). Our study demonstrated that the inclusion of moderate bile acids (0.3%) significantly increased the intestinal lipase and trypsin activity of fish, which is in agreement with previous research conducted on snakehead (Channa argus) (Hou et al., 2019) and largemouth bass (Yin et al., 2021). The results of digestive enzyme assays in the present study were consistent with the enhanced growth performance of the fish. Consequently, as has been demonstrated to augment lipid digestibility in other studies, bile acids could be employed as a feed additive to enhance feed digestibility and utilization in coral trout.
The hydrolysis of dietary lipids within the lumen of the intestine following the uptake of hydrolyzed products by enterocytes is a vital process in the absorption of lipids. To date, several proteins have been identified in fish as being involved in this process of lipid absorption. However, the response of intestinal lipid transporters to dietary bile acids in fish remains largely understudied. Our observations found that NPC1L1, a major transporter involved in the regulation of intestinal cholesterol absorption, was significantly decreased in the groups supplemented with bile acids. A study using human enterocytes found that LXR and LXR+RXR agonists significantly decreased NPC1L1 mRNA expression (Alvaro et al., 2010). In the present study, gene expressions of LXR, FXR and TGR5 were up-regulated in the dietary bile acids 0.3% group. Similar result was also recorded in the liver of hybrid grouper that fish fed bile acids inclusion diets altered FXR and TGR5 genes expression, and lipid metabolism (Xu et al., 2022a). In addition, research has demonstrated that receptor LXR acts as an oxysterol sensor of intracellular cholesterol homeostasis, and mice treated with the LXR agonist have decreased fractional absorption of dietary cholesterol (Levy et al., 2007). The digestive system is largely responsible for maintenance of cholesterol balance in the body. The synthesis of bile salts from cholesterol necessitates an accounting of cholesterol balance, which requires consideration of both bile salts and cholesterol (Cohen, 2008). It was reported that bile acids could inhibit intestinal cholesterol absorption to prevent cholesterol gallstone formation in several mice models (Wang et al., 2003). Study in white shrimp (Litopenaeus vannamei) exhibited that whole-body, hepatopancreas and hemolymph cholesterol concentration were significantly decreased in soybean meal-based bile acids supplementation diet compared with fishmeal diet (Lin et al., 2022). Additionally, the present results also showed that intestinal CD36 mRNA expression was significantly decreased as inclusion of the bile acids. It has been reported that CD36 could involve in cholesterol homeostasis through dietary cholesterol absorption, cholesterol synthesis, lipoprotein formation, reverse cholesterol transport, and synthesis and reabsorption of bile acids (Ulug and Nergiz-Unal, 2021). In vitro investigation of mouse enterocytes has demonstrated that dephosphorylation of CD36 by the co-localized intestinal IAP augments fatty acid uptake (Lynes et al., 2011). However, no significant effect of dietary bile acids on the expression of fish intestine IAP genes was observed.
FABP2 and FATP4 are cytosolic long-chain fatty acids transporter, mainly expressed in cells of intestinal tissue (Kaitetzidou et al., 2015; Xu et al., 2017). In this study, the supplement of bile acids (0.3%) significantly increased fish intestine FABP2 gene expression. The binding of fatty acids to FABPs could reduce fatty acid-mediated intracellular toxicity. To mitigate the toxicity of free fatty acids, rapid conversion to acyl-CoA followed by triglyceride synthesis has been proposed. In addition, our study revealed that the genes FASN and ACACA, which encode rate-limiting enzymes involved in fatty acid biosynthesis, were significantly down-regulated in response to the inclusion of dietary bile acids, indicating that bile acids may facilitate the intestine’s uptake of fatty acids.
Several studies have demonstrated that dietary bile acids could reduce body lipid deposition in fish fed high-lipid diets. For instance, similar whole-body crude lipid was recorded between the control group (7%) and high-lipid (13%) with bile acids group in black seabream (Jin et al., 2019). Similarly, we also observed a significant reduction in whole-body crude lipid content in the 0.3% group compared with the control group. Contrary to the findings of a study on largemouth bass (Yin et al., 2021), which showed that muscle lipid was decreased in a high-lipid diet supplemented with suitable bile acids, we did not observe a significant decrease in muscle lipid content across all treatments. The carcass characteristics of fish are of commercial significance, as lower lipid deposition in the liver or viscera is indicative of a higher filet yield at similar body weight. Our study revealed that the 0.3% group had lower HSI and VSI values compared with the control group, while no significant difference was found between these treatments. Similarly, study in hybrid grouper also showed that VSI was not significantly affected in high-fat diets supplement with bile acids, but HSI was significant lower in optimum bile acids group (0.09%) compared with high-fat diet control group (Xu et al., 2022b). The above variations in results may be attributed to the species of fish, dietary lipid content and bile acids levels.
In conclusion, this study has demonstrated that supplement suitable level (0.3%) of dietary bile acids can improve the growth performance and feed efficiency of juvenile coral trout. Bile acids could also enhance lipid digestion and absorption by modulating intestinal digestive enzyme activity and lipids transporters gene expression. Furthermore, bile acids could reduce body lipid deposition in juvenile coral trout. Therefore, bile acids can be used as a feed additive to enhance feed digestibility and utilization in coral trout. The findings of this study provide valuable information for the formulation of diets for juvenile coral trout.
Data availability statement
The raw data supporting the conclusions of this article will be made available by the authors, without undue reservation.
Ethics statement
The animal study was reviewed and approved by Committee of Animal Welfare and Ethics of Hainan University.
Author contributions
YG: manuscript preparation. YY: fish management, experimental analysis, and data processing. JH, YS, QW, and DG: sampling and experimental analysis. SW: conceptualization and writing-review and editing. All authors have read and agreed to the published version of the manuscript. All authors contributed to the article.
Funding
This research was supported by the Key Research Project of Hainan Province (ZDYF2019099) and the National Natural Science Foundation of China (32260919).
Conflict of interest
Author SW is employed by Shandong Longchang Animal Health Product CO., Ltd.
The remaining authors declare that the research was conducted in the absence of any commercial or financial relationships that could be construed as a potential conflict of interest.’
Publisher’s note
All claims expressed in this article are solely those of the authors and do not necessarily represent those of their affiliated organizations, or those of the publisher, the editors and the reviewers. Any product that may be evaluated in this article, or claim that may be made by its manufacturer, is not guaranteed or endorsed by the publisher.
References
Alam M. S., Teshima S., Ishikawa M., Koshio S. (2002). Effects of ursodeoxycholic acid on growth and digestive enzyme activities of Japanese flounder paralichthys olivaceus (Temminck & schlegel). Aquac. Res. 32, 235–243. doi: 10.1046/j.1355-557x.2001.00020.x
Alvaro A., Rosales R., Masana L., Vallvé J. C. (2010). Polyunsaturated fatty acids down-regulate in vitro expression of the key intestinal cholesterol absorption protein NPC1L1: no effect of monounsaturated nor saturated fatty acids. J. Nutr. Biochem. 21 (6), 518–525. doi: 10.1016/j.jnutbio.2009.02.010
Chiang J. Y. (2013). Bile acid metabolism and signaling. Compr. Physiol. 3 (3), 1191. doi: 10.1002/cphy.c120023
Cohen D. E. (2008). Balancing cholesterol synthesis and absorption in the gastrointestinal tract. J. Clin. Lipidol. 2 (2), S1–S3. doi: 10.1016/j.jacl.2008.01.004
Deng J. M., Bi B. L., Kang B., Kong L. F., Wang Q. J., Zhang X. (2013). Improving the growth performance and cholesterol metabolism of rainbow trout (Oncorhynchus mykiss) fed soyabean meal-based diets using dietary cholesterol supplementation. Br. J. Nutr. 110 (1), 29–39. doi: 10.1017/S0007114512004680
Deshimaru O., Kuroki K., Yone Y. (1982). Suitable levels of lipids and ursodeoxycholic acid in diet for yellowtail. Bull. Japanese Soc. Sci. Fish. 48, 1265–1270. doi: 10.2331/suisan.48.1256
Di Ciaula A., Garruti G., Baccetto R. L., Molina-Molina E., Bonfrate L., Portincasa P., et al. (2018). Bile acid physiology. Ann. Hepatol. 16 (1), 4–14. doi: 10.5604/01.3001.0010.5493
Ding T., Xu N., Liu Y. T., Du J. L., Xiang X. J., Xu D., et al. (2020). Effect of dietary bile acid (BA) on the growth performance, body composition, antioxidant responses and expression of lipid metabolism-related genes of juvenile large yellow croaker (Larimichthys crocea) fed high-lipid diets. Aquaculture 518, 734768. doi: 10.1016/j.aquaculture.2019.734768
Gass J., Vora H., Hofmann A. F., Gray G. M., Khosla C. (2007). Enhancement of dietary protein digestion by conjugated bile acids. Gastroenterology 133 (1), 16–23. doi: 10.1053/j.gastro.2007.04.008
Giri N. A., Astuti N. W. W., Sudewi, Marzuqi M., Asih Y. N. (2021). Fish hydrolysate supplemented diet improved feed efficiency and growth of coral trout (Plectropomus leopardus). IOP. Conf. Ser.: Earth. Environ. Sci. 890, 12024. doi: 10.1088/1755-1315/890/1/012024
Hang Y., Fu Y., Jin C. X., Hua X. M. (2022). Effects of supplemental amino acids and bile acid in a completely replaced fish meal by enzymatically hydrolysed soybean meal diet on growth performance, liver health and fillet quality of rainbow trout (Oncorhynchus mykiss). Aquac. Res. 53 (9), 3297–3308. doi: 10.1111/are.15837
Hou Y. B., Hou Y., Yao L., Chen S., Fan J. H., Qian L. C. (2019). Effects of chromium yeast, tributyrin and bile acid on growth performance, digestion and metabolism of Channa argus. Aquac. Res. 50 (3), 836–846. doi: 10.1111/are.13954
Iwashita Y., Suzuki N., Matsunari H., Sugita T., Yamamoto T. (2009). Influence of soya saponin, soya lectin and cholytuarine supplemented to a casein-based semipurified diet on intestinal morphology and biliary bile status in fingerling rainbow trout Oncorhynchus mykiss. Fish. Sci. 75, 1307–1315. doi: 10.1007/s12562-009-0158-1
Jin M., Pan T. T., Cheng X., Zhu T. T., Sun P., Zhou F., et al. (2019). Effects of supplemental dietary l-carnitine and bile acids on growth performance, antioxidant and immune ability, histopathological changes and inflammatory response in juvenile black seabream (Acanthopagrus schlegelii) fed high-fat diet. Aquaculture 504, 199–209. doi: 10.1016/j.aquaculture.2019.01.063
Kaitetzidou E., Chatzifotis S., Antonopoulou E., Sarropoulou E. (2015). Identification, phylogeny, and function of fabp2 paralogs in two non-model teleost fish species. Mar. Biotechnol. 17, 663–677. doi: 10.1007/s10126-015-9648-6
Kortner T. M., Gu J., Krogdahl A., Bakke A. M. (2013). Transcriptional regulation of cholesterol and bile acid metabolism after dietary soyabean meal treatment in Atlantic salmon (Salmo salar L.). Br. J. Nutr. 109 (4), 593–604. doi: 10.1017/S0007114512002024
Kortner T. M., Penn M. H., Björkhem I., Måsøval K., Krogdahl Å. (2016). Bile components and lecithin supplemented to plant based diets do not diminish diet related intestinal inflammation in Atlantic salmon. BMC. Vet. Res. 12, 1–12. doi: 10.1186/s12917-016-0819-0
Kuhre R. E., Albrechtsen N. J. W., Larsen O., Jepsen S. L., Balk-Moller E., Andersen D. B., et al. (2018). Bile acids are important direct and indirect regulators of the secretion of appetite-and metabolism-regulating hormones from the gut and pancreas. Mol. Metab. 11, 84–95. doi: 10.1016/j.molmet.2018.03.007
Lefebvre P., Cariou B., Lien F., Kuipers F., Staels B. (2009). Role of bile acids and bile acid receptors in metabolic regulation. Physiol. Rev. 89 (1), 147–191. doi: 10.1152/physrev.00010.2008
Levy E., Spahis S., Sinnett D., Peretti N., Maupas-Schwalm F., Delvin E., et al. (2007). Intestinal cholesterol transport proteins: an update and beyond. Curr. Opin. Lipidol. 18 (3), 310–318. doi: 10.1097/MOL.0b013e32813fa2e2
Li T., Yan X. B., Dong X. H., Pan S. M., Tan B. P., Zhang S., et al. (2022). Choline alleviates disorders of lipid metabolism in hybrid grouper (♀ Epinephelus fuscoguttatus × ♂ E. lanceolatus) caused by high-lipid diet. Aquacult. Nutr. 2022, 8998849. doi: 10.1155/2022/8998849
Lin Y. H., Cheng W., Huang Y. S. (2022). Effects of bile acids supplementation in soybean meal-based diet on growth, cholesterol status, digestibility and moulting-related gene expression in white shrimp Litopenaeus vannamei. Aquac. Res. 53 (15), 5375–5381. doi: 10.1111/are.16020
Livak K. J., Schmittgen T. D. (2001). Analysis of relative gene expression data using real-time quantitative PCR and the 2(-delta delta C(T)) method. Methods 25 (4), 402–408. doi: 10.1006/meth.2001.1262
Loh K., Herzog H., Shi Y. C. (2015). Regulation of energy homeostasis by the NPY system. Trends. Endocrin. Met 26 (3), 125–135. doi: 10.1016/j.tem.2015.01.003
Lynes M., Narisawa S., Millán J. L., Widmaier E. P. (2011). Interactions between CD36 and global intestinal alkaline phosphatase in mouse small intestine and effects of high-fat diet. Am. J. PHYSIOL-REG. I 301 (6), R1738–R1747. doi: 10.1152/ajpregu.00235.2011
Macierzanka A., Torcello-Gómez A., Jungnickel C., Maldonado-Valderrama J. (2019). Bile salts in digestion and transport of lipids. Adv. Colloid. Interface. Sci. 274, 102045. doi: 10.1016/j.cis.2019.102045
Ni Q., Cai C. F., Ren S. J., Zhang J. B., Zhao Y. J., Wei X. Y., et al. (2021). Pectin and soybean meal induce stronger inflammatory responses and dysregulation of bile acid (BA) homeostasis than cellulose and cottonseed meal, respectively, in largemouth bass (Micropterus salmoides), which might be attributed to their BA binding capacity. Aquac. Res. 52 (7), 2963–2979. doi: 10.1111/are.15140
Peng X. R., Feng L., Jiang W. D., Wu P., Liu Y., Jiang J., et al. (2019). Supplementation exogenous bile acid improved growth and intestinal immune function associated with NF-κB and TOR signalling pathways in on-growing grass carp (Ctenopharyngodon idella): enhancement the effect of protein-sparing by dietary lipid. Fish. Shellfish. Immun. 92, 552–569. doi: 10.1016/j.fsi.2019.06.047
Rolen S. H., Caprio J. (2008). Bile salts are effective taste stimuli in channel catfish. J. Exp. Biol. 211 (17), 2786–2791. doi: 10.1242/jeb.018648
Romano N., Kumar V., Yang G., Kajbaf K., Rubio M. B., Overturf K., et al. (2020). Bile acid metabolism in fish: disturbances caused by fishmeal alternatives and some mitigating effects from dietary bile inclusions. Rev. Aquacult 12 (3), 1792–1817. doi: 10.1111/raq.12410
Shulpekova Y., Shirokova E., Zharkova M., Tkachenko P., Tikhonov I., Stepanov A., et al. (2022). A recent ten-year perspective: bile acid metabolism and signaling. Molecules 27 (6), 1983. doi: 10.3390/molecules27061983
Sun J. Z., Wang J. Y., Ma J. J., Li B. S., Hao T. T., Sun Y. Z., et al. (2014). Effects of dietary bile acids on growth, body composition and lipid metabolism of juvenile turbot (Scophthalmus maximus) at different lipid levels. Oceanol. Limnol. Sin. 45 (3), 617–625. doi: 10.11693/hyhz20140300092
Tan X. H., Sun Z. Z., Ye C. X. (2019). Dietary lycium barbarum extract administration improved growth, meat quality and lipid metabolism in hybrid grouper (Epinephelus lanceolatus♂ × E. fuscoguttatus♀) fed high lipid diets. Aquaculture 504, 190–198. doi: 10.1016/j.aquaculture.2019.01.044
Ulug E., Nergiz-Unal R. (2021). Dietary fatty acids and CD36-mediated cholesterol homeostasis: potential mechanisms. Nutr. Res. Rev. 34 (1), 64–77. doi: 10.1017/S0954422420000128
Unniappan S., Peter R. E. (2005). Structure, distribution and physiological functions of ghrelin in fish. Comp. Biochem. Phys. A 140 (4), 396–408. doi: 10.1016/j.cbpb.2005.02.011
Wang D. Q. H., Tazuma S., Cohen D. E., Carey M. C. (2003). Feeding natural hydrophilic bile acids inhibits intestinal cholesterol absorption: studies in the gallstone-susceptible mouse. Am. J. Physiol-Gastr. L 285 (3), G494–G502. doi: 10.1152/ajpgi.00156.2003
Xia S. D., Sun J. H., Li M., Zhao W., Zhang D., You H. Z., et al. (2020). Influence of dietary protein level on growth performance, digestibility and activity of immunity-related enzymes of leopard coral grouper, Plectropomus leopardus (Lacépède 1802). Aquacult. Nutr. 26 (2), 242–247. doi: 10.1111/anu.12985
Xiong F., Wu S. G., Zhang J., Jakovlic I., Li W. X., Zou H., et al. (2018). Dietary bile salt types influence the composition of biliary bile acids and gut microbiota in grass carp. Front. Microbiol. 9. doi: 10.3389/fmicb.2018.02209
Xu J., He G., Chen L., Xie S., Chi S., Zhang S., et al. (2022a). Farnesoid X receptor (FXR) and G protein-coupled bile acid receptor 1 (TGR5) signaling pathways improved the hepatic lipid metabolism in hybrid grouper. Aquacult. Rep. 22, 100997. doi: 10.1016/j.aqrep.2021.100997
Xu J., Xie S. W., Chi S. Y., Zhang S., Cao J. M., Tan B. P. (2022b). Protective effects of taurocholic acid on excessive hepatic lipid accumulation via regulation of bile acid metabolism in grouper. Food. Funct. 13 (5), 3050–3062. doi: 10.1039/d1fo04085e
Xu H., Zhang Y., Wang C., Wei Y., Zheng K., Liang M. (2017). Cloning and characterization of fatty acid transport proteins in Japanese seabass lateolabrax japonicus, and their gene expressions in response to dietary arachidonic acid. Aquac. Res. 48 (12), 5718–5728. doi: 10.1111/are.13395
Yamamoto T., Suzuki N., Furuita H., Sugita T., Tanaka N., Goto T. (2007). Supplemental effect of bile salts to soybean mealbased diet on growth and feed utilization of rainbow trout Oncorhynchus mykiss. Fish. Sci. 73, 123–131. doi: 10.1111/j.1444-2906.2007.01310.x
Yao T., Gu X., Liang X. F., Fall F. N., Cao A. Z., Zhang S. S., et al. (2021). Tolerance assessment of dietary bile acids in common carp (Cyprinus carpio L.) fed a high plant protein diet. Aquaculture 543, 737012. doi: 10.1016/j.aquaculture.2021.737012
Yin P., Xie S. W., Zhuang Z. X., He X. S., Tang X. P., Tian L. X., et al. (2021). Dietary supplementation of bile acid attenuate adverse effects of high-fat diet on growth performance, antioxidant ability, lipid accumulation and intestinal health in juvenile largemouth bass (Micropterus salmoides). Aquaculture 531, 735864. doi: 10.1016/j.aquaculture.2020.735864
Zhou Q., Guo X. Y., Huang Y., Gao H. Y., Xu H., Liu S. S., et al. (2020). De novo sequencing and chromosomal-scale genome assembly of leopard coral grouper, Plectropomus leopardus. Mol. Ecol. Resour. 20 (5), 1403–1413. doi: 10.1111/1755-0998.13207
Keywords: bile acids, Plectropomus leopardus, feed intake, lipid digestion, fatty acid transport
Citation: Gao Y, Yao Y, Huang J, Sun Y, Wu Q, Guo D and Wang S (2023) Effect of dietary bile acids supplementation on growth performance, feed utilization, intestinal digestive enzyme activity and fatty acid transporters gene expression in juvenile leopard coral grouper (Plectropomus leopardus). Front. Mar. Sci. 10:1171344. doi: 10.3389/fmars.2023.1171344
Received: 22 February 2023; Accepted: 11 April 2023;
Published: 21 April 2023.
Edited by:
Ming Li, Ningbo University, ChinaCopyright © 2023 Gao, Yao, Huang, Sun, Wu, Guo and Wang. This is an open-access article distributed under the terms of the Creative Commons Attribution License (CC BY). The use, distribution or reproduction in other forums is permitted, provided the original author(s) and the copyright owner(s) are credited and that the original publication in this journal is cited, in accordance with accepted academic practice. No use, distribution or reproduction is permitted which does not comply with these terms.
*Correspondence: Shengpeng Wang, d3NoZW5ncEBtYWlsMi5zeXN1LmVkdS5jbg==
†These authors have contributed equally to this work