- 1Center for Marine Studies, University of Parana, Pontal do Paraná, Brazil
- 2Collections and Research, Delaware Museum of Nature and Science, Wilmington, DE, United States
Life cycle definitions provide the background for conceptualizing meaningful questions to address the mechanisms that generate different life cycle patterns. This review provides explicit definitions and explanations of the steps in a cephalopod life cycle, from fertilization to death. Each large step, or phase, is characterized by a particular developmental process and morphology. Each phase is composed of smaller developmentally distinct steps, or stages. The cephalopod life cycle is comprised of all or some of the following phases: Embryonic, Paralarval, Juvenile, Subadult, Adult and Senescent, and each life cycle is taxon-specific. All cephalopods have direct development and maintain a consistent body plan throughout ontogeny (i.e., no true larval phase and no metamorphosis). Most cephalopods have a life cycle marked by a long early life and a short adult life followed by senescence. Cephalopods have two developmental modes: they produce either small planktonic hatchlings as paralarvae, or large hatchlings as juveniles. All cephalopods go through a Hatchling stage soon after eclosion during which they rely on two modes of nutrition: endogenous (yolk) and exogenous (prey). Many cephalopods with planktonic paralarvae will become benthic early in their life cycle during their Settlement stage or remain pelagic during their Metapelagic stage. Juvenile growth is fast and ontogenetic changes (outside of gonadal maturation) generally cease at the end of the Juvenile phase. The Subadult phase begins when the definitive adult morphology (except for size and body proportions) is acquired (e.g., full complement of photophores). Sexual organs undergo most of their development during the Subadult phase. The Adult phase starts with spawning competency and concludes when gonads are spent. The Senescent phase begins with spent gonads and ends with death. Using this new terminology, we examine the patterns of cephalopod life cycles and find that there are four main patterns based on the presence of a Paralarval phase and the habitat occupied by each phase: Holopelagic (all phases are pelagic), Holobenthic (all phases are benthic), Merobenthic and Meropelagic (phases alternate between benthic and pelagic environments). In these two last patterns, the main difference is the presence of a Paralarval phase in Merobenthic species. The definitions and terminology proposed here provide a unifying framework for future ecological, evolutionary and life cycles research on cephalopods.
1 Introduction
Metazoans have sequential developmental steps that are characterized by particular morphological, physiological, ecological and behavioral features (Roff, 1992; Stearns, 1992; Moran, 1994). The majority of marine invertebrates have complex life cycles with distinct steps or phases that differ radically from their adult forms (Levin and Bridges, 1995; Hejnol and Vellutini, 2017). Each phase can be subdivided into a series of stages that refers to any particular moment in development (Nesis, 1979; Eckman, 1996).
Molluscs possess a large diversity of body plans and developmental patterns. In non-cephalopod molluscs, development is indirect as it proceeds after hatching with a distinct larval phase (e.g., trochophore followed by veliger, pericalimma, or glochidia, Page, 2009; Ponder et al., 2019). These larvae undergo a radical metamorphosis where their entire body plan is reorganized to become a juvenile (Page, 2009; Ponder et al., 2021). Cephalopod life cycles and early development is dramatically different from other mollusks (Boletzky, 1987; Lee et al., 2003; Huan et al., 2020; Yang et al., 2020).
All cephalopods are direct developers. There is no larval phase or a true larva in the strict sense of the definition where larval parts are radically changed and new morphological features are formed from new anlagen (Geigy and Portmann, 1941; Nielsen, 2018) There is no metamorphosis. All structural features of late embryos are present in the post-embryonic and adult body plan with a few minor exceptions (Naef, 1928; Boletzky, 1974; Boletzky, 1987; Lee et al., 2003; Shigeno et al., 2010). All cephalopods hatch with a similar body plan: circumoral arm-crown, head with well-developed eyes and brain, beak and radula, ventral funnel, and a mantle covering the viscera (Figure 1) (Boletzky, 1974; Boletzky, 2003; Lee et al., 2003; Shigeno et al., 2010).
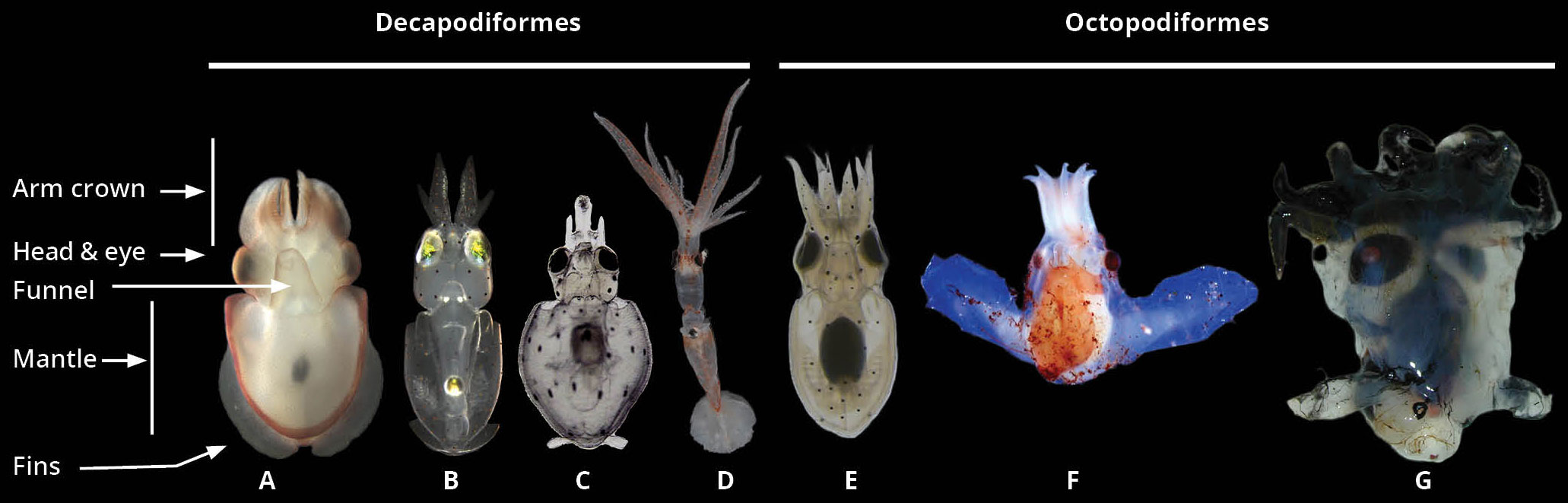
Figure 1 Body plan organization in coleoid cephalopod early life stages. Superorder Decapodiformes, (A) Sepiida, Sepiidae, (B) Myopsida, Loliginidae, (C) Oegopsida, Ommastrephidae, (D) Oegopsida, Chiroteuthidae and Octopodiformes, (E) Incirrata, Octopodidae, (F) Cirrata, Cirroteuthidae Photo credit: R.E. Young and (G) Vampyromorpha, Vampyroteuthidae.
Cephalopods have two developmental modes. Some species hatch as planktonic paralarvae that are morphologically distinct from the adults and occupy a different habitat from their neritic, pelagic, or benthic adult conspecifics. Other species hatch as juveniles that are morphologically similar to the adults and found in the same habitat. Regardless of mode, development is direct. The body plan of the planktonic hatchlings is the same as all other phases; however, there are transient morphological structures that facilitate temporary planktonic and planktotrophic lifestyle. These species may have a prolonged period of development before the Juvenile phase is reached (Sweeney et al., 1992; McEdward, 2000).
Within the cephalopod literature, these two modes of development have caused considerable confusion and controversy and have contributed to a lack of consensus in terminology (e.g. Boletzky, 1974; Nesis, 1979; Young and Harman, 1988; Boletzky, 2003) (see Section 2). Often, the term “indirect development” is misused as a proxy for species with planktonic hatchlings, and “direct development” as a proxy for those that hatch as juveniles (e.g. Mangold-Wirz, 1963; Nesis, 1979; Fioroni, 1982; Arkhipkin, 1992; Nesis, 1995; Nesis, 1999; Nesis, 2002; Uriarte et al., 2011; Ibáñez et al., 2014; Robin et al., 2014; García-Flores et al., 2022).
Inconsistent terminology confounds interpretations of developmental processes and obscures the similarities and dissimilarities in morphogenesis among the cephalopods. Clarifying life cycle definitions and terminology based on established criteria can provide a common background for analyzing the developmental diversity and, the mechanisms that have generated different life cycles patterns in cephalopod (Boyle and Rodhouse, 2005; Rosa et al., 2013a, b; Vidal, 2014).
Here, we develop a consensus terminology for cephalopod life cycles that can be used regardless of taxon and across all research disciplines. Using these new, explicit terms we classify the main life cycle patterns of cephalopods, considering the transitions in habitat relationships among the phases of the life cycles.
2 Early life cycle terminology
Cephalopod development has been described most often as direct (Akimushkin, 1963; Boletzky, 1974; Chia, 1974; McEdward and Janies, 1993; Bonnaud-Ponticelli and Bassaglia, 2014; Nielsen, 2018), but also as indirect (Fioroni, 1982), and both (Mangold-Wirz, 1963; Nesis, 1979; Nesis, 1999; Nesis, 2002). Cephalopod early life phases have been called hatchlings, larvae, paralarvae, and juveniles, with varying degrees of attention to definitions (e.g., Robin et al., 2014; Fernández-Gago et al., 2019; García-Flores et al., 2022; McCormick et al., 2022). Cephalopods have also been broken into functional groups separating adults (mature and maturing) from “juveniles” (paralarvae and immature) (Laptikhovsky et al., 2017b) to circumvent the difficulty of parsing out the different life cycle phases on a species-by-species basis. The few identification guides that exist for marine plankton occasionally include planktonic cephalopods as “larvae” (Todd et al., 1996; Boltovskoy, 1999), sometimes call them “paralarvae” (Johnson and Allen, 2012), and other times do not treat cephalopods at all (Newell and Newell, 1977).
In the mid-20th century, newly-hatched and small cephalopods were commonly called “larvae” (e.g., Allan, 1945; Rees, 1953; Akimushkin, 1963; Mangold-Wirz, 1963; Okutani and McGowan, 1969; Yamamoto and Okutani, 1975). Allometric analyses provided support for distinguishing between larvae and older conspecifics in oegopsid squids and suggesting important changes to the “mode of life”, particularly behavior, habitat and predation (Clarke, 1966; Kubodera and Okutani, 1977).
Cephalopod researchers have long recognized a suite of morphological characters that are common to the early life stages including a sac-shaped body, paddle-shaped terminal fins, and large chromatophores (Sweeney et al., 1992; Nesis, 1999; Zaragoza et al., 2015). Other more distinctive features are family-specific, such as the fused tentacles in Ommastrephidae or the stalked eyes in Cranchiidae (Boletzky, 1974; Nesis, 1979; Fioroni, 1982). These distinctive features are often cited as major metamorphic differences but they are gradually modified and changed with ontogeny. Gradual modification of morphology is generally considered evidence of direct development (Page, 2009).
Categorizing and contextualizing the degree of morphological differences and whether these differences are significant enough to identify a distinctive larval phase of development has been the subject of much debate. The appropriateness of using larva in cephalopods was hotly debated during the 1985 Cephalopod International Advisory Council (CIAC) meeting in France.
Clearly cephalopods have no reorganization of the body comparable to that of other mollusks. Boletzky (1974) reasoned that many of the previously identified “larval” features (e.g., underdeveloped arm crown) are simply adult characters being expressed at an extremely small size. But Nesis (1979) argued that changes in growth coefficients could be construed as a type of metamorphosis, and continued to use “larva” to describe the early life stages.
Soon after the CIAC meetings, Young and Harman (1988) introduced the term “paralarva” to circumvent the developmental terms and to emphasize ecological concepts. The prefix “para” in Latin means “nearly” and paralarva was formally defined as “a cephalopod of the first post-hatching growth stage that is pelagic in near-surface waters during the day and that has a distinctively different mode of life from that of older conspecific individuals”. The definition explicitly involved an ecological criterion - the daytime habitat differences of paralarvae and adults due to diel vertical migration (DVM) - which had not been previously considered (Boletzky, 2003).
Paralarva was not meant to replace developmental terms, but resulted in a confusing situation where an individual could be both a larva or a juvenile (developmental) and a paralarva (ecological). Using DVM as a marker of the end of the Paralarval phase was a way to distinguish between planktonic and nektonic animals, but requires fine-scale understanding of size-dependent depth distributions which are unknown for most open ocean species. Furthermore, it was later shown that coastal species (e.g., Dorytheuthis opalescens and Octopus vulgaris) perform DVM from the time of hatching (Zeidberg and Hamner, 2002; Roura et al., 2019), and can exert some influence over their fine-scale distribution (Vidal et al., 2018), suggesting that other species may be competent to undergo DVM even if available sampling equipment is not sufficient to document it.
Young and Harman (1988) also suggested morphometric data could mark the end point of the Paralarval phase, as could family-specific morphological features (e.g., fused tentacles in Ommastrephidae or the circular club in Chtenopterygidae). Ecological and morphological methods of identifying the endpoint of the Paralarval phase can be in conflict. Okutani (1989) argued that newly-hatched Vampyroteuthis infernalis could be considered a paralarva based on its morphological features, but hatchings are never found in near-surface waters. In addition, some holopelagic octopods have adults that are found in the surface waters during both the day and night (e.g., Argonautidae, Tremoctopodidae).
Strictly defining the Paralarval phase of a species using Young and Harman’s DVM-based model also requires a compendium of species-specific data, including a well-resolved understanding of collecting depth, a large number of specimens of varying sizes to describe growth, a large set of measurement data, and specimens in good condition to identify a morphological marker (Shea and Vecchione, 2010). Because this is so difficult, “paralarva” has been generally adopted as a shorthand for “planktonic cephalopod” with varying degrees of attention to the original formal definition (see Boletzky, 2003 for multiple examples). Here, we adopt the community usage of paralarva as being roughly equivalent to “planktonic cephalopod”, but we provide a more explicit and largely applied definition, and put this definition into context of the other phases of the cephalopod life cycle.
3 Cephalopod life phases and stages
Although both morphological and ecological factors are essential to understand life cycles (McEdward, 1995; Boyle and Rodhouse, 2005; Hanlon and Messenger, 2018), relying on morphology to define the life steps is consistent with past practice in embryology (Naef, 1928; Arnold, 1965) and maturity scales (Lipínski, 1979; Arkhipkin, 1992). Well-described morphological character states are easy to understand, are stable, and are thus broadly applicable and accessible. Using morphology is independent of any particular ecological framework (e.g., vertical migrations, feeding, survival), which is especially important because there are still fundamental knowledge gaps in many species. Finally, morphology may drive ecology (Vidal et al., 2018) or alternatively constrain behaviors (Kier, 1996; Boletzky, 1997; Hanlon and Messenger, 2018; Vidal and Salvador, 2019).
The terms life history and life cycle are often used interchangeably but are distinctly different. Life history describes how individuals, populations, or species survive, grow and reproduce in response to their physical environment. Important life history traits include longevity, age-at-maturity, and fecundity, all of which inform the ability of a species to survive from generation to generation. Alternatively, life cycle is used to describe the species-specific developmental sequence of morphological changes that begins with fertilization, includes all ontogenetic phases and stages, and ends with death. Life cycle traits may include the development or modification of a morphological feature and/or a change in habitat.
The cephalopod life cycle is composed of a series of major and minor steps that can be reliably delineated using morphology. Phases are major steps that are delimited by important milestone events in the life cycle (e.g. fertilization, eclosion, etc.) (Figure 2A). Each phase of life is marked by a particular developmental process and morphology. Each phase is composed of many smaller morphologically distinct steps called stages (Figure 2B) (Naef, 1928; Nesis, 1979; Eckman, 1996). Some stages are found in all taxa (e.g., Hatchling stage) but others may be species-specific (e.g., Settlement stage in small-egged octopods, see Section 3.7).
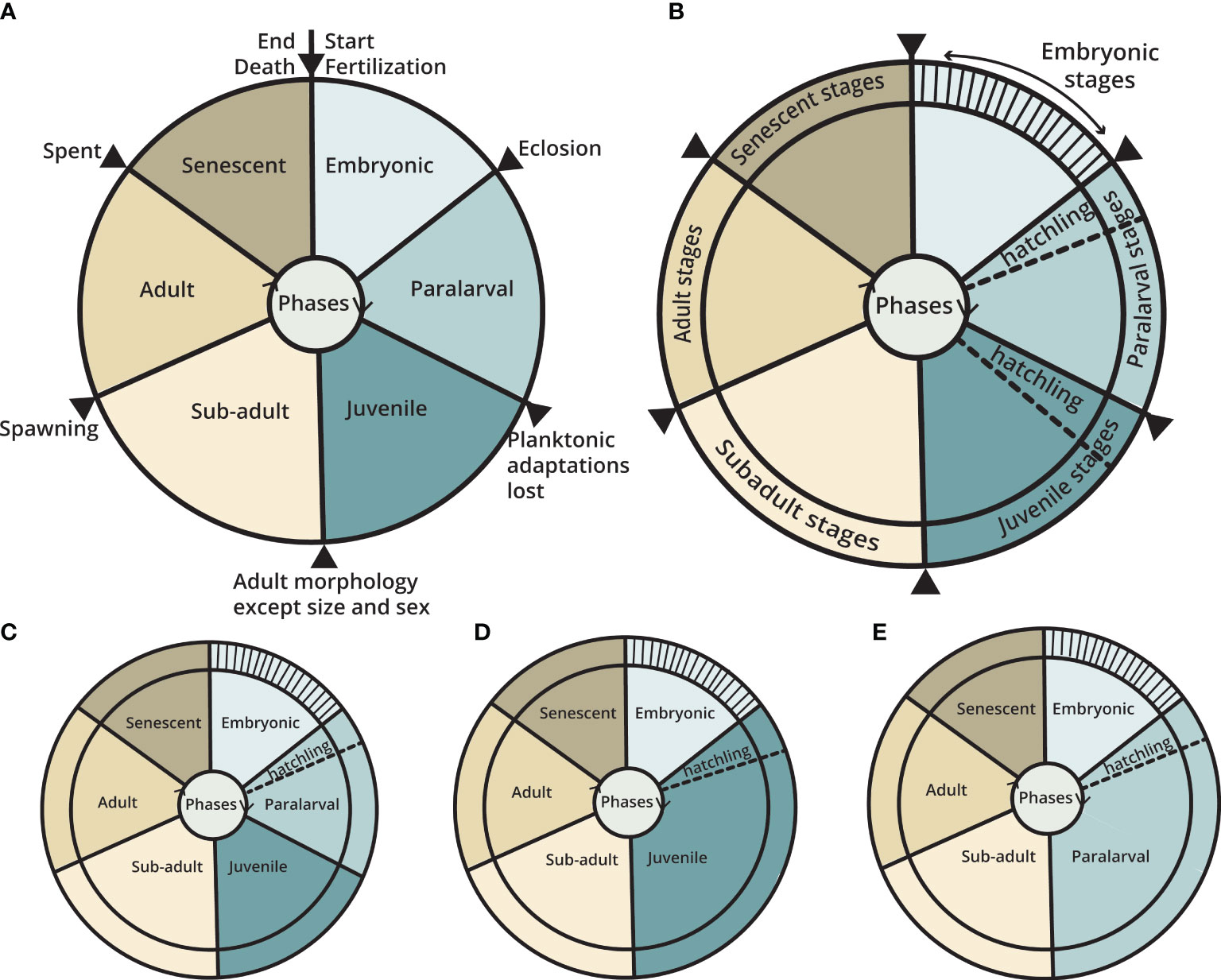
Figure 2 The phases and stages of a single cephalopod life cycle, from fertilization to death. (A) Phases are major divisions of the life cycle. Here, all phases are evenly distributed and color coded for easy comparison. The limit of each phase is marked by a black dividing line and arrowhead and is annotated with the important transitory events or milestones. (B) Stages are smaller, developmental steps within a phase. Here, they are indicated by subdivisions around the circle perimeter. The Hatchling stage is found either in the Paralarval or Juvenile Phase, whichever comes first. (C) Generalized life cycle of a species with a Paralarval phase (D) Generalized life cycle of a species that hatches as a Juvenile and, (E) Generalized life cycle of a species that hatches as a Paralarva and does not have a Juvenile phase. The duration of each phase in relation to the other phases or the whole life cycle are species-specific, and the length of an individual life cycle is influenced by abiotic factors such as temperature.
The timing of transitions between phases and stages is species-specific, with individual and sex-based variations (Nesis, 1979; Okutani, 1987; Vidal, 1994; Shea and Vecchione, 2002). Environmental conditions impact growth rates, but temperature and food availability are particularly important influences (Forsythe et al., 2001) over the size and age at which an individual will transition between phases and stages.
In the following sections, we propose standardized terminology and definitions that are applicable for all cephalopod life cycles.
3.1 Embryonic phase
Definition: The Embryonic phase of the cephalopod life cycle is enclosed in an egg. This phase begins with fertilization and ends with eclosion (=hatching) (Table 1).
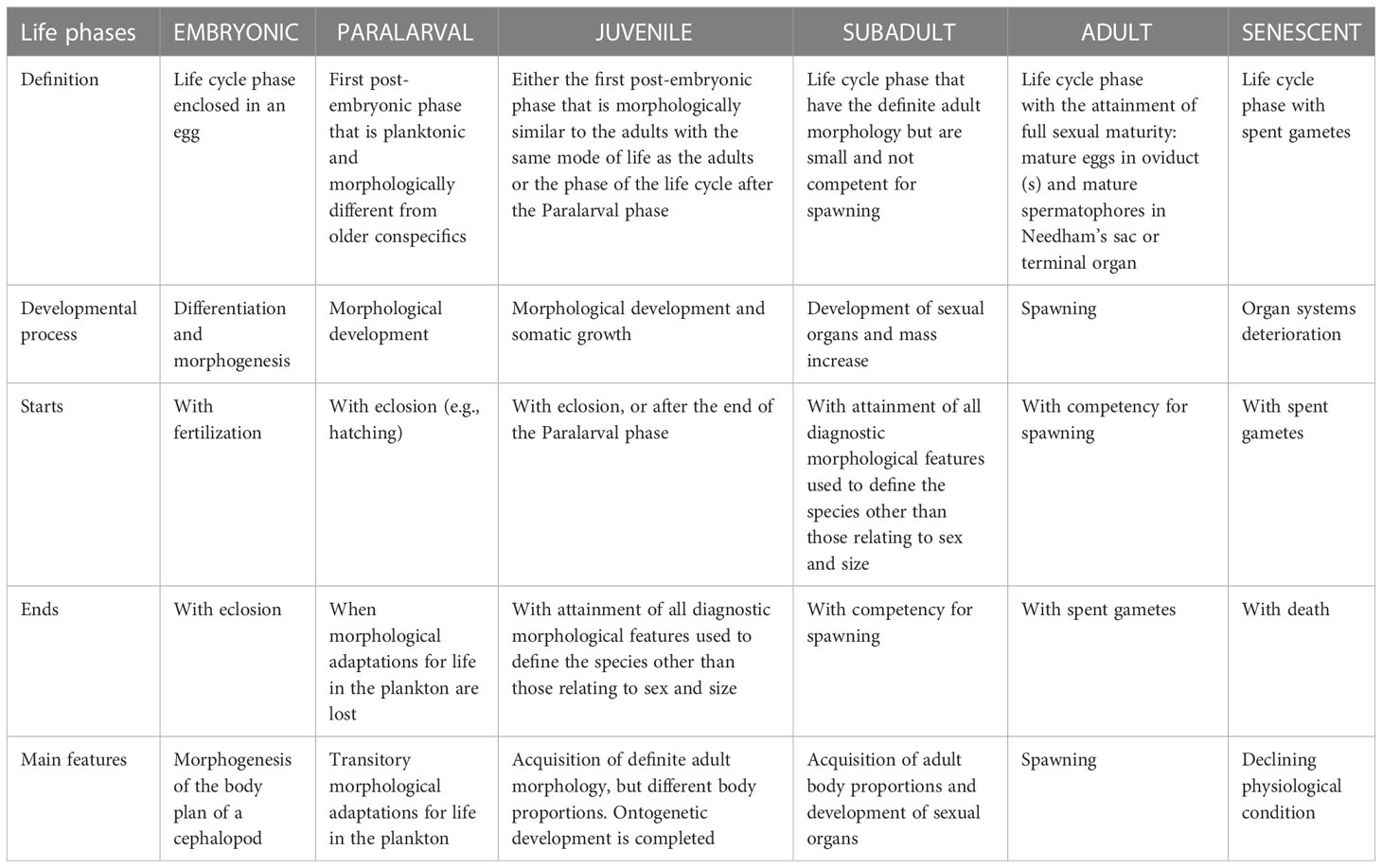
Table 1 Definitions, main developmental process and limits of each phase of the cephalopod life cycle.
The Embryonic phase begins after the egg is fertilized and cell differentiation starts. By the end of this phase, the definitive body plan of a cephalopod is attained.
Cephalopods produce yolk-rich telolecital eggs with large meroblastic discoidal cleavage (Boletzky, 1987). The nutrition of the embryos is provided by yolk within the eggs and composed mainly of proteins, carbohydrates and lipids. Traditional stages of embryogenesis include cleavage, gastrulation, organogenesis and growth (Boletzky et al., 2016).
Descriptive embryology scales range from 18 - 30 morphologically distinguishable, developmental stages, depending on taxon and proposed scale (Naef, 1928; Arnold, 1965; Lemaire, 1970; Boletzky et al., 2016; Deryckere et al., 2020). The duration of development is species-specific, strongly dependent on temperature and egg size. Embryogenesis can take 10-15 days for sepiolid small eggs incubated at warmer temperatures (Nabhitabhata, 2014; Nabhitabhata and Nishiguchi, 2014) or many months to years in large-egged octopus incubated at colder temperatures (Uriarte et al., 2010; Robison et al., 2014; Khen et al., 2022). Within the optimal incubation temperature range for a given species, higher temperatures shorten the time between spawning and hatching and those outside the optimal range increase the incidence of abnormalities and death (Vidal and Boletzky, 2014). The interplay between egg size, incubation temperature and egg-laying timing will be the main determining factors of hatching time and will set the conditions for survival and growth of the hatchlings. There are, however, many other environmental factors that influence embryonic development and hatching success (Vidal et al., 2014).
3.2 Paralarval phase
Definition: The Paralarval phase begins at eclosion and ends when adaptations for life in the plankton have been lost and/or the individual is no longer planktonic. Individuals are morphologically distinct from older conspecifics (Table 1 and Figure 2C).
This phase is marked by unique transitory morphological specializations that are adaptations for dispersal, locomotion, feeding, defense and, camouflage in the plankton regardless of depth (Table 2; Figure 3). These specializations characterize and differentiate a paralarva from species that hatch from large eggs as juveniles and live in the same habitat as the adult conspecific (e.g., Haliphron atlanticus, O’Shea, 2004).
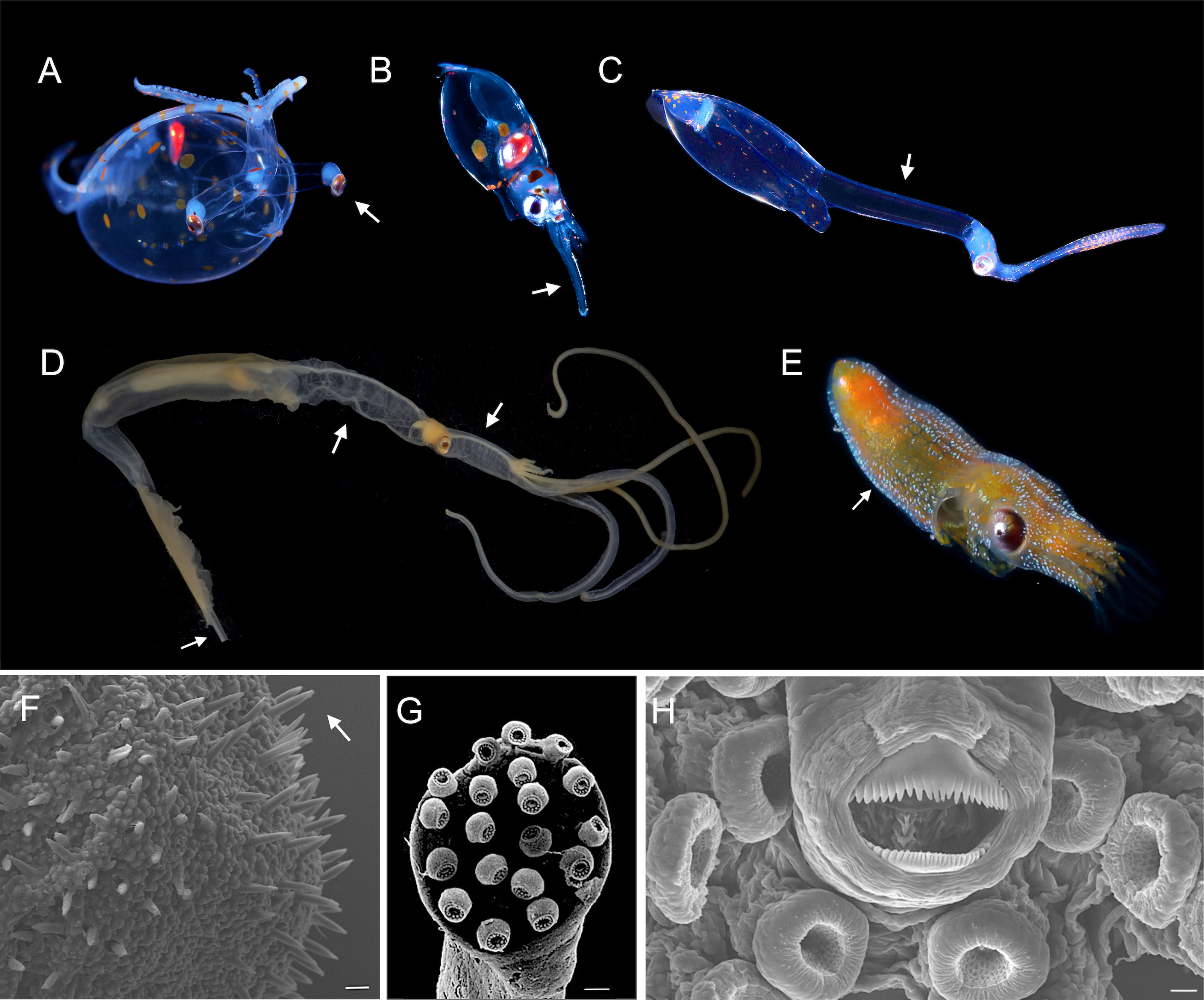
Figure 3 Paralarval transitory morphological adaptations. (A) Stalked-eyes in the cranchiid, Leachia, Photo credit: R. Minemizu. (B) Proboscis in ommastrephid rhynchoteuthion, Photo credit: L. Ianniello. (C) Neck in Brachioteuthis, Photo credit: R. Minemizu. (D) Neck, brachial pillar and long tail with most part broken off in Chiroteuthis spoeli, Photo credit: R.E. Young. (E) Kölliker organs in Octopodidae paralarvae (light blue points), Photo credit: R. Minemizu. (F) Kölliker organs on the posterior mantle of Octopus americanus, scale bar= 20 µm. (G) Circular club in Chtenopteryx sicula, scale bar= 15 µm. (H) Buccal mass showing denticulated upper and lower beaks in Octopus americanus, scale bar= 10 µm.
During the Paralarval phase the main developmental process is morphological development. Changes can vary from minor differences in proportions (e.g., Sepiolidae) to pronounced changes in structure and overall morphology (e.g., Chiroteuthidae).
After eclosion, paralarval body parts develop fast and allometry operates at high rates. The arm crown and fins are often rudimentary or underdeveloped at eclosion but develop quickly (e.g. Boletzky, 1974, 2003; Okutani, 1987; Vidal, 1994; Villanueva, 1995; Shea and Vecchione, 2002; Wakabayashi et al., 2005), particularly tentacular clubs and stalks (Sweeney et al., 1992; Vidal and Salvador, 2019). In some species development may lead to degeneration of tentacles (e.g. Gonatopsis, Okutani, 1987). Chromatophores increase in number and patterns become more complex (e.g. Young et al., 1985; Young and Harman, 1985b; Sweeney et al., 1992; Vidal, 1994; Zaragoza et al., 2015), photophores might develop (Nesis, 1979; Wakabayashi et al., 2002). Although many paralarvae lack photophores as well as hooks, both may develop later in some species (Young, 1972; Boletzky, 2003).
Some paralarval specializations are unique to particular families or genera (Table 2; Figure 3). Chiroteuthide doratopsis paralarvae are marked by a branchial pillar, a long neck and a long tail (Figure 3D). In Ommastrephidae, the tentacles are fused into a proboscis (Figure 3B), which progressively split into the two tentacles. The circular club of Cthenopterygidae is unique (Figure 3G), as is the circumbrachial cuff-shaped membranes in Argonauthidae and Tremoctopodidae (Sweeney et al., 1992). Even more remarkable and morphologically distinct are the conspicuous stalked eyes of most Cranchiidae paralarvae (Figure 3A) and the two fins stage in Vampyroteuthidae (Young and Vecchione, 1999).
Other less obvious changes also occur during this time. In many families, the fragile, lightly pigmented and denticulated beaks of hatchling paralarvae change during this phase of life into more heavily pigmented, tooth-less beaks with pointed rostra. This change likely represents a change from external pre-digestion and suction of body fluids of crustacean prey to biting and tearing flesh (Franco-Santos and Vidal, 2014; Franco-Santos and Vidal, 2020). Thus, the set of characters that defines a paralarva include their relatively small hatching size and morphological adaptations to the planktonic habitat. These adaptations create a distinct morphology from older conspecifics, but are contained within the basic cephalopod body plan (Figure 1, 3).
Cephalopods with a Paralarval phase have larger dispersal potential and thus wider adult geographical distribution than species without a Paralarval phase (Villanueva et al., 2016). The length of time an individual spends as a paralarva is determined in part by the size at eclosion and environmental temperature, which in turn impacts the fitness, dispersal potential, trophic niche and mortality (Levin and Bridges, 1995; Morgan, 1995). The relative duration of the Paralarval phase has been directly observed for only a few coastal cephalopod species raised under laboratory conditions. Results show that hatchlings that are morphologically similar to the adults have a shorter Paralarval phase than species that are morphology dissimilar (Table 3).
The Paralarval pahse may be brief, lasting for hours to days with paralarvae found in roughly the same location as adults (e.g., Sepioloida, Idiosepiidae). At the other end of the spectrum, the Paralarval phase may last several months in colder waters species, which allows for considerable dispersal (e.g., Enteroctopus dofleini, Table 3). Although a longer planktonic period is often associated with increased mortality and advection (Morgan, 1995), it can also be advantageous for maximizing dispersal when benthic habitats are constantly changing or when habitat choice for settlement has important implications for survival. Some benthic octopods, such as Octopus rubescens (Hochberg et al., 1992), Pteroctopus tetracirrhus (Villanueva et al, 2020), and Macrotritopus spp. (Judkins and Vecchione, 2020) can attain large sizes in the water column in coastal and oceanic waters (Villanueva and Norman, 2008).
3.3 Juvenile phase
Definition: The Juvenile phase begins either after eclosion or after the Paralarval phase is complete and ends when all diagnostic morphological features used to define the species other than those relating to sex and size have been attained (Table 1).
In species that hatch as juveniles, the hatchlings are large, well developed and morphologically similar to the adults and often occupy the same habitat of the adults.
Complex behaviors are common in large juvenile hatchlings. For example, Sepia officinalis hatchlings are capable of changing color and texture as fast as and effectively as adults and can display almost every adult body pattern, even though leucophores and iridophores have not yet developed and patterns and displays refine during ontogeny (Hanlon and Messenger, 2018). Similarly, feeding behavior in cuttlefish hatchlings is as accurate as in adults (Messenger, 1977), contrary to the basic feeding behavior of Octopus and loliginid paralarvae (Villanueva, 1995; Vidal and Salvador, 2019).
These abilities involve advanced development of the visual (binocular depth-detection) and neuromotor (camouflage and swimming coordination) systems (Hanlon and Messenger, 2018). Intricate body patterns are important for camouflage, predator avoidance and efficient predation in the benthos. Thus, the set of character states that defines a juvenile are their relatively large size at eclosion, a well-developed nervous system, and an overall morphology that is similar to the adult conspecific.
The largest coleoid cephalopod hatchlings are benthic/demersal juveniles. The Antarctic incirrate, Megaleledone setebos, is likely the largest based on its egg size (41.5 mm, Allcock et al., 2003). Other large juvenile hatchlings are found in subtropical coastal reef areas (e.g. Sepia latimanus, 14 mm ML), in temperate to polar waters (e.g. Eledone moschata, 10.5 mm ML) and in the deep-sea [e.g. Graneledone boreopacifica, 28 mm ML (Villanueva et al., 2016); Octopus californicus, 12 mm ML (Khen et al., 2022); Grimpoteuthis sp., 13 mm ML (Shea et al., 2018)].
In species that have a planktonic paralarva, the Juvenile phase is reached after the attainment of morphological development required to adopt the full adult habitat (Figure 2C). It is noteworthy that the few studies available for small-egged Octopodidae species, have shown that the morphology of post-settlement juveniles is quite similar to that of large-egged juvenile benthic hatchlings (see Section 3.7) (Promboon et al., 2011; Dan et al., 2022; Roura et al., 2023).
Morphological development continues in the Juvenile phase but the phase is primarily characterized by extremely fast, even exponential growth rates in both the wild and under laboratory conditions (Forsythe and Van Heukelem, 1987). Growth in length is intensified in relation to other body parts and thus, body proportions and shape changes. This is an important developmental process because variation in a wide variety of morphological and physiological traits are highly correlated with variation in organism size (Kubodera and Okutani, 1977; Hernandéz-Garcia et al, 1998; Pélabon et al., 2014). These morphological changes in body proportions and growth have been well documented in many families.
High growth rates are possible because of high feeding rates, capacity for exceptional food conversion efficiencies and the allocation of energy to gonad production is reserved until late in the life cycle (Boyle and Rodhouse, 2005). Individual growth rates are subject to considerable plasticity both in laboratory conditions and in wild populations (e.g. Forsythe and Van Heukelem, 1987; Semmens and Moltschaniwskyj, 2000), which causes large variability in size-at-age estimation in wild populations (Boyle and Rodhouse, 2005).
In addition to fast growth, juveniles develop species-specific morphological features. For example, body proportions change and morphological features such as hooks are formed in onychoteuthids and pterygioteuthids (Kubodera and Okutani, 1977; Young and Harman, 1988). Photophores develop in many families (Young, 1975a; Sweeney et al., 1992; Roper and Jereb, 2010). In Pterygioteuthis microlampas the end of the Juvenile phase is marked by the development of arm hooks at 9-11 mm gladius length (Young and Harman, 1988).
The development of these species-specific features, particularly chromatophores and photophores, play key roles in the recognition of and visual communication with conspecifics (Hanlon and Messenger, 2018). In Thysanoteuthidae, the development of the anal photophore and the relative length of arms are important in the formation of pairs of same-sized juvenile males and females (<100 mm ML), that are believed to remain together during their lifetimes, a unique social organization among cephalopods (Nigmatullin and Arkhipkin, 1998; Roper and Jereb, 2010). Also, sexual dimorphism can be expressed in some families as a result of different growth rates and heterochrony between males and females. The Juvenile phase ends with the acquisition of the adult morphology, even though body proportions are different from the adults.
The Juvenile phase is absent in some families. This is particularly evident in the Chiroteuthidae (except in Planctoteuthis spp.), when the doratopsis paralarval clubs are resorbed to give place to the adult clubs, indicating the transition from the Paralarval phase to the Subadult phase (Young, 1991; Bitondo, 2016) (Figure 2E).
3.4 Subadult phase
Definition: The Subadult phase begins with the attainment of all diagnostic morphological features used to define the species other than those relating to sex and size, and ends with competency for spawning (Table 1).
The Subadult phase or “adolescent” phase as coined by Nesis (2002), is the life phase where the definite adult morphology exists but individuals are not full grown and not competent to spawn. Although for many families there is uncertainty in the differentiation of juveniles from subadults, the subadults have the definitive adult morphology, that is, ontogenetic development is completed. The definitive species features are found in the patterns, shapes and relative sizes of subadults, which have the appearance of adults but are smaller than adults and are not competent to spawn (e.g. Roper and Young, 1975; Young and Harman, 1988; Arkhipkin, 1992).
The main developmental process in this phase is gonadal growth and mass increase that progress until the attainment of the adult body proportions and full competence to spawn. By the end of the Subadult phase somatic growth is virtually completed and the body proportions of the adults achieved or nearly so (Table 1).
The onset of maturation (e.g. microscopic development of sexual cells) in the Subadult phase marks the end of the logarithmic growth phase in coleoid cephalopods (Forsythe and Van Heukelem, 1987). Both feeding and food conversion rates slow down at larger body size and consequently growth rates slow down. There are, however, exceptions to this pattern; argonauts and cirrate octopods continue feeding and growing over a wide range of sizes (Villanueva, 1992; Laptikhovsky and Salman, 2003). If sexual dimorphism was not yet expressed in the Juvenile phase, it will become evident in the Subadult phase due to the different growth rates between males and females and the development of the reproductive system (Nesis, 1985, 1995; Boyle and Rodhouse, 2005).
The set of conditions that determine the onset of sexual maturation are complex, not fully understood, entangling many intrinsic and extrinsic factors (Arkhipkin, 1992; Boyle and Rodhouse, 2005; Hoving et al., 2014). Environmental factors are known to trigger the onset of sexual maturation and indirectly reduce feeding and growth rates (e.g. temperature, nutrition, photoperiod). In addition, transition from somatic to gonadal growth is hormonally mediated by the optic gland secretions, which seems to be controlled by photopheriod (O’Dor and Wells, 1978; Arnold, 1984).
Maturation is characterized by the development, packaging, and storage of eggs and sperm. Females can store sperm and delay fertilization and production of eggs. The degree of maturation is based on descriptive scales (e.g., Lipínski, 1979; Arkhipkin, 1992). Full sexual maturity is attained when males and females are competent to spawn (= spawning competency).
Spawning competency is reached when males have mature spermatophores in Needham’s sac or terminal organ and females have visible mature eggs in oviducts (Arkhipkin, 1992; Boyle and Rodhouse, 2005). Spermatophores are transferred from males to females by the hectocolylized modified arm or by the penis. The end of the Subadult phase is marked by attainment of the full adult body proportions and competency to spawn.
Not all species will have a Subadult phase if the acquisition of the adult morphology and diagnostic features takes place simultaneous with the attainment of sexual maturity. This is the case of the life cycle of Leachia pacifica that transition from the Juvenile to the Adult phase (Young, 1975b).
3.5 Adult phase
Definition: The Adult phase begins with the attainment of full sexual maturity as determined by the presence of mature eggs in oviduct (s) and mature spermatophores in Needham’s sac or terminal organ and ends when gametes are spent (i.e. senescence) (Table 1).
The Adult phase of life is marked by storage and use of functional gametes and the spawning process. Reproduction is seasonal in most species. Females may spawn all their eggs over a short period of time (terminal spawning) or distinct bouts of spawining in one season (separate batch or repeated spawning), or during different spawning seasons and, some spawn continuously (Rocha et al., 2001). Species with terminal spawing do not grow between batches and are likely to have a short life span and to die soon after spawning, while those with prolonged spawning periods usually feed and grow between spawnings (Mangold, 1987; Rocha et al., 2001).
Encapsulation of eggs is a feature of cephalopods with a great diversity of capsule structure that varies phylogenetically (Boletzky, 1986; Boletzky, 1998). Among oegopsids, eggs are generally small (1-3 mm) and egg masses are still poorly known. Some species spawn single eggs, others neutraly boyant large egg masses and others “brood” their eggs. In many species spawning is bottom-associated (Nesis, 1995). Myopsid squid eggs are deposited in benthic “mops” where few to multiple eggs (2-10 mm) are organized into capsules (Jereb and Roper, 2010). Sepiidae and Sepiolidae have medium to large eggs (3-10 mm) which are either laid individually or in small clusters in the bottom or in benthic structures (Jereb and Roper, 2005). Cirrate octopods lay large, individual eggs encased in a leathery outer layer on a variety of bottom structures (Boletzky, 1982; Ziegler et al., 2021). In incirrate octopods, both benthic and pelagic, eggs vary greatly in size (1-45 mm) and are laid in batches or individually and brooding takes place until the eggs hatch (Jereb et al., 2016). There is a trade-off between egg size and fecundity. Spawning demands high energy and can occur continually or intermittently for a protracted period of weeks or months and is generally accompanied by an overall physiological deterioration.
The Adult phase of life is generally short, but there are exceptions. A life-span of several years are expected in all cold-water octopod species (Robison et al., 2014) and in nautilids.
After reaching maturity, nautlids lay a few eggs every year (Dunstan et al., 2011a) and can live at least 20 years (Barord and Basil, 2014). There is no information available on the life-span of cirrates.
3.6 Senescent phase
Definition: The Senescence phase begins when gametes are spent and ends with death (Table 1).
The Senescent phase is the final phase of the cephalopod life cycles characterized by overall deterioration of the animal. This phase can last for weeks or months, and is species-specific and temperature dependent. Organ systems degenerate and they cease to function. Declining physiological condition leads to considerable morpho-physiological transformation that characterizes the senescent individual. The morphology of a senescent individual may be quite different from that of the adult, and were even described as a new species (Chaunoteuthis mollis, synonym of Onychoteuthis banksii, Nesis, 1995).
The physiological processes that trigger senescence are not fully understood but senesence is associated with the optic gland control of gonad maturation, spawning and feeding inhibition (Roumbedakis and Guerra, 2019). These changes are likely triggered by reduction or cessation of feeding leading to weight loss and starvation, re-mobilization of somatic protein that causes muscle breakdown and flaccid tissues, skin lesions, cloudy eyes and retraction of the skin around the eyes, loss of coordination, parasite infection, among others (Chichery and Chichery, 1992; Jackson and Mladenov, 1994; Anderson et al., 2002; Roumbedakis and Guerra, 2019).
In the oegopsids, senesence is reported as degraded musculature, with spent females undergoing a gelatinous degeneration and losing their tentacles (Jackson and Mladenov, 1994; Nesis, 1995; Seibel et al., 2000; Laptikhovsky et al., 2019). In Illex argentinus, the mantle becomes thinner and alongated and degeneration of body parts is extreme (Laptikhovsky and Nigmatullin, 1992). Spent females are known to float passively to the surface becoming available for seabirds to forage (Xavier et al., 2014). This is documented in Ancistrocheirinae, Octopoteuthidae, Gonatidae, Histioteuthidae, Cranchiidae among other families (Nesis, 1995).
This phase of life is best-known and described in shallow water species, particularly incirrate female octopods that brood their eggs for weeks to months and even years without feeding (Guerra, 1993; Anderson et al., 2002; Robison et al., 2014; Roumbedakis and Guerra, 2019). Some species are known to use the energy and nutrient reserves of their somatic tissues to fuel the brooding process and this results in loss of muscle mass and physiological condition that contributes to senescence.
In cirrates, sexual maturity likely takes place during most of their lives and egg laying is not related to senescence (Boyle and Rodhouse, 2005). Nautilids are the only cephalopods without optic glands (Arnold, 1984) and senescent individuals have not yet been described.
3.7 Stages in cephalopod life cycles
Each phase of the life cycle is composed of several stages. A stage is defined here as a morphologically distinguishable step in development (Naef, 1928; Nesis, 1979; Eckman, 1996). The number of stages in each life phase and their beginning and end point are species-specific, but stable within a species. Ideally, the complete set of stages within each phase of the life cycle should be known, but this requires an in-depth knowledge of the life cycle and a comprehensive size series that does not exist for most cephalopods. Delineating stages represents an important next step in life cycle research.
The Embryonic stages of inshore and commercially important species are most thoroughly described. Naef (1928), described and illustrated these stages for several species that served as a template for subsequent comparative studies (e.g. Arnold, 1965; Lemaire, 1970; Shigeno et al., 2010; Boletzky et al., 2016; Deryckere et al., 2020). Even so, detailed Embryonic stages of most oegopids and cirrates are still completely unknown. We define the Embryonic stages as: Distinct development steps that occur within the egg during morphogenesis of the cephalopod body plan.
The Hatchling stage is also common to all cephalopods, but usage of the term Hatchling has been highly variable, referring to a variety of life stages from newly-hatched individuals to older paralarvae and even juveniles of unknown age (e.g. Vidal et al., 2002; Robin et al., 2014; Kingston et al., 2015; Fernandéz-Gago et al, 2019; Bazarini and Crook, 2020). We define the Hatchling stage as: The first post-embryonic stage of the cephalopod life cycle. It begins after eclosion and ends with complete absorption of yolk reserves and loss of structures required for hatching (Figure 2B).
During development, and for a short time after eclosion, all cephalopods have an inner yolk sack containing maternal reserves, which are the remnant of the embryonic ‘yolk organ’ (Boletzky, 2010) and/or transitory morphological structures specialized for hatching (e.g., Hoyle organ) even after the onset of exogenous feeding. Yolk absorption takes place independent of and concomitant with the digestion of captured prey and there is a temporary overlap of two modes of nutrition: endogenous (embryonic nutritional system for digesting yolk, i.e., lecithotrophy) and exogenous (digestion of exogenous prey, i.e., planktotrophy or predation on benthic prey) (Vidal et al., 2002; Boletzky, 2010). This mixotrophic nutrition allows the hatchling to cope with shortages of suitable prey, the transition to competent predation, and generally mitigates failure at first feeding (Vecchione, 1987; Boletzky, 2010). Consequently, the Hatchling stage is characterized by high mortality rates that have been well documented in laboratory studies (Vidal et al., 2002, Vidal et al., 2014; Iglesias et al., 2007; Braga et al., 2022).
Individual yolk content at eclosion is highly variable and hatchlings can be premature (Figure 4A), normal or late. Premature hatchlings eclose without complete absorption of the outer yolk sac; normal hatchlings eclose after complete absorption of the outer yolk sack and late hatchlings eclose when nearly all the inner yolk sac has been absorbed (Vidal and Boletzky, 2014). The Hatchling stage is easily recognized in the laboratory by monitoring hatching day and the absorption of the inner yolk sack (Vidal et al., 2002), but more difficult in the wild and must be inferred from the presence of the Hoyle organ (Figure 4B) and/or an inner (Figure 4C) or outer yolk sack (Figure 4A).
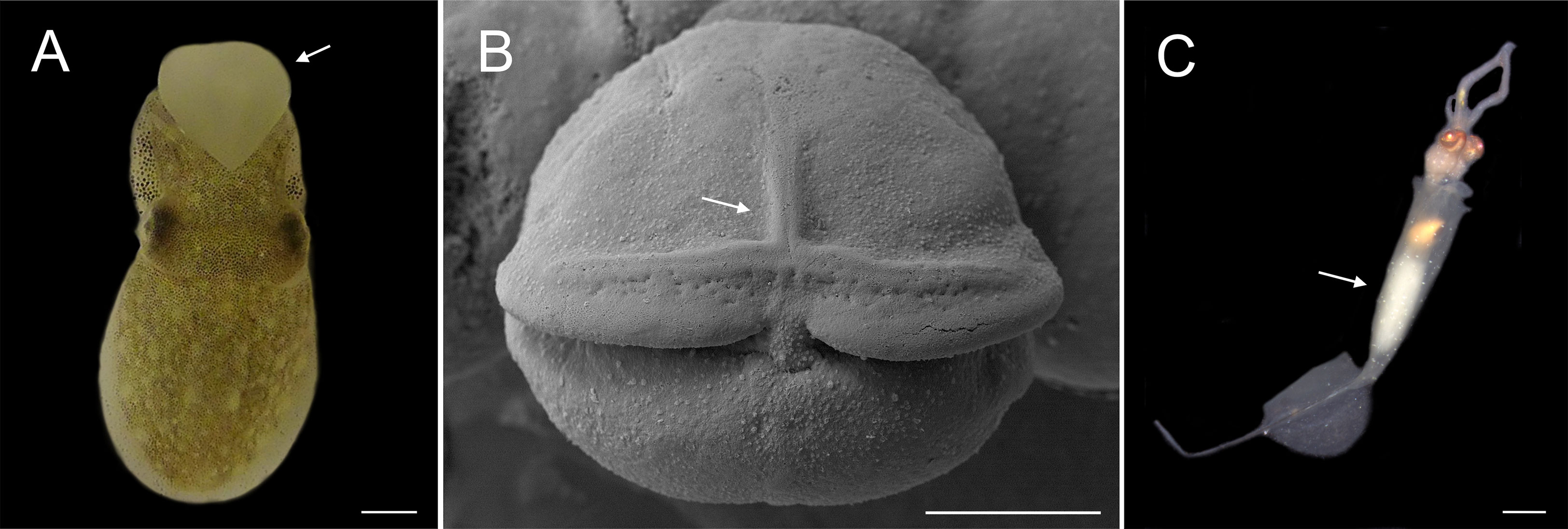
Figure 4 Hatchling stage transitory morphological features. (A) Sepia officinalis premature hatchling with outer yolk sack (arrow), Photo credit: C. O´Brien, scale bar= 1 mm. (B) Sepia officinalis Hoyle organ, anchor-shaped structure on posterior dorsal mantle (arrow), scale bar= 500 µm, Photo credit: N. Cyran. (C) Mastigoteuthid paralarva with inner yolk sack (arrow), scale bar= 1 mm, Photo credit: Danielle Ortiz de Ortiz.
The Hatchling stage may last days, weeks or months (Figure 2B). Sepia officinalis hatchlings go through a major process of reabsorbing the Hoyle organ, which may take up to seven days while yolk reserves are being absorbed (Cyran et al., 2018). For loliginid squid and small-egged Octopus hatchlings, the inner yolk sack can last from a few days to weeks (Vidal et al., 2002; Nande et al., 2017). Juvenile hatchlings from colder and/or deep-waters likely digest yolk for months due to the large size of the inner yolk sack in Grimpoteuthis (Shea et al., 2018) and Graneledone boreopacifica (Voight and Drazen, 2004).
Some cephalopod species go through a Settlement stage, where the paralarva gradually leaves the plankton and adopts a benthic lifestyle. In the larger marine invertebrate literature, this transition is called “settlement” and that terminology has been adopted in cephalopods (Villanueva and Norman, 2008; Roura et al., 2023). This stage has been only documented under laboratory conditions in a few Sepiolida and small-egged Octopodidae species, but it must be common across all taxa that has planktonic paralarvae and benthic juveniles (Figure 2C).
The Settlement stage is the first stage of the Juvenile phase and marked by a gradual transition from the plankton to the benthos that may involve alternating periods in each environment before a fully benthic lifestyle is adopted. For example, in Euprymna hyllebergi, the settlement stage lasts up to 25-30 days (at 28°C and 21-25°C, respectively) during which individuals alternate between the plankton during the day and the benthos at night, before becoming fully benthic (Nabhitabhata and Nishiguchi, 2014) (Table 3). In Octopus sinensis, individuals alternated between clinging on the tank walls or sheltering on the bottom during the day with nocturnal swimming in the water column (Dan et al., 2021). While for O. vulgaris, the settlement stage lasts around 40 to 60 days (at 18-22°C) and no alternating environments between day and night was reported. In this species the settlement stage comprises of three sub-stages each one with particular morphology and behavior (Roura et al., 2023). It was also reported that the transition between environments in Macrotritopus spp. is particularly long and estimated to last from 70 to 140 days (Hanlon et al., 1985). Alternating between the plankton and the benthos during settlement may be an important strategy for finding preferred substrate.
A similar, parallel, stage exists for species that transition from passive plankton drifters to gradually adopt a fully active nektonic lifestyle as juveniles (Roper and Young, 1975). The recognition of this transition to a new life phase is based on development of swimming abilities and strength for individuals to move against surface currents early in life (Bartol et al., 2009; Vidal et al., 2018) for which our understanding is still limited.
Here, we propose the term “Metapelagic stage” to describe the transition from plankton to nekton that has been recognized and described in a few species but unnamed (Sugimoto and Ikeda, 2012; Vidal et al., 2018). The prefix Meta- is Greek and implies a change or shift between two states. Recently, meta- has also been used in self-reference (e.g., metadata is data about data), which also parallels our intent to express that the Metapelagic stage is a transition within the pelagic environment. In species with a Metapelagic stage that form schools (e.g. some loliginid and ommastrephid squids), the transition is easily recognized by advanced swimming control that culminates with the ability to swim in schools (Sugimoto and Ikeda, 2012; Vidal et al., 2018) (Table 3).
The Settlement and the Metapelagic stages are examples of transitional stages based on known life cycles, but still require more studies to be precisely defined in more species. There are several other stages that are common to all cephalopods, including the maturity stages of subadults (Arkhipkin, 1992), and the mating and spawning stages of adults (Puneeta et al., 2015). These stages are discussed elsewhere, and fit easily into this new framework of phases and stages. Clearly there are other stages yet to be described. For example, is the association of argonautoids with gelatinous megaplankton opportunistic, or a long-term association that constitutes a stage (i.e., the Hitchhiking stage)? The description of other intriguing stages of the cephalopod life cycle awaits new research to delineate.
4 Patterns of cephalopod life cycles
Cephalopod life cycles are markedly different from long-lived fish and other marine mollusks. All cephalopods are dioecious, oviparous (except Ocythöe tuberculate and Vitreledonella richardi which are ovoviviparous). Fertilization is generally described as internal, although the details are unknown for most oegopsids (Hoving et al., 2014). Except for Nautilus which can live longer than 20 years (Dunstan et al., 2011a), and some deep-sea and polar species, life spans are short, marked by fast growth, a long early life history, a short adult phase with sexual maturity occurring late in the life cycle, and senescence and death generally, but not always, following reproduction. Cephalopods have flexible reproductive strategies (Rocha et al., 2001; Boyle and Rodhouse, 2005; Ibáñez et al., 2021).
Commercially important shelf species and laboratory reared species have provided the basis for much of what we know about cephalopod life cycles (Boyle, 1983; Boyle and Rodhouse, 2005; Rosa et al., 2013a; Rosa et al., 2013b). These species are well studied, and provide the opportunity for detailed morphological measurements and behavioral observations which is not yet possible for a majority of open ocean species. Oegopsid data must be pieced together from a variety of indirect methods, including stable isotopes and other elemental analyses of beaks and gladii. These methods have been essential in understanding how deep-sea species occupy different water masses or localities throughout their life cycles (e.g., Cherel and Hobson, 2005; Semmens et al., 2007; Zumholz et al., 2007; Lukeneder et al., 2008; Staudinger et al., 2013; Golikov et al., 2018; Golikov et al, 2022b).
Cephalopods exhibit four main life cycle patterns characterized by where each phase of life is lived and whether they have planktonic paralarvae. Life cycles may be fully pelagic, fully benthic or alternating between benthic and pelagic environments (Figure 5). Species with alternating life cycles may hatch as paralarvae or juveniles (Table 4) and the Juvenile, Subadult, and Adult phases may live on bottom, near-bottom or away from the bottom.
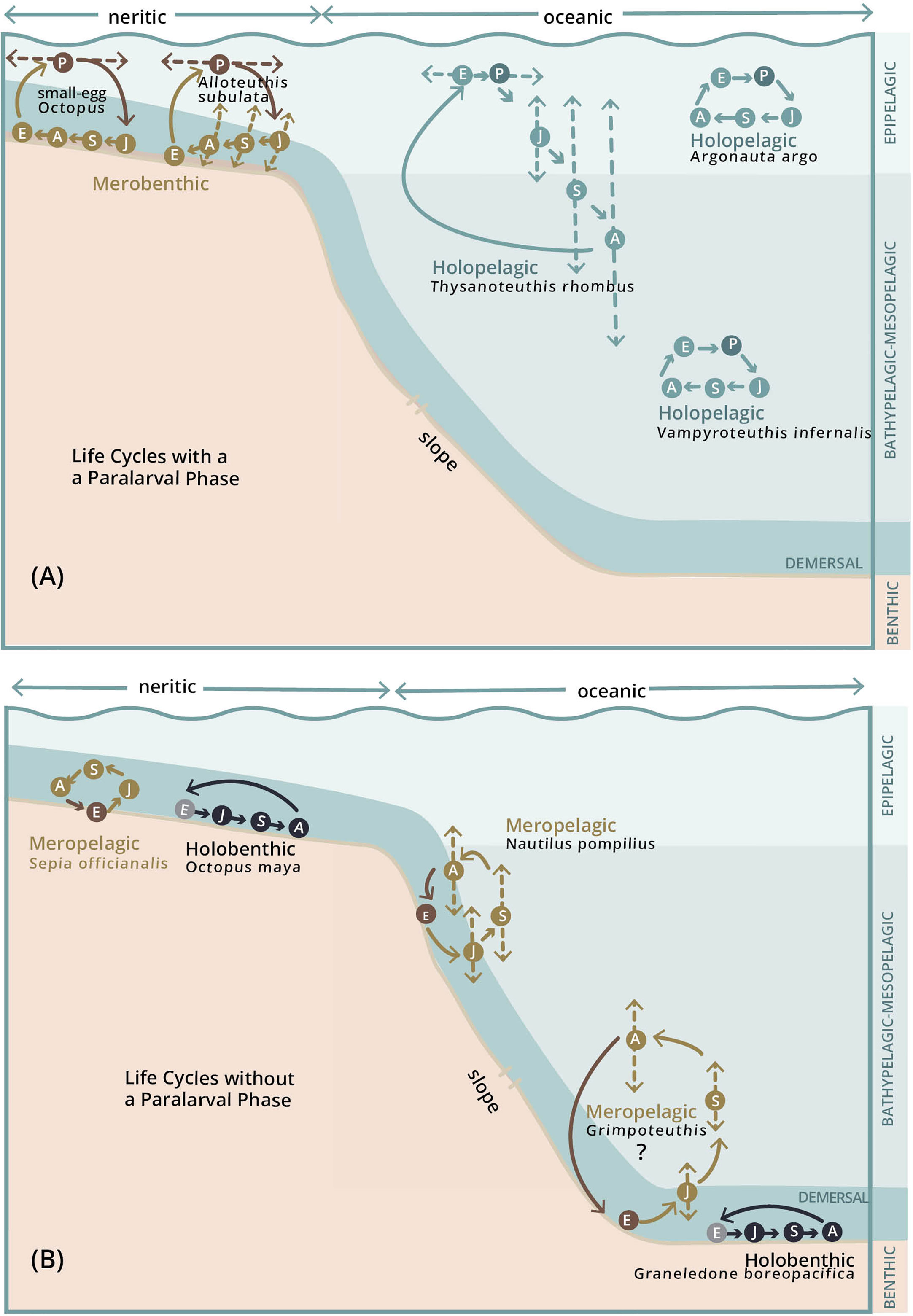
Figure 5 Cephalopod life cycle patterns. All known cephalopod life cycles can be distilled into four major categories: (A) Life cycles with a Paralarval phase. These species are either Merobenthic (brown circles) or Holopelagic (blue-green circles). E, Embryonic; P, Paralarval; J, Juvenile; S, Subadult; A, Adult Phases. Merobenthic species are primarily found on the shelf, and are characterized by having benthic eggs and planktonic paralarvae (P), and JSA phases that live either near bottom or on bottom. Holopelagic species live all phases of their life cycle in the water column, either entirely within a depth horizon, or moving between multiple depth horizons. (B) Life cycles without a Paralarval phase. Species without a Paralarval phase are either Meropelagic (brown circles) or Holobenthic (black circles). Meropelagic life cycles are characterized by having benthic eggs, and JSA that live near-bottom or off-bottom and into the water column. In some of these species, the JSA are highly mobile and may move extensively in the water column as suggested by the dashed arrows. Holobenthic species live all phases of their life cycle on bottom.
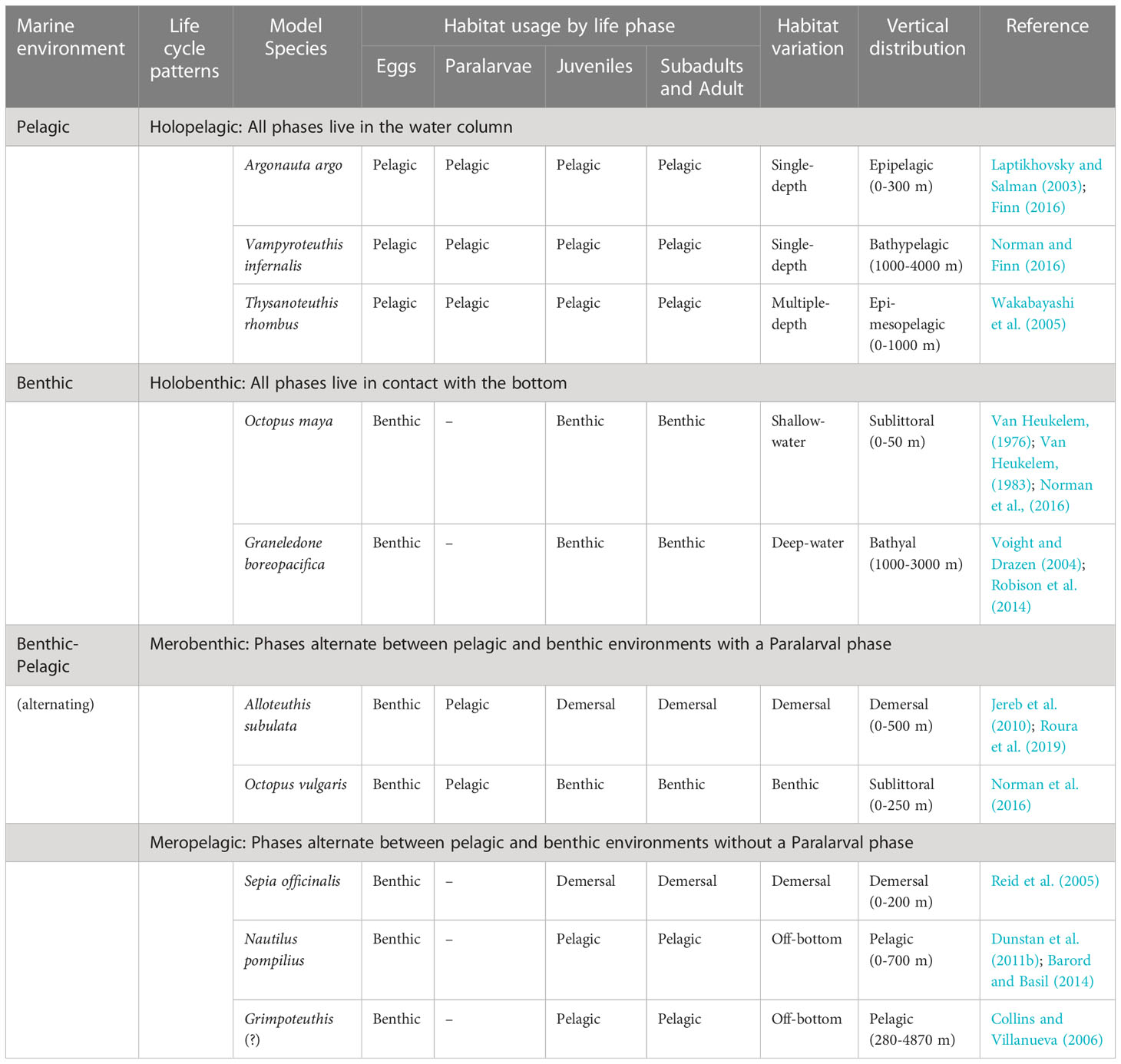
Table 4 Cephalopod life cycle patterns, main variations and habitat usage by each life phase, except for senescent which is widely reported for only a few species.
Each of these four main patterns have variations categorized primarily on the habitat of the adults. These four patterns do not take into account temporary behavioral variations such as bottom associated spawning in many oceanic squids (Nesis, 1995), bottom resting behaviors in adult Illex illecebrosus (Bradbury and Aldrich, 1969; Vecchione and Young, 2018), air-water interface resting behaviors in juvenile Octopus maya (Van Heukelem, 1976), or other responses that may move a benthic animal temporarily off bottom (sepiolids, Bello and Biagi, 1995) or a pelagic animal into the air (Ommastrephidae, Muramatsu et al., 2013). How senescence impacts these life cycles is currently unknown because senescent animals are rarely found, likely due to predation. However, senescent specimens either remain in the same habitat as adults (small-egged octopods), float to the surface (Rodhouse et al., 1987; Xavier et al., 2013) or may sink to the bottom (Roper and Vecchione, 1996; Nesis et al., 1998; Hoving et al., 2017). Based on this and the fact that these habitat changes are not under active control of the organism, the Senescent phase was not treated in Figure 5. A fuller description of how the Senescent phase impacts the cephalopod life cycle awaits new research.
Here, we describe and define four life cycle patterns and their variations. Each variation is explained using the known life cycle of a model species.
4.1 Holopelagic
Definition: A life cycle characterized by all phases and stages living in the water column away from the bottom (Table 4).
All holopelagic species known have a Paralarval phase, although Haliphron eggs are very large (16 mm) (O'Shea, 2004) and juveniles are often caught in epipelagic waters (Hochberg et al., 1992). It is possible that Haliphron atlanticus hatch as juvenile. Some deep-sea species such as the mastigoteuthids, Spirula spirula and Vampyroteuthis infernalis may have mesopelagic paralarvae and juveniles (Clarke, 1969; Clarke, 1970; Clarke and Lu, 1975; Young and Vecchione, 1999) as they are rarely found in the epipelagic plankton. Many holopelagic adult squids have a spawning stage that will occur near the bottom (Nesis, 1995).
Holopelagic species either occupy a single depth horizon (e.g., Argonautidae) or multiple depth horizons over their life cycle (Figure 5A). The multi-depth variation presented below is particularly synoptic and will likely need to be revised as meso- and bathypelagic life cycles become better known. Currently, this category includes species that occupy deeper depth horizons as they grow and develop (e.g., ontogenetic descent), traditional diel vertical migration, vertical spreading and other depth variations (Roper and Young, 1975; Shea and Vecchione, 2010; Judkins and Vecchione, 2020). Vertical migration patterns are typically described for species that move across hundreds or thousands of meters of vertical space (Roper and Young, 1975). Judkins and Vecchione (2020) identify three patterns of vertical migration based on the depths occupied and whether the patterns are synchronous or asynchronous, and provide evidence that some species may undergo an ontogenic “ascent” where larger individuals are found at shallower depths.
Holopelagic - Single depth: This variation refers to species that occupy a single major depth horizon throughout their entire life cycle. For example, Argonauta argo remains in the epipelagic and Vampyroteuthis infernalis remains in the mesopelagic during its entire life cycle. This category includes the non-migrating epipelagic, mesopelagic and bathypelagic diel behaviors identified by Judkins and Vecchione (2020) (Figure 5A).
Argonauta argo is a small epipelagic octopod found worldwide in tropical and subtropical open waters (Finn, 2016). They are holoepipelagic non-migrators (Judkins and Vecchione, 2020) and all life phases are found in the epipelagic zone (Figure 5A) (Table 4). Adult females lay very small eggs (1.5 mm) that are attached to the internal axis of the brood case, and are brooded until hatching. There may be up to 86,000 eggs (Laptikhovsky and Salman, 2003) with up to five different developmental stages developing simultaneously (Finn, 2016). Planktonic paralarvae hatch at about 0.7- 1.0 mm ML (Hochberg et al., 1992). Juvenile females and adult males are found 0 – 300 m. Adult females begin to brood eggs at smaller sizes and continue feeding, growing and producing eggs as they age and are found at the surface during the day, dusk, and night (Laptikhovsky and Salman, 2003; Finn, 2016). Senescent individuals have not been described.
Holopelagic - Multi-depth: This variation includes species with life phases that occur in two or more different depth horizons. Most commonly, this category includes species that have epipelagic paralarvae that gradually move into the meso- or bathypelagic waters with ontogeny. These species may move through multiple pelagic depth horizons every day (Figure 5A).
Thysanoteuthis rhombus is a large oegopsid squid found worldwide in tropical and subtropical open waters and has a 1-year life span. Adult females lay large (up to 2m long), pelagic, cylindrical egg masses that drift in the open surface waters and contain 32,000 to 75,000 developing embryos (Roper and Jereb, 2010). Planktonic paralarvae hatch at about 1.5 mm ML. Paralarvae and juveniles are commonly found in the upper 50 -100 m of the epipelagic zone (Figure 5A) (Table 4). The Juvenile phase begins at about 15 mm ML when the body shape is similar to the adult, but arms are proportionally much longer than in the adult (Wakabayashi et al., 2005). Late juveniles form pairs at approximately 100 mm ML, and probably remain together during their lifetimes. Subadults and adults are found at 600 – 800 m during the day, and 0-50 m at night indicating an extensive diel vertical migration (Roper and Jereb, 2010).
4.2 Holobenthic
Definition: A life cycle characterized by all phases and stages living in contact with the bottom (Table 4).
In holobenthic species, benthic eggs hatch to benthic juveniles which grow into benthic adults. There is no Paralarval phase and consequently these species have more restricted distributions. All phases and stages occupy the same water masses, and consequently invading new spaces is difficult (Figure 5B). The benthic eggs may be laid directly on the substrate, or on other biotic substrate such as corals and sponges. Two depth variations are named according to water depth which roughly correlates with temperature and impacts development time and the relative robustness of the hatchlings.
Holobenthic - Shallow-water: This variation is generally found in species that occur in shallow shelf waters, including tropical coral reefs.
Octopus maya is a large, muscular octopus species that is endemic to the Gulf of Mexico. Adult females lay festoons of large benthic eggs (11-17 mm) in 2-5 m water (Van Heukelem, 1976), then enter senescence. Juvenile hatchlings (7.0 mm ML) immediately adopt a benthic life style. Maximum size is reached at about 8.5 months, and the total life cycle is 1-2 years (Van Heukelem, 1976; Van Heukelem, 1983; Norman et al., 2016) (Figure 5B) (Table 4).
Holobenthic - Deep-water: This variation is generally found in species that occur in deep-water, where water temperature is very cold and the environment is very stable. Although here it is illustrated with a deep-sea benthic species, shallow polar areas may have similar life cycle patterns.
Graneledone boreopacifica is a boreal deep-sea benthic species found in the North Pacific. Adult females lay small batches of large eggs and brood them for about 4 years at depth of 1000-2000 m, while they are in their Senescent phase of life (Robison et al., 2014). Premature hatchlings are very large, 23 – 30 mm ML and 55 mm in total length (Voight and Drazen, 2004). Males and females could be distinguished by examination of internal sex organs at hatching. Males had a hectocotylus with a full complement of suckers, suggesting that this species may hatch as a subadult (Voight and Drazen, 2004). Adults are epibenthic on muddy to rocky bottoms (Jorgensen, 2009) and total life span is expected to last several years (Figure 5B) (Table 4).
4.3 Merobenthic
Definition: A life cycle that alternates between pelagic and benthic environments. Merobenthic species have benthic eggs that hatch as planktonic paralarvae, then settle back to the benthos or near the bottom to live their Juvenile, Subadult and Adult phases (Table 4).
There are many synonyms for this life cycle pattern in the literature including merobenthic (e.g. Boletzky, 1992; Doubleday et al., 2011; Villanueva et al., 2016), pelago-benthic (e.g. Page, 2009; Nielsen, 2013; Ibáñez et al., 2014) and benthopelagic (Bhaud and Duchêne, 1996; Nixon and Mangold, 1998). Merobenthic is adopted here because it has been used in previous research on octopods and avoids the use of a term that has a well-known meaning when used to describe adult habitats. The prefix “Mero” from Greek means “partial”, and benthic refers to the adult habitat, thus Merobenthic is a life cycle that is partially benthic. Merobenthic species are mainly known to inhabit the continental shelf and upper slope.
Merobenthic - Demersal: Alloteuthis subulata is found in shallow, coastal waters associated with sandy and muddy bottoms (Figure 5A, Table 4). Adults may form dense aggregations and undergo seasonal migrations (Jereb et al., 2010). Adults mature at a wide size range, but 50% are mature between 70-80 mm ML. Mature females lay small eggs in balloon-shaped capsules that are attached to hard benthic substrates. Embryonic phase lasts 2-3 weeks. Hatchlings are planktonic and emerge at 1.0 – 2.2 mm ML. Paralarvae have coastal dispersal pattern and live in the plankton for about two months before settling into a demersal habitat (Roura et al., 2019). Estimated life span ranges from 6 months to a year.
Merobenthic - Benthic: Octopus vulgaris is a sublittoral species, living on rocky, sandy, or muddy bottoms of the Mediterranean Sea and central and north-east Atlantic Ocean, typically in < 100 m. Females lay strings of 100-500K small eggs and attach them to the roof of a sheltered den (Figure 5A) (Table 4). Brooding can take from 1.5 to 5 months depending on water temperature (Iglesias et al., 2004). Hatchlings emerge from the benthic eggs at 1-2 mm ML then swim to the surface waters where they live as planktonic paralarvae. Paralarvae spatio-temporal distribution is strongly associated with upwelling events; and paralarvae display a coastal-oceanic dispersal patterns being carried out to oceanic waters and returning to the shelf close to settlement to the benthos (Roura et al., 2019). In a laboratory setting, the Paralarval phase lasts about 40-60 days (Villanueva, 1995; Iglesias et al., 2007) (Table 3). The Juvenile phase begins with the Settlement stage around day 45 after hatching and lasts until day 90 (at 18-20°C) (Roura et al., 2023). At the end of Settlement stage, juveniles have bodies with sculptural components, horizontal pupils, > 35 suckers per arm and are capable of camouflage. Adults have been reported 0 – 250 m; the total life cycle last about 11.5 – 24 months (Iglesias et al., 2004; Norman et al., 2016). Females go through a conspicuous Senescent phase while brooding the eggs for weeks or months, with loss of muscle mass and considerable morpho-physiological deterioration (Roumbedakis and Guerra, 2019) (see Section 3.6)
4.4 Meropelagic
Definition: A life cycle that alternates between pelagic and benthic environments. Meropelagic species hatch as benthic juveniles and move off bottom to become demersal or pelagic juveniles, subadults, and adults (Table 4).
We coin the term Meropelagic as a parallel term to Merobenthic. “Mero” means “partial”, and pelagic refers to the adult habitat, thus Merobenthic is a life cycle partially pelagic or demersal. Meropelagic species lay large, benthic eggs that hatch as juveniles. Because there is no Paralarval phase, these species often have a narrow geographic distribution, although cirrate distribution may be broad (Collins and Villanueva, 2006). In all cases, juveniles, subadults and adults can move away from the bottom and into the water column (Figure 5B).
The distance that these taxa live away from the bottom as an adult distinguishes the different variations. The off-bottom category is very broad and can include any species that has a benthic egg, juvenile hatchlings but that moves up into the water column.
Meropelagic - Demersal: Sepia officinalis is a neritic demersal species found in the Eastern Atlantic and Mediterranean Sea from subtidal waters to up to 200 m (Reid et al., 2005). In the spring and summer, the species is found inshore; in the fall and winter individuals migrate out to the shelf (Figure 5B) (Table 4). Adult females carry between 150 – 4000 eggs depending on their size. Spawning occurs in shallow waters (13 – 15°C). Eggs are 8-10 mm in diameter and attached to benthic substrates. Juvenile hatchlings emerge at 6 – 8 mm ML after 30 – 90 days of development. The juveniles immediately adopt a benthic life style and live in 50 – 80 m of water, including burying behaviors. Juveniles, subadults and adults are demersal, from 0 – 200 m. The life span is one to two years (Reid et al., 2005).
Meropelagic - Off-bottom: In these species, the adults lay benthic eggs, but the Juvenile, Subadult, and Adult phases occupy pelagic spaces and have a pelagic mode of life that is not dependent on the bottom (e.g., swimming is the primary form of movement). This variation encapsulates many taxa whose life cycle phases are poorly known.
Like other extant nautilids, Nautilus pompilius lives on the coral fore reef. Nautilids are slow growing, reaching maturity at 12 – 15 years (Landman et al., 2010). Adult females lay single, encapsulated eggs and attach them to hard structures, likely in shallow waters, 80 – 100 m (Jereb, 2005). Nautilids do not die post-breeding (Saunders, 1984). Rearing studies show that embryos develop for 14 months and hatch as juveniles (Okubo et al., 1995; Uchiyama and Tanabe, 1996). Juveniles, subadults and adults are mobile and occupy similar habitats over reef slopes (Dunstan et al., 2011b) (Figure 5B) (Table 4). It was suggested that adult nautilids have limited dispersal ability, because 200 km of water deeper than 800 m is sufficient to prevent gene flow (Barord et al., 2023).
Cirrates lay single, large, encapsulated eggs in and on deep sea habitats and structures including corals and sponges (Collins and Villanueva, 2006; Boletzky, 2012; Vecchione, 2019). Other information on life cycles is generally lacking and identification is often difficult (Boletzky, 1978-79; Ziegler et al., 2021). Opisthoteuthid cirrates are benthic throughout their life cycle, but other cirrate adults may be collected in deep water pelagic tows. Juvenile hatchlings of Grimpoteuthis are morphologically similar to the adults with large functional fins and a large internal yolk reserves (Shea et al., 2018), which suggests a long juvenile Hatchling stage. Two small cirrate hatchlings of Grimpoteuthis wulkeri (9 and 11 mm ML) were collected between 1489 and 1997 m depth (i.e., 655 – 147 m off-bottom) (Vecchione et al., 2010), suggesting that after eclosion, they could move up into the water column. Four juvenile cirrates (13-15 mm ML) without an inner yolk sack were collected in deep waters off West Greenland (Golikov et al., 2022a). Because adult cirrates have been collected and observed in the midwater with fins that make them highly mobile, we classify their life cycle here as Meropelagic-Off-bottom. However, the degree to which juveniles, subadults and adults are associated with the benthos or the meso – and bathypelagic water still is an open question (Figure 5B) (Table 4).
5 Discussion
We have provided explicit definitions of cephalopods life phases and stages based on established criteria and in line with the vast marine invertebrates literature (McEdward, 1995; Carrier et al., 2018). Definitions can frame how meaningful problems are conceptualized and how results are interpreted. It is important to build consensus towards a standard terminology and a clear conceptual foundation for analyzing the diversity of developmental patterns and the essence of cephalopod life cycles.
The proposed definitions rest on the foundation that cephalopods are direct developers without a true, metamorphic larva as in other mollusks. At the time of eclosion, the cephalopod body plan exists, and is maintained during ontogeny. We delineate the phases and two stages of the life cycle using morphological criteria. The phases are based on major milestones such as having all morphological features that define a species, whereas the stages represent smaller, incremental morphological changes. Some phases and stages are common to all taxa (e.g., Embryonic phase and stages, Hatchling stage, Adult phase), but others are specific to a subset of taxa (e.g., Paralarval phase, Settlement stage). The time elapse of each phase in the life cycle is variable, likely sex related, strongly dependent on temperature and taxon and species-specific in cephalopods.
Different phases of the life cycle utilize different resources and occupy separate habitats. Physical and biological processes and their stressors impact each phase differently, meaning there are phase-specific growth and survival rates that combined control the duration of each phase, and the probability that an individual will successfully transition to the next phase (Morgan, 1995; Boyle and Rodhouse, 2005; Byrne et al., 2018)
It is critical that phases and stages be clearly delimited to understand how susceptibility to these processes and their stressors vary across the life cycle. Each phase represents an optimal adaptation, varying with body size, for acquiring resources for survival and growth under different ecological conditions (Love and Strathmann, 2018). Successful completion of each phase contributes to the overall balance between population growth and mortality, depending on the vulnerability to intrinsic and extrinsic factors. Phases or stages that are more susceptible to mortality are ‘weak links’ in the overall connectivity of life cycles phases (Boyle and Rodhouse, 2005).
In theory, if one phase confers a competitive advantage, selection should favor expanding that phase. Conversely, if a phase results in excessive mortality, selection should favor reducing or eliminating that phase. The absence of a phase can be observed in species without a Paralarval phase (Holobenthic and Meropelagic cycles) or without the Juvenile phase (chiroteuthids). Expansion of a phase can be found in species with exceptionally long Embryonic and Senescent phases (e.g., Graneledone boreopacifica).
The early life, particularly the embryonic and larval phases of marine invertebrates and fish, are negatively affected by extrinsic stressors and generally have a narrow thermal window when compared to older stages (e.g. Pörtner and Farrell, 2008; Pandori and Sorte, 2019; Onthank et al., 2021). This seems also true for cephalopods (e.g. Rosa et al., 2012; Zakroff et al., 2019), substantiating the notion that the Paralarval phase is a period of intense mortality. In addition to the thermal stressors, predation, starvation and advection should negatively impact paralarval mortality. This impact is inferred by the very large number of eggs produced in species with a Paralarval phase, including most commercially important species (Calow, 1987; Boyle and Rodhouse, 2005). Nevertheless, estimates of mortality due to predation, starvation and advection are scarce (Okutani and Watanabe, 1983; Bigelow, 1992; Roberts and van den Berg, 2002; Vidal et al., 2006; González et al., 2010). Even less is known about the factors impacting survival of the juveniles in Holobenthic, Merobenthic and Meropelagic species. Estimates of early mortality require accurate identification of early stages, accurate measures of their distribution, abundance and growth rates, as well as their main predators and prey. These topics are currently unexamined, and represent essential future studies.
Different stressors may impact the survivorship of particular stages within a phase. The Hatchling stage and the Settlement stage have been associated with intense mortality in laboratory studies due to nutritional transitions, from lecithotrophy to planktotrophy in hatchlings, and from planktotrophy to benthic prey in settlers. These stages represent weak links in the life cycle (Vidal et al., 2014; Roura et al., 2023). Identifying these weak links is particularly important for proper management for commercial species (Rodhouse et al., 2014) and increasingly necessary for recognition of how pollution and climate change will impact species. Climate change may cause cephalopods to hatch out smaller, grow faster, mature younger and at a smaller size with shorter life spans (Pecl and Jackson, 2008). Evidence also shows that warming oceans have increased the overall number of cephalopod landings (Doubleday et al., 2016). Cephalopods are notable for their potential for quick population-level responses to environmental change (Seibel, 2007; Fuchs et al., 2020). Will cephalopod grow even faster, shortening life phases and size at maturity under warming conditions? Having a baseline understanding of cephalopod life cycles in a broadly-accepted framework will facilitate our understanding of how they respond to rapid change.
Based on stable definitions of life cycle phases and a survey of the literature, we propose four distinct life cycles patterns: Holopelagic, Merobenthic, Meropelagic and Holobenthic. These patterns were distinguishable based on two main factors: the presence of a Paralarval phase and the degree of association with the bottom. Three of the four patterns have variations identified, and additional variations may be found as we learn more about oegopsid and cirrate life cycles in particular.
Reliance on the benthic environment is strongest in Holobenthic species, but the bottom becomes less essential in the Meropelagic where only the eggs are benthic. We estimate approximately 70% of all cephalopod taxa have an Embryonic phase that takes place either directly on the bottom or attached to benthic structures (Jereb and Roper, 2005; Reid et al., 2005; Jereb and Roper, 2010; Norman et al., 2016). About 30% of all cephalopods are Holopelagic, with an Embryonic phase that is entirely disassociated from the bottom. In Holopelagic species, we find a wide array of adaptations for egg development, from large neutrally buoyant egg masses to the release of single eggs [Brachioteuthis (Young et al., 1985), Enoploteuthidae (Young and Harman, 1985a), the unique production of a chamber for brooding eggs (Argonauthidae) and brooding (e.g. Gonatus onyx (Seibel et al., 2000)), Japetella diaphana (Schwarz et al., 2020), and Bathyteuthis berryi (Bush et al., 2012)].
Holopelagic and Merobenthic species produce paralarvae (Figure 5A). One of the recognized consequences of planktonic development is dispersal to such an extent that it can influence the distributional range of species (Villanueva et al., 2016), favoring transport into new environments. Paralarvae feeding on the plankton represent a reduction in the maternal sources investments per offspring, as feeding is served in the plankton supporting high growth and survival. The Paralarval phase has these advantages for the life cycle and partially explain why planktotrophic development has persisted in many invertebrate taxa (Levin and Bridges, 1995; Love and Strathmann, 2018). However, advection and turbulence can be efficient in spreading drifting paralarvae and carry them away from suitable habitats increasing mortality. That along with predation and high dependence on environmental conditions for recruitment success to the adult population can result in wide inter-annual fluctuations in abundance (Boyle and Rodhouse, 2005). As expected, the Paralarval phase is increasingly seen as being important in fisheries modelling (Bruggeman et al., 2022).
The absence of a Paralarval phase in the Holobenthic and Meropelagic cycles (Figure 5B) suggests a suppression of its dispersal features during the early life stages and life phases might occupy the same or closer habitats. Juveniles are large and similar to the adults, minimizing early life mortality and accelerating the acquisition of the adult morphology (Boletzky, 2003). Alternatively, hatchling juveniles occupy the same habitat as their parents and may be at a disadvantage if the environment has changed and competition with adults for resources has increased (Iwasa et al., 2022). For these species, spawning and embryonic development depend on the ability to find suitable high-quality benthic environments. The microenvironments that these phases occupy may be warmer and highly variable in shallow water species, or colder and stable in deep water species. In contrast, Holopelagic cycles occur in one ecosystem and species are not substantially faced with the hunt for a suitable environment mitigating the harm of turnover habitat between life cycle phases.
The Merobenthic cycles are quite complex as ontogeny progresses between two different ecosystems. Paralarvae adapted for a planktonic lifestyle go through elaborate morphological and behavioral changes to become bottom-dwelling juveniles and find suitable benthic habitat during the Settlement stage to ensure survival of the subsequent life phases. Meropelagic species also alternate between habitats but have larger eggs that hatch as juveniles. As the animal grows through the Subadult and Adult phases, they tend to move up and off of the bottom. Sepia officianalis is a Meropelagic demersal species that may only move slightly off bottom, whereas deep sea species may make more use of the meso- and bathypelagic water depths
These observations inevitably raise the question of the ancestral life cycle pattern of cephalopods. Two competing hypotheses exist about the ancestral life cycle in all bilaterians, including cephalopod mollusks. The “larva first” hypothesis suggests that ancient bilaterians had a pelagic larva and a benthic adult. The “intercalation” hypothesis suggests that larval stages have evolved as specializations from an ancestral with direct development (Page, 2009). The question is thus, whether the ancestral cephalopod produced paralarvae or juvenile hatchlings.
The early embryology of nautilids and coleoids is similar to other gastropod mollusks (Shigeno et al., 2010) and coleoids evolved from ancestors with external shells (Kröger et al., 2011). Extant nautilids hatch as juveniles. However, some cephalopod research generally supports the paralarva first hypothesis in coleoids (Fuchs et al., 2020). In this scenario, the Paralarval phase was reduced over evolutionary time, and the derived condition has an increased egg size and produces juvenile hatchlings (Laptikhovsky et al., 2017a; Fuchs et al., 2020). Indeed, other evidences suggest that the origin of the Holobenthic life cycle in benthic octopuses has evolved from a Merobenthic ancestral by the elimination of the Paralarval phase from the life cycle (Boletzky, 1992; Ibáñez et al., 2014). Resolving whether a life cycle with a paralarva or benthic juvenile is the ancestral condition of cephalopods requires more extensive information about the early life phases and stages of many species, as well as a highly-resolved phylogeny.
Life cycle information is still lacking for many species. Indeed, our knowledge of life phases and stages of many species has increased rather slowly for oceanic, deep sea and polar species, but surprisingly also for coastal species from remote and under sampled areas such as the costs of Africa, India and Indonesia (Jereb and Roper, 2005; Reid et al., 2005; Jereb and Roper, 2010; Norman et al., 2016).
More life cycle oriented research is clearly required (Lipínski, 1998), particularly on defining life stages within phases for which our understanding represent the next level to be accomplished. The Hatchling stage is certainly common across taxa. Other stages belong to particular life cycles, such as the Settlement stage in Merobenthic species (Roura et al., 2023) or the newly proposed Metapelagic stage. Very little work has been directed towards describing important stages within each life cycle phase. This conclusion is strengthened by the observation that only for a few species have been a recent surge of studies defining and delimiting species-specific size or growth stages within the Paralarval and Juvenile phases of squid and octopods based on morphological, behavioral and ecological data (Wakabayashi et al., 2005; Vidal et al., 2018; Franco-Santos and Vidal, 2020; Dan et al., 2022; Roura et al., 2023). Holopelagic juveniles and subadults from many families are generally poorly known (Vecchione, 1987; Sweeney et al., 1992), but expected to be an important part of the diet of midwater fishes (Staudinger et al., 2013) making them an important but understudied part of the marine food web.
Many other life phases are still virtually unknown because of the difficulty of sampling their environments, small catch numbers due to net avoidance and patchy distributions, and collecting deep-sea species alive (Boyle, 1983; Vecchione, 1987; Sweeney et al., 1992; Hoving et al., 2014). Species that are fisheries targets often have large numbers and extensive information but even still there are holes in the life cycle (e.g., Katugin et al., 2013). Species with the most complete information are generally those that can be reared in the laboratory, and have some concurrent information about their wild population. This combination of factors only occurs in some coastal species of the loliginids and octopods, and increasingly model organisms like Euprymna spp. (Hanlon et al., 1997; Nyholm and McFall-Ngai, 2021; Jolly et al., 2022). Laboratory-based studies likely represents the shortest path for studying ecology and behavior of many life stages of coastal and oceanic cephalopods. Interestingly, many of the species treated in Boyle (1983) are the same species we use as models here, reinforcing the idea that only a few species are well studied, even 40 years later.
There are certainly more life cycles patterns, variations and arrays of amazing new behaviors to discover as we expand our cephalopod knowledge. In recent years, that has been accomplished by the use of Remotely Operated Vehicles to obtain in-situ video recordings from great depths describing fine-scale habitat, coloration, and behaviors never before seen. The first in situ observations of Spirula spirula showed that it swims oriented nearly vertically with its head upward (Lindsay et al., 2020), while magnapinnid squid were observed coiling the arm/tentacle filaments while trailing parallel just above the seafloor (Osterhage et al., 2020). These studies were quite revealing and reinforce the value and potential of such observations for a proper understanding of the ecology, behavior and life cycle of deep-water cephalopods.
Using the proposed framework of phases, stages and life cycle patterns will help us standardize our language and provide the opportunity to apply the results from field-based disciplines to lab-based disciplines in a synergistic way, thus maximizing our communication ability to learn about this diverse, charismatic group of mollusks. Hoping for a unifying ground, we encourage the cephalopod community to use the definitions and terminology proposed in this paper.
Author contributions
EAGV conceived the idea of this paper, conceptualization and design, wrote the original draft of sections 1, 3 and 5, and produced tables and figures. ES conceptualization and design, wrote the original draft of sections 2, 4 and 5, and produced tables and figures. Both authors contributed to manuscript revision, read, and approved the submitted version.
Acknowledgments
We would like to thank Dick Young, Mike Vecchione, Chingis Nigmatullin and Vladimir Laptikhovsky and the two reviewers for their comments and suggestions that greatly improved this paper. We also would like to thank Ryo Mimemizu, Linda Ianniello, Norbert Cyran, Caitlin O´Brien, Danielle Ortiz de Ortiz and Dick Young for providing the superb images included in Figures 3, 4. Our thanks to Heather Judkins for enjoyable discussions on cephalopods early development and life cycles. ES would like to thank Teresa Bonaddio and Jennifer Acord (DelMNS) for their essential work on the illustrations and Chief Scientist Trevor Kenchington (DFO Canada), for organizing cruises where Figure 1D was taken, and EAGV would like to thank Irina D. Ozogovski for her help with some figures, the Multi-User Center for Materials Characterization - CMCM of UTFPR where Figure 3F and 3H were taken and the support from the Brazilian National Research Council (CNPq, grants # 426797/2018-5 and # 316391/2021-2).
Conflict of interest
The authors declare that the research was conducted in the absence of any commercial or financial relationships that could be construed as a potential conflict of interest.
Publisher’s note
All claims expressed in this article are solely those of the authors and do not necessarily represent those of their affiliated organizations, or those of the publisher, the editors and the reviewers. Any product that may be evaluated in this article, or claim that may be made by its manufacturer, is not guaranteed or endorsed by the publisher.
References
Akimushkin I. I. (1963). Cephalopod mollusks of the Sea of soviet Russia. Inst. Okean. Akad. Nauk SSR Moskwa Leningrad.
Allan J. (1945). Planktonic cephalopod larvae from the eastern Australian coast. Rec. Austral. Mus. 21, 317–350. doi: 10.3853/j.0067-1975.21.1945.549
Allcock A. L., Hochberg F. G., Stranks T. N. (2003). Re-evaluation of Graneledone setebos (Cephalopoda: Octopodidae) and allocation to the genus Megaleledone. J. Mar. Biol. Assoc. UK 83, 319–328. doi: 10.1017/S0025315403007148h
Anderson R. C., Wood J. B., Byrne R. A. (2002). Octopus senescence: the beginning of the end. J. Appl. Anim. Welf. Sci. 5, 275–283. doi: 10.1207/S15327604JAWS0504_02
Arkhipkin A. I. (1992). Reproductive system structure, development and function in cephalopods with a new general scale for maturity stages. J. Northwest Atlant. Fish. Sci. 12, 63–74. doi: 10.2960/J.v12.a7
Arnold J. M. (1965). Normal embryonic stages of the squid Loligo pealii (LeSueur). Biol. Bull. 128, 24–30. doi: 10.2307/1539386
Arnold J. M. (1984). “Cephalopods,” in The Mollusca, vol. 7 (Reproduction). Eds. Tompa A. S., Verdonk N. H., van den Biggelaar J. A. M. (London: Academic Press), 419–454.
Barord G. J., Basil J. A. (2014). “Nautilus,” in Cephalopod Culture. Eds. Iglesias J., Fuentes L., Villanueva R. (Dordrecht: Springer), 165–174.
Barord G. J., Combosch D. J., Giribet G., Landman N., Lemer S., Veloso J., et al. (2023). Three new species of Nautilus Linneaus, 1758 (Mollusca, Cephalopoda) from the coral Sea and South Pacific. ZooKeys. 1143, 51–69. doi: 10.3897/zookeys.1143.844273
Bartol I. K., Krueger P. S., Stewart W. J., Thompson J. T. (2009). Pulsed jet dynamics of squid hatchlings at intermediate Reynold's numbers. J. Exp. Biol. 212, 1506–1518. doi: 10.1242/jeb.026948
Bazarini S. N., Crook R. J. (2020). Environmental estrogen exposure disrupts sensory processing and nociceptive plasticity in the cephalopod Euprymna scolopes. J. Exp. Biol. 223 (12), jeb218008. doi: 10.1242/jeb.218008
Bello G., Biagi V. (1995). How benthic are sepiolids? Bull. l’Institut Oceanographique Monaco Numero Special 16, 57–61.
Bhaud M., Duchêne J. C. (1996). Change from planktonic to benthic development: is life cycle evolution an adaptive answer to the constraints of dispersal? Oceanol. Acta 19, 335–346.
Bigelow K. A. (1992). Age and growth in paralarvae of the mesopelagic squid Abralia trigonura based on daily growth increments in statoliths. Mar. Ecol. Progr. Ser. 82, 31–40. doi: 10.3354/meps082031
Bitondo A. (2016). Defining the doratopsis: investigating the endpoint of the paralarval stage in Chiroteuthis calyx (Monterey Bay CA: California State University).
Boletzky S. v. (1971). Mandibules denticulées chez les larves des teuthoidés et des octopodes. C. R. Acad. Sci. Paris Ser. 272, 2904–2906.
Boletzky S. v. (1978-79). Nos connaissances actuelles sur le développement des octopodes. Vie Milieu 28-29, 85–120.
Boletzky S. v. (1986). Encapsulation of cephalopod embryos a search for functional correlations. Am. Malacol. Bull. 4 (2), 217–227.
Boletzky S. v. (1987). “Juvenile behavior,” in Cephalopod life cycles, vol. II. Ed. Boyle P. R. (London: Academic Press), 45–60.
Boletzky S. v. (1992). Evolutionary aspects of development, life style, and reproductive mode in incirrate octopods (Mollusca, Cephalopoda). Rev. Suisse Zool. 99 (4), 755–770. doi: 10.5962/bhl.part.79852
Boletzky S. v. (1997). Developmental constraints and heterochrony: a new look at offspring size in cephalopod molluscs. Geobios. 30, 267–275. doi: 10.1016/S0016-6995(97)80102-7
Boletzky S. v. (1998). “Cephalopod eggs and egg masses,” in Oceanography and marine biology: an annual review (Boca Raton, FL: CRC Press), 349–378.
Boletzky S. v. (2003). Biology of early life stages in cephalopod molluscs. Adv. Mar. Biol. 44, 144–204. doi: 10.1016/S0065-2881(03)44003-0
Boletzky S. v. (2010). The ‘yolk organ’ of cephalopod embryos: on transient functions from crawling substratum to provisional knapsack. Ferrantia. 59, 14–21.
Boletzky S. v. (2012). Hatch-as-hatch-can: tricks of the trade in coleoid hatchlings (Mollusca: Cephalopoda). N. Jb. Geol. Palaontol. Abh. 266, 67–76. doi: 10.1127/0077-7749/2012/0261
Boletzky S. v., Andouche A., Bonnaud-Ponticelli L. (2016). A developmental table of embryogenesis in Sepia officinalis. Vie Milieu. 66, 11–23.
Boletzky S. v., Nishiguchi M., Nabhitabhata J., Nugranad J. (2005). (ed.) Idiosepius: ecology, biology and biogeography of a mini-maximalist. Phuket Mar. Biol. Cent. Res. Bull. 66, 11–22.
Bonnaud-Ponticelli L., Bassaglia Y. (2014). Cephalopod development: what we can learn from differences. OA Biol. 2, 6.
Bradbury H. E., Aldrich F. A. (1969). Observations on locomotion of the short-finned squid, Illex illecebrosus illecebrosus (Lesueur), in captivity. Can. J. Zool. 47, 741–744. doi: 10.1139/z69-128
Braga R., van der Molen S., Rodriguez Y. E., Fernández-Giménez A. V., Battini N., Rosas C., et al. (2022). Morphophysiological responses of Octopus tehuelchus juveniles during the transition period between endogenous and exogenous feeding. Aquaculture 556, 738269. doi: 10.1016/j.aquaculture.2022.738269
Bruggeman J., Jacobs Z. L., Popova E., Sauer W. H., Gornall J. M., Brewin R. J., et al. (2022). The paralarval stage as key to predicting squid catch: hints from a process-based model. Deep Sea Res. Part II Top. Stud. Oceanogr. 202, 105123. doi: 10.1016/j.dsr2.2022.105123
Bush S. L., Hoving H. J., Huffard C. L., Robison B. H., Zeidberg L. D. (2012). Brooding and sperm storage by the deep-sea squid Bathyteuthis berryi (Cephalopoda: Decapodiformes). J. Mar. Biol. Assoc. U.K. 92, 1629–1636. doi: 10.1017/S0025315411002165
Byrne M., Ross P. M., Dworjanyn S. A., Parker L. (2018). “Larval ecology in the face of changing climate – impacts of ocean warming and ocean acidification,” in Evolutionary ecology of marine invertebrate larvae. Eds. Carrier T., Reitzel A., Heyland A. (Oxford: Oxford University Press), 251–272.
Calow P. (1987). “Fact and theory: an overview,” in Cephalopod life cycles, vol. II. Ed. Boyle P. R. (London: Academic Press), 351–366.
Carrasco J. F., Arronte J. C., Rodríguez C. (2006). Paralarval rearing of the common octopus, Octopus vulgaris (Cuvier). Aquac. Res. 37, 1601–1605. doi: 10.1111/j.1365-2109.2006.01594.x
Carrier T. J., Reitzel A. M., Heyland A. (2018). Evolutionary ecology of marine invertebrate larvae (Oxford: Oxford University Press).
Cherel Y., Hobson K. A. (2005). Stable isotopes, beaks and predators: a new tool to study the trophic ecology of cephalopods, including giant and colossal squids. Proc. R. Soc Lond. B Biol. Sci. 272, 1601–1607. doi: 10.1098/rspb.2005.3115
Chia F. S. (1974). Classification and adaptive significance of developmental patterns in marine invertebrates. Thalassia Jugoslav. 10, 121–130.
Chichery M. P., Chichery R. (1992). Behavioural and neurohistological changes in aging Sepia. Brain Res. 574 (1-2), 77–84. doi: 10.1016/0006-8993(92)90802-G
Clarke M. R. (1966). A review of the systematics and ecology of oceanic squids. Adv. Mar. Biol. 4, 91–300. doi: 10.1016/S0065-2881(08)60314-4
Clarke M. R. (1969). Cephalopoda collected on the SOND cruise. J. Mar. Biol. Ass. U.K. 49, 961–976. doi: 10.1017/S0025315400038042
Clarke M. R. (1970). Growth and development of Spirula spirula. J. Mar. Biol. Ass. U. K. 50, 53–64. doi: 10.1017/S002531540000059X
Clarke M. R., Lu C. C. (1975). Vertical distribution of cephalopods at 18°N 25°W in the north Atlantic. J. Mar. Biol. Ass. UK. 55, 65–182. doi: 10.1017/S0025315400015812
Collins M. A., Villanueva R. (2006). “Taxonomy, ecology and behaviour of the cirrate octopods,” in Oceanography and marine biology: an annual review, vol. 44. Eds. Gibson R. N., Atkinson R. J. A., Gordon J. D. M. (Boca Raton, FL: Chapman and Hall/CRC Press), 277–322.
Cyran N., Palumbo A., Klepal W., Vidal E. A. G., Staedler Y., Schönenberger J. (2018). The short life of the Hoyle organ of Sepia officinalis: formation, differentiation and degradation by programmed cell death. Hydrobiologia. 808, 35–55. doi: 10.1007/s10750-017-3291-3
Dan S., Kamei Y., Takeshima S., Yamashita K., Hamasaki K. (2022). Stepwise changes in morphology during the settlement process in a merobenthic octopus, Octopus sinensis, raised in the laboratory. Invertebr. Biol. 141, e12358. doi: 10.1111/ivb.12358
Dan S., Shibasaki S., Takasugi A., Takeshima S., Yamazaki H., Ito A., et al. (2021). Changes in behavioural patterns from swimming to clinging, shelter utilization and prey preference of East Asian common octopus Octopus sinensis during the settlement process under laboratory conditions. J. Exp. Mar. Biol. 539, 151537. doi: 10.1016/j.jembe.2021.151537
Deryckere A., Styfhals R., Vidal E. A. G., Almansa E., Seuntjens E. (2020). A practical staging atlas to study embryonic development of Octopus vulgaris under controlled laboratory conditions. BMC Dev. Biol. 20, 1–18. doi: 10.1186/s12861-020-00212-6
Doubleday Z. A., Prowse T. A., Arkhipkin A., Pierce G. J., Semmens J., Steer M., et al. (2016). Global proliferation of cephalopods. Curr. Biol. 26 (10), R406–R407. doi: 10.1016/j.cub.2016.04.002
Doubleday Z. A., White J., Pecl G. T., Semmens J. M. (2011). Age determination in merobenthic octopuses using stylet increment analysis: assessing future challenges using Macroctopus maorum as a model. ICES J. Mar. Sci. 68, 2059–2063. doi: 10.1093/icesjms/fsr135
Dunstan A. J., Ward P. D., Marshall N. J. (2011a). Nautilus pompilius life history and demographics at the osprey reef seamount, coral Sea, Australia. PloS One 6 (2), e16312. doi: 10.1371/journal.pone.0016312
Dunstan A. J., Ward P. D., Marshall N. J. (2011b). Vertical distribution and migration patterns of Nautilus pompilius. PloS One 6 (2), e16311. doi: 10.1371/journal.pone.0016311
Eckman J. E. (1996). Closing the larval loop: linking larval ecology to the population dynamics of marine benthic invertebrates. J. Exp. Mar. Biol. 200, 207–237. doi: 10.1016/S0022-0981(96)02644-5
Fernández-Gago R., Molist P., Anadón R. (2019). “Tissues of paralarvae and juvenile cephalopods,” in Handbook of pathogens and diseases in cephalopods. Eds. Gestal C., Pascual S., Guerra Á., Fiorito G., Vieites. J. M. (New York: Springer), 207–211. doi: 10.1007/978-3-030-11330-85
Finn J. K. (2016). “Family Argonautidae,” in Cephalopods of the world. an annotated and illustrated catalogue of cephalopod species known to date. volume 3. Octopods and Vampire Squids. Eds. Jereb P., Roper C. F. E., Norman M. D., Finn J. K. (Rome: FAO), 228–237.
Fioroni P. (1982). Larval organs, larvae, metamorphosis and types of development of Mollusca - a comprehensive review. Zool. Jb. Anat. 108, 375–420.
Forsythe J. W., Toll R. B. (1991). Clarification of the western Atlantic ocean pygmy octopus complex: the identity and life history of Octopus joubini (Cephalopoda: octopodi nae). Bull. Mar. Sci. 49 (1-2), 88–97.
Forsythe J. W., Van Heukelem W. F. (1987). “Growth,” in Cephalopod life cycles. vol. II. Ed. Boyle P. R. (London: Academic Press), 135–156.
Forsythe J. W., Walsh L. S., Turk P. E., Lee P. G. (2001). Impact of temperature on juvenile growth and age at first egg-laying of the pacific reef squid Sepioteuthis lessoniana reared in captivity. Mar. Biol. 138, 103–112. doi: 10.1007/s002270000450
Franco-Santos R. M., Vidal E. A. G. (2014). Beak development of early squid paralarvae (Cephalopoda: Teuthoidea) may reflect an adaptation to a specialized feeding mode. Hydrobiologia 725, 85–103. doi: 10.1007/s10750-013-1715-2
Franco-Santos R. M., Vidal E. A. G. (2020). Tied hands: synchronism between beak development and feeding-related morphological changes in ommastrephid squid paralarvae. Hydrobiologia. 847, 1943–1960. doi: 10.1007/s10750-020-04223-z
Fuchs D., Laptikhovsky V., Nikolaeva S., Ippolitov A., Rogov M. (2020). Evolution of reproductive strategies in coleoid mollusks. Paleobiology 46 (1), 82–103. doi: 10.1017/pab.2019.41
García-Flores M., Ceballos-Vázquez B. P., Rosales-Velázquez M. O. (2022). Embryonic development and fecundity of the pacific pygmy octopus, Paroctopus digueti. J. Shellfish Res. 41, 125–134. doi: 10.2983/035.41.0110
Geigy R., Portmann A. (1941). Versuch einer morphologischen ordnung der tierischen entwicklungsgange. Sci. Nat. 29, 734–743. doi: 10.1007/978-3-642-51845-4_79
Golikov A. V., Artemev G. M., Blicher M. E., Gudmundsson G., Jørgensen L. L., Olafsdottir S. H., et al. (2022a). Deep and cold: are Boreal and Arctic finned octopods, Stauroteuthis syrtensis and Cirroteuthis muelleri (Cephalopoda: Octopoda: Cirrata), ecological analogues? Deep Sea Res. Part I: Oceanographic Res. Papers. 181, 103706. doi: 10.1016/j.dsr.2022.103706
Golikov A. V., Ceia F. R., Hoving H. J., Queirós J. P., Sabirov R. M., Blicher M. E., et al. (2022b). Life history of the Arctic squid Gonatus fabricii (Cephalopoda: Oegopsida) reconstructed by analysis of individual ontogenetic stable isotopic trajectories. Animals. 12, 3548. doi: 10.3390/ani12243548
Golikov A. V., Ceia F. R., Sabirov R. M., Zaripova Z. I., Blicher M. E. (2018). Ontogenetic changes in stable isotope (δ13C and δ15N) values in squid Gonatus fabricii (Cephalopoda) reveal its important ecological role in the Arctic. Mar. Ecol. Progr. Ser. 606, 65–78. doi: 10.3354/meps12767
González Á.F., Otero J., Pierce G. J., Guerra A. (2010). Age, growth, and mortality of Loligo vulgaris wild paralarvae: implications for understanding of the life cycle and longevity. ICES J. Mar. Sci. 67, 1119–1127. doi: 10.1093/icesjms/fsq014
Guerra Á. (1993). “Ageing in cephalopods,” in The recent advances in cephalopod fisheries biology (Tokyo: Tokai University Press), 684–687.
Hanlon R. T., Forsythe J. W., Boletzky S. v. (1985). Field and laboratory behavior of "Macrotritopus larvae" reared to Octopus defilippi Verany, 1851 (Mollusca: Cephalopoda). Vie Milieu. 35, 237–242.
Hanlon R. T., Turk P. E., Lee P. G., Yang W. T. (1987). Laboratory rearing of the squid Loligo pealei to the juvenile stage: growth comparisons with fishery data. Fish. Bull. 85 (1), 163–167.
Hanlon R. T., Yang W. T., Turk P. E., Lee P. G., Hixon R. F. (1989). Laboratory culture and estimated life span of the Eastern Atlantic squid, Loligo forbesi Steenstrup, 1856 (Mollusca: Cephalopoda). Aquac. Res. 20 (1), 15–34. doi: 10.1111/j.1365-2109.1989.tb00438.x
Hanlon R. T., Claes M. F., Ashcraft S. E., Dunlap P. V. (1997). Laboratory culture of the sepiolid squid Euprymna scolopes: a model system for bacteria-animal symbiosis. Biol. Bull. 192, 364–374. doi: 10.2307/1542746
Hejnol A., Vellutini B. C. (2017). Larval evolution: I’ll tail you later…. Curr. Biol. 27, R21–R24. doi: 10.1016/j.cub.2016.10.057
Hernandéz-Garcia V., Piatkowski U., Clarke M. R. (1998). Development of the darkening of Todarodes sagittatus beaks and its relation to growth and reproduction. J. Mar. Biol. Assoc. UK 20, 363–373. doi: 10.2989/025776198784126485
Hochberg F. G., Nixon M., Toll R. B. (1992). “Order Octopoda Leach 1818,” in “Larval” and juvenile cephalopods: a manual for their identification. Eds. Sweeney M. J., Roper C. F. E., Mangold K. M., Clarke M. R., Boletzky S. v., 213–280.
Hoving H. J. T., Bush S. L., Haddock S. H. D., Robison B. H. (2017). Bathyal feasting: post-spawning squid as a source of carbon for deep-sea benthic communities. Proc. R. Soc B2 84, 20172096. doi: 10.1098/rspb.2017.2096
Hoving H., Perez J. T., Bolstad J. A. A., Braid K., Evans H., Fuchs A. B., et al. (2014). “The study of deep-sea cephalopods,” in Advances in Marine Biology Academic Press, vol. 67. Ed. Vidal E. A. G. (United Kingdom: Academic Press), 235–259. doi: 10.1016/B978-0-12-800287-2.00003-2
Huan P., Wang Q., Tan S., Liu B. (2020). Dorsoventral decoupling of Hox gene expression underpins the diversification of molluscs. Proc. Natl. Acad. Sci. U.S.A. 117 (1), 503–512. doi: 10.1073/pnas.1907328117
Ibáñez C. M., Díaz-Santana-Iturrios M., López-Córdova D. A., Carrasco S. A., Pardo-Gandarillas M. C., Rocha F., et al. (2021). A phylogenetic approach to understand the evolution of reproduction in coleoid cephalopods. Mol. Phylogenet. Evol. 155. doi: 10.3389/fmars.2021.707825
Ibáñez C. M., Peña F., Pardo-Gandarillas M. C., Méndez M. A., Hernández C. E., Poulin E. (2014). Evolution of development type in benthic octopuses: holobenthic or pelago-benthic ancestor? Hydrobiologia. 725, 205–214. doi: 10.1007/s10750-013-1518-5
Iglesias J., Otero J. J., Moxica C., Fuentes L., Sánchez F. J. (2004). The completed life cycle of the octopus (Octopus vulgaris, Cuvier) under culture conditions: paralarval rearing using artemia and zoeae, and first data on juvenile growth up to 8 months of age. Aquac. Int. 12, 481–487 doi: 10.1023/B:AQUI.0000042142.88449.bc
Iglesias J., Sanchez F. J., Bersano J. F. G., Carrasco J. F., Dhont J., Fuentes L., et al. (2007). Rearing of Octopus vulgaris paralarvae: present status, bottlenecks and trends. Aquaculture. 266, 1–15. doi: 10.1016/j.aquaculture.2007.02.019
Itami K., Izawa Y., Maeda S., Nakai K. (1963). Notes on the laboratory culture of octopus larvae. 29, 514–520
Iwasa Y., Yusa Y., Yamaguchi S. (2022). Evolutionary game of life-cycle types in marine benthic invertebrates: feeding larvae versus nonfeeding larvae versus direct development. J. Theor. Biol. 537, 111019. doi: 10.1016/j.jtbi.2022.111019
Jackson G. D., Mladenov P. D. (1994). Terminal spawning in the deepwater squid Moroteuthis ingens (Cephalopoda: Onychoteuthidae). J. Zool. Lond. 234, 189–201. doi: 10.1111/j.1469-7998.1994.tb06067.x
Jereb P. (2005). “Family Nautilidae,” in Cephalopods of the world. an annotated and illustrated catalogue of cephalopod species known to date. volume 1. Chambered Nautiluses and Sepioids. Eds. Jereb P., Roper C. F. E. (Rome: FAO), 51–55.
Jereb P., Roper C. F. E. (2005). Cephalopods of the World. An annotated and illustrated catalogue of cephalopod species known to date. Volume 1. Chambered Nautiluses and Sepioids (Rome: FAO).
Jereb P., Roper C. F. E. (2010). Cephalopods of the world. an annotated and illustrated catalogue of cephalopod species known to date. volume 2. Myopsid and Oegopsid Squids (Rome: FAO).
Jereb P., Vecchione M., Roper C. F. E. (2010). “Family Loliginidae,” in Cephalopod of the world,” in An annotated and illustrated catalogue of cephalopod species known to date. volume 2. Myosida and Oegopsid Squids. Eds. Jereb P., Roper C. F. E. (Rome: FAO), 38–117.
Jereb P., Roper C. F. E., Norman M. D., Finn J. K. (2016). “Cephalopods of the world. an annotated and illustrated catalogue of cephalopod species known to date. volume 3,” Octopods and Vampire Squids (Rome: FAO).
Johnson W. S., Allen D. M. (2012). Zooplankton of the Atlantic and gulf coasts: a guide to their identification and ecology (Baltimore: The Johns Hopkins University Press).
Jolly J., Hasegawa Y., Sugimoto C., Zhang L., Kawaura R., Sanchez G., et al. (2022). Life cycle, culture, and maintenance of the emerging cephalopod models Euprymna berryi and Euprymna morsei. Front. Mar. Sci. 9. doi: 10.3389/fmars.2022.1039775
Jones N. J., Richardson C. A. (2010). Laboratory culture, growth, and the life cycle of the little cuttlefish Sepiola atlantica (Cephalopoda: sepiolidae). J. Shell. Res. 29 (1), 241–246. doi: 10.2983/035.029.0121
Jorgensen E. M. (2009). Field guide to squids and octopods of the Eastern north pacific and Bering Sea (Alaska: Alaska Sea Grant College Program).
Judkins H., Vecchione M. (2020). Vertical distribution patterns of cephalopods in the northern gulf of Mexico. Front. Mar. Sci. 7. doi: 10.3389/fmars.2020.00047
Katugin O. N., Shevtsov G. A., Zuev M. A., Didenko V. D., Kulik V. V., Vanin N. S. (2013). “Berryteuthis magister, schoolmaster gonate squid,” in Advances in squid biology, ecology and fisheries, part II Oegopsid squids. Eds. Rosa R., Pierce G. J., O’Dor R. (New York: Nova Science Publishers, Inc), 1–48.
Khen A., McCormick L. R., Steinke C. A., Rouse G. W., Zerofski P. J. (2022). First known observations of brooding, development, and hatching of fertilized eggs for the north pacific bigeye, Octopus californicus. Ecol. Evol. 12 (11), p.e9481. doi: 10.1002/ece3.9481
Kier W. M. (1996). Muscle development in squid: the ultrastructural differentiation of a specialized muscle type. J. Morphol. 229, 271–288.
Kingston A. C., Wardill T. J., Hanlon R. T., Cronin T. W. (2015). An unexpected diversity of photoreceptor classes in the longfin squid, Doryteuthis pealeii. PloS One 10 (9), e0135381. doi: 10.1371/journal.pone.0135381
Kröger B., Vinther J., Fuchs D. (2011). Cephalopod origin and evolution: a congruent picture emerging from fossils, development and molecules: extant cephalopods are younger than previously realised and were under major selection to become agile, shell-less predators. Bioessays 33 (8), 602–613. doi: 10.1002/bies.201100001
Kubodera T., Okutani T. (1977). Description of a new species of gonatid squid, Gonatus madokai, n. sp., from the Northwest pacific, with notes on morphological changes with growth and distribution in immature stages (Cephalopoda: Oegopsida). Jap. J. Malacol. (Venus) 36, 123–151.
Landman N. H., Cochran J. K., Saunders W. B. (2010). “Growth and longevity of Nautilus,” in Nautilus: biology and paleobiology of a living fossil. Eds. Saunders W. B., Landman N. H. (New York: Plenum Press), 401–420.
Laptikhovsky V., Nigmatullin C. (1992). Caracteristicas reproductivas de machos y hembras del calamar (Illex argentinus). Frente Maritimo 12, 23–37.
Laptikhovsky V., Nikolaeva S., Rogov M. (2017a). Cephalopod embryonic shells as a tool to reconstruct reproductive strategies in extinct taxa. Biol. Rev. 93, 270–283. doi: 10.1111/brv.12341
Laptikhovsky V., Boersch-Supan P., Bolstad K., Kemp K., Letessier T. (2017b). Cephalopods of the southwest Indian ocean ridge: a hotspot of biological diversity and absence of endemism. Deep-Sea Res. Part II: Topical Stud. Ocean. 136, 98–107. doi: 10.1016/j.dsr2.2015.07.002
Laptikhovsky V., Salman A. (2003). On reproductive strategies of the epipelagic octopods of the superfamily Argonautoidea (Cephalopoda: Octopoda). Mar. Biol. 142, 321–326. doi: 10.1007/s00227-002-0959-6
Laptikhovsky V. V., Fock H., Piatkowski U., Schwarz R., Hoving H. J. T. (2019). Reproductive strategies of deep-sea squid (Mastigoteuthidae, Chiroteuthidae, Batoteuthidae and Cranchiidae). Mar. Biol. 166, 85. doi: 10.1007/s00227-019-3532-2
Lee P. N., Callaerts P., de Couet H. G., Martindale M. Q. (2003). Cephalopod hox genes and the origin of morphological novelties. Nature 424, 1061–1065. doi: 10.1038/nature01872
Lemaire J. (1970). Table de développement embryonnaire de Sepia officinalis l. (Mollusque céphalopode). Bull. Soc Zool. Fr. 95, 773–782.
Levin L. A., Bridges T. S. (1995). “Pattern and diversity in reproduction and development,” in Ecology of marine invertebrate larvae. Ed. McEdward L. R. (Boca Raton, FL: CRC Press), 1–48.
Lindsay D. J., Hunt J. C., McNeil M., Beaman R. J., Vecchione M. (2020). The first in situ observation of the ram’s horn squid Spirula spirula turns “common knowledge” upside down. Diversity 12, 449. doi: 10.3390/d12120449
Lipínski M. (1979). “Universal maturity scale for the commercially important squids (Cephalopoda: Teuthoidea),” in The results of maturity classification of Illex illecebrosus (Lesueur 1821) population for years 1973–1977 ICNAF. res. doc. 79/38, 1–20.
Lipínski M. (1998). Cephalopod life cycles: patterns and exceptions. S. Afr. J. Mar. Sci. 20, 439–447. doi: 10.2989/025776198784126205
Love A. C., Strathmann R. R. (2018). “Marine invertebrate larvae: model life histories for development, ecology, and evolution,” in Evolutionary ecology of marine invertebrate larvae. Eds. Carrier T., Reitzel A., Heyland A. (Oxford: Oxford University Press), 306–321.
Lukeneder A., Harzhauser M., Müllegger S., Piller W. E. (2008). Stable isotopes (δ 18O and δ 13C) in Spirula spirula shells from three major oceans indicate developmental changes paralleling depth distributions. Mar. Biol. 154, 175–182. doi: 10.1007/s00227-008-0911-5
Mangold K. (1987). “Reproduction,” in Cephalopod life cycles, vol. II. Ed. Boyle P. R. (London: Academic Press), 157–200.
Mangold-Wirz K. (1963). Biologie des céphalopodes benthiques et nectoniques de la mer cata-lane. Vie Milieu. Suppl. 13, 1–285.
McCormick L. R., Levin L. A., Oesch N. W. (2022). Reduced oxygen impairs photobehavior in marine invertebrate larvae. Biol. Bull. 243, 255–271. doi: 10.1086/717565
McEdward L. R. (2000). Adaptive evolution of larvae and life cycles. Cell Dev. Biol. 11, 403–409. doi: 10.1006/scdb.2000.0193
McEdward L. R., Janies D. A. (1993). Life cycle evolution in asteroids: what is a larva? Biol. Bull. 84, 255–268. doi: 10.2307/1542444
Messenger J. B. (1977). Prey-capture and learning in the cuttlefish, Sepia. Symp. Zoological Soc. London 38, 347–376.
Moran N. A. (1994). Adaptation and constraint in the complex life cycles of animals. Annu. Rev. Ecol. Evol. Syst. 25, 573–600. doi: 10.1146/annurev.es.25.110194.003041
Morgan S. G. (1995). “Life and death in the plankton: larval mortality and adaptation,” in Ecology of marine invertebrate larvae. Ed. McEdward L. R. (Boca Raton, FL: CRC Press), 279–321.
Muramatsu K., Yamamoto J., Abe T., Sekiguchi K., Hoshi N., Sakurai Y. (2013). Oceanic squid do fly. Mar. Biol. 160, 1171–1175. doi: 10.1007/s00227-013-2169-9
Nabhitabhata J. (1997). Life cycle of three cultured generations of spineless cuttlefish, Sepiella inermis (Férussac and D’Orbigny). Phuket Mar. Biol. Cent. Res. Bull. Special Publ. 17 (1), 289–298.
Nabhitabhata J. (2014). “Amphioctopus aegina,” in Cephalopod Culture. eds. Iglesias J., Fuentes L., Villanueva R. (Dordrecht: Springer), 249–363.
Nabhitabhata J., Nishiguchi M. K. (2014). “Euprymna hyllebergi and Euprymna tasmanica” in Cephalopod Culture” eds. Iglesias J., Fuentes L., Villanueva R. (Dordrecht: Springer), 253–269.
Naef A. (1928). Die cephalopoden (Embryologie). fauna e flora del golfo di Napoli 35(I-2), 1-363 [English translation) (Washington, D.C., 20560, U.S.A: Smithsonian Institution Libraries).
Nande M., Iglesias J., Domingues P., Pérez M. (2017). Effect of temperature on energetic demands during the last stages of embryonic development and early life of Octopus vulgaris (Cuvier, 1797) paralarvae. Aquacult. Res. 48, 1951–1961. doi: 10.1111/are.13032
Nesis K. N. (1995). Mating, spawning, and death in oceanic cephalopods, a review. Ruthenica. 6, 23–64.
Nesis K. N. (1999). “Cephalopoda,” in South Atlantic zooplankton Vol.1. Ed. Boltovskoy D. (Leiden: Bakhuys), 707–795.
Nesis K. N. (2002). Life style strategies of recent cephalopods: a review. Bull. Mar. Sci. 71, 561–579.
Nesis K. N., Nigmatullin C. M., Nikitina I. V. (1998). Spent females of deepwater squid Galiteuthis glacialis under the ice at the surface of the Weddel Sea (Antarctic). J. Zool. 244, 185–200. doi: 10.1111/j.1469-7998.1998.tb00024.x
Newell G. E., Newell R. C. (1977). Marine plankton: a practical guide. 5th Edition (London: Hutchinson Educational).
Nielsen C. (2013). Life cycle evolution: was the eumetazoan ancestor a holopelagic, planktotrophic gastraea? BMC Evol. Biol. 13, 1–18. doi: 10.1186/1471-2148-13-171
Nielsen C. (2018). Origin of the trochophora larva. R. Soc Open Sci. 5, 180042. doi: 10.1098/rsos.180042
Nigmatullin C. M., Arkhipkin A. I. (1998). “A review of the biology of the diamondback squid, Thysanoteuthis rhombus (Oegopsida: Thysanoteuthidae),” in Large Pelagic squids. Ed. Okutani T. (Tokyo: Japan Marine Fishery Resources Research Center), 156–181.
Nixon M., Mangold K. (1998). The early life of Octopus vulgaris (Cephalopoda: Octopodidae) in the plankton and at settlement: a change in lifestyle. J. Zool. 239, 301–327. doi: 10.1111/j.1469-7998.1996.tb05453.x
Norman M. D., Finn J. K. (2016). “Family Vampyroteuthidae”, in Cephalopods of the world. an annotated and illustrated catalogue of cephalopod species known to date. Volume 3. Octopods and Vampire Squids, Eds. Jereb P., Roper C. F. E., Norman M. D., Finn J. K. (Rome: FAO), 268–270.
Norman M. D., Finn J. K., Hochberg F. G. (2016). “Family Octopodidae,” in Cephalopods of the world. an annotated and illustrated catalogue of cephalopod species known to date. Volume 3. Octopds and Vampire Squids. Eds. Jereb P., Roper C. F. E., Norman M. D., Finn J. K. (Rome: FAO) 3, 36–215.
Nyholm S. V., McFall-Ngai M. J. (2021). A lasting symbiosis: how the Hawaiian bobtail squid finds and keeps its bioluminescent bacterial partner. Nat. Rev. Microbiol. 19, 666–679. doi: 10.1038/s41579-021-00567-y
O'Shea (2004). The giant octopus Haliphron atlanticus (Mollusca: Octopoda) in new Zealand waters. N. Z. J. Zool 31, 7–13. doi: 10.1080/03014223.2004.9518353
O’Dor R. K., Wells M. J. (1978). Reproduction versus somatic growth, hormonal control in Octopus vulgaris. J. Exp. Biol. 77, 15–31. doi: 10.1242/jeb.77.1.15
Okubo S. (1979). Spawning and rearing of hatchlings of the giant pacific octopus. Anim. Nature 9, 2–6.
Okubo S., Tsujii T., Watabe N., Williams D. F. (1995). Hatching of Nautilus belauensis Saunders, 1981, in captivity - culture, growth and stable-isotope compositions of shells, and histology and immunohistochemistry of the mantle epithelium of the juveniles. Veliger 38, 192–202.
Okutani T. (1987). “Juvenile morphology,” in Cephalopod life cycles, volume II. Ed. Boyle P. R. (London: Academic Press), 33–44.
Okutani T., McGowan J. (1969). Systematics, distribution, and abundance of the epiplanktonic squid (Cephalopoda, Decapoda) larvae of the California Current, April, 1954 – March, 1957. Bull. Scripps Inst. Oceanogr. Univ. Calif 14, 1–90.
Okutani T., Watanabe T. (1983). Stock assessment by larval surveys of the winter population of Todarodes pacificus Steenstrup (Cephalopoda: Ommastrephidae), with a review of early works. Biol. Oceanogr. 2 (2-4), 401–431.
Onthank K. L., Trueblood L. A., Schrock-Duff T., Kore L. G. (2021). Impact of short-and long-term exposure to elevated seawater PCO2 on metabolic rate and hypoxia tolerance in Octopus rubenscens. Physiol. Biochem. Zool. 94, 1–11. doi: 10.1086/712207
Osterhage D., MacIntosh H., Althaus F., Ross A. (2020). Multiple observations of bigfin squid (Magnapinna sp.) in the great Australian bight reveal distribution patterns, morphological characteristics, and rarely seen behaviour. PloS One 15 (11), e0241066. doi: 10.1371/journal.pone.0241066
Page L. R. (2009). Molluscan larvae: pelagic juveniles or slowly metamorphosing larvae? Biol. Bull. 216, 216–225. doi: 10.1086/BBLv216n3p216
Pandori L. L., Sorte C. J. (2019). The weakest link: sensitivity to climate extremes across life stages of marine invertebrates. Oikos. 128, 621–629. doi: 10.1111/oik.05886
Pecl G. T., Jackson G. D. (2008). The potential impacts of climate change on inshore squid: biology, ecology and fisheries. Rev. Fish. Biol. Fish. 18, 373–385
Pélabon C., Firmat C., Bolstad G. H., Voje K. L., Houle D., Cassara J., et al. (2014). Evolution of morphological allometry. Ann. N. Y. Acad. Sci. 1320, 58–75. doi: 10.1111/nyas.12470
Ponder W. F., Lindberg D. R., Ponder J. M. (2019). Biology and evolution of the Mollusca, volume 1 (Boca Raton, FL: CRC Press).
Ponder W. F., Lindberg D. R., Ponder J. M. (2021). Biology and evolution of the Mollusca, volume 2 (Boca Raton, FL: CRC Press).
Pörtner H. O., Farrell A. P. (2008). Physiology and climate change. Science 322, 690–692. doi: 10.1126/science.1163156
Promboon P., Nabhitabhata J., Duengdee T. (2011). Life cycle of the marbled octopus, Amphioctopus aegina (Gray)(Cephalopoda: Octopodidae) reared in the laboratory. Sci. Mar. 75 (4), 811–821. doi: 10.3989/scimar.2011.75n4811
Puneeta P., Vijai D., Yoo H. K., Matsui H., Sakurai Y. (2015). Observations on the spawning behavior, egg masses and paralarval development of the ommastrephid squid Todarodes pacificus in a laboratory mesocosm. J. Exp. Biol. 218 (23), 3825–3835. doi: 10.1242/jeb.127670
Reid A., Jereb P., Roper C. F. E. (2005). “Family Sepiidae,” in Cephalopods of the world. An annotated and illustrated catalogue of species known to date. volume 1. chambered nautiluses and sepioids. Eds. Jereb P., Roper C. F. E. (Rome: FAO), 57–152.
Roberts M. J., van den Berg M. (2002). Recruitment variability of chokka squid (Loligo vulgaris reynaudii) - role of currents on the Agulhas Bank (South Africa) in paralarvae distribution and food abundance. Bull. Mar. Sci. 71, 691–710
Robin J. P., Roberts M., Zeidberg L., Bloor I., Rodriguez A., Briceno F., et al. (2014). “Transitions during cephalopod life history: the role of habitat, environment, functional morphology and behaviour,” in Advances in Marine Biology Vol. 67. Ed. Vidal E. A. G. (Oxford: United Kingdom: Academic Press), 361–437. doi: 10.1016/B978-0-12-800287-2.00004-4
Robison B., Seibel B., Drazen J. (2014). Deep-sea octopus (Graneledone boreopacifica) conducts the longest-known egg-brooding period of any animal. PloS One 9 (7), e103437. doi: 10.1371/journal.pone.0103437 PMID:25075745
Rocha F., Guerra Á., González Á.F. (2001). A review of reproductive strategies in cephalopods. Biol. Rev. 76, 291–304. doi: 10.1017/S1464793101005681
Rodhouse P. G., Clarke M. R., Murray A. W. A. (1987). Cephalopod prey of the wandering albatross Diomedea exulans. Mar. Biol. 96, 1–10. doi: 10.1007/BF00394833
Rodhouse P. G., Pierce G. J., Nichols O. C., Sauer W. H., Arkhipkin A. I., Laptikhovsky V., et al. (2014). “Environmental effects on cephalopod population dynamics: implications for management of fisheries,” in Advances in Marine Biology Vol. 67. Ed. Vidal E. A. G. (United Kingdom: Academic Press), 99–233. doi: 10.1016/B978-0-12-800287-2.00002-0
Roff D. A. (1992). The evolution of life histories, theory and analyses (New York: Chapman and Hall).
Roper C. F. E., Jereb P. (2010). “Family Thysanoteuthidae,” in Cephalopods of the world. an annotated and illustrated catalogue of species known to date volume 2. Myopsid and Oegopsid Squids. Eds. Jereb P., Roper C. F. E. (Rome: FAO), 384–387.
Roper C. F. E., Vecchione M. (1996). In situ observations on Brachioteuthis beanii Verrill: paired behavior, probably mating (Cephalopoda, Oegopsida). Am. Malacol. Bull. 13, 55–60.
Roper C. F. E., Young R. E. (1975) Vertical distribution of pelagic cephalopods. Smith. Contrib. Zool. 209, 1–51.
Rosa R., O'Dor R., Pierce G. J. (2013a). Advances in squid biology, ecology and fisheries. part I: Myopsid squids (New York: NOVA Science Publishers, Inc).
Rosa R., Pierce G. J., O’Dor R. (2013b). Advances in squid biology, ecology and fisheries, part II: Oegopsid squids (New York: NOVA Science Publishers, Inc).
Rosa R., Pimentel M. S., Boavida-Portugal J., Teixeira T., Truebenbach K., Diniz M. (2012). Ocean warming enhances malformations, premature hatching, metabolic suppression and oxidative stress in the early life stages of a keystone squid. PloS One 7 (6), e38282. doi: 10.1371/journal.pone.0038282
Roumbedakis K., Guerra Á. (2019). “Cephalopod senescence and parasitology,”. Eds. Gestal C., Pascual S., Guerra Á., Fiorito G., Vieites J. Handbook of Pathogens and Diseases in Cephalopods (Cham: Springer), 207–211. doi: 10.1007/978-3-030-11330-816
Roura Á., Amor M., González Á.F., Guerra Á., Barton E. D., Strugnell J. M. (2019). Oceanographic processes shape genetic signatures of planktonic cephalopod paralarvae in two upwelling regions. Prog. Oceanogr. 170, 11–27. doi: 10.1016/j.pocean.2018.10.005
Roura Á., Castro-Bugallo A., Martínez-Pérez M. (2023). The settlement stage in the common octopus Octopus vulgaris Cuvier, 1797: a complex transition between planktonic and benthic life-styles. Mar. Biol. 170 (5), 1–2. doi: 10.1007/s00227-023-04188-2
Saunders W. B. (1984). Nautilus growth and longevity: evidence from marked and recaptured animals. Science 224 (4652), 990–992.
Schwarz R., Piatkowski U., Robison B. H., Laptikhovsky V. V., Hoving H. J. (2020). Life history traits of the deep-sea pelagic cephalopods Japetella diaphana and Vampyroteuthis infernalis. Deep Sea Res. Part I Oceanogr. Res. Pap. 164, 103365. doi: 10.1016/j.dsr.2020.103365
Segawa S. (1987). Life history of oval squid Sepioteuthis lessoniana in Kominatoand adjacent waters central Honshu. Jpn. J. Tokyo Univ. Fish. 74, 67–105.
Seibel B. A. (2007). On the depth and scale of metabolic rate variation: scaling of oxygen consumption rates and enzymatic activity in the class Cephalopoda (Mollusca). J. Exp. Biol. 210 (1), 1–11. doi: 10.1242/jeb.02588
Seibel B. A., Hochberg F. G., Carlini D. B. (2000). Life history of Gonatus onyx (Cephalopoda: Teuthoidea), deep-sea spawning and post-spawning egg care. Mar. Biol. 137, 519–526. doi: 10.1007/s002270000359
Semmens J. M., Moltschaniwskyj N. A. (2000). An examination of variable growth in the loliginid squid Sepioteuthis lessoniana: a whole animal and reductionist approach. Mar. Ecol. Prog. Ser. 193, 135–141. doi: 10.3354/meps193135
Semmens J. M., Pecl G. T., Gillanders B. M., Waluda C. M., Shea E. K., Jouffre D., et al. (2007). Approaches to resolving cephalopod movement and migration patterns. Rev. Fish. Biol. Fish. 17, 401–423. doi: 10.1007/s11160-007-9048-8
Shea E. K., Vecchione M. (2002). Quantification of ontogenetic discontinuities in three species of oegopsid squids using model II piecewise linear regression. Mar. Biol. 140, 971–979. doi: 10.1007/s00227-001-0772-7
Shea E. K., Vecchione M. (2010). Ontogenetic changes in diel vertical migration patterns com-pared with known allometric changes in three mesopelagic squid species suggest an expanded definition of a paralarva. ICES J. Mar. Sci. 67, 1436–1443. doi: 10.1093/icesjms/fsq104
Shea E. K., Ziegler A., Faber C., Shank T. M. (2018). Dumbo octopod hatchling provides insight into early cirrate life cycle. Cur. Biol. 28, R144–R145. doi: 10.1016/j.cub.2018.01.032
Shigeno S., Takenori S., Boletzky S. V. (2010). “The origins of cephalopod body plans: a geometrical and developmental basis for the evolution of vertebrate-like organ systems,” in Cephalopods - Present and Past. Eds. Tanabe K., Shigeta Y., Sasaki T., Hirano H. (Tokyo: Tokai University Press), 23–34.
Snyder S. (1986a). “Successful rearing of Octopus dofleini from hatchling to settlement,” in Annual Conference Proceedings American Association of Zoological Parks and Aquariums, 781–783.
Snyder S. (1986b). Laboratory culture of Octopus dofleini from hatching to settlement. Am. Malacol. Bull. 4 (2), 241.
Staudinger M. D., Juanes F., Salmon B., Teffer A. K. (2013). The distribution, diversity, and importance of cephalopods in top predator diets from offshore habitats of the Northwest Atlantic ocean. Deep-Sea Res. II. 95, 182–192. doi: 10.1016/j.dsr2.2012.06.004
Sugimoto C., Ikeda Y. (2012). Ontogeny of schooling behavior in the oval squid Sepioteuthis lessoniana. Fish. Sci. 78, 287–294. doi: 10.1007/s12562-011-0464-2
Sweeney M. J., Roper C. F., Mangold K. M., Clark M. R., Boletzky S. V. (1992). "Larval" and juvenile cephalopods: a manual for their identification. Smith. Contrib. Zool. 513, 1–282. doi: 10.5479/si.00810282.513
Todd C. D., Laverack M. S., Boxshall G. (1996). Coastal marine zooplankton: a practical manual for students (United Kingdom: Cambridge University Press).
Uchiyama K., Tanabe K. (1996). “Hatching of Nautilus macromphalus in Toba Aquarium, Japan” in Advancing Research on Living and Fossil Cephalopods. Proceedings of the IV International Symposium on Cephalopods: Present and Past, eds. Oloriz F., Rodriguez-Tovar F. J. (Grenada, Kluwer Academic), 13–16.
Uriarte I., Farías A. (2014). “Enteroctopus megalocyathus,” in Cephalopod culture. Eds. Iglesias J., Fuentes L., Villanueva R. (Dordrecht: Springer). 365–382.
Uriarte I., Hernandez J., Dorner J., Paschke K., Farías A., Crovetto E., et al. (2010). Rearing and growth of the octopus Robsonella fontaniana (Cephalopoda: Octopodidae) from planktonic hatchlings to benthic juveniles. Biol. Bull. 218, 200–210. doi: 10.1086/BBLv218n2p200
Uriarte I., Iglesias J., Domingues P., Rosas C., Viana M. T., Navarro J. C., et al. (2011). Current status and bottle neck of octopod aquaculture: the case of American species. J. World Aquacult. Soc 42, 735–752. doi: 10.1111/j.1749-7345.2011.00524.x
Van Heukelem W. F. (1976). Growth, bioenergetics and life-span of Octopus cyanea and Octopus maya. [Ph.D. thesis] (Honolulu HI: University of Hawai at Manoa).
Van Heukelem W. F. (1983). “Octopus maya,” in Cephalopod life cycles vol. I. Ed. Boyle P. R. (Lon-don: Academic Press), 311–324.
Vecchione M. (1987). “Juvenile ecology,” in Cephalopod life cycles, vol. II. Ed. Boyle P. R. (London: Academic Press), 61–84.
Vecchione M. (2019). ROV observations on reproduction by deep-sea cephalopods in the central Pacific ocean. Front. Mar. Sci. 6. doi: 10.3389/fmars.2019.00403
Vecchione M., Young R. E. (2018) Illicinae Posselt 1891. Illex Steenstrup 1880 version 20 February 2018 (under construction). Available at: http://tolweb.org/Illex/19940/2018.02.20 (Accessed January 2023).
Vecchione M., Young R. E., Piatkowski U. (2010). Cephalopods of the northern mid-Atlantic ridge. Mar. Biol. Res. 6, 25–52. doi: 10.1080/17451000902810751
Vidal E. A. G. (1994). Relative growth of paralarvae and juveniles of Illex argentinus (Castellanos, 1960) in southern Brazil. Antarct. Sci. 6, 275–282. doi: 10.1017/S0954102094000416
Vidal E. A. G., DiMarco F. P., Wormuth J. H., Lee P. G. (2002). Influence of temperature and food availability on survival, growth and yolk utilization in hatchling squid. Bull. Mar. Sci. 71, 915–931.
Vidal E. A. G., DiMarco P., Lee P. (2006). Effects of starvation and recovery on the survival, growth and RNA/DNA ratio in loliginid squid paralarvae. Aquaculture. 260, 94–105. doi: 10.1016/j.aquaculture.2006.05.056
Vidal E. A. G. (2014). Advances in cephalopod science: biology, ecology, cultivation and fisheries. Adv. Mar. Biol. (London: Academic Press) 67.
Vidal E. A. G., Villanueva R., Andrade J. P., Gleadall I. G., Iglesias J., Koueta N., et al. (2014). “Cephalopod culture: current status of main biological models and research priorities,” in Advances in Marine Biology vol. 67. Ed. Vidal E. A. G. (United Kingdom: Academic Press), 1–98. doi: 10.1016/B978-0-12-800287-2.00001-9
Vidal E. A. G., Boletzky S. V. (2014). “Loligo vulgaris and Dorytheuthis opalescens,” in Cephalopod Culture. Eds. Iglesias J., Fuentes L., Villanueva R. (London: Springer-Verlag), 271–313.
Vidal E. A. G., Zeidberg L. D., Buskey E. J. (2018). Development of swimming abilities in squid paralarvae: behavioral and ecological implications for dispersal. Front. Physiol. 9. doi: 10.3389/fphys.2018.00954
Vidal E. A. G., Salvador B. (2019). The tentacular strike behavior in squid: functional interdependency of morphology and predatory behaviors during ontogeny. Front. Physiol. 10. doi: 10.3389/fphys.2019.01558
Villanueva R. (1992). Continuous spawning in the cirrate octopods Opisthoteuthis agassizii and O. vossi: features of sexual maturation defining a reproductive strategy in cephalopods. Mar. Biol. 114, 265–275. doi: 10.1007/BF00349529
Villanueva R. (1995). Experimental rearing and growth of planktonic Octopus vulgaris from hatching to settlement. Can. J. Fish. Aquat. 52, 2639–2650. doi: 10.1139/f95-853
Villanueva R., Coll-Lladó M., Bonnaud-Ponticelli L., Carrasco S. A., Escolar O., Fernández-Álvarez F.Á., et al. (2021). Born with bristles: new insights on the Kölliker’s organs of Octopus skin. Front. Mar. Sci. 8. doi: 10.3389/fmars.2021.645738
Villanueva R., Laptikhovsky V. V., Piertney S. B., Fernández-Álvarez F.Á., Collins M. A., Ablett J. D., et al. (2020). Extended pelagic life in a bathybenthic octopus. Front. Mar. Sci. 7. doi: 10.3389/fmars.2020.561125
Villanueva R., Norman M. (2008). Biology of the planktonic stages of benthic octopuses. Oceanogr. Mar. Biol. 46, 105–202. doi: 10.1201/9781420065756.ch4
Villanueva R., Vidal E. A. G., Fernández-Álvarez F.Á., Nabhitabhata J. (2016). Early mode of life and hatchling size in cephalopod molluscs: influence on the species distributional ranges. PloS One 11 (11), e0165334. doi: 10.1371/journal.pone.0165334
Voight J. R., Drazen J. C. (2004). Hatchlings of the deep sea octopus Graneledone boreopacifica are the largest and most advanced known. J. Molluscan Stud. 70, 400–402. doi: 10.1093/mollus/70.4.400
Wakabayashi T., Saito K., Tsuchiya K., Segawa S. (2002). Descriptions of Eucleoteuthis luminosa (Sasaki, 1915) and Ornithoteuthis volatilis (Sasaki, 1915) paralarvae in the northwestern Pacific. Jap. J. Malacol. (Venus) 60, 237–260.
Wakabayashi T., Tsuchiya K., Segawa S. (2005). Morphological changes with growth in the paralarvae of the diamondback squid Thysanoteuthis rhombus Troschel, 1857. Phuket Mar. Biol. Cent. Res. Bull. 66, 167–174.
Xavier J. C., Cherel Y., Roberts J., Piatkowski U. (2013). How do cephalopods become available to seabirds: can fish gut contents from tuna fishing vessels be a major food source of deep-dwelling cephalopods? ICES J. Mar. Sci. 70, 46–49. doi: 10.1093/icesjms/fss167
Xavier J. C., Walker K., Elliot G., Cherel Y., Thompson D. (2014). Cephalopod fauna of South Pacific waters: new information from breeding New Zealand wandering albatrosses. Mar. Ecol. Prog. Ser. 513, 131–142. doi: 10.3354/meps10957
Yamamoto K., Okutani T. (1975). Studies on early life history of decapodan Mollusca V. Systematics and distribution of epipelagic larvae of decapod cephalopods in the southwestern waters of Japan during the summer. Bull. Tokai Reg. Fish. Res. Lab. 83, 45–96.
Yang Z., Zhang L., Hu J., Wang J., Bao Z., Wang S. (2020). The evo-devo of molluscs: insights from a genomic perspective. Evol. Dev. 22, 409–424. doi: 10.1111/ede.12336
Young R. E. (1972). The systematics and areal distribution of pelagic cephalopods from the seas off southern California. Smithson. Contr. Zool. 97, 1–159. doi: 10.5479/si.00810282.97
Young R. E. (1975a). Bioluminescent countershading in midwater animals, evidence from living squid. Pac. Sci. 191, 1046–1048. doi: 10.1126/science.1251214
Young. R. E. (1975b). Leachia pacifica (Cephalopoda, Teuthoidea): spawning habitat and function of the brachial photophores. Pac. Sci. 29, 19–25.
Young R. E., Harman R. F. (1985a). Early life history stages of enoploteuthid squids (Cephalopoda:Teuthoidea: Enoploteuthidae) from Hawaiian waters. Vie Milieu 35, 181–201.
Young R. E., Harman R. F. (1985b). The larvae of ommastrephidae squids from Hawaiian waters. Vie Milieu 35, 211–222.
Young R. E., Harman R. F., Mangold K. M. (1985). The eggs and larvae of Brachioteuthis sp. (Cephalopoda: Teuthoidea) from Hawaiian waters. Vie Milieu. 35 (3/4), 203–209.
Young R. E., Harman (1988). "Larva", "paralarva" and "subadult" in cephalopod terminology. Malacologia. 29, 201–207.
Young R. E. (1991). Chiroteuthid and related paralarvae from Hawaiian waters. Bull. Mar. Sci. 49, 162–185.
Young R. E., Vecchione M. (1999). Morphological observations on a hatchling and a paralarva of the vampire squid, Vampyroteuthis infernalis Chun (Mollusca: Cephalopoda). Proc. Biol. Soc Wash. 112, 661–666.
Young R. E., Roper C. F. E., Vecchione M. (2019) Chiroteuthidae Gray, 1849 Version 26 March 2019 (under construction). Available at: http://tolweb.org/Chiroteuthidae/19451/2019.03.26 (Accessed January 2023).
Zakroff C., Mooney T. A., Berumen M. L. (2019). Dose-dependence and small scale variability in responses to ocean acidification during squid, Dorytheuthis pealeii, development. Mar. Biol. 166, 62. doi: 10.1007/s00227-019-3510-8
Zaragoza N., Quetglas A., Moreno A. (2015). Identification guide for cephalopod paralarvae from the Mediterranean Sea. ICES Cooperative Res. Rep. 324, 1–91.
Zeidberg L. D., Hamner W. M. (2002). Distribution of squid paralarvae, Loligo opalescens (Cephalopoda: Myopsida), in the southern California bight in the three years following the 1997–1998 El niño. Mar. Biol. 141, 111–122. doi: 10.1007/s00227-002-0813-x
Zheng X. D., Lin X. Z., Liu Z. S., Wang Z. K., Zheng W. B. (2014). Sepiella japonica, in Cephalopod Culture. Eds. Iglesias J., Fuentes L., Villanueva R. (London: Springer-Verlag), 241–252.
Ziegler A., Miller A., Nagelmann N. (2021). Novel insights into early life stages of finned octopods (Octopoda: Cirrata). Swiss J. Paleontol. 140, 1–20. doi: 10.1186/s13358-021-00240-0
Keywords: definitions, hatchling, meropelagic, metapelagic, morphology, Paralarva, senescent, terminology
Citation: Vidal EAG and Shea EK (2023) Cephalopod ontogeny and life cycle patterns. Front. Mar. Sci. 10:1162735. doi: 10.3389/fmars.2023.1162735
Received: 09 February 2023; Accepted: 25 May 2023;
Published: 07 July 2023.
Edited by:
Marco Casu, University of Sassari, ItalyReviewed by:
Danila Cuccu, University of Cagliari, ItalyJin Gao, Memorial University of Newfoundland, Canada
Copyright © 2023 Vidal and Shea. This is an open-access article distributed under the terms of the Creative Commons Attribution License (CC BY). The use, distribution or reproduction in other forums is permitted, provided the original author(s) and the copyright owner(s) are credited and that the original publication in this journal is cited, in accordance with accepted academic practice. No use, distribution or reproduction is permitted which does not comply with these terms.
*Correspondence: Erica A. G. Vidal, ericagvidal@gmail.com