- 1School of Zoology, Faculty of Life Sciences, Tel- Aviv University, Tel-Aviv, Israel
- 2The Steinhardt Museum of Natural History, Tel- Aviv University, Tel-Aviv, Israel
- 3Ruppin Academic Center, Faculty of Marine Sciences, Michmoret, Israel
- 4School of Life Sciences and Biotechnology, Shanghai Jiao Tong University, Shanghai, China
Climate change is predicted to have detrimental impacts on sessile invertebrates, including sponges. Mesophotic ecosystems have been suggested to play a major role as refugia for coral reef sponge species, however knowledge regarding the ability of mesophotic sponges to cope with thermal stress is scarce. In this study we compared the response of the sponge Diacarnus erythraeanus, a widespread Red Sea sponge, from the shallow and mesophotic reef, to moderate and acute temperature elevation (2°C and 6°C, respectively) for short and long term periods (two and 35 days, respectively) by measuring physiological parameters (respiration, oxygen removal, pumping rates, and photosynthetic efficiency), and the microbiome composition change. The results indicated that mesophotic and shallow populations of D. erythraeanus are highly tolerant to both moderate and acute heat stress, demonstrating a high survival rate (100%) across the experimental treatments, with no visible signs of bleaching or necrosis. Exposure to heat stress resulted in significant alterations in the physiological parameters of sponges, including higher respiration rate and lower photosynthetic efficiency. These alterations were accompanied by correspondingly significant microbial adjustments, thus emphasizing the essential role of the microbiome in the host’s ability to persist when facing essential environmental stress. Moreover, while shallow and mesophotic sponges showed similar physiological tolerance to heat stress, their microbial response differed: while the microbiome diversity of the mesophotic sponges remained stable throughout the experiment, the shallow one significantly changed. This result suggests that their underlying coping mechanisms might differ between mesophotic and shallow populations. Since the associated-microbiome is largely regulated by the sponge-host genetics, difference in microbial adjustments to stress between populations, could indicate genetic variability between hosts. Therefore, while the results of this study support the hypothesis that mesophotic coral reefs could serve as thermal refugia for some sponge species, it raises the question regarding the validity of MCEs as a refuge for shallow populations. Finally, it emphasizes the crucial need to elucidate the underlying mechanisms governing the sponge-microbiome interactions, specifically in the context of the anticipated climate change scenarios.
1 Introduction
Global climate changes have already been shown to have detrimental effects on marine coastal ecosystems (Hughes et al., 2018a; Forster et al., 2021). These effects are predicted to worsen as the intensity, frequency, and duration of marine heatwave events are expected to increase in the future (Frölicher et al., 2018; Oliver et al., 2018; Muñiz-Castillo et al., 2019; IPCC, 2023). The magnitude of the climate change effects varies among the different habitats. Mesophotic coral ecosystems (MCEs), considered as those between 30-150 m depth (Lesser et al., 2009; Kahng et al., 2010), are less affected compared to their adjacent shallower reefs (Hoegh-Guldberg et al., 2007; Kahng et al., 2017). Due to their distance from the sea surface, MCEs are considered to be more stable and environmentally predictable than shallow reefs (Baker et al., 2016). Accordingly, thermal anomalies are reduced in MCEs and the water temperature is cooler compared to that of the shallow depths (Lesser et al., 2009; Shlesinger et al., 2018; Turner et al., 2019). Since the former are genetically and physically associate with their shallow reefs counterparts, they have been suggested to play a major role as refugia and as a larval source for species unable to tolerate the current changing conditions in the shallow waters (Bongaerts et al., 2010; Weiss, 2017). A recent example of the mesophotic depth serving as a thermal refugium is the case of the Mediterranean sponge Agelas oroides. This sponge vanished from the shallow habitats along the Israeli coast in the 1970s, but was recently re-discovered in the mesophotic sponge grounds (100–120 m) in the same area. An experiment transplanting mesophotic individuals to the shallow waters, revealed that the sponges survived well until the temperature exceeded 28°C, when they rapidly perished, suggesting that this sponge’s disappearance from the Israeli shallow waters might have occurred following prolonged periods of elevated temperature (Idan et al., 2020). Another example is that of coral bleaching after a heatwave event in French Polynesia. The bleaching significantly decreased with depth, with minor to no bleaching observed at the mesophotic depths (Pérez-Rosales et al., 2021). Such examples emphasize the potential of mesophotic depths to serve as a thermal shelters for various benthic organisms.
Among the variety of organisms affected by these environmental changes, sessile organisms, such as sponges and corals, are presumably the most sensitive, since they are unable to relocate (Hughes et al., 2018b). Sponges (Phylum Porifera) are widespread benthic organisms, fulfilling fundamental roles in ecosystem functioning (Bell, 2008; Rix et al., 2018). In some coral reefs, sponges are considered the second most abundant component in the benthic assemblage after corals (Diaz and Rützler, 2001). Accumulating evidence from recent years suggests that sponges’ responses to thermal stress vary, with some species more tolerant to a rise in temperature (Luter et al., 2020; Ribeiro et al., 2021; Vargas et al., 2021; Posadas et al., 2022), while others are more sensitive, manifesting bleaching, necrosis, and death (Cebrian et al., 2011; Ramsby et al., 2018; Beepat et al., 2020; Rubio-Portillo et al., 2021). How a sponge responds to environmental stress, however, might be largely a function of its specific associated microbial community (Marangon et al., 2021). The sponge’s microbiome benefits the host by providing key functions that support the sponge-host’s health and fitness (Hentschel et al., 2012; Webster and Thomas, 2016; Zhang et al., 2019; Engelberts et al., 2020; Marangon et al., 2021; Taylor et al., 2021), particularly when subjected to environmental stress (Webster and Reusch, 2017; Pita et al., 2018; Marangon et al., 2021; Posadas et al., 2022).
The diverse associations and dependence between the sponge-host and its microbiome have led to the consortium of microorganisms and their host being regarded as a “meta-organism” or holobiont (Bosch and McFall-Ngai, 2011; Webster and Thomas, 2016), rather than as autonomous components. Upon experiencing environmental stress, the sponge-microbiome might respond in several ways, such as dysbiosis (a break-down of the association), which mostly results in host health decline, disease, and possible mortality. Once dysbiosis occurs, it is characterized by a sharp increase (usually of opportunistic bacteria) or decrease of the natural microbial community (Fan et al., 2013; Lesser et al., 2016; Rondon et al., 2020). For example, the microbiome of the barrel sponge, Xestospongia muta, was destabilized following exposure to low pH and thermal stress conditions, leading to a decline in the host’s nutrient budget (e.g., carbohydrates) (Lesser et al., 2016). In the Great Barrier Reef (GBR) sponge, Rhopaloeide odorabile, various symbiotic functions were lost due to interruption of the host-symbiotic interactions following thermal stress, while pathogenic functions or virulent protein-associated functions were enriched (Fan et al., 2013). However, the microbiome can also be reconstructed, either by changes in the bacterial abundance or by acquiring new bacteria (Ribes et al., 2016; Vargas et al., 2021), leading to a more suitable and beneficial composition that might enhance the host’s resilience under stress (Reshef et al., 2006; Webster and Reusch, 2017). For example, a sponge’s ability to restructure its microbial assemblage was identified as a major factor contributing to its tolerance following exposure to acidification conditions (Ribes et al., 2016). Similarly, in the common aquarium sponge Lendenfeldia chondroides, re-accommodation of bacterial members was associated with the sponge’s acclimation to heat stress (Vargas et al., 2021). Alternatively, resilience might be an outcome of a microbial stable state, in which no change occurs in the bacterial diversity and composition up to a certain temperature (Webster et al., 2008; Simister et al., 2012). These various interactions suggest that the sponge host’s stability is largely attributed to its dynamic equilibrium with its associated microbiome (Fan et al., 2013; Glasl et al., 2018).
While there are accumulating experimental data regarding the microbial response of sponges to thermal stress (Luter et al., 2020; Moreno-Pino et al., 2020; Ribeiro et al., 2021; Vargas et al., 2021), studies on the combined response of the holobiont (both host and microbiome) are sparse (reviewed in Pita et al., 2018). Moreover, although MCEs are being considered as potential thermal refugia, the research to date has been mainly focused on the response of sponges from shallow sites to thermal stress and not that of mesophotic sponges (Simister et al., 2012; Fan et al., 2013; Guzman and Conaco, 2016; Rubio-Portillo et al., 2021). The latter are not exposed to extreme temperature fluctuations and, therefore, may not be adapted to thermal stress, unlike their shallow counterparts. Since MCEs are not immune to the predicted climate change effects (Neal et al., 2014; Nir et al., 2014), it is of importance to determine the ability of sponges from MCEs to tolerate thermal stress (Morrow et al., 2016; Gould et al., 2021), as well as that of the adjacent shallow sponges. Finally, in the face of an increase in global warming, understanding the spatial dynamics (shallow vs. mesophotic) of the holobiont is crucial in order to obtain new insights into the resilience of already degraded coral reefs (Birkeland, 2019).
The coral reefs of Eilat, located at the northern tip of the Gulf of Aqaba, are among the most diverse coral reefs worldwide (Loya, 2004). They are characterized by a steep depth gradient, with only a very short distance (~50-250 m) separating the shallow from the mesophotic reefs (Kramer et al., 2019). Additionally, fauna in the Gulf of Eilat/Aqaba are characterized by their heat tolerance and resilience to thermal stress, so much that the shallow corals in Eilat, were described as ‘super-corals’ (Fine et al., 2013; Grottoli et al., 2017). Despite the study of coral resilience, the heat tolerance of sponges in the Gulf of Eilat/Aqaba has yet to be evaluated. Moreover, while various studies examined the role of MCEs to serve as coral refugia in the Gulf of Eilat/Aqaba, they have neither been evaluated for sponges, nor in other coral reefs worldwide. The endemic Red Sea sponge Diacarnus erythraeanus (Kelly-Borges and Vacelet, 1995, Demospongiae, Poecilosclerida) is widespread in the benthic communities from shallow to mesophotic depths, and hosts a diverse microbial community, including cyanobacteria (Oren et al., 2005). Hence it offers a unique opportunity to examine possible differences in resilience to heat stress, of shallow vs. mesophotic individuals. It also allows for the first time to evaluate the role of MCEs as thermal sponge refugia at the Gulf of Eilat/Aqaba. In this study, we compared the response of D. erythraeanus individuals from both shallow (5-10 m) and mesophotic reefs (45-55 m) to thermal stress in vitro. The response of both the sponge host and its microbiome were assessed for short- and long-term periods (two and after 35 days of heat stress exposure), using physiological measurements and meta-barcoding sequencing of the bacterial assemblage (16S rRNA gene).
We hypothesized that, in response to elevated temperature scenarios, the mesophotic sponges would: a) exhibit higher stress and lower survival compared to shallow sponges, expressed in terms of physiological performance (lower survival, higher respiration rates, lower photosynthetic activity, etc.); and b) exhibit extreme shifts in their microbial composition compared to the shallow sponges.
2 Materials and methods
2.1 Sponge sampling
Diacarnus erythraeanus specimens were collected from the northern Gulf of Aqaba (Eilat) (29°31’19.0”N 34°56’09.3”E) in July 2019. Six individuals were sampled using SCUBA diving from shallow reefs (5-10 m) and six from mesophotic reefs (40-50 m) using a Remotely Operated Vehicle (ROV, ECA-Robotics H800) onboard the R/V Sam Rothberg (Permit no. 2018/42031 and 2019/42248). Immediately after collection each sponge was cut into 15-17 clones (~18 cm3). One clone from each original specimen was flash-frozen in liquid nitrogen and stored (-80°C) to await further characterization of the sponges’ wild-type bacterial composition. All the other clones were tagged to enable identification of the individual sponge, and secured to underwater nursery structures in situ (~10 m depth), for ~5 months of regeneration and acclimation, before being translocated to the experimental system (Supplementary Figures 1A, B).
2.2 Experimental design
The experiment was conducted in 40 L aquaria in a flow-through mesocosm system, Red Sea Simulator, located at the Interuniversity Institute for Marine Sciences (IUI) in Eilat, Israel (for technical details see Bellworthy and Fine (2018)). Temperature treatments comprised of the current ambient temperature (~24°C), the predicted local temperature for 2100, as calculated by IPCC RCP8.5 of 2°C (~26°C, ‘Moderate’ treatment) (Hoegh-Guldberg et al., 2014) and an acute increase of 6°C above ambient (~30°C, ‘Acute’ treatment). The clones were distributed across the three treatments to ensure that each sponge-individual had clones in each of the treatments and at each sampling point. Moderate and acute treatments were each incorporated in four aquaria replicates, together containing a total of 48 clones (24 per treatment), six per aquarium. Each aquarium contained sponge clones from both mesophotic and shallow habitats (Figure 1A), randomly assigned to the different aquaria. Following an acclimation period of five days at ambient temperature (24°C), temperature in the aquarium was then increased by 1°C/day until the desired treatment temperatures were reached (Figure 1B). Since each thermal treatment reached the target temperature at a different time, two groups of control ambient temperature were assigned, one group to each thermal treatment, each comprising of 24 sponge clones which were placed in eight aquaria replicates. The experiment lasted 35 days.
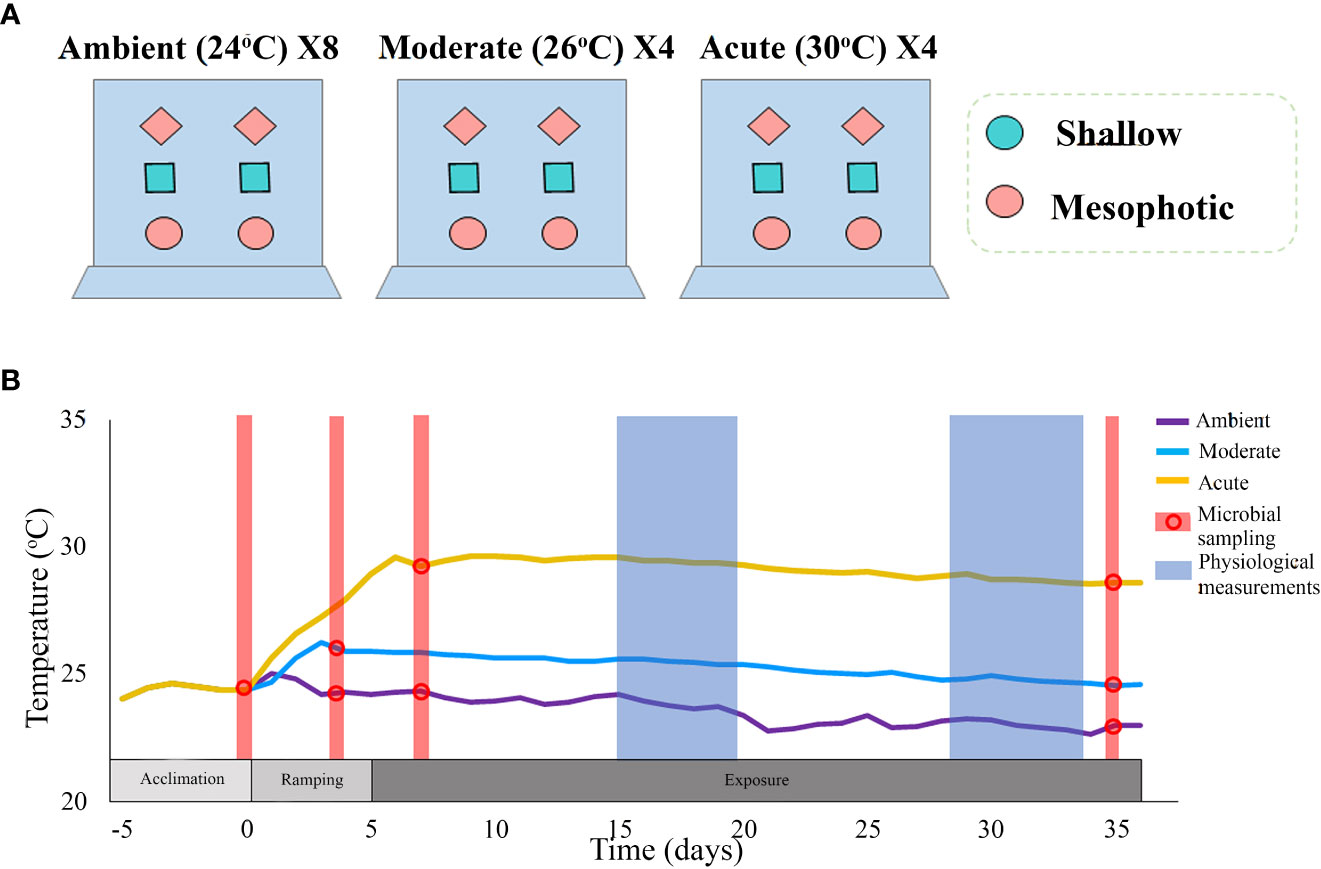
Figure 1 (A) An illustrated description of the experimental set-up. The experiment comprised two thermal treatments: moderate (~26°C) and acute (~30°C), each with four aquaria replicates; and a control ambient temperature (~24°C), with eight replicates. Each thermal treatment comprised 24 clones (six clones per aquarium), originating from 12 different sponge individuals: six from a shallow reef and six from a mesophotic reef. The sponge clones were randomly assigned to the aquaria with each aquarium containing sponges from both mesophotic and shallow habitats, and with each type of individual-clone present in every treatment. (B) Timeline and temperature throughout the duration of the experiment. Different colors represent the various treatments. Dots represent the time-points at which microbial sampling was performed. Physiological measurements comprised respiration rate, oxygen removal, pumping rate, buoyant weight, and photosynthetic efficiency (measured only at Tf).
2.3 Physiological measurements
Sponge pumping activity [Lpumped hr-1ind-1] was evaluated using a modification of the Dye Front Speed method (DFS) as described by (Yahel et al., 2005; Morganti et al., 2019). Each DFS measurement was calculated as the average of repeated measurements (4-6 time) per sponge.
Dissolved oxygen (DO) removal [µmol O2 L-1], was measured using GO2 FireSting (PyroScience, German, hereafter “optodes”). Each measurement lasted for 10 min at 10 s intervals. Optodes were located in the sponge excurrent water ( ), near the osculum, using custom-made manipulators that minimized interruption of the sponge clone during the measurement and stabilized the sensor (Supplementary Figure 1C). Oxygen concentration measurement in the aquarium surrounding water ( took place before each measurement of the sponge-oxygen removal. Oxygen concentration median values (for 10 min measurement) were then calculated for both, and , and the DO removal per liter (ΔO2, µmol L-1) was calculated as follows (Moskovish et al., 2022):
Eq. 1.
Respiration rate [µmol O2 Lpumped-1 hr-1 ind-1] was calculated by multiplying the sponge’s pumping rate by its DO removal for consecutive measurements.
Changes in buoyant weight were measured using a digital scale (ViBRA AJ-320CE) following Osinga (1999).
Sponge physiological measurements were conducted in the middle (days 15-20) and at the end (days 28-35) of the experiment (Figure 1B).
Changes in photosynthetic efficiency of the sponge-associated cyanobacteria under the different thermal treatments were thus assessed by determining the changes in maximal quantum yields (Fv/Fm) of Photosystem II in dark-adapted samples. For this, we used a Diving-Pulse Amplitude Modulation fluorometer (Diving-PAM; Walz, Germany), in which the ground fluorescence in the dark-adapted state signal (Fo) was detected by emitting red modulated measuring light (a weak pulsed light; < 1μmol photons m−2 s−1), followed by a short saturating pulse, to estimate the maximal fluorescence level (Fm). The maximal quantum yield was obtained from the ratio of Fv/Fm, where the variable fluorescence of dark-adapted samples (Fv) was calculated as the subtraction of (Fo-Fm). The measurement was repeated 3-5 times for each sponge clone, at different locations on the clone surface. Measurements took place on day 34 of the experiment, two hours after sunset, to ensure dark acclimation of the PSII.
2.4 Statistical analyses of the physiological measurements
Differences in the physiological measurements were evaluated using a permuted ANOVA test (n=5000 permutations) followed by pairwise comparisons with FDR correction for multiple comparisons, where p value<0.05 was defined as the significant threshold in all analyses. A mixed model was designed to examine the interaction of treatment and depth as fixed factors, and, when relevant, sponge-individual was considered as a random effect within treatment or sampling time. The respiration and pumping rates from the middle and end of the experiment did not diverge in their variance of medians. This, and since only a small number of consecutive measurements (of sponge pumping rate and oxygen removal) were made, their values were pooled to increase power analysis.
Statistical analyses were carried out using the permuco (Frossard and Renaud, 2021), rcompanion (Mangiafico and Mangiafico, 2017), and ggplot2 (Wickham, 2016) packages within RStudio environment (V. 2021.09.0 + 351) (Allaire, 2012).
2.5 Microbial sampling, gene sequencing, and bioinformatic processing
All sponges were sampled for microbiome analysis at four sampling points: on the day of sponge collection from their natural habitat (“wild-type”, as noted in section 2.1); after the five-day acclimation period at an ambient temperature of 24°C, prior to temperature raising (“pre-experiment”); and on 2 days after the aquarium water had reached the desired temperatures (“T2”) and at the last day of the experiment (“Tf”, Figure 1B). At each sampling point, one clone from the shallow waters and one from the mesophotic waters were randomly selected from each aquarium and immediately flash-frozen in liquid nitrogen and stored at −80°C for later DNA extraction.
Genomic DNA was extracted from the sponges using the DNeasy Blood & Tissue Kit (Qiagen) following the manufacturer’s protocol. DNA quantity and purity and were assessed using Qubit 3.0 (Thermofisher, USA) and gel electrophoresis (respectively). The 16S V4 rRNA gene region was amplified using modified 515f forward and 806r reverse primers (Apprill et al., 2015; Parada et al., 2016), and sequencing of 2X250 bp paired-end reads was performed on the Illumina NovaSeq 6000 platform (San Diego, CA, USA) at Magigene Biotechnology Co., Ltd. (Guangdong, China). Error sequencing correction, chimeric removal and denoising of the paired reads into contigs were applied on the resulting FASTQ files using DADA2 package (Callahan et al., 2016) and grouped into Amplicon Sequence Variants (ASVs) based in the naive Bayesian classifier method (Wang et al., 2007) against the SILVA v138.1 database (Quast et al., 2012). Sequences originating from Archaea, chloroplasts, mitochondria, and eukaryotes were removed. Raw sequences were deposited in the NCBI BioProject database under the accession number PRJNA931995.
2.6 Microbiome composition and diversity analyses
The sponges’ microbial community composition and diversity indices (richness and Shannon) were calculated at each sampling point for all the sampled clones (see section 2.5). A Phyloseq object was constructed to import sample data (metadata, abundance, and taxonomy tables) and to calculate microbiome dissimilarities between treatments and sampling points using the RStudio environment (V. 2021.09.0 + 351). Microbial dissimilarities were calculated with weighted-Unifrac distance matrix based on the samples’ relative abundance. In addition, to examine the effect of treatments and depths on microbial diversity, while considering sponge-individual origin, a mixed model was designed, with treatment and depth as fixed effects and sponge-individual as a random effect (nested within treatment). When examining the effect of time on the microbial diversity indices, time was defined as a fixed effect and sponge-individual as a random effect nested within time. Statistical differences were examined using permuted ANOVA (n=999), followed by post-hoc testing for pairwise comparisons, using the Friedman-Nemenyi test. Differences in microbial composition of the samples among treatments and depths were visualized using non-metric multidimensional distance analysis (nMDS) 3D ordinations, based on weighted UniFrac distance matrix. Statistical differences in the microbial communities’ composition among treatments, depths, and sampling points were examined using permutational MANOVA and pairwise comparisons with 999 permutations, where treatment, depth, and sponge-individual were set as the main factors and the interactions of treatment*depth and treatment*sponge-individual were also assessed. In addition, the 20 most abundant ASVs for each sampling point*treatment were calculated and then visualized using bar plots displaying the bacterial relative abundance on the order level. To further investigate which ASVs contributed to the difference across treatments and depths, differentially abundant ASV changes (log fold change ≥ |2 |,Wald test, adjusted p. value ≤ 0.01) were identified using Phyloseq-DESeq and DESeq function.
Microbial diversity calculations and visualizations were carried out using the following packages: phyloseq (McMurdie and Holmes, 2013), microbiome (Lahti and Shetty, 2018), ggpubr (Kassambara and Kassambara, 2020), ggplot2 (Wickham, 2016), vegan (Oksanen et al., 2013), Tidyverse (Wickham et al., 2019), DESeq2 (Love et al., 2014), permuco (Frossard and Renaud, 2021) and PMCMRplus (Pohlert and Pohlert, 2018).
3 Results
3.1 Sponge physiological responses to elevated temperature
All Diacarnus erythraeanus clones appeared to be intact and healthy throughout the 35 days of the experiment, with no signs of bleaching or necrosis, and without mortality under both the ambient and thermal treatments. However, temperature had a significant effect on the sponges’ respiration rate, with those exposed to acute temperature exhibiting a significantly higher rate (14.5 ± 1 µmol O2LpH-1Ind-1) compared to both ambient (4.9 ± 0.8 µmol O2LpH-1Ind-1) and moderate treatments (4.5 ± 1.6 µmol O2LpH-1Ind-1, p<0.005, Figure 2A, Table 1; Supplementary Table 1). Neither depth nor the interaction of temperature*depth had a significant effect on the sponges’ respiration rate (p>0.05. Table 1). Similarly, the sponges’ oxygen removal was significantly higher in those under acute heat treatment (29.6 ± 5.3 µmol O2L-1) compared to both ambient (13.3 ± 3.1 µmol O2L-1) and moderate treatments (14.7 ± 2.9 µmol O2 L-1), which did not differ from one another (Figure 2B, Table 1; Supplementary Table 1). Interestingly, the oxygen removal rate in both thermal treatments significantly decreased over the course of the experiment, while the ambient treatment oxygen removal remained stable. Consequently, at the end of the experiment the oxygen removal of the acute treatment sponges was similar to that of the other treatments (acute: 12 ± 3.6 µmol O2L-1, moderate: 5.0 ± 2.3 µmol O2L-1, ambient: 13.2 ± 1.7 µmol O2L-1, Figure 2B, Table 1; Supplementary Table 1). In addition, while no differences in the sponge’s oxygen removal were attributed to the sponge’s depth-origin, at the end of the experiment, the oxygen removal was significantly affected by the treatment*depth interaction (Table 1).
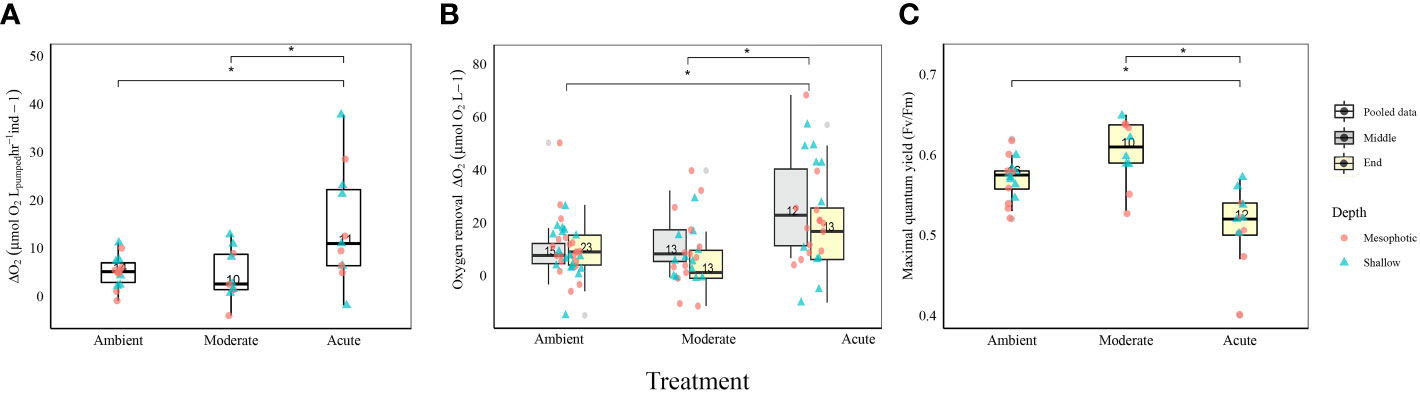
Figure 2 Physiological measurements of D. erythraeanus shallow (triangle) and mesophotic (circle) sponges exposed to ambient, moderate, or acute temperature. (A) Respiration rates (µmol ΔO2 LpH-1), pooled data. (B) Oxygen removal (µmol O2L-1) in the middle (gray) and at the end (yellow) of the experiment. Significant difference refers to the oxygen removal measurements in the middle of the experiment (C) Photosynthetic efficiency (maximal quantum yield Fv/Fm), pooled data. Median and 25-75 percent quartiles are indicated by the box. Dots are measurement values. The number of measurements is indicated within each box. Significant difference between treatments indicated by an asterisk. See Table 1 for detailed statistical information.
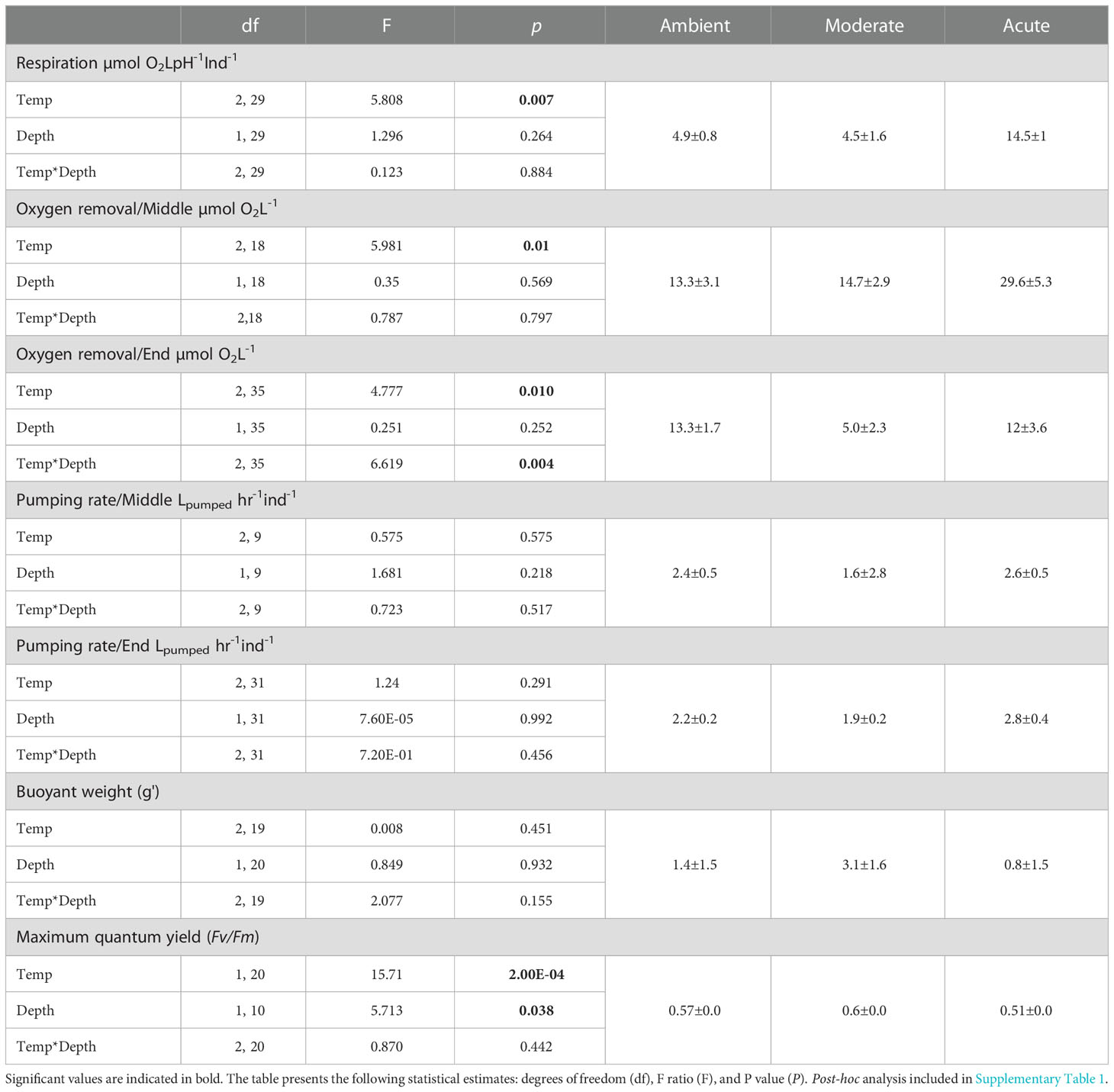
Table 1 Physiological measurements’, mean values + SE for each treatment (right) and summary statistics of temperature, depth-origin and their combined effect on the sponge respiration, oxygen removal rate, pumping rate, buoyant weight, and photosynthetic efficiency (Fv/Fm).
The sponges’ pumping rates remained stable throughout the experiment, with no significant effect of temperature, depth, or temperature*depth interaction. Likewise, the sponges’ buoyant weight remained stable throughout the course of the experiment, and was similar for both the ambient and the thermal treatments, with no effect of treatment*depth (Table 1).
3.2 Sponge microbiome response to elevated temperature
3.2.1 Photosymbionts function decrease
Photosymbionts exposed to the acute treatment exhibited a significantly lower photosynthetic efficiency (0.51 ± 0.01) compared to the moderate (0.6 ± 0.01) and the ambient treatment sponges (0.57 ± 0.00, Figure 2C, Table 1; Supplementary Table 1). The results also indicated that a sponge’s depth-origin had a significant effect on its photosynthetic efficiency, with a generally lower photosynthetic efficiency of the mesophotic sponges (0.55 ± 0.0) compared to the shallow ones (0.57 ± 0.0). However, no significant effect on the photosymbionts’ photosynthetic efficiency was found for the interaction between depth and temperature (Table 1).
3.2.2 16S rRNA bioinformatics analysis
A total of 110 samples were successfully sequenced, achieving 24,228,075 16S V4 rRNA raw sequences. After quality filtering, 18,317,714 reads remained, with an average of 166,524.6± 2631.3 reads per sample, which were classified into 7305 ASVs. Rarefaction curves indicated a sufficient bacterial diversity coverage, with curves reaching asymptotes in all samples (Supplementary Figure 2). The obtained ASVs affiliated with 37 bacterial phyla and 74 classes. In addition, 12 samples of the wild-type sponges were successfully sequenced, achieving 608,078 16S V4 rRNA raw sequences. After quality filtering, 278,840 reads remained, with an average of 23,236.6± 2485.1 reads per sample, which were classified into a sum of 1387 ASVs.
3.2.3 Microbial diversity and species composition change across time, temperature, and depth
Microbial diversity indices (richness, Shannon) of the wild-type sponges were similar for both shallow and mesophotic sponges (Table 2; Supplementary Table 3A). In addition, the microbial communities were mostly composed of similar orders. They differed, however, in their relative abundance (Figure 3A), and significantly differed in their microbial composition (weighted UniFrac, R2 = 0.21, p=0.009, Figure 4A, Table 2). The wild-type shallow samples were primarily composed of the orders HOC36 (Proteobacteria, 38.5%), Caldilineales (15%) and JTB23 (Proteobacteria, 7.5%), while in the mesophotic samples the orders HOC36 (21%), KI89A_clade (Proteobacteria, 21%) and Nitrospirales (20.1%) were the most dominant. Moreover, Rhodobacterales and Puniceispirillales appeared only in the mesophotic samples, while SBR1031 (Chloroflexi) and Dadabacteriales were present only in the shallow ones (Figure 3A). Likewise, several bacterial members significantly changed in abundance between depths. For example, a significant change in ASV abundance (log-fold change, p<0.01) was affiliated with the families Flavobacteriaceae (ASV592), Caldilineaceae (ASV1), Rhodobacteraceae (ASV42), SAR11_clade_lb (ASV769), Bdellovibrionaceae (ASV 392), Prevotellaceae (ASV181), and Kiloniellaceae (ASV135, Figure 5).
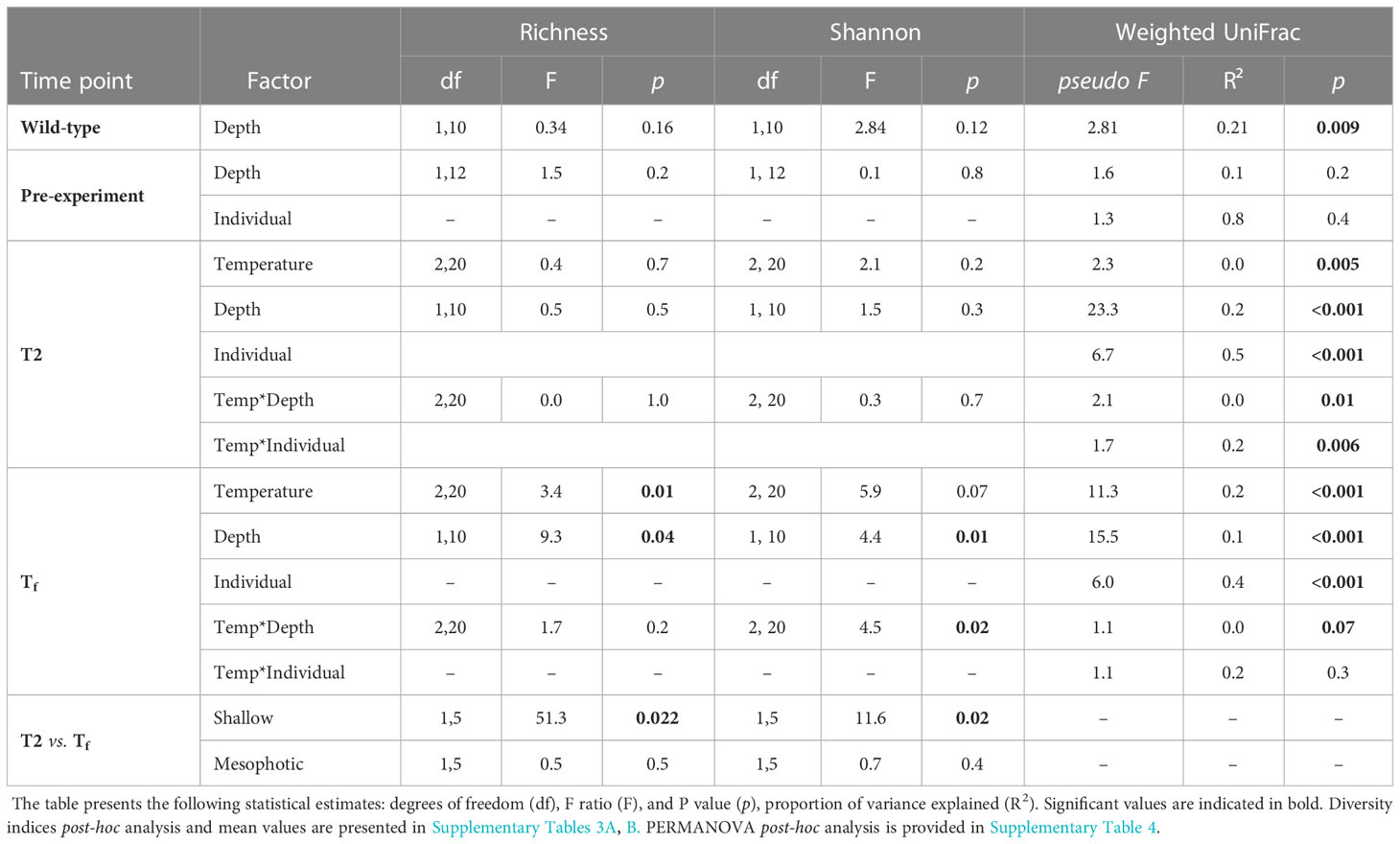
Table 2 Summary statistics for the effects of temperature, depth-origin, sponge-individual and their interaction at each time point on microbial diversity indices (richness and Shannon) and on the microbiome community composition at each time point (PERMANOVA results using weighted -unifrac distance).
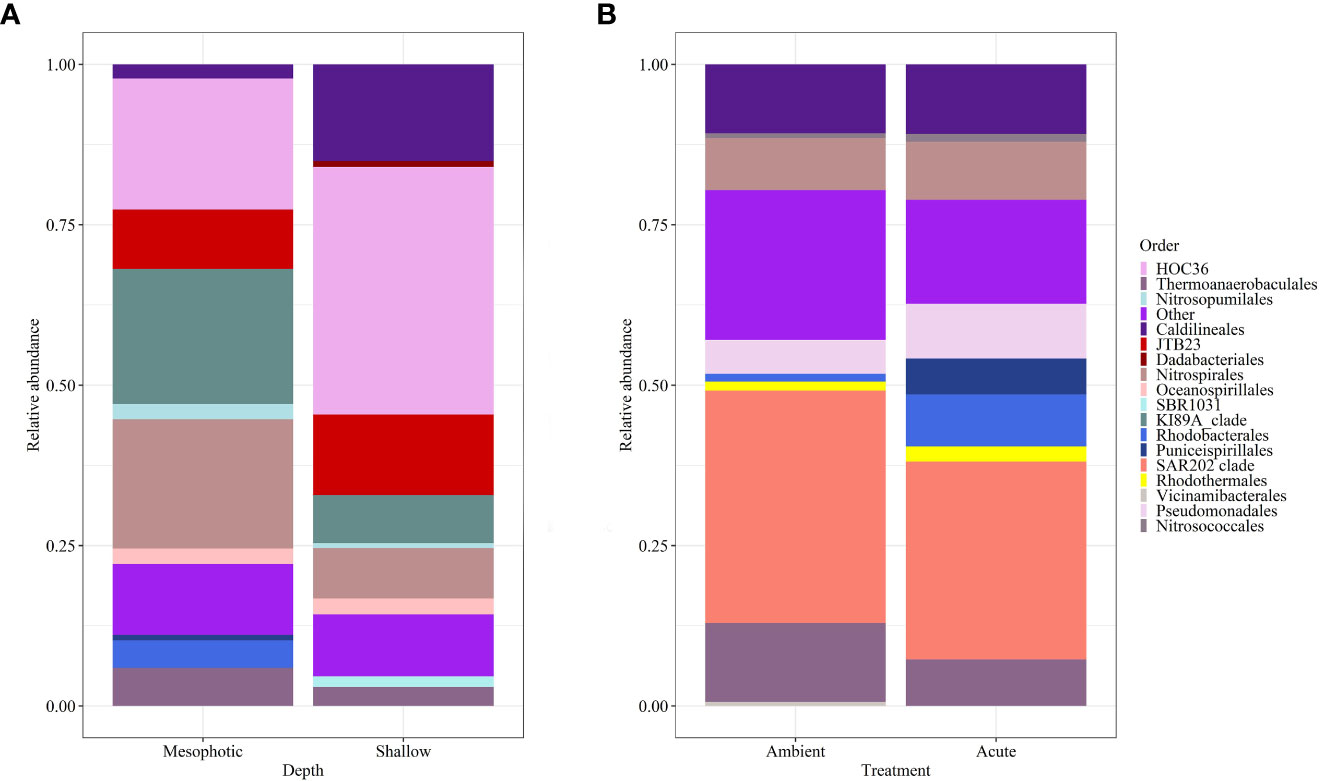
Figure 3 Relative abundance of D. erythraeanus microbial ASVs at the order level of (A) wild-type shallow vs. mesophotic sponges; and (B) sponges exposed to ambient and acute temperature treatment at the end of the experiment -Tf.
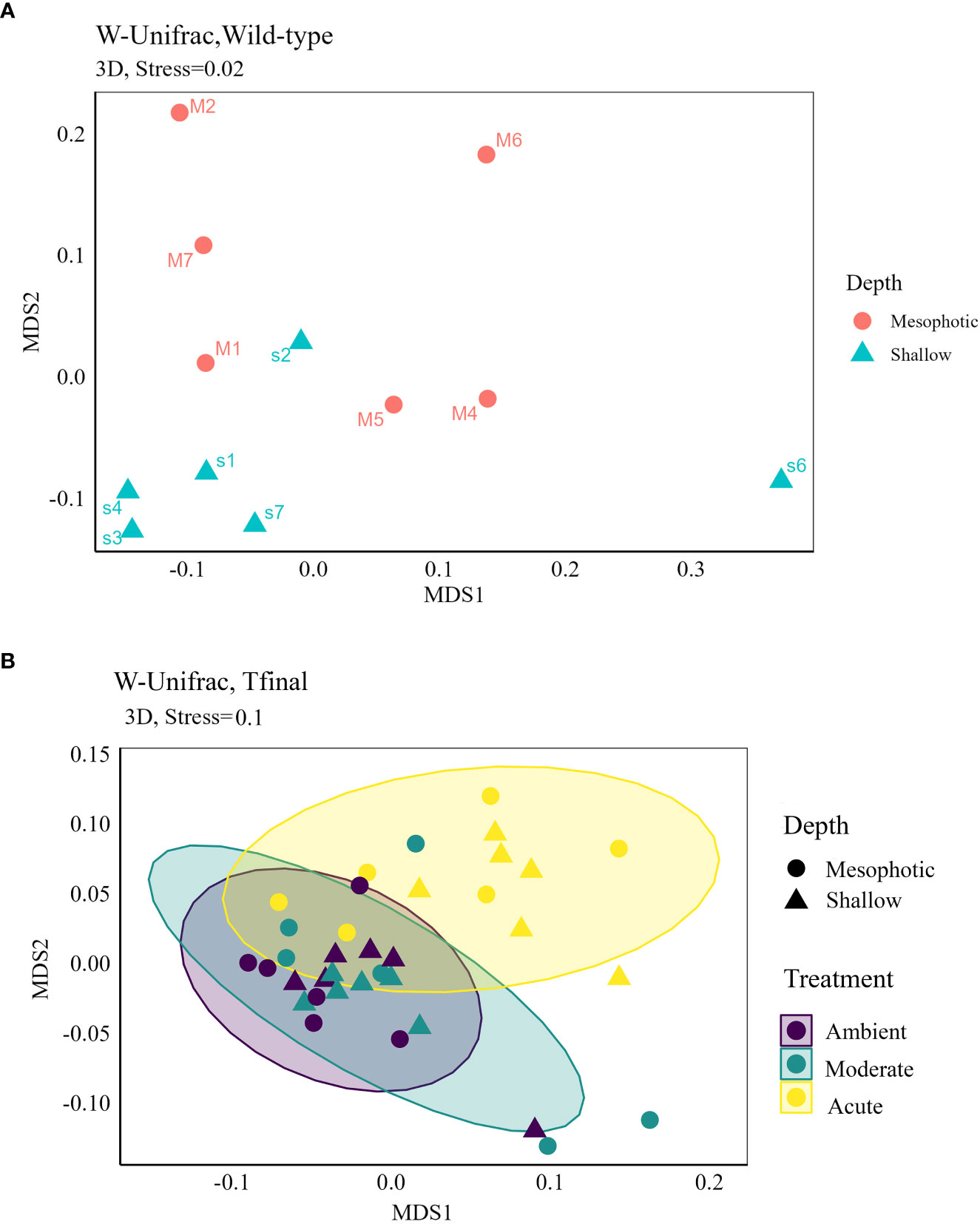
Figure 4 Non-metric multidimensional scaling (NMDS) 3D ordinations based on weighted UniFrac distance matrix of D. erythraeanus microbial community for (A) wild-type samples of shallow and mesophotic sponges; and (B) ambient, moderate and acute treatments of shallow and mesophotic sponges at Tf. Each dot represents one sponge sample (mesophotic=circle, shallow=triangle). See also Table 2 and Supplementary Table 4.
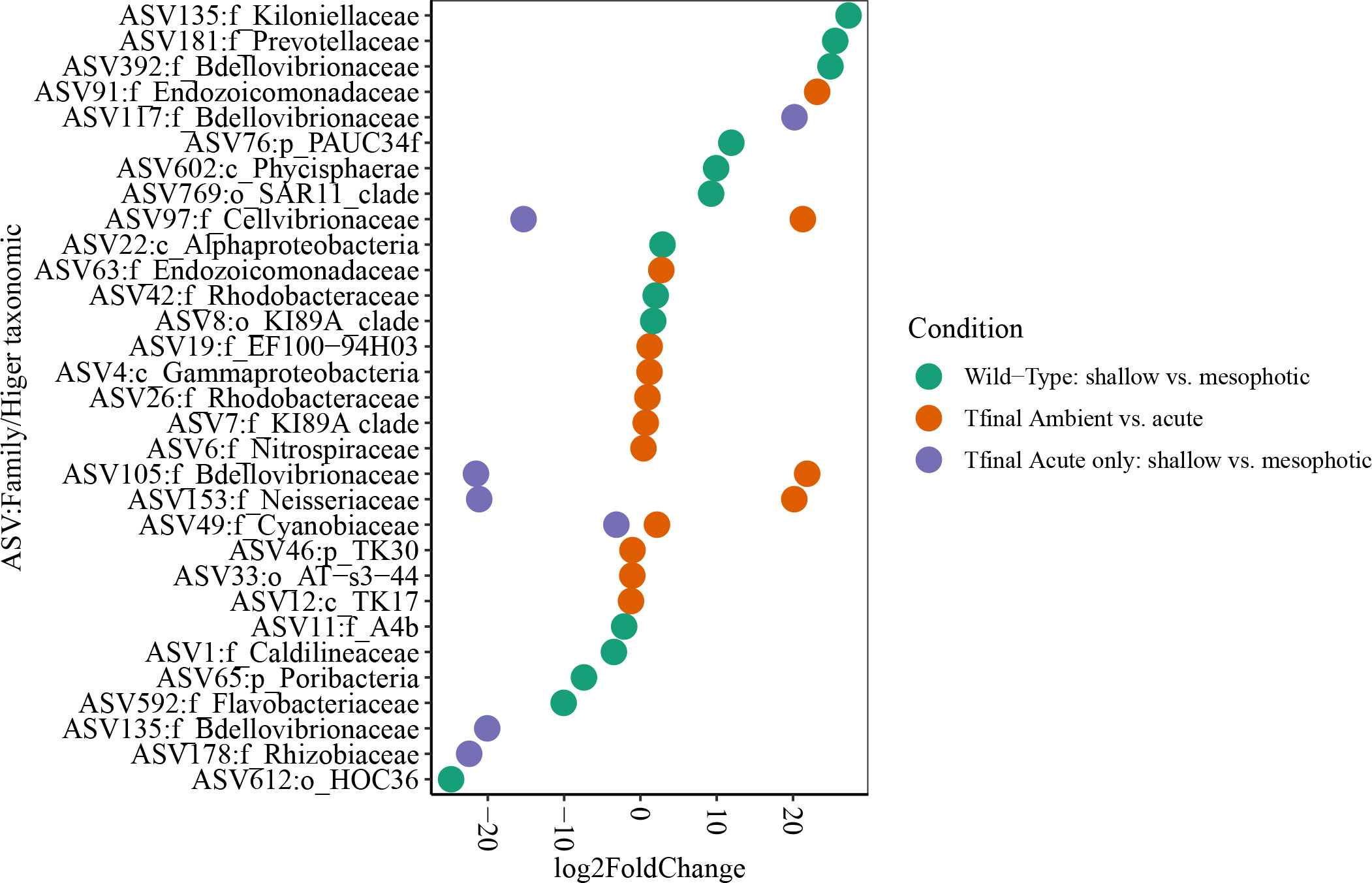
Figure 5 Differentially AVS plot (log fold change ≥ |2 |,Wald test, adjusted p. value ≤ 0.01) displayed at family (or higher) taxonomic level, in D. erythraeanus across depths and treatments. Colors indicate the different comparisons. Shallow sponges and ambient treatment (separately) were used as the reference group at each comparison.
Following the 5-month regeneration period and five-day acclimation at ambient temperature (24°C), and before any temperature increase in the aquaria (pre-experiment sampling point), the microbial communities of the shallow and mesophotic sponges did not differ substantially from each other. No significant differences were detected in diversity indices and microbial composition as a function of their depth origin (weighted UniFrac, R2 = 0.03, p=0.23, Table 2). Similarly, at T2, that is, two days after temperature in the aquaria had reached the target temperature values (26° vs 30 °C), the microbial diversity indices remained similar across temperature treatments and depth origin (Table 2; Supplementary Table 3A).
These results suggest that a 5-month regeneration period under identical field conditions caused the original microbiomes of mesophotic and shallow-water individuals, which differed initially (Figures 3A , 4A, weighted UniFrac, R2 = 0.21, p=0.009, Table 2) to shift and largely converge in composition and abundance.
Moreover, samples from the pre-experiment sampling-point and at T2 shared similar orders, primarily composed of SAR202 clade (27 vs. 30%, respectively), Caldilineales (15 vs. 14%), and Thermoanaerobaculales (10.7 vs. 10.2%, respectively). No unique pattern emerged in the bacterial orders’ identity or in their abundance between depths for the pre-experiment sampling-point, or in treatments between depths for the T2 samples, except for the order Thermoanaerobaculales, which was higher in the shallow samples, and Vicinamibacterales, which was unique to the shallow pre-experiment samples (Supplementary Figure 3).
At the end of the experiment, however, after 35 days, changes in the microbial diversity indices and community composition could be observed, mostly in sponges exposed to the acute temperature stress. Thus, at Tf, the richness and Shannon indices of the acute samples were significantly lower (142 ± 8.1 and 3.74 ± 0.03, respectively) compared to the moderate (162 ± 4.3, 3.78 ± 0.03) and ambient samples (162 ± 4.9, 3.8 ± 0.02), which were similar to one another (Figure 6, Table 2; Supplementary Tables 3A, B). Moreover, while the diversity indices of the mesophotic samples remained stable throughout the experiment under the acute treatment, those of the shallow samples significantly decreased (Table 2; Supplementary Table 3A). In addition, the microbial community composition of the acute treatment sponges significantly differed from the moderate treatment and ambient samples, which were similar to one another (Weighted UniFrac, Figure 4B, Table 2; Supplementary Table 4). For example, a significant increase in ASV abundance in the acute treatment sponges (compared to the ambient treatment) at Tf was associated with members of the families Endozoicomonadaceae (ASV63, ASV91), Nitrospiraceae (ASV6), Neisseriaceae (ASV153), Bdellovibrionaceae (ASV105), Cyanobiaceae (ASV49), Cellvibrionaceae (ASV97), and Rhodobacteraceae (ASV 26, Figure 5). A change in microbial composition of the acute vs. ambient sponge treatments at Tf was also observed in the bacterial orders. For example, the relative abundance of the orders Pseudomonadale and Rhodobacterale was higher in the acute treatment sponges (8.5%; 8% respectively) compared to the ambient samples (5.3%; 1% respectively). In contrast, the SAR202 clade was less abundant in the acute treatment samples (30%) compared to the ambient treatment ones (36%), as were the Thermoanaerobaculales (acute: 7%, ambient: 12%). In addition, at Tf only the acute samples possessed members of the order Puniceispirillales (5%), while Vicinamibacterales were present only in the ambient samples, although at low abundance (0.6%) (Figure 3B).
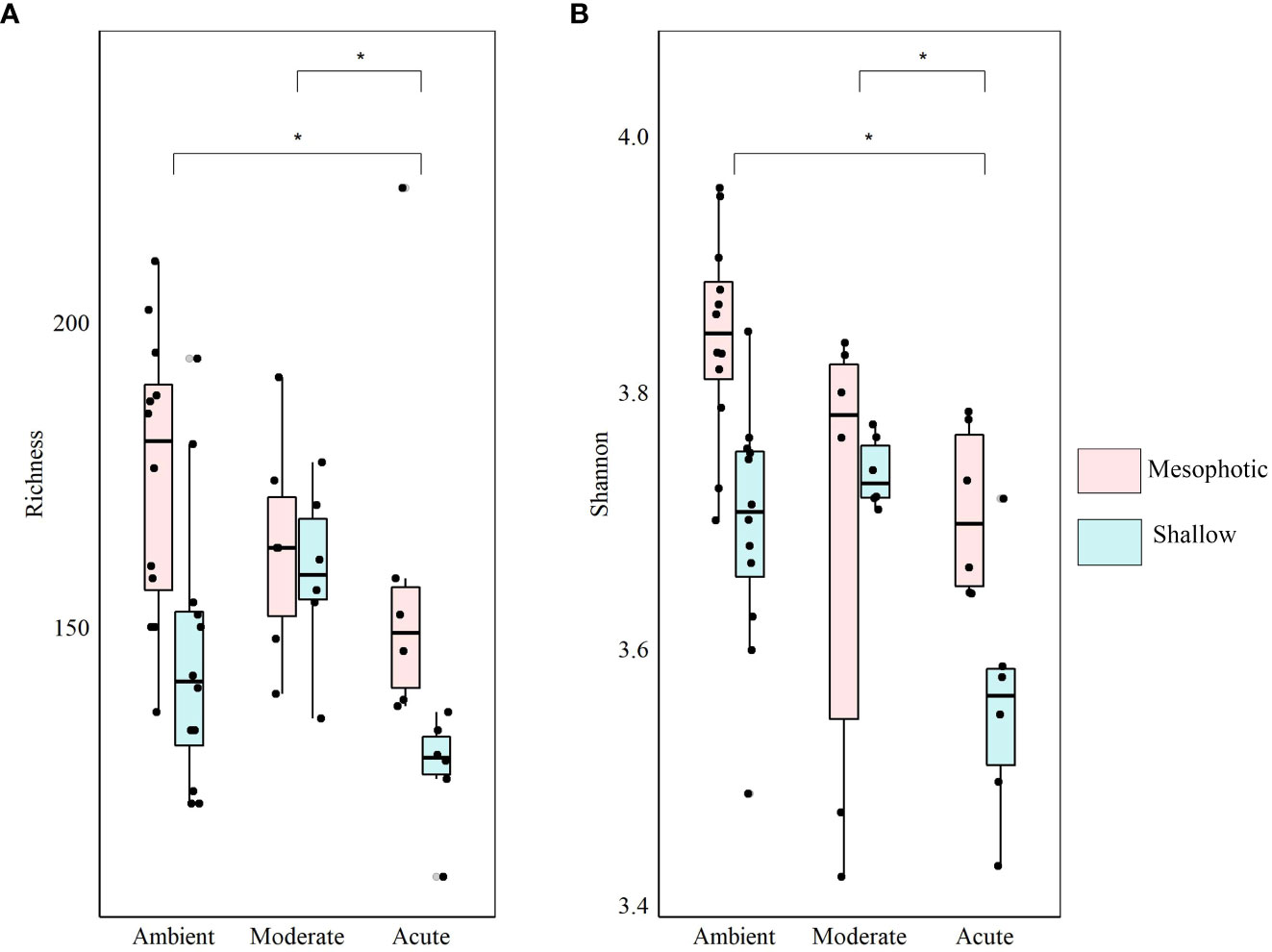
Figure 6 Diversity indices of shallow (light-blue) and mesophotic (pink) D. erythraeanus associated microbiome in clones exposed to ambient, moderate, or acute temperature treatment at Tf. (A) Richness; (B) Shannon diversity index. Median and 25-75 percent quartiles are indicated by the box. Dots are the diversity index values. Significant difference between treatments indicated by an asterisk. Mean values are provided in Supplementary Table S3A. The statistical inference is detailed in Table 2 and Supplementary Table S3B.
At Tf differences in the microbial composition were also associated with the sponge’s depth-origin (Weighted UniFrac R2 = 0.11, p<0.01, Figure 4B, Table 2; Supplementary Table 3A). Numerous bacterial members were found to significantly change in abundance between the shallow and mesophotic samples under the acute treatment. For example, the mesophotic samples were associated with a decreased abundance of members of the families Rhizobiaceae (ASV178), Neisseriaceae (ASV153), Bdellovibrionaceae (ASV105, 135), Cyanobiaceae (ASV49), and Cellvibrionaceae (ASV97), and an increased abundance of members of the family Bdellovibrionaceae (ASV117) (log-fold change, p<0.01, Figure 5). In addition, as previously described for the wild-type samples, also at Tf, the shallow and mesophotic samples in the acute treatment generally shared similar bacterial orders, with a few exceptions, and with differences in the orders’ relative abundance (Supplementary Figure 3). For example, Pseudomonadale and Puniceispirillale abundance was higher in the shallow samples (11%, 7% respectively) compared to the mesophotic ones (5%, 3%, respectively). In addition, Nitrosococcales were unique to the shallow samples and Caldilineales were generally higher in the mesophotic samples.
Finally, the sponge-host individual was found to be the most meaningful factor in shaping the microbial composition, both at T2 and Tf (Weighted UniFrac, R2 = 0.5, p<0.001; R2 = 0.4, p<0.001, respectively, Table 2). Nevertheless, for the pre-experiment sponges, the effect of sponge individual origin was not significant. It was, however, the only factor meaningfully contributing to the explained variance in microbial composition (R2 = 0.78, p=0.37, Table 2).
4 Discussion
We characterized the effects of temperature elevation on the common Red Sea sponge Diacarnus erythraeanus, as a holobiont, while comparing the responses of shallow vs. mesophotic populations. The results indicate that a temperature rise of up to 2°C (“moderate”), as predicted for the Gulf of Eilat/Aqaba in 2100 (Fine et al., 2013; Hoegh-Guldberg et al., 2014), will probably not have a detrimental effect on the D. erythraeanus holobiont. In addition, this sponge exhibited a high stress tolerance under the acute treatment as well, suggesting its survival even under the extremer climate scenarios for 2100 in this region. Moreover, while no difference in sponge survival or any external noticeable effect was detected, irrespective of collection depth, the microbiome of shallow vs. their mesophotic counterparts, responded differently. Although, during the 5-month regeneration period, the microbiomes of mesophotic and shallow-water individuals largely converged in diversity and composition, it is likely that characteristic members of each persisted in low abundance, proliferating differentially during the long-term acute temperature treatment to produce differential responses depending on depth origin.
4.1 Sponge physiological responses imply a tolerance to elevated temperatures
Our results suggest that a temperature increase of up to 6°C for 35 days might not affect the survival of D. erythraeanus, which survived throughout the experiment under the different thermal treatments. However, the experiment was conducted during the autumn, when the ambient temperature was generally lower (mean 5 m: 23.4°C, 45 m: 23.2°C) than the maximum temperature that these sponges experience seasonally during the summer (max temp 5 m: 28.9°C, 45 m: 27.1°C, Shlesinger et al., 2018; Liberman et al., 2022). Therefore, although the moderate temperature treatment (~26°C) exceeded the typical autumn temperatures that these sponges encounter, it was not beyond the temperature range they are exposed to throughout the year. Thus, it is not surprising that the moderate treatment had only a minor effect on the sponge physiology. In contrast, the acute treatment (~30°C) reached above the maximum temperature in present-day to which these sponges are exposed locally during the summer (see above). Although all the clones survived the experimental increased temperatures, changes were observed in their respiration and in their microbial composition. The respiration rate of D. erythraeanus clones in the acute treatment was significantly higher than those in the ambient and moderate treatments (Figure 2A, Table 1). Congruent with our findings, increased respiration rates have previously been reported for several sponge species exposed in situ to thermal stress (Coma, 2002), as well as in aquaria experimental systems (Strand et al., 2017; Beepat et al., 2020). It has been suggested that increased respiration in sponges acts as a coping mechanism under elevated temperature in order to maintain cellular homeostasis through the activation of intra-cellular repair and protection processes (Guzman and Conaco, 2016). This could also be the result of an increased carbon fixation rate by the sponge microsymbionts (Fang et al., 2014). Congruent with the respiration rate, in the middle of the experiment (days 15-20), the oxygen removal was significantly higher in the acute treatment compared to the ambient and moderate treatments. However, by the end of the experiment (days 28-35), the oxygen removal of sponges in the acute treatment had decreased and was similar to that of the ambient treatment sponges (Figure 2B, Table 1). This could imply, on the one hand, that D. erythraeanus might have become adapted to the high temperature stress; or, on the other hand, that the prolonged exposure to an elevated temperature might have resulted in a cumulative thermal stress, followed by physiological dysfunctioning (Rodolfo-Metalpa et al, 2014), with no external signs. Similarly, the respiration rate of the sponge Cliona orientalis was shown to be generally higher under an intermediate temperature stress (compared to the control), while sponges that were exposed to the high temperature treatment (32°C) and were bleached, presented a respiration rate similar to that of the control (Ramsby et al., 2018). Conversely, our D. erythraeanus specimens revealed no external signs of stress and their buoyant weight did not change during the experiment (Table 1), supporting the assumption that an adaptation mechanism, rather than sensitivity, underlay the decrease in the sponge’s oxygen removal consumption under the acute temperature treatment. It is possible, however, that the high spicule and collagen content of this species (Kelly and Samaai, 2002) might have obscured possible changes in tissue weight. Another possibility is that under a longer stress period (over 35 days), this sponge would eventually succumb to disease and possibly mortality. Further focused metabolic analysis, such as examination of the tissue metabolic profile (Strand et al., 2017; Ramsby et al., 2018) or gene expression analysis (Fan et al., 2013; Guzman and Conaco, 2016), could shed additional light on the sponge’s physiological condition following heat stress.
In addition, our findings indicate that the respiration rate of shallow and mesophotic sponges were similarly affected by the rise in temperature (Figure 2A, Table 1). A similar phenomenon was reported from four coral species in Bermuda, which demonstrated a similar thermal tolerance (including respiration rate) by both mesophotic and shallow individuals (Gould et al., 2021). The respiration rate of the Mediterranean sponge Chondrosia reniformis was also found to be depth-independent (Gökalp et al., 2020). Since both shallow and mesophotic individuals of D. erythraeanus exhibited similar rates of respiration under elevated temperature, it is plausible to assume that, in the case of D. erythraeanus, local adaptation toward thermal stress did not take place, at least at the sponge-host level. The latter conclusion is reinforced when considering the potential for vertical connectivity between the shallow and mesophotic populations in the Gulf of Aqaba (Berenshtein, 2018).
4.2 Sponge microbiome response to elevated temperature
4.2.1 Reduction in photo-symbiont functioning
Although none of the D. Erythraeanus clones bleached, a significant decline in photosynthetic efficiency (ΔF/FM) was observed in the sponges under acute temperature treatment (Figure 2C, Table 1). A decrease in photosynthesis in organisms experiencing heat stress has been documented in multiple studies on sponges (Cebrian et al., 2011; Bennett et al., 2017; Ramsby et al., 2018; Beepat et al., 2020). The reduced functioning of photosymbionts could further result in a potential reduction in the translocation of photosynthesis-derived products from the photosymbionts to the sponge-host (Wilkinson and Fay, 1979). This might severely affect the sponge’s energetic budget and threaten its continued survival. Various mechanisms have been suggested to compensate the host’s nutritional loss following a decline in photosymbiont functioning. For example, the high survivorship of the bleached sponge Xestospongia muta was attributed to its low nutritional dependence on photosymbionts, and greater reliance on heterotrophic sources (McMurray et al., 2011). Another mechanism was demonstrated in bleached individuals of the sponge Cliona orientalis, which used its lipid stores to overcome periods of low or no photoautotrophic-derived food-sources (Fang et al., 2014). Thus, it can be assumed that similar mechanisms are also involved in the case of D. Erythraeanus, enabling its high survival rate during a reduction in photosynthetic-derived nutrition sources.
4.2.2 Mesophotic and shallow microbial communities: general description
The bacterial community composition of the wild-type mesophotic sponges differed significantly from that of the shallow-reef sponges (Figure 4A, Table 2). These differences were mainly attributed to the relative abundance of the bacterial orders rather than their identity, which was similar at all depths (Figure 3A). These findings are in line with previous studies that compared microbial assemblages in relation to depth, and revealed similar patterns (Morrow et al., 2016; Indraningrat et al., 2022). Along the shallow to mesophotic depths the physical, biochemical, and biotic factors undergo change (Lesser et al., 2018). Some of those factors have been identified as meaningful drivers in shaping microbial communities (Busch et al., 2022). For example, temperature and light intensity decrease along shallow to mesophotic depths, while nutrient concentration is usually higher (Kahng et al., 2017; Lesser et al., 2018). All of those factors were previously indicated to drive changes in microbial communities composition (Olson and Gao, 2013; Morrow et al., 2016; Steffen et al., 2022). The shift in the bacterial composition with depth could therefore indicate the holobiont’s functional adjustment to the changing ecological conditions along this depth range (shallow and mesophotic). In the Gulf of Aqaba, light availability, temperature, sedimentation rate, and the hydrodynamic regimes differ between shallow and mesophotic habitats (Eyal et al., 2019). These environmental variations could significantly affect the microbial community of D. erythraeanus populations from different depths. Many bacterial members have been found to change in abundance between the wild-type shallow and mesophotic sponges. For example, ASV members of the order Caldilineales (Chloroflexi) were enriched in the wild-shallow samples, as found for the sponges X. muta and Agelas sventres (Indraningrat et al., 2022). In the mesophotic sponges, a higher abundance of Nitrospirota bacteria was found (Figure 3A). Considering the reduced light availability at the mesophotic depths (Kahng et al., 2019), it is plausible that the increased abundance of Nitrospirota could constitute a sort of “metabolic compensation”, whereby the photoautotrophy efficiency is reduced. In addition, sponges from the shallow and the mesophotic waters in the pre-experiment (post-acclimation) sampling possessed similar bacterial communities, as opposed to the distinct bacterial communities found in the wild-type shallow and mesophotic sponges, sampled immediately after collection (Figure 4A, Table 2). However, because the pre-experiment sampling occurred after a recovery (acclimation) period (~5 months) of the sponges at 10 m depth, it is possible that the transplantation of both the mesophotic and the shallow samples to the (shallow) recovery site, induced a microbiome transitioning towards microbial communities resembling those of the transplanted site. These results emphasize the flexibility of microorganisms in rapidly adapting to environmental changes (Reshef et al., 2006; Lesser et al., 2020; Voolstra and Ziegler, 2020; Maldonado et al., 2021; Vargas et al., 2021), while facilitating their sponge-host’s adjustment to the new conditions.
4.2.3 Microbiome adjustments under elevated temperature
Sponges rely on intimate associations with diverse microorganisms (Thomas et al., 2016; Webster and Thomas, 2016), which fulfill various crucial roles in the host’s functioning and stability (Pita et al., 2018; Engelberts et al., 2020; Moreno-Pino et al., 2020; Posadas et al., 2022; Schmittmann et al., 2022). In the present study, a meaningful change in the microbial community was observed only at the end of the experiment (Tf), and mainly under the acute temperature treatment (Figures 3-6, Tables 1, 2). Changes in microbial community diversity and composition under heat stress often result in deterioration of the holobiont’s physical condition, as previously documented in numerous sponge species (Fan et al., 2013; Lesser et al., 2016; Ramsby et al., 2018; Rubio-Portillo et al., 2021). In the current study, however, the external appearance of D. erythraeanus remained stable throughout the experiment, with no visible signs of bleaching or necrosis. It is thus plausible that the changes in the microbial community were part of a resilience process rather than a collapse (Pita et al., 2018; Vargas et al., 2021).
Our results indicate that under acute thermal stress the microbial community of D. erythraeanus undergoes restructuring, mostly through a change in the relative abundance of the ASVs (Figures 3B, 4B, 5). We found that while the increased abundance of some bacterial members might reflect sponge-host stress, other bacteria were found to potentially possess essential functions that could support sponge-host resilience under the acute elevated temperature. For example, the SAR202 clade (Chloroflexi) in sponges was suggested to have a potential for DOM recalcitrant degradation. In addition, this clade possesses biosynthetic pathways for cofactor biosynthesis (Thiamine) (Bayer et al., 2018), possibly supporting the host’s metabolism. SAR202 significantly decreased in abundance in the acute heat treatment sponges at the end of the experiment (LFC, p<0.01, Figure 5), possibly reflecting a nutritional impediment to the sponge-host. Other bacteria that might have negative effects on their sponge-host are the Neisseria (ASV153), which significantly increased in abundance under the acute heat treatment (LFC, p<0.01, Figure 5). Neisseria are recognized as pathogenic bacteria (Tinsley and Nassif, 1996; Livorsi et al., 2011) and were found to be predominant in coral tissue invaded by sponges (Thinesh et al., 2020). Nevertheless, except of Neisseria, no other pathogenic bacteria were detected in the microbiome of the acute or moderate temperature treatment sponges. As opposed to that, the members of the families Cyanobiaceae (ASV49), Endozoicomonadaceae and Nitrospira significantly increased in abundance in the acute-stressed sponges (ASV91, ASV63 and ASV6, LFC, p<0.01), possibly possessing key functions for the sponge-host’s stability under stress, such as increased carbon supply and nutrient cycling functions (Raina et al., 2009; Fiore et al., 2013; Morrow et al., 2015; Neave et al., 2016; Moitinho-Silva et al., 2017; Zhang et al., 2019).
Similarly, a dynamic relationship between the host and its associated microbiome under environmental stress, known as the “Coral probiotic hypothesis” (Reshef et al., 2006), was suggested to promote the coral holobiont’s resilience. In sponges, several studies reported a microbial shift following environmental stress, suggesting that these changes are involved in the host resilience (Morrow et al., 2015; Ribes et al., 2016; Webster and Reusch, 2017; Ribeiro et al., 2021; Vargas et al., 2021).
4.2.4 Possible different coping mechanisms underly the shallow and mesophotic sponges’ responses to elevated temperature
The microbial response of shallow sponges significantly differed from that of their mesophotic counterparts under elevated temperature stress. While the microbial diversity indices of the shallow sponges decreased throughout the experiment under the acute temperature treatment, those of the mesophotic sponges remained stable and similar to those of the ambient treatment sponges (Table 2; Supplementary Table 3A). The flexibility in microbial community response of the shallow and mesophotic sponges to elevated temperatures, suggests the existence of different underlying coping mechanisms for each sponge population (Guzman and Conaco, 2016). Whereas at the mesophotic depths the environmental conditions are relatively stable, shallow sponges (and hence their microbiome) often encounter environmental disturbances, such as increased temperature fluctuations (Lesser et al., 2009; Bongaerts et al., 2010; Kahng et al., 2010). A prior exposure to environmental stress (e.g., elevated temperatures) could improve the organism’s response to subsequent and more extreme exposures (Guzman and Conaco, 2016; Hilker et al., 2016; Hackerott et al., 2021). For example, in the sponge Haliclona tubifera, heat stress induced gene activation related to innate immunity and various processes such as initiation of cellular damage repair and heat shock proteins pathways (Guzman and Conaco, 2016). Likewise, the survivorship of the sponge Neopetrosia compacta to acidification and warming stress was related to changes in the sponge-host immune functions, microbiome stability and increased abundance of beneficial microbial groups (Posadas et al., 2022). Therefore, under chronic or sequentially occurring environmental stresses, microbial adjustments might act as a key feature in a sponge acclimation (Ribes et al., 2016; Pita et al., 2018; Turon et al., 2018; Voolstra and Ziegler, 2020; Marangon et al., 2021; Ribeiro et al., 2021; Vargas et al., 2021; Posadas et al., 2022; Schmittmann et al., 2022) and possibly even lead to population adaptation (Webster and Reusch, 2017). Thus, the observed shift in the microbial diversity indices of the shallow sponges in the present study, could be an expression of acclimatization or adaptation, following previous events of exposure to elevated temperatures in the shallow waters. In contrast, the stability of the microbial diversity indices in the mesophotic sponges, might suggest that their healthy condition was maintained by means of resistance mechanisms. Microbial resistance is considered to occur when a microbial community remains unchanged following perturbation (Moya and Ferrer, 2016; Zaneveld et al., 2017). Nevertheless, changes in the relative abundance of the microbial community were observed for both, the shallow and mesophotic sponges under elevated temperatures (Figures 4-6), indicating that both are resilient to such increases (Allison and Martiny, 2008; Comte et al., 2013).
Finally, our results indicate that the sponge-host individual (the original clonal individual sponge) was the most meaningful factor in shaping the microbial community’s structure, irrespective of the other factors examined in the current study (temperature, time, depth). Correspondly, host genotype was previously suggested to act as a main factor in shaping the microbial assemblage of a sponge’s microbiome (Easson and Thacker, 2014; Reveillaud et al., 2014; Chaib De Mares et al., 2017; Glasl et al., 2018).
5 Conclusion
We examined the holobiont sponge D. erythraeanus’s response to elevated temperature. The findings indicate that both shallow and mesophotic individuals of D. erythraeanus are highly tolerant to moderate temperature elevation of 2°C, as predicted for 2100 in the Red Sea (Hoegh-Guldberg et al., 2014), and acute heat stress of 6°C above the ambient. Furthermore, we posit that its high survival rate coincides with microbial adjustments, suggesting the essential role of the microbiome to the host’s adaptation and stability when facing significant environmental stressors. We found that both shallow and mesophotic sponges are tolerant to heat stress, hence, our hypothesis that mesophotic sponges will be less resilient to elevated temperature was not supported. However, our results suggest that their underlying coping mechanisms might differ. We argue that while D. erythraeanus mesophotic sponges are resistant to heat stress, their shallow water counterparts might have become adapted to elevated temperatures, following previous exposure to increased heat events in the shallow waters. Therefore, these results cast doubt on the potential function of mesophotic coral ecosystems (MCEs) as thermal refugia for some sponge species. While both shallow and mesophotic sponge individuals demonstrated high levels of heat tolerance, their variability in the microbial response to heat stress might reflect local ecological adaptation and questions the validity of MCEs as a refuge for shallow populations. The variability in the responses of these populations further highlights the importance of uncovering the mechanisms that structure the sponge-microbiome interactions, particularly in regard to the predicted climate change scenarios.
Data availability statement
The datasets presented in this study can be found in online repositories. The names of the repository/repositories and accession number(s) can be found below: https://www.ncbi.nlm.nih.gov/bioproject, PRJNA931995.
Author contributions
LR-N, LG, SS and MI conceived the idea. LR wrote the manuscript. All authors read, critically reviewed, and edited the manuscript. RM assisted with the physiological measurements and analysis. ZL conducted the samples sequencing. All authors contributed to the article and approved the submitted version.
Funding
This research was supported by the ISF-NSFC joint research program (Grant No. 2577/18 to MI & 31861143020 to ZL). LR was supported by the Lev Tzion scholarship from the Israeli council for higher education.
Acknowledgments
We acknowledge the invaluable support from the M. Fine Lab in providing access to the experimental system. Our special thanks go to Komet and T. Yamin for their assistance in maintaining the experiment. We would also like to express our sincere gratitude to the editor and reviewers for their major contributions in improving this manuscript. We appreciate N. Paz for the scientific editorial support and the lab members of Ilan’s lab for their support in both laboratory and fieldwork. Our gratitude also extends to the staff of the R/V Sam Rothberg and the Interuniversity Institute for Marine Sciences in Eilat (IUI) for their ongoing support and access to facilities. Finally, we would like to thank Dr. M. Lalzar and Prof. L. Steindler for their inspiring guidance in the result interpretation and microbiome analysis and Dr. T. Shlesinger for generously sharing the temperature measurements with us.
Conflict of interest
The authors declare that the research was conducted in the absence of any commercial or financial relationships that could be construed as a potential conflict of interest.
Publisher’s note
All claims expressed in this article are solely those of the authors and do not necessarily represent those of their affiliated organizations, or those of the publisher, the editors and the reviewers. Any product that may be evaluated in this article, or claim that may be made by its manufacturer, is not guaranteed or endorsed by the publisher.
Supplementary material
The Supplementary Material for this article can be found online at: https://www.frontiersin.org/articles/10.3389/fmars.2023.1161648/full#supplementary-material
References
Allaire J. (2012). RStudio: integrated development environment for r. (Boston, MA: RStudio Inc) 537, 538.
Allison S. D., Martiny J. B. (2008). Resistance, resilience, and redundancy in microbial communities. Proc. Natl. Acad. Sci. 105 (supplement_1), 11512–11519. doi: 10.1073/pnas.0801925105
Apprill A., McNally S., Parsons R., Weber L. (2015). Minor revision to V4 region SSU rRNA 806R gene primer greatly increases detection of SAR11 bacterioplankton. Aquat. Microbial Ecol. 75 (2), 129–137. doi: 10.3354/ame01753
Baker E., Thygesen K., Harris P., Andradi-Brown D., Appeldoorn R. S., Ballantine D., et al. (2016). Mesophotic coral ecosystems: a lifeboat for coral reefs? The United Nations Environment Programme (UNEP) and GRID-Arendal, Nairobi and Arendal, 98.
Bayer K., Jahn M. T., Slaby B. M., Moitinho-Silva L., Hentschel U. (2018). Marine sponges as chloroflexi hot spots: genomic insights and high-resolution visualization of an abundant and diverse symbiotic clade. MSystems 3 (6), e00150–e00118. doi: 10.1128/mSystems.00150-18
Beepat S. S., Davy S. K., Woods L., Bell J. J. (2020). Short-term responses of tropical lagoon sponges to elevated temperature and nitrate. Mar. Environ. Res. 157, 104922. doi: 10.1016/j.marenvres.2020.104922
Bell J. J. (2008). The functional roles of marine sponges. Estuarine Coast. shelf Sci. 79 (3), 341–353. doi: 10.1016/j.ecss.2008.05.002
Bellworthy J., Fine M. (2018). The red Sea simulator: a high-precision climate change mesocosm with automated monitoring for the long-term study of coral reef organisms. Limnology Oceanography: Methods 16 (6), 367–375. doi: 10.1002/lom3.10250
Bennett H. M., Altenrath C., Woods L., Davy S. K., Webster N. S., Bell J. J. (2017). Interactive effects of temperature and pCO 2 on sponges: from the cradle to the grave. Global Change Biol. 23 (5), 2031–2046. doi: 10.1111/gcb.13474
Berenshtein I. (2018). “Connectivity in the gulf of Aqaba/Eilat,” in Israel Nature and parks authority report, 27. doi: 10.13140/rg.2.2.17500.64643
Birkeland C. (2019). “Global status of coral reefs: in combination, disturbances and stressors become ratchets,” in World seas: an environmental evaluation (Amsterdam: Elsevier), 35–56.
Bongaerts P., Ridgway T., Sampayo E., Hoegh-Guldberg O. (2010). Assessing the ‘deep reef refugia’hypothesis: focus on Caribbean reefs. Coral reefs 29 (2), 309–327. doi: 10.1007/s00338-009-0581-x
Bosch T. C., McFall-Ngai M. J. (2011). Metaorganisms as the new frontier. Zoology 114 (4), 185–190. doi: 10.1016/j.zool.2011.04.001
Busch K., Slaby B. M., Bach W., Boetius A., Clefsen I., Colaço A., et al. (2022). Biodiversity, environmental drivers, and sustainability of the global deep-sea sponge microbiome. Nat. Commun. 13 (1), 1–16. doi: 10.1038/s41467-022-32684-4
Callahan B. J., McMurdie P. J., Rosen M. J., Han A. W., Johnson A. J. A., Holmes S. P. (2016). DADA2: high-resolution sample inference from illumina amplicon data. Nat. Methods 13 (7), 581–583. doi: 10.1038/nmeth.3869
Cebrian E., Uriz M. J., Garrabou J., Ballesteros E. (2011). Sponge mass mortalities in a warming Mediterranean Sea: are cyanobacteria-harboring species worse off? PloS One 6 (6), e20211. doi: 10.1371/journal.pone.0020211
Chaib De Mares M., Sipkema D., Huang S., Bunk B., Overmann J., Van Elsas J. D. (2017). Host specificity for bacterial, archaeal and fungal communities determined for high-and low-microbial abundance sponge species in two genera. Front. Microbiol. 8, 2560. doi: 10.3389/fmicb.2017.02560
Coma R. (2002). Seasonality of in situ respiration rate in three temperate benthic suspension feeders. Limnology oceanography 47 (1), 324–331. doi: 10.4319/lo.2002.47.1.0324
Comte J., Fauteux L., Giorgio P. (2013). Links between metabolic plasticity and functional redundancy in freshwater bacterioplankton communities. Front. Microbiol. 4, 112. doi: 10.3389/fmicb.2013.00112
Diaz M. C., Rützler K. (2001). Sponges: an essential component of Caribbean coral reefs. Bull. Mar. Sci. 69 (2), 535–546.
Easson C. G., Thacker R. W. (2014). Phylogenetic signal in the community structure of host-specific microbiomes of tropical marine sponges. Front. Microbiol. 5, 532. doi: 10.3389/fmicb.2014.00532
Engelberts J. P., Robbins S. J., de Goeij J. M., Aranda M., Bell S. C., Webster N. S. (2020). Characterization of a sponge microbiome using an integrative genome-centric approach. ISME J. 14 (5), 1100–1110. doi: 10.1038/s41396-020-0591-9
Eyal G., Tamir R., Kramer N., Eyal-Shaham L., Loya Y. (2019). “The red sea: Israel,” in Mesophotic coral ecosystems. Eds. Loya Y., Puglise K. A., Bridge T. C. L. (New York: Springer), 199–214.
Fan L., Liu M., Simister R., Webster N. S., Thomas T. (2013). Marine microbial symbiosis heats up: the phylogenetic and functional response of a sponge holobiont to thermal stress. ISME J. 7 (5), 991. doi: 10.1038/ismej.2012.165
Fang J. K., Schönberg C. H., Mello-Athayde M. A., Hoegh-Guldberg O., Dove S. (2014). Effects of ocean warming and acidification on the energy budget of an excavating sponge. Global Change Biol. 20 (4), 1043–1054. doi: 10.1111/gcb.12369
Fine M., Gildor H., Genin A. (2013). A coral reef refuge in the red Sea. Global Change Biol. 19 (12), 3640–3647. doi: 10.1111/gcb.12356
Fiore C. L., Jarett J. K., Lesser M. P. (2013). Symbiotic prokaryotic communities from different populations of the giant barrel sponge, Xestospongia muta. Microbiologyopen 2 (6), 938–952. doi: 10.1002/mbo3.135
Forster P., Storelvmo T., Armour K., Collins W., Dufresne J. L., Frame D., et al. (2021). “Earth’s energy budget, climate feedbacks, and climate sensitivity,” in Climate change 2021: the physical science basis. contribution of working group I to the sixth assessment report of the intergovernmental panel on climate change. Eds. Masson-Delmotte V., et al (Cambridge Univ. Press), 923–1054.
Frölicher T. L., Fischer E. M., Gruber N. (2018). Marine heatwaves under global warming. Nature 560 (7718), 360–364. doi: 10.1038/s41586-018-0383-9
Frossard J., Renaud O. (2021). Permutation tests for regression, ANOVA, and comparison of signals: the permuco package. J. Stat. Software 99, 1–32. doi: 10.18637/jss.v099.i15
Glasl B., Smith C. E., Bourne D. G., Webster N. S. (2018). Exploring the diversity-stability paradigm using sponge microbial communities. Sci. Rep. 8 (1), 1–9. doi: 10.1038/s41598-018-26641-9
Gökalp M., Kooistra T., Rocha M. S., Silva T. H., Osinga R., Murk A. J., et al. (2020). The effect of depth on the morphology, bacterial clearance, and respiration of the mediterranean sponge chondrosia reniformis (Nardo 1847). Mar. Drugs 18 (7), 358. doi: 10.3390/md18070358
Gould K., Bruno J. F., Ju R., Goodbody-Gringley G. (2021). Upper-mesophotic and shallow reef corals exhibit similar thermal tolerance, sensitivity and optima. Coral Reefs 40 (3), 907–920. doi: 10.1007/s00338-021-02095-w
Grottoli A. G., Tchernov D., Winters G. (2017). Physiological and biogeochemical responses of super-corals to thermal stress from the northern gulf of aqaba, red Sea. Front. Mar. Sci. 215. doi: 10.3389/fmars.2017.00215
Guzman C., Conaco C. (2016). Gene expression dynamics accompanying the sponge thermal stress response. PloS One 11 (10), e0165368. doi: 10.1371/journal.pone.0165368
Hackerott S., Martell H. A., Eirin-Lopez J. M. (2021). Coral environmental memory: causes, mechanisms, and consequences for future reefs. Trends Ecol. Evol. 36 (11), 1011–1023. doi: 10.1016/j.tree.2021.06.014
Hentschel U., Piel J., Degnan S. M., Taylor M. W. (2012). Genomic insights into the marine sponge microbiome. Nat. Rev. Microbiol. 10 (9), 641–654. doi: 10.1038/nrmicro2839
Hilker M., Schwachtje J., Baier M., Balazadeh S., Bäurle I., Geiselhardt S., et al. (2016). Priming and memory of stress responses in organisms lacking a nervous system. Biol. Rev. 91 (4), 1118–1133. doi: 10.1111/brv.12215
Hoegh-Guldberg O., Cai R., Poloczanska E. S., Brewer P. G., Sundby S., Hilmi K., et al. (2014). “The ocean,” in Climate change 2014: impacts, adaptation, and vulnerability. part b: regional aspects. contributionof working group II to the fifth assessment report of the intergovernmental panel on climate change. Eds. Barros V. R., Field C. B., Dokken D. J., Mastrandrea M. D., Mach K. J., Bilir T. E., Chatterjee M., Ebi K. L., Estrada Y. O., Genova R. C., Girma B., Kissel E. S., Levy A. N., MacCracken S., Mastrandrea P. R., White L. L. (Cambridge, United Kingdom and New York, NY, USA: Cambridge University Press), 1655–1731.
Hoegh-Guldberg O., Mumby P. J., Hooten A. J., Steneck R. S., Greenfield P., Gomez E., et al. (2007). Coral reefs under rapid climate change and ocean acidification. science 318 (5857), 1737–1742. doi: 10.1126/science.1152509
Hughes T. P., Anderson K. D., Connolly S. R., Heron S. F., Kerry J. T., Lough J. M., et al. (2018a). Spatial and temporal patterns of mass bleaching of corals in the anthropocene. Science 359 (6371), 80–83. doi: 10.1126/science.aan8048
Hughes T. P., Kerry J. T., Baird A. H., Connolly S. R., Dietzel A., Eakin C. M., et al. (2018b). Global warming transforms coral reef assemblages. Nature 556 (7702), 492–496. doi: 10.1038/s41586-018-0041-2
Idan T., Goren L., Shefer S., Ilan M. (2020). Sponges in a changing climate: survival of Agelas oroides in a warming Mediterranean Sea. Front. Mar. Science. 7. doi: 10.3389/fmars.2020.603593
Indraningrat A. A. G., Steinert G., Becking L. E., Mueller B., de Goeij J. M., Smidt H., et al. (2022). Sponge holobionts shift their prokaryotic communities and antimicrobial activity from shallow to lower mesophotic depths. Antonie van Leeuwenhoek 115, 1265–1283. doi: 10.1007/s10482-022-01770-4
IPCC (2023). Summary for policymakers. In limate change 2023: synthesis report. A report of the intergovernmental panel on climate change. Contribution of working groups I, II and III to the sixth assessment report of the intergovernmental panel on climate change Core Writing Team, Lee H., Romero J. eds. (Geneva, Switzerland: IPCC), 36. (in press).
Kahng S. E., Akkaynak D., Shlesinger T., Hochberg E. J., Wiedenmann J., Tamir R., et al. (2019). “Light, temperature, photosynthesis, heterotrophy, and the lower depth limits of mesophotic coral ecosystems,” in Mesophotic Coral Ecosystems. Coral Reefs of the World. Eds. Loya Y., Puglise K., Bridge T. (Cham: Springer) 12, 801–828.
Kahng S., Copus J. M., Wagner D. (2017). Mesophotic coral ecosystems. Mar. Anim. forests 10, 978–973. doi: 10.1007/978-3-319-21012-4_4
Kahng S., Garcia-Sais J., Spalding H., Brokovich E., Wagner D., Weil E., et al. (2010). Community ecology of mesophotic coral reef ecosystems. Coral Reefs 29 (2), 255–275. doi: 10.1007/s00338-010-0593-6
Kelly M., Samaai T. (2002). “Family podospongiidae de laubenfel,” in Systema Porifera (New York: Springer), 694–702. doi: 10.1007/978-1-4615-0747-5_75
Kelly-Borges M., Vacelet J. (1995). A revision of Diacamus Burton and Negombata de laubenfels (Demospongiae: latrunculiidae) with descriptions of new species from the west central pacific and the red Sea. Memoirs-Queensland Museum 38, 477–504.
Kramer N., Eyal G., Tamir R., Loya Y. (2019). Upper mesophotic depths in the coral reefs of eilat, red Sea, offer suitable refuge grounds for coral settlement. Sci. Rep. 9 (1), 1–12. doi: 10.1038/s41598-019-38795-1
Lesser M. P., Fiore C., Slattery M., Zaneveld J. (2016). Climate change stressors destabilize the microbiome of the Caribbean barrel sponge, Xestospongia muta. J. Exp. Mar. Biol. Ecol. 475, 11–18. doi: 10.1016/j.jembe.2015.11.004
Lesser M. P., Mueller B., Pankey M. S., Macartney K. J., Slattery M., de Goeij J. M. (2020). Depth-dependent detritus production in the sponge, Halisarca caerulea. Limnology Oceanography 65 (6), 1200–1216. doi: 10.1002/lno.11384
Lesser M. P., Slattery M., Leichter J. J. (2009). Ecology of mesophotic coral reefs. J. Exp. Mar. Biol. Ecol. 375 (1-2), 1–8. doi: 10.1016/j.jembe.2009.05.009
Lesser M. P., Slattery M., Mobley C. D. (2018). Biodiversity and functional ecology of mesophotic coral reefs. Annu. Rev. Ecology Evolution Systematics 49 (1), 49–71. doi: 10.1146/annurev-ecolsys-110617-062423
Liberman R., Shlesinger T., Loya Y., Benayahu Y. (2022). Soft coral reproductive phenology along a depth gradient: can “going deeper” provide a viable refuge? Ecology 103 (9), e3760. doi: 10.1002/ecy.3760
Livorsi D. J., Stenehjem E., Stephens D. S. (2011). Virulence factors of gram-negative bacteria in sepsis with a focus on Neisseria meningitidis. Sepsis-Pro-Inflammatory Anti-Inflammatory Responses 17, 31–47. doi: 10.1159/000324008
Love M. I., Huber W., Anders S. (2014). Moderated estimation of fold change and dispersion for RNA-seq data with DESeq2. Genome Biol. 15 (12), 1–21. doi: 10.1186/s13059-014-0550-8
Loya Y. (2004). “The coral reefs of eilat–past, present and future: three decades of coral community structure studies,” in: (Eds.) Rosenberg E., Loya Y. Coral Reef Health and Disease (Berlin: Springer), 1–34.
Luter H. M., Andersen M., Versteegen E., Laffy P., Uthicke S., Bell J. J., et al. (2020). Cross-generational effects of climate change on the microbiome of a photosynthetic sponge. Environ. Microbiol. 22 (11), 4732–4744. doi: 10.1111/1462-2920.15222
Maldonado M., López-Acosta M., Busch K., Slaby B. M., Bayer K., Beazley L., et al. (2021). A microbial nitrogen engine modulated by bacteriosyncytia in hexactinellid sponges: ecological implications for deep-sea communities. Front. Mar. Sci. 8, 638505. doi: 10.3389/fmars.2021.638505
Mangiafico S., Mangiafico M. S. (2017). Package ‘rcompanion’. Cran Repos 20, 1–71. Available at: https://CRAN.R-project.org/package=rcompanion.
Marangon E., Laffy P. W., Bourne D. G., Webster N. S. (2021). Microbiome-mediated mechanisms contributing to the environmental tolerance of reef invertebrate species. Mar. Biol. 168 (6), 1–18. doi: 10.1007/s00227-021-03893-0
McMurdie P. J., Holmes S. (2013). Phyloseq: an r package for reproducible interactive analysis and graphics of microbiome census data. PloS One 8 (4), e61217. doi: 10.1371/journal.pone.0061217
McMurray S. E., Blum J. E., Leichter J. J., Pawlik J. R. (2011). Bleaching of the giant barrel sponge Xestospongia muta in the Florida keys. Limnology Oceanography 56 (6), 2243–2250. doi: 10.4319/lo.2011.56.6.2243
Moitinho-Silva L., Díez-Vives C., Batani G., Esteves A. I., Jahn M. T., Thomas T. (2017). Integrated metabolism in sponge–microbe symbiosis revealed by genome-centered metatranscriptomics. ISME J. 11 (7), 1651–1666. doi: 10.1038/ismej.2017.25
Moreno-Pino M., Cristi A., Gillooly J. F., Trefault N. (2020). Characterizing the microbiomes of Antarctic sponges: a functional metagenomic approach. Sci. Rep. 10 (1), 1–12. doi: 10.1038/s41598-020-57464-2
Morganti T. M., Ribes M., Yahel G., Coma R. (2019). Size is the major determinant of pumping rates in marine sponges. Front. Physiol. 10. doi: 10.3389/fphys.2019.01474
Morrow K. M., Bourne D. G., Humphrey C., Botté E. S., Laffy P., Zaneveld J., et al. (2015). Natural volcanic CO 2 seeps reveal future trajectories for host–microbial associations in corals and sponges. ISME J. 9 (4), 894–908. doi: 10.1038/ismej.2014.188
Morrow K. M., Fiore C. L., Lesser M. P. (2016). Environmental drivers of microbial community shifts in the giant barrel sponge, Xestospongia muta, over a shallow to mesophotic depth gradient. Environ. Microbiol. 18 (6), 2025–2038. doi: 10.1111/1462-2920.13226
Moskovish R., Diga R., Ilan M., Yahel G. (2022). High variability and enhanced nocturnal oxygen uptake in coral-reef sponges. Limnol. Oceanogr. doi: 10.1002/lno.12361
Moya A., Ferrer M. (2016). Functional redundancy-induced stability of gut microbiota subjected to disturbance. Trends Microbiol. 24 (5), 402–413. doi: 10.1016/j.tim.2016.02.002
Muñiz-Castillo A. I., Rivera-Sosa A., Chollett I., Eakin C. M., Andrade-Gómez L., McField M., et al. (2019). Three decades of heat stress exposure in Caribbean coral reefs: a new regional delineation to enhance conservation. Sci. Rep. 9 (1), 1–14. doi: 10.1038/s41598-019-47307-0
Neal B., Condit C., Liu G., dos Santos S., Kahru M., Mitchell B., et al. (2014). When depth is no refuge: cumulative thermal stress increases with depth in bocas del toro, Panama. Coral Reefs 33, 193–205. doi: 10.1007/s00338-013-1081-6
Neave M. J., Apprill A., Ferrier-Pagès C., Voolstra C. R. (2016). Diversity and function of prevalent symbiotic marine bacteria in the genus endozoicomonas. Appl. Microbiol. Biotechnol. 100 (19), 8315–8324. doi: 10.1007/s00253-016-7777-0
Nir O., Gruber D. F., Shemesh E., Glasser E., Tchernov D. (2014). Seasonal mesophotic coral bleaching of Stylophora pistillata in the northern red Sea. PloS One 9 (1), e84968. doi: 10.1371/journal.pone.0084968
Oksanen J., Blanchet F. G., Kindt R., Legendre P., Minchin P. R., O’hara R., et al. (2013). Package ‘vegan’. Community Ecol. package version 2 (9). Available at: https://github.com/vegandevs/vegan.
Oliver E. C., Donat M. G., Burrows M. T., Moore P. J., Smale D. A., Alexander L. V., et al. (2018). Longer and more frequent marine heatwaves over the past century. Nat. Commun. 9 (1), 1–12. doi: 10.1038/s41467-018-03732-9
Olson J. B., Gao X. (2013). Characterizing the bacterial associates of three Caribbean sponges along a gradient from shallow to mesophotic depths. FEMS Microbiol. Ecol. 85 (1), 74–84. doi: 10.1111/1574-6941.12099
Oren M., Steindler L., Ilan M. (2005). Transmission, plasticity and the molecular identification of cyanobacterial symbionts in the red Sea sponge Diacarnus erythraenus. Mar. Biol. 148 (1), 35–41. doi: 10.1007/s00227-005-0064-8
Osinga R. (1999). Measurement of sponge growth by projected body area and underwater weight. Mem Queense Mus 44, 419–426.
Parada A. E., Needham D. M., Fuhrman J. A. (2016). Every base matters: assessing small subunit rRNA primers for marine microbiomes with mock communities, time series and global field samples. Environ. Microbiol. 18 (5), 1403–1414. doi: 10.1111/1462-2920.13023
Pérez-Rosales G., Rouzé H., Torda G., Bongaerts P., Pichon M., Consortium U. T. P., et al. (2021). Mesophotic coral communities escape thermal coral bleaching in French Polynesia. R. Soc. Open Sci. 8 (11), 210139. doi: 10.1098/rsos.210139
Pita L., Rix L., Slaby B. M., Franke A., Hentschel U. (2018). The sponge holobiont in a changing ocean: from microbes to ecosystems. Microbiome 6 (1), 1–18. doi: 10.1186/s40168-018-0428-1
Pohlert T., Pohlert M. T. (2018). Package ‘PMCMRplus’ (Vienna, Austria: R Foundation for Statistical Computing). Available at: https://cran.r-project.org/package=PMCMRplus.
Posadas N., Baquiran J. I. P., Nada M. A. L., Kelly M., Conaco C. (2022). Microbiome diversity and host immune functions influence survivorship of sponge holobionts under future ocean conditions. ISME J. 16 (1), 58–67. doi: 10.1038/s41396-021-01050-5
Quast C., Pruesse E., Yilmaz P., Gerken J., Schweer T., Yarza P., et al. (2012). The SILVA ribosomal RNA gene database project: improved data processing and web-based tools. Nucleic Acids Res. 41 (D1), D590–D596. doi: 10.1093/nar/gks1219
Raina J.-B., Tapiolas D., Willis B. L., Bourne D. G. (2009). Coral-associated bacteria and their role in the biogeochemical cycling of sulfur. Appl. Environ. Microbiol. 75 (11), 3492–3501. doi: 10.1128/AEM.02567-08
Ramsby B. D., Hoogenboom M. O., Smith H. A., Whalan S., Webster N. S. (2018). The bioeroding sponge Cliona orientalis will not tolerate future projected ocean warming. Sci. Rep. 8 (1), 1–13. doi: 10.1038/s41598-018-26535-w
Reshef L., Koren O., Loya Y., Zilber-Rosenberg I., Rosenberg E. (2006). The coral probiotic hypothesis. Environ. Microbiol. 8 (12), 2068–2073. doi: 10.1111/j.1462-2920.2006.01148.x
Reveillaud J., Maignien L., Eren A. M., Huber J. A., Apprill A., Sogin M. L., et al. (2014). Host-specificity among abundant and rare taxa in the sponge microbiome. ISME J. 8 (6), 1198–1209. doi: 10.1038/ismej.2013.227
Ribeiro B., Padua A., Barno A., Villela H., Duarte G., Rossi A., et al. (2021). Assessing skeleton and microbiome responses of a calcareous sponge under thermal and pH stresses. ICES J. Mar. Sci. 78 (3), 855–866. doi: 10.1093/icesjms/fsaa231
Ribes M., Calvo E., Movilla J., Logares R., Coma R., Pelejero C. (2016). Restructuring of the sponge microbiome favors tolerance to ocean acidification. Environ. Microbiol. Rep. 8 (4), 536–544. doi: 10.1111/1758-2229.12430
Rix L., de Goeij J. M., van Oevelen D., Struck U., Al-Horani F. A., Wild C., et al. (2018). Reef sponges facilitate the transfer of coral-derived organic matter to their associated fauna via the sponge loop. Mar. Ecol. Prog. Ser. 589, 85–96. doi: 10.3354/meps12443
Rodolfo-Metalpa R., Hoogenboom M. O., Rottier C., Ramos-Esplá A., Baker A. C., Fine M., et al. (2014). Thermally tolerant corals have limited capacity to acclimatize to future warming. Global Change Biol. 20 (10), 3036–3049. doi: 10.1111/gcb.12571
Rondon R., González-Aravena M., Font A., Osorio M., Cárdenas C. A. (2020). Effects of climate change stressors on the prokaryotic communities of the Antarctic sponge Isodictya kerguelenensis. Front. Ecol. Evol. 262. doi: 10.3389/fevo.2020.00262
Rubio-Portillo E., Ramos-Esplá A. A., Antón J. (2021). Shifts in marine invertebrate bacterial assemblages associated with tissue necrosis during a heat wave. Coral Reefs 40 (2), 395–404. doi: 10.1007/s00338-021-02075-0
Schmittmann L., Rahn T., Busch K., Fraune S., Pita L., Hentschel U. (2022). Stability of a dominant sponge-symbiont in spite of antibiotic-induced microbiome disturbance. Environ. Microbiol. 24 (12), 6392–6410. doi: 10.1111/1462-2920.16249
Shlesinger T., Grinblat M., Rapuano H., Amit T., Loya Y. (2018). Can mesophotic reefs replenish shallow reefs? reduced coral reproductive performance casts a doubt. Ecology 99 (2), 421–437. doi: 10.1002/ecy.2098
Simister R., Taylor M. W., Tsai P., Fan L., Bruxner T. J., Crowe M. L., et al. (2012). Thermal stress responses in the bacterial biosphere of the great barrier reef sponge, Rhopaloeides odorabile. Environ. Microbiol. 14 (12), 3232–3246. doi: 10.1111/1462-2920.12010
Steffen K., Indraningrat A. A. G., Erngren I., Haglöf J., Becking L. E., Smidt H., et al. (2022). Oceanographic setting influences the prokaryotic community and metabolome in deep-sea sponges. Sci. Rep. 12 (1), 1–16. doi: 10.1038/s41598-022-07292-3
Strand R., Whalan S., Webster N. S., Kutti T., Fang J. K.-H., Luter H. M., et al. (2017). The response of a boreal deep-sea sponge holobiont to acute thermal stress. Sci. Rep. 7 (1), 1–12. doi: 10.1038/s41598-017-01091-x
Taylor J. A., Palladino G., Wemheuer B., Steinert G., Sipkema D., Williams T. J., et al. (2021). Phylogeny resolved, metabolism revealed: functional radiation within a widespread and divergent clade of sponge symbionts. ISME J. 15 (2), 503–519. doi: 10.1038/s41396-020-00791-z
Thinesh T., Meenatchi R., Lipton A. N., Anandham R., Jose P. A., Tang S.-L., et al. (2020). Metagenomic sequencing reveals altered bacterial abundance during coral-sponge interaction: insights into the invasive process of coral-killing sponge Terpios hoshinota. Microbiological Res. 240, 126553. doi: 10.1016/j.micres.2020.126553
Thomas T., Moitinho-Silva L., Lurgi M., Björk J. R., Easson C., Astudillo-García C., et al. (2016). Diversity, structure and convergent evolution of the global sponge microbiome. Nat. Commun. 7, 11870. doi: 10.1038/ncomms11870
Tinsley C., Nassif X. (1996). Analysis of the genetic differences between Neisseria meningitidis and Neisseria gonorrhoeae: two closely related bacteria expressing two different pathogenicities. Proc. Natl. Acad. Sci. 93 (20), 11109–11114. doi: 10.1073/pnas.93.20.11109
Turner J. A., Andradi-Brown D. A., Gori A., Bongaerts P., Burdett H. L., Ferrier-Pagès C., et al. (2019). “Key questions for research and conservation of mesophotic coral ecosystems and temperate mesophotic ecosystems,” in Mesophotic coral ecosystems: Coral reefs of the world. Eds. Loya Y., Puglise K., Bridge T. (Cham: Springer) 12, 989–1003.
Turon M., Cáliz J., Garate L., Casamayor E. O., Uriz M. J. (2018). Showcasing the role of seawater in bacteria recruitment and microbiome stability in sponges. Sci. Rep. 8 (1), 1–10. doi: 10.1038/s41598-018-33545-1
Vargas S., Leiva L., Wörheide G. (2021). Short-term exposure to high-temperature water causes a shift in the microbiome of the common aquarium sponge Lendenfeldia chondrodes. Microbial Ecol. 81 (1), 213–222. doi: 10.1007/s00248-020-01556-z
Voolstra C. R., Ziegler M. (2020). Adapting with microbial help: microbiome flexibility facilitates rapid responses to environmental change. BioEssays 42 (7), 2000004. doi: 10.1002/bies.202000004
Wang Q., Garrity G. M., Tiedje J. M., Cole J. R. (2007). Naive Bayesian classifier for rapid assignment of rRNA sequences into the new bacterial taxonomy. Appl. Environ. Microbiol. 73 (16), 5261–5267. doi: 10.1128/AEM.00062-07
Webster N. S., Cobb R. E., Negri A. P. (2008). Temperature thresholds for bacterial symbiosis with a sponge. ISME J. 2 (8), 830–842. doi: 10.1038/ismej.2008.42
Webster N. S., Reusch T. B. (2017). Microbial contributions to the persistence of coral reefs. ISME J. 11 (10), 2167–2174. doi: 10.1038/ismej.2017.66
Webster N. S., Thomas T. (2016). The sponge hologenome. MBio 7 (2), e00135–e00116. doi: 10.1128/mBio.00135-16
Weiss K. R. (2017). Can deep reefs rescue shallow ones? Science 355 (6328), 903–903. doi: 10.1126/science.355.6328.903
Wickham H., Averick M., Bryan J., Chang W., McGowan L. D. A., François R., et al. (2019). Welcome to the tidyverse. J. Open Source software 4 (43), 1686. doi: 10.21105/joss.01686
Wilkinson C., Fay P. (1979). Nitrogen fixation in coral reef sponges with symbiotic cyanobacteria. Nature 279 (5713), 527–529. doi: 10.1038/279527a0
Yahel G., Marie D., Genin A. (2005). InEx–a direct in situ method to measure filtration rates, nutrition, and metabolism of active suspension feeders. Limnology Oceanography: Methods 3 (2), 46–58. doi: 10.4319/lom.2005.3.46
Zaneveld J. R., McMinds R., Vega Thurber R. (2017). Stress and stability: applying the Anna karenina principle to animal microbiomes. Nat. Microbiol. 2 (9), 1–8. doi: 10.1038/nmicrobiol.2017.121
Keywords: bacteria, climate change, Diacarnus erythraeanus, holobiont, mesophotic, microbiome, ocean warming, sponges
Citation: Raijman-Nagar L, Goren L, Shefer S, Moskovich R, Li Z and Ilan M (2023) A comparison of mesophotic and shallow sponge holobionts resilience to predicted future temperature elevation. Front. Mar. Sci. 10:1161648. doi: 10.3389/fmars.2023.1161648
Received: 08 February 2023; Accepted: 18 May 2023;
Published: 05 June 2023.
Edited by:
Manuel Maldonado, Center for Advanced Studies of Blanes (CSIC), SpainReviewed by:
Cristiane Cassiolato Pires Hardoim, São Paulo State University, BrazilLucia Pita, Institute of Marine Sciences (CSIC), Spain
Copyright © 2023 Raijman-Nagar, Goren, Shefer, Moskovich, Li and Ilan. This is an open-access article distributed under the terms of the Creative Commons Attribution License (CC BY). The use, distribution or reproduction in other forums is permitted, provided the original author(s) and the copyright owner(s) are credited and that the original publication in this journal is cited, in accordance with accepted academic practice. No use, distribution or reproduction is permitted which does not comply with these terms.
*Correspondence: Lilach Raijman-Nagar, cmFsaWxhaDJAZ21haWwuY29t