- IHCantabria - Instituto de Hidráulica Ambiental de la Universidad de Cantabria, Santander, Spain
The Manila clam (Ruditapes philippinarum) has been introduced into the Atlantic European coast for commercial exploitation. In this region, the population dynamics of this clam species may be determined by the interaction with the native dwarf eelgrass (Zostera noltei). We performed an experiment in the tidal flats of an estuary located in northern Spain to analyse the interaction between Z. noltei and juvenile Manila clams. Based on existing knowledge, it was hypothesised that seagrasses act as a nursery protecting juveniles from predation, which is an important cause of natural mortality, and that seagrass density influences the survival, growth and condition of clams. The results indicate that the effects of seagrasses on juvenile clams depends on shoot density, which mainly determine the relative prevalence of positive versus negative interactions. Dense seagrass meadows protect juvenile clams from predation, likely decreasing their visibility and reducing the efficiency of predators. However, in these dense meadows, a decline in the condition of clams was also observed, although not in shell growth. This decline can be due to the fact that food supply in dense meadows is slow and does not fulfil the replenishment rates required by filter-feeding organisms or because competition for space with the seagrass causes stress. Clams are larger in populations where predator access is prevented, possibly indicating selective predation on larger organisms when this interaction is allowed. The knowledge gained from this experiment is of great interest for analysing possible future trends in clam populations and the derived social implications given its high economic value.
Introduction
Due to their structural and functional characteristics, seagrasses are ecosystem engineers that generate a tridimensional structure within and above the sediment in which they grow, in estuarine and coastal waters. They are also capable of further modifying the existing abiotic (both physical and chemical) conditions, providing many ecological functions, such as refuge, nesting and resting places, nutritional resources, and geochemical and nutritional pathways. This explains why seagrass habitats are able to support more species and density of benthic invertebrates than non-vegetated habitats (Glaspie and Seitz, 2017; Brun et al., 2021). Such rich fauna diversity involves a complex network of interactions with seagrasses (e.g. competition, mutualism or facilitation) resulting in multiple bottom-up and top-down, positive and negative effects, which affect the population dynamics of the species themselves (e.g. death, reproduction and recruitment rates) regulating their abundance and size structure. Thus, an appropriate understanding of the specific effects of habitat modifications driven by seagrasses on the different associated species has great relevance for the conservation and management of marine and estuarine ecosystems.
Given the capacity of seagrasses to house and support a large biodiversity, the health of seagrass meadows has been considered a robust indicator of the general health of their associated communities and ecosystems. Consequently, ecological indicators have been developed relying on metrics retrieved from these same seagrass meadows. The biomass-density relations of seagrass, and its associated phalanx and guerrilla clonal growth strategies have been on the core on such attempts (Sintes et al., 2005; Romero et al., 2007; Cabaço et al., 2008; García-Marín et al., 2013; Vieira et al., 2018; Vieira et al., 2022). The consensual approach is that denser meadows with larger biomass are healthier, and thus, so are its associated communities and ecosystems. However, recent evidences suggests that, although generally correct, this assumption may not hold for extremely dense meadows where negative effects on benthic fauna could emerge.
In northern Spain, the most abundant seagrasses are those belonging to the genus Zostera, which coexists in estuaries with different species of bivalves, such as commercially valuable clams of the genus Ruditapes. This coexistence leads to reciprocal interactions that generate effects and mechanisms as diverse as protection against predation and disturbance, food or oxygen availability (by production and water renovation), in the case of effects of seagrass on bivalves, and reduction of turbidity, decreased anoxia or drought resistance, in the case of effects of bivalves on plants, among others (Gagnon et al., 2020).
Clams can be positively or negatively affected by seagrasses in different ways. Seagrass leaves reduce prey visibility and provide physical predator-free refuge. Not only adult individuals benefit from this service, but also juveniles, in agreement with the well-known nursery function hypothesis (Beck et al., 2001). The juvenile stages of individuals, which are more vulnerable to predation, are critical for the sustainability of populations in the medium and long term, since they determine recruitment and replacement rates. Thus, seagrasses, through their function as nurseries for juvenile clams, foster a greater contribution to adult populations, increasing the recruitment success and ensuring their renewal (Barbier et al., 2017). In general, the presence of seagrasses is almost always negatively related to predation efficiency, with an increased seagrass density being often associated with a decreased predation efficiency on the associated benthic fauna, including clams (Heck and Orth, 2006). Nevertheless, this general effect of seagrasses on predator-prey dynamics depends not only on plant features but also on predator and prey characteristics. For example, clam size determines whether they can fit into the interstitial space available within the complex physical structure of seagrass meadows and thus avoid predation.
Other types of interactions beyond trophic ones also play a role in the overall balance of positive-negative effects of seagrasses on clams (Fales et al., 2020). Just like survival and richness, the growth of macrofaunal species is enhanced within seagrass meadows compared to unvegetated substrates (Heck et al., 2003), by preventing predation and allowing species to spend more time and effort feeding (where food availability is also higher, Irlandi and Peterson, 1991). However, at the same time, there are some evidences showing that high shoot seagrass densities can reduce growth and condition of benthic fauna, despite increasing their survival (Carroll and Peterson, 2013). This finding deserves further study to understand the factors that modulate this response and whether it applies to different biotic and abiotic conditions. The aerial structure of seagrass meadows characteristically reduces water flow (Ondiviela et al., 2014; Fonseca et al., 2019) and modifies resource availability within the meadows, which could both limit or promote the growth and condition of benthic and sedentary filter-feeding animals such as clams. Sparse seagrass meadows increase the supply of food for filter feeders and oxygen. Conversely, although dense meadows favour deposition and retention of suspended particles, food and oxygen can become limiting due to reduced water flow and consequent reduced supply of food particles, which may even become lower than its consumption rate by clams (González-Ortiz et al., 2014). Therefore, the capacity of seagrasses to attenuate water flow and increase or decrease nutrient supply for filter feeders and oxygen depends on plant density. The oxygen could be also limiting within dense seagrass meadows due to the intense deposition of organic matter that can lead to anoxia in the sediment and interstitial water, with deep impact on the benthic fauna, both on the health condition of the individuals, their abundances, and shifts in community structure with loss of biodiversity. Such events of extreme organic load to the sediment may even have deleterious impact on the own root system of seagrasses, eventually driving their decline.
The subterranean structure of seagrasses (i.e. their rhizomes) can also lead to strong competition for space with benthic infauna at high plant densities (Gribben and Wright, 2014). Stressful conditions induced by this competitive scenario potentially decrease clam survival, growth and condition. Belowground seagrass biomass can reduce the burial capacity of clams in shallow and deep sediment, which is necessary for them to avoid predation and drift due to water currents and waves, and to tolerate stress conditions caused by exposure to air at low tide. The magnitude of this competition for space also depends on the size of the clam and thus its age. Small juvenile clams can more easily be accommodated into the spaces between rhizomes even at very high plant densities, decreasing intra and interspecies competition compared to larger adult individuals. Thus, the carrying capacity of clams within seagrass meadows depends on such space availability and it is conditioned by both seagrass density and clam size.
According to the previous paragraph, the effect of seagrass meadows on clams depends on the size of the clams. And not only clam size, but also other structural characteristics, functional traits and behaviour of clams that change with ontogeny can influence the outcome of their interaction with seagrasses. Despite the important role that different life cycle stages of clams may play in species interactions, most of the existing studies focus on analysing the overall effects of seagrass meadows on the survival, growth and condition of adult clams. However, there are still major knowledge gaps of how juvenile clams modify the effects of seagrass-clam interaction.
From a management and conservation perspective, it is important to consider the role of these biological interactions on the population dynamics of highly pressured species, such as the native clam Ruditapes decussatus (Carpet clam) and the non-native farmed clam Ruditapes philippinarum (Manila clam) in Europe. In recent years, many bivalve stocks around the world have declined drastically, and specifically these two clam species have severely reduced their main populations in the estuaries of Cantabria (Bay of Biscay). This decline has been associated with different natural and anthropogenic pressures, either by increasing (e.g., increased frequency and intensity of heatwaves associated with climate change) or decreasing (e.g., reduced nutrient inputs due to the implementation of wastewater treatment systems) them on the ecosystem (Bidegain et al., 2013). Natural recovery is often slow or absent (e.g., due to recruitment failure), and even active restoration initiatives are difficult, because changes in the abiotic conditions and altered biotic interactions persist in the ecosystem (van der Heide et al., 2014). Understanding and analysing possible future trends in clam populations and management options requires considering not only the direct impacts of pressures but also the indirect impacts of changes in the populations of other species with which they interact, such as Zostera noltei (Ondiviela et al., 2020). Z. noltei, which coexists and interacts with clams in soft-bottom intertidal flats of estuaries, is currently increasing its extension and density in some European estuaries such as the Bay of Santander (Calleja et al., 2017). In addition, R. philippinarum has been introduced in the estuaries of northern Spain, where it is listed as a non-native exotic species and where it has created new interactions with the native seagrass Z. noltei that need to be studied further to understand clam population dynamics and their potential ecological and economic impacts.
Therefore, the primary objective of this study is to investigate the interactions between the seagrass species Zostera noltei and juvenile Manila clams (Ruditapes philippinarum) in sandy intertidal areas. Specifically, we aim to determine how the density of seagrass and predation affect the overall balance of biological interactions between the two species. By investigating these factors, we hope to gain a better understanding of the mechanisms that underlie the expansion of Manila clams beyond their native area and their interactions with seagrass along the Atlantic European coastline. In accordance with this objective, the working hypotheses are: (1) the main cause of mortality of juvenile clams is predation from above (e.g. by fish, birds or crabs); (2) seagrasses act as a nursery protecting juvenile clams from predation; (3) the density of seagrasses determines the overall balance of positive and negative interactions with juvenile clams, such that positive interactions dominate at low cover (e.g. protection from predators) and negative interactions are detected at high cover (e.g. difficulty of burial or reduced food availability). The experimental approach developed in this study focuses on testing the three hypothesis.
Materials and methods
Study site
A field experiment was conducted in the intertidal flats of Santander Bay, the largest estuary along the Northern Spanish coastline (Figure 1) (Juanes et al., 2020). It is a mesotidal estuary with a complex morphology and large intertidal areas (Galván et al., 2010). The experimental site within the estuary is an area characterized by the presence of dwarf seagrass meadows (Zostera noltei) and located near culture parks of the non-native Manila clam (Ruditapes philippinarum) (Juanes et al., 2012). Natural populations of the native Carpet clam (Ruditapes decussatus) are also commercially exploited. The study site and surrounding areas are suitable habitats for both clam species (Bidegain et al., 2015). The substrate is composed of sandy sediments (> 95% of sand) with low concentrations of organic matter (0.5%), nutrients (Nitrogen: 230 mg/kg; Phosphorous: 98 mg/kg), metals (low levels below standard reference toxicity values) and PCBs (< 0.05 mg/kg), typical of non-contaminated by human activities sites (Puente et al., 2022). The sediment and associated biota are exposed to air around 45% of the time. During immersion times, they are under polyhaline (average salinity around 28), low water velocities (average values around 0.1 m/s) and weak shear stress (maximum values around 0.1 N/m2) conditions (Galván et al., 2021).
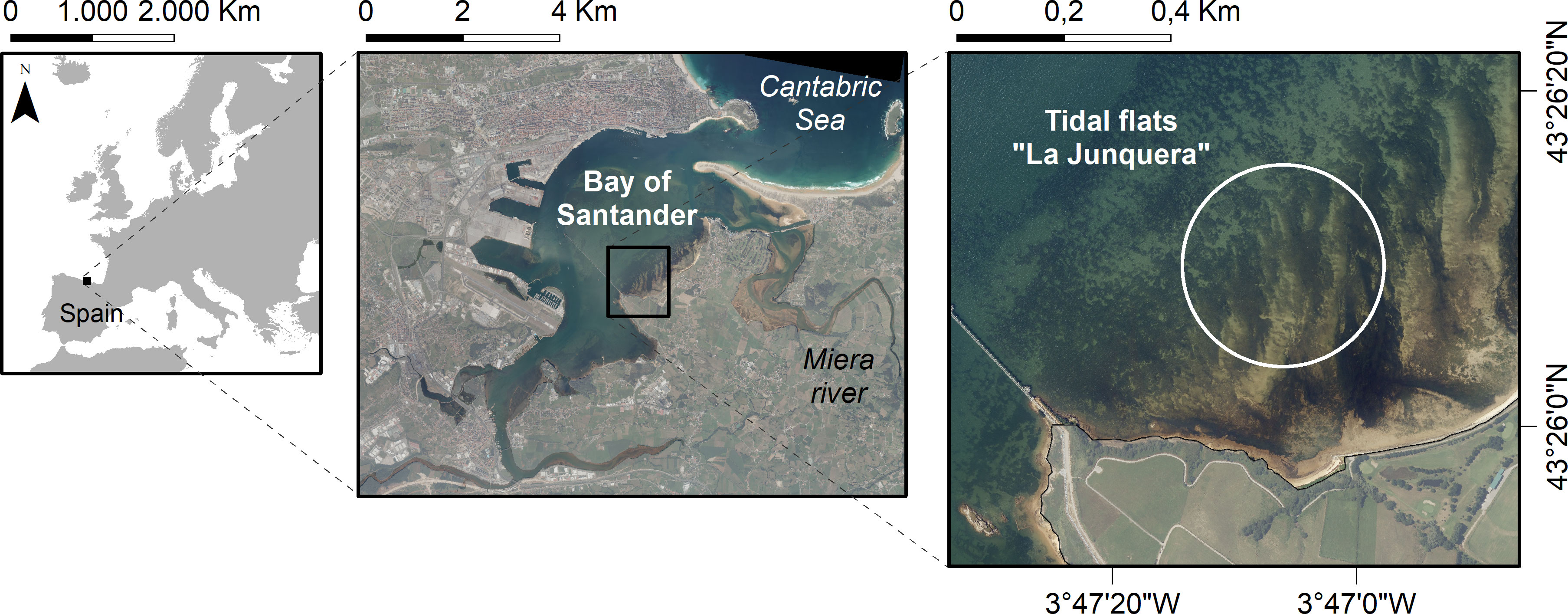
Figure 1 Experimental site (white circle) in the intertidal flats of Santander Bay (northern Spain, Europe). CRS: World Geodetic System 1984 (WGS84).
Experimental design
To test our hypotheses, we completed a field experiment using a split plot design (Altman and Krzywinski, 2015) with two factors: seagrass density and predation (Figure 2). The whole plot treatment factor was seagrass density, with three levels: (1) high seagrass density, with an aerial coverage higher than 90%; (2) medium seagrass density, with an aerial coverage between 40-60%; and (3) absent or low seagrass density, with an aerial coverage lower than 1%. The subplot treatment factor was predation that was a binary factor considering (1) non-access from predators from above; and (2) free access from predators. Predation levels were assigned to the subplots within each plot using a completely randomized design (CRD). Five replicates per split-plot treatment were performed.
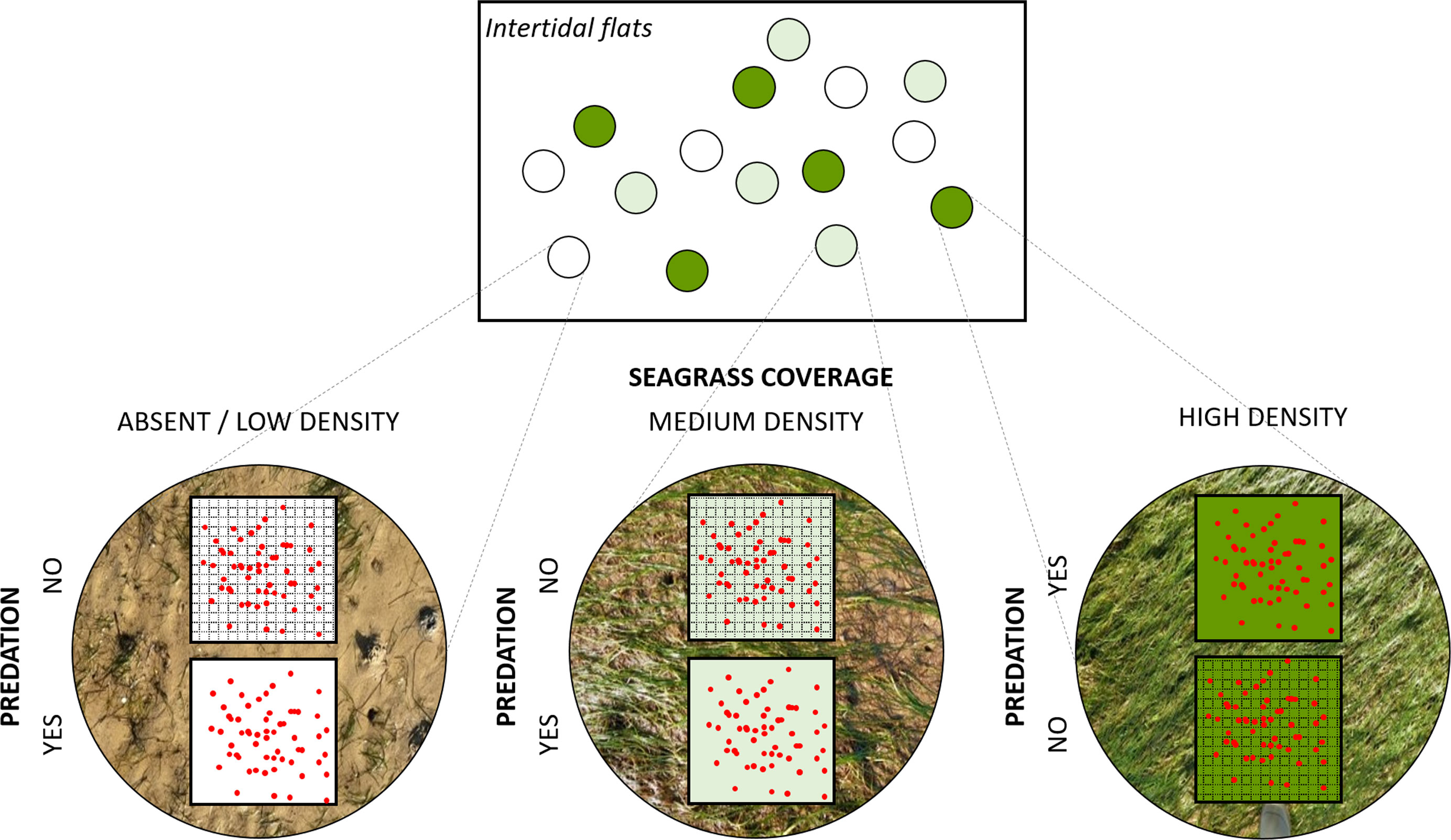
Figure 2 Experimental design with five replicates of six treatments resulting from the combination of two factors: seagrass coverage and predation. Predation level was experimentally manipulated using a protective mesh against predators. The red dots represent clam individuals.
Fifteen whole plots were distributed along the study site in places where seagrass coverage complied with the previously described treatments (i.e. five plots per seagrass density level) (Figure 2). Seagrass densities in the plots were not artificially modified because the natural patchy distribution of Z. noltei provides enough areas with the required three seagrass cover categories within the study site. A separation of at least 1 m between plots was maintained to reduce spatial effects. In each plot, two subplots of 0.0625 m2 (i.e. squares of 25 x 25 cm) were delimited by bamboo sticks in the field. One of the two subplots was randomly selected and covered with a protective 5-mm plastic mesh against predation of clams by fishes, crustaceans and/or birds. The mesh was placed 5 cm above the sediment to allow the leaves to elongate vertically. The other subplot was considered a control with a natural access from predators. All plots were set up in places with similar physical conditions, avoiding pools and shell or debris accumulations.
The density of experimental Manila clams in the subplots was manipulated to obtain homogeneous populations. Juvenile clam individuals (size 9-11 mm) were obtained from culture parks located in Galicia, in the same bioregion as the study site. Clams were transported to the lab in cold conditions. In the lab, they were maintained for 48 hours in aquaria with filtered seawater and aeration at 18°C, and fed with Isochrysis sp. At the experimental site, a total of 30 individuals of juvenile clams were put in each subplot, to obtain a density of 480 individual/m2, which is lower than that used in clam culture fields but higher than natural values. Individuals were marked with a permanent black marker and buried 1 cm into the sediment to facilitate their adaptation and survival (e.g., reduce predation, dragging the clam out of the plot by water currents and stress by air exposure) before the tide covered them.
The experiment was conducted between 24 June 2021 and 22 July 2021. These dates were selected because (1) it is a suitable period for the survival and growth of clam juveniles; (2) macroalgae blooms that could cover the plots generating anoxic conditions are rare; (3) the probability of extreme events, such as high river flows or heatwaves, is low. A 1-month duration of the experiment was selected because it is enough time to assess clam survival and mortality rates and growth, and at the same time reduce the risk of vandalism and catastrophic events.
At the beginning of the experiment, the seagrass coverage in each subplot was estimated visually using expert criteria. At the end of the experiment, all the sediment contained in each subplot to a depth of 15 cm was collected. The sediment was sieved with a 1 mm mesh to recover the juvenile clams. In the lab, clams were counted and classified as dead or alive. Various parameters were measured in the marked clams recovered alive. First, the monthly instantaneous survival rate in each treatment was estimated as:
Where ‘Nend’ is the number of live clams at the end of the experiment (1 month) and ‘Ninitial’ is the initial number of clams in each subplot at the beginning of the experiment (30 clams). Second, the size of each live clam (maximum length) was measured. Lastly, the flesh mass (soft body) was separated from the shell and both fragments were dried at 70°C for 24 hours (Yin et al., 2017). Dry body weight and shell body weight were then obtained using a precision laboratory balance with a 0.001 g resolution. Based on this data, the Condition Index of each specimen was calculated using the following equation (Walne, 1976; Filgueira et al., 2013):
A sample of seagrasses was collected in a plot of 25x25 cm for each seagrass cover treatment in order to estimate seagrass density and biomass. Aboveground and belowground biomass of Z. noltei were estimated as the dry weight after drying at 70 °C for 72 hours. Additionally, a sediment sample was obtained for characterizing the grain size distribution (Wentworth scale) and the organic matter content of the sediment was also analysed.
Statistical analyses
We determined if different levels of seagrass coverage and predation from above (i.e., two independent variables) cause the same mean instantaneous survival rate, condition and growth of juvenile clams (i.e., dependent or response variables) by using Linear Mixed-effect Models (LMM). Linear mixed-effect models allow accommodating split plot designs. Seagrass coverage (whole plot factor) and predation (subplot factor) treatments were included in the model as fixed effects, and plot (block factor) was a random effect.
For each dependent variable, we built a model that contains as fixed effects seagrass coverage, predation and the interaction between them. Based on this fixed structure, we fitted a random intercept model and also a more complex random intercept and slope model with REML (Restricted Maximum Likelihood). We compare both models to select the random structure that fits the data significantly better using the Likelihood Ratio Test (Zuur et al., 2009). Then, we estimated the coefficients and variances of all fixed effect parameters in the model considering the selected random structure. The significance of the contribution of fixed terms were analysed using F-test based on the Kenward-Roger approach (Kenward and Roger, 1997). Finally, a Sidak test (Sidak, 1967) was carried out to perform post-hoc pairwise comparisons and to identify statistically significant differences between specific treatments. This test adjusts the significance level for multiple comparison and determines whether there is a difference between the mean of all possible pairs using a studentized range distribution.
The statistical assumptions of linear mixed-effect models were checked. Spatial autocorrelation in residuals was analysed to assess the independence of samples. Normality of residuals was visually assessed using histograms, while homoscedasticity was checked using plots of residuals versus fitted values. In addition, both normality and homoscedasticity assumptions were statistically analysed using the Shapiro-Wilk and Levene’s tests, respectively. If the residuals were non-normally distributed or with unequal variances, data (dependent variable) was transformed before fitting the model.
For all tests, a significance level of 0.05 was considered. The models were built using the lmer function of the lmerTest package, F-test were applied with the anova function in base R, the post-hoc comparisons were done using emmeans and multcomp packages, and normality and homoscedasticity were assessed using the stats and car package in the R software version 4.3.1 (R Core Team, 2022).
Results
The average values of instantaneous survival rate, condition index and shell length responded in a different way to the experimental treatments (Table 1) (Supplementary Material). The survival rate was clearly lower in the low seagrass coverage treatments exposed to predation. On the contrary, the condition index was lower in the high seagrass coverage treatments, independent of predation level. Last, shell length was larger in those treatments that are protected against predation from above independently of seagrass coverage levels.
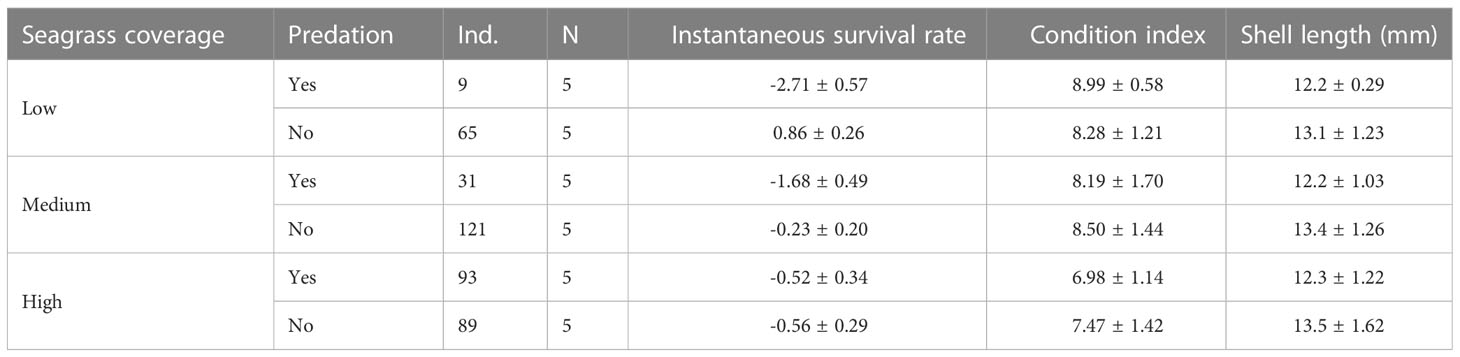
Table 1 Number of clams at the end of the experiment (Ind.), number of independent samples (N) and mean and standard deviation ( ± SD) of instantaneous survival rate, condition index and shell length of juvenile clams by treatment (all combinations of the three levels of seagrass coverage and the two levels of predation from above).
For the three response variables (i.e., survival rate, clam length and condition index), the linear mixed-effect model that fit the data significantly better is the model with the less complex random intercepts (lower AIC, Akaike Information Criteria, Table 2), in comparison with the more complex model with a random intercept and slope (higher AIC, Table 2). The value of the parameters of fixed factors in each model and their individual significance are shown in Section 6.2 of the Supplementary Material.
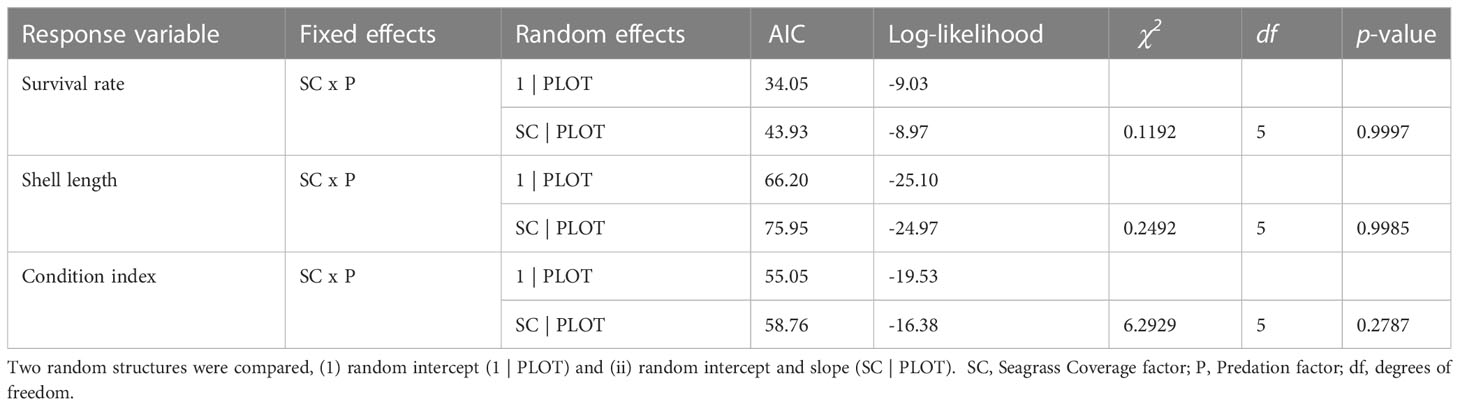
Table 2 Selection of the random structure for the model of survival rate, shell length and condition index, based on the AIC (lower AIC values indicate a better model) and the likelihood ratio test (p-value > 0.05 indicates that the model does not fit the data significantly better than the other model compared).
The statistical analysis revealed that seagrass coverage, predation and their interaction have significant effects on clam instantaneous survival rates (Table 3, Figure 3) (Supplementary Material). Pairwise comparisons between treatments showed that clam survival in dense seagrass meadows was significantly higher than in meadows with low and medium shoot density (Table 4). Additionally, instantaneous survival rates were lower with decreasing plant densities. This pattern was observed under conditions of exposure to natural levels of predation. In contrast, when artificial physical barriers prevented clam predation from above, their instantaneous survival rates were similar in all seagrass meadows regardless of seagrass density. Moreover, these survival values were equivalent to those recorded in high-density meadows exposed to predation. Finally, it should be noted that within low and medium density meadows, instantaneous survival rates were significantly higher when predator access to clams was prevented than when predator access was allowed.
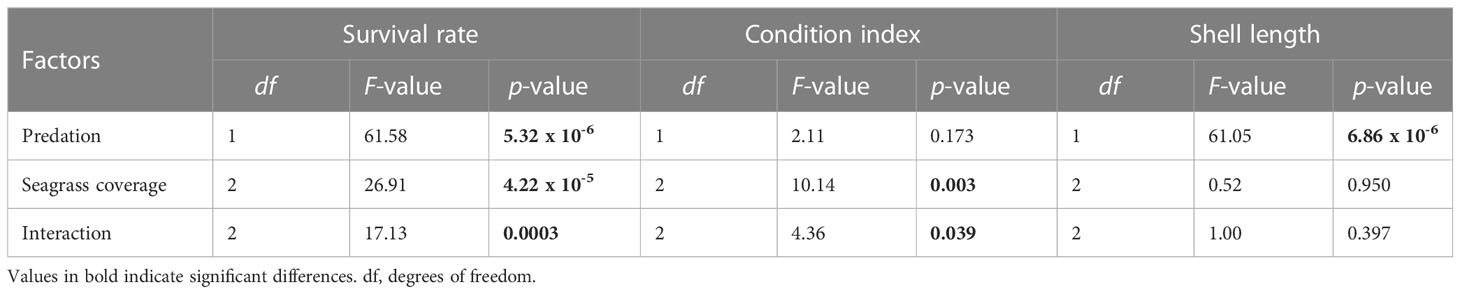
Table 3 Effects of seagrass coverage and predation on the survival rate, condition index and shell length of juvenile clams.
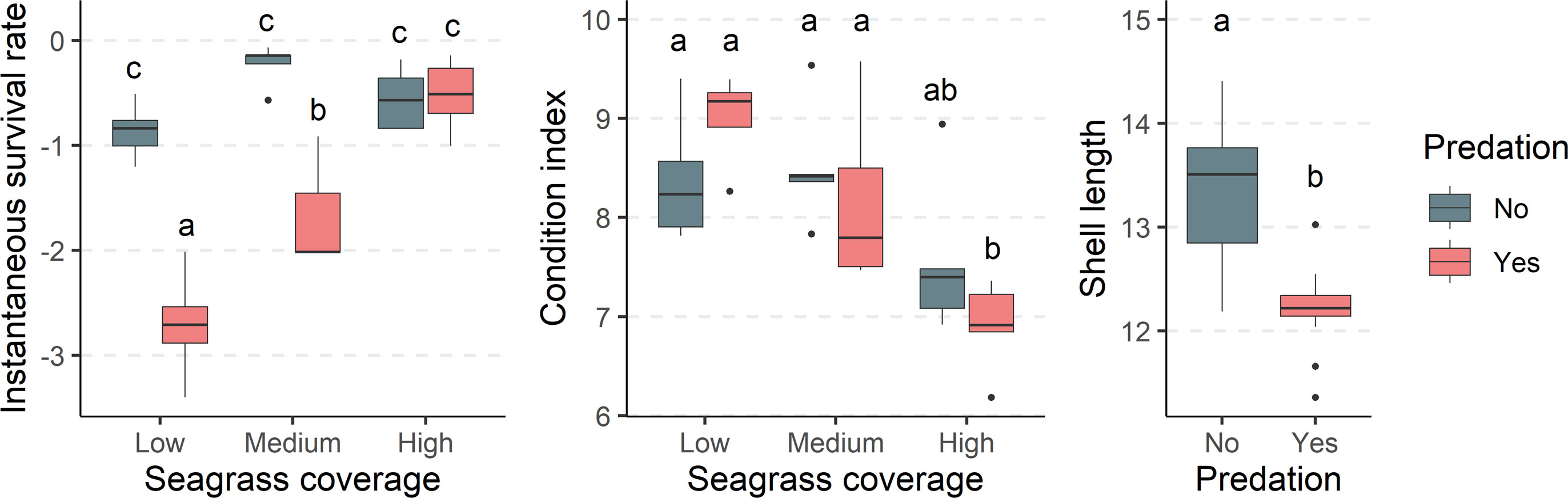
Figure 3 Boxplots and post-hoc test results showing differences in the monthly instantaneous survival rate and condition index of clams at the various seagrass coverage and predation levels analysed, and the shell length of clams with and without predation. Boxplots represent the median, interquartile range (25th and 75th percentiles), largest and smallest value within 1.5 times below the 25th percentile and above the 75th percentile, respectively, and outliers. Different letters indicate statistically significant differences at a confidence level of 95%.
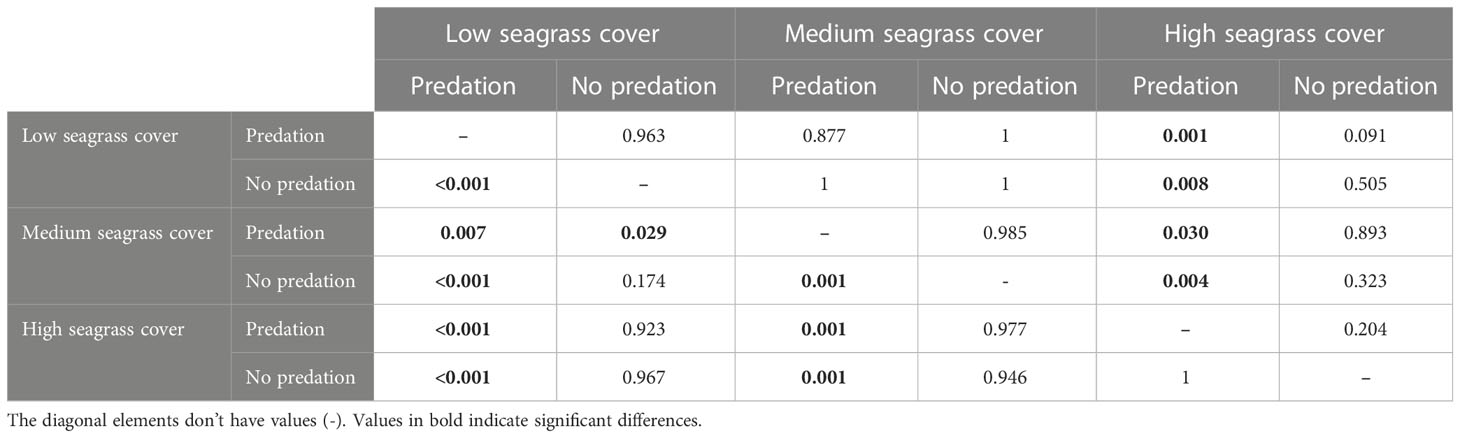
Table 4 Pairwise multiple comparison (Sidak test) results (p-values) of clam survival rate (Lower left diagonal) and condition index (Upper right diagonal) comparisons between seagrass coverage and predation levels.
With regard to clam condition, this variable required transformation for statistical analysis to improve normality of residuals. Unlike the patterns observed for survival rate, clam condition showed no statistically significant differences between predation levels (Table 3, Figure 3) (Supplementary Material). Instead, there were significant differences associated with the level of seagrass cover and the interaction of this factor with predation. A more detailed pairwise analysis of the effects of seagrass density revealed significantly lower values of condition index for clams located within dense seagrass meadows (Table 4). This effect was detected both with and without free access of clam predators to the experimental units.
An average increase of 2-3 mm (approximately 20%) was observed over the month-long trial. Clam length was significantly and negatively affected by predation (Table 3, Figure 3) (Supplementary Material). Clams were significantly smaller when exposed to natural levels of predation from above than when predation was restricted (Table 4). Contrary to what was observed for clam survival and condition, seagrass density had no significant effect on clam size.
The seagrasses and sediment properties are different among the three seagrass treatments. The low, medium and high seagrass cover treatments correspond to mean density values of 240, 2,832 and 3,552 shoots/m2, respectively. Biomass levels correspond to average values of 6.1 g/m2 (aboveground: 2.1 g/m2; belowground: 4.0 g/m2), 33.3 g/m2 (aboveground: 11.7 g/m2; belowground: 21.6 g/m2) and 137.4 g/m2 (aboveground: 47.5 g/m2; belowground: 89.8 g/m2) in the low, medium and high seagrass coverage plots, respectively. The length of the leaves of Z. noltei was also higher in the high seagrass density plots (23.8 ± 2.5 cm) than in the medium and low seagrass coverage plots (9.4 ± 0.8 cm). The organic matter content in the sediment was similar in the low and medium seagrass coverage treatments (0.4%), and lower in both cases than in the high coverage treatment (1.17%). Sediment in the study site was dominated by sand fractions (97.9%), with less than 1% of gravel.
Discussion
This study contributes to understand the role of biological interactions in seagrass systems by disentangling the positive and negative effects of seagrass density and large predators on juvenile clam survival, condition and growth (Figure 4). Our results demonstrate that seagrasses and predators affect the survival, condition, and growth of juvenile clams (Gagnon et al., 2020), providing quantitative evidence of the effects of dwarf seagrass (Z. noltei) on juvenile non-native Manila clams (R. philippinarum). Specifically, the experiment results confirm the starting hypothesis of this study. The main threat to the survival of clams during juvenile stages is predation by large predators from above (hypothesis 1). Likewise, the density of seagrasses is positively related with clam survival (hypothesis 2) and negatively related with condition of clams (hypothesis 3). This work has added further insights into the R. philippinarum’s autoecology and is one of the few studies looking at these interaction effects on juveniles inhabiting a non-native area.
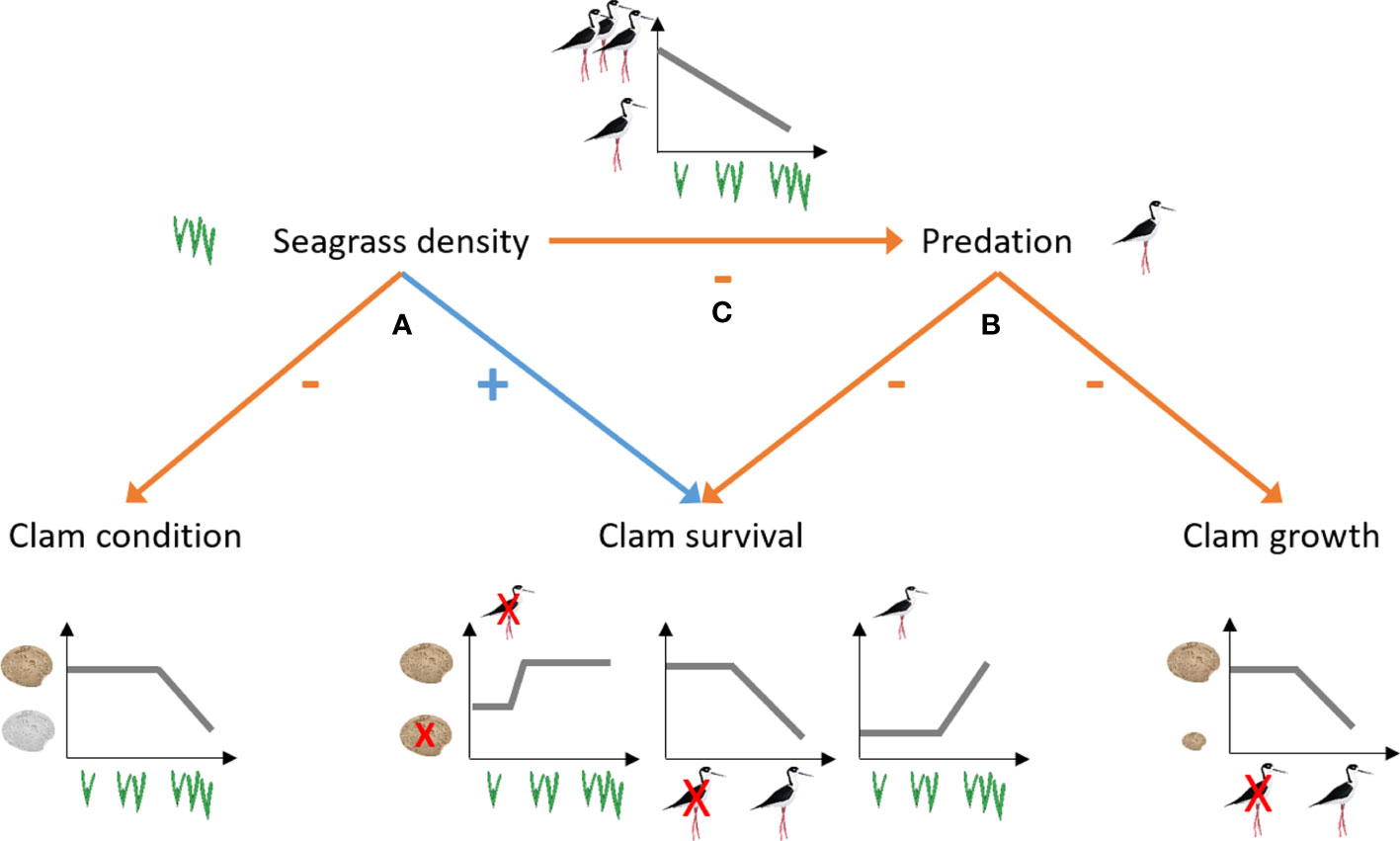
Figure 4 Diagram of the effects emerging from positive and negative interactions between native seagrass Z. noltei and non-native clam R. philippinarum. Seagrass density has direct effects on clam condition and survival (A), and indirect effects on clam survival and growth (B) through their capacity to modify the efficiency of clam predators (C). Symbols used on the figure were taken from IAN symbol libraries (https://ian.umces.edu/media-library).
Our study supports previous evidence (McArthur, 1998; Hiddink et al., 2002; Ruesink et al., 2014) showing that one of the main causes of clam mortality is predation, especially in the early stages of their life cycle. Specifically, in this study, mortality by large predators was estimated to range from ca. 25% to more than 50% in juvenile Manila clams that were not protected by a mesh. Predators from above, such as birds, fishes or crabs, have high feeding rates and mobility, and exert a strong predation pressure on the clams (Dethier et al., 2019; Domínguez et al., 2021). For example, Carcinus maenas is a native abundant predator of juvenile clams, both in natural and farmed populations, in intertidal flats of North East Atlantic estuaries, which can have a negative impact on clam abundance (Grosholz et al., 2001). Beyond predation, there are other causes of clam mortality with significant effects on juvenile populations. The observed mortality in predator-free plots could be explained because exclusion nets above the sediment were not an efficient protection against infaunal predators (e.g., worms or polychaetes) and because of the vulnerability of clams to physical stress (Cigarría and Fernández, 2000). Other hypothesis to explain the mortality of clams is the potential decrease of oxygen within the sediment and interstitial water. Low water renovation and the sedimentation of large amounts of organic matter from seagrasses may lead to anoxic conditions, which could be facilitated by low tide or high temperatures. In the study area, the anoxic layer is located at about 2 cm depth in dense seagrass meadows. This indicates that anoxia is not a chronic pressure for clams.
We also observed that there was a lower mortality of juvenile clams in the presence of seagrasses, which supports the hypothesis that seagrass beds are important nurseries for clam species. This nursery role of seagrass meadows has been extensively analysed, several studies concluding that the enhanced survival of juveniles within seagrass habitats compared to non-vegetated habitats is mainly due to the structure provided by them rather than any intrinsic property of seagrasses (Heck et al., 2003). The higher density, the higher above and below ground structure complexity and the higher protection against predation. In this regard, the main challenge is to quantify how the protection change with seagrass density and detect whether threshold levels of seagrass density exist, below which there is no clam protection effect and above which protection increases rapidly. Different authors have found the existence of such threshold; it means that a minimum seagrass density is required to obtain a significant reduction in predation rates (Heck and Ortz, 2006). The results obtained in this study are not conclusive to support or reject this finding. Additional experiments or field observations along seagrass density gradients with a higher resolution should be carried out to detect whether such thresholds really exist and to better quantify the protection response above and below the density threshold.
Since protection of juvenile clams depends on seagrass density, which shows a seasonal variability, the seasonal dynamics of both species should be taken into account to assess the nursery function of seagrasses. Seagrasses growing on the European Atlantic coast show a marked temporal variation in abundance and biomass that responds to a unimodal curve, with a maximum in spring (April-May) and a minimum in winter (January) in the case of Z. noltei (Ondiviela et al., 2018; Sousa et al., 2019). This peak in biomass coincides with one of the recruitment periods of Manila clams (Humphreys et al., 2007), thus contributing to increase the recruitment success by decreasing mortality rate due to predation. Furthermore, since predation pressure on clams also depends on density of predators, it is necessary to include in this equation not only the seasonal cycle of clams and seagrasses, but also that of clam predator species (e.g. intra-annual change in abundance of different predators with different capture efficiency of juvenile clams).
Numerous studies in marine ecosystems have found effects of predators on the size distribution of their prey, affecting their population dynamics (Sih et al., 1985; Wilson, 1990; Turchin, 1995; Glaspie and Seitz, 2018). The same effect was observed on juvenile clams in this work. Large predators, such as birds, crabs and fishes, exhibit a preference for a specific prey size, in this case the larger sizes of juvenile clams (O’Brien et al., 2005). Maybe, a higher visibility or a lower burial capacity of large clams (e.g. due to a complex underground matrix of seagrass rhizomes and roots) can determine this result.
In accordance with the above-mentioned seagrass nursery hypothesis, seagrass meadows are considered to contribute not only to the increased survival of juvenile clams but also to an enhanced length and condition of the individuals. However, the results of this study do not fully support this statement, as it is concluded that, unlike clam survival rate, clam length and condition are not directly related to seagrass density. On the contrary, it is here concluded that seagrass density and clam condition have an inverse relationship, in accordance with Tsai et al. (2010). A variety of studies show different results in this respect, highlighting the complexity of the issue and the existence of multiple factors conditioning clam response (Gagnon et al., 2020). Specifically, the condition index has been positively correlated with food availability in many species of bivalves (Norkko et al., 2005). In this regard, seagrass meadows have the potential to influence the availability of food for clams, as well as their uptake rate. Food availability for filter feeders depends on current velocity and water renewal inside the meadow, which in turn depends on seagrass density (Ondiviela et al., 2014). In addition, the density of seagrass meadows also determines the degree of protection from predators provided to the clams and thus the amount of time they can spend feeding. The trade-off between seagrass density and these effects on food availability and protection therefore gives rise to multiple possible responses depending on the local conditions of the study site. Manipulative field experiments are key to understand the relative importance of factors affecting the dynamics of clam populations. By conducting the same experiment at several locations at the same time, variations in the relative importance of local ecological processes at broader spatial scales can be assessed.
The essential role of environmental factors other than food availability, that directly influence clam condition, should not be forgotten. Several environmental factors such as temperature and salinity have been correlated with the condition index in bivalves. An impaired condition in clams due to the cumulative effects of suboptimal conditions in water temperature and sediment characteristics have also been observed in previous studies (Norkko and Thrush, 2006). Under less suitable scenarios, clam condition and fitness are reduced, and seagrasses can play a relevant role by modifying environmental conditions within the meadows (e.g. reduced current velocity or reduced turbidity). These modifications, however, can occur in different directions, either enhancing or buffering unfavorable conditions for clam juveniles, through processes of antagonism or synergy. This is especially important under a climate change scenario, since seagrasses can reduce sediment temperature inside meadows compared to adjacent areas of bare sediment, and thus buffer negative effects of rising temperatures and heatwaves on clam growth and survival (Román et al., 2022b).
Much of the variation of results concerning seagrass-clam interactions found in the bibliography and in this study, can be explained by differences in environmental conditions. The experiment carried out in this study is not replicated in time and space, which limits the ability of the authors to conclude about the consistency of interactions across the estuarine environmental range in which both species coexist. Nevertheless, it is important to note that this study adds new evidences to the state of the art and supports the development of future experiments to address some of the limitations identified here. The accumulation of context- and perspective-dependent experimental evidences, such as that provided in this study, is essential for meta-analyses studies focused on asses the consistency of seagrass-clam interactions.
The introduction and exploitation of non-native species, such as the Manila clam, in European estuaries, creates new ecological, social and economic challenges that need to be analysed in depth in order to design appropriate management strategies for sustainability (Coelho et al., 2021). On the one hand, the intense harvesting of Ruditapes philippinarum, and other infaunal shellfish resources, has negative impacts on the native local benthic communities and on Zostera meadows in Iberian estuaries (Román et al., 2022a), driving their decline in some estuaries (Alexandre et al., 2005; Cabaço et al., 2005; Garmendia et al., 2017; Vieira et al., 2022). Besides the Iberian case, the negative impact of clam harvesting on seagrass meadows, particularly concerning their biomass and shoot density, is generalized worldwide (Peterson et al., 1987; Boese, 2002; McLaughlin et al., 2007; Ferriss et al., 2019; Herrera et al., 2022). On the other hand, the sustainability of exploited clam resources requires detailed information about population characteristics such as survival, growth, recruitment rate and how these parameters change spatially and temporally. Taking this two aspects into account, management decisions must have the dual objective of ensuring the sustainability of the exploited resource and the conservation of native biodiversity. To this end, it is essential to increase and deepen our knowledge of the effects of interactions between exploited species and native estuarine species to which this study contributes.
Biological and ecological information is usually scarce and decisions about harvest levels and exploitation areas, for example, are usually limited to estimates of standing stocks and size frequencies (Juanes et al., 2012), without taking into account species interactions and impacts on other species. As we have mentioned previously, the impacts of clam harvesting on seagrasses are well known, just like many of the key descriptors of Manila clam population dynamics. However, new knowledge gaps emerge from its status as a non-native species in Europe (among other sites), and the poorly studied interspecific interactions occurring with local species’ population dynamics and ecosystem functioning. Given the crucial role of seagrass meadows on bivalve populations, they must also be integrated in management perspectives aimed at preserving the seagrasses and the valuable goods and services provided by clams. A reduction in recruitment and thus in the renewal of the adult clam population associated with changes in seagrass extent and condition, among other causes, makes it difficult for clams to cope with catastrophic events and/or overexploitation. The influence of anthropogenic pressures and climate change in coastal zones is increasing, with cascading effects on clams and seagrasses (Juanes et al., 2020). Complementary studies are needed to determine the impact of the combined effects of these two stressors on both clams and seagrasses at different spatial scales. Additionally, all the available knowledge and information (e.g. experimental data, field data, remote sensing data or modelling data) about the ecology of both species can also be put together to model their potential or actual past, present or projected future distribution areas (Ondiviela et al., 2014; Bidegain et al., 2015; Galván et al., 2021). These predictive tools are critical for understanding clam population dynamics and designing management approaches that are compatible with conservation under different scenarios.
Data availability statement
The original contributions presented in the study are included in the article/Supplementary Material. Further inquiries can be directed to the corresponding author.
Ethics statement
The manuscript presents research on animals that do not require ethical approval for their study.
Author contributions
All authors have made substantial contributions to the research and have been involved in drafting the manuscript and approving the final submitted manuscript.
Funding
This research was part of the ECOTOPO project (RTI2018-096409-B-I00), financially supported by the Spanish Ministry of Science and Innovation through the National Plan for Scientific Research, and the project “Profundizando en el conocimiento de la Biodiversidad en estuarios: el efecto de la escala y la presión en los patrones biológicos funcionales” (56.VP76.64662), financially supported by the regional government through the Consejería de Universidades, Igualdad, Cultura y Deporte del Gobierno de Cantabria (Convocatoria Financiada por el Contrato Programa Gobierno de Cantabria-UC). This study was also supported by the regional government through the Consejería de Desarrollo Rural, Ganadería, Pesca y Alimentación.
Conflict of interest
The authors declare that the research was conducted in the absence of any commercial or financial relationships that could be construed as a potential conflict of interest.
Publisher’s note
All claims expressed in this article are solely those of the authors and do not necessarily represent those of their affiliated organizations, or those of the publisher, the editors and the reviewers. Any product that may be evaluated in this article, or claim that may be made by its manufacturer, is not guaranteed or endorsed by the publisher.
Supplementary material
The Supplementary Material for this article can be found online at: https://www.frontiersin.org/articles/10.3389/fmars.2023.1160734/full#supplementary-material
References
Alexandre A., Santos R., Serrão E. (2005). Effects of clam harvesting on sexual reproduction of the seagrass Zostera noltii. Mar. Ecol. Prog. Ser. 298, 115–122. doi: 10.3354/meps298115
Altman N., Krzywinski M. (2015). Split plot design. Nat. Methods 12, 165–166. doi: 10.1038/nmeth.3293
Barbier P., Meziane T., Forêt M., Tremblay R., Robert R., Olivier F. (2017). Nursery function of coastal temperate benthic habitats: New insight from the bivalve recruitment perspective. J. Sea Res. 121, 11–23. doi: 10.1016/j.seares.2016.12.007
Beck M. W., Heck K. L., Able K. W., Childers D. L., Eggleston D. B., Gillanders B. M., et al. (2001). The identification, conservation, and management of estuarine and marine nurseries for fish and invertebrates. BioScience 51, 633–641. doi: 10.1641/0006-3568(2001)051[0633:TICAMO]2.0.CO;2
Bidegain G., Bárcena J. F., García A., Juanes J. A. (2015). Predicting coexistence and predominance patterns between the introduced Manila clam (Ruditapes philippinarum) and the European native clam (Ruditapes decussatus). Estuarine Coast. Shelf Sci. 152, 162–172. doi: 10.1016/j.ecss.2014.11.018
Bidegain G., Sestelo M., Roca-Pardiñas J., Juanes J. A. (2013). Estimating a new suitable catch size for two clam species: Implications for shellfishery management. Ocean Coast. Manage. 71, 52–63. doi: 10.1016/j.ocecoaman.2012.09.009
Boese B. L. (2002). Effects of recreational clam harvesting on eelgrass (Zostera marina) and associated infaunal invertebrates: in situ manipulative experiments. Aquat. Bot. 73, 63–74. doi: 10.1016/S0304-3770(02)00004-9
Brun F. G., Cobo-Díaz J. F., González-Ortiz V., Varela J. L., Pérez-Lloréns J. L., Vergara J. J. (2021). Seagrass patch complexity affects macroinfaunal community structure in intertidal areas: an in situ experiment using seagrass mimics. Diversity 13, 572. doi: 10.3390/d13110572
Cabaço S., Alexandre A., Santos R. (2005). Population-level effects of clam harvesting on the seagrass Zostera noltii. Mar. Ecol. Prog. Ser. 298, 123–129. doi: 10.3354/meps298123
Cabaço S., Santos R., Duarte C. M. (2008). The impact of sediment burial and erosion on seagrasses: A review. Estuarine Coast. Shelf Sci. 79, 354–366. doi: 10.1016/j.ecss.2008.04.021
Calleja F., Galván C., Silió-Calzada A., Juanes J. A., Ondiviela B. (2017). Long-term analysis of Zostera noltei: A retrospective approach for understanding seagrasses’ dynamics. Mar. Environ. Res. 130, 93–105. doi: 10.1016/j.marenvres.2017.07.017
Carroll J. M., Peterson B. J. (2013). Ecological trade-offs in seascape ecology: bay scallop survival and growth across a seagrass seascape. Landscape Ecol. 28, 1401–1413. doi: 10.1007/s10980-013-9893-x
Cigarría J., Fernández J. M. (2000). Management of Manila clam beds 1. Influence of seed size, type of substratum and protection on initial mortality. Aquaculture 182, 173–182. doi: 10.1016/S0044-8486(99)00257-4
Coelho P., Carvalho F., Goulding T. A., Chainho P., Guerreiro J. (2021). Management models of the manila clam (Ruditapes philippinarum) fisheries in invaded European coastal systems. Front. Mar. Sci. 8. doi: 10.3389/fmars.2021.685307
Dethier M. N., Dobkowski K., Noreen A., Yun M., Moosmiller A. (2019). Vulnerability of juvenile clams to predation by shore crabs. Aquaculture 506, 350–354. doi: 10.1016/j.aquaculture.2019.03.070
Domínguez R., Vázquez E., Smallegange I. M., Woodin S. A., Wethey D. S., Peteiro L. G., et al. (2021). Predation risk increases in estuarine bivalves stressed by low salinity. Mar. Biol. 168, 132. doi: 10.1007/s00227-021-03942-8
Fales R. J., Boardman F. C., Ruesink J. L. (2020). Reciprocal interactions between bivalve molluscs and seagrass: a review and meta-analysis. J. Shellfish Res. 39 (3), 547–562. doi: 10.2983/035.039.0305
Ferriss B. E., Conway-Cranos L. L., Sanderson B. L., Hoberecht L. (2019). Bivalve aquaculture and eelgrass: A global meta-analysis. Aquaculture 498, 254–262. doi: 10.1016/j.aquaculture.2018.08.046
Filgueira R., Comeau L. A., Landry T., Grant J., Guyondet T., Mallet A. (2013). Bivalve condition index as an indicator of aquaculture intensity: A meta-analysis. Ecol. Indic. 25, 215–229. doi: 10.1016/j.ecolind.2012.10.001
Fonseca M. S. J., Fourqurean W., Koehl M. A. R. (2019). Effect of seagrass on current speed: importance of flexibility v. shoot density. Front. Mar. Sci. 6. doi: 10.3389/fmars.2019.00376
Gagnon K., Rinde E., Bengil E. G. T., Carugati L., Christianen M. J. A., Danovaro R., et al. (2020). Facilitating foundation species: The potential for plant-bivalve interactions to improve habitat restoration success. J. Appl. Ecol. 57, 1161–1179. doi: 10.1111/1365-2664.13605
Galván C., Juanes J. A., Puente A. (2010). Ecological classification of European transitional waters in the North-East Atlantic eco-region. Estuarine Coast. Shelf Sci. 87 (3), 442–450. doi: 10.1016/j.ecss.2010.01.026
Galván C., Puente A., Juanes J. A. (2021). Nested socio-ecological maps as a spatial planning instrument for estuary conservation and ecosystem-based management. Front. Mar. Sci. 8. doi: 10.3389/fmars.2021.730762
García-Marín P., Cabaço S., Hern andez I., Vergara J. J., Silva J., Santos R. (2013). Multi-metric index based on the seagrass Zostera noltii (ZoNI) for ecological quality assessment of coastal and estuarine systems in SW Iberian Peninsula. Mar. Pollut. Bull. 68, 48–54. doi: 10.1016/j.marpolbul.2012.12.025
Garmendia J. M., Valle M., Borja Á., Chust G., Lee D.-J., Rodríguez J. G., et al. (2017). Effect of trampling and digging from shellfishing on Zostera noltei (Zosteraceae) intertidal seagrass beds. Scientia Marina 81 (1), 1–8. doi: 10.3989/scimar.04482.17A
Glaspie C. N., Seitz R. D. (2017). Role of habitat and predators in maintaining functional diversity of estuarine bivalves. Mar. Ecol. Prog. Ser. 570, 113–125. doi: 10.3354/meps12103
Glaspie C. N., Seitz R. D. (2018). Habitat complexity and benthic predator-prey interactions in Chesapeake Bay. PloS One 13 (10), e0205162. doi: 10.1371/journal.pone.0205162
González-Ortiz V., Egea L. G., Jiménez-Ramos R., Moreno-Marín F., Pérez-Lloréns J. L., Bouma T. J., et al. (2014). Interactions between seagrass complexity, hydrodynamic flow and biomixing alter food availability for associated filter-feeding organisms. PloS One 9 (8), e104949. doi: 10.1371/journal.pone.0104949
Gribben P. E., Wright J. T. (2014). Habitat-former effects on prey behaviour increase predation and non-predation mortality. J. Anim. Ecol. 83, 388–396. doi: 10.1111/1365-2656.12139
Grosholz E., Olin P., Williams B., Tinsman R. (2001). Reducing predation on Manila clams by nonindigenous European green crabs. J. Shellfish Res. 20 (3), 913–919.
Heck K. L., Hays G., Orth R. J. (2003). Critical evaluation of the nursery role hypothesis for seagrass meadows. Mar. Ecol. Prog. Ser. 253, 123–136. doi: 10.3354/meps253123
Heck K. L., Orth R. J. (2006). “Predation in seagrass beds,” in Seagrasses: biology, ecology, and conservation. Eds. Larkum A. W. D., Orth R. J., Duarte C. M.. (Dordrecht, Netherlands:Springer). doi: 10.1007/978-1-4020-2983-7_22
Herrera M., Tubío A., Pita P., Vázquez E., Olabarria C., Duarte C. M., et al. (2022). Trade-offs and synergies between seagrass ecosystems and fishing activities: A global literature review. Front. Mar. Sci. 9. doi: 10.3389/fmars.2022.781713
Hiddink J. G., Marijnissen S. A. E., Troost K., Wolff W. J. (2002). Predation on 0-group and older year classes of the bivalve Macoma balthica: interaction of size selection and intertidal distribution of epibenthic predators. J. Exp. Mar. Biol. Ecol. 269 (2), 223–248. doi: 10.1016/S0022-0981(02)00002-3
Humphreys J., Caldow R. W. G., McGrorty S., West A. D., Jensen A. C. (2007). Population dynamics of naturalised Manila clams Ruditapes philippinarum in British coastal waters. Mar. Biol. 151, 2255–2270. doi: 10.1007/s00227-007-0660-x
Irlandi E. A., Peterson C. H. (1991). Modification of animal habitat by large plants: Mechanisms by which seagrasses influence clam growth. Oecologia 87 (3), 307–318. doi: 10.1007/BF00634584
Juanes J. A., Bidegain G., Echavarri-Erasun B., Puente A., García A., García A., et al. (2012). Differential distribution pattern of native Ruditapes decussatus and introduced Ruditapes phillippinarum clam populations in the Bay of Santander (Gulf of Biscay): Considerations for fisheries management. Ocean Coast. Manage. 69, 316–326. doi: 10.1016/j.ocecoaman.2012.08.007
Juanes J. A., Gómez A. G., Rubio S., García-Castrillo G., Puente A., Ondiviela B., et al. (2020). Santander Bay: Multiuse and multiuser socioecological space. Regional Stud. Mar. Sci. 34, 101034. doi: 10.1016/j.rsma.2019.1010134
Kenward M. G., Roger J. H. (1997). Small sample inference for fixed effects from restricted maximum likelihood. Biometrics 53, 983–997. doi: 10.2307/2533558
McArthur V. E. (1998). Predation and the survival of juvenile Cerastoderma glaucum Bruguiere (Mollusca: Bivalvia) in a coastal lagoon. J. Exp. Mar. Biol. Ecol. 225 (1), 79–97. doi: 10.1016/S0022-0981(97)00211-6
McLaughlin E., Portig A., Johnson M. P. (2007). Can traditional harvesting methods for cockles be accommodated in a Special Area of Conservation? ICES J. Mar. Sci. 64, 309–317. doi: 10.1093/icesjms/fsl037
Norkko J., Pilditch C. A., Thrush S. F., Wells R. M. G. (2005). Effects of food availability and hypoxia on bivalves: the value of using multiple parameters to measure bivalve condition in environmental studies. Mar. Ecol. Prog. Ser. 298, 205–218. doi: 10.3354/meps298205
Norkko J., Thrush S. (2006). Ecophysiology in environmental impact assessment: implications of spatial differences in seasonal variability of bivalve condition. Mar. Ecol. Prog. Ser. 326, 175–186. doi: 10.3354/meps326175
O’Brien E. L., Burger A. E., Dawson R. D. (2005). Foraging decision rules and prey species preferences of northwestern crows (Corvus caurinus). Ethology 111 (1), 77–87. doi: 10.1111/j.1439-0310.2004.01041.x
Ondiviela B., Fernández L., Puente A., García-Castrillo G., Juanes J. A. (2018). Characterization of a resilient seagrass meadow during a decline period. Scientia Marina 82 (1), 67–75. doi: 10.3989/scimar.04616.18A
Ondiviela B., Galván C., Recio M., Jiménez M., Juanes J. A., Puente A. (2020). Vulnerability of Zostera noltei to sea level rise: the use of clustering techniques in climate change studies. Estuaries Coasts 43, 2063–2075. doi: 10.1007/s12237-020-00742-z
Ondiviela B., Losada I. J., Maza M., Galván C., Lara J. L., Bouma T., et al. (2014). The role of seagrasses in coastal protection in a changing climate. Coast. Eng. 87, 158–168. doi: 10.1016/j.coastaleng.2013.11.005
Peterson C. H., Summerson H. C., Fegley S. R. (1987). Ecological consequences of mechanical harvesting of clams. Fishery Bull. 85 (2), 281–291. doi: 10.17615/2rb3-rm41
Puente A., Gómez A. G., de los Ríos A., Galván C. (2022). Natural stress vs. anthropogenic pressure. How do they affect benthic communities? Mar. pollut. Bull. 181, 113935. doi: 10.1016/j.marpolbul.2022.113935
R Core Team (2022). R: A language and environment for statistical computing (Vienna, Austria: R Foundation for Statistical Computing). Available at: https://www.R-project.org/.
Román M., de los Santos C. B., Román S., Santos R., Troncoso J. S., Vázquez E., et al. (2022a). Loss of surficial sedimentary carbon stocks in seagrass meadows subjected to intensive clam harvesting. Mar. Environ. Res. 175, 105570. doi: 10.1016/j.marenvres.2022.105570
Román S., Vázquez E., Román M., Viejo R. M., Woodin S. A., Wethey D. S., et al. (2022b). Effects of warming on biological interactions between clams and the seagrass Zostera noltei: A case study using open top chambers. Estuarine Coast. Shelf Sci. 276, 108027. doi: 10.1016/j.ecss.2022.108027
Romero J., Martínez-Crego B., Alcoverro T., Pérez M. (2007). A multivariate index based on the seagrass Posidonia oceanica (POMI) to assess ecological status of coastal waters under the Water Framework Directive (WFD). Mar. pollut. Bull. 55, 196–204. doi: 10.1016/j.marpolbul.2006.08.032
Ruesink J. L., Freshley N., Herrold S., Trimble A. C., Patten K. (2014). Influence of substratum on non-native clam recruitment in Willapa Bay, Washington, USA. J. Exp. Mar. Biol. Ecol. 459, 23–30. doi: 10.1016/j.jembe.2014.05.010
Sidak Z. (1967). Rectangular confidence regions for the means of multivariate normal distributions. J. Am. Stat. Assoc. 62, 626–633. doi: 10.2307/2283989
Sih A., Crowley P., McPeek M., Petranka J., Strohmeier K. (1985). Predation, competition, and prey communities: a review of field experiments. Annu. Rev. Ecol. Systematics 16, 269–311. doi: 10.1146/annurev.es.16.110185.001413
Sintes T., Marbá N., Duarte C. M., Kendrick G. A. (2005). Non-linear processes in seagrass colonisation explained by simple clonal growth rules. Oikos 108, 165–175. doi: 10.1111/j.0030-1299.2005.13331.x
Sousa A. I., da Silva J. F., Azevedo A., Lillebo A. I. (2019). Blue Carbon stock in Zostera noltei meadows at Ria de Aveiro coastal lagoon (Portugal) over a decade. Sci. Rep. 9, 14387. doi: 10.1038/s41598-019-50425-4
Tsai C., Yang S., Trimble A. C., Ruesink J. L. (2010). Interactions between two introduced species: Zostera japonica (dwarf eelgrass) facilitates itself and reduces condition of Ruditapes philippinarum (Manila clam) on intertidal flats. Mar. Biol. 157, 1929–2936. doi: 10.1007/s00227-010-1462-0
Turchin P. (1995). “Population regulation: old arguments and a new synthesis,” in Population dynamics: new approaches and synthesis. Eds. Cappuccino N., Price P. W. (New York, NY: Academic Press), 19–40. doi: 10.1016/b978-012159270-7/50003-8
van der Heide T., Tielens E., van der Zee E. M., Weerman E. J., Holthuijsen S., Eriksson B. K., et al. (2014). Predation and habitat modification synergistically interact to control bivalve recruitment on intertidal mudflats. Biol. Conserv. 172, 163–169. doi: 10.1016/j.biocon.2014.02.036
Vieira V. N. C. S., Lobo-Arteaga J., Santos R., Leitão-Silva D., Veronez A., Neves J. M., et al. (2022). Seagrasses benefit from mild anthropogenic nutrient additions. Front. Mar. Sci. 9. doi: 10.3389/fmars.2022.960249
Vieira V.M.N.C.S., Lopes I. E., Creed J. C. (2018). The biomass–density relationship in seagrasses and its use as an ecological indicator. BMC Ecol. 18, 44. doi: 10.1186/s12898-018-0200-1
Walne P. R. (1976). Experiments on the culture in the sea of the Butterfish Venerupis decussata L. Aquaculture 8, 371–381. doi: 10.1016/0044-8486(76)90119-8
Wilson W. H. (1990). Competition and predation in marine soft-sediment communities. Annu. Rev. Ecol. Systematics 21, 221–241. doi: 10.1146/annurev.es.21.110190.001253
Yin X., Chen P., Chen H., Jin W., Yan X. (2017). Physiological performance of the intertidal Manila clam (Ruditapes philippinarum) to long-term daily rhythms of air exposure. Sci. Rep. 7, 41648. doi: 10.1038/srep41648
Keywords: seagrasses, clams, interspecific interactions, estuaries, survival, predation, juvenile stages
Citation: Galván C and Puente A (2023) Exploring the newly emerging effects of native seagrasses on survival and growth of non-native juvenile clams. Front. Mar. Sci. 10:1160734. doi: 10.3389/fmars.2023.1160734
Received: 07 February 2023; Accepted: 01 August 2023;
Published: 11 September 2023.
Edited by:
Emilio Fernández, University of Vigo, SpainReviewed by:
Jennifer Li Ruesink, University of Washington, United StatesVasco Manuel Nobre de Carvalho da Silva Vieira, University of Lisbon, Portugal
Copyright © 2023 Galván and Puente. This is an open-access article distributed under the terms of the Creative Commons Attribution License (CC BY). The use, distribution or reproduction in other forums is permitted, provided the original author(s) and the copyright owner(s) are credited and that the original publication in this journal is cited, in accordance with accepted academic practice. No use, distribution or reproduction is permitted which does not comply with these terms.
*Correspondence: Cristina Galván, Z2FsdmFuY0B1bmljYW4uZXM=