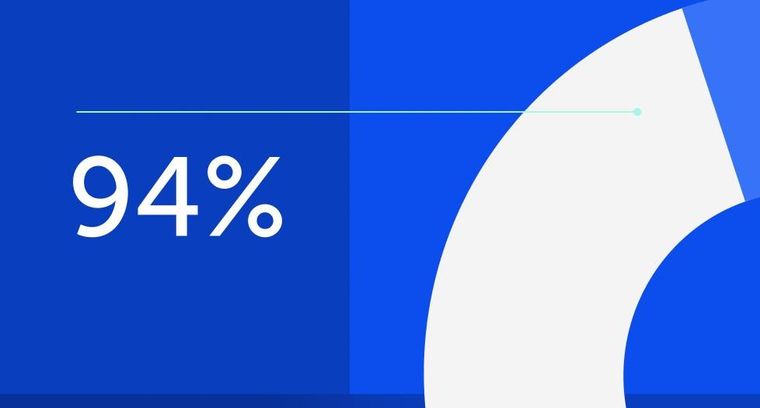
94% of researchers rate our articles as excellent or good
Learn more about the work of our research integrity team to safeguard the quality of each article we publish.
Find out more
REVIEW article
Front. Mar. Sci., 24 April 2023
Sec. Marine Biogeochemistry
Volume 10 - 2023 | https://doi.org/10.3389/fmars.2023.1157879
This article is part of the Research TopicChemical and Biogeochemical Processes at Methane and Other Cold SeepsView all 15 articles
Cold seep foraminifera have attracted considerable attention as they provide valuable insights into the study of cold seeps. This study provides a comprehensive overview of the manifestations of foraminifera in cold seep environments and methane seepage activities. Certain taxa of benthic foraminifera, such as Uvigerina, Bolivina, Bulimina, Chilostomella, Globobulimina, Nonionella, Melonis, Epistominella, Cibicidoides, and Globocassidulina, are known to inhabit geochemical conditions induced by methane-rich environments and may feed on associated methanotrophic microbial communities. Secondary mineralization on foraminifera shells is a widespread manifestation in seep sediments, and alters the microstructure, elementary composition, and isotopic signatures of foraminifera. On one hand, the precipitation of secondary authigenic Mg-rich, Mn-rich, Sr-rich, and Ba-rich calcite coatings have been observed on microfossils. On the other hand, micron-sized crystal pyrite and gypsum aggregates can also grow on the foraminifera walls. The negative δ13C and positive δ18O anomalies in both planktonic and benthic foraminifera from seep-associated sediments can serve as proxies for tracing past seepage activities, either in their live form or being adulterated by methane-derived authigenic carbonate after deposition. Seeping activities are recognized with a significant impact on benthic foraminifera, and the presence of cold seep-related species and significant isotopic anomalies in shells can be used to reconstruct past methane seepage events. Intensive methane seepage tends to suppress benthic foraminifera populations, while moderate intensity seepage may lead to a thriving benthic foraminiferal community, with hypoxia-enduring taxa such as Uvigerina, Melonis, and Bulimina being predominant. In contrast, oxygen-loving epibenthic taxa such as Cibicidoides often occur in areas of low methane flux. Compared to planktonic foraminifera, the single species of benthic foraminifera can provide a more comprehensive record of seepage evolution. Live benthic specimens are preferred for in-situ seep studies, while the superimposition of secondary minerals on the original shells should not be ignored when observing dead individuals. The significance of the evolution of methane seepage, changes in environmental parameters of the living habitat, and species sensitivity in cold seeps are emphasized in explaining the variation in foraminiferal assemblages and fluctuations in stable isotopes.
Marine cold seeps, frequently observed in the vicinity of active and passive continental margin slopes, have been linked to the destabilization of gas hydrate reservoirs (Ceramicola et al., 2018; Suess, 2020). Variations in sea level increase in bottom water temperature, tectonic activity, and fluctuations in deposition rates can trigger instability in gas hydrate reservoirs and lead to repeated episodes of methane seepage (Karstens et al., 2018; Wallmann et al., 2018; Ketzer et al., 2020; Suess, 2020; Sultan et al., 2020). The seep fluids often saturate the pore water with hydrocarbon gases, an essential process of methane oxidation in methane-charged sediments via the action of anaerobic oxidation of methane (AOM, Eq. 1, Caldwell et al., 2008; Knittel and Boetius, 2009). This process is performed by a consortium of anaerobic methane-oxidizing archaea (ANME) and sulfate-reducing bacteria (SRB) (Knittel and Boetius, 2009). The AOM and methane aerobic oxidation processes (Eq. 2, Paull et al., 2005; Reeburgh, 2007) generate carbon dioxide (CO2) and hydrogen sulfide (H2S), which, in turn, resulting in local acidic and anoxic conditions (Niewöhner et al., 1998; Aharon and Fu, 2000; Joye et al., 2010). Elevated carbonate alkalinity promoted by AOM results in the formation of authigenic carbonate minerals. Typically, methane-derived authigenic carbonate (MDAC) is a universal mineral indicator in seep areas and is primarily composed of acicular aragonite, high-Mg calcite, and dolomite (Boetius et al., 2000; Judd et al., 2007; Naehr et al., 2007; Buckman et al., 2020; Judd et al., 2020). However, in some cases, Fe- and Mn-carbonates may also be present (Suess, 2020). Meanwhile, a direct consequence of such stressful conditions is the occurrence of symbolic benthic seep communities. Tube worms, clams, mussels, and microbial mats, are indicator organisms to survive in seep ecological environments (Suess, 2020), and these organisms may provide carbon and energy via AOM (Boetius et al., 2000). Implications are that the association of methane fluids, authigenic minerals, and benthic biota has been regarded as a biogeochemical footprint to identify cold seeps.
Foraminifera, single-celled eukaryotes widely distributed in seawater and deep-sea sediments, have been established as a crucial micro-paleontological foundation for the study of paleoceanography and paleoclimatology (Jorissen, 1992; Schiebel et al., 2018). Environmental information can be obtained through the examination of foraminifera assemblage on a macro-scale, and as well as shell elements and isotopic compositions on a micro scale (Gooday, 1994; Kucera, 2007; Ravelo and Hillaire-Marcel, 2007; Pawlowski and Holzmann, 2008; Schiebel et al., 2018). In recent decades, evidence of deep-sea benthic foraminifera in seep areas has been discovered (e.g. Brooks et al., 1991; Sen Gupta and Aharon, 1994; Martin et al., 2010), and growing interests begins to focus on the identification of seep environments and methane seepage activities via foraminifera research. Apparently, cold seep foraminifera have been served as archives of seep activity, and differences in foraminiferal assemblages, secondary minerals alteration on the shells, and isotopic compositions between seep and non-seep areas can be detected.
The community assemblage of benthic foraminifera (composition, abundance, diversity, and dominant species) have been recognized as an advantageous indicator for the identification of cold seep environments. Data from different oceanic basins spread across the Pacific Rim shelf (Bernhard and Reimers, 1991; Hill et al., 2003; Heinz et al., 2005; Khusid et al., 2006; Zhou et al., 2009; Chen et al., 2010; Martin et al., 2010; Xiang et al., 2012; Saeidi Ortakand et al., 2016; Pan et al., 2018; Xin et al., 2020; Zhou et al., 2020; Li et al., 2021), the North and east Atlantic (Jones, 1993; Sen Gupta and Aharon, 1994; Sen Gupta et al., 1997; Rathburn et al., 2000; Bernhard et al., 2001; Rathburn et al., 2003; Robinson et al., 2004; Bhaumik and Gupta, 2005; Panieri, 2005; Bhaumik and Gupta, 2007; Lobegeier and Sen Gupta, 2008; Panieri and Gupta, 2008; Wilfert et al., 2015), the North Indian Ocean (Erbacher and Nelskamp, 2006), the Arctic marginal seas (Dessandier et al., 2019; Melaniuk, 2021), and Mediterranean (Panieri, 2003; Panieri, 2006) demonstrate the opportunism of benthic foraminifera associates with cold seep habitats (Figure 1). Some benthic foraminifera, including endobenthic taxa such as Uvigerina, Bolivina, Bulimina, Chilostomella, Globobulimina, Nonionella and Melonis, as well as epibenthic taxa such as Epistominella, Cibicidoides and Globocassidulina, are known to withstand methane- and sulfide-enriched seepage conditions. However, no seep-endemic species have been reported to date, as these taxa are well-documented in normal marine environments as well. It should be noted that benthic foraminiferal assemblages (e.g., abundance and diversity) are not congruent in these cold seep areas on a global scale and are influenced by multiple factors. Despite the absence of consistent global patterns in seep benthic foraminiferal assemblages, their sensitive response to seepage environments provides valuable evidence for identifying past methane seepage events (Bhaumik and Gupta, 2007; Zhou et al., 2009; Pan et al., 2018; Li et al., 2021).
Figure 1 Foraminifera associated with the cold seeps in the world. The research areas, where isotopic analyses and/or species studies have already been carried out, are denoted by filled circles, among which green filled symbols indicate the sites with the community composition determined and electron microscopy images or optical microscope images of typical benthic foraminifera given next to them. As for the locations of the red dots, no foraminifera photographs are found, but it is certain that foraminifera related studies were involved. Please refer to Appendix Table 1 for relevant references.
Visible changes in the primary microstructure and elemental composition of seep foraminifera due to diagenetic alteration have been obtained through diversified macro-scale observations. This phenomenon has been described by Electron Probe Micro Analysis (EPMA) (Panieri et al., 2017), Energy Dispersive Spectrometer (EDS) (Schneider et al., 2017; Dessandier et al., 2020; Zhou et al., 2020; Miao et al., 2021), Scanning Electron Microscopy (SEM) (Fontanier et al., 2014; Consolaro et al., 2015; Panieri et al., 2017; Schneider et al., 2017; Wan et al., 2018; Dessandier et al., 2020; Zhou et al., 2020; Miao et al., 2021), Transmission Electron Microscopy (TEM) (Panieri et al., 2017), and Electron Back Scatter Diffraction (EBSD) (Panieri et al., 2017). However, the current understanding from in-situ observations of seep foraminifera may be deficient, as better micro-observations of specimens after visible diagenetic alteration were just from active seep sites of the Arctic Ocean (Consolaro et al., 2015; Panieri et al., 2017; Schneider et al., 2017; Dessandier et al., 2020), the Atlantic Ocean (Merinero et al., 2008; Fontanier et al., 2014; Dessandier et al., 2020), the South China Sea (Wan et al., 2018; Zhou et al., 2020), and fossil seep sites of the Greenland Sea (Millo et al., 2005), the Barents Sea (Argentino et al., 2021), the Oregon Washington coast and Vancouver Island (Torres et al., 2010). In these methane-charged sediments, the geochemical cycles of elements are interconnected with authigenic minerals such as carbonate, pyrite, and gypsum (Ritger et al., 1987; Tribovillard et al., 2006; McQuay et al., 2008; Griffith and Paytan, 2012; Large et al., 2017; Smrzka et al., 2020). Available observations indicate that foraminifera shell components are significantly influenced by seep authigenic mineral precipitation, as evidenced by the formation of authigenic Mg-, Sr-, Mn-, and Ba-rich carbonate coatings, and the micron-sized euhedral gypsum and pyrite growth on the exterior and interior walls of seep foraminifera.
Currently, isotopic signals recorded in foraminifera tests are an ideal index for identifying methane release from cold seeps. The isotopic composition of seawater, including both the pore water in seafloor sediments and the upper water body, undergoes significant changes as a result of the reformative processes of seepage fluids and methane-related biogeochemical processes, making the observation of low δ13C and high δ18O anomalies recorded by foraminifera shells to be widely considered as a marker of methane release at cold seeps (Li et al., 2010; Martin et al., 2010; Panieri et al., 2012). So far, the isotopic composition analysis of foraminifera has revealed the occurrence of methane-release events from various cold seep environments, including the Gulf of California (Keigwin, 2002), the Santa Barbara Basin (Kennett et al., 2000; Hill et al., 2003; Cannariato and Stott, 2004), the Black Ridge (Bhaumik and Gupta, 2007), the Cascadia margin (Hill et al., 2004; Li et al., 2010), the Peru shelf margin (Wefer et al., 1994), the West Svalbard continental margin (Panieri et al., 2014), the Norwegian continental margin (Hill et al., 2012), the Greenland Sea (Smith et al., 2001; Millo et al., 2005), the Fram Strait (Consolaro et al., 2015), the Mediterranean Sea (Panieri et al., 2012), the Japan Sea (Ohkushi et al., 2005; Uchida et al., 2008), the South China Sea (Lei et al., 2012; Wang et al., 2013; Chang et al., 2015; Zhuang et al., 2016), and the Weddell Sea (Thomas et al., 2002) (Figure 1). Additionally, the application of the isotopic composition analysis of foraminifera has enabled the interpretation of previous methane-release events in various geological settings, including the Northern Apennines (Italy) (Panieri et al., 2009), Western Washington State (USA) (Martin et al., 2007), and the Sunda arc (Indonesia) (Wiedicke and Weiss, 2006). Consequently, reconstructions of methane fluxes and the historical evolution of seepage can be performed through the analysis of fluctuations in foraminifera isotopic ratios (Kennett et al., 2000; Li et al., 2010; Wang et al., 2013; Panieri et al., 2014; Consolaro et al., 2015; Zhuang et al., 2016; Zhou et al., 2020).
While multiple proxies based on cold seep foraminifera offer insights into contemporaneous seep areas and seep activities during geological history, certain issues remain unresolved. For example, the selection of scientifically valuable cold seep-related benthic foraminifera species, the mechanism of the foraminifera community assembly response to dynamic seeping activities, and the explanation of fluctuations in foraminifera isotopic values. Additionally, the ability of foraminifera to fully assimilate seawater or pore water methane-derived carbon during biomineralization, and the differing information recorded by living and fossil, planktonic and benthic foraminifera in cold seep areas also contribute to the uncertainty in interpreting the results. Meanwhile, there still have difficulty in distinguishing the stable isotope signatures between primary biogenic calcite and secondary authigenic calcite precipitate driven by AOM on dead specimens. Thus, while the approach of identifying cold seep environments through multiple foraminifera proxies offers significant potential, the influence of mixed interference from other factors necessitates further research to resolve these knowledge gaps.
Benthic foraminifera are widely recognized as valuable indicators of cold seep activities in the seafloor sediment community. In general, the superficial seep sediments exhibit high methane fluxes (up to 90 mmol m–2 d–1; Hill et al., 2004), sulfide concentrations (up to 63 mmol m–2 d–1; Heinz et al., 2005), and total organic carbon content (up to 4%; Bhaumik and Gupta, 2005). The stressful microhabitat of active cold seepages presents a challenge for foraminifera to survive. Numerous studies have investigated the responses of benthic foraminiferal assemblages to seep activity in various seep areas, including the Hydrate Ridge (Torres et al., 2003; Hill et al., 2004; Heinz et al., 2005), the Eel River (Rathburn et al., 2000), the Santa Barbara Basin (Hill et al., 2003), the Kimki Ridge (McGann and Conrad, 2018), the Peru shelf margin (Wefer et al., 1994), the Japan Sea (Uchida et al., 2008; Saeidi Ortakand et al., 2016), the Sea of Okhotsk (Khusid et al., 2006), the South China Sea (Zhou et al., 2009; Xiang et al., 2012; Pan et al., 2018; Xin et al., 2020), the Blake Ridge (Robinson et al., 2004; Bhaumik and Gupta, 2005; Bhaumik and Gupta, 2007), the Gulf of Mexico (Sen Gupta and Aharon, 1994; Sen Gupta et al., 1997; Robinson et al., 2004), the Monterey Bay (Bernhard et al., 2001; Rathburn et al., 2003; Martin et al., 2004), the Irish Sea (Panieri, 2005), the North Sea (Wilfert et al., 2015), the Mediterranean Sea (Panieri, 2003; Panieri, 2006), the Adriatic Sea (Panieri, 2003; Panieri, 2006), the Arabian Sea (Erbacher and Nelskamp, 2006), the Arctic shelf margin (Dessandier et al., 2019; Melaniuk, 2021), and the New Zealand shelf margin (Martin et al., 2010). The distribution of benthic foraminifera in seep sediments is remarkably skewed toward adjacent non-seep-affected environments, which suggests that some organisms may opportunistic to adapt to the challenging geochemical conditions brought by methane seepages (Jones, 1993; Bernhard et al., 2001; Panieri, 2005; Bhaumik and Gupta, 2007; Burkett et al., 2016; Dessandier et al., 2019). In these cold seep areas, the foraminifera assemblage is largely hyaline perforate calcitic or aragonitic species, while imperforate porcelaneous and coarsely agglutinated taxa are infrequent. Some hyaline taxa, such as Uvigerina, Bolivina, Bulimina, Chilostomella, Globobulimina, Nonionella, Melonis, Epistominella, Cibicidoides, and Globocassidulina have been considered as seep-related species due to their higher adaptation to the high organic, low oxygen, reducing conditions, and hypoxia environments rich in CH4 and H2S commonly found in cold seep environments (Appendix Table 1). However, evidence suggests that these foraminifera may not be restricted to the seep environments (e.g. Sen Gupta et al., 1997; Rathburn et al., 2000; Bernhard et al., 2001).
Typically, endobenthic benthic foraminifera exhibit superior tolerance to the harsh conditions of methane seeps, these methane-loving taxa include Uvigerina, Bolivina, Bulimina, Chilostomella, Globobulimina, Nonionella, and Melonis. Uvigerina has been observed to be adaptable to high organic matter, low oxygen, and methane-rich conditions and is likely specialized in methane-oxidizing bacteria (Sen Gupta and Aharon, 1994; Rathburn et al., 2000; Bernhard et al., 2001; Torres et al., 2003). Bolivina is associated with dysoxic bottom waters due to high methane emissions and is known to be resistant to anoxia and sulfide toxicity (Sen Gupta et al., 1997; Hill et al., 2003; Bhaumik and Gupta, 2005). Bulimina is commonly found in seep sediments with high organic matter content (Rathburn et al., 2000; Rathburn et al., 2003; Martin et al., 2004; Wiedicke and Weiss, 2006; Zhou et al., 2009; Xin et al., 2020). Chilostomella is noted to tolerate hypoxia and hostile environments rich in methane and hydrogen sulfide (Corliss, 1985; Pan et al., 2018). Globobulimina is frequently found in Pacific and Atlantic methane seep areas (Sen Gupta et al., 1997; Rathburn et al., 2000; Rathburn et al., 2003; Hill et al., 2004; Martin et al., 2004; Bhaumik and Gupta, 2007). And food supply provided by microbial mats appears to facilitate the survival of Melonis in high-latitude methane-rich environments (Panieri, 2005; Dessandier et al., 2019; Melaniuk, 2021). These hypoxia-tolerant benthic foraminifera are often abundant in methane-rich sediments and serve as proxies for tolerance to stressful seep environments.
However, there are exceptions where densities of a few oxygen-loving epibenthic taxa increase in certain cold seep areas, such as Epistominella, Cibicidoides, and Globocassidulina. Epistominella has been observed to be dominant taxa in seep areas of the North Sea (Jones, 1993), the Monterey Bay (Bernhard et al., 2001; Rathburn et al., 2003), the Gulf of Mexico (Robinson et al., 2004), the northern Adriatic Sea (Panieri, 2006), the Hydrate Ridge (Heinz et al., 2005), and the Santa Barbara Basin (Hill et al., 2003). Cibicidoides has been found in methane-rich bottom waters of the South China Sea (Pan et al., 2018; Xin et al., 2020), the Hydrate Ridge (Torres et al., 2003; Hill et al., 2004), the Gulf of Mexico (Robinson et al., 2004), and the Vestnesa Ridge (Melaniuk et al., 2022). In some cases, like the East Greenland slope (Smith et al., 2001; Millo et al., 2005), the Blake Ridge (Bhaumik and Gupta, 2005), the Northern North Sea (Wilfert et al., 2015), the Vestnesa Ridge (Dessandier et al., 2019), the Margin of Eastern New Zealand (Martin et al., 2010), and the South China Sea (Zhou et al., 2009; Xiang et al., 2012), Cibicidoides also holds a larger advantage. Meanwhile, Globocassidulina is dominant in methane seep sediments associated with oxygen depletion and organic-rich conditions (Sen Gupta and Aharon, 1994; Rathburn et al., 2000; Panieri and Gupta, 2008; Zhou et al., 2009). Previous studies have indicated that these benthic foraminifera commonly attach to the wall of the worm tubes and live a few centimeters to tens of centimeters above the bottom sediments (Mackensen et al., 2006; Lobegeier and Sen Gupta, 2008; Rathburn et al., 2009). This attached lifestyle is likely to allow them to escape extreme seep habitats (Gupta et al., 2007).
Many studies have taken emphasized on the distinction in benthic foraminiferal assemblages between seep and non-seep habitats (Rathburn et al., 2000; Bernhard et al., 2001; Panieri, 2003; Torres et al., 2003; Wiedicke and Weiss, 2006; Dessandier et al., 2019; Li et al., 2021), and environmental parameters such as microhabitat conditions and seepage activities are known to play a crucial role in the survival of these organisms (Figure 2).
Figure 2 Living foraminiferal assemblages associated with methane seepages (after Borowski et al., 1996; Consolaro et al., 2015; Li et al., 2021). (A) Disappear period of benthic foraminifera during times of high methane flux. (B) Boom period of benthic foraminifera during times of moderate methane flux. (C) Stable period of benthic foraminifera during times of low methane flux. (D) Benthic foraminiferal assemblages in different seep microhabitats.
Overall, benthic foraminifera are commonly found in a range of seep ecosystems, such as clam beds, microbial mats, mud volcanoes, pockmarks, and algal reefs (Appendix Table 1). The preference of benthic foraminifera for specific seep microhabitats is influenced by substrate type, oxygen level, and total organic carbon content. Fine-grained sediments rich in clay and mud, typically charged with methane, are abundant in endobenthic taxa (e.g. Bhaumik and Gupta, 2005; Zhou et al., 2009; Xin et al., 2020). While epibenthic benthic foraminifera tend to inhabit in coarse and hard areas, such as algal reefs or environments precipitated with carbonate nodules (e.g. Panieri, 2006; Xiang et al., 2012) (Figure 2D). In general, habitats with high oxygen (O2) levels and low carbon dioxide (CO2) concentrations are conducive to the survival of foraminifera, as a decrease in O2 and an increase in CO2 can limit their survival or symbiotic existence (Herguera et al., 2014). Agglutinate foraminifera thrive in microhabitats with adequate O2 levels and high CO2 concentrations, while the growth of calcareous foraminifera is limited in these conditions (Herguera et al., 2014). During periods of high methane flux and anoxic conditions associated with active seepages, endobenthic benthic foraminifera tend to predominate, while with a decrease in seepage activity and an increase in O2 levels, epibenthic taxa gradually become dominant (Zhou et al., 2009; Pan et al., 2018). In addition, benthic foraminifera have also been found to show sensitivity to the organic flux to the sea floor (Abu-Zied et al., 2008). In seep areas, organic matter can provide additional food sources for foraminifera, and foraminifera with food tendencies, such as Cibicidoides, Bulimina, Uvigerina, Nonionella, and Melonis, have been observed (Wefer et al., 1994; Torres et al., 2003; Dessandier et al., 2019; Xin et al., 2020). Compared to non-seep sites, the significantly lower abundance of agglutinate foraminifera (approximately 10% of the total) in seep sites suggests that they may not be well adapted to the seep geochemical environment (Zhou et al., 2009; Martin et al., 2010; Wilfert et al., 2015; Dessandier et al., 2019). There are, however, exceptions, such as the dominance of agglutinate taxa in the Sea of Okhotsk Deryugin seep area (Khusid et al., 2006).
What’s more, the point is that the benthic foraminiferal communities in cold seep environments are strongly affected by seepage activities. Studies have shown variable results in terms of foraminiferal abundance in seep areas (from several to thousands per 10 cm3), with reports of both decreased (e.g. Panieri, 2003; Bhaumik and Gupta, 2007; McGann and Conrad, 2018; Dessandier et al., 2019), increased (e.g. Rathburn et al., 2000; Torres et al., 2003; Wiedicke and Weiss, 2006), as well as no significant differences from non-seepage sites (e.g. Bernhard et al., 2001; Rathburn et al., 2003). Although seep environments are known to be harmful to the survival of benthic foraminifera (Dickens et al., 1995; Dickens et al., 1997; McConnaughey et al., 1997; Bernhard and Bowser, 1999), recent studies suggest that the relationship between seepage activities and benthic foraminifera abundance is more complex (Figure 2). Foraminifera are generally thought to prefer an aerobic methane environment with low methane flux, but the bacteria that flourished during the period of moderate-flux methane seepage may provide a food source for benthic foraminifera, resulting in higher abundance (Figure 2B, Li et al., 2021). The poisonous sulfidic environment during high-methane flux periods will lead to the death of the foraminifera, while the benthic foraminifera community in low-methane flux periods may be similar to that of the normal marine sediments (Figure 2, Consolaro et al., 2015; Li et al., 2021; Melaniuk et al., 2022). Additionally, the density of living foraminifera is related to the thickness of microbial mats, with higher taxa observed in thicker mats due to a greater food supply (Robinson et al., 2004). The depth distribution of benthic foraminifera is also influenced by redox conditions in methane-rich sediments. Typically, the redox boundary is close to the sediment-water interface and may be lower than 1 cm (Sen Gupta and Aharon, 1994). which restricts the distribution of living foraminifera within 0.5-3 cm below the sediments, where they are primarily hypoxia-tolerant epibenthic species (Rathburn et al., 2000; Erbacher and Nelskamp, 2006; Melaniuk, 2021).
The precipitation of authigenic calcite is an anticipated process due to the typically intense AOM and inherent saturation of CaCO3 in seep-associated sediments (Figure 3A). Authigenic Mg-calcite overgrowth is the dominant form of secondary mineral coatings observed on the macroscopic tests of foraminifera, with a contribution to the shell weight reaching up to 58 wt% (Millo et al., 2005; Schneider et al., 2017; Wan et al., 2018). This microcrystalline Mg-calcite overgrowth may overlay the whole foraminifera surface and is structurally identical to the primary biogenic calcite (Panieri et al., 2017). As diagenetic alteration intensifies, the precipitation of MDAC on the shell is cumulatively added. The shell surfaces from “glassy” to “translucent,” and even “fuzzy,” eventually forming a solid inorganic carbonate crust, which results in the loss of the shell’s smooth surface texture, with both the interior and exterior walls covered and thicken (Schneider et al., 2017). The “glassy” shells under biomineralization processes usually show low magnesium content below 0.2 wt% MgCO3 (Blackmon and Todd, 1959; Bentov and Erez, 2006), whereas in modern active seep sites the Mg/Ca ratios of benthic foraminifera tests can higher than 10 mmol/mol (Panieri et al., 2017; Wan et al., 2018). Furthermore, diagenesis-altered benthic foraminifera tests from ancient seep sites have been reported to exhibit spots with high Mg (Cassidulina neoteretis, Cassidulina reniforme, and Melonis barleeanum, Argentino et al., 2021), and the Mg/Ca ratios could up to 69 mmol/mol (Globobulimina pacifica, Torres et al., 2010). The elevated Mg content of original shells is considered to benefit from Mg-rich interlayers with Mg/Ca ratios even reaching 220 mmol/mol (Panieri et al., 2017) (Figure 3B). To some extent, the increasing Mg/Ca ratio of altered foraminifera shells reflects the heightened activity of AOM and authigenic carbonate precipitation, which in turn signifies changes in the activities of seepage (e.g. Torres et al., 2003; Li et al., 2010).
Figure 3 Authigenic secondary minerals precipitation and their alteration to foraminifera shells in seep-associated sediments. (A) Typical authigenic secondary minerals precipitation, and the equations utilized to depict this process are derived from the works of Berner (1982); Borowski et al. (2013); Liu et al. (2018), and Miao et al. (2021). (B) Altered foraminifera coated with high-Mg carbonate interlayers. (C) Altered foraminifera infilled with authigenic pyrites.
Secondary mineralization can also be traced with the use of Sr, Mn, and Ba content of foraminifera. Sr and Mn are typical elements to determine the extent of alteration associated with meteoric diagenesis, as Mn can be incorporated into carbonate while Sr will separate from carbonate during diagenetic alteration (Kaufman and Knoll, 1995). The Sr/Ca of benthic foraminifera in seep areas is usually around 1 mmol/mol, but individual tests could reach up to 36.48 mmol/mol (Torres et al., 2010), which may be related to the precipitation of Sr-rich aragonite (Tesoriero and Pankow, 1996; Fontanier et al., 2014). The Mn/Ca ratio of foraminifera in case of natural calcification ranges from 0.05 to 0.3 mmol/mol (Klinkhammer et al., 2009), while the Mn/Ca ratio of benthic foraminifera in seep-associated sediments can exceed 1 mmol/mol, or even up to 12 mmol/mol (Torres et al., 2010). Moreover, foraminifera modified by cold seeps in high latitudes display spots of high Ba content under EDS images (Dessandier et al., 2020). These trace elements can mask microbial shells by incorporating carbonates after the alteration of diagenesis and may play an important role in seep elemental cycling.
An association of authigenic gypsum with pyrite tends to occur within the sulfate-methane transition zone (SMTZ), which also marks the methane seepage events (Figure 3A; Lin et al., 2016a, b; Zhou et al., 2020; Dantas et al., 2022). The fluid released by natural gas hydrate decomposition or seepage carries dissolved barium (Ba2+) (Hu et al., 2014), resulting in a higher Ba content in bottom water and usually precipitated in the form of gypsum (CaSO4·2H2O) in surface sediments (Pierre, 2017). Macroscopic observations of gypsum in methane-charged sediments reveal rhombic, bladed, lenticular, or trapezoidal shapes (Kocherla, 2013; Lin et al., 2016a). By now, crystal growth of gypsum microcrystals on foraminifera shells in the seep sediments of the Nansha Trough (Zhou et al., 2020) and the Santos Basin (Dantas et al., 2022) has been observed.
Pyrite (FeS2) is a ubiquitous sulfide mineral in marine sediments and plays a crucial role in the global Fe-S cycle (Berner, 1985). In cold seep environments, the formation of pyrite is closely linked to the presence of methane upwelling, as hydrogen sulfide produced by AOM action may be the main electron donor for pyrite formation (Schippers and Jorgensen, 2002; Zhang M. et al., 2018). Framboidal pyrite, which forms via authigenic crystallization, is known as the typical morphology in methane-charged sediments (Peckmann et al., 2001; Lin et al., 2016b). Additionally, pyrite may also precipitate in bacterial forms, such as spheroids, rod-chains, and worm-like structures (Chen et al., 2006). Pyrite infill in foraminifera chambers is not extraordinary and typically exhibits framboids and aggregates forms (Figure 3C). In recent years, numerous studies have reported the presence of pyrite infillings in foraminifera shells from various cold seep sites, including the western Svalbard (Panieri et al., 2017), the Haima seep (Miao et al., 2021), the Nansha Trough (Zhou et al., 2020), the South China Sea (Zhuang et al., 2016), and the Gulf of Cadiz (Merinero et al., 2008). From another perspective, many trace elements can incorporate into the pyrite structure as solid solution or as sulfide/metal microinclusions (e.g. As, Cu, Zn, Pb, Sb, Mo, Ag, Cd, Mn, Hg, Au). or directly through ionic substitution (e.g. Ni2+, Co2+, Cu2+, Se2-, Te2-) (Large et al., 2017), These pyrite-filling aggregates can influence the elemental composition of foraminifera shells, but the quantification of trace element cycling processes remains challenging due to a lack of relevant findings.
It is generally believed that the apparent lack of 13C in foraminifera shells is invoked as an indicator of methane seepages. There have been published that use foraminifera carbon isotopic signatures to reconstruct ancient seepages and variable methane fluxes (e.g. Consolaro et al., 2015; Panieri et al., 2016; Zhou et al., 2020). During the destabilization of hydrate reservoirs, large amounts of 13C-depleted methane are released and anaerobically consumed when they reach the seafloor (Ruppel and Kessler, 2017; Egger et al., 2018). Carbon isotope signals will be conveyed into the pore water dissolved inorganic carbon (DIC) pool in the form of via AOM (Ussler and Paull, 2008). And by so doing, benthic foraminifera often have low δ13C values by incorporating 13C-depleted DIC during calcification (Hill et al., 2003; Rathburn et al., 2003). Given that AOM mainly occurs within the SMTZ near the top of the sediments, the carbon isotope deficit is most pronounced in this region (e.g. Torres et al., 2003; Ussler and Paull, 2008).
Most carbon isotopic composition of benthic foraminifera shells, both living and fossil, from methane seepage environments have been observed to range from 3‰ to -22‰ PDB, with one exception of -35.7‰ PDB (Martin et al., 2010), in contrast, the isotopic composition of ancient seep specimens have been observed to be lower than -50‰ PDB (Appendix Table 2, Figure 4). Methane in gas hydrates can have a variety of sources, such as thermogenic, biogenic or a mixture, with a typical carbon isotope value of methane ranging from -30% to -110% PDB, and typically lower than -60% PDB (Sackett, 1978; Kvenvolden, 1993; Whiticar, 1999). The carbon isotope of pore water DIC (δ13CDIC) in normal marine sediments is typically between -1 and 1‰ PDB (Tagliabue and Bopp, 2008), but can become lower than -45‰ PDB when corporate by methane-derived carbon (Rathburn et al., 2003; Torres et al., 2003). Apart from that, the carbon isotope of authigenic carbonate (δ13Ccarbonate) precipitates related to methane oxidation processes is commonly lower than -40‰ PDB (Whiticar, 1999). Although carbon isotopic values of benthic foraminifera (δ13CBF) in seep sediments exhibit discernable negative shifts compared to those living in normal marine sedimentary environments (-1 to 1‰ PDB, McCorkle et al., 1990), the shifts still remain far from the magnitude observed in δ13CDIC or δ13Ccarbonate influenced by methane (Figure 4). Overall, most bulk values of δ13CBF have been observed to be concentrated between 0‰ and -16‰ PDB, which suggests a disequilibrium between δ13CBF and methane-derived δ13CDIC.
Figure 4 Summary of carbon and oxygen isotopic records of benthic foraminifera in seep sediments. The most commonly adopted endobenthic species (Uvigerina spp., Bolivina spp., Bulimina mexicana, and Globobulimina spp.) and epibenthic species (Cibicidoides spp.) are selected. δ13C values of live and dead benthic specimens are represented by solid dots and open circles, respectively. Similarly, δ18O values are represented by triangles. The black square represents the δ13C results of ancient seep samples. The solid gray line in the middle of two symbols represents the fluctuation range of the isotopic value, but it does not mean continuous change. Dotted blue line is a guide for the normal marine average δ13CBF value, which is selected as 0‰ PDB here. Please refer to Appendix Table 2 for data sources.
Planktonic foraminifera living in the upper water column are generally considered to be isolated from the light carbon isotope signals from methane seepage, due to processes such as AOM in sediments, diffusion, and dissolution in seawater, as well as consumption by methanotrophic microbes (Damm et al., 2005; Steinle et al., 2015; Ruppel and Kessler, 2017; Egger et al., 2018). Despite this, several studies have reported the occurrence of simultaneous negative shifts in both δ13CBF and carbon isotopic values of planktonic foraminifera (δ13CPF), which could potentially be indicative of methane emissions. For example, Kennett et al. (2000) observed that δ13CPF of the Santa Barbara Basin sediments changed over the past 6000 years, but this change was not significant (higher than -3.5‰). Additionally, Hill et al. (2012) found that methane venting not only had an impact on the isotopic composition of benthic foraminifera, but also caused significant negative shifts in the δ13CPF of Neogloboquadrina pachyderma in the Nyegga pockmark field. Zhuang et al. (2016) pointed out that the δ13CPF of Globigerinoides ruber was depleted (as low as -5.68‰ PDB), but not to the same extent as the benthic foraminifera Uvigerina peregrina, which was depleted as low as -15.85‰ PDB. McGann and Conrad (2018) found the same lower δ13C peaks in both benthic and planktonic tests, which may provide the evidence of methane releases large enough to influence the water column. Zhou et al. (2020) determined two major paleo-methane seepage events according to anomalously δ13CPF shifts of Globigerinoides ruber from seep sediments in the South China Sea. Although benthic foraminifera generally exhibit more obvious shifts in isotopic composition, there are also instances where δ13CPF shifts are significantly depleted (e.g. Smith et al., 2001; Ohkushi et al., 2005). In these cases, careful analysis and consideration of the potential impact of secondary environmental factors on the death of planktonic foraminifera shells is necessary.
The high δ18O values of foraminifera are also considered as evidence of seep activity due to the release of large amounts of 18O-rich water molecules during hydrate decomposition (Hesse and Harrison, 1981; Davidson et al., 1983; Matsumoto and Borowski, 2000). This process occurs deeper than SMTZ, thus its influence extends deeper than the record of 13C depletion (Dessandier et al., 2020). Positive δ18O shifts have been observed in the shells of both living and fossil benthic foraminifera at modern seeps in various locations, such as the South China Sea (Chang et al., 2015), the Barents Sea (Mackensen et al., 2006), the Hikurangi Margin of eastern New Zealand (Martin et al., 2010), the Niger delta (Fontanier et al., 2014), and core records from the Cascadia Margin of northeastern Pacific (Li et al., 2010), the Weddell Sea of Southern Ocean (Thomas et al., 2002), and the Western Mediterranean Sea (Panieri et al., 2012). These shifts of oxygen isotope of benthic foraminifera (δ18OBF), which range from 0‰ to 4.5‰ PDB in bulk analyses, are positively correlated with the period of methane emission but not remarkable compared to δ13CBF in the same tests (Appendix Table 2).
The oxygen isotope of planktonic foraminifera (δ18OPF) is of critical importance on detecting abnormal climate events since the last glacial period. The marine oxygen isotope record (MIS) has been constructed based on this proxy, which largely reflects the expected changes in paleotemperature (Broecker and Van Donk, 1970; Schiebel et al., 2018). As a result, the δ18OPF is frequently used to infer both paleotemperature changes and sea-level changes that may have induced methane discharge (Smith et al., 2001; Thomas et al., 2002; Hill et al., 2003). Although some clues of seepage can be detected through δ18OPF tests, it is more likely to be represented by the carbonate deposits precipitated by AOM rather than the original calcification record (e.g. Dessandier et al., 2020).
Compared to δ13C records, the δ18O signatures of foraminifera are less informative of methane seepages, leading to a lack of correlation between δ13C and δ18O views. Three main factors contribute to this phenomenon. Firstly, there may not be a clear synergy between δ18OBF and δ13CBF, as δ18OBF is more prone to isotopic equilibrium zero-point rather than reflecting δ18O values in surrounding water bodies (McCorkle et al., 1990). Secondly, δ18OPF is highly sensitive to temperature changes, which is often used to reconstruct seawater temperature. It has been shown that δ18OBF becomes heavier with deeper water depth (lower temperature) (Sen Gupta and Aharon, 1994; Cheng et al., 2005; Erbacher and Nelskamp, 2006; Chang et al., 2015). Finally, the post-depositional alteration to dead individuals during the burial process, such as the precipitation of authigenic carbonates, cannot be disregarded and is difficult to measure.
The carbon and oxygen isotopes of foraminifera are primarily influenced by vital effects, ecological preferences and environmental parameters such as pH and temperature in vivo (Duplessy et al., 1970; Zahn et al., 1986; Bemis et al., 1998; Barras et al., 2010). However, it is also important to consider the secondary environmental impacts on the shells of dead individuals. In the context of cold seep research, the interpretation of the carbon and oxygen stable isotope values of foraminifera shells remains a subject of controversy and can be summarized into five aspects.
Since pore water δ13C is often a negative shift due to the decomposition of organic matter in sediments, making δ13CDIC is closely related to organic carbon fluxes from the overlying seawater (McCorkle et al., 1990). In a previous study, Stott et al. (2002) posited that sedimentation of photosynthetically produced organic matter in the upper water column led to the release of into pore water through anaerobic oxidation (Eq. 3), which ultimately resulted in a negative shift in δ13CBF. In low-seepage or non-seepage environments, the anaerobic oxidation of organic carbon was found to be the primary factor affecting the δ13CBF (Chang et al., 2015). On the other hand, some studies have shown that there may not be a direct relationship between the organic carbon content and the δ13C of foraminifera in areas with high methane flux, as the δ13CBF is primarily influenced by methane seepage (Wefer et al., 1994; Chen et al., 2007).
Despite previous assumptions, some studies have uncovered that the carbon and oxygen isotope fluctuations of benthic foraminifera may not necessarily support the presence of methane release events, but rather demonstrate stronger correlations with the glacial-interglacial cycle during the Quaternary period. For instance, Hill et al. (2012) found that the δ18OBF and δ18OPF in a pockmark of the Storegga Slide complex demonstrated a clear transition between glacial and deglacial stages. In Site 973-4 of the South China Sea, benthic foraminifera Uvigerina spp. failed to record a methane-derived signature during the last sea-level lowstand, but instead reflected an increase in organic carbon input during the ice age (-2.26‰ PDB during Last Glacial Maximum, Zhang M et al., 2018). Additionally, the δ13CPB of Pulleniatina obliquiloculata showed a positive shift (0.25-1.25‰ PDB) during the seepage period, although the source of short-term light carbon remains uncertain (Zhang B et al., 2018). Similar observations were made in the Shenhu area (Chen et al., 2010) and the Adriatic Sea (Panieri, 2006), where the stable isotope records of foraminifera were deemed to be independent of methane seepages.
The carbon and oxygen isotope signals of foraminifera are influenced by various vital activities, including predation, metabolism, and symbionts. During their lifespan, benthic foraminifera exhibit restricted incorporation of 13C depleted methane-derived carbon (Herguera et al., 2014). Spero and Lea (1996) found that food uptake accounted for 8% to 15% of the δ13CPF in Globigerina bulloides. Additionally, metabolic processes may mix depleted δ13C into foraminifera shells (Erez, 1978; Grossman, 1987; Herguera et al., 2014). Bacteria thriving in methane-influenced environments, such as Beggiatoa (Panieri, 2006), may provide food for benthic foraminifera, and these microbes have a distinct light carbon isotope composition (Jiasheng et al., 2007). Some taxa incorporate the carbon derived from methane into their shells via predation and/or the incorporation of ambient DIC (Bernhard and Reimers, 1991; Panieri, 2006; Melaniuk et al., 2022). Recent studies suggest that benthic foraminifera species, such as Uvigerina peregrina (Bernhard et al., 2001; Torres et al., 2003), Nonionella auris (Wefer et al., 1994), and Melonis barleeanus (Dessandier et al., 2019) could record light δ13C values by feeding on methanotrophic bacteria and/or incorporating surrounding DIC (Melaniuk et al., 2022).
Apart from this, the presence of symbiotic bacteria within the foraminifera body may have a significant impact on the isotopic signals of living species. The symbiotic relationship between foraminifera and their endosymbiotic bacteria has been noted by several studies (e.g. Bernhard et al., 2001; Barbieri and Panieri, 2004). Despite the recent discovery of chemoautotrophic symbionts in some living seep-related species in 2010, the positive staining reaction of these endobionts with Rose Bengal raises questions about the reliability of isotopic signals produced by living foraminifera (Bernhard et al., 2010). This highlights the need to further investigate the influence of symbiotic bacteria on the isotopic composition of living foraminifera.
Studies have consistently shown that the analysis of seep activity is often performed by comparing isotopic records obtained from the same species or taxa of foraminifera. This is because the sensitivity of foraminifera to their microhabitat plays a crucial role in the interpretation of seepage intensity (Zahn et al., 1986; McCorkle et al., 1990; McGann and Conrad, 2018). The shallow infaunal species, such as Uvigerina peregrina, are known to reflect the isotopic signature of the pore water below the sediment-water interface, while deep infaunal species, such as Globobulimina pacifica, record the isotopic signature in the depth of dissolved oxygen concentration near zero, in contrast, epifaunal species, such as Cibicidoides wuellerstorfi, primarily record the bottom water above the sediment-water interface (McCorkle et al., 1990; Keigwin, 1998; McGann and Conrad, 2018). Similarly, planktonic foraminifera, such as Globigerinoides ruber, Neogloboquadrina dutertrei and Pulleniatina obliquiloculata, mainly record seawater information corresponding to the depth of their living environments (Hemleben et al., 1989). It is suggested that benthic individuals are more likely to in-situ record isotopic signals from seep environments compared to their planktonic counterparts, and therefore may have recorded the excursion prior to the latter (Thomas et al., 2002; Martin et al., 2004).
The vital effect refers to the difference in isotopic fractionation between different species of foraminifera, as well as between juveniles and adults of the same species, due to biological processes, such as metabolism. This effect has been shown to result in fluctuations of ~1-2‰ δ13C values within a single specimen (Rathburn et al., 2000; Rathburn et al., 2003; Panieri, 2006; Melaniuk et al., 2022). Corliss (1985) proposed that reduced absorption of 12C during low-productivity periods leads to the depletion of 13C and lower carbon isotope values of foraminifera. Rathburn et al. (2003) pointed out that different species of benthic foraminifera exhibit distinct ranges of isotope fluctuations, but results from a single species have the potential to be valuable in evaluating methane seepage, assuming no carbonate diagenesis. McGann and Conrad (2018) observed δ13C depletion in benthic foraminifera from the Kimki Ridge seep, however, multiple fluctuations were evident among species living above or below the water-sediment interface and in shallow or deep sediments. Additionally, the shell size of planktonic foraminifera has also been proposed as a factor in paleoenvironment reconstruction, with a size of 270-320 μm being suggested as most suitable by Spero and Lea (1996). However, Dessandier et al. (2020) found no significant effect of shell size on the results of stable isotope tests in seep environments. While the mechanisms of microhabitat preference and species-specific vital effects are still unclear, it is widely acknowledged that signals from the same species can provide valuable information in investigating and interpreting cold seep environments.
It can be stated that the δ13C values of benthic foraminifera reflect ambient methane concentration to some extent, serving as an indicator of the intensity of methane seepages. Previous studies have demonstrated that a significant negative shift in foraminifera δ13C values is commonly associated with high-efficiency AOM during periods of intense methane release (e.g. Millo et al., 2005; Uchida et al., 2008; Consolaro et al., 2015; McGann and Conrad, 2018). On the other hand, normal marine δ13C values may be observed during periods of no or low methane seepage, where AOM cannot affect the shallow pore water DIC and living foraminifera (Sen Gupta and Aharon, 1994; Chen et al., 2007). However, the relationship between methane seepage intensity and foraminifera activity is complex and not straightforward. A recent study thought that foraminifera communities tend to thrive and actively incorporate methane-derived carbon during periods of moderate seepage, whereas high methane discharge could result in the death of foraminifera and secondary signals begin to superimpose (Melaniuk et al., 2022). Additionally, it should be considered that the growth of foraminifera shells is not continuous and that methane seepage events in geological history are dynamic and transient (Thatcher et al., 2013), making it challenging to discern short-term or rapid methane emissions through shell samples (Dessandier et al., 2020).
To explain the mismatch between δ13C values of benthic foraminifera and pore water DIC, a lot of research has made the efforts. Early research suggested that periods of excessive methane flux have the potential to induce foraminiferal migration, thereby leading to an imbalance carbon isotope records between the biogenic carbonate and pore water DIC (Bernhard and Bowser, 1999). Another explanation is that the extreme circumstance of active AOM may inhibit the metabolism of foraminifera (Sen Gupta and Aharon, 1994). From the results of methane seepage-emulating culture experiments, it is true that methane release can continue to affect the isotopic composition of deep-sea benthic foraminifera (Wollenburg et al., 2015). As for the variability of foraminifera isotopic values, the mixing of different methane sources (e.g. thermogenic and biogenic) with DIC and marine organic carbon seems to be the most plausible explanation (Martin et al., 2007). Despite numerous studies addressing this discrepancy, there remains a lack of clarity regarding the mechanism by which foraminifera fail to record equivalent DIC signals in seep areas.
It has been confirmed that MDAC can capture methane-derived 13C-depleted (may lower than 60 ‰ PDB) and 18O-enriched signatures (may higher than 8.9 ‰ PDB) from seep environments (Naehr et al., 2007; Feng et al., 2018; Buckman et al., 2020). Foraminifera fossil shells in sediments are susceptible to MDAC secondary overgrowth after diagenetic alteration, which will obscure the original information contained in the shells (e.g. Torres et al., 2003; Millo et al., 2005; Martin et al., 2007; Sexton and Wilson, 2009; Panieri et al., 2012; Edgar et al., 2013; Consolaro et al., 2015). Both planktonic and benthic foraminifera shells can be affected by this process, especially in deep sediments and specimens from ancient cold seep locations (Figure 5).
Figure 5 Impact on foraminifera stable isotopic signatures from methane seepage intensity and the MDAC-covered coating. (A) Impact during high methane flux period. (B) Impact during low methane flux period. (C) Zoom schematic of the MDAC-covered coating on foraminifera shell.
Indeed, the extreme values in stable isotopes of both benthic and planktonic foraminifera tests are believed to show an information superimposition by secondary overgrowth of MDAC (e.g. Martin et al., 2010; Fontanier et al., 2014; Panieri et al., 2014). These altered shells possess highly variable and extremely depleted δ13C signals, ranging from -1.6‰ to -55.3‰ PDB (Torres et al., 2003; Hill et al., 2004; Martin et al., 2007; Uchida et al., 2008; Panieri et al., 2009; Martin et al., 2010; Fontanier et al., 2014; Panieri et al., 2016), and false positive shifts in δ18O, ranging from 1.5‰ to 5.3‰ PDB (Ohkushi et al., 2005; Uchida et al., 2008; Naehr et al., 2009; Dessandier et al., 2020).
To quantify the relative amount of MDAC overgrowth on foraminifera shells, Schneider et al. (2017) introduced a formula that takes into account the primary calcification and secondary overgrowth of MDAC (Eq. 4). This method is on the basis that the δ13C of foraminifera is composed of two segments: the primary calcification and the secondary overgrowth of MDAC (Figure 5C). Results based on this formula indicate that in the Vestnesa Ridge seep sediments, the δ13CBF signals of Cassidulina neoteretis were remarkably mixed by MDAC with a contribution ranging from 55-58% (Schneider et al., 2017). Similarly, in the South China Sea, where seepage activity is relatively weak, MDAC-coupled δ13C signals were found to contribute 20-30% in benthic specimens (Uvigerina peregrina and Cibicidoides spp.) and 11-20% in planktonic specimens (Globigerinoides ruber and Pulleniatina obliquiloculata) (Wan et al., 2018)
δ13Cbulk-foram represents the δ13C of 15 to 28 individual foraminifera, δ13Cpristine-foram represents assumed δ13C of pristine foraminifera, and δ13CMDAC represents an average δ13C of some MADC samples.
Notice that the presence of MDAC on foraminifera shells obscure the original information of primary biomass shells, proper cleaning procedures may necessary to obtain the raw isotopic signals. Three cleaning protocols have been established for now, including the use of methanol proposed by Chen et al. (2005), a comprehensive chemical method designed by Barker et al. (2003), and an acid-leaching approach by Millo et al. (2005) and Uchida et al. (2008). The high contribution rates of the MDAC coating to the results may emphasize the importance of properly distinguishing primary and secondary signals in foraminifera shell tests. Results from these procedures revealed that MDAC can dominate up to 10-20% of the δ13C signals in foraminifera shells from Quaternary sediments in the Southwest Greenland Sea (Millo et al., 2005), and can contribute up to 22% for planktonic foraminifera and 15% for benthic foraminifera in sediments from Hokkaido, Japan (Uchida et al., 2008). Despite attempts to remove MDAC coatings through leaching experiments, negative δ13C and positive δ18O values persisted, suggesting that both primary calcification and secondary overgrowth contribute to the excursions of isotopic signatures in foraminifera shells (Panieri et al., 2012; Consolaro et al., 2015). It can be concluded that seepage signatures can be achieved through both primary calcification and secondary overgrowth of MDAC. And by so, foraminifera calcite could still able to exhibit visible excursions in δ13C even after cleaning by a standard method, and major ancient methane release events or modern seep environments could be identified (e.g. Millo et al., 2005; Wan et al., 2018; Zhou et al., 2020).
However, the altered specimens may difficult to identify via light microscopy, and there still remains arduous to selectively eliminate MDAC from the unaltered shells through the process of exhaustive cleaning (Panieri et al., 2017), making the cleaning process to be a subject of debate. Several publications show specimens with pristine microstructures free from secondary mineral overgrowths in methane-charged sediments (Hill et al., 2003; Hill et al., 2004; Martin et al., 2007), and the stable isotopic composition of foraminiferal shells may be not significantly affected by authigenic calcite precipitates (Hill et al., 2004; Mackensen et al., 2006; Panieri et al., 2012). To mitigate the influence of secondary minerals on isotope results, some studies have opted to focus exclusively on specimens that are well-preserved, with complete individuals and distinct rims or bright surfaces (e.g. Fontanier et al., 2014; Zhuang et al., 2016; McGann and Conrad, 2018). However, the effort might somewhat limited since the secondary coatings may thin or infill the interior shell walls (e.g. Torres et al., 2003; Hill et al., 2004; Hill et al., 2012). Typically, the diagenetic alteration of foraminifera calcite is comparatively less problematic in benthic as opposed to planktic foraminifera (Uchida et al., 2008; Edgar et al., 2013). There are indeed reports to reconstruct ancient seepage history by distinguishing negative excursions in untreated benthic foraminiferal δ13C (e.g. Hill et al., 2012; Panieri et al., 2014; Panieri et al., 2016). Additionally, the negative δ13C values of planktonic foraminifera tests buried in seep environments are largely believed to be amplified by diagenetic overprinting with MDAC during methane seepage, rather than a true signal of biogenic carbonates (e.g. Cannariato and Stott, 2004; Ohkushi et al., 2005; Hill et al., 2012; Consolaro et al., 2015; Wan et al., 2018). Incidentally, almost all of the extreme isotopic values reported in prior studies were obtained from dead specimens that appear to have undergone post-depositional alteration (e.g. Torres et al., 2003; Hill et al., 2004; Millo et al., 2005; Martin et al., 2010). However, it is undeniable that the remarkably depleted δ13C values of altered foraminifera indirectly confirm the formation of authigenic carbonates in proximity to the shift of the SMTZ and the associated alteration in past methane release events.
(1) The presence of specific types and assemblages of benthic foraminifera in cold seep sediments reflects the micro-environmental conditions associated with dynamic methane seepages. Despite significant research efforts, the endemic species of benthic foraminifera in seep environments remain to be identified. The dominated assemblages are typically hyaline, some taxa may able to thrive in seep areas due to their high tolerance to the challenging conditions and/or feed on associated methanotrophic microbial communities. These benthic foraminifera, including endobenthic taxa such as Uvigerina, Bolivina, Bulimina, Chilostomella, Globobulimina, Nonionella, and Melonis, as well as epibenthic taxa such as Epistominella, Cibicidoides, and Globocassidulina, provide valuable opportunities to study the seep environments.
(2) The assemblage patterns of benthic foraminifera in cold seep environments are found to be inconsistent and influenced by various environmental factors, such as seepage activity, oxygen levels, total organic carbon content, substrate type, and microhabitat conditions. In areas of high seeping intensity, hypoxia-tolerant endobenthic species tend to dominate the community within the sediments closest to the seafloor. Meanwhile, agglutinate taxa may experience survival difficulties in these harsh conditions. In contrast, when seepage intensity decreases and environmental conditions become less harsh, oxygen-loving epibenthic species may become abundant. It is expected that benthic foraminifera communities will experience maximal flourishing during periods of moderate seepage intensity, where food supplies are abundant and conditions are more favorable for microbial growth.
(3) In methane-charged sediments, a pristine shell of foraminifera may undergo widespread alteration by secondary mineralization after the death of the organisms. It can be inferred that foraminifera shells buried in seep-associated sediments may exhibit overprinting signals of secondary authigenic carbonate-bound (Mg, Sr, Mn, Ba) coatings and the growth of gypsum and pyrite microcrystals. The alteration of secondary mineralization to seep foraminifera reveals a magnesium, barium, and strontium incorporation in carbonates formed within SMTZ, and the appearance of gypsum and pyrite shows a typical signature of cold seep environments. Typically, the degrees of secondary mineralization may be served as a valuable proxy for the intensity of AOM and associated post-depositional alteration during methane seepages.
(4) The detection of abnormal negative δ13C and positive δ18O values in foraminifera shells is considered a unique fingerprinting of methane release events. Benthic foraminifera, which live above or below the surface of seafloor sediments, are known to be reliable recorders of methane-derived light carbon isotopes and heavy oxygen isotopes released from hydrate decomposition. While this isotopic imprinting may also be observed in planktonic foraminifera tests which are likely to have been altered by methane-derived authigenic carbonate.
(5) The variations in δ13C and δ18O signatures preserved in foraminifera shells may reflect the influence of external organic matter inputs, biochemical processes, microhabitat parameters, seeping activities, and post-burial secondary authigenic carbonate overgrowth. To minimize these uncertainties, it is advisable to use live foraminifera to study modern cold seep activities, as they provide the best record of in situ isotopic data. Benthic species are preferable to planktonic individuals as they are thought to have a higher potential to capture methane-derived light carbon isotopes and heavy oxygen isotopes. To effectively evaluate the evolutionary history of a seepage, the isotopic results of a single species must be considered in conjunction with other geological and environmental data. Cleaning protocols can be utilized before stable isotopic analyses to distinguish the signals originating from primary biogenic calcite and secondary carbonate coatings. Foraminifera whether after strict cleaning procedures, or selected under microscopic to minimize authigenic contamination, or in their original state, cannot completely eliminate the influence of secondary carbonate isotopes signals, but all have the potential to exhibit a response to the cold seep environments.
YHL: Data collection, Writing original draft; HY: Data collection, Writing–review and editing; BH and YJL: Writing–review and editing; HL: Conceptualization, Supervision, Writing–review and editing. BH supervised the study; YHL wrote the original draft; HY, HL, and YJL contributed to refining the draft. All authors contributed to the article and approved the submitted version.
The authors declare that the research was conducted in the absence of any commercial or financial relationships that could be construed as a potential conflict of interest.
All claims expressed in this article are solely those of the authors and do not necessarily represent those of their affiliated organizations, or those of the publisher, the editors and the reviewers. Any product that may be evaluated in this article, or claim that may be made by its manufacturer, is not guaranteed or endorsed by the publisher.
The Supplementary Material for this article can be found online at: https://www.frontiersin.org/articles/10.3389/fmars.2023.1157879/full#supplementary-material
Appendix Table 1 | Summary of cold seeps benthic foraminiferal assemblages.
Appendix Table 2 | Summary of stable isotopic records of cold seep related benthic foraminifera.
Abu-Zied R. H., Rohling E. J., Jorissen F. J., Fontanier C., Casford J. S., Cooke S. (2008). Benthic foraminiferal response to changes in bottom-water oxygenation and organic carbon flux in the eastern Mediterranean during LGM to recent times. Mar. Micropaleontol 67 (1-2), 46–68. doi: 10.1016/j.marmicro.2007.08.006
Aharon P., Fu B. (2000). Microbial sulfate reduction rates and sulfur and oxygen isotope fractionations at oil and gas seeps in deepwater gulf of Mexico. Geochimica Cosmochimica Acta 64 (2), 233–246. doi: 10.1016/s0016-7037(99)00292-6
Argentino C., Waghorn K. A., Vadakkepuliyambatta S., Polteau S., Bünz S., Panieri G. (2021). Dynamic and history of methane seepage in the SW barents Sea: new insights from leirdjupet fault complex. Sci. Rep. 11 (1), 1–13. doi: 10.1038/s41598-021-83542-0
Barbieri R., Panieri G. (2004). How are benthic foraminiferal faunas influenced by cold seeps? evidence from the Miocene of Italy. Palaeogeography Palaeoclimatol Palaeoecol. 204 (3-4), 257–275. doi: 10.1016/S0031-0182(03)00730-2
Barker S., Greaves M., Elderfield H. (2003). A study of cleaning procedures used for foraminiferal Mg/Ca paleothermometry. Geochemistry Geophysics Geosystems 4 (9), 1–20. doi: 10.1029/2003gc000559
Barras C., Duplessy J., Geslin E., Michel E., Jorissen F. J. (2010). Calibration of δ18O of cultured benthic foraminiferal calcite as a function of temperature. Biogeosciences 7 (4), 1349–1356. doi: 10.5194/bg-7-1349-2010
Bemis B. E., Spero H. J., Bijma J., Lea D. W. (1998). Reevaluation of the oxygen isotopic composition of planktonic foraminifera: experimental results and revised paleotemperature equations. Paleoceanography 13 (2), 150–160. doi: 10.1029/98PA00070
Bentov S., Erez J. (2006). Impact of biomineralization processes on the mg content of foraminiferal shells: a biological perspective. Geochemistry Geophysics Geosystems 7 (1), 1–11. doi: 10.1029/2005GC001015
Berner R. A. (1982). Burial of organic carbon and pyrite sulfur in the modern ocean: its geochemical and environmental significance. Am. J. Sci. 282 (4), 451–473. doi: 10.2475/ajs.282.4.451
Berner R. A. (1985). Sulphate reduction, organic matter decomposition and pyrite formation. Philos. Trans. R. Soc. London. Ser. A Math. Phys. Sci. 315 (1531), 25–38. doi: 10.1098/rsta.1985.0027
Bernhard J. M., Bowser S. S. (1999). Benthic foraminifera of dysoxic sediments: chloroplast sequestration and functional morphology. Earth-Science Rev. 46 (1-4), 149–165. doi: 10.1016/S0012-8252(99)00017-3
Bernhard J. M., Buck K. R., Barry J. P. (2001). Monterey Bay cold-seep biota: assemblages, abundance, and ultrastructure of living foraminifera. Deep Sea Res. Part I 48 (10), 2233–2249. doi: 10.1016/s0967-0637(01)00017-6
Bernhard J. M., Martin J. B., Rathburn A. E. (2010). Combined carbonate carbon isotopic and cellular ultrastructural studies of individual benthic foraminifera: 2. toward an understanding of apparent disequilibrium in hydrocarbon seeps. Paleoceanography 25 (4), 1–12. doi: 10.1029/2009PA001846
Bernhard J. M., Reimers C. E. (1991). Benthic foraminiferal population fluctuations related to anoxia: Santa Barbara basin. Biogeochemistry 15 (2), 127–149. doi: 10.1007/BF00003221
Bhaumik A. K., Gupta A. K. (2005). Deep-sea benthic foraminifera from gas hydrate-rich zone, Blake ridge, Northwest Atlantic (ODP hole 997A). Curr. Sci. 88 (12), 1969–1973. doi: 10.1016/j.rsma.2021.101949
Bhaumik A. K., Gupta A. K. (2007). Evidence of methane release from Blake ridge ODP hole 997A during the plio-pleistocene: benthic foraminifer fauna and total organic carbon. Curr. Sci. 92 (2), 192–199. Available at: https://www.jstor.org/stable/24096688.
Blackmon P. D., Todd R. (1959). Mineralogy of some foraminifera as related to their classification and ecology. J. Paleontol 33 (1), 1–15.
Boetius A., Ravenschlag K., Schubert C. J., Rickert D., Widdel F., Gieseke A., et al. (2000). A marine microbial consortium apparently mediating anaerobic oxidation of methane. Nature 407 (6804), 623–626. doi: 10.1038/35036572
Borowski W. S., Paull C. K., Ussler W. III (1996). Marine pore-water sulfate profiles indicate in situ methane flux from underlying gas hydrate. Geology 24 (7), 655–658. doi: 10.1130/0091-7613(1996)024<0655:mpwspi>2.3.co;2
Borowski W. S., Rodriguez N. M., Paull C. K., Ussler I. I. I. (2013). Are 34S-enriched authigenic sulfide minerals a proxy for elevated methane flux and gas hydrates in the geologic record? Mar. Petroleum Geology 43, 381–395. doi: 10.1016/j.marpetgeo.2012.12.009
Broecker W. S., Van Donk J. (1970). Insolation changes, ice volumes, and the O18 record in deep-sea cores. Rev. Geophysics 8 (1), 169–198. doi: 10.1029/rg008i001p00169
Brooks J. M., Field M. E., Kennicutt M. C. II (1991). Observations of gas hydrates in marine sediments, offshore northern California. Mar. Geology 96 (1-2), 103–109. doi: 10.1016/0025-3227(91)90204-H
Buckman J., Donnelly T., Jiang Z., Lewis H., Ruffell A. (2020). Methane derived authigenic carbonate (MDAC) aragonite cemented quaternary hardground from a methane cold seep, rathlin basin, northern Ireland: δ13C and δ18O isotopes, environment, porosity and permeability. Geosciences 10 (7), 255. doi: 10.3390/geosciences10070255
Burkett A. M., Rathburn A. E., Elena Pérez M., Levin L. A., Martin J. B. (2016). Colonization of over a thousand cibicidoides wuellerstorfi (foraminifera: schwager 1866) on artificial substrates in seep and adjacent off-seep locations in dysoxic, deep-sea environments. Deep Sea Res. Part I 117, 39–50. doi: 10.1016/j.dsr.2016.08.011
Caldwell S. L., Laidler J. R., Brewer E. A., Eberly J. O., Sandborgh S. C., Colwell F. S. (2008). Anaerobic oxidation of methane: mechanisms, bioenergetics, and the ecology of associated microorganisms. Environ. Sci. Technol. 42 (18), 6791–6799. doi: 10.1021/es800120b
Cannariato K. G., Stott L. D. (2004). Evidence against clathrate-derived methane release to Santa Barbara basin surface waters? Geochemistry Geophysics Geosystems 5 (5), 1–9. doi: 10.1029/2003GC000600
Ceramicola S., Dupré S., Somoza L., Woodside J. (2018). “Cold seep systems,” in Submarine geomorphology. Eds. Micallef A., Krastel S., Savini A. (Switzerland: Springer Geology. Springer, Cham), 367–387. doi: 10.1007/978-3-319-57852-1_19
Chang Z., Fang C., Sihai C., Hongfeng L., Yang Z., Guanghu L. (2015). Stable isotopic characteristics and their influencing factors of benthic foraminifera in the prospective gas hydrate area from the northern south China Sea since the last glacial. Quaternary Sci. 35 (2), 422–432. doi: 10.11928/j.issn.1001-7410.2015.02.17
Chen D. F., Feng D., Su Z., Song Z. G., Chen G. Q., Cathles L. M. (2006). Pyrite crystallization in seep carbonates at gas vent and hydrate site. Materials Sci. Engineering: C 26 (4), 602–605. doi: 10.1016/j.msec.2005.08.037
Chen D. F., Huang Y. Y., Yuan X. L., Cathles III, L.M. (2005). Seep carbonates and preserved methane oxidizing archaea and sulfate reducing bacteria fossils suggest recent gas venting on the seafloor in the northeastern south China Sea. Mar. petroleum Geology 22 (5), 613–621. doi: 10.1016/j.marpetgeo.2005.05.002
Chen F., Su X., Lu H., Chen C., Zhou Y., Cheng S., et al. (2007). Carbon stable isotopic composition of benthic foraminifers from the north of the south China Sea: indicator of methane-rich environment. Mar. Geology Quaternary Geology 27 (4), 1–7. doi: 10.1016/j.palaeo.2006.10.002
Chen F., Zhou Y., Su X., Lu H., Liu G., Zhuang C. (2010). Benthic foraminifera and stable isotopic composition of gas hydrate-bearing sediments from shenhu area in the northern south China Sea. Mar. Geology Quaternary Geology 30 (2), 1–8. doi: 10.3724/SP.J.1140.2010.02001
Cheng X., Huang B., Jian Z., Zhao Q., Tian J., Li J. (2005). Foraminiferal isotopic evidence for monsoonal activity in the south China Sea: a present-LGM comparison. Mar. Micropaleontol 54 (1-2), 125–139. doi: 10.1016/j.marmicro.2004.09.007
Consolaro C., Rasmussen T. L., Panieri G., Mienert J., Bünz S., Sztybor K. (2015). Carbon isotope (δ13C) excursions suggest times of major methane release during the last 14 kyr in fram strait, the deep-water gateway to the Arctic. Climate Past 11 (4), 669–685. doi: 10.5194/cp-11-669-2015
Corliss B. H. (1985). Microhabitats of benthic foraminifera within deep-sea sediments. Nature 314 (6010), 435–438. doi: 10.1038/314435a0
Damm E., Mackensen A., Budéus G., Faber E., Hanfland C. (2005). Pathways of methane in seawater: plume spreading in an Arctic shelf environment (SW-spitsbergen). Continental Shelf Res. 25 (12-13), 1453–1472. doi: 10.1016/j.csr.2005.03.003
Dantas R. C., Hassan M. B., Cruz F. W., Jovane L. (2022). Evidence for methane seepage in south Atlantic from the occurrence of authigenic gypsum and framboidal pyrite in deep-sea sediments. Mar. Petroleum Geology 142, 105727. doi: 10.1016/j.marpetgeo.2022.105727
Davidson D. W., Leaist D. G., Hesse R. (1983). Oxygen-18 enrichment in the water of a clathrate hydrate. Geochimica Cosmochimica Acta 47 (12), 2293–2295. doi: 10.1016/0016-7037(83)90053-4
Dessandier P., Borrelli C., Kalenitchenko D., Panieri G. (2019). Benthic foraminifera in Arctic methane hydrate bearing sediments. Front. Mar. Sci. 6. doi: 10.3389/fmars.2019.00765
Dessandier P., Borrelli C., Yao H., Sauer S., Hong W., Panieri G. (2020). Foraminiferal δ18O reveals gas hydrate dissociation in Arctic and north Atlantic ocean sediments. Geo-Marine Lett. 40 (4), 507–523. doi: 10.1007/s00367-019-00635-6
Dickens G. R., Castillo M. M., Walker J. C. (1997). A blast of gas in the latest Paleocene: simulating first-order effects of massive dissociation of oceanic methane hydrate. Geology 25 (3), 259–262. doi: 10.1130/0091-7613(1997)025<0259:abogit>2.3.co;2
Dickens G. R., O'Neil J. R., Rea D. K., Owen R. M. (1995). Dissociation of oceanic methane hydrate as a cause of the carbon isotope excursion at the end of the Paleocene. Paleoceanography 10 (6), 965–971. doi: 10.1029/95pa02087
Duplessy J. C., Lalou C., Vinot A. C. (1970). Differential isotopic fractionation in benthic foraminifera and paleotemperatures reassessed. Science 168 (3928), 250–251. doi: 10.1126/science.168.3928.250
Edgar K. M., Pälike H., Wilson P. A. (2013). Testing the impact of diagenesis on the δ18O and δ13C of benthic foraminiferal calcite from a sediment burial depth transect in the equatorial pacific. Paleoceanography 28 (3), 468–480. doi: 10.1002/palo.20045
Egger M., Riedinger N., Mogollón J. M., Jørgensen B. B. (2018). Global diffusive fluxes of methane in marine sediments. Nat. Geosci. 11 (6), 421–425. doi: 10.1038/s41561-018-0122-8
Erbacher J., Nelskamp S. (2006). Comparison of benthic foraminifera inside and outside a sulphur-oxidizing bacterial mat from the present oxygen-minimum zone off Pakistan (NE Arabian Sea). Deep Sea Res. Part I: Oceanographic Res. Papers 53 (5), 751–775. doi: 10.1016/j.dsr.2006.02.003
Erez J. (1978). Vital effect on stable-isotope composition seen in foraminifera and coral skeletons. Nature 273 (5659), 199–202. doi: 10.1038/273199a0
Feng D., Qiu J., Hu Y., Peckmann J., Guan H., Tong H., et al. (2018). Cold seep systems in the south China Sea: an overview. J. Asian Earth Sci. 168, 3–16. doi: 10.1016/j.jseaes.2018.09.021
Fontanier C., Koho K. A., Goñi-Urriza M. S., Deflandre B., Galaup S., Ivanovsky A., et al. (2014). Benthic foraminifera from the deep-water Niger delta (Gulf of guinea): assessing present-day and past activity of hydrate pockmarks. Deep Sea Res. Part I: Oceanographic Res. Papers 94, 87–106. doi: 10.1016/j.dsr.2014.08.011
Gooday A. J. (1994). The biology of deep-sea foraminifera: a review of some advances and their applications in paleoceanography. Palaios 9 (1), 14–31. doi: 10.2307/3515075
Griffith E. M., Paytan A. (2012). Barite in the ocean–occurrence, geochemistry and palaeoceanographic applications. Sedimentology 59 (6), 1817–1835. doi: 10.1111/j.1365-3091.2012.01327.x
Grossman E. L. (1987). Stable isotopes in modern benthic foraminifera; a study of vital effect. J. Foraminiferal Res. 17 (1), 48–61. doi: 10.2113/gsjfr.17.1.48
Gupta B. K. S., Smith L. E., Lobegeier M. K. (2007). Attachment of foraminifera to vestimentiferan tubeworms at cold seeps: refuge from seafloor hypoxia and sulfide toxicity. Mar. Micropaleontol 62 (1), 1–6. doi: 10.1016/j.marmicro.2006.06.007
Heinz P., Sommer S., Pfannkuche O., Hemleben C. (2005). Living benthic foraminifera in sediments influenced by gas hydrates at the cascadia convergent margin, NE pacific. Mar. ecology. Prog. Ser. (Halstenbek) 304, 77–89. doi: 10.3354/meps304077
Hemleben C., Spindler M., Anderson O. R. (1989). Modern planktonic foraminifera. Springer New York NY. 363, 77–89. doi: 10.1007/978-1-4612-3544-6
Herguera J. C., Paull C. K., Perez E., Ussler W. III, Peltzer E. (2014). Limits to the sensitivity of living benthic foraminifera to pore water carbon isotope anomalies in methane vent environments. Paleoceanography 29 (3), 273–289. doi: 10.1002/2013PA002457
Hesse R., Harrison W. E. (1981). Gas hydrates (clathrates) causing pore-water freshening and oxygen isotope fractionation in deep-water sedimentary sections of terrigenous continental margins. Earth planetary Sci. Lett. 55 (3), 453–462. doi: 10.1016/0012-821X(81)90172-2
Hill T. M., Kennett J. P., Spero H. J. (2003). Foraminifera as indicators of methane-rich environments: a study of modern methane seeps in Santa Barbara channel, California. Mar. Micropaleontol 49 (1-2), 123–138. doi: 10.1016/S0377-8398(03)00032-X
Hill T. M., Kennett J. P., Valentine D. L. (2004). Isotopic evidence for the incorporation of methane-derived carbon into foraminifera from modern methane seeps, hydrate ridge, northeast pacific. Geochimica Cosmochimica Acta 68 (22), 4619–4627. doi: 10.1016/j.gca.2004.07.012
Hill T. M., Paull C. K., Critser R. B. (2012). Glacial and deglacial seafloor methane emissions from pockmarks on the northern flank of the storegga slide complex. Geo-Marine Lett. 32 (1), 73–84. doi: 10.1007/s00367-011-0258-7
Hu Y., Feng D., Peckmann J., Roberts H. H., Chen D. (2014). New insights into cerium anomalies and mechanisms of trace metal enrichment in authigenic carbonate from hydrocarbon seeps. Chem. Geology 381 (14), 55–66. doi: 10.1016/j.chemgeo.2014.05.014
Jiasheng W., Wang Y., Li Q. (2007). Potential contributions of extremophiles to hydrocarbon resources in marine extreme environments: a review. Front. Earth Sci. China 1 (4), 444–451. doi: 10.1007/s11707-007-0055-9
Jones R. W. (1993). “Preliminary observations on benthonic foramininera associated with biogenic gas seep in the north Sea”. in Applied Micropalaeontology. Eds. Jenkins D. G. (Dordrecht: Springer), 69–91. doi: 10.1007/978-94-017-0763-3_3
Jorissen F. J. (1992). Ecology and palaeoecology of benthic foraminifera. Palaeogeography Palaeoclimatol Palaeoecol. 95 (3–4), 349–350. doi: 10.1016/0031-0182(92)90153-V
Joye S. B., Bowles M. W., Samarkin V. A., Hunter K. S., Niemann H. (2010). Biogeochemical signatures and microbial activity of different cold-seep habitats along the gulf of Mexico deep slope. Deep Sea Res. Part II: Topical Stud. Oceanography 57 (21-23), 1990–2001. doi: 10.1016/j.dsr2.2010.06.001
Judd A., Croker P., Tizzard L., Voisey C. (2007). Extensive methane-derived authigenic carbonates in the Irish Sea. Geo-Marine Lett. 27, 259–267. doi: 10.1007/s00367-007-0079-x
Judd A., Noble-James T., Golding N., Eggett A., Diesing M., Clare D., et al. (2020). The croker carbonate slabs: extensive methane-derived authigenic carbonate in the Irish Sea–nature, origin, longevity and environmental significance. Geo-Marine Lett. 40 (4), 423–438. doi: 10.1007/s00367-019-00584-0
Karstens J., Haflidason H., Becker L. W. M., Berndt C., Rüpke L., Planke S., et al. (2018). Glacigenic sedimentation pulses triggered post-glacial gas hydrate dissociation. Nat. Commun. 9 (1), 635. doi: 10.1038/s41467-018-03043-z
Kaufman A. J., Knoll A. H. (1995). Neoproterozoic variations in the c-isotopic composition of seawater: stratigraphic and biogeochemical implications. Precambrian Res. 73 (1-4), 27–49. doi: 10.1016/0301-9268(94)00070-8
Keigwin L. D. (1998). Glacial-age hydrography of the far northwest pacific ocean. Paleoceanography 13 (4), 323–339. doi: 10.1029/98PA00874
Keigwin L. D. (2002). Late pleistocene-Holocene paleoceanography and ventilation of the gulf of California. J. Oceanography 58 (2), 421–432. doi: 10.1023/A:1015830313175
Kennett J. P., Cannariato K. G., Hendy I. L., Behl R. J. (2000). Carbon isotopic evidence for methane hydrate instability during quaternary interstadials. Science 288 (5463), 128–133. doi: 10.1126/science.288.5463.128
Ketzer M., Praeg D., Rodrigues L. F., Augustin A., Pivel M. A. G., Rahmati-Abkenar M., et al. (2020). Gas hydrate dissociation linked to contemporary ocean warming in the southern hemisphere. Nat. Commun. 11 (1), 3788–3789. doi: 10.1038/s41467-020-17289-z
Khusid T. A., Domanov M. M., Svininnikov A. M. (2006). Species composition and distribution of foraminifers in the deryugin basin (Sea of Okhotsk). Biol. Bull. 33 (2), 172–178. doi: 10.1134/S1062359006020129
Klinkhammer G. P., Mix A. C., Haley B. A. (2009). Increased dissolved terrestrial input to the coastal ocean during the last deglaciation. Geochemistry Geophysics Geosystems 10 (3), 1–11. doi: 10.1029/2008GC002219
Knittel K., Boetius A. (2009). Anaerobic oxidation of methane: progress with an unknown process. Annu. Rev. Microbiol. 63, 311–334. doi: 10.1146/annurev.micro.61.080706.093130
Kocherla M. (2013). Authigenic gypsum in gas-hydrate associated sediments from the East coast of India (Bay of Bengal). Acta Geologica Sinica-English Edition 87 (3), 749–760. doi: 10.1111/1755-6724.12086
Kucera M. (2007). Chapter six planktonic foraminifera as tracers of past oceanic environments. Developments Mar. geology 1, 213–262. doi: 10.1016/S1572-5480(07)01011-1
Kvenvolden K. A. (1993). Gas hydrates–geological perspective and global change. Rev. geophysics 31 (2), 173–187. doi: 10.1029/93RG00268
Large R. R., Mukherjee I., Gregory D. D., Steadman J. A., Maslennikov V. V., Meffre S. (2017). Ocean and atmosphere geochemical proxies derived from trace elements in marine pyrite; implications for ore genesis in sedimentary basins. Economic geology Bull. Soc. Economic Geologists 112 (2), 423–450. doi: 10.2113/econgeo.112.2.423
Lei H., Cao C., Ou W., Gong C., Shi C. (2012). Carbon and oxygen isotope characteristics of foraminiferan from northern south China Sea sediments and their significance to late quaternary hydrate decomposition. J. Cent. South Univ. 19 (6), 1728–1740. doi: 10.1007/s11771-012-1200-5
Li N., Feng D., Wan S., Peckmann J., Guan H., Wang X., et al. (2021). Impact of methane seepage dynamics on the abundance of benthic foraminifera in gas hydrate bearing sediments: new insights from the south China Sea. Ore Geology Rev. 136, 104247. doi: 10.1016/j.oregeorev.2021.104247
Li Q., Wang J., Chen J., Wei Q. (2010). Stable carbon isotopes of benthic foraminifers from iodp expedition 311 as possible indicators of episodic methane seep events in a gas hydrate geosystem. Palaios 25 (10), 671–681. doi: 10.2307/40865499
Lin Q., Wang J., Algeo T. J., Su P., Hu G. (2016a). Formation mechanism of authigenic gypsum in marine methane hydrate settings: evidence from the northern south China Sea. Deep Sea Res. Part I: Oceanographic Res. Papers 115, 210–220. doi: 10.1016/j.dsr.2016.06.010
Lin Q., Wang J., Algeo T. J., Sun F., Lin R. (2016b). Enhanced framboidal pyrite formation related to anaerobic oxidation of methane in the sulfate-methane transition zone of the northern south China Sea. Mar. Geology 379 (1), 100–108. doi: 10.1016/j.margeo.2016.05.016
Liu X., Li A., Dong J., Zhuang G., Xu F., Wan S. (2018). Nonevaporative origin for gypsum in mud sediments from the East China Sea shelf. Mar. Chem. 205 (20), 90–97. doi: 10.1016/j.marchem.2018.08.009
Lobegeier M. K., Sen Gupta B. K. (2008). Foraminifera of hydrocarbon seeps, gulf of Mexico. J. Foraminiferal Res. 38 (2), 93–116. doi: 10.1175/JTECH2026.1
Mackensen A., Wollenburg J., Licari L. (2006). Low δ13C in tests of live epibenthic and endobenthic foraminifera at a site of active methane seepage. Paleoceanography 21 (2), 1–12. doi: 10.1029/2005PA001196
Martin J. B., Day S. A., Rathburn A. E., Perez M. E., Mahn C., Gieskes J. (2004). Relationships between the stable isotopic signatures of living and fossil foraminifera in Monterey bay, California. Geochemistry Geophysics Geosystems 5 (4), 1–25. doi: 10.1029/2003GC000629
Martin R. A., Nesbitt E. A., Campbell K. A. (2007). Carbon stable isotopic composition of benthic foraminifera from pliocene cold methane seeps, cascadia accretionary margin. Palaeogeography Palaeoclimatol Palaeoecol. 246 (2-4), 260–277. doi: 10.1016/j.palaeo.2006.10.002
Martin R. A., Nesbitt E. A., Campbell K. A. (2010). The effects of anaerobic methane oxidation on benthic foraminiferal assemblages and stable isotopes on the hikurangi margin of eastern new Zealand. Mar. Geology 272 (1-4), 270–284. doi: 10.1016/j.margeo.2009.03.024
Matsumoto R., Borowski W. S. (2000). Gas hydrate estimates from newly determined oxygen isotopic fractionation (αGH-IW) and δ18O anomalies of the interstitial waters: leg 164, Blake ridge. Proc. ODP Sci. Results 164, 59–66. doi: 10.2973/odp.proc.sr.164.206.2000
McConnaughey T. A., Burdett J., Whelan J. F., Paull C. K. (1997). Carbon isotopes in biological carbonates: respiration and photosynthesis. Geochimica Cosmochimica Acta 61 (3), 611–622. doi: 10.1016/S0016-7037(96)00361-4
McCorkle D. C., Keigwin L. D., Corliss B. H., Emerson S. R. (1990). The influence of microhabitats on the carbon isotopic composition of deep-sea benthic foraminifera. Paleoceanography 5 (2), 161–185. doi: 10.1029/PA005i002p00161
McGann M., Conrad J. E. (2018). Faunal and stable isotopic analyses of benthic foraminifera from the southeast seep on kimki ridge offshore southern California, USA. Deep Sea Res. Part II 150, 92–117. doi: 10.1016/j.dsr2.2018.01.011
McQuay E. L., Torres M. E., Collier R. W., Huh C., McManus J. (2008). Contribution of cold seep barite to the barium geochemical budget of a marginal basin. Deep Sea Res. Part I 55 (6), 801–811. doi: 10.1016/j.dsr.2008.03.001
Melaniuk K. (2021). Effectiveness of fluorescent viability assays in studies of arctic cold seep foraminifera. Front. Mar. Sci. 8. doi: 10.3389/fmars.2021.587748
Melaniuk K., Sztybor K., Treude T., Sommer S., Rasmussen T. L. (2022). Influence of methane seepage on isotopic signatures in living deep-sea benthic foraminifera, 79° n. Sci. Rep. 12 (1) 1169. doi: 10.1038/s41598-022-05175-1
Merinero R., Lunar R., Martinez-Frias J., Somoza L., Diaz-del-Rio V. (2008). Iron oxyhydroxide and sulphide mineralization in hydrocarbon seep-related carbonate submarine chimneys, gulf of cadiz (SW Iberian peninsula). Mar. Petroleum Geology 25 (8), 706–713. doi: 10.1016/j.marpetgeo.2008.03.005
Miao X., Feng X., Liu X., Li J., Wei J. (2021). Effects of methane seepage activity on the morphology and geochemistry of authigenic pyrite. Mar. Petroleum Geology 133, 105231. doi: 10.1016/j.marpetgeo.2021.105231
Millo C., Sarnthein M., Erlenkeuser H., Frederichs T. (2005). Methane-driven late pleistocene δ13C minima and overflow reversals in the southwestern Greenland Sea. Geology 33 (11), 873–876. doi: 10.1130/G21790.1
Naehr T. H., Birgel D., Bohrmann G., MacDonald I. R., Kasten S. (2009). Biogeochemical controls on authigenic carbonate formation at the chapopote “asphalt volcano”, bay of campeche. Chem. Geology 266 (3-4), 390–402. doi: 10.1016/j.chemgeo.2009.07.002
Naehr T. H., Eichhubl P., Orphan V. J., Hovland M., Paull C. K., Ussler W. III, et al. (2007). Authigenic carbonate formation at hydrocarbon seeps in continental margin sediments: a comparative study. Deep Sea Res. Part II 54 (11-13), 1268–1291. doi: 10.1016/j.dsr2.2007.04.010
Niewöhner C., Hensen C., Kasten S., Zabel M., Schulz H. D. (1998). Deep sulfate reduction completely mediated by anaerobic methane oxidation in sediments of the upwelling area off Namibia. Geochimica cosmochimica Acta 62 (3), 455–464. doi: 10.1016/S0016-7037(98)00055-6
Ohkushi K., Ahagon N., Uchida M., Shibata Y. (2005). Foraminiferal isotope anomalies from northwestern pacific marginal sediments. Geochemistry Geophysics Geosystems 6 (4), 1–15. doi: 10.1029/2004gc000787
Pan M., Wu D., Yang F., Sun T., Wu N., Liu L. (2018). Geochemical sedimentary evidence from core 973-2 for methane activity near the jiulong methane reef in the northern south China Sea. Interpretation 6 (1), 163–174. doi: 10.1190/int-2017-0001.1
Panieri G. (2003). Benthic foraminifera response to methane release in an Adriatic Sea pockmark. Rivista Italiana di Paleontologia e Stratigrafia 109 (3), 549–562. doi: 10.13130/2039-4942/5523
Panieri G. (2005). Benthic foraminifera associated with a hydrocarbon seep in the rockall trough (NE Atlantic). Geobios 38 (2), 247–255. doi: 10.1016/j.geobios.2003.10.004
Panieri G. (2006). Foraminiferal response to an active methane seep environment: a case study from the Adriatic Sea. Mar. Micropaleontol 61 (1-3), 116–130. doi: 10.1016/j.marmicro.2006.05.008
Panieri G., Camerlenghi A., Cacho I., Cervera C. S., Canals M., Lafuerza S., et al. (2012). Tracing seafloor methane emissions with benthic foraminifera: results from the ana submarine landslide (Eivissa channel, Western Mediterranean Sea). Mar. Geology 291-294 (1), 97–112. doi: 10.1016/j.margeo.2011.11.005
Panieri G., Camerlenghi A., Conti S., Pini G. A., Cacho I. (2009). Methane seepages recorded in benthic foraminifera from Miocene seep carbonates, northern Apennines (Italy). Palaeogeography Palaeoclimatol Palaeoecol. 284 (3-4), 271–282. doi: 10.1016/j.palaeo.2009.10.006
Panieri G., Graves C. A., James R. H. (2016). Paleo-methane emissions recorded in foraminifera near the landward limit of the gas hydrate stability zone offshore western Svalbard. Geochemistry Geophysics Geosystems 17 (2), 521–537. doi: 10.1002/2015GC006153
Panieri G., Gupta B. K. S. (2008). Benthic foraminifera of the Blake ridge hydrate mound, western north Atlantic ocean. Mar. Micropaleontol 66 (2), 91–102. doi: 10.1016/j.marmicro.2007.08.002
Panieri G., James R. H., Camerlenghi A., Westbrook G. K., Consolaro C., Cacho I., et al. (2014). Record of methane emissions from the West Svalbard continental margin during the last 23.500 yrs revealed by δ13C of benthic foraminifera. Global Planetary Change 122, 151–160. doi: 10.1016/j.gloplacha.2014.08.014
Panieri G., Lepland A., Whitehouse M. J., Wirth R., Raanes M. P., James R. H., et al. (2017). Diagenetic mg-calcite overgrowths on foraminiferal tests in the vicinity of methane seeps. Earth Planetary Sci. Lett. 458 (15), 203–212. doi: 10.1016/j.epsl.2016.10.024
Paull C. K., Ussler W., Lorenson T., Winters W., Dougherty J. (2005). Geochemical constraints on the distribution of gas hydrates in the gulf of Mexico. Geo-Marine Lett. 25 (5), 273–280. doi: 10.1007/s00367-005-0001-3
Pawlowski J., Holzmann M. (2008). Diversity and geographic distribution of benthic foraminifera: a molecular perspective. Biodiversity Conserv. 17 (2), 317–328. doi: 10.1007/s10531-007-9253-8
Peckmann J., Reimer A., Luth U., Luth C., Hansen B. T., Heinicke C., et al. (2001). Methane-derived carbonates and authigenic pyrite from the northwestern black Sea. Mar. geology 177 (1-2), 129–150. doi: 10.1016/S0025-3227(01)00128-1
Pierre C. (2017). Origin of the authigenic gypsum and pyrite from active methane seeps of the southwest African margin. Chem. Geology 449 (20), 158–164. doi: 10.1016/j.chemgeo.2016.11.005
Rathburn A. E., Levin L. A., Held Z., Lohmann K. C. (2000). Benthic foraminifera associated with cold methane seeps on the northern California margin: ecology and stable isotopic composition. Mar. Micropaleontol 38 (3-4), 247–266. doi: 10.1016/S0377-8398(00)00005-0
Rathburn A. E., Levin L. A., Tryon M., Gieskes J. M., Martin J. B., Pérez M. E., et al. (2009). Geological and biological heterogeneity of the Aleutian margin, (1965–4822 m). Prog. Oceanography 80 (1-2), 22–50. doi: 10.1016/j.pocean.2008.12.002
Rathburn A. E., Pérez M. E., Martin J. B., Day S. A., Mahn C., Gieskes J., et al. (2003). Relationships between the distribution and stable isotopic composition of living benthic foraminifera and cold methane seep biogeochemistry in Monterey bay, California. Geochemistry Geophysics Geosystems 4 (12), 1–28. doi: 10.1029/2003gc000595
Ravelo A. C., Hillaire-Marcel C. (2007). Chapter eighteen the use of oxygen and carbon isotopes of foraminifera in paleoceanography. Developments Mar. geology 1, 735–764. doi: 10.1016/S1572-5480(07)01023-8
Reeburgh W. S. (2007). Oceanic methane biogeochemistry. Chem. Rev. 107 (2), 486–513. doi: 10.1021/cr050362v
Ritger S., Carson B., Suess E. (1987). Methane-derived authigenic carbonates formed by subduction-induced pore-water expulsion along the Oregon/Washington margin. Geological Soc. America Bull. 98 (2), 147–156. doi: 10.1130/0016-7606(1987)98<147:MACFBS>2.0.CO;2
Robinson C. A., Bernhard J. M., Levin L. A., Mendoza G. F., Blanks J. K. (2004). Surficial hydrocarbon seep infauna from the Blake ridge (Atlantic ocean 2150 m) and the gulf of Mexico (690–2240 m). Mar. Ecol. 25 (4), 313–336. doi: 10.1111/j.1439-0485.2004.00034.x
Ruppel C. D., Kessler J. D. (2017). The interaction of climate change and methane hydrates. Rev. Geophysics 55 (1), 126–168. doi: 10.1002/2016RG000534
Sackett W. M. (1978). Carbon and hydrogen isotope effects during the thermocatalytic production of hydrocarbons in laboratory simulation experiments. Geochimica Cosmochimica Acta 42 (6), 571–580. doi: 10.1016/0016-7037(78)90002-9
Saeidi Ortakand M., Hasegawa S., Matsumoto R., Matsuda H. (2016). Correlating biostratigraphy and palaeoceanographic changes during late quaternary at cold seeps with gas hydrate-free areas of the Japan Sea using foraminifera paleoecology. Island Arc 25 (1), 55–71. doi: 10.1111/iar.12131
Schiebel R., Smart S. M., Jentzen A., Jonkers L., Morard R., Meilland J., et al. (2018). Advances in planktonic foraminifer research: new perspectives for paleoceanography. Rev. Micropaléontologie 61 (3-4), 113–138. doi: 10.1016/j.revmic.2018.10.001
Schippers A., Jorgensen B. B. (2002). Biogeochemistry of pyrite and iron sulfide oxidation in marine sediments. Geochimica et cosmochimica acta 66(1), 85–92. doi: 10.1016/S0016-7037(01)00745-1
Schneider A., Crémière A., Panieri G., Lepland A., Knies J. (2017). Diagenetic alteration of benthic foraminifera from a methane seep site on vestnesa ridge (NW Svalbard). Deep Sea Res. Part I 123, 22–34. doi: 10.1016/j.dsr.2017.03.001
Sen Gupta B. K., Aharon P. (1994). Benthic foraminifera of bathyal hydrocarbon vents of the gulf of Mexico: initial report on communities and stable isotopes. Geo-Marine Lett. 14 (2), 88–96. doi: 10.1007/BF01203719
Sen Gupta B. K., Platon E., Bernhard J. M., Aharon P. (1997). Foraminiferal colonization of hydrocarbon-seep bacterial mats and underlying sediment, gulf of Mexico slope. J. Foraminiferal Res. 27 (4), 292–300. doi: 10.2113/gsjfr.27.4.292
Sexton P. F., Wilson P. A. (2009). Preservation of benthic foraminifera and reliability of deep-sea temperature records: importance of sedimentation rates, lithology, and the need to examine test wall structure. Paleoceanography 24 (2), 1–14. doi: 10.1029/2008PA001650
Smith L. M., Sachs J. P., Jennings A. E., Anderson D. M., DeVernal A. (2001). Light δ13C events during deglaciation of the East Greenland continental shelf attributed to methane release from gas hydrates. Geophysical Res. Lett. 28 (11), 2217–2220. doi: 10.1029/2000GL012627
Smrzka D., Feng D., Himmler T., Zwicker J., Hu Y., Monien P., et al. (2020). Trace elements in methane-seep carbonates: potentials, limitations, and perspectives. Earth-Science Rev. 208, 103263. doi: 10.1016/j.earscirev.2020.103263
Spero H. J., Lea D. W. (1996). Experimental determination of stable isotope variability in globigerina bulloides: implications for paleoceanographic reconstructions. Mar. Micropaleontol 28 (3-4), 231–246. doi: 10.1016/0377-8398(96)00003-5
Steinle L., Graves C. A., Treude T., Ferré B., Biastoch A., Bussmann I., et al. (2015). Water column methanotrophy controlled by a rapid oceanographic switch. Nat. Geosci. 8 (5), 378–382. doi: 10.1038/NGEO2420
Stott L. D., Bunn T., Prokopenko M., Mahn C., Gieskes J., Bernhard J. M. (2002). Does the oxidation of methane leave an isotopic fingerprint in the geologic record? Geochemistry Geophysics Geosystems 3 (2), 1–16. doi: 10.1029/2001gc000196
Suess E. (2020). “Marine cold seeps: background and recent advances,” in Hydrocarbons, oils and lipids: diversity, origin, chemistry and fate. handbook of hydrocarbon and lipid microbiology. Eds. Wilkes H. (Cham: Springer), 747–767. doi: 10.1007/978-3-319-90569-3_27
Sultan N., Plaza-Faverola A., Vadakkepuliyambatta S., Buenz S., Knies J. (2020). Impact of tides and sea-level on deep-sea Arctic methane emissions. Nat. Commun. 11 (1), 5087. doi: 10.1038/s41467-020-18899-3
Tagliabue A., Bopp L. (2008). Towards understanding global variability in ocean carbon-13. Global biogeochemical cycles 22 (1), 1–13. doi: 10.1029/2007GB003037
Tesoriero A. J., Pankow J. F. (1996). Solid solution partitioning of Sr2+, Ba2+, and Cd2+ to calcite. Geochimica Cosmochimica Acta 60 (6), 1053–1063. doi: 10.1016/0016-7037(95)00449-1
Thatcher K. E., Westbrook G. K., Sarkar S., Minshull T. A. (2013). Methane release from warming-induced hydrate dissociation in the West Svalbard continental margin: timing, rates, and geological controls. J. Geophysical Res. 118 (1), 22–38. doi: 10.1029/2012JB009605
Thomas D. J., Zachos J. C., Bralower T. J., Thomas E., Bohaty S. (2002). Warming the fuel for the fire: evidence for the thermal dissociation of methane hydrate during the Paleocene-Eocene thermal maximum. Geology 30 (12), 1067–1070. doi: 10.1130/0091-7613(2002)030<1067:WTFFTF>2.0.CO;2
Torres M. E., Martin R. A., Klinkhammer G. P., Nesbitt E. A. (2010). Post depositional alteration of foraminiferal shells in cold seep settings: new insights from flow-through time-resolved analyses of biogenic and inorganic seep carbonates. Earth Planetary Sci. Lett. 299 (1-2), 10–22. doi: 10.1016/j.epsl.2010.07.048
Torres M. E., Mix A. C., Kinports K., Haley B., Klinkhammer G. P., McManus J., et al. (2003). Is methane venting at the seafloor recorded by δ13C of benthic foraminifera shells? Paleoceanography 18 (3), 1–7. doi: 10.1029/2002PA000824
Tribovillard N., Algeo T. J., Lyons T., Riboulleau A. (2006). Trace metals as paleoredox and paleoproductivity proxies: an update. Chem. Geology 232 (1-2), 12–32. doi: 10.1016/j.chemgeo.2006.02.012
Uchida M., Ohkushi K., Kimoto K., Inagaki F., Ishimura T., Tsunogai U., et al. (2008). Radiocarbon-based carbon source quantification of anomalous isotopic foraminifera in last glacial sediments in the western north pacific. Geochemistry Geophysics Geosystems 9 (4), 1–26. doi: 10.1029/2006GC001558
Ussler W. III, Paull C. K. (2008). Rates of anaerobic oxidation of methane and authigenic carbonate mineralization in methane-rich deep-sea sediments inferred from models and geochemical profiles. Earth Planetary Sci. Lett. 266 (3-4), 271–287. doi: 10.1016/j.epsl.2007.10.056
Wallmann K., Riedel M., Hong W. L., Patton H., Hubbard A., Pape T., et al. (2018). Gas hydrate dissociation off Svalbard induced by isostatic rebound rather than global warming. Nat. Commun. 9 (1), 83. doi: 10.1038/s41467-017-02550-9
Wan S., Feng D., Chen F., Zhuang C., Chen D. (2018). Foraminifera from gas hydrate-bearing sediments of the northeastern south China Sea: proxy evaluation and application for methane release activity. J. Asian Earth Sci. 168, 125–136. doi: 10.1016/j.jseaes.2018.04.036
Wang S., Yan B., Yan W. (2013). Tracing seafloor methane emissions with benthic foraminifera in the baiyun sag of the northern south China Sea. Environ. Earth Sci. 70 (3), 1143–1150. doi: 10.1007/s12665-012-2201-2
Wefer G., Heinze P., Berger W. H. (1994). Clues to ancient methane release. Nature 369, 282–282. doi: 10.1038/369282a0
Whiticar M. J. (1999). Carbon and hydrogen isotope systematics of bacterial formation and oxidation of methane. Chem. Geology 161 (1-3), 291–314. doi: 10.1016/S0009-2541(99)00092-3
Wiedicke M., Weiss W. (2006). Stable carbon isotope records of carbonates tracing fossil seep activity off Indonesia. Geochemistry Geophysics Geosystems 7 (11), 1–22. doi: 10.1029/2006GC001292
Wilfert P., Krause S., Liebetrau V., Schönfeld J., Haeckel M., Linke P., et al. (2015). Response of anaerobic methanotrophs and benthic foraminifera to 20 years of methane emission from a gas blowout in the north Sea. Mar. Petroleum Geology 68, 731–742. doi: 10.1016/j.marpetgeo.2015.07.012
Wollenburg J. E., Raitzsch M., Tiedemann R. (2015). Novel high-pressure culture experiments on deep-sea benthic foraminifera–evidence for methane seepage-related δ13C of cibicides wuellerstorfi. Mar. Micropaleontol 117, 47–64. doi: 10.1016/j.marmicro.2015.04.003
Xiang R., Fang L., Chen Z., Zhang L., Yan W., Chen M. (2012). Carbon isotope of benthic foraminifera and its implications for cold seepage in the southwestern area off Dongsha islands, South China Sea. Marine Geology & Quaternary Geology 32(4), 17–24. doi: 10.3724/SP.J.1140.2012.04017
Xin S., Ying Q., Fang C., Shengxiong Y., Yang Z., Hongpeng C., et al. (2020). Deep sea benthic foraminifera from the taixinan basin and changes of their cold seep microhabitats during the past 50000 years. Earth Sci. Front. 27 (6), 255. doi: 10.13745/j.esf.sf.2020.6.13
Zahn R., Winn K., Sarnthein M. (1986). Benthic foraminiferal δ13C and accumulation rates of organic carbon: uvigerina peregrina group and cibicidoides wuellerstorfi. Paleoceanography 1 (1), 27–42. doi: 10.1029/PA001i001p00027
Zhang M. Z. M., Lu H. L. H., Guan H. G. H., Liu L. L. L., Wu D. W. D., Wu N. W. N. (2018). Methane seepage intensities traced by sulfur isotopes of pyrite and gypsum in sediment from the shenhu area, south China Sea. Acta Oceanologica Sin. 37 (7), 20–27. doi: 10.1007/s13131-018-1241-1
Zhang B., Pan M., Wu D., Wu N. (2018). Distribution and isotopic composition of foraminifera at cold-seep site 973-4 in the dongsha area, northeastern south China Sea. J. Asian Earth Sci. 168, 145–154. doi: 10.1016/j.jseaes.2018.05.007
Zhou Y., Chen F., Su X., Liu G., Chen C. (2009). Benthic foraminifera communities in methane-rich environment showed by core hd319 in dongsha sea area of the south China Sea. Mar. Geology Quaternary Geology 29 (3), 1–8. doi: 10.3724/SP.J.1140.2009.03001
Zhou Y. A., Di P. B. C. D., Li N. B. C. D., Chen F. A., Su X. F., Zhang J. A. (2020). Unique authigenic mineral assemblages and planktonic foraminifera reveal dynamic cold seepage in the southern south China sea. Minerals 10 (3), 275. doi: 10.3390/min10030275
Keywords: cold seep, marine foraminifera, methane seepage, species composition, secondary mineralization, stable isotopes
Citation: Lu Y, Yang H, Huang B, Liu Y and Lu H (2023) Foraminifera associated with cold seeps in marine sediments. Front. Mar. Sci. 10:1157879. doi: 10.3389/fmars.2023.1157879
Received: 03 February 2023; Accepted: 06 April 2023;
Published: 24 April 2023.
Edited by:
Dong Feng, Shanghai Ocean University, ChinaReviewed by:
Jiasheng Wang, China University of Geosciences Wuhan, ChinaCopyright © 2023 Lu, Yang, Huang, Liu and Lu. This is an open-access article distributed under the terms of the Creative Commons Attribution License (CC BY). The use, distribution or reproduction in other forums is permitted, provided the original author(s) and the copyright owner(s) are credited and that the original publication in this journal is cited, in accordance with accepted academic practice. No use, distribution or reproduction is permitted which does not comply with these terms.
*Correspondence: Hailong Lu, aGx1QHBrdS5lZHUuY24=
Disclaimer: All claims expressed in this article are solely those of the authors and do not necessarily represent those of their affiliated organizations, or those of the publisher, the editors and the reviewers. Any product that may be evaluated in this article or claim that may be made by its manufacturer is not guaranteed or endorsed by the publisher.
Research integrity at Frontiers
Learn more about the work of our research integrity team to safeguard the quality of each article we publish.