- 1Marine Animal Ecology, Wageningen University & Research, Wageningen, Netherlands
- 2Zoology Department, Kenyatta University, Nairobi, Kenya
Monitoring of reef restoration efforts and artificial reefs (ARs) has typically been limited to coral fragment survival, hampering evaluation of broader objectives such as ecosystem recovery. This study aimed to determine to what extent AR design influences the ecological recovery of restored reefs by monitoring outplanted coral fragments, benthic cover, coral recruitment and fish and invertebrate communities for two years. Four AR designs (16 m2), unrestored controls and natural reef patches as reference (n = 10) were established in Mkwiro, Kenya. ARs consisted either of concrete disks with bottles, layered concrete disks, metal cages or a combination thereof. A mixture of 18 branching coral species (mainly Acropora spp.) was outplanted on ARs at a density of 7 corals m-2. After two years, 60% of all outplanted fragments had survived, already resulting in coral cover on most ARs comparable (though Acropora-dominated) to reference patches. Coral survival differed between ARs, with highest survival on cages due to the absence of crown-of-thorns sea star predation on this design. In total, 32 coral genera recruited on ARs and recruit densities were highest on reference patches, moderate on concrete ARs and low on cages. ARs and reference patches featured nearly twice the fish species richness and around an order of magnitude higher fish abundance and biomass compared to control patches. Fish abundance and biomass strongly correlated with coral cover on ARs. AR, reference and control patches all had distinct fish species compositions, but AR and reference patches were similar in terms of trophic structure of their fish communities. Motile invertebrates including gastropods, sea urchins, sea cucumbers and sea stars were present at ARs, but generally more abundant and diverse at natural reference patches. Taken together, all studied ecological parameters progressed towards reef ecosystem recovery, with varying influences of AR design and material. We recommend a combination of metal cages and layered concrete ARs to promote high fragment survival as well as natural coral recruitment. Ultimately, a longer period of monitoring is needed to fully determine the effectiveness reef restoration as conservation tool to support coral reef ecosystem recovery.
Introduction
Coral reefs have been deteriorating worldwide due to local human impacts such as overfishing and pollution (Burke et al., 2011) and declines are rapidly worsening with climate change (Heron et al., 2017). In the Western Indian Ocean, coral reefs and coastal communities are especially vulnerable due to the high dependence and utilization of reefs by people for their livelihoods, including artisanal fishing and tourism (Obura et al., 2022). Reef managers – unable to influence climate change – aim to strengthen reef resilience locally to reduce impacts of large-scale disturbances beyond their control (Nyström et al., 2008; Anthony et al., 2011; Graham et al., 2013; Anthony et al., 2015). In addition to the crucial reduction of local threats (Anthony et al., 2017; Mcleod et al., 2019), active interventions such as restoration of damaged and deteriorated reefs are now deemed necessary to reverse the ongoing loss of biodiversity and reef resilience (Suding et al., 2015; Rinkevich, 2019; Duarte et al., 2020). Alongside with climate action on an international level, such active local reef management might give reefs a better chance to resist or recover from disturbances whilst providing crucial ecosystem services and buying time for coral adaptation to increasing temperatures (Hein et al., 2020b; Knowlton et al., 2021).
A commonly used and recommended method for reef restoration is the two-phase coral gardening approach in which coral fragments are first cultured in nurseries and then outplanted onto degraded reefs or artificial reef (AR) structures (Rinkevich, 1995). The nursery phase of coral gardening has been well established: high coral growth rates (Lirman et al., 2010) in combination with low costs (Levy et al., 2010) have made this an effective way to generate considerable coral stock, especially when integrated with natural processes such as herbivory to maintain coral health (Frias-Torres and Van de Geer, 2015; Knoester et al., 2019). The outplanting phase remains more costly and is not always successful in effectively increasing coral cover (Omori, 2019), partially due to ineffective AR design (Hylkema et al., 2021; Higgins et al., 2022) and a lack of understanding of ecological processes that determine coral survival such as coral predation and competition among benthic species (Ladd and Shantz, 2020). Although it is the ambition to substantially upscale restoration efforts (Vaughan, 2021), scientifically documented projects are currently still both small in size and high in costs (Bayraktarov et al., 2016; Bayraktarov et al., 2019). Furthermore, projects often (cl)aim to restore ecosystem functionality and ecosystem services, but monitoring generally lacks clear aims and mostly tracks item-based successes such as outplanted coral fragment survival (Bostrom-Einarsson et al., 2020; Hein et al., 2020b). Improved monitoring is needed to evaluate both AR design as well as the broader ecosystem impact of reef restoration as this could create the scientific credibility needed to further upscale restoration efforts (Abelson et al., 2020).
Traditionally, ARs have been widely used to exploit rather than restore marine ecosystems (Higgins et al., 2022). AR monitoring studies have therefore mainly focused on optimizing fisheries yields for a select group of commercial species by adjusting the design, site selection and management of ARs (Bohnsack and Sutherland, 1985; Baine, 2001). Studies that include the development of the whole reef community around ARs in comparison to natural reference reefs are needed to evaluate ecosystem restoration success, however such studies remain scarce (Carr and Hixon, 1997; Ceccarelli et al., 2020; Hylkema et al., 2021). Nonetheless, structural complexity has been identified as a driving factor supporting fish and invertebrate communities on both natural reefs (Graham and Nash, 2013) and ARs of different design (Baine, 2001; Hunter and Sayer, 2009). In return, accommodated reef communities can facilitate reef restoration by providing ecological functions such as herbivory or top-down control of coral predators that benefit coral survival and growth (Ladd and Shantz, 2020). Thus, monitoring how the development of ecological communities is influenced by AR design and complexity has the potential to improve the cost-effectiveness of reef restoration by evaluating to what extent critical ecological processes are re-established that drive ecosystem recovery (Horoszowski-fridman and Rinkevich, 2016). Since most reef restoration projects intend to initiate reef recovery (not to rebuild the entire reef) by using pioneer coral species such as branching Acropora spp., key indicators of a functional ecosystem need to be monitored including benthic cover, coral diversity, coral recruitment and the fish and invertebrate community (Bostrom-Einarsson et al., 2020; Ferse et al., 2021). Ultimately, monitoring at the relevant ecological scales (as opposed to item-based monitoring) allows for the appropriate evaluation of the central restoration goal of re-establishing self-sustaining reefs (Hein et al., 2020b; Ferse et al., 2021).
To restore a self-sustaining coral reef ecosystem that ultimately provides ecosystems services, ecological recovery on three levels is required (NASEM, 2018): individual coral colonies (survival and growth), coral population (reproduction and recruitment) and reef community (functional diversity). This study evaluated whether the outplanting of pioneer coral species onto ARs could initiate recovery on these three levels and to what extent recovery is influenced by AR design (Figure 1). Detailed benthic and fish surveys were performed on four different types of AR patches of increasing structural complexity, unrestored control patches and natural reference reefs over a period of two years to answer three research questions (RQs). RQ1: How does AR design influence the extent to which outplanted corals survive and grow sufficiently to establish themselves and outperform benthic competitors? RQ2: How does AR design affect coral recruitment? RQ3: How do the community composition and ecological functionalities of fish and invertebrates that develop on different AR designs compare to those communities on surrounding natural reefs? We expected to observe: 1) Differences between growth and survival of corals among ARs due to the impact of AR design on associating reef communities such as benthic competitors, coral predators and herbivores; 2) Intermediate coral recruitment on ARs compared to control and references reef patches due to the addition of moderately complex substrate, with further specific differences between ARs due to design, materials and associated communities; 3) Early stage ARs supporting the establishment of a moderately diverse fish and invertebrate community, with diversity of species and dietary guilds increasing with increasing complexity of AR designs (see Table S1 in the Supplementary Materials for more details).
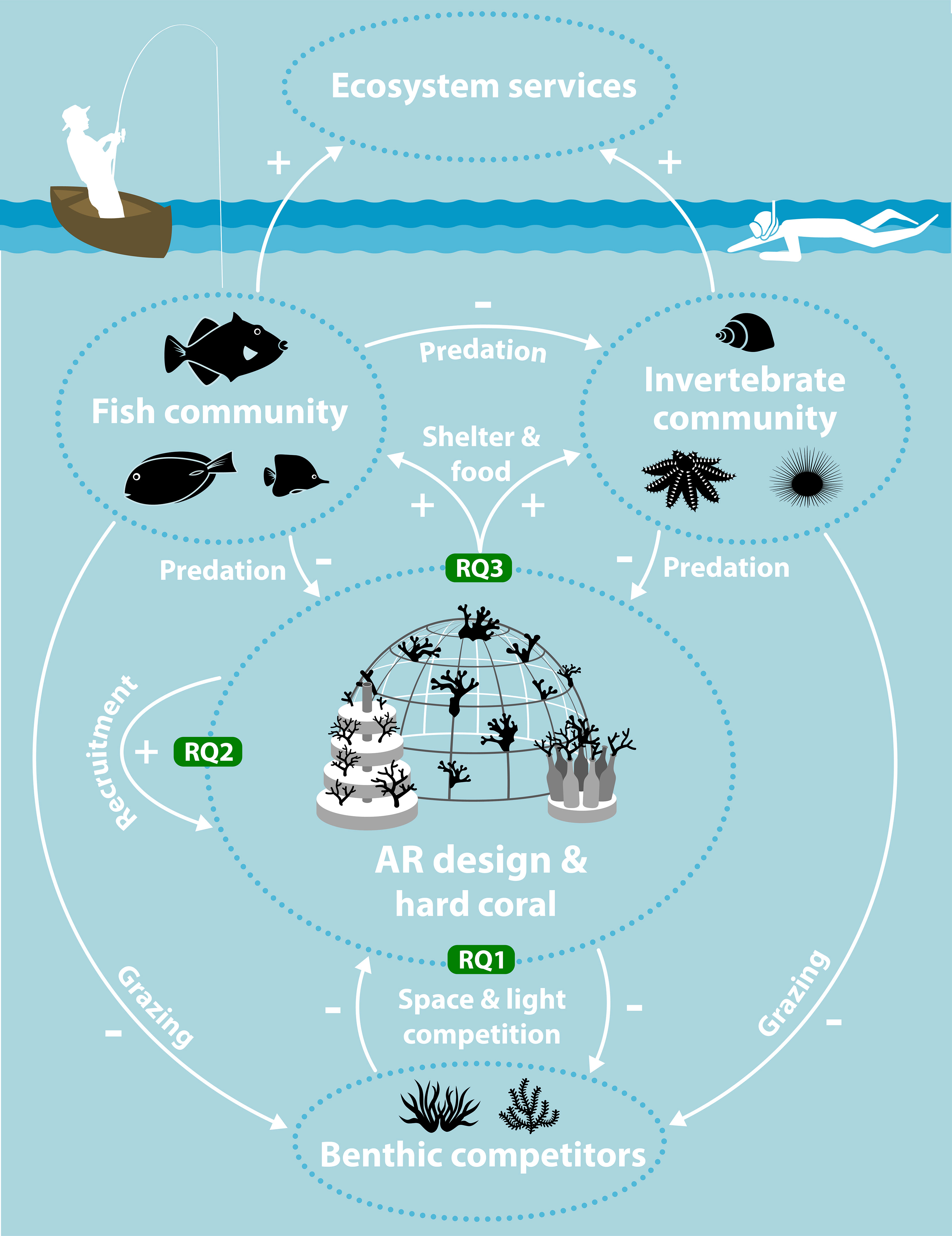
Figure 1 Schematic overview of the study, research questions (RQs) and wider socio-ecological setting. Growth and survival of hard corals outplanted onto artificial reefs (ARs) was evaluated and compared to benthic competitors (RQ1). The effect of AR design on coral recruitment was monitored (RQ2). The development of fish and invertebrate communities and their functions on different AR designs was compared (RQ3). A functional fish community can graze on benthic competitors, predate on invertebrates and predate on corals. A functional motile invertebrate community can also graze on benthic competitors and impact coral through predation. Both the fish and invertebrate community can also support ecosystem services such as artisanal fishing and tourism. Artwork by Vrijlansier.
Methods
Area description
The study area (-4.659, 39.381) covered a 1.6-km stretch of coastline between the villages of Mkwiro and Wasini on the north coast of Wasini Island, Kenya (Figure 2A). A kilometre-wide sea strait separated the study area from a headland of the Kenyan mainland featuring the central village Shimoni. Extensive mangrove forests surrounding two river mouths envelope both sides of the headland. The sea strait is subjected to semi-diurnal tides that cause differences in seawater surface levels of up to 4 m. Due to this specific combination of environmental settings, the study area experienced moderately strong currents, relatively low visibility (8 m yearly average) and moderate wave exposure (max significant wave height of ~1.5 m during the NE monsoon). Long-term average sea surface temperature range from 25˚C in August to 29˚C in April (NOAA, 2022). During the study period, however, water temperatures peaked above 30˚C in April 2019 and again in April 2020, culminating in a temperature stress of respectively 6 and 10 degree heating weeks in those two years (Liu et al., 2006).
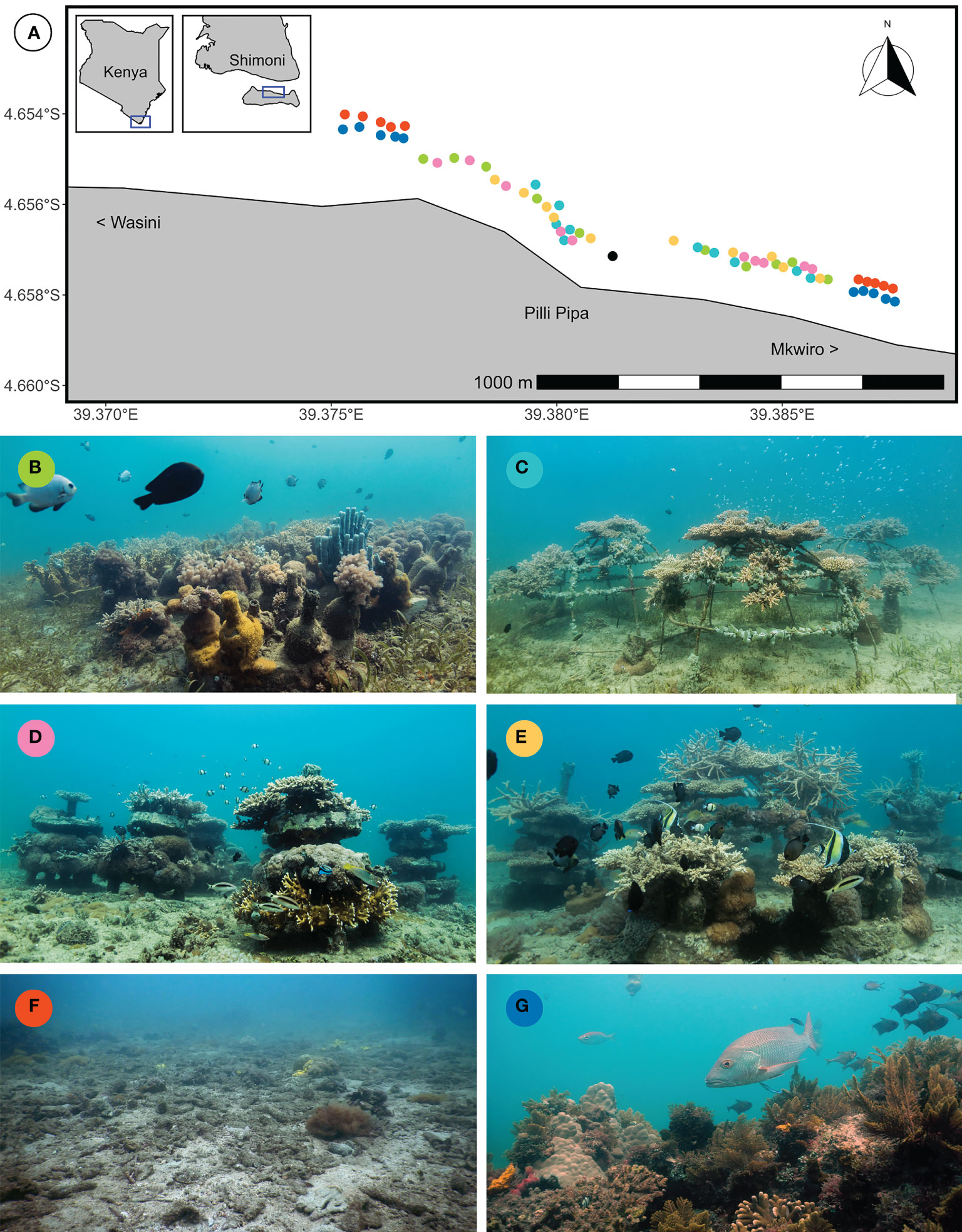
Figure 2 (A) Map of the study area (insets showing position within Kenya) and representative pictures of the six treatment patches two years after starting the experiment: (B) Bottle patch consisting of 16 bottle modules, (C) Cage patch consisting of 4 metal cages, (D) Cake patch consisting of 8 layered cakes, (E) Compound patch consisting of 4 bottle modules, 1 metal cage and 2 layered cakes, (F) Control patch that was not restored and (G) Reference reefs that retained natural structural complexity. Each coloured point on the map represents a patch (n = 10 per treatment) and matches with the treatment colour. The black point indicates the coral nursery. The land marker of Pilli Pipa Restaurant (also known as Panga Tatu) is indicated, as are the directions of the nearby villages of Mkwiro and Wasini.
Natural coral reefs in the study area were limited to a narrow strip (2 to 5 m depth at low tide) of discontinuous patches interspersed with extensive fields of unconsolidated rubble, which were occasionally overgrown by sheets of soft coral. Seagrass and macroalgae typically formed dense canopies in shallower water, whereas a diverse assemblage of soft corals and sponges covered the sandy slope into deeper water. The patch reefs had moderately high and diverse coral cover, with remaining hard substrate largely covered by macroalgae and sessile invertebrates such as sponges, hydroids, soft corals and tunicates. Encrusting and (sub)massive hard corals prevailed, but extensive heaps of rubble indicated that branching corals and especially Acropora spp. once were more common. Throughout the region, Acropora populations had been dominant (McClanahan et al., 1999) until diminished by the severe 1998 temperature anomaly (McClanahan et al., 2001). Acropora and other temperature-sensitive genera have not recovered since (McClanahan, 2014). Recovery of these predominantly branching and delicate corals is further hampered by ongoing destructive fishing practices such as the use of beach seines (Samoilys et al., 2017).
Study context: Reef restoration in Mkwiro
The study area falls within the waters of Mkwiro village, which is heavily dependent on its marine resources for fishing and, increasingly, tourism (Arthurton and Korateng, 2006). To promote community participation in the sustainable management of marine resources, Mkwiro Beach Management Unit (BMU) was established in 2007 as a local fishery stakeholder association under the State Department of Fisheries (Kawaka et al., 2017). In 2018, Mkwiro BMU started a collaboration with the REEFolution Foundation (the Netherlands) to improve the status of their reefs. The REEFolution Foundation, currently represented by an independent Kenyan branch named REEFolution Trust, aims to train and educate coastal communities to restore and protect their coral reefs and thereby safeguard local livelihoods. The REEFolution Trust collaborates with Wageningen University and Research (WUR) to develop scientifically validated and effective restoration methods. A co-management plan was drafted in which restoration and protection of a protected community managed area (CMA) for Mkwiro were proposed. Implementation of the co-management plan started in 2019 with the training of community members and students, coral gardening activities and demarcation of the Mkwiro CMA, though enforcement of the no-take zone has been largely lacking. As part of this collaboration between Mkwiro BMU, REEFolution Foundation, REEFolution Trust and WUR, the current experiment was set up under research license NACOSTI/P/21/8896.
Experimental setup
A total of 40 AR patches were deployed and filled with coral fragments between April and November 2019. Each AR patch covered approximately 16 m2, resulting in a total restored reef areal dimension (sensu Goergen et al., 2020) of 640 m2. Four different types of AR patches were created (n = 10): Bottle patches, Cage patches, Cake patches and Compound patches combining all AR types (Figures 2B–E). In addition, 10 rubble fields were left unrestored as Control patches (Figure 2F) and 10 natural reef patches that retained moderate structural complexity were chosen as Reference patches (Figure 2G). The AR patches were separated by at least 50 m from the Control and Reference patches (Figure 2A). The distance (measured from edge to edge) between adjacent AR patches was 12 ± 5 m (mean ± SD) and the distance between AR patches and any nearest natural reef structure was 14 ± 5 m. The distance between Control patches and natural reef was 13 ± 6 m. The depth of control patches (8 ± 2 m) and AR patches (8 ± 1 m) was greater than for Reference patches (5 ± 1 m). The Control and AR patches were positioned slightly deeper for two reasons: 1) natural reef patches were smaller (< 16 m2) and spaced more widely in deeper waters, leaving extensive fields of rubble where deployed AR patches could be considered independent of each other and the natural reef and 2) it was expected that deeper restored patches would be less impacted by temperature anomalies, benefitting the long-term objectives of the ongoing restoration project. Details on the exact placement of each patch can be found in the Supplementary Materials, Table S2.
The following modules were used to build each AR patch: Bottle patch (16 bottle modules), Cage patch (4 cages), Cake patch (8 layered cakes) and Compound patch (4 bottle modules, 1 cage and 2 layered cakes). A bottle module consisted of a concrete disk with about eight glass bottles (Figure 2B). A cage was made of metal and consisted of three vertical crossbows and four horizontal rings (Figure 2C). A layered cake consisted of four concrete disks, each separated from the next by PVC pipes and held together by a central PVC pipe (Figure 2D). Details on exact dimensions of modules can be found in Table S3. Coral fragments (10 – 15 cm length) were attached using tie-wraps (4.8 x 300 mm) to bottle necks, metal intersections on cages and PPR (plastic) pins that were embedded in both the top and third layer of cakes. Coral fragments were sourced from coral nursery trees (Nedimyer et al., 2011) in the study area (Figure 2A), which had been filled with naturally broken coral fragments (corals of opportunity) one year earlier. Coral species have not been confirmed, but a presumed 19 branching species of five genera have been outplanted: 12 Acropora spp., 2 Millepora spp., 2 Pocillopora spp., 1 Porites sp. and 2 Stylophora spp. Since fragments were collected as corals of opportunity, a high genotypic diversity is expected, but this remains unconfirmed. An outplanting density of 7 corals m-2 was realized at the start of monitoring, totalling to 4256 outplanted fragments (3580 Acropora spp., 201 Millepora spp., 107 Pocillopora spp., 145 Porites sp. and 223 Stylophora spp.). Species were haphazardly outplanted on AR patches. After outplanting, no maintenance (e.g. predator or fouling removal) was performed.
Monitoring
To monitor the survival of outplanted fragments, all AR modules were photographed at the start (Dec 2019) and near the end of the study (Dec 2021). Throughout the study, AR patches were visited at least quarterly to identify recent or ongoing causes of coral mortality. For example, the presence of crown of thorns sea stars (CoTS; Acanthaster sp.), Drupella sp. snails, sea turtles and fishing line would inform the likely cause of mortality or detachment, and these would often also leave characteristic marks behind (Figure S1). Benthic surveys were performed just after the start (Feb – Mar 2020) and at the end of the study (Feb – Mar 2022) to monitor benthic cover and motile invertebrates. To avoid sampling excessive amounts of rubble around the small AR patches, two perpendicular 5-m point intercept lines were used (crossing in the middle). This approach was used to monitor all AR, Control and Reference patches. The lines were sampled every 0.25 m and benthic cover divided into the following categories: hard coral to genus level (including the reef-building hydrozoan Millepora), soft coral, macroalgae (fleshy algae > 1 cm), hard substrate (including bare substrate, crustose coralline algae and turf algae < 1 cm), soft substrate (rubble, sand and seagrass) and other (mainly sponges, tunicates and hydroids). Motile invertebrates were sampled in a circle around the intersecting survey lines, with the radius depending on the size of the invertebrates: corallivorous snails (Drupella spp. and Coralliophila spp.) were counted within a 2.5-m radius (i.e. sampling area of 20 m2), sea urchins were identified and counted within a 3.6-m radius (40 m2) and larger invertebrates such as sea cucumbers, sea stars, large (> 5 cm) gastropods, octopus and lobsters were identified and counted within a 5.6-m radius (100 m2). Each patch was surveyed once in 2022, but due to COVID-19 fewer patches were sampled in 2020. At the end of the study (Mar 2022), coral recruits (1 – 10 cm diameter) were counted and identified to genus level where possible. At AR patches, coral recruits on each AR module were counted, the material type noted (glass, concrete, iron, PPR or PVC) and for recruits on concrete the orientation (horizontal or vertical) was noted as well. At each Control and Reference patch, coral recruits were sampled within 16 replicate 1 m2 quadrats.
Fish surveys were performed just after the start (Feb – Mar 2020) and at the end of the study (Dec 2021 – Mar 2022). A stationary fish census with standard 5-min initial sampling period was used to quantify the composition and abundance of all diurnal (surveys were performed between 0800 h and 1400 h), non-cryptic fish (Bohnsack and Bannerot, 1986). The radius of the fish census was typically 7.5 m, but lower when visibility was below average (to a minimum radius of 6 m). Surveys were performed about two meters distance from AR patches, and the large radius was chosen so that wary fish could be observed as well. Fish sizes (fork length) were estimated in classes of 5 cm for fishes smaller than 20 cm, in 10-cm size classes up to 50 cm and in 50-cm bins for larger fishes (i.e. trumpetfishes, cornetfishes and morays), so that fish biomasses (kg ha-1) could be estimated using known length-weight relations and the midpoint of each size class (Froese and Pauly, 2015). At each of the 60 patches, two to three fish surveys were performed in 2022, but not all patches were sampled in 2020 due to COVID-19 disruptions. Control and Reference patches had also been also surveyed in Apr – Jun 2019, before large-scale deployment of AR patches started. Surveys were performed by various observers, but always trained and tested by EGK on species identification and size estimation. A number of surveys were conducted by two observers (EGK and JJR) simultaneously on the same patch and comparisons did not show significant differences in observed fish richness, abundance or biomass.
Analyses
All analyses were performed in R (R Core Team, 2020). The comparison of fragment survival between AR patches included only the genus Acropora (representing 84% of outplanted fragments) due to the unequal distribution of the other genera among patches. Fragment survival was averaged per patch and compared between the four AR patch types using a generalized linear model with beta distribution from the betareg package (Cribari-Neto and Zeileis, 2010), thereby accounting for the proportional nature of the survival data. Model assumptions were validated by visual inspection of residual plots. A Wald Chi-Squared Test from the car package (Fox and Weisberg, 2018) was used to determine significance, and pairwise comparisons with Tukey adjustments were made using the emmeans package (Lenth, 2020). Putative causes of fragments mortality were summarized descriptively per AR patch type and per coral genus.
Coral recruits were summed per patch and divided by the projected area (i.e. 16 m2) to determine recruit density. Recruit density was log-transformed and compared between all six treatment patches with a linear model using the nlme package (DebRoy, 2006). Checking model assumptions and performing significance tests were implemented as outlined above. Genus-specific recruit densities were summarized descriptively. For AR patches specifically, recruit densities were explored further by material type and orientation. To get material-specific recruit densities, recruits were summed per material type and divided by the respective materials’ surface area per patch. For orientation-specific recruit densities the same procedure was repeated, but only concrete was sampled due its clear horizontal – vertical distinction. In addition, these results were further split by coral genus. As surface area and replicate numbers varied substantially for material type and orientation, these results are presented descriptively only.
Hard coral cover was compared between the six treatment patches using a generalized linear model with beta distribution as described above. Hard coral genera were summarized descriptively, as were data on other benthic categories. Given the similar patterns across years but limited number of replicates for 2020 and associated model convergence issues, the focus has been put on the more recent and complete 2022 data. Densities of motile invertebrates were all square-root transformed and compared between treatment patches using linear models of the nlme package as described above. The richness within each group was presented descriptively on either genus or family level. Due to their limited numbers found across all surveys, octopuses (9) and lobsters (5) were not included in the analysis. To explore the association between coral cover and recruit densities, a Pearson correlation was performed using AR module averages.
Fish communities were compared between treatment patches on three parameters: species richness, abundance and biomass. As for benthic communities, the focus has been put on the final 2022 data. Surveys performed simultaneously by two observers at the same patch were averaged. Silversides (Atherinomorus spp.) were excluded due to their highly variable abundance. Depth and distance to nearest AR or natural reef patch were evaluated as covariates in model selection based on Akaike’s Information Criterion (AIC) using a threshold of ΔAIC > 6 (Fox et al., 2015). For all three parameters, mixed-effects linear models were fitted using the lme4 package (Bates et al., 2015) with patch as random factor to account for non-independence of repeated surveys. Abundance and biomass were log-transformed and checking assumptions and performing significance tests were implemented as outlined for the models described above. The procedures were repeated for survey-area corrected abundance and biomass data: to get an estimate of how much fish there could be if the entire surveyed area had been restored, abundance and biomass values were divided by patch size (i.e. 16 m2) instead of the whole survey area (177 m2). Furthermore, for Control and Reference patches, the effect of year (2019, 2020 and 2022) on all three (uncorrected) parameters was compared using the mixed-effects linear model approach just described. Pearson correlation tests between average AR patch hard coral cover against fish species richness, abundance and biomass were performed. Lastly, fish community composition was compared between and within treatment patches using Bray-Curtis dissimilarity matrices based on average fish biomass per patch on both species and dietary guild level, following Morais & Bellwood (2020) for diet categories. Community differences were statistically evaluated using multivariate permutation tests (PERMANOVA) with Bonferroni adjustment and visually presented with non-metric multidimensional scaling (NMDS) plots using the vegan package (Oksanen et al., 2022).
Results
Benthic cover, fragment survival and coral recruitment
Hard coral cover was largely similar across AR patches at the start of the study (averaging 19%) and differences appeared over time (Figure 3). Reductions or increases in hard coral cover on AR patches were frequently mirrored by reciprocal increases or reductions in soft coral cover (mainly Cespitularia spp. and Rhytisma sp.), whereas macroalgae (mainly Dictyota sp. and Sargassum spp.) were only commonly seen at Reference patches (Figure 3). Benthic cover on Control and Reference patches remained largely unchanged throughout the study (Figure 3). At the end of the 2-year study, hard coral cover differed significantly between the six treatment patches (Figure 4; X2 = 373.43, df = 5, p < 0.001). Control patches remained devoid of hard coral (1%), whereas Cake (18%), Cage (32%) and Compound patches (29%) featured moderately high coral cover, comparable to Reference patches (26%); Bottle patches featured intermediate and therefore rather low coral cover (9%). Genus-level coral richness differed greatly between treatments (Figure 4): all AR patches were dominated almost exclusively by Acropora (though Cake patches featured several additional genera at low cover), whereas Reference patches showed a moderately diverse assemblage of genera (with very limited Acropora cover).
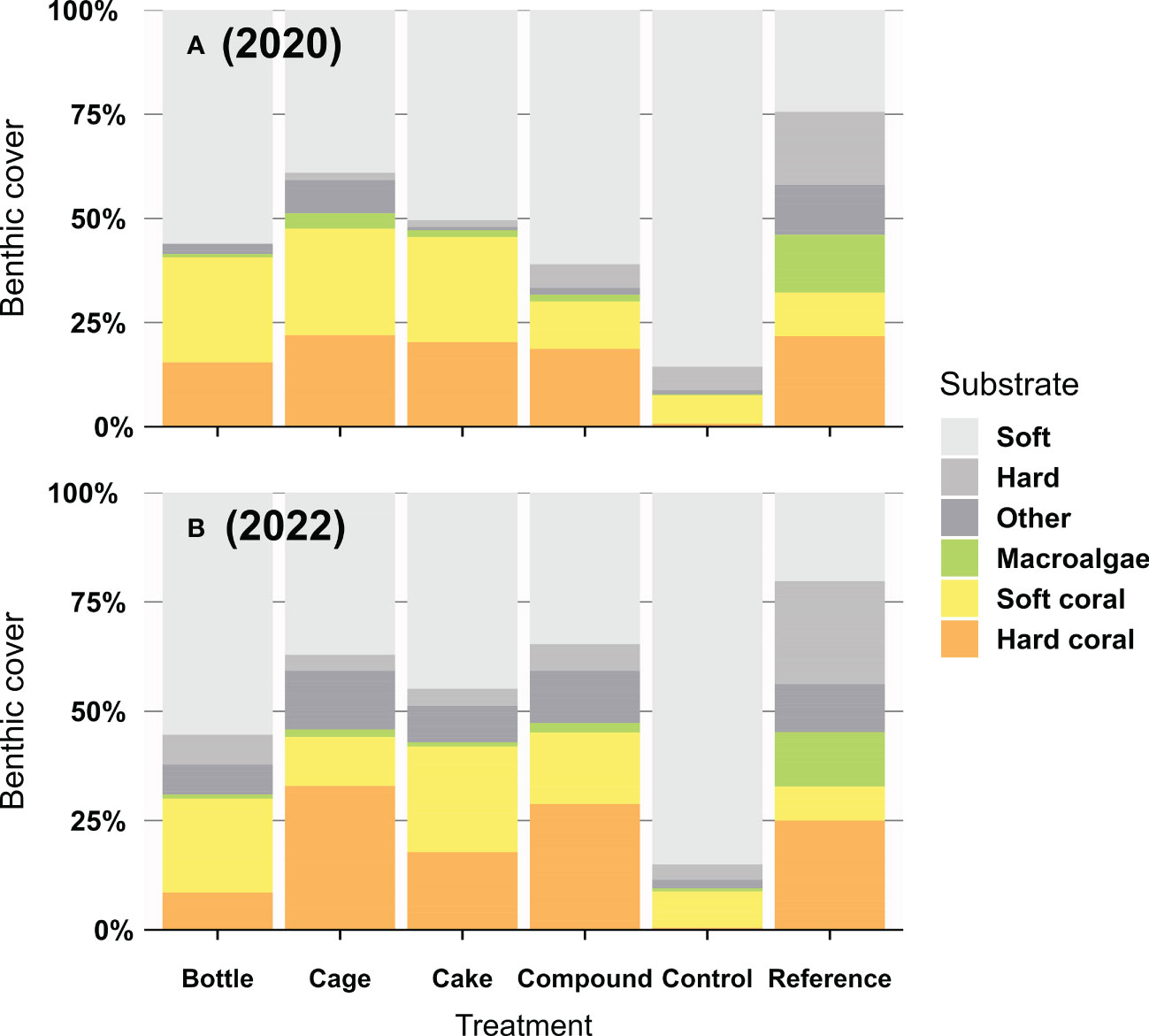
Figure 3 Percentage benthic cover (A) a few months and (B) two years after deployment of artificial reef patches, compared to unrestored Control patches and natural Reference reefs (n = 3 – 10 for 2020; n = 10 for 2022). Benthic substrate was divided into the following groups: Soft substrate (including sand, rubble and seagrass), Hard substrate (including bare rock and rocky substrate covered by crustose coralline algae or turf algae < 1 cm), Macroalgae (fleshy algae > 1 cm), Soft coral, Hard coral and the group Other including rarer sessile invertebrates such as sponges, tunicates and hydroids.
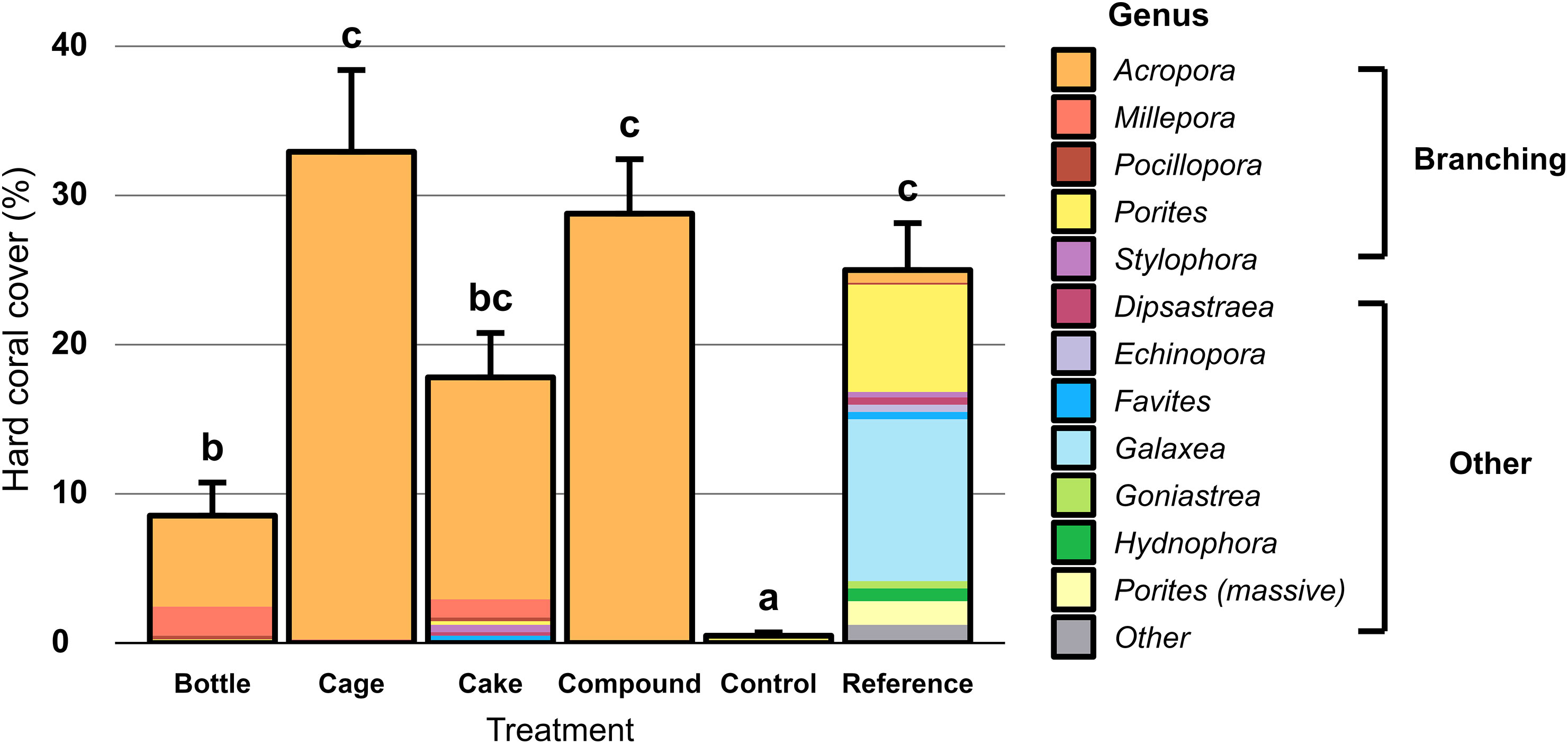
Figure 4 Percentage hard coral cover (including the hydrozoan Millepora) on artificial reef patches two years after deployment, unrestored Control patches and natural Reference patches. Error bars denote SE (n = 10) and treatments not sharing lowercase letters differ significantly (p < 0.05). Colours represent coral genera, split between branching corals and other growth forms.
Of all 4256 outplanted coral fragments, 2552 remained alive for two years (60%), 1300 died (31%) and 404 were dislodged (9%). Survival of Acropora fragments differed significantly between AR patches (X2 = 15.35, df = 3, p = 0.0015) and was higher on Cage and Compound patches compared to Bottle patches, with intermediate results for Cakes (Figure 5). Across all fragments and genera. CoTS were chiefly responsible for predation mortality (14% of outplanted fragments), with the remainder of predation mortality caused by Drupella spp. (1%). Other mortality causes included: dislodgement due to turtles (Eretmochelys imbricata and Chelonia mydas) scraping their carapace (5%), detachment after entanglement with fishing gear (4%) and benthic competition with neighbouring hard corals, sponges, tunicates or soft corals (2%). Bleaching caused the demise of 2% of coral fragments. For the remainder of dead coral fragments (10%), no clear cause could be identified; no symptoms of diseases were observed throughout the study. Identified mortality causes for Acropora were distinct between AR patches (Figure 5): predation caused substantial losses at all patches except Cages, which suffered more from detachment by fishing gear. Only Bottle patches suffered substantial dislodgement by sea turtles. Clear differences could also be observed between coral genera, both in terms of survival and mortality causes (Figure S2). Across all patches, survival was highest for Millepora spp. (85%) and Acropora spp. (64%), with much lower survival for Stylophora spp. (29%), Pocillopora spp. (16%) and Porites sp. (12%). All genera except Millepora suffered from predation, Acropora and Millepora suffered relatively little from competition, only Pocillopora was impacted by bleaching and for Porites the mortality causes remained largely unknown (Figure S2).
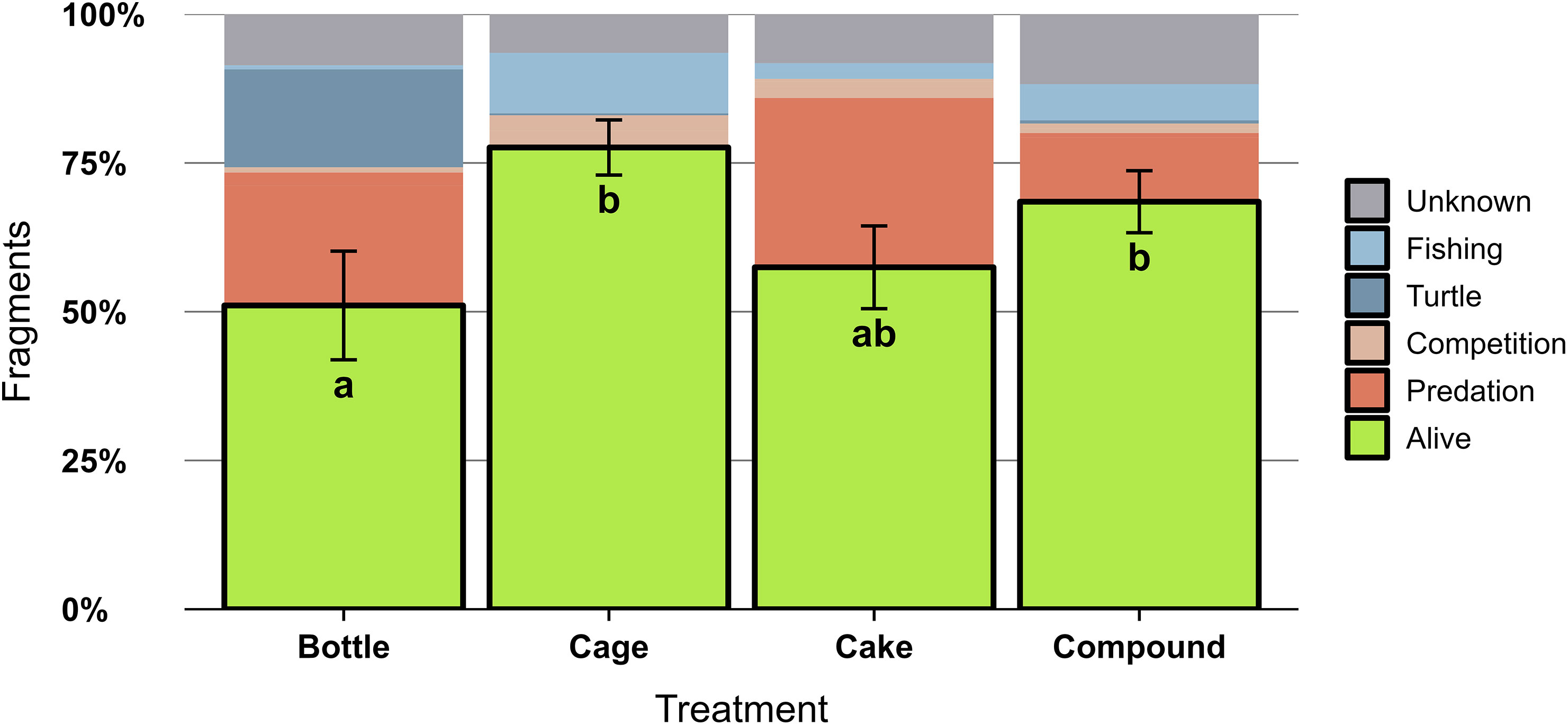
Figure 5 Survival of Acropora spp. coral fragments (N = 3580 fragments) two years after outplanting onto four different types of artificial reef patches (n = 10 per treatment). The percentage of surviving fragments are outlined in black with SE noted by error bars. Patches not sharing lowercase letter differ significantly (p < 0.05) in percentage of surviving fragments. The remainder of fragments did not survive and their putative mortality causes have been indicated: bleaching (mortality due to above-average water temperatures), fishing (detachment due to entanglement in fishing gear), turtle (detachment due to interaction with sea turtles), competition (mortality due to competing benthic organisms such as tunicates, sponges and soft coral), predation (consumption by Acanthaster sp. sea stars or Drupella spp. snails) or unknown cause of death.
Hard coral recruit density differed significantly between the six treatment patches (X2 = 148.62, df = 5, p < 0.001). Recruit density was very low at both Control (0.4 m-2) and Cage patches (0.2 m-2), significantly higher at Bottle (1.8 m-2), Compound (1.8 m-2) and Cake patches (2.9 m-2) and highest at Reference patches (4.8 m-2; Figure 6); differences between Cake and Reference patches were not significant. Genus-level richness of coral recruits mirrored this pattern (Figure 6), with highest number of genera found on Reference reefs. Recruits of Stylophora and Porites were dominant across patches. In total, 1401 recruits were observed on the ARs. Concrete featured both highest recruit densities (7 recruits m-2 versus < 4 recruits m-2 on all other material types) and genus-level richness (Figure S3). Nearly all of the 32 coral genera that settled onto concrete preferred to settle on vertical surfaces, which typically featured three-fold higher recruit densities than horizontal surfaces (Figure S4). Only Porites, Coscinaraea, Leptastrea and genera from the Merulinidae family (Favites, Dipsastraea, Cyphastrea and Goniastrea) were found in roughly equal densities on both orientations (Figure S4). Recruit densities were not correlated to hard coral cover on AR modules (r = 0.025, df = 868, p = 0.46).
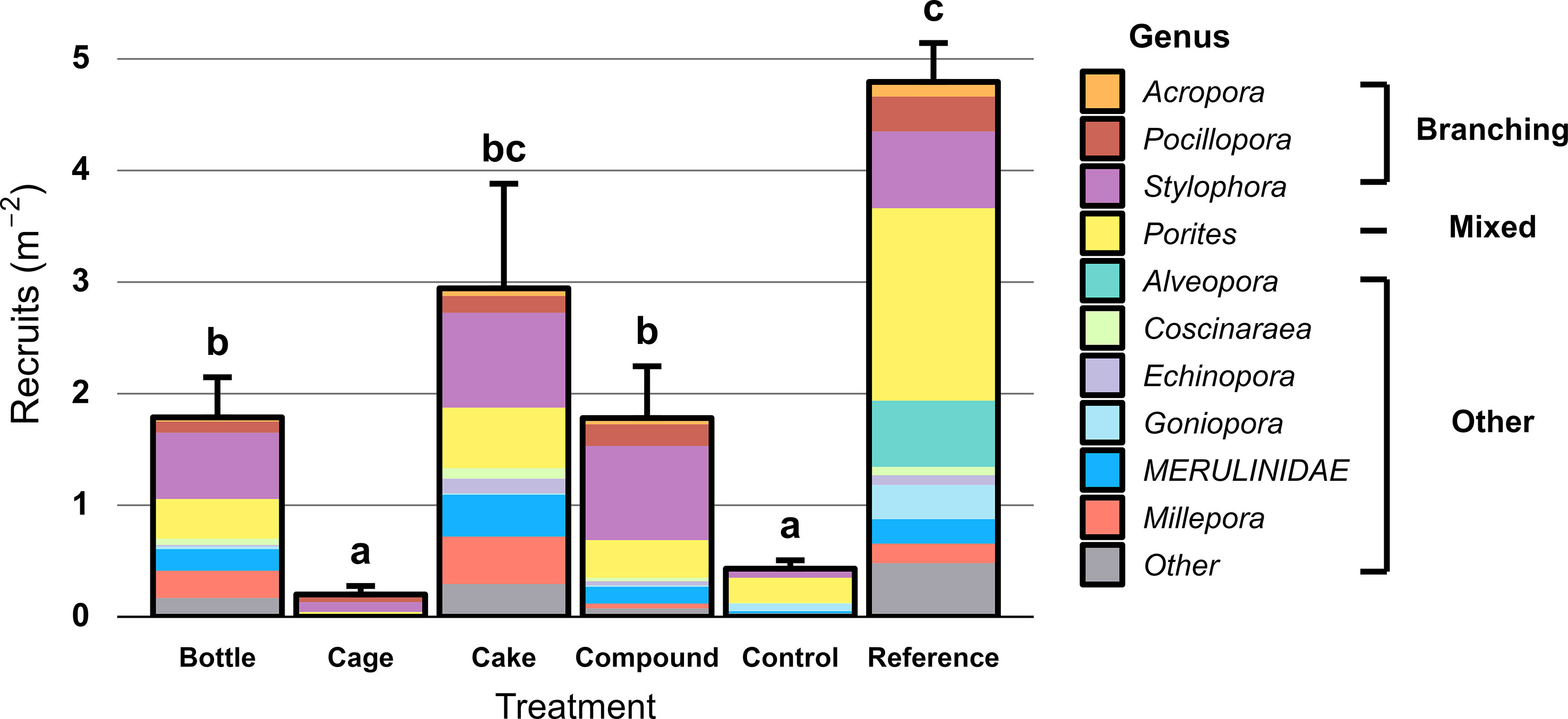
Figure 6 Hard coral recruit density (colonies 1 -10 cm diameter, including hydrozoan Millepora) two years after deployment at restored patches, compared to unrestored Control patches and natural Reference reefs. Error bars denote SE (n = 10) and treatments not sharing lowercase letters differ significantly (p < 0.05). Colours represent coral genera, split between branching corals and other growth forms.
Fish and invertebrate communities
There were significant differences between the six treatment patches in terms of fish species richness (X2 = 47.57, df = 5, p < 0.001), fish abundance (X2 = 54.90, df = 5, p < 0.001) and fish biomass (X2 = 69.46, df = 5, p < 0.001). Depth was a significant covariate in the model for fish species richness (deeper patches showed lower species richness: mean ± SE of beta estimate -2.3 ± 0.44 species m-1) and therefore depth-corrected values for richness are shown (Figure 7A). Patterns across treatment patches were similar for all three parameters: Control patches featured significantly lower fish species richness, abundance and biomass than all other patches, which did not differ among each other (Figures 7A–C). At Control patches, fish species richness (13 species) was just over half that of other patch types (21 – 24 species). Both the abundance (0.1 fish m-2) and biomass (8 kg ha-1) at Control patches were between five to twelvefold lower compared to all other patch types (abundance: 0.45 – 1.2 fish m-2; biomass: 38 – 92 kg ha-1). When standardized to actual area restored (i.e. excluding the unrestored but surveyed rubble areas surrounding the patches), all AR patches except Bottle patches featured significantly higher abundances (three to eightfold higher) and biomasses (two to sixfold higher) than natural Reference reefs (Figure S5). Coral cover on AR patches was positively correlated with fish abundance (r = 0.71, df = 38, p < 0.001) and fish biomass (r = 0.54, df = 38, p < 0.001), and a trend was seen for species richness (r = 0.31, df = 38, p = 0.055; Figure S6). The abundance and biomass of fish remained constant on both Control and Reference patches before and after restoration (Figure S7), though average fish richness increased (from 7 to 10 species on Control patches and from 19 to 27 species on Reference patches).
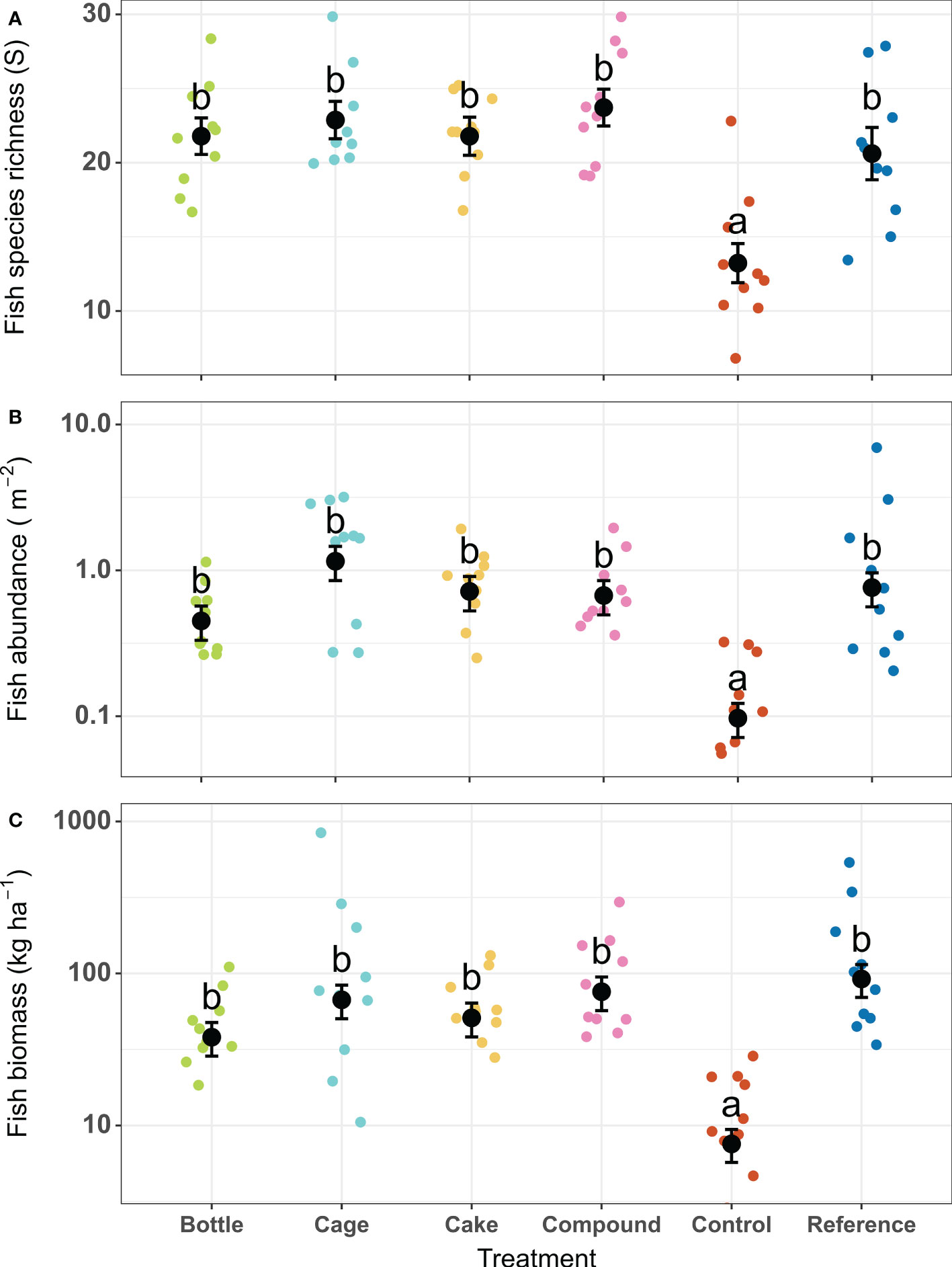
Figure 7 (A) Fish species richness per survey (corrected for covariate depth), (B) Fish abundance and (C) Fish biomass two years after deployment of restored patches, compared to unrestored Control patches and natural Reference patches. Error bars denote SE (n = 10) and treatments not sharing lowercase letters differ significantly (p < 0.05). Coloured points indicate values for replicate patches within each treatment type.
Fish species composition was significantly different between the six treatment patches (F = 2.77, df = 5, p < 0.001), see Table S4 for the Bray-Curtis dissimilarity matrix. The fish community at Control and Reference patches were different from each other (p < 0.001) and both were different from AR patches (all p < 0.05; Figure 8A). AR patches were similar to each other, except Bottle patches, which differed from Cage (p = 0.011) and Compound patches (p = 0.0077), and a significant difference between Cage and Cake patches (p = 0.024). The total number of fish species encountered was higher on Reference patches (145 species) than Bottle (108), Cage (103), Cake (101), Compound (113) and Control patches (72). Fish community composition on dietary guild level also differed between treatment patches (F = 3.49, df = 5, p < 0.001; Table S5), but now Reference patches were similar to all AR patches, while Control patches remained distinct (all p < 0.01; Figure 8B). The similar dietary guilds but different species compositions among restored and reference patches can be highlighted by some common planktivorous and omnivorous species such as Dascyllus trimaculatus and Dascyllus carneus (common on AR patches) compared to Plectroglphidodon lacrymatus and Chromis dimidiata (common Reference patches). Control patches hosted a fish community consisting mainly of invertivores, whereas herbivores were more associated with both Reference and AR patches. Predatory fish (e.g. Diagramma pictum and Lutjanus fulviflamma) were more associated with Cage patches, though not significantly so.
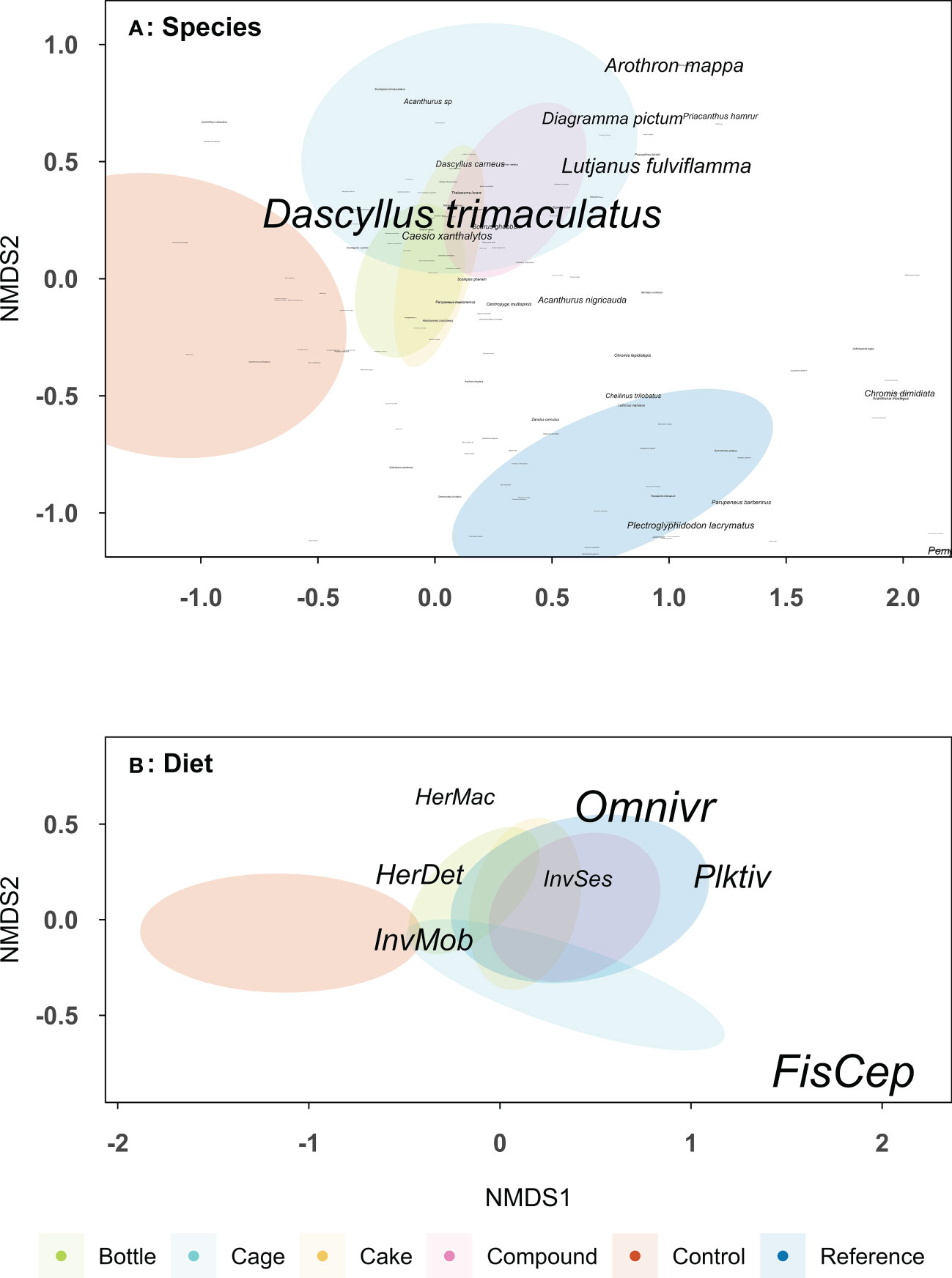
Figure 8 Non-metric multidimensional scaling (NMDS) plots based on Bray-Curtis dissimilarity matrices on (A) fish species (2 dimensions, stress: 0.199) and (B) fish dietary guild (2 dimensions, stress: 0.138). Font sizes increases with fish abundance. Data based on 10 replicate patches of four different types of artificial reef, unrestored Control patches and natural Reference patches (as indicated by colours). Data on the fish community was collected two years after deployment of the artificial reef patches. Ellipses show a 99% confidence interval around the centroid for each treatment. Dietary guilds are as follows: FisCep, fish and cephalopod predators, HerDet, herbivores/detritivores; HerMac, herbivores/macroalgivores; InvMob, invertivores (motile prey); InvSes, invertivores (sessile prey); Omnivr, omnivores; Plktiv, planktivores.
Motile invertebrates were generally more abundant and diverse at Reference patches compared to other patches, but patterns varied among key invertebrate groups (Figures 9A–E). Significant differences were found in abundances of corallivorous snails (X2 = 56.52, df = 5, p < 0.001) and sea urchins (X2 = 78.26, df = 5, p < 0.001) between treatment patches. Both groups were significantly more abundant at Reference patches compared to all other patches (Figures 9A, B). In addition, highest genus richness was found on References patches as well for these two invertebrate groups. Sea stars showed a similar pattern (Figure 9C), but differences were not significant. CoTS were rarely encountered, but some were seen on Bottle patches. No clear patterns nor statistical differences were seen for sea cucumbers (Figure 9D). Average abundance of gastropods did not differ significantly between Reference and AR patches, but family-level richness was higher at Reference patches. In contrast, AR patches were dominated by Cypraeidae gastropods (mainly Cypraea tigris), which were rarely encountered on Reference patches.
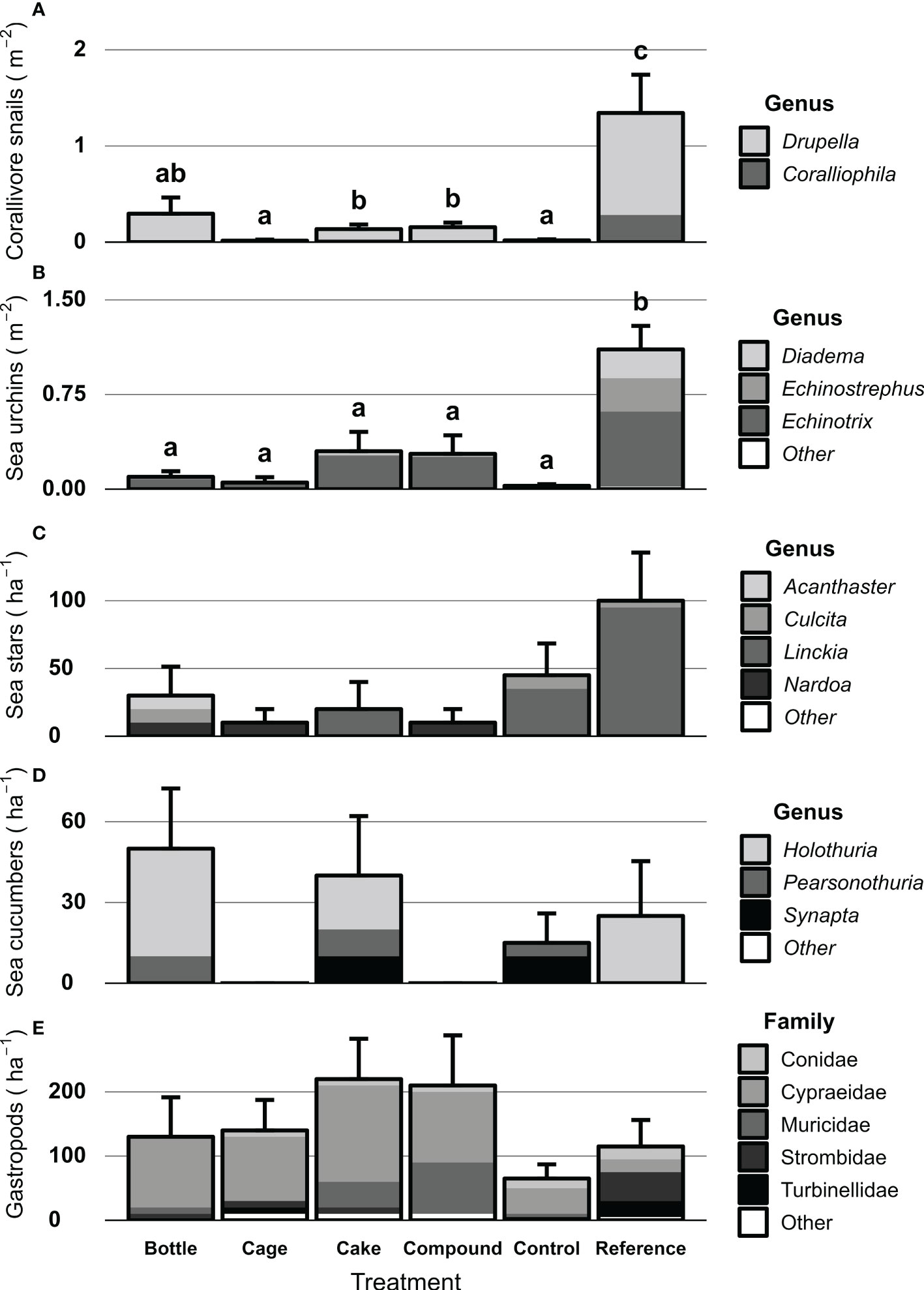
Figure 9 Densities of key motile invertebrates at artificial reef patches two years after deployment, unrestored Control patches and natural Reference patches. Key groups shown are (A) Corallivorous snails m-2, (B) Sea urchins m-2, (C) Sea stars ha-1, (D) Sea cucumbers ha-1 and (E) Large (> 5 cm) gastropods ha-1. Error bars denote SE (n = 10) and treatments not sharing lowercase letters differ significantly (p < 0.05). No significant difference between treatment patches were found for sea stars, sea cucumbers and large gastropods. Octopus and lobsters were rarely observed and not included here. Shades represent genera or families that make up the invertebrate communities.
Discussion
This study aimed to determine to what extent AR design influences the recovery of restored reefs using a uniquely broad ecological approach (Hein et al., 2020a) that monitored the development of outplanted coral fragments, coral recruitment and fish and invertebrate communities. Two years after their deployment, the AR patches with outplanted corals have shown positive development towards recovery of reef ecosystem functionality. Coral fragment survival was on par with reported global averages (Bayraktarov et al., 2019; Bostrom-Einarsson et al., 2020), but clearly differed between AR designs. Metal cages featured highest fragment survival and coral cover by preventing access to invertebrate coral predators, whereas a combination of predation by CoTS and dislodgement by sea turtles reduced fragment survival and increased soft coral cover on (especially low-set) concrete ARs. In contrast, coral recruitment was negligible on metal cages mostly due to their low surface area whereas coral recruitment was moderately high on concrete ARs, which featured both a higher surface area and a more suitable settlement substrate. Thus, metal cages featured high coral cover which remained dominated by outplanted branching corals (principally Acropora spp.), whereas concrete ARs featuring lower coral cover showed higher potential to increase coral diversity through natural recruitment. Fish abundance and biomass were similar across all AR designs, and were already after two years comparable to natural reference reefs. Abundance and biomass of fish are even expected to surpass levels of reference reefs when larger areas are restored, since these parameters already exceeded levels detected at reference reefs when unrestored areas around AR patches were excluded from the census counts. Fish species richness and trophic composition were also similar between all AR designs and reference reefs, though exact species compositions remained distinct between natural and restored reefs. Motile invertebrate communities remained less abundant and less diverse on AR patches and might need more time or specific habitat to get established. Taken together, all studied ecological parameters progressed towards reef recovery, with unique and varying influences of AR design. We recommend reef restoration with a combination of metal cages and layered concrete ARs to promote high fragment survival as well as natural coral recruitment. Further considerations on AR design, ecological facilitation and restoration recommendations are discussed below.
AR design
AR design clearly affected the performance of outplanted coral fragments by mediating the effects of reef organisms causing coral predation and detachment. CoTS predation was less intense than reported by earlier restoration studies in the region (Tamelander et al., 2000; Mbije et al., 2013), but was still causing substantial coral mortality on bottle and cake patches despite low observed densities of the sea star. CoTS were not able to climb metal cages, which explains the higher fragment survival on these ARs. Similarly, predation by corallivorous snails can be reduced using cages, especially when recruitment by snail larvae from the water column is low and colonization of ARs would have to happen by ground-dwelling adult snails (Williams et al., 2014). Coral predation can increase hard coral diversity by selectively targeting fast growing genera (Neudecker, 1979; Cox, 1986), but extensive predation might also induce overgrowth of coral colonies and even full ARs by benthic competitors such as macroalgae (Rice et al., 2019) and soft corals (Bruno et al., 2009; Norström et al., 2009). In this study, fragments that died due to predation were quickly overgrown by soft corals. This space occupation by soft corals can prevent hard coral recruitment, succession and reef recovery (Sammarco et al., 1985; Norström et al., 2009). Remarkably, also sea turtles hampered efforts to increase hard coral cover by dislodging fragments when scraping the underside of their carapaces on bottle modules. This, in combination with high predation on the same modules, has rendered the bottle design least successful for coral survival. Besides AR design, coral genus was also an important determinant of fragment survival with clear genus-specific mortality causes such as predation (Acropora and Stylophora) and bleaching (Pocillopora). The high but unexplained mortality of Porites sp. could possibly indicate that for this species, larger fragment sizes could be needed for outplanting (Seebauer, 2001).
Hard coral recruitment on concrete ARs was moderately high and diverse compared to reference reefs and falls within the range of regional averages of around 2 – 8 recruits m-2 (Obura et al., 2008; Visram et al., 2009), highlighting the potential of ARs and reef restoration to support key ecological processes and recovery towards a functional reef (Montoya-Maya et al., 2016; Hein et al., 2020a). The finding that recruit densities on concrete approached densities on natural reef substrates demonstrates that concrete is a suitable substrate for coral recruitment, especially when vertically oriented. Also, metal appears a suitable substrate for the settlement of at least certain coral genera, but the low surface area of the cage module resulted in low overall coral recruitment at this AR design. Sufficiently high coral recruitment (Graham et al., 2014) and high outplanted fragment densities (Ladd et al., 2016) can prevent shifts to benthic competitors which could otherwise inhibit reef recovery (Norström et al., 2009; Ladd et al., 2018). The absence of an association between coral cover and recruit densities on AR modules, though, suggests that other factors than nearby hard corals per se are important for coral settlement and survival, such as provision of suitable hard substrate (Hata et al., 2017). Nonetheless, over time, outplanted corals are expected to contribute to reproduction (Horoszowski-Fridman et al., 2011) and local recruitment (Montoya-Maya et al., 2016). For the moment, common recruits mainly included opportunistic brooding genera such as Pocillopora and Stylophora and the stress-tolerant genus Porites (sensu Darling et al., 2012), and it remains unclear if outplanting of once dominant competitive species such as broadcasting Acropora will assist their recruitment and comeback in absence of natural recovery (McClanahan et al., 2001; McClanahan, 2008; McClanahan et al., 2014).
Contrary to our expectations, AR design did not affect the composition of fish or invertebrate communities that developed around the restored patches. This contrasts earlier studies, as both AR design (Bohnsack and Sutherland, 1985; Hylkema et al., 2020) and structural complexity (Graham and Nash, 2013) have been shown to be determinants of fish communities (Seraphim et al., 2020). The levels of structural complexity provided by the various studied ARs differed in rugosity and more detailed quantification of this structural complexity could possibly help explain the different impacts on reef communities. Provision of fine-scale habitat and food by corals is likely the main driver for development of fish and invertebrate communities on these ARs, as indicated by the association between AR coral cover and fish abundance and biomass in this study. This suggests that hard coral cover is more crucial for reef fish communities than artificially created structural complexity (Coker et al., 2014; Pratchett et al., 2014). Follow-up factorial studies that separate the effects of ARs and outplanted corals on reef communities can help to clarify the benefits of each restoration approach. Interestingly, fish species richness appeared unrelated to both AR design and coral cover, implying that these factors are less important if restoration of fish species richness is the only goal. The difference in fish species composition between AR patches and reference reefs is commonly observed (Higgins et al., 2022), and likely relates to the different coral species composition (Berumen and Pratchett, 2006) and probably additional factors such as AR material, complexity, relative size or age (Hylkema et al., 2021). Especially age could be an important yet largely unstudied factor, as communities can undergo successional changes and the studied ARs were still very young compared to natural reefs. On a level of dietary guilds, however, the similarity between ARs and reference reefs shows that restored fish communities can support similar trophic roles as natural fish communities (in agreement with Paxton et al., 2020). The effects of AR patch size and condition of the direct surroundings remain to be tested, but extrapolation (Figure S5) indicates that substantial potential exists for AR patch size to further enhance fish communities, especially in combination with hierarchical spatial arrangement (Bohnsack et al., 1994). The absence of clear patterns for invertebrates might relate to their natural low abundances and high variability (McClanahan, 1989) and comparisons and interpretation are further complicated by the lack of data on this diverse functional group in restoration projects (Hylkema et al., 2021; Higgins et al., 2022).
Ecological facilitation
The identified fish and invertebrate communities can improve reef recovery when mediating ecological processes in favour of hard cover growth and recruitment (Shaver and Silliman, 2017; Ladd et al., 2018) and measuring these functional processes directly will contribute to a better mechanistic understanding of coral reef restoration ecology. For example, herbivores can prevent the establishment of macroalgae and thereby create a competitive advantage for corals (Hughes et al., 2007). Local herbivorous key species such as grazing surgeonfish (Knoester et al., 2019), sea urchins (Humphries et al., 2020) and browsing unicornfish (Knoester et al., 2022) might indeed have controlled macroalgae on ARs in this study, but sessile benthic invertebrates became more abundant over time compared to natural reefs, including contentious competitors of hard coral such as soft corals, tunicates and sponges (Stobart et al., 2005; Bruno et al., 2009). The current grazer community around ARs (despite high abundances of, for example, the sponge and soft-coral eating gastropod Cypraea tigris) might not be able to provide sufficient top down control on these often toxic sessile invertebrates (La Barre et al., 1986; Pawlik et al., 2018), which might additionally benefit from elevated nutrient levels in the relatively turbid study area (Pastorok and Bilyard, 1985; Norström et al., 2009). Pre-emptive space occupation by outplanting more hard corals and thereby intensify grazing pressure on smaller areas of remaining open substrate could help to prevent the establishment benthic competitors, but requires outplanting corals at appropriate densities and subsequent high fragment survival (Ladd et al., 2016). Outplant densities used in this study appear sufficiently high to sustain coral cover, except for the Bottle ARs that suffered high predation and dislodgement. Quantification of these important ecological processes can further improve reef restoration techniques.
Additional ways of ecological facilitation include control of corallivores through predation or for example nutrient cycling by fish (Shaver and Silliman, 2017; Ladd et al., 2018). Natural control of coral-predating invertebrates is important to prevent pest-like outbreaks (Rotjan and Lewis, 2008; Rice et al., 2019), especially as restoration projects generally use coral species susceptible to predation (Cole et al., 2008). As broad trophic roles of the fish community in this study were similar among ARs and reference reefs this could indicate that such functions are being re-established, but more detailed and empirical data on key species would be needed to confirm this. Also, top-down control on adult CoTS was not sufficient to prevent substantial coral predation. To maintain sufficient coral cover on recently established ARs, reducing coral predation directly through AR design or outplanted species selection appears more effective than attempting to regulate corallivore populations indirectly by facilitating their predators. This suggestion applies in particular to larger adult corallivores, which are less sensitive to predation (Cowan et al., 2017; Shaver et al., 2020). Excess nutrient inputs by humans are generally detrimental to reef functioning and could promote corallivores (Shantz and Burkepile, 2014; Pratchett et al., 2017), but nutrient recycling by fish communities has been shown to benefit corals on natural reefs (Shantz et al., 2015) and might help to facilitate recovery of degraded reefs (Ladd and Shantz, 2020). For example, the colonization of outplanted corals by planktivorous damselfish can be expected to enhance coupling of pelagic nutrients to restored reefs (Seraphim et al., 2020) and schooling predatory fish can create nutrient hotspots (Shantz et al., 2015). A better understanding of such processes could further improve reef restoration effectiveness (Shaver and Silliman, 2017; Ladd et al., 2018).
Methodological considerations
This study provides a more holistic evaluation of reef ecosystem restoration performance than item-based monitoring only (Bayraktarov et al., 2019; Abelson et al., 2020). For future work, three further methodological improvements are recommended. Firstly, two years is a short time in ecology and this study therefore only represents the early successional stage of reef recovery, although longer than most reef restoration studies (Bayraktarov et al., 2016). Over time, when coral recruits grow, outplanted corals become fertile and benthic cover further increases complexity, AR communities are expected to become more diverse and resemble natural reefs more closely (Thanner et al., 2006), while likely still remaining distinct (Hylkema et al., 2021). Long term monitoring (> 5 – 10 years) will therefore be vital to track succession (Hein et al., 2020b), understand ecological interactions (Seraphim et al., 2020) and ensure functional reefs which continue to provide ecosystem services (Abelson et al., 2020; Hein et al., 2021). Secondly, the long-standing debate on relative contributions of attraction versus production of fish and invertebrates around ARs needs to be clarified (Pickering and Whitmarsh, 1997), especially if the restoration goal is to support sustainable exploitation (Lima et al., 2019; Hylkema et al., 2021). On the studied ARs, the high abundance of damselfish species not regularly encountered on nearby reefs indicate that local production of these small planktivorous fishes is likely, which might in turn support production on higher trophic levels. Abundance and biomass of fishes at nearby natural reefs did not decrease, despite the substantial addition of ARs, which further suggests that ARs were supporting local production. Of course, the relative size of the restored area compared to the surroundings and distance to healthy reefs will be of great influence on this. Incorporation of age cohort monitoring over time will provide more certainty on this aspect (Brickhill et al., 2005). Thirdly, biases in the used methodologies should be noted: observer bias might explain the observed increase in fish species richness over the different years on control and reference patches and this could be addressed by monitoring methods using artificial intelligence (Barbedo, 2022), and the discrepancy between small AR size and large surveyed area for fishes as well as the small areal coverage for benthic surveys ideally are solved by upscaling restoration efforts rather than through adjusted monitoring methodologies.
Restoration recommendations
Concluding, we highlight several recommendations with broader relevance. Firstly, as clearly outlined in restoration guidelines (Precht, 2006; Edwards et al., 2010), causes of coral decline need to be addressed for restoration to be successful. During the current study, fishing efforts still interfered with restoration efforts both directly by dislodging fragments and likely indirectly by affecting the community composition of fish – also on natural reference reefs (McClanahan et al., 2008). Continued efforts to align restoration with protection are therefore crucial (Hylkema et al., 2021). Secondly, at least equally important, adaptation to climate change impacts must be considered. To address this potential threat to restoration success, we used, where possible, presumed temperature-resilient corals (outplanted Acropora spp. and other corals that had survived previous bleaching episodes) and we placed ARs slightly deeper to reduce combined heat and light stress. This might have contributed to the low bleaching mortality among most outplanted corals despite significant heat stress during the study. However, the bleaching mortality of Pocillopora spp. and the anticipated ever-increasing temperatures clearly show more research is needed to combine ongoing restoration efforts with new techniques such as assisted evolution (van Oppen et al., 2017; NASEM, 2018; Rinkevich, 2019). There should be no doubt, however, that long-term success of coral reef conservation ultimately depends on how soon global greenhouse gas emissions are curbed (Knowlton et al., 2021). Thirdly, the clear effects of AR design and species selection on coral survival highlight the importance of these factors for reef restoration success. A combination of AR designs with species-specific outplanting strategies is recommended to realize high coral cover and diversity: predation-sensitive genera such as Acropora can be placed on elevated structures such as cages, whereas predation-resilient corals such as Millepora and Porites can be put on concrete structures such as layered cakes. In this way, coral cover can be increased quickly using pioneer species while coral recruitment is also facilitated, supporting the development of a more diverse and resilient coral community, which can in turn support a fish community that is functionally similar to natural reefs (Horoszowski-fridman and Rinkevich, 2016). Low concrete structures such as bottle reefs are not recommended for coral outplanting, but could be placed specifically to create turtle hangouts in the studied area. Further improvements in coral performance are likely possible by varying outplant density (Ladd et al., 2018) and species composition (Cabaitan et al., 2015). Altogether, considerations of these ecology-based processes have the potential to improve outplanting success even further (Shaver and Silliman, 2017; Ladd et al., 2018). Ultimately, monitoring at socio-ecological relevant scales will determine if reef restoration can support the recovery of coral reefs and their services and thus can be considered an effective, efficient and engaging conservation tool (McDonald et al., 2016; Goergen et al., 2020).
Data availability statement
The datasets presented in this study can be found in online repositories. The names of the repository/repositories and accession number(s) can be found below: https://github.com/ewoutknoester/SurveysAR.
Author contributions
EK: Conceptualization, methodology, formal analysis, investigation, data curation, writing – original draft, visualization. JR: Methodology, investigation, formal analysis, writing – review & editing. QS: Methodology, investigation, writing – review & editing. AW: Methodology, investigation, writing – review & editing. AM: Conceptualization, resources, writing – review & editing, supervision. RO: Conceptualization, methodology, writing – review & editing, supervision, project administration. All authors contributed to the article and approved the submitted version.
Funding
This study was funded through the University Fund Wageningen and an anonymous donor. This study would not have been possible without the Global Environment Facility Small Grants Programme supporting the ongoing restoration activities of Mkwiro Beach Management Unit.
Acknowledgments
We like to thank members of Mkwiro Beach Management Unit, REEFolution Foundation and REEFolution Trust for collaborating on this large field study. A special thanks to all REEF Rangers and students who helped with the deployment of artificial reefs and outplanting of corals. We thank Pilli Pipa Dhow Safari for their logistic support for all activities. We thank the reviewers for their time taken to provide valuable input.
Conflict of interest
The authors declare that the research was conducted in the absence of any commercial or financial relationships that could be construed as a potential conflict of interest.
Publisher’s note
All claims expressed in this article are solely those of the authors and do not necessarily represent those of their affiliated organizations, or those of the publisher, the editors and the reviewers. Any product that may be evaluated in this article, or claim that may be made by its manufacturer, is not guaranteed or endorsed by the publisher.
Supplementary material
The Supplementary Material for this article can be found online at: https://www.frontiersin.org/articles/10.3389/fmars.2023.1152106/full#supplementary-material
References
Abelson A., Reed D. C., Edgar G. J., Smith C. S., Kendrick G. A., Orth R. J., et al. (2020). Challenges for restoration of coastal marine ecosystems in the anthropocene. Front. Mar. Sci. 7. doi: 10.3389/fmars.2020.544105
Anthony K. R. N., Bay L., Costanza R., Firn J., Gunn J., Harrison P., et al. (2017). New interventions are needed to save coral reefs. Nat. Ecol. Evol. 1, 1420–1422. doi: 10.1038/s41559-017-0313-5
Anthony K. R. N., Marshall P. A., Abdulla A., Beeden R., Bergh C., Black R., et al. (2015). Operationalizing resilience for adaptive coral reef management under global environmental change. Glob. Change Biol. 21, 48–61. doi: 10.1111/gcb.12700
Anthony K. R. N., Maynard J. A., Diaz-Pulido G., Mumby P. J., Marshall P. A., Cao L., et al. (2011). Ocean acidification and warming will lower coral reef resilience. Glob. Change Biol. 17, 1798–1808. doi: 10.1111/j.1365-2486.2010.02364.x
Arthurton R., Korateng K. (2006). “Coastal and marine environments,” in Africa Environment outlook 2. our environment, our wealth (Nairobi, Kenya: United Nations Environment Programme), 155–195.
Baine M. (2001). Artificial reefs: a review of their design, application, management and performance. Ocean Coast. Manage. 44, 441–259. doi: 10.1038/s41559-020-1281-8
Barbedo J. G. A. (2022). A review on the use of computer vision and artificial intelligence for fish recognition, monitoring, and management. Fishes 7, 335. doi: 10.3390/fishes7060335
Bates D., Mächler M., Bolker B. M., Walker S. C. (2015). Fitting linear mixed-effects models using lme4. J. Stat. Software 67. doi: 10.18637/jss.v067.i01
Bayraktarov E., Saunders M. I., Abdullah S., Mills M., Beher J., Possingham H. P., et al. (2016). The cost and feasibility of marine coastal restoration. Ecol. Appl. 26, 1055–1074. doi: 10.5061/dryad.rc0jn
Bayraktarov E., Stewart-Sinclair P. J., Brisbane S., Boström-Einarsson L., Saunders M. I., Lovelock C. E., et al. (2019). Motivations, success, and cost of coral reef restoration. Restor. Ecol. 27, 981–991. doi: 10.1111/rec.12977
Berumen M. L., Pratchett M. S. (2006). Recovery without resilience: persistent disturbance and long-term shifts in the structure of fish and coral communities at tiahura. Coral Reefs 25, 647–653. doi: 10.1007/s00338-006-0145-2
Bohnsack J. A., Bannerot S. P. (1986). A stationary visual census technique for quantitatively assessing community structure of coral reef fishes. NOAA Tech. Rep. NMFS 41, 21.
Bohnsack J. A., Harper D. E., McClellan D. B., Hulsbeck M. (1994). Effects of reef size on colonization and assemblage structure of fishes at artificial reefs off southeastern Florida, U.S.A. Bull. Mar. Sci. 55, 796–823.
Bohnsack J. A., Sutherland D. L. (1985). Artificial reef research: a review with recommendations for future priorities. Bull. Mar. Sci. 37, 11–39.
Bostrom-Einarsson L., Babcock R. C., Bayraktarov E., Ceccarelli D. M., Cook N., Ferse S. C. A., et al. (2020). Coral restoration – a systematic review of current methods, successes, failures and future directions. PloS One 15 (1), 1–24. doi: 10.1371/journal.pone.0226631
Brickhill M. J., Lee S. Y., Connolly R. M. (2005). Fishes associated with artificial reefs: attributing changes to attraction or production using novel approaches. J. Fish Biol. 67, 53–71. doi: 10.1111/j.0022-1112.2005.00915.x
Bruno J. F., Sweatman H., Precht W. F., Selig E. R., Schutte V. G. W. (2009). Assessing evidence of phase shifts from coral to macroalgal dominance on coral reefs. Ecology 90, 1478–1484. doi: 10.1890/08-1781.1
Burke L., Reytar K., Spalding M. D., Perry A. (2011). Reefs at risk revisited (Washington, DC: World Resources Institute). doi: 10.1016/0022-0981(79)90136-9
Cabaitan P. C., Yap H. T., Gomez E. D. (2015). Performance of single versus mixed coral species for transplantation to restore degraded reefs. Restor. Ecol. 23, 349–356. doi: 10.1111/rec.12205
Carr M. H., Hixon M. A. (1997). Artificial reefs: The importance of comparisons with natural reefs. Fisheries 22, 28–33. doi: 10.1577/1548-8446(1997)022<0028:artioc>2.0.co;2
Ceccarelli D. M., McLeod I. M., Bostrom-Einarsson L., Bryan S. E., Chartrand K. M., Emslie M. J., et al. (2020). Substrate stabilisation and small structures in coral restoration: State of knowledge, and considerations for management and implementation. PloS One 15, 1–27. doi: 10.1371/journal.pone.0240846
Coker D. J., Wilson S. K., Pratchett M. S. (2014). Importance of live coral habitat for reef fishes. Rev. Fish Biol. Fish. 24, 89–126. doi: 10.1007/s11160-013-9319-5
Cole A. J., Pratchett M. S., Jones G. P. (2008). Diversity and functional importance of coral-feeding fishes on tropical coral reefs. Fish Fish. 9, 286–307. doi: 10.1111/j.1467-2979.2008.00290.x
Cowan Z. L., Pratchett M. S., Messmer V., Ling S. (2017). Known predators of crown-of-thorns starfish (Acanthaster spp.) and their role in mitigating, if not preventing, population outbreaks. Diversity 9, 1–19. doi: 10.3390/d9010007
Cox E. F. (1986). The effects of a selective corallivore on growth rates and competition for space between two species of Hawaiian corals. J. Exp. Mar. Bio. Ecol. 101, 161–174. doi: 10.1016/0022-0981(86)90047-X
Cribari-Neto F., Zeileis A. (2010). Beta regression in r. J. Stat. Software 34, 129–150. doi: 10.1201/9781315119403-7
Darling E. S., Alvarez-Filip L., Oliver T. A., Mcclanahan T. R., Côté I. M. (2012). Evaluating life-history strategies of reef corals from species traits. Ecol. Lett. 15, 1378–1386. doi: 10.1111/j.1461-0248.2012.01861.x
Duarte C. M., Agusti S., Barbier E., Britten G. L., Castilla J. C., Gattuso J. P., et al. (2020). Rebuilding marine life. Nature 580, 39–51. doi: 10.1038/s41586-020-2146-7
Edwards A. J., Guest J. R., Shafir S., Fisk D., Gomez E. D., Rinkevich B., et al. (2010). Reef rehabilitation manual (Australia: St Lucia).
Ferse S. C. A., Hein M. Y., Rölfer L. (2021). A survey of current trends and suggested future directions in coral transplantation for reef restoration. PloS One 16, 1–21. doi: 10.1371/journal.pone.0249966
Fox G. A., Negrete-Yankelevich S., Sosa V. J. (2015). Ecological statistics: Contemporary theory and application (USA: Oxford University Press).
Frias-Torres S., Van de Geer C. (2015). Testing animal-assisted cleaning prior to transplantation in coral reef restoration. PeerJ 3, e1287. doi: 10.7717/peerj.1287
Froese R., Pauly D. (2015) FishBase. Available at: www.fishbase.org (Accessed January 3, 2015).
Goergen E. A., Schopmeyer S., Moulding A., Moura A., Kramer P., Viehman S. (2020). Coral reef restoration monitoring guide: methods to evaluate restoration success from local to ecosystem scales. Silver Spring, MD: National Oceanic and Atmospheric Administration. doi: 10.25923/xndz-h538
Graham N. A. J., Bellwood D. R., Cinner J. E., Hughes T. P., Norström A. V., Nyström M. (2013). Managing resilience to reverse phase shifts in coral reefs. Front. Ecol. Environ. 11, 541–548. doi: 10.1890/120305
Graham N. A. J., Jennings S., Macneil M. A., Mouillot D., Wilson S. K. (2014). Predicting climate-driven regime shifts versus rebound potential in coral reefs. Nature 518, 94–97. doi: 10.1038/nature14140
Graham N. A. J., Nash K. L. (2013). The importance of structural complexity in coral reef ecosystems. Coral Reefs 32, 315–326. doi: 10.1007/s00338-012-0984-y
Hata T., Madin J. S., Cumbo V. R., Denny M., Figueiredo J., Harii S., et al. (2017). Coral larvae are poor swimmers and require fine-scale reef structure to settle. Sci. Rep. 7, 1–9. doi: 10.1038/s41598-017-02402-y
Hein M. Y., Beeden R., Birtles A., Gardiner N. M., Le Berre T., Levy J., et al. (2020a). Coral restoration effectiveness: multiregional snapshots of the long-term responses of coral assemblages to restoration. Diversity 12, 1–22. doi: 10.3390/D12040153
Hein M. Y., McLeod I. M., Shaver E., Vardi T., Pioch S., Boström-Einarsson L., et al. (2020b). Coral reef restoration as a strategy to improve ecosystem services - a guide to coral restoration methods. (Nairobi, Kenya: United Nations Environment Programme).
Hein M. Y., Vardi T., Shaver E. C., Pioch S., Boström-Einarsson L., Ahmed M., et al. (2021). Perspectives on the use of coral reef restoration as a strategy to support and improve reef ecosystem services. Front. Mar. Sci. 8. doi: 10.3389/fmars.2021.618303
Heron S. F., Eakin C. M., Douvere F., Anderson K. D., Day J. C., Geiger E. F., et al. (2017). Impacts of climate change on world heritage coral reefs: a first global scientific assessment (Paris: UNESCO World Heritage Centre).
Higgins E., Metaxas A., Scheibling R. E. (2022). A systematic review of artificial reefs as platforms for coral reef research and conservation. PloS One 17, 1–23. doi: 10.1371/journal.pone.0261964
Horoszowski-Fridman Y. B., Izhaki I., Rinkevich B. (2011). Engineering of coral reef larval supply through transplantation of nursery-farmed gravid colonies. J. Exp. Mar. Bio. Ecol. (New York: Springer International Publishing), 399, 162–166. doi: 10.1016/j.jembe.2011.01.005
Horoszowski-fridman Y. B., Rinkevich B. (2016). Restoration of the animal forests: harnessing silviculture biodiversity concepts for coral transplantation. doi: 10.1007/978-3-319-17001-5
Hughes T. P., Rodrigues M. J., Bellwood D. R., Ceccarelli D. M., Hoegh-Guldberg O., McCook L. J., et al. (2007). Phase shifts, herbivory, and the resilience of coral reefs to climate change. Curr. Biol. 17, 360–365. doi: 10.1016/j.cub.2006.12.049
Humphries A. T., McClanahan T. R., McQuaid C. D. (2020). Algal turf consumption by sea urchins and fishes is mediated by fisheries management on coral reefs in Kenya. Coral Reefs 39 (4), 1137–1146. doi: 10.1007/s00338-020-01943-5
Hunter W. R., Sayer M. D. J. (2009). The comparative effects of habitat complexity on faunal assemblages of northern temperate artificial and natural reefs. ICES J. Mar. Sci. 66, 691–698. doi: 10.1093/icesjms/fsp058
Hylkema A., Debrot A. O., Osinga R., Bron P. S., Heesink D. B., Izioka A. K., et al. (2020). Fish assemblages of three common artificial reef designs during early colonization. Ecol. Eng. 157, 105994. doi: 10.1016/j.ecoleng.2020.105994
Hylkema A., Hakkaart Q. C. A., Reid C. B., Osinga R., Murk A. J., Debrot A. O. (2021). Artificial reefs in the Caribbean: a need for comprehensive monitoring and integration into marine management plans. Ocean Coast. Manage. 209, 105672. doi: 10.1016/j.ocecoaman.2021.105672
Kawaka J. A., Samoilys M. A., Murunga M., Church J., Abunge C., Maina G. W. (2017). Developing locally managed marine areas: Lessons learnt from Kenya. Ocean Coast. Manage. 135, 1–10. doi: 10.1016/j.ocecoaman.2016.10.013
Knoester E. G., Murk A. J., Osinga R. (2019). Benefits of herbivorous fish outweigh costs of corallivory in coral nurseries placed close to a Kenyan patch reef. Mar. Ecol. Prog. Ser. 611, 143–155. doi: 10.3354/meps12869
Knoester E. G., Plug V. E., Murk A. J., Sande S. O., Osinga R. (2022). Fisheries restrictions and their cascading effects on herbivore abundance and macroalgae removal at Kenyan coral reefs. J. Exp. Mar. Bio. Ecol. 559, 151850. doi: 10.1016/j.jembe.2022.151850
Knowlton N., Grottoli A. G., Kleypas J., Obura D., Corcoran E., de Goeij J. M., et al. (2021). “Rebuilding coral reefs: A decadal grand challenge,” in Int. coral reef Soc. futur. earth coasts (Bremen, Germany: International Coral Reef Society), 56. Available at: http://coralreefs.org/wp-content/uploads/2021/07/ICRS_2021_Policy_Brief_high_resol.pdf.
La Barre S. C., Coll J. C., Sammarco P. W. (1986). Defensive strategies of soft corals (Coelenterata ;: Octocorallia) of the great barrier reef. II. the relationship between toxicity and feeding deterrence. Biol. Bull. 171, 565–576. doi: 10.2307/1541624
Ladd M. C., Miller M. W., Hunt J. H., Sharp W. C., Burkepile D. E. (2018). Harnessing ecological processes to facilitate coral restoration. Front. Ecol. Environ. 16, 239–247. doi: 10.1002/fee.1792
Ladd M. C., Shantz A. A. (2020). Trophic interactions in coral reef restoration: a review. Food Webs 24, e00149. doi: 10.1016/j.fooweb.2020.e00149
Ladd M. C., Shantz A. A., Nedimyer K., Burkepile D. E. (2016). Density dependence drives habitat production and survivorship of acropora cervicornis used for restoration on a Caribbean coral reef. Front. Mar. Sci. 3. doi: 10.3389/fmars.2016.00261
Lenth R. (2020) Emmeans: Estimated marginal means, aka least-squares means. Available at: https://cran.r-project.org/package=emmeans.
Levy G., Shaish L., Haim A., Rinkevich B. (2010). Mid-water rope nursery: testing design and performance of a novel reef restoration instrument. Ecol. Eng. 36, 560–569. doi: 10.1016/j.ecoleng.2009.12.003
Lima J. S., Zalmon I. R., Love M. (2019). Overview and trends of ecological and socioeconomic research on artificial reefs. Mar. Environ. Res. 145, 81–96. doi: 10.1016/j.marenvres.2019.01.010
Lirman D., Thyberg T., Herlan J., Hill C., Young-Lahiff C., Schopmeyer S. A., et al. (2010). Propagation of the threatened staghorn coral acropora cervicornis: methods to minimize the impacts of fragment collection and maximize production. Coral Reefs 29, 729–735. doi: 10.1007/s00338-010-0621-6
Liu G., Strong A. E., Skirving W. J., Arzayus L. F. (2006). Overview of NOAA coral reef watch program’s near-real-time satellite global coral bleaching monitoring activities. Proc. 10th Int. Coral Reef Symp. 1793, 1783–1793.
Mbije N. E., Spanier E., Rinkevich B. (2013). A first endeavour in restoring denuded, post-bleached reefs in Tanzania. Estuar. Coast. Shelf Sci. 128, 41–51. doi: 10.1016/j.ecss.2013.04.021
McClanahan T. R. (1989). Kenyan Coral reef-associated gastropod fauna: a comparison between protected and unprotected reefs. Mar. Ecol. Prog. Ser. 53, 11–20. doi: 10.3354/meps053011
McClanahan T. R. (2008). Response of the coral reef benthos and herbivory to fishery closure management and the 1998 ENSO disturbance. Oecologia 155, 169–177. doi: 10.1007/s00442-007-0890-0
McClanahan T. R. (2014). Recovery of functional groups and trophic relationships in tropical fisheries closures. Mar. Ecol. Prog. Ser. 497, 13–23. doi: 10.3354/meps10605
McClanahan T. R., Ateweberhan M., Darling E. S., Graham N. A. J., Muthiga N. A. (2014). Biogeography and change among regional coral communities across the western Indian ocean. PloS One 9, 1–9. doi: 10.1371/journal.pone.0093385
McClanahan T. R., Hicks C. C., Darling E. S. (2008). Malthusian Overfishing and efforts to overcome it on Kenyan coral reefs. Ecol. Appl. 18, 1516–1529. doi: 10.1890/07-0876.1
McClanahan T. R., Muthiga N. A., Kamukuru A. T., Machano H., Kiambo R. W. (1999). The effects of marine parks and fishing on coral reefs of northern Tanzania. Biol. Conserv. 89, 161–182. doi: 10.1016/S0006-3207(98)00123-2
McClanahan T. R., Muthiga N. A., Mangi S. C. (2001). Coral and algal changes after the 1998 coral bleaching: Interaction with reef management and herbivores on Kenyan reefs. Coral Reefs 19, 380–391. doi: 10.1007/s003380000133
McDonald T., Gann G. D., Jonson J., Dixon K. W. (2016). International standards for the practice of ecological restoration – including principles and key concepts (Washington D.C: Society for Ecological Restoration). Available at: http://c.ymcdn.com/sites/www.ser.org/resource/resmgr/docs/SER_International_Standards.pdf.
Mcleod I. M., Newlands M., Hein M. Y., Boström-Einarsson L., Banaszak A. T., Grimsditch G., et al. (2019). Mapping current and future priorities for coral restoration and adaptation programs. Interim Rep. (North Queensland, Australia: James Cook University), 44.
Montoya-Maya P. H., Smit K. P., Burt A. J., Frias-Torres S. (2016). Large-Scale coral reef restoration could assist natural recovery: A case study in Seychelles, Indian ocean. Nat. Conserv. 16, 1–17. doi: 10.3897/natureconservation.16.8604
Morais R. A., Bellwood D. R. (2020). Principles for estimating fish productivity on coral reefs. Coral Reefs 39, 1221–1231. doi: 10.1007/s00338-020-01969-9
NASEM (2018). A research review of interventions to increase the persistence and resilience of coral reefs (Washington, DC: The National Academies Press). doi: 10.17226/25279
Nedimyer K., Gaines K., Roach S. (2011). Coral tree nursery: An innovative approach to growing corals in an ocean-based field nursery. AACL Bioflux 4, 442–446.
Neudecker S. (1979). Effect of grazing and browsing fishes on the zonation of corals in Guam. Ecology 60, 666–672. doi: 10.2307/1936602
NOAA, C. R. W. (2022) NOAA Coral reef watch version 2.16 daily near-Real-Time global 5-km satellite coral bleaching monitoring products. oct. 20, 2019 - mar. 20, 2020 (Maryland: USA NOAA Coral Reef Watch) (Accessed 2022-02-24).
Norström A. V., Nyström M., Lokrantz J., Folke C. S. (2009). Alternative states on coral reefs: beyond coral – macroalgal phase shifts. Mar. Ecol. Prog. Ser. 376, 295–306. doi: 10.3354/meps07815
Nyström M., Graham N. A. J., Lokrantz J., Norström A. V. (2008). Capturing the cornerstones of coral reef resilience: linking theory to practice. Coral Reefs 27, 795–809. doi: 10.1007/s00338-008-0426-z
Obura D., Gudka M., Samoilys M., Osuka K., Mbugua J., Keith D. A., et al. (2022). Vulnerability to collapse of coral reef ecosystems in the Western Indian ocean. Nat. Sustain. 5, 104–113. doi: 10.1038/s41893-021-00817-0
Obura D., Tamelander J., Linden O. (2008). Coastal oceans research and development in the Indian ocean: Status report 2008. CORDIO (Mombasa, Kenya: Coastal Oceans Research and Development in the Indian Ocean).
Oksanen J., Simpson G., Blanchet F., Kindt R., Legendre P., Minchin P. (2022). Vegan: community ecology package. r package version 2.6-2.
Omori M. (2019). Coral restoration research and technical developments: What we have learned so far. Mar. Biol. Res. 15, 377–409. doi: 10.1080/17451000.2019.1662050
Pastorok R. A., Bilyard G. R. (1985). Effects of sewage pollution on coral-reef communities. Mar. Ecol. Prog. Ser. 21, 175–189.
Pawlik J. R., Loh T., Mcmurray S. E. (2018). A review of bottom-up vs . top-down control of sponges on Caribbean fore-reefs ;: what ‘ s old , what ‘ s new , and future directions. PeerJ 6, 1–28. doi: 10.7717/peerj.4343
Paxton A. B., Shertzer K. W., Bacheler N. M., Kellison G. T., Riley K. L., Taylor J. C. (2020). Meta-analysis reveals artificial reefs can be effective tools for fish community enhancement but are not one-size-fits-all. Front. Mar. Sci. 7. doi: 10.3389/fmars.2020.00282
Pickering H., Whitmarsh D. (1997). Artificial reefs and fisheries exploitation: A review of the “attraction versus production” debate, the influence of design and its significance for policy. Fish. Res. 31, 39–59. doi: 10.1016/S0165-7836(97)00019-2
Pratchett M. S., Caballes C. F., Wilmes J. C., Matthews S., Mellin C., Sweatman H. P. A., et al. (2017). Thirty years of research on crown-of-thorns starfish, (1986-2016): scientific advances and emerging opportunities. Diversity 9, 41. doi: 10.3390/d9040041
Pratchett M. S., Hoey A. S., Wilson S. K. (2014). Reef degradation and the loss of critical ecosystem goods and services provided by coral reef fishes. Curr. Opin. Environ. Sustain. 7, 37–43. doi: 10.1016/j.cosust.2013.11.022
R Core Team (2020) R: A language and environment for statistical computing. Available at: https://www.r-project.org/.
Rice M. M., Ezzat L., Burkepile D. E. (2019). Corallivory in the anthropocene: interactive effects of anthropogenic stressors and corallivory on coral reefs. Front. Mar. Sci. 5. doi: 10.3389/fmars.2018.00525
Rinkevich B. (1995). Restoration strategies for coral reefs damaged by recreational activities: the use of sexual and asexual recruits. Restor. Ecol. 3, 241–251. doi: 10.1111/j.1526-100X.1995.tb00091.x
Rinkevich B. (2019). The active reef restoration toolbox is a vehicle for coral resilience and adaptation in a changing world. J. Mar. Sci. Eng. 7, 201. doi: 10.3390/jmse7070201
Rotjan R. D., Lewis S. M. (2008). Impact of coral predators on tropical reefs. Mar. Ecol. Prog. Ser. 367, 73–91. doi: 10.3354/meps07531
Sammarco P. W., Coll J. C., La Barre S. (1985). Competitive strategies of soft corals (Coelenterata: Octocorallia). II. variable defensive responses and susceptibility to scleractinian corals. J. Exp. Mar. Bio. Ecol. 91, 199–215. doi: 10.1016/0022-0981(85)90176-5
Samoilys M. A., Osuka K., Maina G. W., Obura D. O. (2017). Artisanal fisheries on kenya’s coral reefs: Decadal trends reveal management needs. Fish. Res. 186, 177–191. doi: 10.1016/j.fishres.2016.07.025
Seebauer J. (2001). Zoology of porites cylindrica: Potential for use in reef-rehabilitation transplantation efforts. Geneseo J. Sci. Math. 2, 26–34.
Seraphim M. J., Sloman K. A., Alexander M. E., Janetski N., Jompa J., Ambo-Rappe R., et al. (2020). Interactions between coral restoration and fish assemblages: implications for reef management. J. Fish Biol. 97 (3), 1–23. doi: 10.1111/jfb.14440
Shantz A. A., Burkepile D. E. (2014). Context-dependent effects of nutrient loading on the coral-algal mutualism. Ecology 95, 1995–2005. doi: 10.1890/13-1407.1
Shantz A. A., Ladd M. C., Schrack E., Burkepile D. E. (2015). Fish-derived nutrient hotspots shape coral reef benthic communities. Ecol. Appl. 25, 2142–2152. doi: 10.1890/14-2209.1
Shaver E. C., Renzi J. J., Bucher M. G., Silliman B. R. (2020). Relationships between a common Caribbean corallivorous snail and protected area status, coral cover, and predator abundance. Sci. Rep. 10, 1–10. doi: 10.1038/s41598-020-73568-1
Shaver E. C., Silliman B. R. (2017). Time to cash in on positive interactions for coral restoration. PeerJ 5, e3499. doi: 10.7717/peerj.3499
Stobart B., Teleki K., Buckley R., Downing N., Callow M. (2005). Coral recovery at aldabra atoll, Seychelles: Five years after the 1998 bleaching event. Philos. Trans. R. Soc A Math. Phys. Eng. Sci. 363, 251–255. doi: 10.1098/rsta.2004.1490
Suding K., Higgs E., Palmer M. A., Callicott J. B., Anderson C. B., Baker M., et al. (2015). Committing to ecological restoration. Sci. (80-. ). 348, 638–640. doi: 10.1126/science.aaa4216
Tamelander J., Visram S., Obura D. (2000). Prospects for coral recovery through transplantation and natural recruitment, Kenya. Proc. 9th Int. Coral Reef Sym Bali Indones. 2, 23–27.
Thanner S. E., McIntosh T. L., Blair S. M. (2006). Development of benthic and fish assemblages on artificial reef materials compared to adjacent natural reef assemblages in Miami-Dade county, Florida. Bull. Mar. Sci. 78, 57–70.
van Oppen M. J. H., Gates R. D., Blackall L. L., Cantin N., Chakravarti L. J., Chan W. Y., et al. (2017). Shifting paradigms in restoration of the world’s coral reefs. Glob. Change Biol. 23, 3437–3448. doi: 10.1111/gcb.13647
Vaughan D. E. (2021). Active coral restoration: Techniques for a changing planet. 1st ed (Florida: J. Ross Publishing).
Visram S., Mwaura J., Obura D. (2009). Assessing coral community recovery from coral bleaching by recruitment in two reserves in Kenya. West. Indian Ocean J. Mar. Sci. 6, 199–205. doi: 10.4314/wiojms.v6i2.48241
Keywords: Acropora, coral gardening, coral predation, coral recruitment, fish community, keystone invertebrates, long-term ecological monitoring, structural complexity
Citation: Knoester EG, Rienstra JJ, Schürmann QJF, Wolma AE, Murk AJ and Osinga R (2023) Community-managed coral reef restoration in southern Kenya initiates reef recovery using various artificial reef designs. Front. Mar. Sci. 10:1152106. doi: 10.3389/fmars.2023.1152106
Received: 27 January 2023; Accepted: 27 March 2023;
Published: 06 April 2023.
Edited by:
Ibon Galparsoro, Technological Center Expert in Marine and Food Innovation (AZTI), SpainReviewed by:
Lisa Boström Einarsson, Lancaster University, United KingdomAmilcar Leví Cupul-Magaña, University of Guadalajara, Mexico
Copyright © 2023 Knoester, Rienstra, Schürmann, Wolma, Murk and Osinga. This is an open-access article distributed under the terms of the Creative Commons Attribution License (CC BY). The use, distribution or reproduction in other forums is permitted, provided the original author(s) and the copyright owner(s) are credited and that the original publication in this journal is cited, in accordance with accepted academic practice. No use, distribution or reproduction is permitted which does not comply with these terms.
*Correspondence: EG. Knoester, ZXdvdXQua25vZXN0ZXJAd3VyLm5s