- 1Institut für Geowissenschaften, Christian-Albrechts-Universität zu Kiel, Kiel, Germany
- 2Palaeobiology Research Group, School of Earth Sciences, University of Bristol, Bristol, United Kingdom
- 3Chemical and Physical Sciences Department, University of Toronto Mississauga, Mississauga, ON, Canada
- 4GEOMAR Helmholtz-Zentrum für Ozeanforschung Kiel, Kiel, Germany
- 5Centre d’Estudis Avançats de Blanes (CEAB, CSIC), Girona, Spain
- 6Instituto de Acuicultura de Torre de la Sal (IATS, CSIC), Castelló, Spain
Coralline algae play important ecological roles throughout the photic zone of the world’s oceans. Recent studies have shown that attached-living coralline algae can contain records of past climate variability. So far, algal-based paleo-reconstructions are mainly available from mid- to high-latitudes, while in low latitude and temperate regions only few examples exist. Here, we investigate samples from the attached-living encrusting coralline algal species Neogoniolithon hauckii (Rothpletz) R.A Townsend & Huisman [= Neogoniolithon mamillosum (Hauck) Setchell & L.R. Mason, nom illeg.] from a temperate site in the Mediterranean Sea to assess its potential as an environmental recorder. The specimens were collected at different water depths (20 and 40 m) in the Columbretes Islands (Spain). Sclerochronological analysis of sectioned samples revealed seasonal growth patterns. Mg/Ca, Li/Ca, and Ba/Ca ratios were measured in the algal skeletons using laser ablation inductively coupled mass spectrometry (LA-ICP-MS) in ultra-high resolution. We report a mean vertical extension rate of 1.1 to 1.2 mm/year (based on analysis of Mg/Ca cycles in 40 m and 20 m samples), representing the first growth rate measurement for this species. In addition, subannual banding patterns were mapped, measured, and could be linked to high frequency variability in laser-analyzed Mg/Ca ratios. Elemental ratios analyzed in Neogoniolithon hauckii were compared to in situ water temperatures measured at the water depth of sample collection. Our results show significant positive relationships between algal Mg/Ca (R=0.55) as well as Li/Ca ratios (R=0.46) and in situ measured temperature data (40 m specimen). Ba/Ca ratios show no significant correlation to temperature and may be influenced by other factors. These data suggest potential of this species for climate reconstructions in warm-temperate regions as Neogoniolithon hauckii is not only widely distributed in the Mediterranean, but also one of the few species that may be used as a temperature archive for mesophotic coralligenous assemblages that are strongly affected by the recent anthropogenic temperature rise. Further calibration studies are needed to test the element-temperature relationships on samples with longer growth records and in different settings and water depths.
1 Introduction
Coralline algae (Corallinophycidae, Rhodophyta) can be found worldwide in coastal ecosystems from the intertidal to mesophotic zones (Adey and Macintyre, 1973). Encrusting coralline algae build important settlement substrates for invertebrates and act as substrate binders from tropical oceans to the Arctic (Adey and Macintyre, 1973; Steneck, 1983; Steneck, 1986; Tebben et al., 2015) and are key ecosystem engineers (Schubert et al., 2020). They form rhodolith beds (free-living forms of coralline algae) in warm-temperate to Arctic regions (Foster, 2001; Hetzinger et al., 2006; Halfar et al., 2012; Teichert et al., 2014) and play an important role in coral reef growth by stabilizing and cementing reef structures in tropical oceans in modern times and on geological time scales (Kiessling, 2009; Rindi et al., 2019; Teichert et al., 2020). Coralline algae precipitate CaCO3 within their cell walls (Adey et al., 2013) and are thus major carbonate producers in marine ecosystems (Canals and Ballesteros, 1997). Due to their abundance in all oceans, they are an important part of the oceanic carbon cycle (Perry et al., 2008). Corallines are well represented in the fossil record, for example in the Mediterranean region, where they have occurred for at least 140 Myr (Rindi et al., 2019), and more than 60 species of corallines have been reported from this region alone (Coll et al., 2010; Rindi et al., 2019).
Similar to tree rings and bivalve mollusks (Cook et al., 2004; Mette et al., 2016), many species of coralline algae form seasonal banding patterns during growth (Halfar et al., 2000; Kamenos et al., 2008; Halfar et al., 2011a; Williams et al., 2011). However, whether the observed growth patterns in the high-Mg calcite skeletons form annually or subannually is not clear in all species. For example, in the crustose coralline algae Clathromorphum sp., the calcification rate declines during seasonal decreases in temperature and light in winter periods. This translates to changes of skeletal anatomy, where a shift from narrow and elongate cells with dense walls takes place to wider and shorter cells with thinner walls, resulting in growth increment demarcations in the fall to early winter (Adey et al., 2013). These incremental growth patterns allow a clear discrimination of individual growth years. In some specimens of Clathromorphum sp. the yearly layering of sporangial conceptacles, i.e. cavities in which the reproductive structures develop, form in fall and early winter (Adey, 1966) and can provide an additional secondary marker for annual growth. In situ field monitoring experiments (Halfar et al., 2008) have confirmed that the attached-living genus Clathromorphum forms annual banding patterns with fastest growth during summer and reduced growth during fall and winter. This is in line with physiological considerations, which suggest that calcification and growth may slow down dramatically at reduced light intensities and temperatures (Adey, 1970). However, in some coralline algal species subseasonal growth banding patterns can make the identification of seasonal growth cycles difficult. In free-living (non-attached rhodoliths) coralline algae the episodical movement by waves, currents (Marrack, 1999), and grazing animals may lead to differences in growth rates depending on the orientation. As rhodoliths are often found on soft substrate, the bottom side of individual rhodoliths may be episodically buried in the sediment. This may influence the growth patterns in different parts of a specimen, even leading to a halt of growth in some area, while growth continues in another, potentially complicating the sclerochronological interpretation (Sletten et al., 2017b).
Coralline algae also incorporate changes in their ambient environment into the geochemistry of their skeletons (Chave and Wheeler, 1965; Moberly, 1968; Williams et al., 2011; Chan et al., 2017). Seasonality seen in trace elements in algal skeletal calcite can help to better identify annual growth banding patterns. Field calibration experiments have confirmed that algal geochemistry provides a reliable indication of ocean temperatures (Halfar et al., 2008). Comparisons between algal proxies and measured surface ocean data have shown that algal Mg/Ca ratios are temperature-dependent (Kamenos et al., 2008; Nash and Adey, 2017; Hetzinger et al., 2018). The substitution of magnesium in calcite is endothermic, favored at higher temperatures, and builds the basis for magnesium paleothermometry in different marine organisms (e.g. Lea, 2003). The direct matching of highly-resolved Mg/Ca time series measured in skeletal calcite along transects parallel to the direction of algal growth, visible in high-resolution microscope photomosaic images, can provide a valuable tool to distinguish subseasonal cyclicity from seasonal cycles and is routinely used as a method to calibrate and refine age models (e.g. Hetzinger et al., 2019). Due to the well-developed regular annual growth banding and the potential of long-lived specimens, crustose coralline algae have been established as biogenic climate archives in recent years (Svendsen et al., 2014; Hetzinger et al., 2021; Siebert et al., 2021). A number of studies have examined various aspects of the chemical and physical structure of attached-living coralline algae in order to reconstruct past changes in large-scale climate variability (Halfar et al., 2007; Gamboa et al., 2010; Williams et al., 2014; Williams et al., 2018a; Williams et al., 2018b; Leclerc et al., 2022).
Even though coralline algae are distributed worldwide the majority of algal-based paleo-reconstructions are based on cold-water species from mid- to high-latitudes, such as Clathromorphum sp. and Lithothamnion glaciale. So far, the longest algal-based climate records have been developed from species that live attached to rocky substrate and form thick crusts with mound-like structures [e.g. Clathromorphum compactum, up to 650 years of continuous growth (Halfar et al., 2013)]. These records from high latitudes stem from colder waters using specimens with relatively slow growth rates (Hetzinger et al., 2019).
Tropical and subtropical coralline algal species have been less used as potential archives of climate and environmental variability [e.g. Lithothamnion spp. (Halfar et al., 2000; Sletten et al., 2017a); Lithophyllum spp. (Caragnano et al., 2014; Nash et al., 2016; Caragnano et al., 2017); Sporolithon durum (Darrenougue et al., 2014; Darrenougue et al., 2018)], mainly due to a lack of long-lived species. Not all coralline algal genera have the potential to be used as climate archives if their vertical growth is limited [e.g. Phymatolithon (Adey et al., 2018)]. In warmer tropical environments growth forms are more common to expand horizontally with only moderate thickening of the thallus, making the identification of annual growth patterns and the establishment of age chronologies difficult. Additionally, overgrowth by different algal species and by other organisms (e.g. bryozoans) is more commonly seen in tropical environments and crustal extension rates can be up to a magnitude higher than in subarctic coralline algae (e.g. Adey and Vassar, 1975). Crustose coralline algal species from warm-temperate settings have received even less attention as potential paleo-proxy archives for the reconstruction of past climate variability. Nevertheless, free-living growth forms in temperate settings have been used as paleo-sea level indicators (Aguirre et al., 2020) and for paleoecological studies (Basso, 1998; Martinuš et al., 2013).
Finding new coralline algal species potentially suitable for paleoclimate reconstructions is important in order to gain insights into past seasonal-resolution climate variability. As measured instrumental climate data, such as surface ocean temperatures, are not available before the mid-20th century for most ocean regions, it is crucial to be able to reconstruct seasonal variability before the impact of anthropogenic warming on marine ecosystems. In the Mediterranean, coralligenous assemblages and other bioconstructed habitats, like reefs and beds of the coral Cladocora caespitosa, are strongly affected by water temperature increase in recent decades with mass mortalities and temperature-dependent diseases occurring more frequently (Garrabou et al., 2009; Kersting et al., 2013; Hereu and Kersting, 2016; Kersting and Linares, 2019; Garrabou et al., 2022). During the past three decades the Mediterranean Sea has warmed up with a trend of 0.041 ± 0.006°C/year (Pisano et al., 2020).
Here, we have analyzed for the first time encrusting coralline alga Neogoniolithon hauckii (Rothpletz) R.A. Townsend & Huisman (Corallinales: Rhodophyta) samples from a temperate site in the Mediterranean Sea using high-resolution LA-ICP-MS and growth banding analysis. In this study, we assess whether this species has the potential to hold records of past environmental variability. First, we examine seasonal growth patterns and measure annual mean extension rates. Second, Mg/Ca, Li/Ca, and Ba/Ca ratios are analyzed in ultra-high resolution and elemental ratios are compared to in situ water temperatures in order to test their potential as paleotemperature proxies. The selection of N. hauckii for this study is specially suitable as it is a species whose distribution includes most of the Mediterranean Sea (Babbini and Bressan, 1997). Moreover, Neogoniolithon hauckii has a wide depth distribution range, that covers most of the shaded Mediterranean habitats, from 1 to 90 m depth. Although it is occasionally found in the shallows and in maërl beds (Bressan and Babbini, 2003), it is more abundant in coralligenous outcrops (Feldmann, 1937), where it is a major component of the coralline algal matrix (Ballesteros, 2006), together with Lithophyllum stictiforme and Mesophyllum spp.
2 Materials and methods
2.1 Study site and regional setting
The Columbretes Islands (NW Mediterranean Sea) are located 30 nautical miles off the nearest coast (Castelló, Spain, NW Mediterranean; Figure 1A). A marine reserve encircles the archipelago, covering an area of 5500 hectares. Illa Grossa (39°53.825′N, 0°41.214′E), the largest of the islets in the Columbretes, is a C-shaped, drowned Quaternary volcanic caldera. A detailed description of the geographic area, including environmental and oceanographic features is available in Kersting et al. (2013).
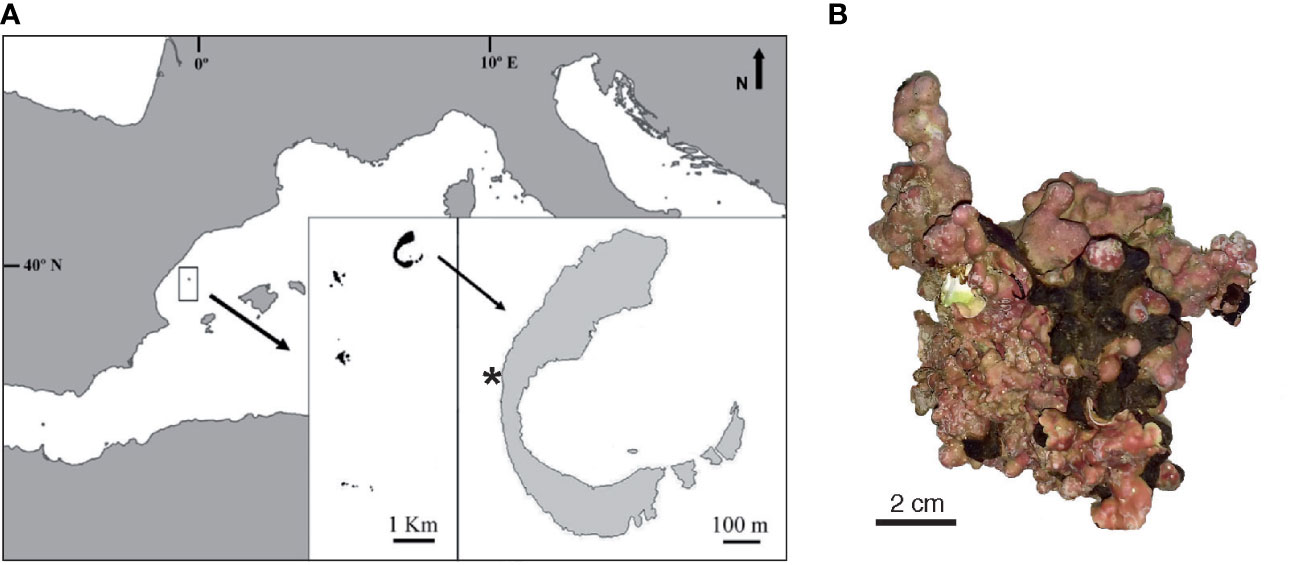
Figure 1 Study site and coralline algal sample. (A) Map of study site Columbretes Islands (Spain) in Mediterranean Sea. Inset shows crescent-shaped Illa Grossa Bay (39°53.825′N, 0°41.214′E), where coralline algae were sampled in October 2017 (by DK) and where water temperature monitoring sensors are installed (*). (B) Attached-living specimen of Neogoniolithon hauckii. Map (A) modified after Kersting et al. (2013).
2.1.1 Temperature measurements
High-resolution (hourly) water temperature data is provided for water depths from 5 to 40 m (in 5 m steps) at the western part of Illa Grossa (Figure 1A) by ONSET HOBO Water Temperature Pro V2 data loggers since 2007. According to the manufacturer the accuracy of the temperature loggers is ±0.2°C. The measured data is available via the international observation network on climate change effects in the Mediterranean marine coastal ecosystems T-MEDnet (https://t-mednet.org). Temperature data downsampled to monthly resolution are shown in Supplementary Figure 1 for water depths 5, 20, and 40 m (data in Supplementary Table 1).
2.1.2 Coralline algae collection
Attached-living crustose coralline algal samples (fruticose Neogoniolithon hauckii, Figure 1B) were collected alive from hard substrate at the western part of Illa Grossa Bay (Figure 1A), exactly where the temperature sensors are located, by SCUBA diving in October 2017 at 20 and 40 m water depth. Identification of the specimens collected agreed with description in Rodriguez-Prieto et al. (2013) under the name Neogoniolithon mamillosum (Hauck) Setchell & L.R.Masson, that according to Algaebase (https://www.algaebase.org/search/species/detail/?species_id=169942) is a heterotypic synonym of N. hauckii. Vouchers have been deposited in HGI Herbarium.
2.2 Sample preparation and LA-ICP-MS analysis
Air-dried coralline specimens were sectioned parallel to the direction of growth using a low speed saw with diamond blades (Figure 2). Thick sections (2 mm) were cut from the slabs. Due to the delicate and fragile skeletal structure of branching growth forms and the tiny size (individual nodules often comprise only few millimeters in length/diameter), it is often difficult to section single branches/nodules exactly parallel to the growth direction. This is further complicated by the mound-like growth structure of individual nodules. In preparation for microscopic scanning, sample surfaces were polished using diamond-polishing suspensions with grit sizes of 9, 3, and 1 μm on a Struers Labopol polishing disk for 45 min per step. High-resolution digital images of the polished surface were produced using an Olympus reflected light microscope (VS-BX) attached to an automated sampling stage/imaging system equipped with the software geo.TS (Olympus Soft Imaging Systems). This setup allows two-dimensional mapping of the surfaces of polished specimens at various magnifications. The resultant high-resolution photomosaics enable the identification and lateral mapping of growth-increment patterns over the entire sample.
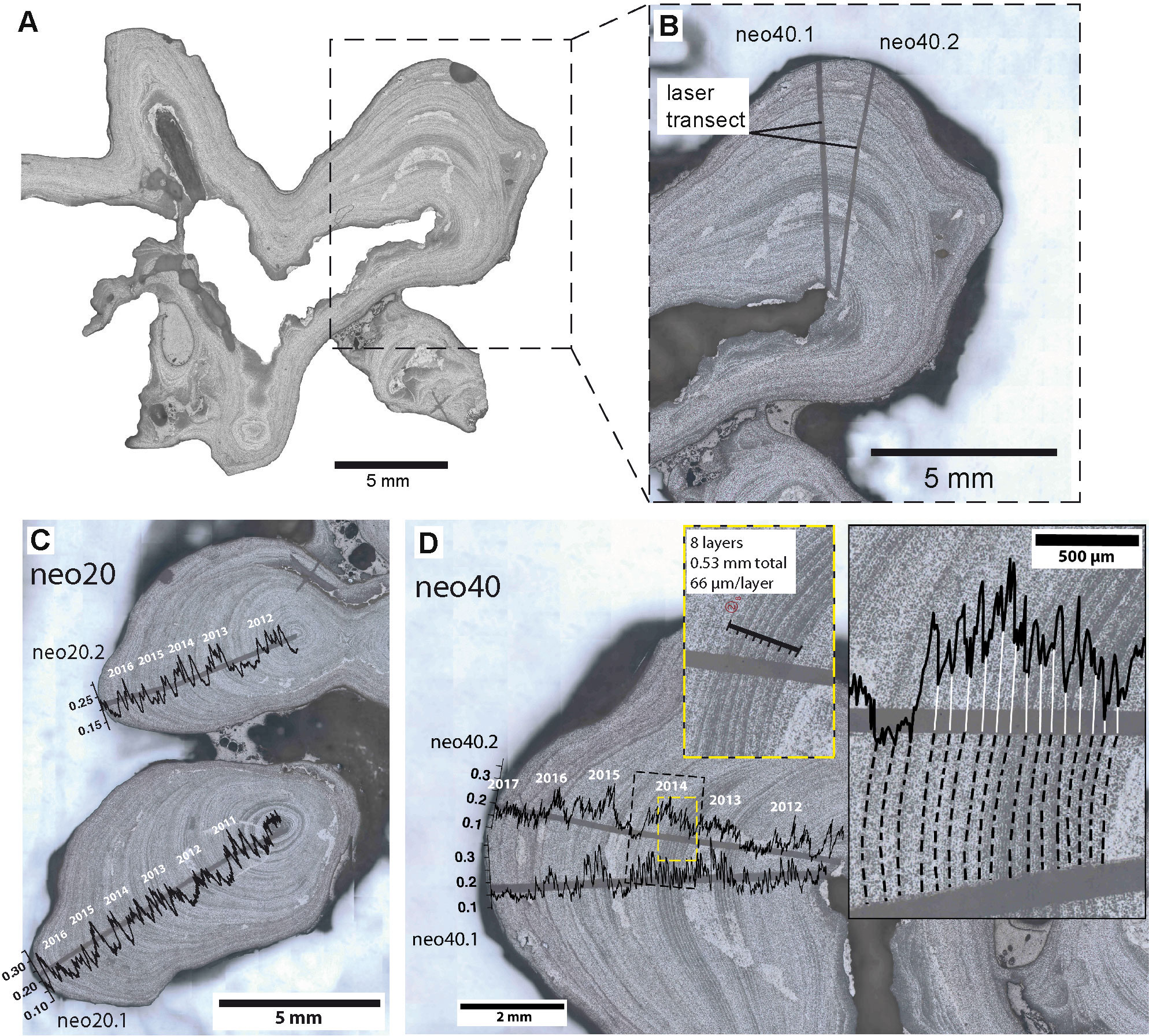
Figure 2 Sectioned and analyzed samples of Neogoniolithon hauckii. (A) Sectioned sample neo40, enlarged inset (B) shows longitudinal section of analyzed protuberance. (B) Magnification of sectioned protuberance with measurement transects after LA-ICP-MS analysis. Samples neo20 (C) and neo40 (D) after LA-ICP-MS analysis. Mg/Ca ratios plotted over measurement transects. Approximate year markers based on Mg/Ca age model (see Supplementary Figure 2) are placed above laser transects in order to illustrate seasonal cycles. (D) Two separate transects on sample neo40. Right inset shows enlarged area marked by stippled line rectangle. Mapped subannual growth lines indicated by stippled lines. White lines link minimum values of subannual cycles in Mg/Ca ratios to mapped subannual banding. Smaller inset (yellow stippled line) shows portion of the annual band 2014 where subannual band thickness was measured directly on photomosaic (8 layers marked with black bold line, total length of 0.53 mm, 66 µm per subannual layer on average, respectively). Mg/Ca ratios in (C, D) in (mol/mol).
Polished thick sections of samples were analyzed by laser ablation inductively coupled plasma - mass spectrometry (ICP-MS) at GEOMAR Helmholtz-Centre for Ocean Research Kiel following methodology described in detail in Hetzinger et al. (2018). Li/Ca, Mg/Ca, and Ba/Ca ratios were measured using a Teledyne Photon Machines Analyte Excite 193nm ArF excimer laser ablation system coupled to a Thermo ElementXR sector field ICP-MS. Individual line transects were analyzed with a scan speed of 5 µm/s (preablation transect with 10 µm/s), a laser spot rectangular mask of 10x155 µm and a 10 Hz pulse rate. Time resolved data were blank subtracted and normalized to Ca using the iolite v3 software and calibrated using repeated analysis of NIST SRM610 and 612 glasses. As the Mg content of the coralline calcite is high and variable, the data were worked up as element/Ca ratios and calibrated with the measured element/Ca ratio of the NIST glasses. Data quality was assessed by analyses of powder pellets of the carbonate reference material JCp-1 during the same session as samples. A pellet of the original JCp-1 reference material powder was used to assess accuracy and values were the same within uncertainties to consensus values determined by solution methods in many laboratories (Hathorne et al., 2013). The exception is Li/Ca for which values are higher for the laser ablation analyses which vaporize and analyze some detrital material in the coral powder as evident in elevated Al contents compared to solution analyses. As the original powder is heterogenous for some trace metals at the small length scales of laser ablation analyses, a nano particulate pellet of the JCp-1 reference material was made (Garbe-Schönberg and Müller, 2014) and used to assess the reproducibility of the analyses. The relative standard deviation (1 sigma) of two 120 s line scans across the JCp-1 nano pellet in the same session and with the same laser conditions as the sample analyses was 41, 10 and 18% for Li/Ca, Mg/Ca and Ba/Ca, respectively. For the 10 point running average which is comparable to the smoothed data shown for the samples, the RSD was 10, 6 and 8% for Li/Ca, Mg/Ca and Ba/Ca, respectively. Detection limits defined as 3.3 times the standard deviation of the gas background intensity during the session were 0.7, 6.7, 96, and 0.04 ug/g for Li, Mg, Ca, and Ba, respectively. Sample concentrations were >>10 times detection limits except for Li which was 2-4 times the detection limit in the samples and mostly above quantification limits.
In order to test and improve the robustness of our data, we analyzed two laser transects on each specimen. We selected two samples (sampled at 20 and 40 m water depth, neo20 and neo40), which show regular banding patterns, for LA-ICP-MS measurements (Figure 2). Laser line transects were placed perpendicular to the growth lines (Figure 2B).
2.3 Age model development
Age models were generated by counting annual growth increments on the mapped and digitized image of the specimens in combination with seasonal algal Mg/Ca cycles. Our samples were collected alive; hence the top layer was assigned to the year of collection. Although yearly growth banding is clearly visible, the samples exhibit 2nd order sub-annual growth cyclicity (Figures 2C, D), which cannot always be clearly separated from annual bands. For example, sample neo20 annual cycles in Mg/Ca ratios were difficult to identify and did not consistently match the banding observed in the microscope image. Thus, the main focus of this study is the analysis of sample neo40, which exhibits clear increments and Mg/Ca ratio cyclicity. Two closely spaced laser line transects on the same protuberance of neo40 allow to test for reproducibility and enhanced matching of cycles in Mg/Ca ratios and growth increments. Sample neo20 is presented as well, although especially in the younger part (growth years 2015 and 2016) the identification of seasonal cycles in Mg/Ca ratios is difficult and cyclicity less clear in both transects (see Supplementary Figure 2, data in Supplementary Table 2), making the presumed age model less reliable than in sample neo40. High Mg values within the skeletons of high-Mg calcite coralline algae are interpreted to correspond to summer periods of growth (Chave and Wheeler, 1965; Halfar et al., 2000). Age models were established based on a combination of the pronounced seasonal cycle in algal Mg/Ca and the annual growth banding. Maximum (minimum) Mg/Ca values were tied to October (April), which is on average the warmest (coolest) month at the study site.
3 Results
3.1 Intra-sample variability
Individual transects on sample neo40, which were analyzed on the same protuberance in close proximity, were cross-matched prior to establishing an age model to check for intra-sample variability and facilitate identification of annual cycles (Figure 2D). For this purpose, a cross-correlation function (ccf) in the software R was used. Time series independent of actual age chronology of both transects on sample neo40 were generated (raw data as well as 10 point running means) and cross-correlations were performed to calculate the best fit with associated lag (Figure 3A).
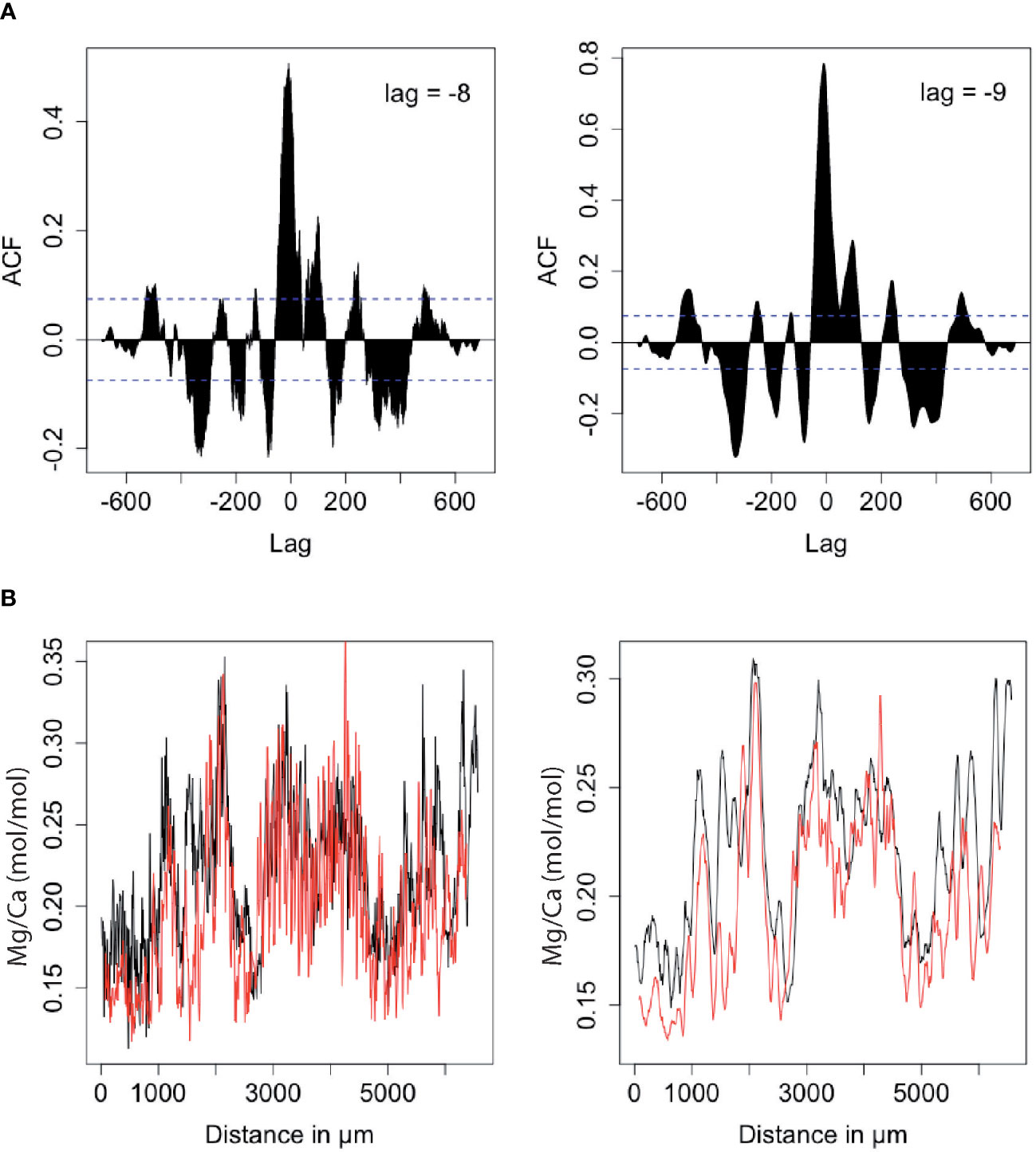
Figure 3 Cross-correlation of Mg/Ca transects (from left to right: 2017-2012). (A) Cross-correlation of Mg/Ca time series neo40.1 and neo40.2; left graph shows full resolution data, right graph 10 point running mean. (B) Best fit of Mg/Ca curve of neo40.2 (black curve) and Mg/Ca curve of neo40.1 (red curve) already corrected for calculated lag; left graph raw data, right graph 10 point running mean. ACF in (A) stands for “auto-correlation function”, which displays coefficients of correlation between time series and its lagged values.
The most dominant cross correlation between neo40.1 and neo40.2 occurs at a lag of -8 for the raw data and -9 for the 10-point-running mean data (Figure 3A). The results of this analysis indicate a significant similarity/overlap of both Mg/Ca ratio transects on sample neo40, when corrected for the calculated lag, and help to identify corresponding maxima and minima (Figure 3B). Mg/Ca, Li/Ca, and Ba/Ca age models for transects samples neo20 and neo40 neo40.1 and neo40.2 transects were created using the time series software AnalySeries (Paillard et al., 1996) to obtain an equidistant proxy time series with a resolution of 12 samples/year (i.e. monthly resolution) and 52 samples/year (i.e. weekly resolution). The developed chronologies were refined and cross-checked for possible errors in the age model by comparing annual extreme values in the Mg/Ca ratios to mapped growth increment patterns for each individual year of algal growth. Sample neo40 displays a record of five years (2012-2017), sample neo20 extends to 2011 (monthly data in Supplementary Table 3).
3.2 Neogoniolithon hauckii annual extension rate
Annual mean extension rates (summer to summer) for the attached-living encrusting specimens of Neogoniolithon hauckii were calculated based on the Mg/Ca ratio age models, where maximum Mg/Ca values were tied to October, representing seasonal maximum temperatures. The distance between maxima was calculated from laser data yielding annual mean extension rates. The mean extension rates are 1.1 mm/year for sample neo40 (transect neo40.1: mean 1.08 mm, minimum 0.83 mm, maximum 1.26 mm per year; transect neo40.2: mean 1.13 mm, minimum 0.97 mm, maximum 1.43 mm per year, Figure 4). In sample neo20 transects were not averaged, since they were measured on separate protuberances (transect neo20.1: mean 1.28 mm, minimum 1.0 mm, maximum 1.42 mm per year; transect neo20.2: mean 1.2 mm, minimum 0.65 mm, maximum 1.74 mm per year). Note that the measured extension rates are based on only four annual cycles (five in transect neo20.1). Extension rates are highly variable in different parts of the specimens (Figure 2A; Supplementary Table 4).
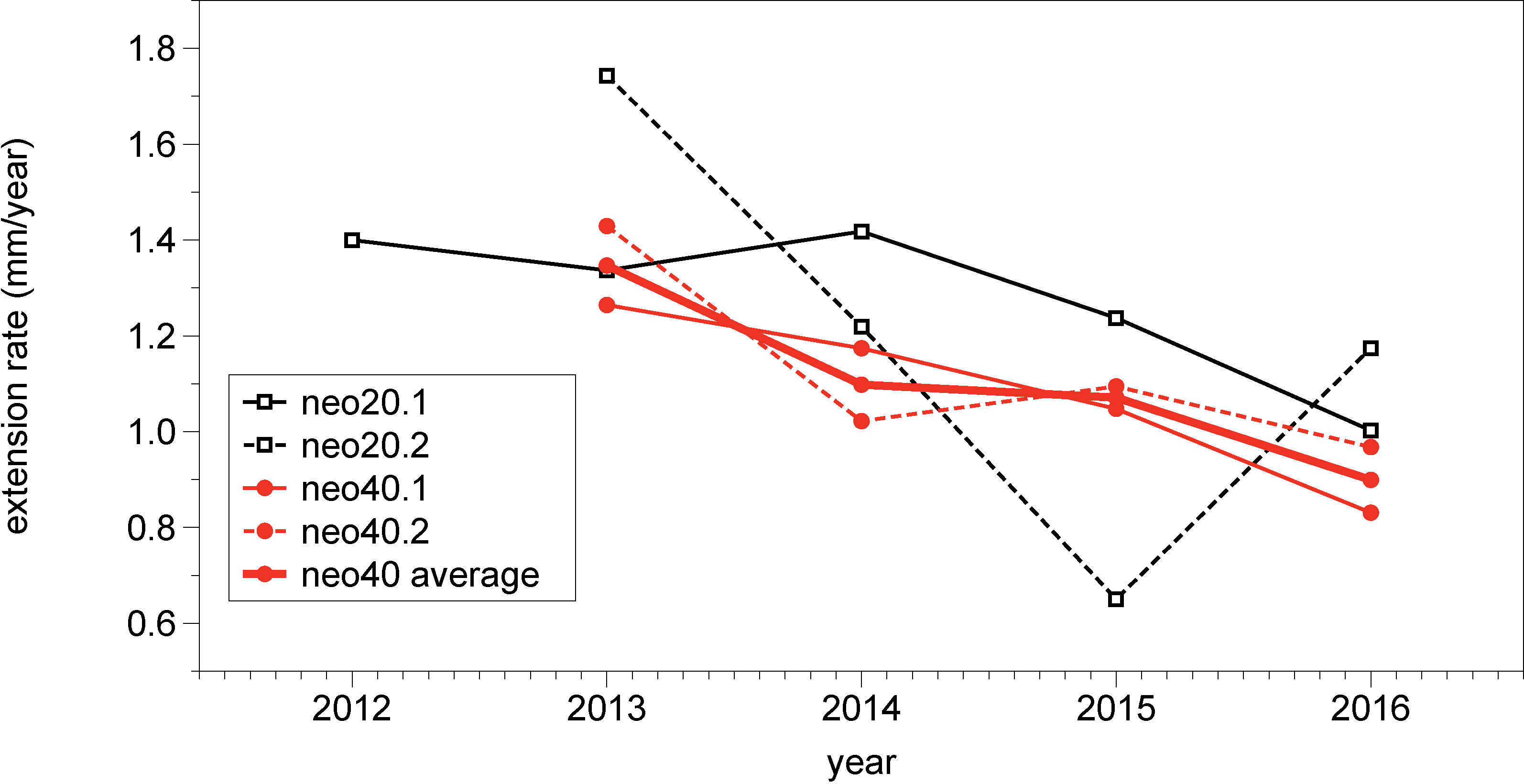
Figure 4 Annual extension rates of samples neo20 (black, open squares) and neo40 (red, filled circles). Sample neo20 - solid line: transect neo20.1; stippled line: transect neo20.2; sample neo40 - solid line: transect neo40.1; stippled line: transect neo40.2, bold line: average of neo40 transects.
3.3 Subannual growth banding
Subannual growth bands were mapped in a portion of sample neo40 in order to study the frequency of banding patterns and measure the banding width (Figure 2D). Approximately thirteen 2nd order bands were successfully linked to cycles in Mg/Ca ratios for the year 2014, indicating that high frequency variability in laser-measured Mg/Ca ratios can be tied to subannual growth patterns. Based on this comparison, an average thickness of 0.066 mm/year was directly measured from eight pronounced subannual growth bands from within one growth year on the microscope photomosaic (see inlets in Figure 2D). However, identification of a complete set of 2nd order subannual banding was only possible in some parts of sample neo40, i.e. in growth years 2014 (Figure 2D) and 2013 (not shown in detail).
3.4 Reproducibility of element/Ca ratio transects
Two laser-ablation transects (Figure 2B) on neo40 were investigated separately for each element/Ca ratio. We found a high reproducibility for Mg/Ca (R=0.62) signals and Li/Ca (R=0.47) when using ordinary least squares (OLS) regression with zero lag (Figure 5; p<0.01). Reproducibility is poor between individual transects for Ba/Ca (R=0.01). Reproducibility for sample neo20 is much lower for Mg/Ca (R=0.34) than in sample neo40, reproducibility is poor for Ba/Ca (R=0.1) and Li/Ca (R=0.1). In a second step, data from both transects on sample neo40 (neo40.1 and neo40.2) were averaged to calculate a sample mean (Figure 5) for each element/Ca ratio, respectively. This decreases noise caused by high intra-sample variability and establishes better comparability (Hetzinger et al., 2018). This step was not undertaken in sample neo20 due to large differences in cycles, especially in years 2015 and 2016 and the poor correlation. Variability between individual transects might also be influenced by the positioning of transects, which have been measured in close proximity to each other (Figure 2B), however, variations between different parts of the sample are expected due to microstructural differences. While transects on sample neo40 were measured in close proximity to each other (Figure 2), transects in sample neo20 were analyzed on different protuberances.
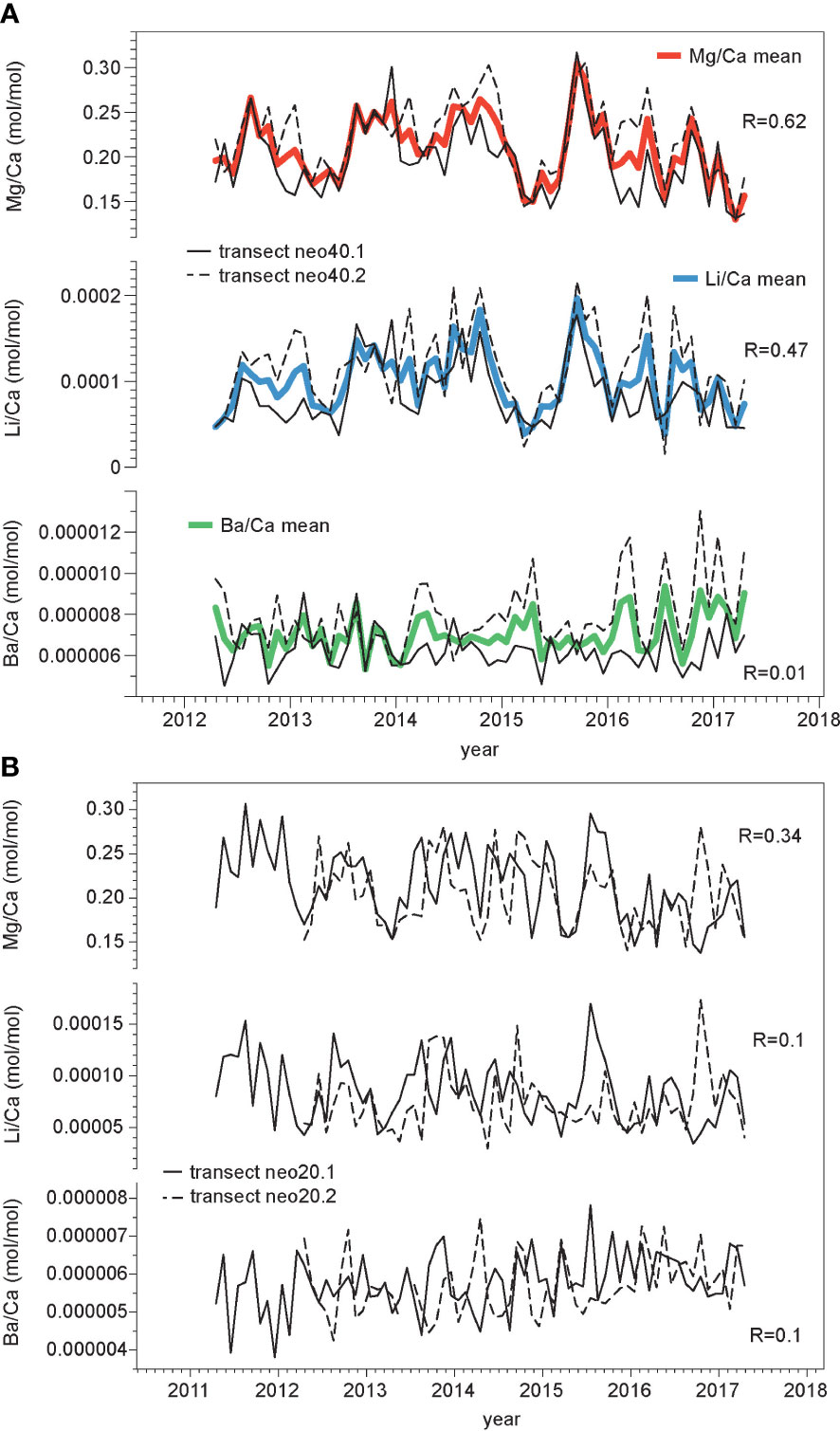
Figure 5 Age models for element/Ca ratios and intraspecimen variability. Changes in the Mg/Ca, Li/Ca, and Ba/Ca ratios (monthly resolution) shown over time; (A) sample neo40 - solid lines: transect neo40.1; stippled lines: transect neo40.2; bold lines: mean of both transects. (B) sample neo20 - solid lines: transect neo20.1; stippled lines: transect neo20.2. Note that due to low correlation and placement of transects on separate protuberances no means were calculated for sample neo20. Correlation coefficients are shown for comparisons between transects for each element/Ca ratio, respectively (p<0.01).
3.5 Comparison between element/Ca ratios
Mg/Ca and Li/Ca ratios display similar variability (Figure 5) and are significantly correlated in both samples (sample neo40: R=0.84, for sample mean; sample neo20: R=0.82 for transect neo20.1, R=0.79 for transect neo20.2; monthly means). Both, Mg/Ca and Li/Ca ratios show a cyclical seasonal pattern (Figure 6) with highest values observed during the Northern Hemisphere summer months, and vice versa. To better illustrate the cyclical variability in Mg/Ca and Li/Ca ratios, higher-resolved weekly data are plotted in comparison to local in situ water temperature (Figure 6). These elemental ratios have been shown to act as temperature proxies in other species of encrusting coralline algae (e.g. Darrenougue et al., 2014). Ba/Ca ratios do not exhibit a similar seasonal variability; Ba/Ca variability stays fairly constant until 2016, thereafter higher values are registered in the sample mean. However, this increase is mainly observed in transect neo40.2 (Figure 5), while Ba/Ca ratios in transect neo40.1 show no deviation. Ba/Ca has previously been used in coralline algae as a proxy for terrestrial input and changes in the upwelling-induced nutrient availability (Chan et al., 2011; Hetzinger et al., 2021). However, as reproducibility between transects is poor, this study concentrates on Mg/Ca and Li/Ca ratios as potential temperature proxies in further analyses.
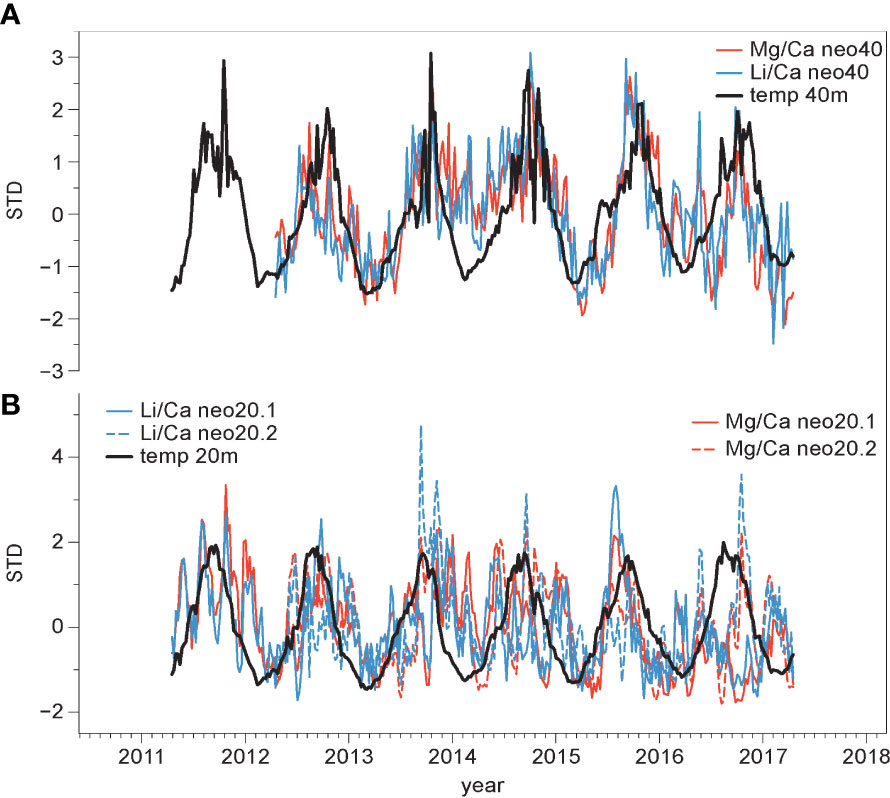
Figure 6 Cyclicity in element/Ca ratios in comparison to temperature (52 samples/annual cycle resolution). Changes in Mg/Ca (red) and Li/Ca (blue) ratios and in situ measured temperature data (black). (A) 40 m water depth, (B) 20 m water depth. Values shown are standardized by removing mean and dividing by standard deviation (STD).
3.6 Relationship to in situ temperature data
In order to study a potential link between the element/Ca ratios and water temperature, element/Ca ratios were tested against in situ temperature (20 and 40 m depth) using OLS regression analysis. For the April 2012 to April 2017 time period, which is covered by the coralline algal records, monthly minimum (maximum) logger-derived temperatures are on average 12.5° (25.8°C) in April (October) at 20 m depth and on average 12.6°C (22.6°C) in April (October) at 40 m. Average annual temperature is 18.1°C at 20 m and 15.9°C at 40 m depth. Note that seasonal maximum temperature peaks may occur 1-2 months earlier at 20 m depth. All monthly element/Ca ratios, except for Ba/Ca, show a significant positive correlation to in situ measured temperature from the study site. Mg/Ca from sample neo40 exhibits the highest correlation with temperature (Figure 7A; R=0.55 for monthly sample mean; R=0.50 for transect neo40.1; R=0.49 for transect neo40.2) followed by Li/Ca (Figure 7B; R=0.46 for monthly sample mean; R=0.47 for transect neo40.1; R=0.34 for transect neo40.2). P-values for all linear regressions are smaller than 0.01. Correlations of Mg/Ca and Li/Ca ratios from sample neo20 to 20 m temperature are lower (Figure 8A, Mg/Ca: R=0.40 for transect neo20.2, R=0.24 for transect neo20.1; Figure 8B, Li/Ca: R=0.33 for transect neo20.2, R=0.29 for transect neo20.1).
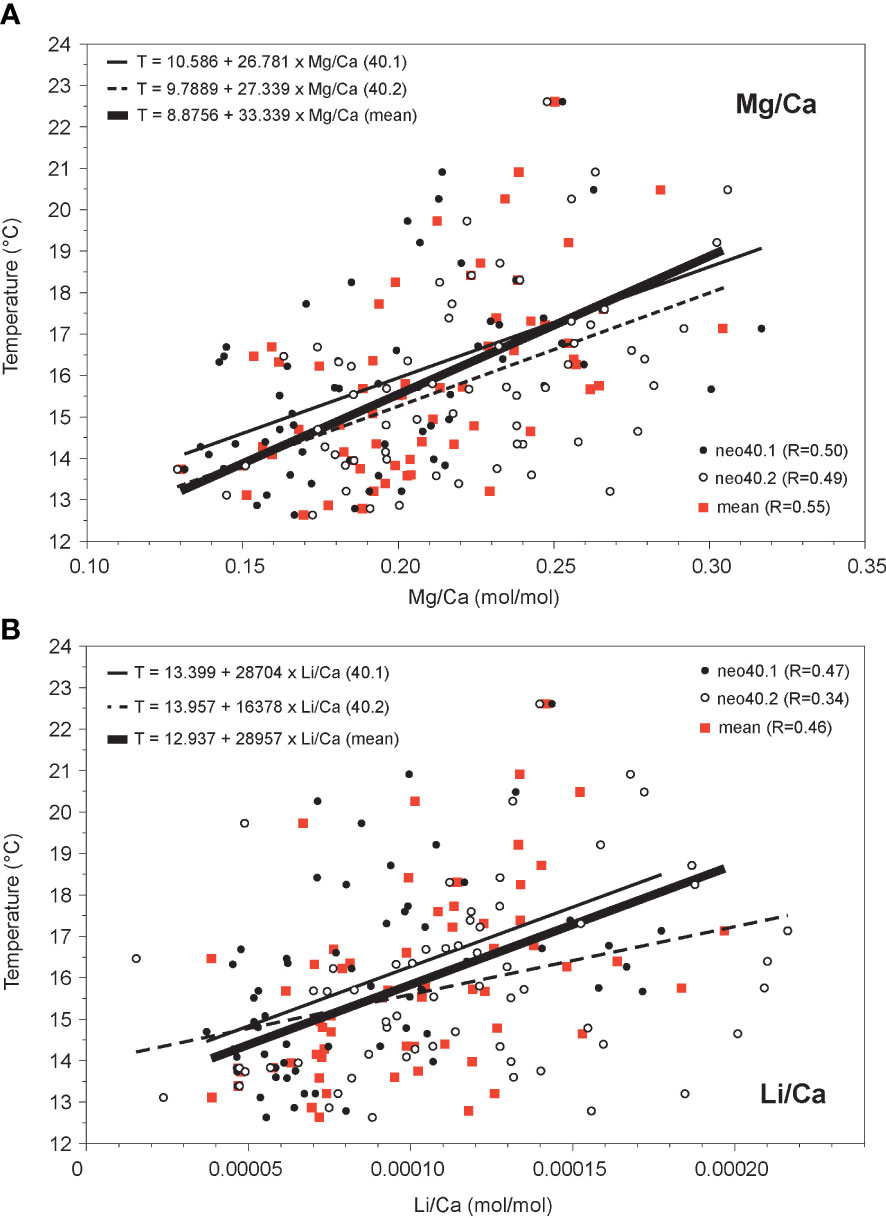
Figure 7 Comparison of (A) Mg/Ca and (B) Li/Ca ratios to in situ temperature (2012-17) for sample neo40. Linear regressions for monthly data are significant (p<0.01), with regression line shown in bold (sample mean), solid (transect neo40.1), and stippled (transect neo40.2). Equations and correlation coefficients (R) are shown for each regression. Both ratios are positively correlated to temperature.
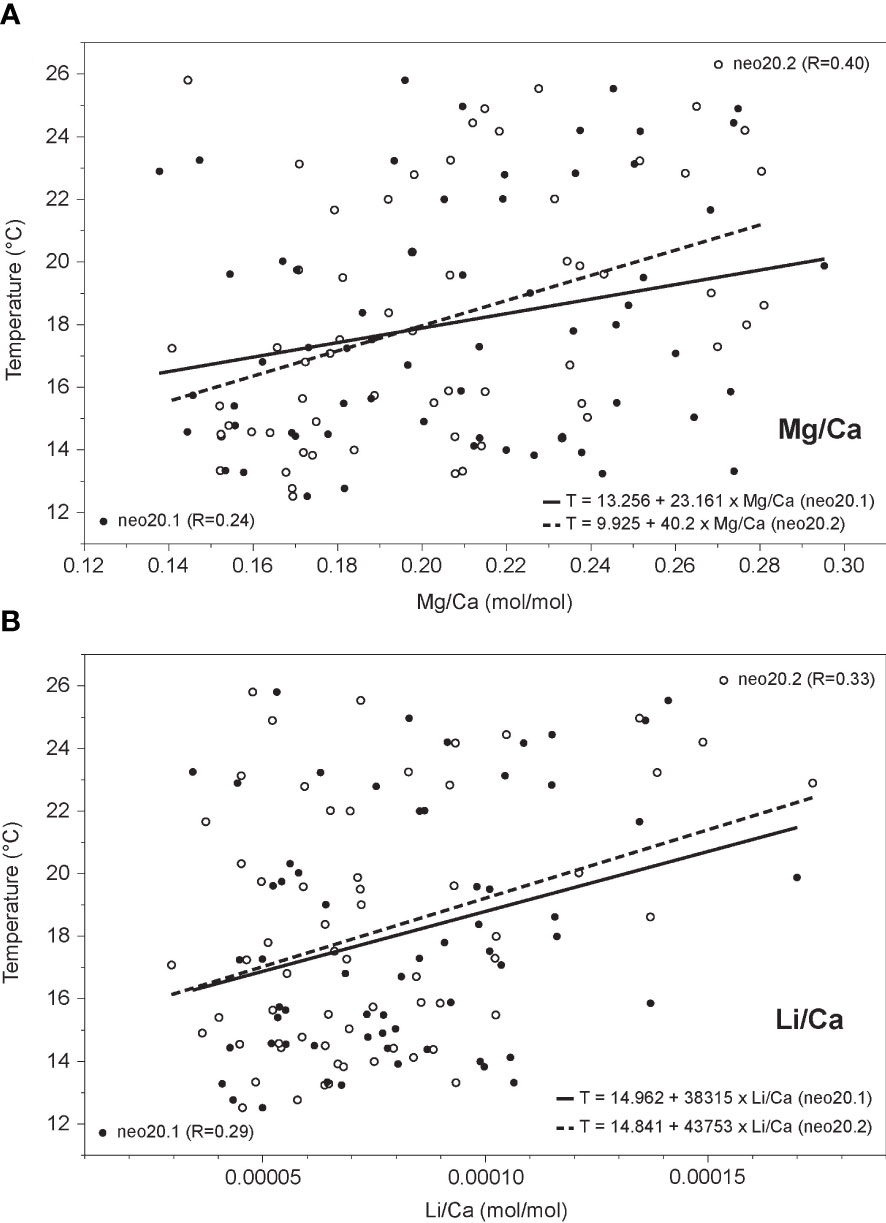
Figure 8 Comparison of (A) Mg/Ca and (B) Li/Ca ratios to in situ temperature (2011-17) for sample neo20. Linear regressions for monthly data are significant (p<0.01), with regression line shown in solid (transect neo20.1) and stippled (transect neo20.2). Equations and correlation coefficients (R) are shown for each regression. Both ratios are positively correlated to temperature.
4 Discussion
A main goal in this study was to assess whether the coralline alga Neogoniolithon hauckii holds any information on past environmental variability in its skeleton. We addressed this question by first making seasonal growth patterns visible using high-resolution photomosaics, and secondly by using ultra-high-resolution laser ablation ICP-MS to analyze trace elemental composition.
In both algal specimens 2nd order subannual cyclicity in growth banding is apparent (Figure 2). This complicates the interpretation of seasonal cycles in growth and elemental ratios. Subannual growth patterns are often difficult to discern from annual banding patterns; also changes in the life history of certain coralline algal species that have been reported from the Mediterranean (Ragazzola et al., 2020). For example, the feasibility of extracting climate records from attached-living encrusting Mediterranean coralline algae was recently tested using different species, such as Lithothamnion minervae, Lithophyllum stictaeforme, and Mesophyllum philippii. Ragazzola et al. (2020) found that L. minervae may be used as a recorder of temperature and its seasonality using Mg/Ca cyclicity, while irregular growth variations were observed when comparing the oldest and youngest parts of a M. philippii specimen, making this species less useful for climate analysis. Hence, it was noted that growth patterns need to be better understood prior to geochemical analysis as they might influence the reliability of certain species as a temperature recorder.
In our specimens, subannual growth banding is clearly visible (Figure 2) and could even be mapped and directly measured in portions of sample neo40 (Figure 2D). In addition to unclear banding patterns on sample neo20, annual cycles in Mg/Ca ratios were more difficult to identify than in sample neo40 and did not consistently match with the banding observed in the microscope image. This was observed for both measured laser transects (Figure 2C) analyzed on separate protuberances of neo20. The poor match, especially in the uppermost years of neo20, among the two adjacent protuberances may reside in different growth rates, lateral pinging-out of layers and/or the occurrence of a hiatus (Schlüter et al., 2021). However, we did not find evidence for a hiatus in sample neo20. In addition, the optimal placement of sectioning angles during sample preparation is a prerequisite for successful age model development in algal specimens, in particular in branching growth morphologies. We speculate that one reason for the deviations in sample neo20 could be that the angle of sectioning may have been chosen in a suboptimal way. The plane of sectioning crosses the inner part of both protuberances (Figure 2C), which is displayed as concentric rings in growth bands. This is not the case in sample neo40, where successful matching between well-defined seasonal cyclicity in Mg/Ca ratios and annual growth patterns was much easier in the microscope photomosaics, although subannual banding was clearly visible, too (Figure 2D). Previous studies on coralline algae have shown secondary growth banding periodicities corresponding to a monthly cycle, e.g. in Porolithon gardineri (Foslie) from Northwestern Hawaii (Agegian, 1981), where a link to lunar periodicity has been suggested. A lunar influence in short-term skeletal density variations has also been seen in hermatypic corals (Buddemeier, 1974). In bivalve mollusk shells a range of periodic growth patterns have been recognized, such as lunar-monthly, fortnightly, solar-daily, lunar-daily, diurnal, and semidiurnal cycles (Hallmann et al., 2009; Schöne and Surge, 2012). In free-living coralline algae (rhodoliths), subannual growth bands have been linked to growth cessations, monthly/lunar rhythms potentially superimposed on annual cycles (Freiwald and Henrich, 1994; Blake and Maggs, 2003), and second order cycles within the annual cycles (Agegian, 1981; Halfar et al., 2000; Sletten et al., 2017a). Hence, subannual banding may limit the potential for long-term climate reconstructions as these may distort or falsify age models.
In future analysis of samples from this species, optimal sample preparation and placement of laser transects will be key to allow a reliable interpretation of algal sclerochronology and geochemistry. Successful paleoclimate reconstructions can only be achieved after proper identification of annual banding patterns to allow the development of accurate age models for geochemical measurements. Eventually, only specimens which have been stained in controlled field experiments and are collected later will deliver reliable growth rates and allow a proper distinction of subannual from annual banding patterns. For example, this was shown for Clathromorphum compactum in a monitoring experiment in the Gulf of Maine using Alizarin-stained samples, which allowed a direct comparison between growth increments, geochemistry and in situ measured water temperatures in ultra-high resolution (Halfar et al., 2008). This study yielded important information on the timing of vertical growth in this species, with highest growth (= calcification) occurring between June and September at this site, when the combined influence of temperature and solar insolation is the highest. Using stained thalli from free-living Lithothamnion glaciale, Kamenos and Law (2010) found an effect of temperature on calcite density, but no consistent relationship with band width. However, similar to our study, they also noted the possible influence of sectioning angles in sample preparation, as individual branches in L. glaciale do not grow in straight lines. Thus, it is impossible to ensure that each growth band is observed parallel to its maximum axis of growth.
Using encrusting Clathromorphum nereostratum samples from Alaska, Halfar et al. (2011b) have shown declining growth rates with increasing water depth as the availability of light decreases with water depth. In the temperate setting of the Columbretes Islands the observed growth rates might be influenced by reduced light intensity at higher water depths (sample collected at 40 m). Neogoniolithon hauckii (as Neogoniolithon mamillosum) has been described to be growing mainly in coralligenous outcrops, where it is a major carbonate framework builder (Ballesteros, 2006). Coralligenous outcrops develop in a wide range of depths across the Mediterranean Sea (20 to 120 m) (Ballesteros, 2006) and the 40 m sample was collected in the normal distribution range for this species in Columbretes Islands (see Kersting et al., 2021). Sea surface temperature at the study site ranges from 29.6°C in summer to 12.0°C in winter (Kersting et al., 2013). However, at 40 m depth, where sample neo40 was collected, water temperatures and seasonal variability are lower, ranging from 22.6°C maximum in summer to 12.6°C minimum in winter. This dampened seasonal cycle at greater depth, when compared to surface temperature seasonality, might potentially influence growth rates, as well as seasonal cyclicity in elemental ratios, which are related to water temperature (in particular Mg/Ca and Li/Ca).
There is no annual growth data available so far for Neogoniolithon sp. We report mean extension rate in specimen neo40 of 1.1 mm/year (two averaged laser measurement transects) and similar growth rates for sample neo20 at lesser depth (transect neo20.1: 1,28 mm/year; transect neo20.2: 1.2 mm/year). Hence, the difference in sampling depth may not have a significant effect on growth rates, albeit more samples are needed to investigate potential differences. The presented annual growth rates fall within the range of published annual extension rate data for other encrusting coralline algal species. Published vertical extension rates of coralline algae have a wide range, for example from around 0.1 mm/year for rhodoliths (Lithothamnion corallioides) from a temperate setting at the Italian coast (Piazza et al., 2022) to mean extension rates measured in rhodoliths from the NW-Indian Ocean (Lithophyllum kotschyanum f. affine, Gulf of Aden, temperatures between 24 and 30°C) of ca. 0.7-1.5 mm/year (Caragnano et al., 2014). Much higher growth rates of 7-20 mm/year were reported for Porolithon gardineri (Foslie) from NW-Hawaii (Agegian, 1981). Growth rates in rhodolith-forming coralline algae can be highly variable over time and in different parts of individual specimens, a large range between approximately 0.015-2.5 mm/year has been reported (Adey and McKibbin, 1970; Adey and Vassar, 1975; Bosence, 1983; Frantz et al., 2000; Halfar et al., 2000; Blake and Maggs, 2003; Kamenos et al., 2008; Schäfer et al., 2011; Darrenougue et al., 2013; Sletten et al., 2017a). When considering the problems in identification of banding patterns and in the differentiation of annual from subannual growth increments, the vastly different growth rates reported in the literature might in some cases be the result of a misidentification of 1st and 2nd order cycles.
In general, previous studies have reported higher annual extension rates in temperate and tropical coralline algae than in samples from colder water temperatures. Slower growth rates (< 1 mm/year) have been observed in encrusting coralline algae in high-latitude settings, where algal growth is limited by low temperatures and available light (e.g. Leclerc et al., 2022). For example, for the long-lived coralline algae C. compactum in the northwestern Atlantic ocean, annual growth rates range between around 100-400 µm/year with decreasing growth rates latitudinally, suggesting a direct relationship to water temperature (Adey et al., 2013). A recent study has suggested that Mg/Ca ratios from massive encrusting growth forms can yield higher correlations to temperature than transects measured on branching morphologies (i.e. individual rhodoliths) of the same species, which often only allow the generation of short uninterrupted time series due to frequent growth irregularities (Williams et al., 2018a).
Here, we report significant positive relationships between ultra-high-resolution Mg/Ca and Li/Ca ratios and water temperature. For the first time, in situ temperature data measured exactly at the same water depth directly at the specimen collection site allowed a temperature calibration of elemental ratios for this temperate algal species (samples were collected in distances of less than 10 meters from the sensors). Results from linear regression indicate that Mg/Ca and Li/Ca are potentially suited as a temperature proxy in the examined coralline samples. Highest correlations are found in sample neo40, correlations are lower for sample neo20, probably due to the difficulties in establishing an age model for this sample as a result of less clear seasonal Mg/Ca cycles (compare Supplementary Figure 2; Figures 7, 8). Mg/Ca shows the best fit, with around 30% of its variance explained by temperature, while 21% of variance of Li/Ca is explained by temperature in sample neo40. Similar to previous studies on other calcifying coralline algal species, we have shown for Neogoniolithon hauckii that Mg incorporation into the skeleton increases with temperature (Moberly, 1968; Kamenos et al., 2008; Williams et al., 2014), yielding similar relationships as shown for other coralline algal species (e.g. Caragnano et al., 2017, their table 2). Significant relationships between temperature and coralline algal Li/Ca and other element/Ca-ratios have been observed in different species (Hetzinger et al., 2011; Caragnano et al., 2014; Darrenougue et al., 2014; Darrenougue et al., 2018). The relationships to temperature may improve when using an intersample average from a larger number of sample specimens in future studies, as has previously been shown for other coralline algal species (Hetzinger et al., 2018). For a more comprehensive study the collection of a larger number of samples from different water depths is a prerequisite that will also allow quality screening to select optimally-grown and longest-lived specimens of N. hauckii for analysis. Ba/Ca ratios may be influenced by other factors, such as the input of sediments from land sources into the coastal ocean (Gillikin et al., 2006), upwelling processes (Lea et al., 1989), or salinity changes (Hetzinger et al., 2013). However, the open-ocean location of Columbretes Islands makes land-based influence very unlikely. Wind-driven upwelling has been observed in the Mediterranean (Bakun and Agostini, 2001), but reproducibility is poor between individual Ba/Ca transects in our sample and the time series is too short for the identification of such events.
5 Conclusion
This study represents the first assessment of Neogoniolithon hauckii as a potential climate archive and may function as a starting point for more detailed analyses. Our results, which suggest that Mg/Ca and Li/Ca are suited as a temperature proxy in the examined species, indicate that N. hauckii may be used as a new temperature archive for mesophotic coralligenous assemblages in the Mediterranean that are strongly affected by the recent anthropogenic temperature rise. However, a better understanding of intra-annual growth patterns is critical, as well as optimal sample preparation, in order to precisely determine periods of growth and calcification in this coralline red algal species. For this task, more samples of this temperate alga from different water depths and environmental settings need to be analyzed in the future.
Data availability statement
The original contributions presented in the study are included in the article/Supplementary Material. Further inquiries can be directed to the corresponding author.
Author contributions
DK conducted the fieldwork, provided sample material and temperature data. EB identified the coralline algal species. JH prepared sample specimens and provided ultra-high-resolution photomosaics. MG, DK, and EH conducted LA-ICP-MS analyses. SH and MG analyzed and interpreted the data and wrote the original draft of the article. All coauthors provided feedback on the manuscript and have read and agreed to the submitted version of the manuscript. All authors contributed to the article.
Funding
JH was supported by a Natural Sciences and Engineering Research Council of Canada Discovery Grant (316003). DK was supported by a Ramon y Cajal postdoctoral grant funded by the Ministry of Science and Innovation (PEICTI 2021- 2023; grant no. RYC2021‐033576‐I) and by the program Beatriu de Pinós funded by the Secretary of Universities and Research (Government of Catalonia) and the Horizon 2020 program of research and innovation of the European Union under the Marie Sklodowska-Curie grant agreement No 801370. This study was partially funded by the Deutsche Forschungsgemeinschaft (DFG, German Research Foundation, project no. 401447620).
Acknowledgments
We thank the Secretaría General de Pesca and the Columbretes Islands Marine Reserve staff for logistic support. We thank two reviewers for their helpful and constructive comments.
Conflict of interest
The authors declare that the research was conducted in the absence of any commercial or financial relationships that could be construed as a potential conflict of interest.
Publisher’s note
All claims expressed in this article are solely those of the authors and do not necessarily represent those of their affiliated organizations, or those of the publisher, the editors and the reviewers. Any product that may be evaluated in this article, or claim that may be made by its manufacturer, is not guaranteed or endorsed by the publisher.
Supplementary material
The Supplementary Material for this article can be found online at: https://www.frontiersin.org/articles/10.3389/fmars.2023.1151592/full#supplementary-material
References
Adey W. H. (1966). Distribution of saxicolous crustose corallines in the northwestern north Atlantic. J. Phycol. 2, 49–54. doi: 10.1111/j.1529-8817.1966.tb04593.x
Adey W. H. (1970). The effects of light and temperature on growth rates in boreal-subarctic crustose corallines. J. Phycol. 6, 269–276. doi: 10.1111/j.1529-8817.1970.tb02392.x
Adey W. H., Halfar J., Williams B. (2013). Biological, physiological and ecological factors controlling high magnesium carbonate formation and producing a precision Arctic/Subarctic marine climate archive: the coralline genus Clathromorphum foslie emend adey. Smithsonian Contributions to Mar. Sci. 40, 1–48. doi: 10.5479/si.1943667X.40.1
Adey W. H., Hernández-Kantún J. J., Gabrielson P. W., Nash M. C., Hayek L. (2018). Phymatolithon (Melobesioideae, hapalidiales) in the Boreal–subarctic transition zone of the north Atlantic: a correlation of plastid DNA markers with morpho-anatomy, ecology, and biogeography. Smithsonian Contributions to Mar. Sci 41, 1–90. doi: 10.5479/si.1943-5667X.5441
Adey W. H., Macintyre I. G. (1973). Crustose coralline algae: a re-evaluation in the geological sciences. Geological Soc. America Bull. 84, 883–904. doi: 10.1130/0016-7606(1973)84<883:CCAARI>2.0.CO;2
Adey W. H., McKibbin D. (1970). Studies on the maerl species Phymatolithon calcareum (Pallas) nov. comb. and Lithothamnium corallioides crouan in the ria de vigo. Botanica Marina 13, 100–106. doi: 10.1515/botm.1970.13.2.100
Adey W. H., Vassar J. M. (1975). Colonization, succession and growth rates of tropical crustose coralline algae (Rhodophyta, cryptonemiales). Phycologia 14 (2), 55–69. doi: 10.2216/i0031-8884-14-2-55.1
Agegian C. R. (1981). Growth of the branched coralline alga, Porolithon gardineri (Foslie) in the Hawaiian archipelago. Proc. Fourth Int. Coral Reef Symposium 2, 420–423.
Aguirre J., Braga J. C., Pujalte V., Orue-Etxebarria X., Salazar-Ortiz E., Rincón-Martínez D., et al. (2020). Middle Eocene rhodoliths from tropical and mid-latitude regions. Diversity 12 (3), 117. doi: 10.3390/d12030117
Babbini L., Bressan G. (1997). Recensement des corallinaceíes de la mer Mediterraneíe et considerations phytogeographiques. Bibliotheca Phycologica 1, 103–121.
Bakun A., Agostini V. N. (2001). Seasonal patterns of wind-induced upwelling/downwelling in the Mediterranean Sea. Scientia Marina 65 (3), 243–257. doi: 10.3989/scimar.2001.65n3243
Ballesteros E. (2006). Mediterranean Coralligenous assemblages: a synthesis of present knowledge. Oceanography Mar. Biol. 44, 123–195. doi: 10.1201/9781420006391.ch4
Basso D. (1998). Deep rhodolith distribution in the pontian islands, Italy: a model for the paleoecology of a temperate sea. Palaeogeography Paleoclimatol. Paleoecol. 137, 173–187. doi: 10.1016/S0031-0182(97)00099-0
Blake C., Maggs C. A. (2003). Comparative growth rates and internal banding periodicity of maerl (Corallinales, rhodophyta) from northern Europe. Phycologia 42 (6), 606–612. doi: 10.2216/i0031-8884-42-6-606.1
Bosence D. W. J. (1983). “The occurrence and ecology of recent rhodoliths- a review,” in Coated grains. Ed. Preyt T. M. (Berlin: Springer-Verlag), 225–241.
Bressan G., Babbini L. (2003). Biodiversità marine delle coste italiane: corallinales del mar mediterraneo. guida alla determinazione. Societá italiana di biologia 10, 237.
Buddemeier R. W. (1974). Environmental controls over annual and lunar monthly cycles in hermatypic coral calcification. Proc. 2nd Int. Coral Reef Symp. 259–267.
Canals M., Ballesteros E. (1997). Production of carbonate particles by phytobenthic communities on the mallorca-menorca shelf, northwestern Mediterranean Sea. Deep Sea Res. Part II: Topical Stud. Oceanography 44 (3), 611–629. doi: 10.1016/S0967-0645(96)00095-1
Caragnano A., Basso D., Jacob D. E., Storz D., Rodondi G., Benzoni F., et al. (2014). The coralline red alga Lithophyllum kotschyanum f. affine as proxy of climate variability in the Yemen coast, gulf of Aden (NW Indian ocean). Geochimica Et Cosmochimica Acta 124 (0), 1–17. doi: 10.1016/j.gca.2013.09.021
Caragnano A., Basso D., Storz D., Jacob D. E., Ragazzola F., Benzoni F., et al. (2017). Elemental variability in the coralline alga Lithophyllum yemenense as an archive of past climate in the gulf of Aden (NW Indian ocean). J. Phycology 53 (2), 381–395. doi: 10.1111/jpy.12509
Chan P., Halfar J., Adey W., Hetzinger S., Zack T., Moore G. W. K., et al. (2017). Multicentennial record of Labrador Sea primary productivity and sea-ice variability archived in coralline algal barium. Nat. Commun. 8, 15543. doi: 10.1038/ncomms15543
Chan P., Halfar J., Williams B., Hetzinger S., Steneck R., Zack T., et al. (2011). Freshening of the Alaska coastal current recorded by coralline algal Ba/Ca ratios. J. Geophysical Res. 116 (G1), G01032. doi: 10.1029/2010JG001548
Chave K. E., Wheeler B. D. Jr (1965). Mineralogic changes during growth in the red alga Clathromorphum compactum. Science 147, 621. doi: 10.1126/science.147.3658.621.a
Coll M., Piroddi C., Steenbeek J., Kaschner K., Ben Rais Lasram F., Aguzzi J., et al. (2010). The biodiversity of the Mediterranean Sea: estimates, patterns, and threats. PloS One 5 (8), e11842. doi: 10.1371/journal.pone.0011842
Cook E. R., Woodhouse C. A., Eakin C. M., Meko D. M., Stahle D. W. (2004). Long-term aridity changes in the Western united states. Science 306 (5698), 1015–1018. doi: 10.1126/science.1102586
Darrenougue N., De Deckker P., Eggins S., Fallon S., Payri C. (2018). A record of mining and industrial activities in new Caledonia based on trace elements in rhodolith-forming coralline red algae. Chem. Geology 493, 24–36. doi: 10.1016/j.chemgeo.2018.05.014
Darrenougue N., De Deckker P., Eggins S., Payri C. (2014). Sea-Surface temperature reconstruction from trace elements variations of tropical coralline red algae. Quaternary Sci. Rev. 93, 34–46. doi: 10.1016/j.quascirev.2014.03.005
Darrenougue N., De Deckker P., Payri C., Eggins S., Fallon S. (2013). Growth and chronology of the rhodolith-forming, coralline red alga Sporolithon durum. Mar. Ecol. Prog. Ser. 474, 105–119. doi: 10.3354/meps10085
Feldmann J. (1937). Recherches sur la végétation marine de la méditerranée. la côte des albères (Rouen: Imprimerie Wolf).
Foster M. S. (2001). Rhodoliths: between rocks and soft places. J. Phycology 37, 659–667. doi: 10.1046/j.1529-8817.2001.00195.x
Frantz B. R., Kashgarian M., Coale K. H., Foster M. S. (2000). Growth rate and potential climate record from a rhodolith using 14C accelerator mass spectrometry. Limnology Oceanography 45 (8), 1773–1777. doi: 10.4319/lo.2000.45.8.1773
Freiwald A., Henrich R. (1994). Reefal coralline algal build-ups within the Arctic circle: morphology and sedimentary dynamics under extreme environmental seasonality. Sedimentology 41, 963–984. doi: 10.1111/j.1365-3091.1994.tb01435.x
Gamboa G., Halfar J., Hetzinger S., Adey W., Zack T., Kunz B., et al. (2010). Mg/Ca ratios in coralline algae record northwest Atlantic temperature variations and north Atlantic oscillation relationships. J. Geophysical Res. 115 (C12), C12044. doi: 10.1029/2010JC006262
Garbe-Schönberg D., Müller S. (2014). Nano-particulate pressed powder tablets for LA-ICP-MS. J. Analytical Atomic Spectrometry 29 (6), 990–1000. doi: 10.1039/C4JA00007B
Garrabou J., Coma R., Bensoussan N., Bally M., Chevaldonné P., Cigliano M., et al. (2009). Mass mortality in northwestern Mediterranean rocky benthic communities: effects of the 2003 heat wave. Global Change Biol. 15 (5), 1090–1103. doi: 10.1111/j.1365-2486.2008.01823.x
Garrabou J., Gómez-Gras D., Medrano A., Cerrano C., Ponti M., Schlegel R., et al. (2022). Marine heatwaves drive recurrent mass mortalities in the Mediterranean Sea. Global Change Biol. 28 (19), 5708–5725. doi: 10.1111/gcb.16301
Gillikin D. P., Dehairs F., Lorrain A., Steenmans D., Baeyens W., André L. (2006). Barium uptake into the shells of the common mussel (Mytilus edulis) and the potential for estuarine paleo-chemistry reconstruction. Geochimica Cosmochimica Acta 70 (2), 395–407. doi: 10.1016/j.gca.2005.09.015
Halfar J., Adey W. H., Kronz A., Hetzinger S., Edinger E., Fitzhugh W. W. (2013). Arctic Sea-ice decline archived by multicentury annual-resolution record from crustose coralline algal proxy. Proc. Natl. Acad. Sci. 110 (49), 19737–19741. doi: 10.1073/pnas.1313775110
Halfar J., Eisele M., Riegl B., Hetzinger S., Godinez-Orta L. (2012). Modern rhodolith-dominated carbonates at punta chivato, Mexico. Geodiversitas 34 (1), 99–113. doi: 10.5252/g2012n1a6
Halfar J., Hetzinger S., Adey W., Zack T., Gamboa G., Kunz B., et al. (2011a). Coralline algal growth-increment widths archive north Atlantic climate variability. Palaeogeography Palaeoclimatol. Palaeoecol. 302 (1-2), 71–80. doi: 10.1016/j.palaeo.2010.04.009
Halfar J., Steneck R. S., Joachimski M., Kronz A., Wanamaker A. D. Jr (2008). Coralline red algae as high-resolution climate recorders. Geology 36 (6), 463–466. doi: 10.1130/G24635A.1
Halfar J., Steneck R., Schöne B. R., Moore G. W. K., Joachimski M. M., Kronz A., et al. (2007). Coralline alga reveals first marine record of subarctic north pacific climate change. Geophysical Res. Lett. 34, L07702. doi: 10.01029/02006GL028811
Halfar J., Williams B., Hetzinger S., Steneck R. S., Lebednik P., Winsborough C., et al. (2011b). 225 years of Bering Sea climate and ecosystem dynamics revealed by coralline algal growth-increment widths. Geology 39 (6), 579–582. doi: 10.1130/G31996.1
Halfar J., Zack T., Kronz A., Zachos J. (2000). Growth and high-resolution paleoenvironmental signals of rhodoliths (coralline red algae): a new biogenic archive. J. Geophysical Res. 105 (C9), 22107–22116. doi: 10.1029/1999JC000128
Hallmann N., Burchell M., Schöne B. R., Irvine G. V., Maxwell D. (2009). High-resolution sclerochronological analysis of the bivalve mollusk saxidomus gigantea from Alaska and British Columbia: techniques for revealing environmental archives and archaeological seasonality. J. Archaeological Sci. 36 (10), 2353–2364. doi: 10.1016/j.jas.2009.06.018
Hathorne E. C., Gagnon A., Felis T., Adkins J., Asami R., Boer W., et al. (2013). Interlaboratory study for coral Sr/Ca and other element/Ca ratio measurements. Geochemistry Geophysics Geosystems 14 (9), 3730–3750. doi: 10.1002/ggge.20230
Hereu B., Kersting D. K. (2016). Diseases of coralline algae in the Mediterranean Sea. Coral Reefs 35 (2), 713–713. doi: 10.1007/s00338-016-1428-x
Hetzinger S., Halfar J., Kronz A., Simon K., Adey W. H., Steneck R. S. (2018). Reproducibility of Clathromorphum compactum coralline algal Mg/Ca ratios and comparison to high-resolution sea surface temperature data. Geochimica Cosmochimica Acta 220, 96–109. doi: 10.1016/j.gca2017.09.044
Hetzinger S., Halfar J., Riegl B., Godinez-Orta L. (2006). Sedimentology and acoustic mapping of modern rhodolith facies on a non-tropical carbonate shelf (Gulf of California, Mexico). J. Sedimentary Res. 76, 670–682. doi: 10.2110/jsr.2006.053
Hetzinger S., Halfar J., Zack T., Gamboa G., Jacob D. E., Kunz B. E., et al. (2011). High-resolution analysis of trace elements in crustose coralline algae from the north Atlantic and north pacific by laser ablation ICP-MS. Palaeogeography Palaeoclimatology Palaeoecol. 302 (1-2), 81–94. doi: 10.1016/j.palaeo.2010.06.004
Hetzinger S., Halfar J., Zack T., Mecking J. V., Kunz B. E., Jacob D. E., et al. (2013). Coralline algal barium as indicator for 20th century northwestern north Atlantic surface ocean freshwater variability. Sci. Rep. 3 (1761). doi: 10.1038/srep01761
Hetzinger S., Halfar J., Zajacz Z., Möller M., Wisshak M. (2021). Late twentieth century increase in northern spitsbergen (Svalbard) glacier-derived runoff tracked by coralline algal Ba/Ca ratios. Climate Dynamics 56 (9), 3295–3303. doi: 10.1007/s00382-021-05642-x
Hetzinger S., Halfar J., Zajacz Z., Wisshak M. (2019). Early start of 20th-century Arctic sea-ice decline recorded in Svalbard coralline algae. Geology 47 (10), 963–967. doi: 10.1130/G46507.1
Kamenos N., Cusack M., Moore P. G. (2008). Coralline algae are global paleothermometers with bi-weekly resolution. Geochimica Cosmochimica Acta 72, 771–779. doi: 10.1016/j.gca.2007.11.019
Kamenos N. A., Law A. (2010). Temperature controls on coralline algal skeletal growth. J. Phycology 46 (2), 331–335. doi: 10.1111/j.1529-8817.2009.00780.x
Kersting D. K., Bensoussan N., Linares C. (2013). Long-term responses of the endemic reef-builder Cladocora caespitosa to Mediterranean warming. PloS One 8 (8), e70820. doi: 10.1371/journal.pone.0070820
Kersting D. K., Linares C. (2019). Living evidence of a fossil survival strategy raises hope for warming-affected corals. Sci. Adv. 5 (10), eaax2950. doi: 10.1126/sciadv.aax2950
Kersting D. K., Mangialajo L., Ballesteros E. (2021). On the edge: segregation and coexistence between epibenthic fish species in Mediterranean mesophotic habitats. Mar. Biol. 168 (10), 155. doi: 10.1007/s00227-021-03955-3
Kiessling W. (2009). Geologic and biologic controls on the evolution of reefs. Annu. Rev. Ecology Evolution Systematics 40 (1), 173–192. doi: 10.1146/annurev.ecolsys.110308.120251
Lea D. W. (2003). “Elemental and isotopic proxies of marine temperatures,” in The oceans and marine geochemistry. Ed. Elderfield H. (Oxford: Elsevier-Pergamon), 365–390.
Lea D., Shen G. T., Boyle E. A. (1989). Coralline barium records temporal variability in equatorial pacific upwelling. Nature 340, 373–376. doi: 10.1038/340373a0
Leclerc N., Halfar J., Hetzinger S., Chan P. T. W., Adey W., Tsay A., et al. (2022). Suitability of the coralline alga Clathromorphum compactum as an Arctic archive for past sea ice cover. Paleoceanogr. Paleoclimatol. 37 (1), e2021PA004286. doi: 10.1029/2021PA004286
Marrack E. C. (1999). The relationship between water motion and living rhodolith beds in the southwestern gulf of California, Mexico. Palaios 14, 159–171. doi: 10.2307/3515371
Martinuš M., Fio K., Pikelj K., Aščić Š. (2013). Middle Miocene warm-temperate carbonates of central paratethys (Mt. zrinska gora, croatia): paleoenvironmental reconstruction based on bryozoans, coralline red algae, foraminifera, and calcareous nannoplankton. Facies 59 (3), 481–504. doi: 10.1007/s10347-012-0327-z
Mette M. J., Wanamaker A. D., Carroll M. L., Ambrose W. G., Retelle M. J. (2016). Linking large-scale climate variability with Arctica islandica shell growth and geochemistry in northern Norway. Limnol. Oceanogr. 61 (2), 748–764. doi: 10.1002/lno.10252
Moberly R. Jr. (1968). Composition of magnesian calcites of algae and pelecypods by electron microprobe analysis. Sedimentology 11, 61–82. doi: 10.1111/j.1365-3091.1968.tb00841.x
Nash M. C., Adey W. (2017). Multiple phases of mg-calcite in crustose coralline algae suggest caution for temperature proxy and ocean acidification assessment: lessons from the ultrastructure and biomineralization in Phymatolithon (Rhodophyta, Corallinales)1. J. Phycology 53 (5), 970–984. doi: 10.1111/jpy.12559
Nash M. C., Martin S., Gattuso J. P. (2016). Mineralogical response of the Mediterranean crustose coralline alga Lithophyllum cabiochae to near-future ocean acidification and warming. Biogeosciences 13 (21), 5937–5945. doi: 10.5194/bg-13-5937-2016
Paillard D., Labeyrie L., Yiou P. (1996). Macintosh program performs time-series analysis. Eos Trans. AGU 77, 379. doi: 10.1029/96EO00259
Perry C. T., Spencer T., Kench P. S. (2008). Carbonate budgets and reef production states: a geomorphic perspective on the ecological phase-shift concept. Coral Reefs 27 (4), 853–866. doi: 10.1007/s00338-008-0418-z
Piazza G., Bracchi V. A., Langone A., Meroni A. N., Basso D. (2022). Growth rate rather than temperature affects the B/Ca ratio in the calcareous red alga Lithothamnion corallioides. Biogeosciences 19, 1047–1065. doi: 10.5194/bg-2021-21
Pisano A., Marullo S., Artale V., Falcini F., Yang C., Leonelli F. E., et al. (2020). New evidence of Mediterranean climate change and variability from Sea surface temperature observations. Remote Sens. 12 (1), 132. doi: 10.3390/rs12010132
Ragazzola F., Caragnano A., Basso D., Schmidt D. N., Fietzke J. (2020). Establishing temperate crustose early Holocene coralline algae as archives for palaeoenvironmental reconstructions of the shallow water habitats of the Mediterranean Sea. Palaeontology 63 (1), 155–170. doi: 10.1111/pala.12447
Rindi F., Braga J. C., Martin S., Peña V., Le Gall L., Caragnano A., et al. (2019). Coralline algae in a changing Mediterranean Sea: how can we predict their future, if we do not know their present? Front. Mar. Sci. 6 (723). doi: 10.3389/fmars.2019.00723
Rodriguez-Prieto C., Ballesteros E., Boisset F., Afonso-Carrillo J. (2013). Guía de las macroalgas y fanerógamas marinas del mediterráneo occidental (Spain: Ediciones OMEGA).
Schäfer P., Fortunato H., Bader B., Liebetrau V., Bauch T., Reijmer J. J. G. (2011). Growth rates and carbonate production by coralline red algae in upwelling and non-upwelling settings along the pacific coast of Panama. PALAIOS 26 (7), 420–432. doi: 10.2110/palo.2010.p10-138r
Schlüter M., Pyko I., Wisshak M., Schulbert C., Teichert S. (2021). Growth interruptions in Arctic rhodoliths correspond to water depth and rhodolith morphology. Minerals 11 (5), 538. doi: 10.3390/min11050538
Schöne B., Surge D. (2012). Treatise Online no. 46: Part N, Revised, Volume 1, Chapter 14: Bivalve sclerochronology and geochemistry. Treatise Online. doi: 10.17161/to.v0i0.4297
Schubert N., Schoenrock K. M., Aguirre J., Kamenos N. A., Silva J., Horta P. A., et al. (2020). Editorial: coralline algae: globally distributed ecosystem engineers. Front. Mar. Sci. 7 (352). doi: 10.3389/fmars.2020.00352
Siebert V., Poitevin P., Chauvaud L., Schöne B. R., Lazure P., Thébault J. (2021). Using growth and geochemical composition of Clathromorphum compactum to track multiscale north Atlantic hydro-climate variability. Palaeogeography Palaeoclimatology Palaeoecol. 562, 110097. doi: 10.1016/j.palaeo.2020.110097
Sletten H. R., Andrus C. F. T., Guzmán H. M., Halfar J. (2017a). Re-evaluation of using rhodolith growth patterns for paleoenvironmental reconstruction: an example from the gulf of Panama. Palaeogeography Palaeoclimatology Palaeoecol. 465, 264–277. doi: 10.1016/j.palaeo.2016.10.038
Sletten H. R., Gillikin D. P., Halfar J., Andrus C. F. T., Guzmán H. M. (2017b). Skeletal growth controls on Mg/Ca and P/Ca ratios in tropical Eastern pacific rhodoliths (coralline red algae). Chem. Geology 465, 1–10. doi: 10.1016/j.chemgeo.2017.05.010
Steneck R. (1983). Escalating herbivory and resulting adaptive trends in cacareous algal crusts. Paleobiology 9, 44–61. doi: 10.1017/S0094837300007375
Steneck R. (1986). The ecology of coralline algal crusts: convergent patterns and adaptive strategies. Ann. Rev. Ecol. Syst. 17, 273–303. doi: 10.1146/annurev.es.17.110186.001421
Svendsen L., Hetzinger S., Keenlyside N., Gao Y. (2014). Marine-based multiproxy reconstruction of Atlantic multidecadal variability. Geophysical Res. Lett. 41. doi: 10.1002/2013gl059076
Tebben J., Motti C. A., Siboni N., Tapiolas D. M., Negri A. P., Schupp P. J., et al. (2015). Chemical mediation of coral larval settlement by crustose coralline algae. Sci. Rep. 5 (1), 10803. doi: 10.1038/srep10803
Teichert S., Steinbauer M., Kiessling W. (2020). A possible link between coral reef success, crustose coralline algae and the evolution of herbivory. Sci. Rep. 10 (1), 17748. doi: 10.1038/s41598-020-73900-9
Teichert S., Woelkerling W., Rüggeberg A., Wisshak M., Piepenburg D., Meyerhöfer M., et al. (2014). Arctic Rhodolith beds and their environmental controls (Spitsbergen, Norway). Facies 60 (1), 15–37. doi: 10.1007/s10347-013-0372-2
Williams B., Halfar J., DeLong K. L., Hetzinger S., Steneck R. S., Jacob D. E. (2014). Multi-specimen and multi-site calibration of Aleutian coralline algal Mg/Ca to sea surface temperature. Geochimica Cosmochimica Acta 139 (0), 190–204. doi: 10.1016/j.gca.2014.04.006
Williams B., Halfar J., Steneck R. S., Wortmann U. G., Hetzinger S., Adey W., et al. (2011). Twentieth century δ13C variability in surface water dissolved inorganic carbon recorded by coralline algae in the northern north pacific ocean and the Bering Sea. Biogeosciences 8, 165–174. doi: 10.5194/bg-8-165-2011
Williams S., Halfar J., Zack T., Hetzinger S., Blicher M., Juul-Pedersen T. (2018a). Comparison of climate signals obtained from encrusting and free-living rhodolith coralline algae. Chem. Geology 476, 418–428. doi: 10.1016/j.chemgeo.2017.11.038
Keywords: coralline algae, LA-ICP-MS, sclerochronology, temperate, Mg/Ca, Li/Ca, climate archive, subannual banding
Citation: Hetzinger S, Grohganz M, Halfar J, Hathorne E, Ballesteros E and Kersting DK (2023) Elemental cycles in the coralline alga Neogoniolithon hauckii as a recorder of temperature variability in the Mediterranean Sea. Front. Mar. Sci. 10:1151592. doi: 10.3389/fmars.2023.1151592
Received: 26 January 2023; Accepted: 19 April 2023;
Published: 01 May 2023.
Edited by:
Travis Blake Meador, Academy of Sciences of the Czech Republic (ASCR), CzechiaReviewed by:
Matthias López Correa, National Research Council (CNR), ItalyAndres Rüggeberg, Université de Fribourg, Switzerland
Copyright © 2023 Hetzinger, Grohganz, Halfar, Hathorne, Ballesteros and Kersting. This is an open-access article distributed under the terms of the Creative Commons Attribution License (CC BY). The use, distribution or reproduction in other forums is permitted, provided the original author(s) and the copyright owner(s) are credited and that the original publication in this journal is cited, in accordance with accepted academic practice. No use, distribution or reproduction is permitted which does not comply with these terms.
*Correspondence: Steffen Hetzinger, c3RlZmZlbi5oZXR6aW5nZXJAaWZnLnVuaS1raWVsLmRl