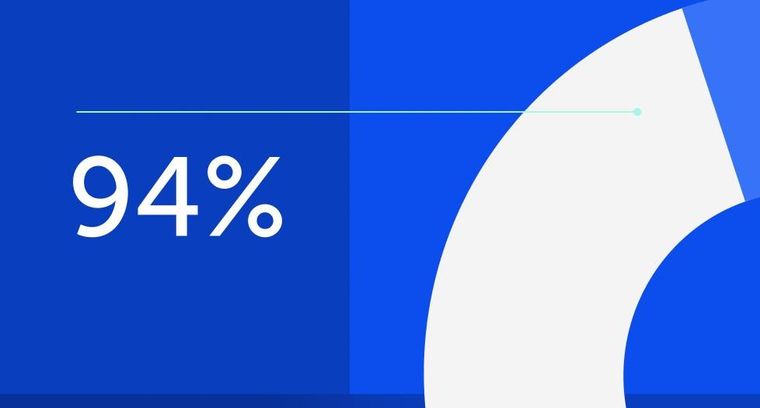
94% of researchers rate our articles as excellent or good
Learn more about the work of our research integrity team to safeguard the quality of each article we publish.
Find out more
METHODS article
Front. Mar. Sci., 12 June 2023
Sec. Marine Biogeochemistry
Volume 10 - 2023 | https://doi.org/10.3389/fmars.2023.1150518
Radiolaria are the primary contributors to biogenic opal in the tropical ocean, and their isotope compositions are potentially useful tool for reconstructing the silicon cycle in mid-depth waters. To date little attention has been paid to their isotopic study, partly because of the difficulties in purifying radiolarian tests from marine sediments. In this study twelve surface sediment samples from the South China Sea and the western Indian Ocean were used in a density separation experiment, with the aim of enabling better separation of radiolarian tests from detrital grains and other siliceous organisms. The results show that sponge spicules, radiolarian tests and diatom frustules from tropical ocean sediments preferentially settle in heavy liquid solutions with different specific gravities, and are constrained to range in the densities from 1.95 to 2.1 g/cm3, 1.85 to 2.0 g/cm3 and 1.7 to 1.95 g/cm3, respectively. These density ranges for radiolarians and diatom frustules in the low-latitude ocean are much lower than that of amorphous silica, probably resulting from the decreased silicification of radiolarians and diatoms due to the limited silica availability in the highly-stratified tropical ocean. According to the components and density ranges of siliceous microfossils observed in this study, an optimized method with detailed procedures is proposed to extract and purify radiolarians from late Quaternary sediments in the tropical ocean that have not undergone substantial dissolution and diagenetic change. Using a combination of wet-chemical treatment, wet sieving, differential settling, and density separations, this method can yield clean radiolarian test in sufficient quantities from tropical ocean sediments for isotope/geochemical analysis.
Silicon (Si) is one of the most important nutrients in the oceanic ecosystem, impacting export efficiency of organic matter to the deep sea through the production of siliceous phytoplankton (e.g., Nelson et al., 1995; Tréguer et al., 2018). Through this, the Si cycle plays a key role in the marine biological pump, the regulation of the carbon cycle and global climate changes on various timescales (e.g., Tréguer and Pondaven, 2000; Conley and Carey, 2015). However, the limited quantitative data about the reservoirs, consumptions, and sources of dissolved silicon (DSi) in the different water layers of the ocean precludes a comprehensive understanding of these processes and the evolution of the marine Si cycle together with its climatic effects over time. To address this, it is necessary to develop effective tools for quantifying changes in the DSi cycle in the modern and past ocean environments so that the relationships between the Si cycle, biological pump efficiency and global climate change can be better assessed.
The silicon isotope composition of siliceous microfossils, composed of diatom frustules, sponge spicules, radiolarians, and silicoflagellates, is an effective tool for quantitative reconstructions of past oceanic DSi concentrations and consumptions (e.g., De La Rocha et al., 1998; Hendry and Robinson, 2012; Doering et al., 2021), and has provided records on various aspects of the past silicon cycle (Brzezinski et al., 2002; De La Rocha, 2003; Hendry et al., 2012; Hendry et al., 2014; Ablemann et al., 2015; Hendry et al., 2016; Fontorbe et al., 2016; Swann et al., 2016; Fontorbe et al., 2017; Swann et al., 2017; Sutton et al., 2018; Worne et al., 2022). This includes changes in surface water silicon utilization (e.g., De La Rocha, 2003; Hendry et al., 2014; Ablemann et al., 2015; Swann et al., 2016; Worne et al., 2022), and deep-water DSi concentrations (e.g., Hendry et al., 2012; Fontorbe et al., 2016; Hendry et al., 2016; Fontorbe et al., 2017). Radiolarians, accounting for 62-99 wt. % of the biogenic opal in the surface sediments of the tropical Pacific Oceans (Lisitzin, 1972; Zhang et al., 2015), have significant potential to extended this by constraining the Si cycle at surface and mid-layer ocean depths (Ablemann et al., 2015; Doering et al., 2021). However, until now the isotope composition of radiolaria has received minimal attentions, partly due to the difficulties in separating and purifying sufficient radiolarian tests from sediment samples for isotopic analysis.
Although approaches have been proposed for the purification of diatoms from marine and lacustrine sediments (Shemesh et al., 1988; Ellwood and Hunter, 1999; Morley et al., 2004; Swann et al., 2013; Swann and Snelling, 2023), few studies have focused on the extraction of radiolarians from marine sediments. Shemesh et al. (1988) proposed a method for the separation and cleaning of both diatoms and radiolarians from Southern Ocean sediments by sieving and settling techniques. These samples did not include sponge spicules, which are a common marine siliceous group and are more difficult to separate from radiolarians due to their similar particle sizes. Ablemann et al. (2015) also developed an approach to separate the radiolarian fraction larger than 125 µm from Southern Ocean sediments through a series of sieving and settling steps combined with ultrasonic treatment. However, when this approach is applied to samples from the tropical oceans in this current study, some sponge spicules remain in the sieved material alongside radiolarians. Additionally, the ultrasonic treatment used by Abelmann et al. (2015) for several hours to remove diatoms from radiolarians would result in the breakage of certain radiolarian species in the tropical ocean, such as the Dictyocoryne profunda/truncatum group, which are common species characterized by three arms (Shiino et al., 2020) and are susceptible to breakage by sonication. Therefore, this method may not be suitable for extracting radiolarian from sediments in low to mid-latitude oceans.
Until now, extracting purified radiolarians for isotope analysis is best achieved by hand-picking under a light microscope after the initial separation of material by a heavy density liquid such as sodium polytungstate (SPT) (Hendry et al., 2014; Fontorbe et al., 2016; Fontorbe et al., 2017). In general, there are three problems with manual picking. Firstly, radiolarian tests from Quaternary sediments in the tropical Pacific Ocean are light with an average weight from 0.063 to 0.136 μg/shell (Moore, 1969; Takahashi, 1982). As such, 7,000 to 15,000 radiolarian tests need to be handpicked per 1 mg of material needed for isotope analysis. Secondly, even if tests were handpicked under a microscope, it is unavoidable to lose some radiolarian tests when transferring samples to a storage vial due to electrostatic adsorption of the light shells to the transfer tool, such as a brush. Finally, the composition of the radiolarian assemblages may be changed substantially because of the randomness of manual picking or priority selection of larger individuals, which may bias the measured isotope compositions.
This study thus aims to 1) constrain the density ranges of the radiolarians, diatoms and sponge spicules through experiments on the heavy liquid separation of various marine sediments, and 2) from this develop a more effective method to separate radiolarians from marine sediments, ensuring that the purity of extracted radiolarian tests is >99%.
A total of 12 sediment samples from the South China Sea (SCS, 9 samples) and the western Indian Ocean (WIO, 3 samples) (Figure S1; Table S1 in Supplementary Material) were used to assess the settling characteristics and density ranges of different siliceous microfossils. Three samples of already purified radiolarian tests, extracted from sediment samples in the SCS by the method shown in the Section 4.2 and Supplementary Material, were also used to further quantify the settling of radiolaria in various SPT solutions.
Heavy liquids, with different specific gravities for the density separation experiments, were made by further diluting prepared SPT solution (~2.3 g/ml) with deionized water (DDW):
Where D is the volume of DDW added into the prepared SPT solution; P1 and P2 are the densities of the prepared SPT solution and the solution to be prepared, respectively; V is the volume of the prepared SPT solution.
Bulk sediment samples were dried in the oven at 55°C, and processed with wet-chemical treatment for the removals of carbonates and organic matters (see details in Section 4.2 and the Supplementary Material). The residuals of the bulk samples after the wet-chemical treatment were sonicated using an Emerson Branson-5800 at 40 KHz and 160W for ~5 minutes, to fully disperse particles and remove detritus particles adhering onto the surface of siliceous microfossils, and then separated twice by SPT solution at a specific gravity of 2.3 g/ml to maximize the extraction of all siliceous microfossils. After centrifugation with a relative centrifuge force (RCF) of approximately 1153g (2500 rpm) for 20 minutes using a Heraeus Megafuge 16 with the rotor radius of 16.5 centimeter, the supernatant was extracted into separate centrifuge tubes before being rinsed three times with DDW by centrifugation at the RCF of approximately 415g (1500 rpm) for 5 minutes each time. Centrifuge tubes were then filled with 10ml 30% H2O2 and allowed to react in a heat block at 75°C for 96 hours. After again being rinsed three times with DDW by centrifugation at the RCF of approximately 415g for 5 minutes each time, particles were well mixed with DDW into homogeneous suspensions and ~0.1 ml of the suspension was extracted by pipette into separate tubes. This material was then separated by SPT solutions at specific gravities from 2.25 g/ml to 1.70 g/ml at 0.5-unit intervals. After every SPT separation, particles both in the supernate and settling (i.e. density fraction) were inspected under an inverted microscope at x100 magnification to determine the compositions of each fraction. Settlings were then dried for weighing, whilst particles in the suspension and supernate were used for the next density separation until there were less than a total of approximately 100 particles (less than 0.1% of the total particles in the sample before the density separation experiment) remaining in the suspension/supernate.
After each SPT separation, samples were rinsed three times with DDW and then mixed into a homogeneous suspension. Three to four drops of the suspension and approximately double amount of the DDW were then dripped onto a petri dish and placed under an inverted microscope. The individual numbers of various components in each sample, which would be used to calculate the proportion of the specific siliceous component relative to each settling, were determined in 2-3 fields of view at x100 magnification. Settling solutions were then dried in the oven at 55°C and weighted. Based on the weight and relative proportions of siliceous microfossils observed under the microscope, the total amount of each siliceous component extracted through the density separation experiments can be calculated as:
Where TM is the total amount of each siliceous component extracted through the density separation experiments, and Pi is the proportion of the specific siliceous component in each settling separated by the SPT solutions from 2.25 to 1.7 g/ml at 0.5-unit intervals, and Wp is the weight of each settling. The relative amount of each siliceous component at an individual specific density can be defined as:
where the RS is the relative amount of the specific siliceous component; Si is the relative proportions of the specific siliceous microfossil in the settling; Wp and TM are same as those in Eq.2.
In order to confirm the purity and cleanliness of radiolarian tests extracted by the method proposed in this study, radiolarian tests were inspected using an inverted microscope and scanning electron microscopy (SEM) coupled with Energy Dispersive X-ray Spectroscopy (EDS). For the purity inspection under the microscope, radiolarian tests were well mixed with DDW into homogeneous suspensions. Three to four drops of the suspension, along with approximately double amount of DDW, were then dripped onto a petri dish and observed under an inverted microscope. The purity of radiolarian tests was assessed based on the average number of non-radiolarian particles present in 100 radiolarian individuals in 4-5 fields of view at x100 magnification.
SEM-EDS was employed to further examine the cleanness and preservation of radiolarian tests using a JEOL JSM-IT200 SEM equipped with an Oxford Instruments Nano Analysis Ultim 100 EDS detector at the Nanoscale and Microscale Research Centre, University of Nottingham. The freeze-dried radiolarian tests were scattered randomly onto an aluminum stub with double-sided carbon tape and coated with a layer of carbon film. The samples were then imaged and subjected to EDS analysis in secondary electron mode, with a spot size of 5 (dimensionless) and an accelerating voltage of 10 kV. To perform the EDS analysis, each spectrum was obtained by collecting over 1,000,000 counts, and element concentrations of Si, O, Fe, Al, Mg, Sr, Na, K and Cl were analyzed using the Oxford Instruments Nanoanlaysis Software called AZtec. These elements are the major components of siliceous microfossils and silicate detritus, and can be used to identify any detrital particles that may remain in pure radiolarian test samples.
The weights, compositions and relative amounts of the siliceous particles settling from different sediment samples through repeated density separations exhibit similar patterns (Figures 1–3). In general, most of the terrigenous material and a very small fraction of siliceous fossils (<1%) settle in the SPT solutions with specific gravities of greater than 2.1 g/ml, and a few terrigenous detritus as well as a large fraction of siliceous microfossils gradually settle with the decrease in the specific gravities below 2.1 g/ml (Figure S2 in the Supplementary Material). After the 1.7 g/ml SPT solution almost no material remains in the supernate (Figure 1; Figure S2 in the Supplementary Material). Based on the weight and composition of the particles in the settling, the settling processes of marine sediments are divided into four stages (Figures 1, 2).
Figure 1 the net weight (Wp) (A) and the percentage (B) of the settling from representative samples through the SPT density separations.
Figure 2 the relative proportion of each siliceous microfossil relative to the total particles (dashed lines) in each settling and to the total amount of specific siliceous component (solid line) separated from various SPT solutions.
Figure 3 Compositions of the supernate and the settling from a representative sample (SCS-NE3) in the tropical ocean by SPT density separation. All photos taken under the inverted microscope at 100x magnification.
Stage I (specific gravity = 2.25 to 2.1 g/ml): settlings are predominately composed of terrigenous material. There is a decrease in detrital particle size as the specific gravity of the SPT solution declines (Figure S2 in the Supplementary Material) and a slight increase of sponge spicules in the 2.1 g/ml SPT solution. The total amounts of siliceous microfossils in the settlings are<1%.
Stage II (specific gravity = 2.1 to 2.0 g/ml): settlings across all sites/samples, are dominated by sponge spicules (~46-78% of settling) (Figures 1–3). Diatoms and radiolaria constitute 0-11% and ~0-16% of the settling respectively, whilst 3-19% of the settling is terrigenous detritus. The relative amounts of sponge spicules (RSspon), radiolarian tests (RSrad), and diatom frustules (RSdiat) ranges from 82-93%, 4-19%, and 0-18%, respectively.
Stage III (specific gravity = 2.0 to 1.85 g/ml): settlings are dominated by radiolarian tests (~44-71% of settling) (Figures 1–3), with the amount varying from ~11-38%, 52-55% and ~1-18% in SPT solutions of 1.95 g/ml, 1.9 g/ml and 1.85 g/ml respectively. With the increase of radiolarian tests in the settling during this stage, RSspon decreases to an average of ~8% in the settling in a solution of 1.95 g/ml and to lower levels (0-3%) in solutions< 1.9 g/ml (Figure 2), indicating that specific gravities of 2.0 to 1.95 g/ml are likely the transition range in the switch in settling from sponge spicules to radiolaria (Figures 1, 2). RSrad and RSdiat in the settling increase to ~79-95% and ~35-62% in this stage respectively.
Stage IV (specific gravity = 1.85 g/ml to 1.70 g/ml): diatoms (~62-86% of the settling) are the primary siliceous microfossils after the density separations (Figures 1–3), with relative abundances varying from ~53-59%, 53-89% and ~62-90% in the 1.8 g/ml, 1.75 g/ml, and 1.7 g/ml SPT solutions, respectively (Figure 2). Although the relative proportion of diatom frustules in the settling increases through this stage, radiolarians still contribute ~11-18% of the settling at 1.8 g/ml and decrease to 0-2% in solutions< 1.8 g/ml, indicating that specific gravities from 1.85 to 1.8 g/ml are the transition range in the switch in settling from radiolarians to diatoms (Figure 1). RSrad and RSdiat vary from ~1-3% and ~23-60% in the settling, respectively.
Experiments on the purified radiolarians show that ~12-15%, 72-78%, and 8-10% of the radiolarians settle in the SPT solutions at specific gravities of 1.95 g/ml, 1.9 g/ml and 1.85 g/ml respectively. Very few radiolarian tests appear in the settlings separated by the SPT solutions >2.0 g/ml or<1.85 g/ml (Figure 1), with maximum settling occurring at ~1.9 g/ml. This reiterates the earlier results indicating that radiolarians preferentially settle in solutions from 2.0 to 1.85 g/ml (Figure 2).
Based on the radiolarian classification system, Radiolaria are classified into three classes: Polycystinea, Acantharea, and Taxopodida (Adl et al., 2019). The majority of radiolaria extracted from both the SCS and WIO belong to Class Polycystinea which have much stronger resistance of dissolution and are generally well preserved in the sediments compared to Acantharea, and Taxopodida (Suzuki and Aita, 2011). These polycystine radiolarians in the studied samples are primarily composed of Orders Spumellaria and Nassellaria, with the absence or less than 1% of Order Collodaria (Figure 4 as well as Figure S3 and Videos 1 and 2 in the Supplementary Material). In samples of pure radiolarian tests extracted from surface sediments using the method proposed in this study, the average number of non-radiolarian particles is less than one for every 100 radiolarian individuals in different visual fields under an inverted microscope at 100 x magnifications (Figure S3 and Videos 1 and 2 in the Supplementary Material), indicating a purity of >99% in radiolarian tests.
Figure 4 SEM and EDS spectrum images of pure radiolarian tests extracted from surface sediment samples in the South China Sea (A and A’) and the western Indian Ocean (B and B’). C and C’ are the SEM image and spectrum of Spongaster tetras (radiolaria), which is characterized by a dense meshwork and thus easy to capture fine detrital particles on the surface of the shell. The white squares in SEM images (A–C) represent the areas analyzed by EDS. The elements in red in the tables of the element composition at the top right of spectrums (A’, B’ and C’) indicate those below the minimum detectable limit for the EDS detector used in this study.
SEM images indicate that radiolarian tests are free from clay minerals or fine detrital particles on their shells, and the majority of radiolarian tests are intact with no apparent changes in shape or distribution of the shell pores (Figure 4). The EDS analysis results show that Si and O are the primary constitutes of pure radiolarian test samples, with a combined concentration exceeding 99%, and the concentrations of other elements (Fe, Al, Mg, Sr, Na, K and Cl) are all below the minimum detectable limit for the EDS detector used in this study. These finding further provide evidence that no impurities were mixed with the radiolarians and that there were no changes in pore material of radiolarian tests which may be induced by diagenesis.
Accurate information about the density of different siliceous microfossils is essential to prepare the correct SPT density solutions for the extraction of radiolarian tests from detrital grains and other siliceous organisms. Although much work has been carried out to determine water contents (e.g., Levi et al., 1989; Mortlock and Froelich, 1989; Herdianita et al., 2000), chemical compositions (Shemesh et al., 1988; Sandford, 2003), and the settling rates of various siliceous microfossils (e.g., Takahashi, 1982; Miklasz and Denny, 2010), only a few authors have reported on the densities of diatoms and sponge spicules (Van Ierland and Peperzak, 1984; Sandford, 2003; Miklasz and Denny, 2010).
The skeleton of siliceous microfossils (biogenic silica) is made of amorphous hydrated silica (SiO2.nH2O) and varies considerably in density depending on the different degrees of hydration and the amount of structural water in the formula SiO2.nH2O (Perry et al., 2003). In general, the density of biogenic silica is lower than that of the pure silica (~2.65 g/cm3), and generally below 2.2 g/cm3 (Perry et al., 2003; Finkel and Kotrc, 2010). Living diatoms with a cytoplasm have densities of 1.03-1.26 g/cm3 (Van Ierland and Peperzak, 1984), with the density of the cytoplasm itself 1.03-1.1g/cm3 (Smayda, 1970). After deposition onto the seafloor the cytoplasm is decomposed, leading to diatom frustules preserved in sediments having a higher density (1.4- 2.2 g/cm3, mean = c. 2.1 g/cm3) than those living in the water column (Miklasz and Denny, 2010; Finkel and Kotrc, 2010). According to the experiments performed in this study on sediments from the tropical oceans, although a few diatoms occurred in the settling of SPT solutions > 2.0 g/cm3, most diatoms (82-100%; mean RSdiat = 92%) settled in solutions at specific gravities from 1.7-1.95 g/cm3 (Figure 2), much lower than those typically reported densities for diatom frustules (2.1 g/cm3) and amorphous hydrated silica (~2.2 g/cm3).
There is limited data on the density of sponge spicules in sediment samples. Levi et al. (1989) reported the density range of sponge spicule as 2.01-2.03 g/cm3 based on three spicule fragments. Sandford (2003) analyzed the physical property and chemical composition of six species of sponge spicules, suggesting that the average density ranged from 2.03-2.13 g/cm3. In this current study, ~96-99% of the sponge spicules (mean RSspon=98%) that settle in SPT solution have slightly lower densities ranging from 1.95-2.10 g/cm3 (Figure 2). To date, little effort has been made to measure the density of radiolarian tests in marine sediments, but based on the current experiments ~79-96% (mean RSrad = 91%) of radiolarian tests which primarily belong to polycystines settle in solutions from 1.85-2.0 g/cm3 (Figure 2).
As discussed above, although siliceous microfossils from tropical sediments are primarily comprised of amorphous silica, the densities of most radiolarian tests (1.85-2.0 g/cm3) and diatom frustules (1.7 to 1.95 g/cm3) are much lower than values typically reported for amorphous silica (~2.2 g/cm3). Considering all siliceous microfossils were reacted with 30% H2O2 in a heat block at 75°C for 96h, and then sonicated for 5 minutes before the density separation experiments, organic matter and any material adhering onto the shells of the biogenic silica should be completely removed, and thus have no impact on densities of siliceous microfossils.
Although both dissolution and diagenetic alteration may affect the densities of siliceous microfossils in the ocean, we suggest that these processes have had no substantial impacts on siliceous organisms extracted from sediment samples used in this study. Whilst dissolution can results in a various degree of breakage to siliceous fossils, most of siliceous microfossils extracted from the sediment samples in this study are intact and well-preserved (Figure 4 and Videos 1 and 2 in the Supplementary Material), indicating they have not undergone significant dissolution. Diagenetic change is sluggish in the majority of deep-sea sediments because of the uniformly low temperatures on the deep ocean floor and moderate to low geothermal gradients that characterize large parts of the ocean basins and continental margins (Hesse and Schacht, 2011). Typically, such changes occur in the strata beneath the seafloor at depths of several hundred meters, induced by an increase in the pressure and geothermal temperature (Hesse and Schacht, 2011). The samples used in this study were primarily derived from unconsolidated surface sediments, and showed no indications of having undergone diagenesis
In addition, the surface samples from the South China Sea and the western Indian Ocean which were used for the density separation experiments in this study covered a range of typical water depths from outer shelf (372 m) to the deep sea (4,778 m) (Table S1 in the Supplementary Material). If dissolution and digenetic alteration had a significant impact on the densities of siliceous microfossils extracted from different oceans in this study, it is possible that the settling characteristics of these microfossils in SPT solutions would differ between areas. However, density separation experiment indicated that the weights, compositions and relative amounts of the siliceous particles settling from different sediment samples exhibited similar patterns (Figures 1, 2 and Figure S2 in the Supplementary Material). This suggests that neither dissolution nor digenetic alteration had a substantial impact on the densities of siliceous microfossils in this study, and that the specific siliceous organism preserved in the surface sediments may have a similar density range in the tropical oceans.
Furthermore, the dissolution of biogenic silica in the ocean is a chemical process during which lighter isotopes are preferentially released into the water, while heavier isotopes will remain in the skeletons of siliceous organisms (Demarest et al., 2009). As a result, the dissolution is expected to result in a slight increase in the density of siliceous organisms, rather than a decrease. The process of diagenesis can also have the similar impact on the density of siliceous fossils as that of dissolution. During the diagenesis process, biogenic opal (SiO2.nH2O) will gradually transform from Opal A to Opal Cristobalite/Tridymite (C/T), and this transformation is a process of dehydration reaction (Davies and Clark, 2006; Davies et al., 2006). The transformation from opal A to opal C/T will decrease the amount of structural water in Opal A, causing its structure to become more stable (dissolution-resistant), compact and denser (Davies et al., 2006). If siliceous organisms in this study underwent substantial diagenesis or dissolution, it would be expected that their densities would slightly increase, and be potentially higher than the density of amorphous silica (2.2 g/cm3). However, the density ranges of various siliceous microfossils obtained from our study are notably lower than typically reported density values. Therefore, we suggest that the process of diagenetic alternation or dissolution had no substantial impacts on the densities of siliceous organisms extracted from sediment samples used in this study.
Since the density of the amorphous hydrated silica (SiO2.nH2O) depends on the relative content of the silica and structural water (Perry et al., 2003), the lower densities of the radiolarians and diatom frustules may indicate that the skeletons of these organisms are characterized by less silica in their amorphous silica structure. It has been suggested that decreasing silicification is a common strategy for organisms to avoid growth limitation in Si-depleted oceans (Paasche, 1975; Brzezinski et al., 1990; Martin-Jézéquel et al., 2000; Finkel and Kotrc, 2010; McNair et al., 2018), and this mechanism has been invoked to explain the decrease in radiolarian shell weight in low-latitude oceans during the Cenozoic (Lazarus et al., 2009). As the water column in the tropical oceans is highly stratified and the dissolved silica is extremely low in the upper water column, a decreased silicification of radiolarians and diatoms may also be responsible for their low densities recorded in the current study.
Due to the overlapping density ranges of the various siliceous microfossils in this study, we suggest it is not possible to only use density separation for the extraction and purification of the radiolarian tests from tropical ocean sediments for isotope/geochemical analysis. Although ~90% of the radiolarians preferentially settle at densities from 1.85-2.0 g/cm3, the ~10% loss of radiolarian tests may cause a composition bias in the radiolarian assemblage. In addition, ~51% of diatom frustules and a small fraction of fine mineral grains would also settle alongside radiolarians in this density range. However, density separation can effectively remove siliceous components (e.g. non-monoaxonic sponge spicules) and silicate grains which are similar in size to radiolarian tests. This would then allow for the further purification of radiolarian tests after the removals of diatom frustules, monoaxonic sponge spicules and fine detrital grains in the settling by sieving at different size fractions.
Based on our findings above, we propose a method to efficiently extract purified radiolarian samples from tropical ocean sediment which have not undergone substantial dissolution and diagenetic change, in sufficient quantities for isotope or geochemical analysis. This method is based on some steps of methods for the extraction of diatoms (Swann and Snelling, 2023), but contains additional stages which reflect the increased difficulty in extracting radiolaria. Marine sediments are generally composed of organic matters, biogenic organisms (calcareous and siliceous organisms) and non-biogenic components (silicate detritus and autogenic mineral grains) (Li and Schoonmaker, 2003). To extract purified radiolarian tests from the tropical marine sediments, wet sieving, differential settling and density separation is applied to remove all non-radiolarian material including organic matter, calcareous organisms, diatoms, sponge spicules, seaweed spines, terrigenous material and autogenic minerals.
The details of the full methodology are outlined in the Supplementary Material. Organic matters and calcareous organisms should be removed first by treatment with 30% H2O2 and 5-8% HCl respectively. After wet chemical treatments, residuals can be filtered with a 53 µm sieve to pre-concentrate radiolarian tests and remove all fine detritus, small diatoms, seaweed spines and a small fraction of sponge spicules (if required, filtrates in the container can be collected and retained for the further separation of diatoms by SPT flotation). After the first sieving, the larger faction would be composed mainly of radiolarian tests, larger diatom frustules (of which there are typically few in most tropical marine sediments), a few coarse detrital grains and many sponge spicules (Figure 5).
The differential settling, sonication treatment, the second sieving, and density separation is applied to further separate radiolaria from other particles that remain after the first sieving. For the removal of large diatoms, samples undergo differential settlings (Shemesh et al., 1988) and sonication treatment for no more than 10 minutes to break down the remaining large diatoms and avoid the breakage of radiolarian tests. The key procedure in the methodology is the removal of the sponge spicules which have a similar density and size to radiolarian tests. Although most sponge spicules are greater than 53 μm in length, the diameter (cross section) of monoaxonic sponge spicules is generally several micrometers (Tang et al., 2019). Therefore, it is possible to remove all monoaxonic spicules and a fraction of smaller non-monoaxonic spicules by wet sieving if the sponge spicules are repeatedly suspended in the water during the sieving process. This can be done by half immersing samples in a container filled with DDW, with the bottom of the sieve tapped gently to ensure that all particles remain suspended in water (steps of Stage 3 shown in the Supplementary Material). This leads to almost all monoaxonic sponge spicules passing through a 53 µm sieve (Figure 5), which if required could be collected from the container flask for further isotope/geochemical analysis of the sponge spicules.
The particles retained in the sieve after the second sieving would be mainly comprised of purified radiolarian tests, with some coarse detritus and potentially a small fraction of non-monoaxonic sponge spicule. Radiolarian test can then be further isolated via an additional set of density separation stages at specific gravities from 2.1-2.0 g/cm3 by 0.2-0.5-unit interval (steps of Stage 4 shown in the Supplementary Material). In our tests, this effectively removes the remaining detrital grains and sponge spicules (Figure 5) with minimal (<2%) loss of radiolarian tests.
Figure 5 The main process for separating and purifying the radiolarian tests from tropical marine sediments. All photos taken under the inverted microscope at 100x magnification.
After the procedure of density separation, the particles should be examined under an inverted microscope to access the purity of the radiolarian test. If the average number of non-radiolarian particles is less than one for every 100 radiolarian individuals in different visual fields under an inverted microscope at 100 x magnifications as shown in Supplementary Videos 1 and 2, it confirms a purity of >99% in radiolarian test. At this point, the purified radiolarian tests can be transferred into a container and freeze-dried in preparation for isotope analysis. Otherwise, steps of stage 2, 3, and 4 outlined in Supplementary Material should be repeated to further remove specific impurities that may be mixed with the radiolarian tests, until the purity level reaches >99% (Figure 4 and Videos 1 and 2 in the Supplementary Material).
Considering the samples used for our experiments on the heavy liquid separation are completely unconsolidated surface sediments from the tropical ocean with no substantial dissolution or diagenetic alteration, we suggest that the method proposed in this study is primarily applied to extract and purify radiolaria primarily from late Quaternary tropical marine sediments which have not undergone substantial dissolution and diagenetic changes. If the sediment samples have undergone diagenetic alternation, the initial three stages (steps 1 to 16 in Supplementary Material) of the method remain applicable. However, as density ranges of siliceous microfossils may be altered during diagenesis, it is necessary to carry out experiments on heavy liquid separation of sediments as described in section “2.2 Separation experiment” to determine the density ranges of various siliceous fossils prior to proceeding with the steps of stage 4 (steps 17 to 23 in Supplementary Material), which aim to further remove the non-monoaxonic spicules and detrital particles from radiolarian tests. This information concerning the density ranges of various siliceous fossils is essential to access the feasibility of preparing appropriate SPT density solutions for the extraction of radiolarian tests from detrital grains and other siliceous organisms.
1) Based on the settling characteristics of siliceous microfossils in SPT solutions, the sponge spicules, radiolarians, and diatom frustules from tropical ocean sediments are constrained to range in density from ~1.95 to 2.1 g/cm3, 1.85 to 2.0 g/cm3 and 1.7 to 1.95 g/cm3, respectively.
2) The density range of radiolarians and diatom frustules in the low-latitude ocean are much lower than that of amorphous silica, probably resulting from the decreased silicification of radiolarians and diatoms due to the limited silica availability in the highly-stratified tropical ocean.
3) An effective method has been proposed to extract and purify radiolaria from tropical marine sediments that were not subjected to substantial dissolution and diagenetic change. In general, after processing by wet-chemical treatment, sieving and differential settling and density separation, late Quaternary sediments from the tropical oceans can yield purified radiolarian tests at a purity of >99%.
The original contributions presented in the study are included in the article/Supplementary Material. Further inquiries can be directed to the corresponding author.
Both of the authors contributed to the design of the experiment and the method proposed in this study. QZ wrote the first draft of the manuscript, and GS contributed to manuscript revision. Both of the authors approved the submitted version.
This work was supported by the NERC Isotope Geosciences Facilities Steering Committee (Grant No. 2230.0320), Guangdong Basic and Applied Basic Research Foundation (Grant Nos. 2020A1515010499, 2022A1515010932), National Natural Science Foundation of China (Grant Nos. 42076073, 41606046), and the CAS Scholarship.
The authors declare that the research was conducted in the absence of any commercial or financial relationships that could be construed as a potential conflict of interest.
All claims expressed in this article are solely those of the authors and do not necessarily represent those of their affiliated organizations, or those of the publisher, the editors and the reviewers. Any product that may be evaluated in this article, or claim that may be made by its manufacturer, is not guaranteed or endorsed by the publisher.
The Supplementary Material for this article can be found online at: https://www.frontiersin.org/articles/10.3389/fmars.2023.1150518/full#supplementary-material
Abelmann A., Gersonde R., Knorr G., Zhang X., Chapligin B., Maier E., et al. (2015). The seasonal sea-ice zone in the glacial Southern Ocean as a carbon sink. Nat Commun 6 (1), 8136. doi: 10.1038/ncomms9136
Adl S. M., Bass D., Lane C. E., Lukeš J., Schoch C. L., Smirnov A., et al. (2019). Revisions to the classification, nomenclature, and diversity of eukaryotes. J. Eukaryotic Microbiol. 66 (1), 4–119. doi: 10.1111/jeu.12691
Brzezinski M. A., Olson R. J., Chisholm S. W. (1990). Silicon availability and cell-cycle progression in marine diatoms. Mar. Ecol. Prog. Ser. 67, 83–96. doi: 10.3354/meps067083
Brzezinski M. A., Sigman D. M., Sarmiento J. L., Matsumoto K., Gruber N., Rau G. H., et al. (2002). A switch from Si(OH)4 to NO3–depletion in the glacial southern ocean. Geophys. Res. Lett. 29, 1564. doi: 10.1029/2001GL014349
Conley D. J., Carey J. C. (2015). Silica cycling over geologic time. Nat. Geosci. 8 (6), 431–432. doi: 10.1038/ngeo2454
Davies R. J., Clark I. R. (2006). Submarine slope failure primed and triggered by silica and its diagenesis. Basin Res. 18 (3), 339–350. doi: 10.1111/j.1365-2117.2006.00297.x
Davies R. J., Huuse M., Hirst P., Cartwright J., Yang Y. (2006). Giant clastic intrusions primed by silica diagenesis. Geology 34 (11), 917–920. doi: 10.1130/G22937A.1
De La Rocha C. L. (2003). Silicon isotope fractionation by marine sponges and the reconstruction of the silicon isotope composition of ancient deep water. Geology 31, 423–426. doi: 10.1130/0091-7613(2003)031<0423:SIFBMS>2.0.CO;2
De La Rocha C. L., Brzezinski M. A., DeNiro M. J., Shemesh A. (1998). Silicon-isotope composition of diatoms as an indicator of past oceanic change. Nature 395 (6703), 680–683. doi: 10.1038/27174
Demarest M. S., Brzezinski M. A., Beucher C. P. (2009). Fractionation of silicon isotopes during biogenic silica dissolution. Geochimica Cosmochimica Acta 73 (19), 5572–5583. doi: 10.1016/j.gca.2009.06.019
Doering K., Ehlert C., Pahnke K., Frank M., Schneider R., Grasse P. (2021). Silicon isotope signatures of radiolaria reveal taxon-specific differences in isotope fractionation. Front. Mar. Sci. 782. doi: 10.3389/fmars.2021.6668
Ellwood M. J., Hunter K. A. (1999). Determination of the Zn/Si ratio in diatom opal: a method for the separation, cleaning and dissolution of diatoms. Mar. Chem. 66 (3-4), 149–160. doi: 10.1016/S0304-4203(99)00037-7
Finkel Z. V., Kotrc B. (2010). Silica use through time: macroevolutionary change in the morphology of the diatom fustule. Geomicrobiol. J. 27 (6-7), 596–608. doi: 10.1080/01490451003702941
Fontorbe G., Frings P. J., Christina L., Hendry K. R., Conley D. J. (2016). A silicon depleted north Atlantic since the palaeogene: evidence from sponge and radiolarian silicon isotopes. Earth Planet. Sci. Lett. 453, 67–77. doi: 10.1016/j.epsl.2016.08.006
Fontorbe G., Frings P. J., de la Rocha C. L., Hendry K. R., Carstensen J., Conley D. J. (2017). Enrichment of dissolved silica in the deep equatorial pacific during the Eocene-oligocene. Paleoceanography 32 (8), 848–863. doi: 10.1002/2017PA003090
Hendry K. R., Gong X., Knorr G., Pike J., Hall I. R. (2016). Deglacial diatom production in the tropical north Atlantic driven by enhanced silicic acid supply. Earth Planet. Sci. Lett. 438, 122–129. doi: 10.1016/j.epsl.2016.01.016
Hendry K. R., Robinson L. F. (2012). The relationship between silicon isotope fractionation in sponges and silicic acid concentration: modern and core-top studies of biogenic opal. Geochimica Cosmochimica Acta 81, 1–12. doi: 10.1016/j.gca.2011.12.010
Hendry K. R., Robinson L. F., McManus J. F., Hays J. D. (2014). Silicon isotopes indicate enhanced carbon export efficiency in the north Atlantic during deglaciation. Nat. Commun. 5 (1), 1–9. doi: 10.1038/ncomms4107
Hendry K. R., Robinson L. F., Meredith M. P., Mulitza S., Chiessi C. M., Arz H. (2012). Abrupt changes in high-latitude nutrient supply to the Atlantic during the last glacial cycle. Geology 40 (2), 123–126. doi: 10.1130/G32779.1
Herdianita N. R., Browne P. R. L., Rodgers K. A., Campbell K. A. (2000). Mineralogical and textural changes accompanying ageing of silica sinter. Mineralium Deposita 35 (1), 48–62. doi: 10.1007/s001260050005
Hesse R., Schacht U. (2011). Early diagenesis of deep-sea sediments. Develop. Sedimentol. 63, 557–713. doi: 10.1016/B978-0-444-53000-4.00009-3
Lazarus D. B., Kotrc B., Wulf G., Schmidt D. N. (2009). Radiolarians decreased silicification as an evolutionary response to reduced Cenozoic ocean silica availability. Proc. Natl. Acad. Sci. 106 (23), 9333–9338. doi: 10.1073/pnas.0812979106
Levi C., Barton J. L., Guillemet C., Le Bras E., Lehuede P. (1989). A remarkably strong natural glassy rod: the anchoring spicule of theMonorhaphis sponge. J. Mater. Sci. Lett. 8 (3), 337–339. doi: 10.1007/BF00725516
Li Y. H., Schoonmaker J. E. (2003). “Chemical composition and mineralogy of marine sediments,” in Treatise on Geochemistry. Eds. Turekian K. K., Holland H. D. (Amsterdam: Elsevier Science Ltd.) 7, 1–35.
Lisitzin A. P. (1972). Sedimentation in the world ocean. Soc Econ. Paleont. Mineral. Spec. Publ. No. 17, 218 pp. doi: 10.2110/pec.72.17
Martin-Jézéquel V., Hildebrand M., Brzezinski M. A. (2000). Silicon metabolism in diatoms: implications for growth. J. Phycol. 36 (5), 821–840. doi: 10.1046/j.1529-8817.2000.00019.x
McNair H. M., Brzezinski M. A., Krause J. W. (2018). Diatom populations in an upwelling environment decrease silica content to avoid growth limitation. Environ. Microbiol. 20 (11), 4184–4193. doi: 10.1111/1462-2920.14431
Miklasz K. A., Denny M. W. (2010). Diatom sinkings speeds: Improved predictions and insight from a modified Stokes' law. Limnol Oceanogr 55 (6), 2513–2525. doi: 10.4319/lo.2010.55.6.2513
Moore T. C. Jr. (1969). Radiolaria: change in skeletal weight and resistance to solution. Geol. Soc. America Bull. 80 (10), 2103–2108. doi: 10.1130/0016-7606(1969)80[2103:RCISWA]2.0.CO;2
Morley D. W., Leng M. J., Mackay A. W., Sloane H. J., Rioual P., Battarbee R. W. (2004). Cleaning of lake sediment samples for diatom oxygen isotope analysis. J. Paleolimnol. 31 (3), 391–401. doi: 10.1023/B:JOPL.0000021854.70714.6b
Mortlock R. A., Froelich P. N. (1989). A simple method for the rapid determination of biogenic opal in pelagic marine sediments. Deep Sea Res. Part A. Oceanogr. Res. Papers 36 (9), 1415–1426. doi: 10.1016/0198-0149(89)90092-7
Nelson D. M., Tréguer P., Brzezinski M. A., Leynaert A., Quéguiner B. (1995). Production and dissolution of biogenic silica in the ocean: revised global estimates, comparison with regional data and relationship to biogenic sedimentation. Global Biogeochem. Cycles 9 (3), 359–372. doi: 10.1029/95GB01070
Paasche E. (1975). Growth of the plankton diatom thalassiosira nordenskioeldii cleve at low silicate concentrations. J. Exp. Mar. Biol. Ecol. 18 (2), 173–183. doi: 10.1016/0022-0981(75)90072-6
Perry C. C., Belton D., Shafran K. (2003). “Studies of biosilicas; structural aspects, chemical principles, model studies and the future,” in Silicon biomineralization: biology–Biochemistry—Molecular biology–biotechnology. Ed. Müller W. E. G. (Berlin; Heidelberg: Springer), 269–299.
Sandford F. (2003). Physical and chemical analysis of the siliceous skeletons in six sponges of two groups (Demospongiae and Hexactinellida). Microsc Res Tech 62 (4), 336–355. doi: 10.1002/jemt.10400
Shemesh A., Mortlock R. A., Smith R. J., Froelich P. N. (1988). Determination of Ge/Si in marine siliceous microfossils: separation, cleaning and dissolution of diatoms and radiolaria. Mar. Chem. 25 (4), 305–323. doi: 10.1016/0304-4203(88)90113-2
Shiino Y., Kurihara T., Ichinohe R., Kishimoto N., Yoshino T., Matsuoka A. (2020). A morphological analysis of the flat-shaped spumellarian radiolarian dictyocoryne: morpho-functional insights into planktonic mode of life. Paleontol. Res. 24 (2), 134–146. doi: 10.2517/2019PR020
Smayda T. J. (1970). The suspension and sinking of phytoplankton in the sea. Oceanogr. Mar. Biol. Annu. Rev. 8, 353–414.
Sutton J. N., André L., Cardinal D., Conley D. J., De Souza G. F., Dean J., et al. (2018). A review of the stable isotope bio-geochemistry of the global silicon cycle and its associated trace elements. Front. Earth Sci. 112. doi: 10.3389/feart.2017.00112
Suzuki N., Aita Y. (2011). Radiolaria: achievements and unresolved issues: taxonomy and cytology. Plankton Benthos Res. 6 (2), 69–91. doi: 10.3800/pbr.6.69
Swann G. E., Pike J., Leng M. J., Sloane H. J., Snelling A. M. (2017). Temporal controls on silicic acid utilization along the West Antarctic peninsula. Nat. Commun. 8 (1), 1–8. doi: 10.1038/ncomms14645
Swann G. E. A., Pike J., Snelling A. M., Leng M. J., Williams M. C. (2013). Seasonally resolved diatom δ18O records from the West Antarctica peninsula over the last deglaciation. Earth Planet. Sci. Lett. 364, 12–23. doi: 10.1016/j.epsl.2012.12.016
Swann G. E., Snelling A. M. (2023). Isotope sample preparation of diatoms for paleoenvironmental research. PloS One 18 (2), e0281511. doi: 10.1371/journal.pone.0281511
Swann G. E., Snelling A. M., Pike J. (2016). Biogeochemical cycling in the Bering Sea over the onset of major northern hemisphere glaciation. Paleoceanography 31 (9), 1261–1269. doi: 10.1002/2016PA002978
Takahashi K. (1982). Vertical flux, ecology and dissolution of radiolaria in tropical oceans: implications for the silica cycle. PhD thesis. (Cambridge MA: Massachusetts Institute of Technology and Woods Hole Oceanographic Institution), pp. 461. doi: 10.1575/1912/2420
Tang Q., Wan B., Yuan X., Muscente A. D., Xiao S. (2019). Spiculogenesis and biomineralization in early sponge animals. Nat. Commun. 10 (1), 1–10. doi: 10.1038/s41467-019-11297-4
Tréguer P., Bowler C., Moriceau B., Dutkiewicz S., Gehlen M., Aumont O., et al. (2018). Influence of diatom diversity on the ocean biological carbon pump. Nat. Geosci. 11 (1), 27–37. doi: 10.1038/s41561-017-0028-x
Tréguer P., Pondaven P. (2000). Silica control of carbon dioxide. Nature 406, 358–359. doi: 10.1038/35019236
Van Ierland E. T., Peperzak L. (1984). Separation of marine seston and density determination of marine diatoms by density gradient centrifugation. J. Plankton Res. 6 (1), 29–44. doi: 10.1093/plankt/6.1.29
Worne S., Swann G. E., Kender S., Lacey J. H., Leng M. J. (2022). Silicic acid cycling in the Bering Sea during the mid-pleistocene transition. Paleoceanogr. Paleoclimatol. 37 (2), e2021PA004284. doi: 10.1029/2021PA004284
Keywords: radiolarian, density separation, marine sediments, tropical ocean, method, isotope
Citation: Zhang Q and Swann GEA (2023) An effective method to extract and purify radiolaria from tropical marine sediments. Front. Mar. Sci. 10:1150518. doi: 10.3389/fmars.2023.1150518
Received: 24 January 2023; Accepted: 23 May 2023;
Published: 12 June 2023.
Edited by:
Jun Sun, China University of Geosciences Wuhan, ChinaReviewed by:
Tatiana Palechek, Geological Institute (RAS), RussiaCopyright © 2023 Zhang and Swann. This is an open-access article distributed under the terms of the Creative Commons Attribution License (CC BY). The use, distribution or reproduction in other forums is permitted, provided the original author(s) and the copyright owner(s) are credited and that the original publication in this journal is cited, in accordance with accepted academic practice. No use, distribution or reproduction is permitted which does not comply with these terms.
*Correspondence: Qiang Zhang, emhhbmdxaWFuZzIxMEBzY3Npby5hYy5jbg==
Disclaimer: All claims expressed in this article are solely those of the authors and do not necessarily represent those of their affiliated organizations, or those of the publisher, the editors and the reviewers. Any product that may be evaluated in this article or claim that may be made by its manufacturer is not guaranteed or endorsed by the publisher.
Research integrity at Frontiers
Learn more about the work of our research integrity team to safeguard the quality of each article we publish.