- 1The Key Laboratory of Mariculture, Ministry of Education, Ocean University of China, Qingdao, China
- 2Institute of Biochemistry, Carleton University, Ottawa, ON, Canada
Background: In the past few decades, heat waves and seasonal variation linked with global warming are causing frequent fluctuations of water temperature in aquatic environments, resulting in further challenges for marine organisms. As a poikilothermic marine animal and a temperate species, sea cucumber A. japonicus is also very sensitive to temperature variation and shows a limited ability to adapt to environmental temperature changes. However, the molecular mechanisms by which A. japonicus perceives signals from such a diverse environment including the trigger of aestivation still remain unclear. TRP (transient receptor potential) superfamily, as the great potential “sentinel” of the organism in response to the changes of the external environment signals, perceived little attention and will be the future great candidates for sensory function research in echinoderm.
Methods: BLAST program was applied to identify the AjTRPs based on A. japonicus transcriptome database. Gene structure analysis of AjTRPs and phylogenetic analysis of TRPs were performed. The expression profiles of AjTRPs in different tissues and in response to different heat stress treatments (chronic/ acute) were measured using qRT-PCR.
Results: A total of 54 TRPs were identified in A. japonicus. Gene structure analysis showed that the exon numbers and the domains of AjTRPs varied greatly. Phylogenetic analysis indicated that AjTPRs were mainly grouped into six subfamilies: TRPA, TRPM, TRPC, TRPV, TRPML, and TRPP. Copy numbers of each TRP member in marine invertebrates including A. japonicus were more than those in vertebrates, however some TRP channel members were lost in invertebrates. Expression profile detection suggested that the representative 15 AjTRP genes belonging to 6 subfamilies were expressed in all tissues examined.
Conclusion: Our findings indicate that the plastic evolutionary history of TRP channel genes and suggest that TRPM2-1, TRPM2-2, TRPM3, and TRPC3-1 are potential thermo-TRP proteins involved in thermal regulation in A. japonicus. This work facilitates the knowledge of TRP-mediated sensory systems in echinoderms, provides new insights into the mechanisms of aestivation and global warming adaptation in marine invertebrates.
1 Introduction
TRP (transient receptor potential) channels were first discovered in the visual conduction system of Drosophila in 1969 (Cosens and Manning, 1969). Minke et al. (1975) named these channels for the first time in 1975 based on their electrophysiological phenotype. In 1989, Montell and Rubin cloned and identified the TRP genes of Drosophila melanogaster and found that mutant DmTRP could rescue the defect of light response (Montell and Rubin, 1989). By 2014, the molecular characteristics, biophysical properties, and pathophysiological functions of the TRP superfamily members began to be extensively studied (Flockerzi and Nilius, 2014) and this work has increased exponentially in recent years.
TRP channels are cation-permeable six transmembrane (S1-S6) ion channels. There is a cation-permeable pore region between S5 and S6, and an amino (N) terminal and a carboxy (C) terminal that are located inside the cell (Clapham, 2003). Most TRP family members are activated in the form of a homo-tetramer (Hoenderop et al., 2003), but some studies have reported that hetero-tetramers can also be formed and activated (Hofmann et al., 2002; Hoenderop et al., 2003; Staruschenko et al., 2010). The overall structural features are highly conserved between different TRP channels, with the specificity of each member being localized to the N-terminal and C-terminal domains. The TRP channel superfamily is classified into seven subfamilies based on amino acid sequence homology rather than ligand function or ion selectivity. They are the TRPC (“canonical”), TRPM (“melastatin”), TRPV (“vanilloid”), TRPA (“ankyrin”), TRPML (“mucolipin”), TRPP (or PKD) (“polycystin”), and TRPN (no mechanoreceptor potential C, or NOMPC). Remarkably, TRPN has not been found in mammals (Walker et al., 2000; Corey et al., 2004; Clapham et al., 2005).
As an important multimodal receptor in cells, TRP channels are mainly involved in the perception processes of vision, smell, taste, temperature, and mechanical stimulation (Perraud et al., 2001; Perraud et al., 2003; Tracey et al., 2003; Kang et al., 2011; Zhan et al., 2016). These channels can be activated by compounds, messenger molecules, osmotic pressure, and temperature changes inside or outside cells. The activation of different TRP channels requires different types of stimuli, and may even be activated by multiple types of stimuli at the same time (Venkatachalam and Montell, 2007). Besides sensory functions, TRP channels are also involved in the regulation of physiological functions such as immunity, inflammation, kidney function, and bone development (Nilius and Owsianik, 2011). Accumulating studies have reported that a number of hereditary and acquired diseases are also related to the dysfunction of TRP channels (Nilius and Owsianik, 2011). In addition, two members of the SpREG (Receptor for Egg Jelly), belonging to TRPP subfamily of the sea urchin, Strongylocentrotus purpuratus, have been reported to be associated with the sperm acrosome reaction (Gunaratne et al., 2007).
Marine invertebrates account for the largest portion of marine animals and play an irreplaceable role in the material cycle and energy flow in marine ecosystems (Branch et al., 2013). In addition, most marine invertebrates are weak in mobility, vulnerable to environmental influences, simple in shape and structure, and lack obvious sensory organs. To date, studies of TRP channels in marine invertebrates are still very limited. Fu et al. (2021) compared the expression profile of TRPs under acute and chronic heat stress in two oyster species, Crassostrea gigas and C. angulata. Peng et al. (2021) systematically identified and characterized 17 TRPs in Zhikong scallop, Chlamys farreri, and analyzed the spatiotemporal expression profile of these sensors. Notably, TRPs were also suggested to be involved in functional phototransduction in squid, Doryteuthis pealei (Kingston et al., 2015), and early brachiopod embryos (Passamaneck and Martindale, 2013). In echinoderms, the studies of TRP channels have focused mainly on sea urchins and starfish. Gunaratne et al. (2007) identified 10 TRPP subfamily members in the sea urchin S. purpuratus and reported a low similarity of their genomic structures and distinct temporal and spatial expression profiles. Previous studies also suggested that TRPA1 could be activated by heat stimulation and was involved in positive thermotaxis in starfish Patiria pectinifera larvae (Saito et al., 2017). Ding et al. (2019) found that the expression levels of TRPA1 increased significantly under long-term low temperature stress in the sea urchin, S. intermedius. TRPs are also reported to play key roles in locomotion and are involved in pigment release by sea urchins, S. purpuratus (Shah et al., 2018). Overall, there is still much to be learned about the functions of TRP subfamily members and their roles in the sensory systems of marine invertebrates.
In the past few decades, heat waves and seasonal variation linked with global warming are causing frequent fluctuations in water temperature in aquatic environments, causing further challenges for marine organisms, particularly those in shallow water locations (Frölicher et al., 2018; Oliver et al., 2018). The sea cucumber, Apostichopus japonicus (Echinodermata, Holothuroidea), is a key benthic organism (Wang, 2018) and plays an important role in the marine ecosystem with involvement in the carbon cycle, bioturbation, and nutrient regeneration (Kitchell et al, 1978; Uthicke, 2001; Schneider et al., 2011; Mactavish et al., 2012). Currently, this species is also getting much attention because of its high nutritional and economic values and special biological characteristics, including a pentamerous radial symmetrical structure, evisceration, autolysis, aestivation, and regeneration (Chen et al., 2021). However, little is known about the sensory system of echinoderm, especially in A. japonicus. And some limited research has suggested that the tube feet, tentacles, and papilla of A. japonicus may be the most important potential sensory organs in response to environmental signals like light (Liu et al., 2020a), chemical (Marquet et al., 2020), and mechanical stimulation (Lin et al., 2020). In addition, as a poikilothermic marine animal and a temperate species, A. japonicus is also very sensitive to temperature variation and shows a limited ability to adapt to environmental temperature changes (Xu et al., 2016). Furthermore, continuous extreme high temperatures (up to 30°C) are lethal to A. japonicus cultured in semi-open regions. Over a long evolutionary process, A. japonicus has acquired a high temperature-induced special physiological behavior, aestivation, to aid survival and reproduction (Yang et al., 2005). To date, the molecular mechanisms by which A. japonicus perceive these signals from their environment including the trigger of aestivation still remain unclear. Most of the research focus on the identification of G protein-coupled receptors (GPCRs) related to light (Liu et al., 2020b) and chemical stimulation (Marquet et al., 2020) at the transcriptional level. However, the TRP superfamily, as the great potential “sentinel” of the organism in response to the changes of the external environment signals, have received little attention and will be great future candidates for sensory function research in echinoderms. In order to better understand the functions of the TRP superfamily in echinoderms, in the present study we identified and characterized the TRP channels of A. japonicus. Expression profiles of AjTRP channels were investigated in different tissues and in response to chronic and acute heat stress. Our work provides a valuable profile on the TRP channels in A. japonicus that will lead to future studies of the TRP-mediated sensory system in echinoderms and offers new insights into global warming adaptation in marine invertebrates.
2 Materials and methods
2.1 BLAST-based identification of TRP superfamily members in A. japonicus
The full-length transcriptomes (NCBI accession number: PRJNA785124) (Wang et al., 2022a) and the larval transcriptomes (NCBI accession numbers: SRR6075435, SRR6075436, SRR6075437, and SRR6075438) (Boyko et al., 2019) of A. japonicus were generated previously and were applied for identification of TRP superfamily members. The full-length transcriptome was constructed based on pooled samples from control (15°C) and 48 h heat-stressed (30°C) A. japonicus coelomocytes. The larval transcriptome samples were from blastula (17-27 hours), gastrula (32-43 hours), dipleurula (88 hours), and pentactules (19 days) stages of development. Initially, 446 TRP gene sequences (Supplementary Table S1) including Acanthaster planci (Tu et al., 2012), Strongylocentrotus purpuratus (Hall et al., 2017), Asterias rubens (Hennebert et al., 2015), Patiria miniata (Accession: PRJNA597964) and Lytechinus variegatus (Bronstein and Kroh, 2019) were submitted as queries for BLAST (ncbi-blast-2.13.0) searches with e-value set to 0.01. Among them, the TRP gene sequences of echinoderms were performed with tBLASTn and used “blastn -query seq-in.fasta -db dbname -out seq-out1.blast -outfmt 6 -evalue 0.01 -num_threads 8” parameters. Other species were performed using BLASTp with “blastp -query seq-in.fasta -db dbname -out seq-out2.blast -outfmt 6 -evalue 0.01 -num_threads 8” parameters. Based on that, we further confirmed the results by BLASTp against NCBI non-redundant (Nr) protein sequence database. Finally, 54 TRPs were identified; detailed information is provided in Supplementary Table S2.
2.2 Sample collection
Adult A. japonicus (80–100g) were collected from an aquaculture farm in Weihai (Shandong, China) in June 2021. The animals were maintained in a seawater aquarium system at 18°C for at least one week and fed with commercial formulated diet once a day. After acclimation, 10 individuals were randomly selected at 18°C as the control (CO) group and different tissues (tube foot, tentacle, nerve ring, intestine, coelomocytes, and papilla) were collected immediately and rapidly frozen in liquid nitrogen, then stored at −80°C for subsequent analysis. A small opening about 1cm was made in the abdomen of A. japonicus, and 5ml of coelom fluid was sucked with a disposable sterile pipette and centrifuged at 1000rpm for 3 min at 4°C, and the supernatant was discarded to preserve the precipitation as coelomocytes. The front tissue of A. japonicus was cut off, the surrounding tentacles, water water-vascular system, and muscle tissue were removed, and the remaining tissue wrapped by a calcium ring as a nerve ring was collected. For the chronic heat stress group, the temperature was raised at a rate of 2°C every 3 days until it reached 26°C and then held at 26°C continuously. Animal tissues were collected at three time points: when the temperature was 22°C (chronic heat stress-1, CH-1), 26°C on first (chronic heat stress-2, CH-2), and sixteenth days (chronic heat stress-3, CH-3). Aestivation is a special physiological behavior for A. japonicus, mainly induced by high summer temperatures to aid survival and reproduction over a long evolutionary process. A previous study has verified that temperatures from 24.5 to 25.5°C were determined to be the threshold for entering into aestivation (EA) for such sized sea cucumbers (Yang et al., 2005). After maintaining this temperature range for at least 15 days, sea cucumbers were observed to stop feeding, and their movements, if any, were very limited, and sea cucumbers were seen to enter into deep aestivation stage (DA) (Zhao et al., 2014). In our present study, the sea cucumbers were slowly induced into aestivation basically following the protocol reported previously (Zhao et al., 2014). CH-2 and CH-3 correspond to EA and DA respectively. For the acute heat stress group (AH), water temperature was raised to 30°C at a rate of 2°C/h and held at 30°C for 48 hours. The tissues of CH-1, CH-2, CH-3, and AH groups were collected. For each group of heat stress, 10 individuals were sacrificed and the tissues were flash frozen in liquid nitrogen and then stored at −80°C. All animal protocols for care, experimentation, and sacrifice had the approval of the Experimental Animal Ethics Committee of the Ocean University of China.
2.3 RNA extraction
Total RNA was isolated from different tissues using Trizol RNA isolation reagent (R401-01; Vazyme, China) according to the manufacturer’s instructions. RNA degradation and contamination were monitored using 1% agarose gels. The purity and concentration of RNA were measured using NanoDrop ND1000 spectrophotometer (NanoDrop Technologies, Rockland, DE, United States) with OD 260/280 reading, and then RNA was stored at −80°C until use.
2.4 cDNA cloning and expression profiles of TRP genes
Two potential temperature sensitive AjTRPM2-1 and AjTRPM2-2 channels and one temperature insensitive AjTRPML3-1 channel were randomly chosen to clone. Specific primers for AjTRPM2-1, AjTRPM2-2, and AjTRPML3-1 were designed using Primer3Plus (https://www.primer3plus.com/index.html ) based on the sequences of AjTRP genes from the results by tBLASTn (Supplementary Table S3). The RNA for SMARTer RACE cDNA came from the intestine tissue of the control group. The SMARTer RACE 5’/3’ Kit (634858; TAKARA, Japan) was used to clone the full-length cDNA sequences following the manufacturer’s instructions. PCR amplification was carried out using ApexHF HS DNA Polymerase CL (AG12204; Accurate, China). The RACE PCR conditions were described as follows: (1) 95°C for 5 min; (2) 5 cycles of 94°C for 30s, 68°C for 30s, and 72°C for 3 min; (3) 5 cycles of 94°C for 30s, 68°C for 30s, and 72°C for 3 min; (4) 5 cycles of 94°C for 30s, 66°C for 30s, and 72°C for 3 min; (5) 20 cycles of 94°C for 30s, 64°C for 30s, and 72°C for 3 min; followed by a final cycle of (6) 72°C for 10 min. The PCR products were purified and eluted by 1% agarose gel, then ligated to the Lineareized pRACE vector (provided with the SMARTer RACE 5’/3’ Kit) and transformed into E. coli DH5α competent cells (9057; TAKARA, Japan). Positive recombinant clones were sequenced by BGI-Qingdao (BGI-Qingdao, China). The sequences and peak maps were analyzed and assembled using DNAStar software (DNAStar Inc., USA) to confirm the accuracy of predicted sequences.
cDNA was synthesized using Hifair III 1st Strand cDNA Synthesis Super Mix (YEASEN; Cat No. 11141ES60) and qRT PCR (Quantitative Real-time PCR) was performed using Hieff UNICON Universal Blue qPCR SYBR Green Master Mix (11184ES08; YEASEN, China) with the TRP gene specific primer pairs (Supplementary Table S3). The cDNA used for qRT-PCR was diluted by 5, 10, 20, 40, and 80 times for evaluating amplification efficiencies of the primers. Standard curves were made with the log value of the dilution multiple being the independent variable and the Ct value being the dependent variable; the slope (k) was then acquired. The formula of the standard curves (E):
Xiao and Xu (2021) have summarized that TRPA1, TRPM2-5, TRPM8, and TRPV1-4 were temperature sensitive molecules in different systems. TRPC3 was reported to be involved in thermal regulation in oysters (Fu et al., 2021). So AjTRPA1, TRPM2, TRPM3, and TRPC3 identified in A. japonicus were selected as potential temperature-sensitive TRP channels. β-actin and β-tubulin were selected as internal controls as previously reported (Zhao et al., 2014; Xu et al., 2018; Huo et al., 2019). Each sample was run in triplicate and melt-curve analysis of the amplification products was performed to confirm specificity. The 2−ΔΔCT method was applied to analyze the comparative expression levels, and the data were subjected to one-way analysis of variance (ANOVA) followed by a Tukey post hoc test (SPSS 25.0 software, Chicago, IL, USA). All results are shown as mean ± S.E.M. (n = 5). Different lowercase letters indicate significant differences (p< 0.05).
2.5 Sequence analysis and number comparisons of TRP genes and phylogenetic analysis
TBtools software was applied to predict structural information about the protein domains (Chen et al., 2020). Isoelectric points and mass fractions of the protein were estimated using the Prot-Param online tool (https://web.org/protparam/) (Swain, 2015). The motifs were predicted by MEME Suite (https://meme-suite.org/meme/tools/meme). BLASTn was applied for aligning the TRP gene sequences with the whole-genome sequences for genomic structure analysis. Then the exon and intron architectures of TRPs were structured based on the website of GSDS v2.0 (http://gsds.gao-lab.org/) (Hu et al., 2015). The number of transmembrane domains of AjTRPs were predicted by Protter (http://wlab.ethz.ch/protter/start http://wlab.ethz.ch/protter/start/). The positions of TRP genes on the chromosomes were determined by annotations based on the genome database (China National GeneBank DataBase (CNGBdb) under BioProject Accession No. CNP0002776) and mapped by TBtools software (Chen et al., 2020; Wang et al., 2022b). To further investigate the relationship of AjTRPs with those from other representative species, ClustalW was used to make multiple sequence alignments of full-length proteins and the phylogenetic analysis based on the Maximum Likelihood method were performed using MEGA 11 with WAG+G+I+F model and then edited with EvolView (https://evolgenius.info//evolview-v2/). All sequence ID numbers used for analysis are listed in Supplementary Table S1.
The copy numbers of TRPs of Homo sapiens, Mus musculus, Danio rerio, Xenopus tropicalis, Branchiostoma belcheri, Drosophila melanogaster, Caenorhabditis elegans, Ciona intestinalis, Exaiptasia pallida, Crassostrea gigas, Crassostrea virginica, Mizuhopecten yessoensi, S. purpuratus, A. planci, and A. japonicus were counted to explore the evolution of TRP genes. The numbers of H. sapiens, M. musculus, D. rerio, X. tropicalis, C. intestinalis, M. yessoensi, C. virginica, and C. gigas were obtained from Fu et al. (2021). The information of TRP genes of D. melanogaster, C. elegans, and E. pallida were obtained from Peng et al. (2021) and B. belcheri, S. purpuratus, and A. planci were retrieved from NCBI database.
3 Results
3.1 Molecular cloning and expression profile of TRPs in different tissues
A total of 54 TRP genes were identified in A. japonicus. The full-length cDNA of TRPM2-1, TRPM2-2, and TRPML3-1 were verified and the sequences were highly consistent with predicted sequences. All sequences were deposited in the NCBI database (accession numbers: OP470643 - OP470696). Among them, TRPA subfamily contained one member, AjTRPA1; TRPC subfamily contained five members, AjTRPC3, AjTRPC4, AjTRPC5, AjTRPC6, and TRP-gamma; TRPM subfamily contained four members, AjTRPM1, AjTRPM2, AjTRPM3, and AjTRPM6; TRPML subfamily contained one member, AjTRPML3; TRPV subfamily contained two members, AjTRPV5 and AjTRPV6; and TRPP subfamily contained six members, AjTRPP1, AjTRPP2, receptor for egg jelly 4, receptor for egg jelly 5, receptor for egg jelly 7, and receptor for egg jelly 9. In our study, for most of the AjTRP members, we selected the longest isoform for qRT-PCR analysis. In addition, for TRPA1-2 and TRPC3-1, we selected the isoforms containing the most comprehensive domains and for TRPM2, we selected the TRPM2-1 for which the complete CDS has been verified. The amplification efficiencies of the primers for qRT-PCR were within 90% - 110% (r2 ≥ 0.95) and shown in Supplementary Table S3. The expression profiles of 15 AjTRP members were measured in various tissues, including tube foot, coelomocytes, tentacle, nerve ring, papilla, and intestine. And the schematic diagram of these organism’s anatomy has been shown in Supplementary Figure S1. We compared the expression levels of the same gene in different tissues. The qRT PCR results showed that these AjTRPs were expressed in all tissues examined (Figure 1). Among them, AjTRPC4-2, AjTRPM1-1, AjTRPM2-1, and AjTRPML3-1 were mainly expressed in tube foot compared with other tissues, and the expression levels of AjTRPML3-1 in tube foot were ten times higher than in other tissues. Expression levels of AjTRPC3-1 and AjTRPV6 were highest in coelomocytes compared with other tissues. The highest expression levels of AjTRPA1-2 and AjTRPC5-2 were observed in tentacles. Nerve ring was the tissue with the highest expression levels of several TRP genes, including AjTRPM3, AjTRPM6, and AjTRPP2. In addition, the expression levels of AjTRPC4-2 and AjTRPML3-1 in nerve ring were approximately ten times higher than in other tissues except for tube foot. The expression levels of AjTRPC6, AjTRPV5, receptor for egg jelly7-2, and AjTRP-gamma-2 were highest in papilla. All AjTRPs assessed in this study were expressed in intestine, but the expression levels were relatively low.
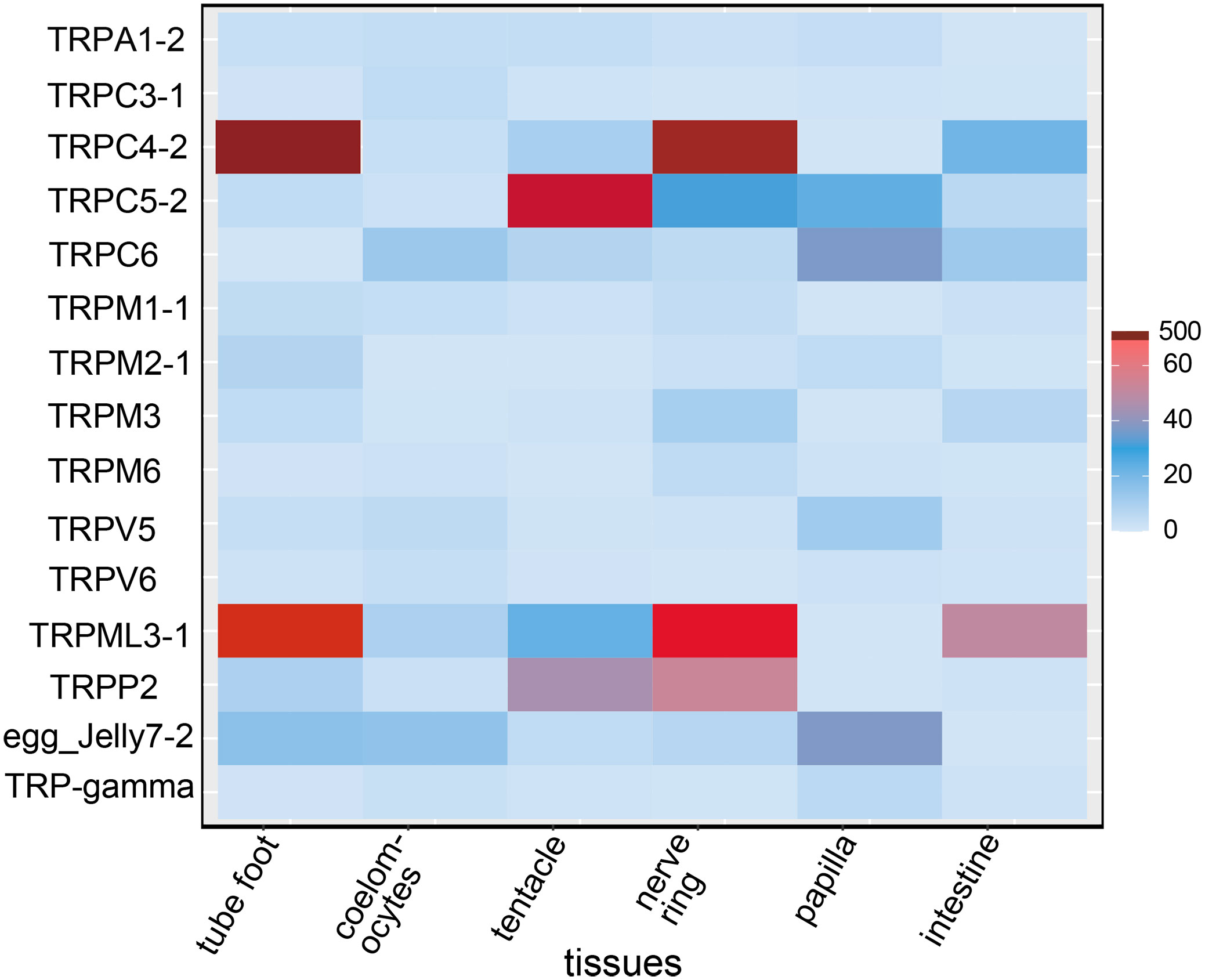
Figure 1 The relative expression levels of TRPs in different tissues. Expression profiles of TRPs in different tissues including tube foot, coelomocytes, tentacle, nerve ring, papilla, and intestine. Data are presented as the mean ± S.E.M. (n = 5). egg_Jelly: receptor for egg jelly, belonging to the TRPP subfamily.
3.2 Sequence analysis
The physicochemical properties of TRP channels showed that the size of TRP superfamily members differed greatly (Supplementary Table S2). The predicted isoelectric points (pI) of the AjTRPs ranged from 4.19 to 9.37 and the mean pI was 6.38. The protein encoding TRPA1-1 was the shortest with only 114 amino acids and the lowest molecular weight (12.35 kDa). The protein encoding the receptor for egg jelly 4 was the longest with 3363 amino acids and the highest molecular weight (376.61 kDa).
Gene structure analysis showed that the exon numbers of AjTRPs varied greatly (Figure 2 and Supplementary Table S2). Among them, TRPP2-2 contained only one exon, TRPA1-1, TRPA1-9, and receptor for egg jelly 5-2 contained 3 exons, while receptor for egg jelly 4 contained 37 exons which contained the largest exon number.
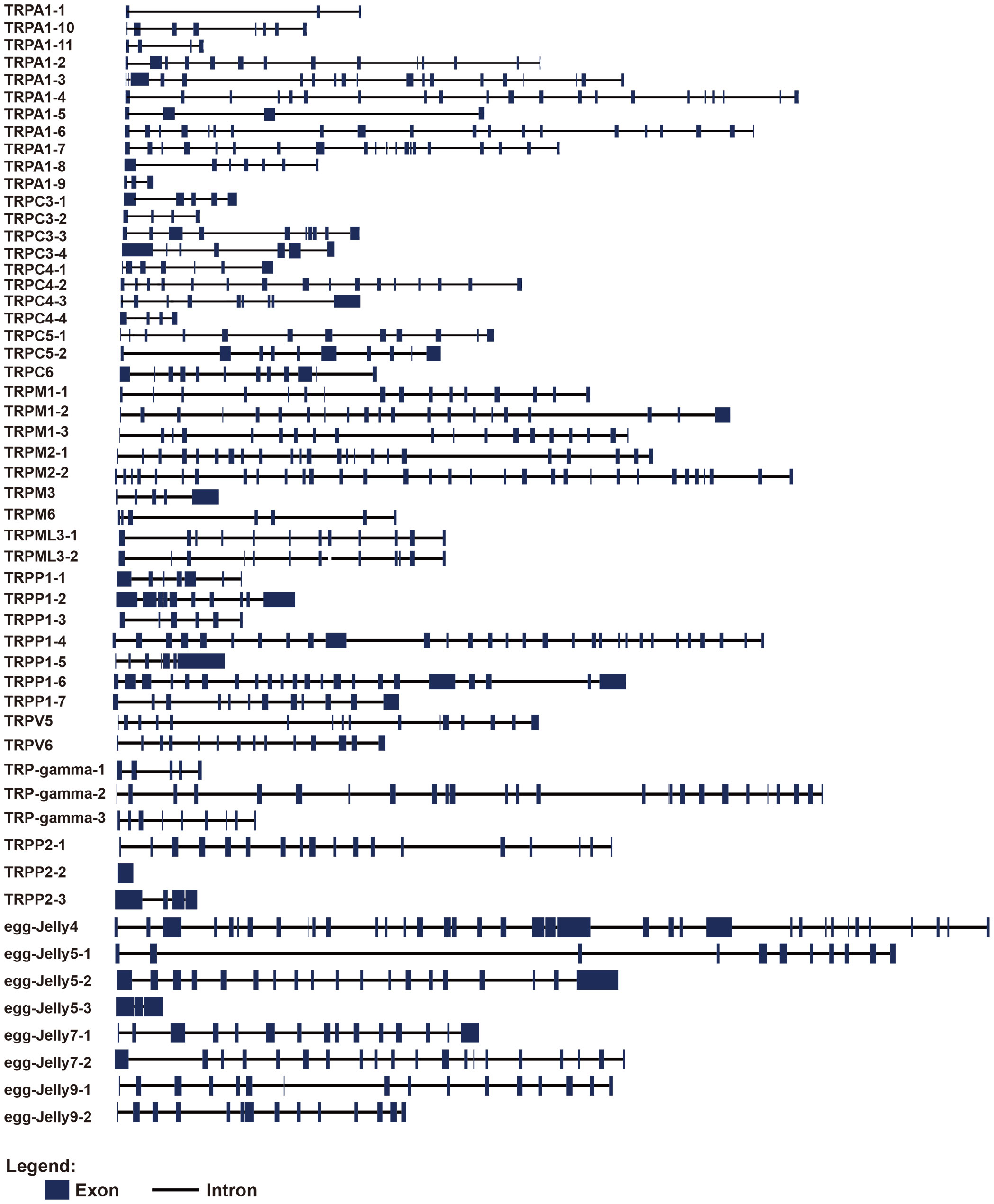
Figure 2 Gene structures of TRPs in A. japonicus. The blue square represents the exon region and the black line represents the intron.
As shown in Figure 3, the structural domains of AjTRPs were complex and there were significant differences among them. Almost all TRPA subfamily members and TRPC3-3, TRPC5-2, TRPC6, TRPV5, and TRPV6 contained multiple ANK domains which were a repetitive sequence containing 33 amino acids and activated non-selective cationic channels through covalent modification of key cysteine residues (Hinman et al., 2006). Among them, TRPA1-3 and TRPA1-6 have the largest number of ANK domains (16 and 15, respectively). The ion_trans, low_complexity_region, transmembrane_domain, and TRP_2 domains were ubiquitous in most TRP channels. TRP_2 domains were mainly distributed in TRPC subfamily. Notably, PKD has an IG-like fold and functions as a ligand binding site in protein-protein or protein-carbohydrate interactions (Bycroft et al., 1999) and REJ domains exist specifically in TRPP and TRPML subfamilies. REJ domain contained 600 amino acids, PKD-like domains, and six conserved cysteine residues that may form disulphide bridges (Moy et al., 1996).
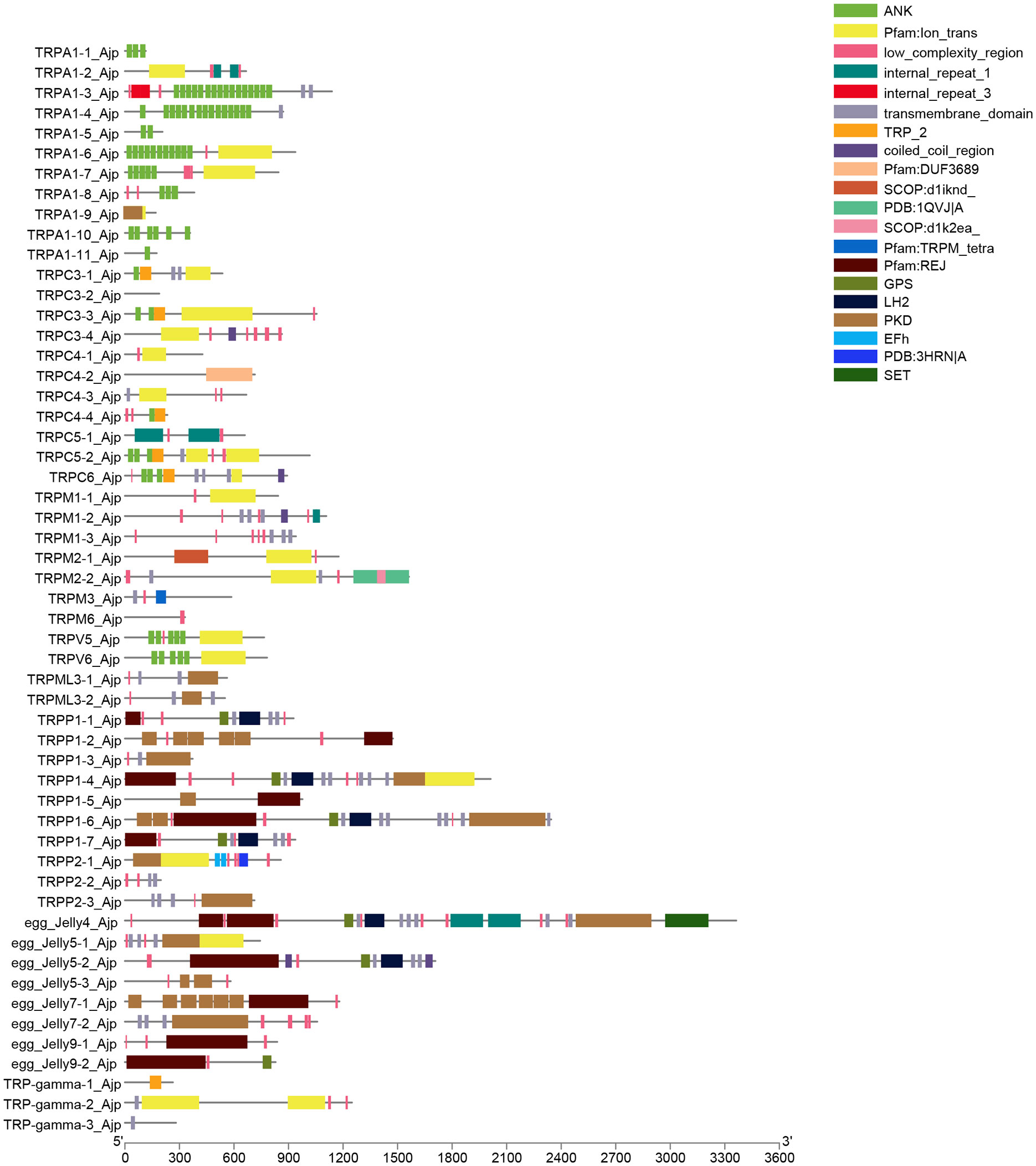
Figure 3 The conserved domains of the TRP proteins. Domains that have been identified are represented in color; the lines indicate that the domain is not recognized.
A total of 15 conserved motifs were identified in AjTRPs using the MEME online website (Supplementary Figures S2, 3). Motif 4 contained TRP box 1 and motif 12 contained TRP box 2. They were part of the TRP domains and mainly distributed in the TRPC and TRPM subfamilies, indicating that the TRP domain plays an important role in the TRP family. Motif 1, motif 3, and motif 13 were the most widely distributed motifs, existing in more than 20 TRPs. As shown in Supplementary Figure S4, TRP domain was mainly distributed among members of the TRPC and TRPM subfamilies. TRPA1-6, TRPA1-7, and TRPV5 also had TRP domain. The conserved amino acid sequences of TRP box1 and TRP box2 were mainly WKFQR and PPPF respectively. In addition, we found some conserved residues named by Cabezas-Bratesco et al. (2022) distributed in AjTRPA1-6, AjTRPA1-7, AjTRPC4-2, AjTRPM1-1, AjTRPM1-2, AjTRPM1-3, AjTRPM2-1, AjTRPM2-2, AjTRPV5, and AjTRPV6; the identities of 434, 441, and 666 residues (according to rTRPV1 numbering) were relatively low in A. japonicus.
3.3 TRPs distribution across chromosomes of A. japonicus
Chromosome localization of TRP genes in A. japonicus was analyzed using TBtools (Supplementary Figure S5). The results showed that 54 TRP genes were distributed on 16 chromosomes, and the distribution was not uniform in quantity. TRPA subfamily members were mainly distributed on chromosomes 8 and 12. TRPP subfamily members were mainly found on chromosomes 3 and 22. The receptor for egg jelly mapped to five chromosomes (2, 5, 7, 14, and 22). TRPM subfamily members were mainly distributed on chromosomes 3 and 5. No TRP genes were found on chromosomes 1, 11, 13, 15, 16, 18, or 23. Chromosomes 12 and 22 had the most TRP members (7) followed by chromosome 14 (6).
3.4 Phylogenetic analysis of TRPs
Phylogenetic analysis was conducted combining TRPs identified from A. japonicus and several other representative species. WAG+G+I+F model was used for maximum likelihood. As shown in Figure 4, a total of 54 TRPs were mainly divided into six subfamilies, namely TRPA, TRPM, TRPC, TRPV, TRPML, and TRPP. Overall, most TRPs were clustered into their corresponding subfamilies. The TRP subfamily members of A. japonicus were firstly clustered with the corresponding subfamilies of other echinoderms including S. purpuratus and A. planci. TRPPs and receptors for egg jelly were in the branch of TRPP subfamily. The bootstrap support value in most nodes was ≥ 70, which shows that the clustering results of the phylogenetic tree are reliable.
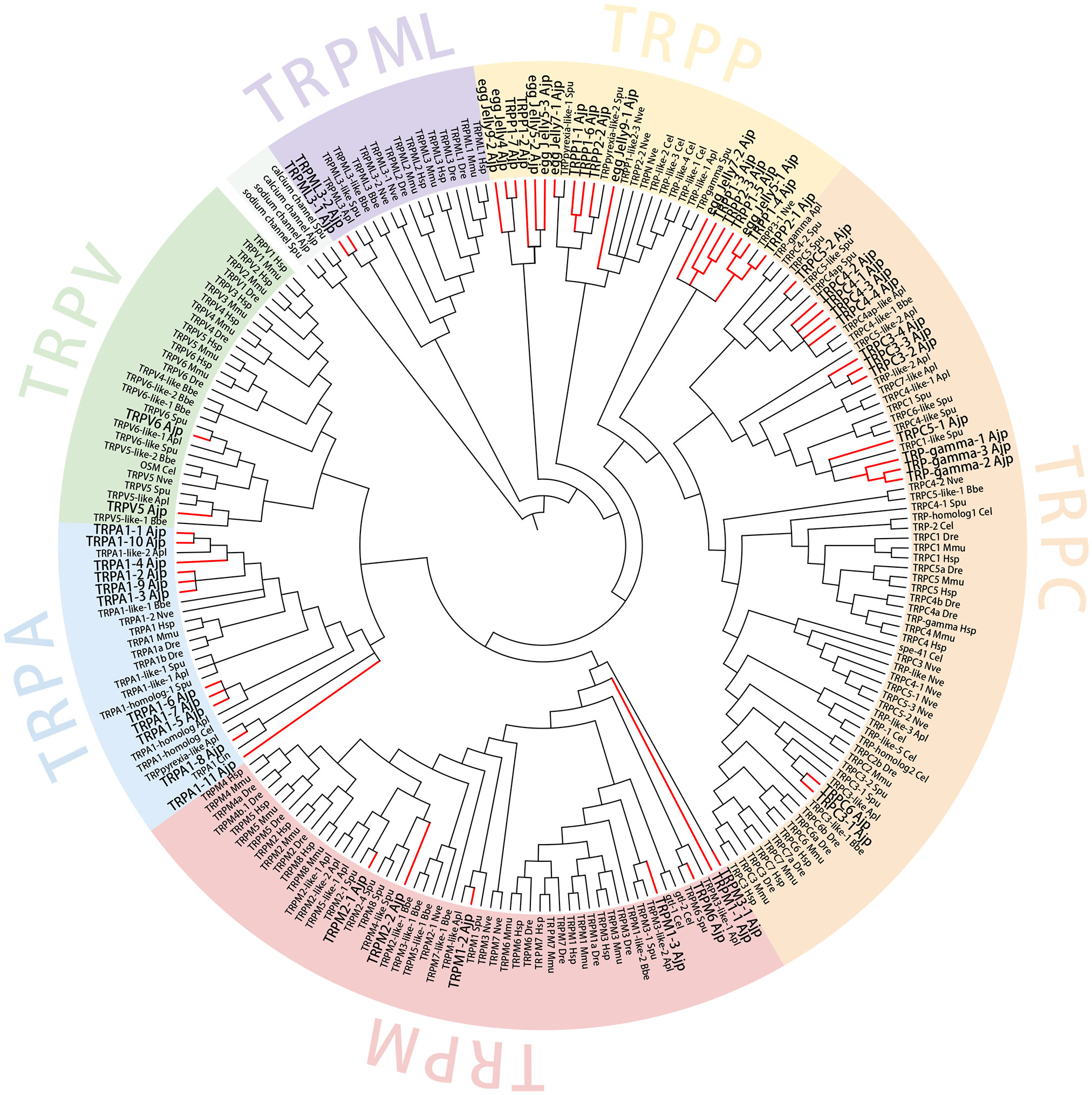
Figure 4 Phylogenetic analysis of TRP genes. AjTRPs are highlighted with red branches and enlarged font. Sodium channel protein of A. japonicus and S. purpuratus and calcium channel protein of A. japonicus and S. purpuratus sequences were included as outgroups. Accession numbers for all sequences are provided in Supplementary Table S1. Hsp, Homo sapiens; Mmu, Mus musculus; Dre, Danio rerio; Cel, Caenorhabditis elegans; Bbe, Branchiostoma belcheri; Apl, Acanthaster planci; Spu, Strongylocentrotus purpuratus; Nve, Nematostella vectensis; Ajp, Apostichopus japonicus.
In order to compare the difference in TRPs copy numbers between invertebrates and vertebrates, we counted the copy numbers of TRPs in a variety of vertebrates and invertebrates. We found that the copy numbers of TRP in invertebrates were larger than in higher vertebrates and this phenomenon was most obvious in marine invertebrates (Table 1). For example, S. purpuratus TRPA1 had 39 copies, E. pallida TRPA1 had 33 copies, C. gigas TRPM2 had 21 copies, and C. virginica TRPM2 had 18 copies. Notably, most TRPs in vertebrates had only one copy. For A. japonicus, many AjTRPs had multiple copies except for AjTRPC6, AjTRPM3, AjTRPM6, AjTRPV5, and AjTRPV6.
3.5 Relative expression of AjTRPs in response to heat stress
The pictures of A. japonicus intestine kept at control conditions and chronic heat stress (CH)-3 are shown in Supplementary Figure S4. Once A. japonicus entered aestivation, the gradual degeneration of the intestine was observed, the mucous epithelium and muscular layer were significantly thinner than that in control group, the volume of respiratory tree became smaller, and the tissue structure did not deteriorate obviously. Histological description of intestine and respiratory tree of the sea cucumbers kept at the different heat conditions have been reported previously (Li and Wang, 2007; Su et al., 2012). The expression profiles of potential temperature-sensitive AjTRP genes in coelomocytes, nerve ring, and intestine under different heat stress treatments were examined by qRT-PCR (Figure 5). As shown in Figure 5, different AjTRPs have different reactions to temperature changes. Under acute heat stress (AH), the expression levels of AjTRPM2-1 were up-regulated significantly (p<0.05) in coelomocytes, nerve ring, and intestine. Notably, the expression levels of AjTRPM2-1 in AH were about 30 times that in the control group (CO) in coelomocytes. And the expression levels of AjTRPM2-1 in CH-3 were significantly higher than the control, CH-1, and CH-2 groups (p<0.05) in nerve ring and intestine. AjTRPM3 showed the highest expression levels in coelomocytes in the CH-3 group (p<0.05), which suggested that AjTRPM2-1 and AjTRPM3 are potential thermo TRPs of A. japonicus. The expression levels of AjTRPM2-2 and AjTRPC3-1 were also up-regulated significantly (p<0.05) in nerve ring in AH. In addition, the expression levels of AjTRPM2-2 and AjTRPC3-1 down-regulated significantly (p<0.05) in CH-1 in coelomocytes. AjTRPA1-2 was down-regulated significantly (p<0.05) in the CH-1 and CH-3 group in coelomocytes. AjTRPA1-2 showed the lowest expression levels in nerve ring in the CH-1 group (p<0.05). The expression levels of AjTRPM3 in nerve ring and intestine and AjTRPA1-2 and AjTRPC3-1 in intestine have no significant difference (p≥0.05) and showed an irregular change.
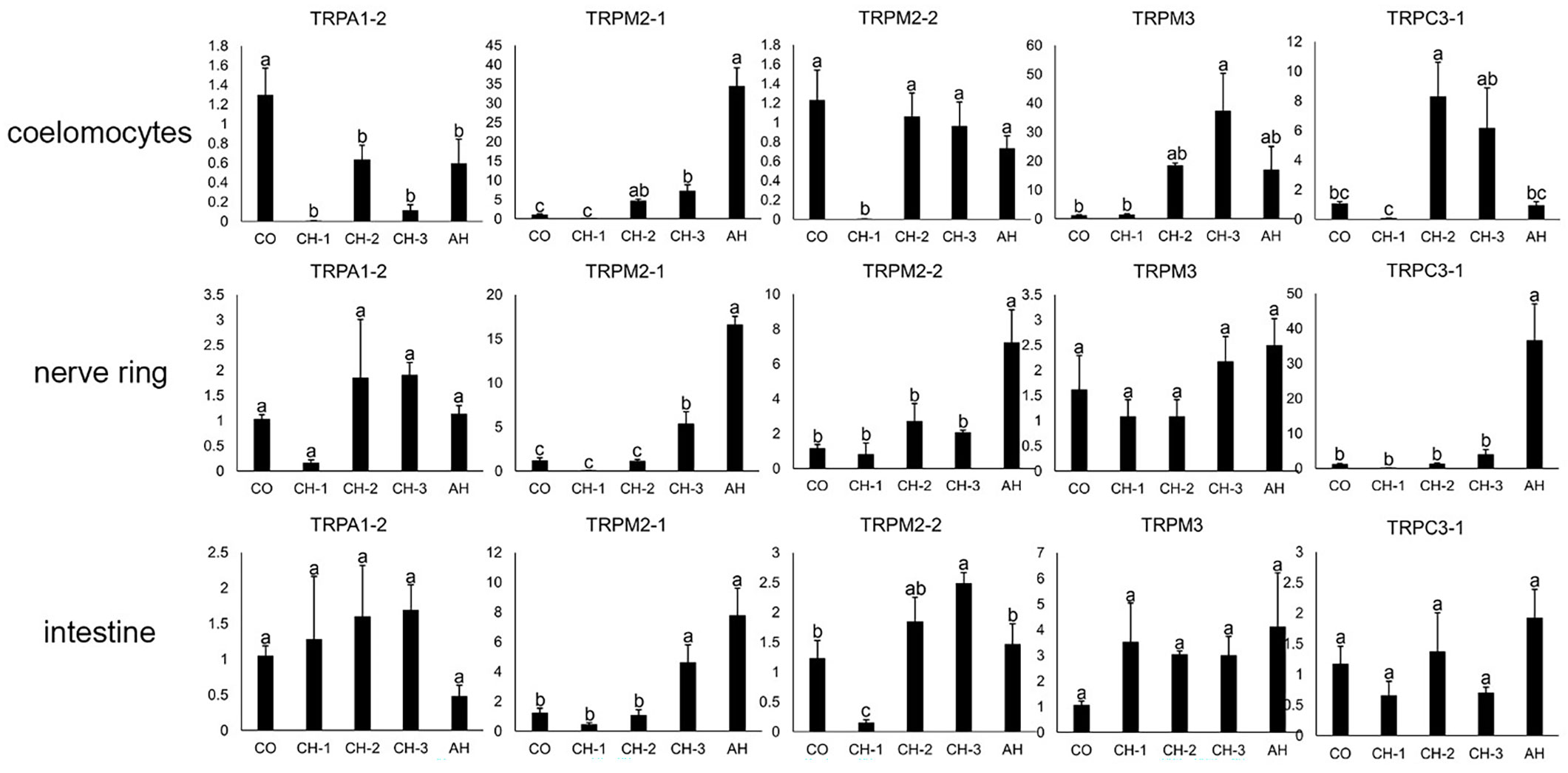
Figure 5 The relative expression level of TRPs in response to different temperature treatments. CO: control group, CH-1: chronic heat stress-1 group (temperature was 22°C), CH-2: chronic heat stress-2 group (maintained at 26°C for 1 day), CH-3: chronic heat stress-3 group (maintained at 26°C for 16 days), AH: acute heat stress group (temperature was raised with the rate of 2°C/h to 30°C for 48 hours). The relative expression levels as vertical coordinate. Data are presented as the mean ± S.E.M. (n = 5). β-actin and β-tubulin were selected as housekeeping genes. Significance testing used one-way ANOVA with Tukey’s Multiple Range Test and p<0.05 accepted as a significant difference. Significant differences are indicated on the bar with different lower-case letters.
4 Discussion
4.1 The characterization and numbers of AjTRPs
The sea cucumber A. japonicus represents a group of important marine invertebrates found in coastal and marine ecosystems (Uthicke, 2001), and is mainly distributed in temperate waters between 35°N and 44°N of the Northwest Pacific Ocean (Liao, 1997). Their ability to survive in frequently changeable marine environments and their special behavior of aestivation make them an ideal model for studying the molecular mechanism of perceiving and adapting to the changes in the marine environment. As molecular sensors, TRPs help animals to perceive external environmental signals and can be activated by sight, heat, touch, smell, and hearing (Perraud et al., 2001; Perraud et al., 2003; Tracey et al., 2003; Kang et al., 2011; Zhan et al., 2016). These channels have been reported to play essential roles in diverse physiological process such as embryonic development, intestinal peristalsis, blood pressure regulation, body fluid balance, cell growth, and apoptosis (Miller, 2006; Dadon and Minke, 2010). However, studies of TRPs in marine invertebrates are very rare and so far are focused mainly on molluscan species such as oysters and scallops and their responses to changeable temperatures (Fu et al., 2021; Peng et al., 2021).
In the present study, a total of 54 TRPs were identified in the sea cucumber A. japonicus. The common features of TRP superfamily members include six transmembrane segments, varying degrees of sequence homology, and permeability to cations. The classification of TRP superfamily is mainly based on the differences of amino acid sequences and topological structures (Dadon and Minke, 2010). TRPC, TRPV, TRPM, TRPN, and TRPA bear the strongest sequence homology to the founding member of the superfamily in Drosophila TRP (Montell and Rubin, 1989). With the exception of the TRPM channels, these subfamilies all have multiple N-terminal ankyrin repeats (Li, 2017). TRPP and TRPML share sequence homology over the transmembrane segments and contain a large loop separating the first two transmembrane domains (Venkatachalam and Montell, 2007). Most of the AjTRPs were clustered together with their orthologs in other echinoderms, the topological structure of the phylogenetic tree being consistent with previous studies (Fu et al., 2021; Peng et al., 2021). Lengths of these 54 TRP proteins ranged from 114 to 3363 amino acids and molecular mass ranged from 12.35 kDa to 376.61 kDa, which is consistent with findings for other marine invertebrates such as C. gigas (Fu et al., 2021) and C. farreri (Peng et al., 2021). AjTRP channels are mainly contained in the transmembrane domain and ion-trans domains reflecting the basic characteristics of transmembrane ion channels. The ANK domain is mainly distributed in the TRPA subfamily and the PKD domain is mainly found in the TRPP subfamily. This suggests the conservation of structure and function of AjTRP channels. The TRP box is very important for ligand activation of TRPs (Taberner et al., 2014; Mori et al., 2022). Aligning multiple sequences in this study showed that the TRP box is mainly distributed in the TRPA, TRPC, and TRPM subfamilies, although certain key amino acids were different. These key amino acids may change the conformation of the TRP channels through hydrogen bonding and further change the stability of the opening state (Taberner et al., 2014; Mori et al., 2022). Cabezas-Bratesco et al. (2022) reported that conserved fingerprint composed of 12 residues in TRP channels played an important role in maintaining proper channel activity. The sequence alignment showed that the fingerprint was distributed in AjTRPA1-6, AjTRPA1-7, AjTRPC4-2, AjTRPM1-1, AjTRPM1-2, AjTRPM1-3, AjTRPM2-1, AjTRPM2-2, AjTRPV5, and AjTRPV6. Interestingly, we found Nudix domain as an important domain for TRPM2 to function was lost in one isoform but existed in others. This phenomenon was also observed in other echinoderm species. Interesting to note, we found that some domains existed specifically in echinoderm TRP channels. For example, A. planci TRPC4 contained Smc (Losada and Hirano, 2005) and Spc7 (Kerres et al., 2004) domains that were usually present in cell cycle. A Lebercilin domain related to light response (Boldt et al., 2011) suggested that the TRPC subfamily may work as a light receptor in echinoderms. TRPC7 of A. planci not only had Smc and Spc7 domains, but also contained a Cast domain that is involved in determining the site of synaptic vesicle fusion (Deguchi-Tawarada et al., 2004) and a dapto-LiaX domain involved in the process of the innate immune response (Khan et al., 2019). However, we did not find these domains in AjTRPs. AjTRPA1-2 contained an Atrophin-1 domain that is related to neurodegenerative disorders (Nucifora et al., 2001). The specific existence of these domains in echinoderms suggested that TRPs might have some potential special functions in these animals.
The TRP superfamily members and copy numbers of each TRP channel are highly variable. In vertebrates, the TRP superfamily consists of more members and each TRP member has only one copy (Wes et al., 1995; Montell, 2005; Venkatachalam and Montell, 2007) whereas in invertebrates each TRP member has multiple copies, but more TRP members were lost as compared with vertebrates. In addition, compared with terrestrial animals, aquatic animals have more copies of TRP members. For example, M. musculus has 30 TRP family members and each member only has one copy, whereas A. japonicus only has 15 TRP family members, most of which have multiple copies: AjTRPA1 had 11 copies, AjTRPP1 seven, AjTRPC3 four, and AjTRPC4 four. This phenomenon was also observed in other invertebrates including marine species (Hu et al., 2019). The number of TRPs in different species showed the plastic evolutionary history of TRP genes. And some TRPs have undergone the expansion or loss in marine invertebrates (Peng et al., 2015). For the invertebrates, especially marine invertebrates, diverse strategies including increased numbers of TRP copies are important for dealing with a highly variable marine environment.
4.2 TRPA subfamily
TRPA1 is generally activated by nociceptive hypothermia as a cold receptor, but is heat-activated in a few animals such as the rattlesnake and is heat-insensitive in humans (Chen et al., 2013). In addition, the electrophilic substances produced by light could inhibit the feeding behavior of insects when combined with TRPA1 (Du et al., 2016). The function of TRPA1 is species-dependent. It has been reported that echinoderm TRPA1 was involved in thermosensing and regulation of ingestion and digestion behaviors (Saito et al., 2017; Ding et al., 2022). TRPA1 positive nociceptive receptors further activated downstream receptors such as tachykinin, neurokinin A, and calcitonin-related peptide to trigger pain and mediate inflammation (Bu et al., 2021). In the present study, AjTRPA1-2 expression levels were only significantly down-regulated in nerve ring under CH-1 and coelomocytes under CH-1 and CH-3, and no significant change was detected in the intestine, which suggested that AjTRPA1-2 had no obvious response to heat stress. One possibility is that the heat sensing function of AjTRPA1 may be performed by other isoforms. Differences in thermal sensitivity of TRPA1 isoforms also existed in D. melanogaster (Du et al., 2016). Another possibility is that AjTRPA1 is activated by nociceptive hypothermia as a cold receptor as in rats and mice (Ding et al., 2022).
4.3 TRPC subfamily
In vertebrates, the TRPC subfamily members are related to the activation of transcription factors (Heineke and Molkentin, 2006), cell proliferation and death (Asghar et al., 2015; Faouzi et al., 2016), synaptic formation and neurotransmitter release (Sukumaran et al., 2017), and other important physiological and pathological regulatory processes. In the present study, we observed the highest expression of TRPC4-2 in tube foot, TRPC5-2 in tentacles, and TRPC6 in papilla, suggesting their potential functions are related to sensory activities. This is also supported by research on the Zhikong scallop C. farreriy that identified the expression of TRPC in eyes and suggested a potential light perception function for this channel (Peng et al., 2021). AjTRPC3-1, which is mainly distributed in coelomocytes in sea cucumbers and up-regulated significantly under CH-2 and CH-3, may be related to immunity in A. japonicus, just like TRPC participates in T lymphocyte apoptosis through the PLC-IP3 signaling pathway in rats (Wu et al., 2015). AjTRPC4-2 was detected as highly expressed in the nerve ring, suggesting that AjTRPC4-2 might be involved in neurophysiological functions. Previous studies also reported that TRPC contributed to neuronal cell death (Sun et al., 2018). In addition, the expression levels of AjTRPC3-1 in nerve ring were low under normal physiological conditions, but up-regulated significantly under acute heat stress. Therefore, the high expression of AjTRPC3 may be related to temperature sensing by regulating neuronal functions (Chrétien et al., 2017).
4.4 TRPM subfamily
The TRPM subfamily including TRPM1-8 is involved in a variety of physiological and pathological processes (Bae et al., 2018). It has been reported that TRPM2, TRPM3, TRPM4, TRPM5, and TRPM8 participate in biological thermoregulation as classical thermoreceptors (Pertusa et al., 2012). TRPM2 and TRPM8 as warm/cold temperature sensors respectively were extensively studied in H. sapiens and M. musculus. Temperature thresholds for TRPM8 are synergistically increased by the activator menthol (McKemy et al., 2002). In addition, several endogenous factors including redox signaling, cytosolic Ca2+, adenosine diphosphate ribose (ADPR), and exogenous H2O2 are reported to be involved in regulating the activity of TRPM2 (Kashio and Tominaga, 2017). In the present study, we identified two thermo TRPM members, TRPM2 and TRPM3, in A. japonicus; TRPM2 had multiple copies. The relative expression levels of AjTRPM2-1 were found to be very sensitive to acute heat stress. The expression levels of AjTRPM2-2 in nerve ring and AjTRPM2-1 in coelomocytes, nerve ring, and intestine were all up-regulated with an increase in environmental temperature. The expression levels of AjTRPM3 were up-regulated in coelomocytes under chronic heat stress. Therefore, we speculated that AjTRPM2 and AjTRPM3 may work as thermoreceptors for temperature perception of A. japonicus, which would also provide a unique perspective for sea cucumber aestivation research. The photoreceptor proteins of the sea urchin S. purpuratus and other echinoderms are mainly distributed in the tube foot. Similarly, the highest expression of A. japonicus TRPM1-1 was in the tube foot and this indicates that A. japonicus TRPM1 may have a potential function in light perception, which is consistent with a previous study that linked TRPM1 to vision (Audo et al., 2009).
4.5 TRPV subfamily
The TRPV subfamily includes six members. TRPV1, -V2, -V3, and -V4 are regulated by physical factors such as hypotonicity, stretch, and temperature (Yin and Kuebler, 2010; Kuipers et al., 2012). TRPV1 is a founding member of TRP channels, directly activated by temperatures greater than 43°C, acidic pH less than 6.0, capsaicin, and a variety of endogenous lipid metabolic products in Rattus norregicus (Venkatachalam and Montell, 2007). TRPV channels are polymodally activated and are capable of integrating various stimuli (Venkatachalam and Montell, 2007). TRPV5 and TRPV6 are essential for osteoclastic bone resorption and Ca2+ reabsorption and are activated by fluid flow (van der Eerden et al., 2005). In the present study, only TRPV5 and TRPV6 were identified as highly expressed in papilla and coelomocytes of A. japonicus. It has been reported that papilla of A. japonicus are located on the back of the body-wall and are involved in connecting the water-vascular system to the outside world (Zhou et al., 2016). Based on this information, we speculate that AjTRPV5 and AjTRPV6 may be involved in pH (Hoenderop et al., 1999) and/or fluid flow perception (Cha et al., 2013).
4.6 TRPN subfamily
TRPN channels were reported to be mechanosensation molecules and mainly play a role in hearing and touching in invertebrates (Göpfert et al., 2006). However, in the present study, we did not find this family in echinoderms. We presume that the mechanosensation of TRPN in A. japonicus may be replaced by another TRP channel. Potential candidates may include TRPA1 (Lennertz et al., 2011), the TRPV subfamily (Yin and Kuebler, 2010), the TRPP subfamily (Nauli et al., 2003), or TRPML3 (Cuajungco and Samie, 2008).
4.7 TRPP subfamily
Mutations in TRPP1 and TRPP2 have been reported to lead to polycystic kidney disease in adult mammals (Hofherr and Köttgen, 2011). In the present study, we also identified eight A. japonicus orthologs of SpREG which were named as receptors for egg jelly. Previous studies have also reported that TRPP can form acid-sensitive channel receptors (Ishimaru et al., 2006) and plays an important role in the development of retina and hair cells (Steigelman et al., 2011). The high expression of TRPP in the nerve ring (around the mouth and tentacles which is related to ingestion) found in our study also suggest that TRPP2 has potential functions in taste sensation or the formation of ciliated cells.
4.8 TRPML subfamily
The mechanosensation function of TRPML3 is still disputed. Cuajungco and Samie (2008) demonstrated that loss of TRPML3 caused deafness in mice whereas Jörs et al. (2010) reported that its loss did not cause deafness or other obvious change. TRPML3 as a salty taste receptor and other physiological roles were reported by Castiglioni et al. (2011) but still with an absence of convincing evidence. In the present study, we observed the highest expression levels of TRPML3 in tube foot and nerve ring but further study is needed to confirm the potential function of AjTRPML3.
5 Conclusions
In this study, we identified 54 AjTRP genes and investigated the expression profiles of different AjTRP members. We found that the representative 15 AjTRP genes belonging to six subfamilies were expressed in the six selected tissues and differed in their expression levels. In addition, we found that AjTRPM2-1, AjTRPM2-2, TRPM3, and AjTRPC3-1 may be heat inducible according to the expression levels of these genes under control, chronic, or acute heat stress. Compared with vertebrate species, each invertebrate TRP member contained multiple copies, but some TRP members were lost. In the future, we need to explore the activation characteristics of AjTRP by combining Calcium Imaging and Patch-clamp Techniques, and further verify the results by behavioral observation. In addition, TRPs can form homo- and heterotetramers. One TRP member mutant could affect cation entry mediated by other TRPs (Hofmann et al., 2002; Staruschenko et al., 2010). The interactions between TRPs could give rise to a novel function that is different from the functions of a single TRP (Hofmann et al., 2002). Therefore, the potential interactions between TRPs should not be ignored. In conclusion, this study enriches the knowledge of TRP-mediated sensory systems in echinoderms and provides a new research perspective for clarifying the mechanism of aestivation of A. japonicus.
Data availability statement
The data presented in the study are deposited in the NCBI database repository, accession number OP470643 - OP470696.
Author contributions
MC originally conceived the study. XW and YW conducted the experiments. MC, XW, YW and YW contributed to the acquisition of data. MC, XW and YW contributed to the interpretation of the data. MC and XW wrote the first draft of the manuscript and KS revised the manuscript. All authors contributed to manuscript revision, read and approved the submitted version.
Funding
This work was supported by National Natural Science Foundation of China (42276103 and 31972767).
Conflict of interest
The authors declare that the research was conducted in the absence of any commercial or financial relationships that could be construed as a potential conflict of interest.
Publisher’s note
All claims expressed in this article are solely those of the authors and do not necessarily represent those of their affiliated organizations, or those of the publisher, the editors and the reviewers. Any product that may be evaluated in this article, or claim that may be made by its manufacturer, is not guaranteed or endorsed by the publisher.
Supplementary material
The Supplementary Material for this article can be found online at: https://www.frontiersin.org/articles/10.3389/fmars.2023.1142982/full#supplementary-material
Supplementary Figure 1 | (A) Diagram showing the anatomy of A. japonicus. CN, nerve ring; T, tentacle; TF, tube foot; IN, intestine; PA, papilla. (B) The enlarged drawing of tentacle, papilla, and tube foot. (C) The intestine anatomy of A. japonicus. (D) The anatomy of A. japonicus without intestine.
Supplementary Figure 2 | Protein motifs of TRP superfamily members in A. japonicus. Conserved amino acid sequences of motif 1, motif 3, motif 4, motif 12, and motif 13. The size of letters indicates the degree of amino acid conservation at this site. The larger the letter, the more conservative the amino acid at this site.
Supplementary Figure 3 | Distribution of conserved motifs in A. japonicus TRPs.
Supplementary Figure 4 | Multi-sequence comparison of the TRPs domain and fingerprint residues of AjTRPs. (A) Multi-sequence comparison of the TRPs domain. (B) Multi-sequence comparison of the fingerprint residues. Φ denotes six carbon aromatic residues (i.e., Tyr or Phe). X denotes any amino acid residue. rTRPV1, transient receptor potential cation channel, subfamily V, member 1 [Rattus norvegicus (Norway rat)]. The conserved residues in box 1, box 2, and fingerprint residues are shown in black and gray shadows.
Supplementary Figure 5 | Distribution of TRP genes across the chromosomes of A. japonicus. The scale on the left is in million bases (Mb). Chromosome numbers are shown at the top of each vertical bar. Genomic locations of TRP genes are marked with the black lines.
Supplementary Figure 6 | Intestinal anatomy of A. japonicus in (A) control group and (B) chronic heat stress-3.
References
Asghar M. Y., Magnusson M., Kemppainen K., Sukumaran P., Löf C., Pulli I., et al. (2015). Transient receptor potential canonical 1 (TRPC1) channels as regulators of sphingolipid and vegf receptor expression: implications for thyroid cancer cell migration and proliferation. J. Biol. Chem. 290, 16116–16131. doi: 10.1074/jbc.M115.643668
Audo I., Kohl S., Leroy B. P., Munier F. L., Guillonneau X., Mohand-Saïd S., et al. (2009). TRPM1 is mutated in patients with autosomal-recessive complete congenital stationary night blindness. Am. J. Hum. Genet. 85, 720–729. doi: 10.1016/j.ajhg.2009.10.013
Bae C., Jara-Oseguera A., Swartz K. J. (2018). TRPM channels come into focus. Science. 539, 160–161. doi: 10.1126/science.aar6205
Boldt K., Mans D. A., Won J., van Reeuwijk J., Vogt A., Kinkl N., et al. (2011). Disruption of intraflagellar protein transport in photoreceptor cilia causes leber congenital amaurosis in humans and mice. J. Clin. Invest. 121, 2169–2180. doi: 10.1172/JCI45627
Boyko A. V., Girich A. S., Eliseikina M. G., Maslennikov S. I., Dolmatov I. Y. (2019). Reference assembly and gene expression analysis of Apostichopus japonicus larval development. Sci. Rep. 9 (1), 1131. doi: 10.1038/s41598-018-37755-5
Branch T. A., DeJoseph B. M., Ray L. J., Wagner C. A. (2013). Impacts of ocean acidification on marine seafood. Trends Ecol. Evol. 28, 178–186. doi: 10.1016/j.tree.2012.10.001
Bronstein O., Kroh A. (2019). The first mitochondrial genome of the model echinoid lytechinus variegatus and insights into odontophoran phylogenetics. Genomics 111 (4), 710–718. doi: 10.1016/j.ygeno.2018.04.008
Bu F., Zhang Y., Xie j. (2021). Research progress of trpa1 ion channel and its function. Chin. J. Modern Appl. Pharmacy. 38, 1618–1626. doi: 10.13748/j.cnki.issn1007-7693.2021.13.014
Bycroft M., Bateman A., Clarke J., Hamill S. J., Sandford R., Thomas R. L., et al. (1999). The structure of a PKD domain from polycystin-1: implications for polycystic kidney disease. EMBO J. 18 (2), 297–305. doi: 10.1093/emboj/18.2.297
Cabezas-Bratesco D., Mcgee F. A., Colenso C. K., Zavala K., Granata D., Carnevale V., et al. (2022). Sequence and structural conservation reveal fingerprint residues in TRP channels. ELife 11, e73645. doi: 10.7554/eLife.73645
Castiglioni A. J., Remis N. N., Flores E. N., García-Añoveros J. (2011). Expression and vesicular localization of mouse Trpml3 in stria vascularis, hair cells, and vomeronasal and olfactory receptor neurons. J. Comp. Neurol. 519, 1095–1114. doi: 10.1002/cne.22554
Cha S., Kim J. H., Huang C. (2013). Flow-induced activation of TRPV5 and TRPV6 channels stimulates Ca2+ activated k+ channel causing membrane hyperpolarization. Biochim. Biophys. Acta 1833, 3046–3053. doi: 10.1016/j.bbamcr.2013.08.017
Chen C., Chen H., Zhang Y., Thomas H. R., Frank M. H., He Y., et al. (2020). TBtools: An integrative toolkit developed for interactive analyses of big biological data. Mol. Plant 13, 1194–1202. doi: 10.1016/j.molp.2020.06.009
Chen J., Kang D., Xu J., Lake M., Hogan J. O., Sun C., et al. (2013). Species differences and molecular determinant of TRPA1 cold sensitivity. Nat. Commun. 4, 1–8. doi: 10.1038/ncomms3501
Chen M., Zheng Y., Cong X. (2021). Research advances in neuropeptides of echinoderm. Periodical Ocean Univ. China. 51, 21–34. doi: 10.16441/j.cnki.hdxb.20200163
Chrétien C., Fenech C., Liénard F., Grall S., Chevalier C., Chaudy S., et al. (2017). Transient receptor potential canonical 3 (TRPC3) channels are required for hypothalamic glucose detection and energy homeostasis. Diabetes. 66, 314–324. doi: 10.2337/db16-1114
Clapham D. E. (2003). TRP channels as cellular sensors. Nature. 426, 517–524. doi: 10.1038/nature02196
Clapham D. E., Julius D., Montell C., Schultz G. (2005). International union of pharmacology. XLIX. nomenclature and structure-function relationships of transient receptor potential channels. Pharmacol. Rev. 57, 427–450. doi: 10.1124/pr.57.4.6
Corey D. P., García-Añoveros J., Holt J. R., Kwan K. Y., Lin S. Y., Vollrath M. A., et al. (2004). TRPA1 is a candidate for the mechanosensitive transduction channel of vertebrate hair cells. Nature. 432, 723–730. doi: 10.1038/nature03066
Cosens D. J., Manning A. (1969). Abnormal electroretinogram from a drosophila mutant. Nature. 224, 285–287. doi: 10.1038/224285a0
Cuajungco M. P., Samie M. A. (2008). The varitint-waddler mouse phenotypes and the TRPML3 ion channel mutation: cause and consequence. Pflug Arch. Eur. J. Phy. 457, 463–473. doi: 10.1007/s00424-008-0523-4
Dadon D., Minke B. (2010). Cellular functions of transient receptor potential channels. Int. J. Biochem. Cell Biol. 42, 1430–1445. doi: 10.1016/j.biocel.2010.04.006
Deguchi-Tawarada M., Inoue E., Takao-Rikitsu E., Inoue M., Ohtsuka T., Takai Y. (2004). CAST2: identification and characterization of a protein structurally related to the presynaptic cytomatrix protein CAST. Genes Cells 9, 15–23. doi: 10.1111/j.1356-9597.2004.00697.x
Ding J., Wang H., Li Z., Sun J., Ding P., Chi X., et al. (2019). TRPA1 expression provides new insights into thermal perception by the sea urchin Strongylocentrotus intermedius. Biology. 11, 503. doi: 10.1017/S0025315419000882
Ding J., Wang H., Li Z., Sun J., Ding P., Chi X., et al. (2022). Digestive enzyme activities and gut emptying are correlated with the reciprocal regulation of TRPA1 ion channel and serotonin in the gut of the sea urchin Strongylocentrotus intermedius. Biology. 11, 503. doi: 10.3390/biology11040503
Du E. J., Ahn T. J., Wen X., Seo D. W., Na D. L., Kwon J. Y., et al. (2016). Nucleophile sensitivity of drosophila TRPA1 underlies light-induced feeding deterrence. eLife. 5, e18425. doi: 10.7554/eLife.18425
Faouzi M., Hague F., Geerts D., Ay A. S., Potier-Cartereau M., Ahidouch A., et al. (2016). Functional cooperation between KCa3.1 and TRPC1 channels in human breast cancer: Role in cell proliferation and patient prognosis. Oncotarget. 7 (24), 36419–36435. doi: 10.18632/oncotarget.9261
Flockerzi V., Nilius B. (2014). TRPs: truly remarkable proteins. Handb. Exp. Pharmacol. 222, 1–12. doi: 10.1007/978-3-642-54215-2_1
Frölicher T. L., Fischer E. M., Gruber N. (2018). Marine heatwaves under global warming. Nature 560, 360–364. doi: 10.1038/s41586-018-0383-9
Fu H., Jiao Z., Li Y., Tian J., Ren L., Zhang F., et al. (2021). Transient receptor potential (TRP) channels in the pacific oyster (Crassostrea gigas): Genome-wide identification and expression profiling after heat stress between C. gigas and C. angulata. Int. J. Mol. Sci. 22, 3222. doi: 10.3390/ijms22063222
Göpfert M. C., Albert J. T., Nadrowski B., Kamikouchi A. (2006). Specification of auditory sensitivity by drosophila TRP channels. Nat. Neurosci. 9, 999–1000. doi: 10.1038/nn1735
Gunaratne H. J., Moy G. W., Kinukawa M., Miyata S., Mah S. A., Vacquier V. D. (2007). The 10 sea urchin receptor for egg jelly proteins (SpREJ) are members of the polycystic kidney disease-1 (PKD1) family. BMC Genomics 8, 235. doi: 10.1186/1471-2164-8-235
Hall M. R., Kocot K. M., Baughman K. W., Fernandez-Valverde S. L., Gauthier M., Hatleberg W. L., et al. (2017). The crown-of-thorns starfish genome as a guide for biocontrol of this coral reef pest. Nature. 544, 231–234. doi: 10.1038/nature22033
Heineke J., Molkentin J. D. (2006). Regulation of cardiac hypertrophy by intracellular signalling pathways. Nat. Rev. Mol. Cell Bio. 7, 589–600. doi: 10.1038/nrm1983
Hennebert E., Leroy B., Wattiez R., Ladurner P. (2015). An integrated transcriptomic and proteomic analysis of sea star epidermal secretions identifies proteins involved in defense and adhesion. J. Proteomics 128, 83–91. doi: 10.1016/j.jprot.2015.07.002
Hinman A., Chuang H. H., Bautista D. M., Julius D. (2006). TRP channel activation by reversible covalent modification. Proc. Natl. Acad. Sci. U.S.A. 103, 19564–19568. doi: 10.1073/pnas.0609598103
Hoenderop J. G., van der Kemp A. W., Hartog A., van de Graaf S. F., van Os C. H., Willems P. H., et al. (1999). Molecular identification of the apical Ca2+ channel in 1, 25-dihydroxyvitamin D3-responsive epithelia. J. Biol. Chem. 274, 8375–8378. doi: 10.1074/jbc.274.13.8375
Hoenderop J. G., Voets T., Hoefs S., Weidema F., Prenen J., Nilius B., et al. (2003). Homo- and heterotetrameric architecture of the epithelial Ca2+ channels TRPV5 and TRPV6. EMBO J. 22, 776–785. doi: 10.1093/emboj/cdg080
Hofherr A., Köttgen M. (2011). TRPP channels and polycystins. Adv. Exp. Med. Biol. 704, 287–313. doi: 10.1007/978-94-007-0265-3_16
Hofmann T., Schaefer M., Schultz G., Gudermann T. (2002). Subunit composition of mammalian transient receptor potential channels in living cells. P Natl. Acad. Sci. U.S.A. 99, 7461–7466. doi: 10.1073/pnas.102596199
Hu B., Jin J., Guo A. Y., Zhang H., Luo J., Gao G. (2015). GSDS 2.0: An upgraded gene feature visualization server. Bioinformatics. 31, 1296–1297. doi: 10.1093/bioinformatics/btu817
Hu B., Li M., Yu X., Xun X., Lu W., Li X., et al. (2019). Diverse expression regulation of Hsp70 genes in scallops after exposure to toxic Alexandrium dinoflagellates. Chemosphere. 234, 62–69. doi: 10.1016/j.chemosphere.2019.06.034
Huo D., Sun L., Zhang L., Yang H., Liu S., Sun J., et al. (2019). Time course analysis of immunity-related gene expression in the sea cucumber Apostichopus japonicus during exposure to thermal and hypoxic stress. Fish Shellfish Immun. 95, 383–390. doi: 10.1016/j.fsi.2019.09.073
Ishimaru Y., Inada H., Kubota M., Zhuang H., Tominaga M., Matsunami H. (2006). Transient receptor potential family members PKD1L3 and PKD2L1 form a candidate sour taste receptor. P Natl. Acad. Sci. U.S.A. 103, 12569–12574. doi: 10.1073/pnas.0602702103
Jörs S., Grimm C., Becker L., Heller S. (2010). Genetic inactivation of TRPML3 does not lead to hearing and vestibular impairment in mice. PloS One 5, e14317. doi: 10.1371/journal.pone.0014317
Kang K., Panzano V. C., Chang E. C., Ni L., Dainis A. M., Jenkins A. M., et al. (2011). Modulation of TRPA1 thermal sensitivity enables sensory discrimination in drosophila. Nature. 481, 76–80. doi: 10.1038/nature10715
Kashio M., Tominaga M. (2017). The TRPM2 channel: A thermo-sensitive metabolic sensor. Channels (Austin). 11 (5), 426–433. doi: 10.1080/19336950.2017.1344801
Kerres A., Vietmeier-Decker C., Ortiz J., Karig I., Beuter C., Hegemann J., et al. (2004). The fission yeast kinetochore component Spc7 associates with the EB1 family member Mal3 and is required for kinetochore-spindle association. Mol. Biol. Cell. 15, 5255–5267. doi: 10.1091/mbc.e04-06-0443
Khan A., Davlieva M., Panesso D., Rincon S., Miller W. R., Diaz L., et al. (2019). Antimicrobial sensing coupled with cell membrane remodeling mediates antibiotic resistance and virulence in Enterococcus faecalis. P Natl. Acad. Sci. U.S.A. 116, 26925–26932. doi: 10.1073/pnas.1916037116
Kingston A. C., Kuzirian A. M., Hanlon R. T., Cronin T. W. (2015). Visual phototransduction components in cephalopod chromatophores suggest dermal photoreception. J. Exp. Biol. 218, 1596–1602. doi: 10.1242/jeb.117945
Kitchell J. A., Kitchell J. F., Johnson G. L., Hunkins K. L. (1978). Abyssal traces and megafauna: comparison of productivity, diversity and density in the Arctic and Antarctic. Palaeobiology. 4, 171–180. doi: 10.2307/2400285
Kuipers A. J., Middelbeek J., van Leeuwen F. N. (2012). Mechanoregulation of cytoskeletal dynamics by TRP channels. Eur. J. Cell Biol. 91, 834–846. doi: 10.1016/j.ejcb.2012.05.006
Lennertz R. C., Kossyreva E. A., Smith A. K., Stucky C. L. (2011). TRPA1 mediates mechanical sensitization in nociceptors during inflammation. PloS One 7, e43597. doi: 10.1371/journal.pone.0043597
Li H. (2017). TRP channel classification. Adv. Exp. Med. Biol. 976, 1–8. doi: 10.1007/978-94-024-1088-4_1
Li X., Wang X. (2007). The histological observation of alimentary tract and respiratory tree in sea cucumber, Apostichopus japonicus during aestivation induced in lab. J. Dalian Fisheries University. 02, 001.
Liao Y. (1997). FAUNA SINICA, phylum Echinodermata, class holothuroidea Vol. 1997 (Beijing, China: Science Press), 334.
Lin C., Liu X., Sun L., Liu S., Sun J., Yang H. (2020). Effects of different flow velocities on behavior and TRPA1 expression in the sea cucumber. Apostichopus japonicus J. Oceanology Limnology. 38 (04), 1328–1340.
Liu X., Lin C., Sun L., Liu S., Sun J., Yang H. (2020a). Behavioural response of different epithelial tissues of sea cucumber (Apostichopus japonicus) to light and differential expression of the light-related gene Pax6. Mar. Freshw. Behav. Physiol. 53 (2), 73–85.
Liu X., Lin C., Sun L., Liu S., Sun J., Zhang L., et al. (2020b). Transcriptome analysis of phototransduction-related genes in tentacles of the sea cucumber Apostichopus japonicus. Comp. Biochem. Physiol. Part D Genomics Proteomics 34, 100675. doi: 10.1016/j.cbd.2020.100675
Losada A., Hirano T. (2005). Dynamic molecular linkers of the genome: the first decade of SMC proteins. Gene Dev. 19, 1269–1287. doi: 10.1101/gad.1320505
Mactavish T., Stenton-Dozey J., Vopel K., Savage C. (2012). Deposit-feeding sea cucumbers enhance mineralization and nutrient cycling in organically-enriched coastal sediments. PloS One 7, e50031. doi: 10.1371/journal.pone.0050031
Marquet N., Cardoso J., Louro B., Fernandes S. A., Silva S. C., Canário A. (2020). Holothurians have a reduced GPCR and odorant receptor-like repertoire compared to other echinoderms. Sci. Rep. 10 (1), 3348. doi: 10.1038/s41598-020-60167-3
McKemy D. D., Neuhausser W. M., Julius D. (2002). Identification of a cold receptor reveals a general role for TRP channels in thermosensation. Nature 416 (6876), 52–58. doi: 10.1038/nature719
Miller B. A (2006). The Role of TRP channels in oxidative stress-induced cell death. Membr. Biol. 209, 31–41. doi: 10.1007/s00232-005-0839-3
Minke B., Wu C., Pak W. L. (1975). Induction of photoreceptor voltage noise in the dark in drosophila mutant. Nature. 258, 84–87. doi: 10.1038/258084a0
Montell C. (2005). The TRP superfamily of cation channels. Sci. STKE. 2005, re3. doi: 10.1126/stke.2722005re3
Montell C., Rubin G. M. (1989). Molecular characterization of the drosophila trp locus: a putative integral membrane protein required for phototransduction. Neuron. 2, 313–1323. doi: 10.1016/0896-6273(89)90069-x
Mori M. X., Okada R., Sakaguchi R., Hase H., Imai Y., Polat O. K., et al. (2022). Critical contributions of pre-S1 shoulder and distal TRP box in DAG-activated TRPC6 channel by PIP2 regulation. Sci. Rep. 12, 10766. doi: 10.1038/s41598-022-14766-x
Moy G. W., Mendoza L. M., Schulz J. R., Swanson W. J., Glabe C. G., Vacquier V. D. (1996). The sea urchin sperm receptor for egg jelly is a modular protein with extensive homology to the human polycystic kidney disease protein, PKD1. J. Cell Biol. 133 (4), 809–817. doi: 10.1083/jcb.133.4.809
Nauli S. M., Alenghat F. J., Luo Y., Williams E., Vassilev P., Li X., et al. (2003). Polycystins 1 and 2 mediate mechanosensation in the primary cilium of kidney cells. Nat. Genet. 33, 129–137. doi: 10.1038/ng1076
Nilius B., Owsianik G. (2011). The transient receptor potential family of ion channels. Genome Biol. 12, 218. doi: 10.1186/gb-2011-12-3-218
Nucifora F.C. C.OMMAJ.R.X.X.X, Sasaki M., Peters M. F., Huang H., Cooper J. K., Yamada M., et al. (2001). Interference by huntingtin and atrophin-1 with cbp-mediated transcription leading to cellular toxicity. Science. 291, 2423–2428. doi: 10.1126/science.1056784
Oliver E. C. J., Donat M. G., Burrows M. T., Moore P. J., Smale D. A., Alexander L. V., et al. (2018). Longer and more frequent marine heatwaves over the past century. Nat. Commun. 9, 1324. doi: 10.1038/s41467-01803732-9
Passamaneck Y. J., Martindale M. Q. (2013). Evidence for a phototransduction cascade in an early brachiopod embryo. Integr. Comp. Biol. 53, 17–26. doi: 10.1093/icb/ict037
Peng G., Shi X., Kadowaki T. (2015). Evolution of TRP channels inferred by their classification in diverse animal species. Mol. Phylogenet Evol. 84, 145–157. doi: 10.1016/j.ympev.2014.06.016
Peng C., Yang Z., Liu Z., Wang S., Yu H., Cui C., et al. (2021). A systematical survey on the trp channels provides new insight into its functional diversity in zhikong scallop (Chlamys farreri). Int. J. Mol. Sci. 22, 11075. doi: 10.3390/ijms222011075
Perraud A. L., Fleig A., Dunn C. A., Bagley L. A., Launay P., Schmitz C., et al. (2001). ADP-ribose gating of the calcium-permeable LTRPC2 channel revealed by nudix motif homology. Nature. 411, 595–599. doi: 10.1038/35079100
Perraud A. L., Schmitz C., Scharenberg A. M. (2003). TRPM2 Ca2+ permeable cation channels: from gene to biological function. Cell calcium. 33, 519–531. doi: 10.1016/s0143-4160(03)00057-5
Pertusa M., Moldenhauer H., Brauchi S., Latorre R., Madrid R., Orio P. (2012). Mutagenesis and temperature-sensitive little machines. In: Mutagenesis, ed. Rajnikant M. (Rijeka: IntechOpen). 221–246.
Saito S., Hamanaka G., Kawai N., Furukawa R., Gojobori J., Tominaga M., et al. (2017). Characterization of TRPA channels in the starfish Patiria pectinifera: involvement of thermally activated TRPA1 in thermotaxis in marine planktonic larvae. Sci. Rep. 7, 2173. doi: 10.1038/s41598-017-02171-8
Schneider K., Silverman J., Woolsey E., Eriksson H., Byrne M., Caldeira K. (2011). Potential influence of sea cucumbers on coral reef CaCO3 budget: a case study at one tree reef. Geophys. Res. 116, G04032. doi: 10.1029/2011jg001755
Shah M. A., Kirkman L. M., Sitver P. J., Shelley C. (2018). Pharmacological disruption of sea urchin tube foot motility and behavior. Biol. Bull. 234, 96–105. doi: 10.1086/697378
Staruschenko A., Jeske N. A., Akopian A. N. (2010). Contribution of TRPV1-TRPA1 interaction to the single channel properties of the TRPA1 channel. J. Biol. Chem. 285, 15167–15177. doi: 10.1074/jbc.M110.106153
Steigelman K. A., Lelli A., Wu X., Gao J., Lin S., Piontek K., et al. (2011). Polycystin-1 is required for stereocilia structure but not for mechanotransduction in inner ear hair cells. J. Neurosci. 31, 12241–12250. doi: 10.1523/JNEUROSCI.6531-10.2011
Su L., Chen M., Wang T., Liu J., Zhao Y., Yang H. (2012). The division of different stages of aestivation and quantitative analysis of tissue layers thickness of anterior intestine in sea cucumber Apostichopus japonicus. Mar. Sci. 36, 1–5.
Sukumaran P., Sun Y., Schaar A., Selvaraj S., Singh B. B. (2017). TRPC channels and parkinson's disease. Adv. Exp. Med. Biol. 976, 85–94. doi: 10.1007/978-94-024-1088-4_8
Sun D., Ma H., Ma J., Wang J., Deng X., Hu C., et al. (2018). Canonical transient receptor potential channel 3 contributes to febrile seizure inducing neuronal cell death and neuroinflammation. Cell Mol. Neurobiol. 38, 1215–1226. doi: 10.1007/s10571-018-0586-5
Swain P. (2015). In-silico analysis predicting the best model for photosystemiid2 protein of spinaciaolearacea using multiple templates. Comput. Mol. Biol. 4, 1–6. doi: 10.5376/cmb.2014.04.0013
Taberner F. J., López-Córdoba A., Fernández-Ballester G., Korchev Y., Ferrer-Montiel A. (2014). The region adjacent to the c-end of the inner gate in transient receptor potential melastatin 8 (TRPM8) channels plays a central role in allosteric channel. J. Biol. Chem. 289, 28579–28594. doi: 10.1074/jbc.M114.577478
Tracey W. D. J., Wilson R. I., Laurent G., Benzer S. (2003). painless, a drosophila gene essential for nociception. Cell. 113, 261–273. doi: 10.1016/s0092-8674(03)00272-1
Tu Q., Cameron R. A., Worley K. C., Gibbs R. A., Davidson E. H. (2012). Gene structure in the sea urchin Strongylocentrotus purpuratus based on transcriptome analysis. Genome Res. 22 (10), 2079–2087. doi: 10.1101/gr.139170.112
Uthicke S. (2001). Nutrient regeneration by abundant coral reef holothurians. Exp. Mar. Bio. Ecol. 265, 153–170. doi: 10.1016/S0022-0981(01)00329-X
van der Eerden B. C., Hoenderop J. G., de Vries T. J., Schoenmaker T., Buurman C. J., Uitterlinden A. G., et al. (2005). The epithelial Ca2+ channel TRPV5 is essential for proper osteoclastic bone resorption. P Natl. Acad. Sci. U.S.A. 102, 17507–17512. doi: 10.1073/pnas.0505789102
Venkatachalam K., Montell C. (2007). TRP channels. Annu. Rev. Biochem. 76, 387–417. doi: 10.1146/annurev.biochem.75.103004.142819
Walker R. G., Willingham A. T., Zuker C. S. (2000). A drosophila mechanosensory transduction channel. Science. 287, 2229–2234. doi: 10.1126/science.287.5461.2229
Wang Z. (2018). The research on heterosis of molecular mechanism for Chinese sea cucumber,Russian sea cucumber and their hybrids (Dalian, China: Master-Dalian Ocean University).
Wang Y., Yang Y., Li Y., Chen M. (2022b). Identification of sex determination locus in sea cucumber Apostichopus japonicus using genome-wide association study. BMC Genomics 23, 1–14. doi: 10.1186/s12864-022-08632-3
Wang Y., Yin Y., Cong X., Storey K. B., Chen M. (2022a). PacBio isoform sequencing and illumina RNA sequencing provide novel insights on responses to acute heat stress in Apostichopus japonicus coelomocytes. Front. Mar. Sci. 8. doi: 10.3389/fmars.2021.815109
Wes P. D., Chevesich J., Jeromin A., Rosenberg C., Stetten G., Montell C. (1995). TRPC1, a human homolog of a drosophila store-operated channel. P Natl. Acad. Sci. U.S.A. 92 (21), 9652–9656. doi: 10.1073/pnas.92.21.9652
Wu Q., Sun M., Wu C., Li Y., Du J., Zeng J., et al. (2015). Activation of calcium-sensing receptor increases TRPC3/6 expression in T lymphocyte in sepsis. Mol. Immunol. 64, 18–25. doi: 10.1016/j.molimm.2014.10.018
Wu Q., Sun M., Wu C., Li Y., Du J., Zeng J., et al. (2015). Activation of calcium-sensing receptor increases TRPC3/6 expression in T lymphocyte in sepsis. Mol. Immunol. 64, 18–25. doi: 10.1016/j.molimm.2014.10.018
Xiao R., Xu X. Z. S. (2016). Temperature sensation: from molecular thermosensors to neural circuits and coding principles. Annu. Rev. Physiol., 83, 205–230. doi: 10.1146/annurev-physiol-031220-095215
Xu D., Zhou S., Sun L. (2018). RNA-Seq based transcriptional analysis reveals dynamic genes expression profiles and immune-associated regulation under heat stress in Apostichopus japonicus. Fish Shellfish Immun. 78, 169–176. doi: 10.1016/j.fsi.2018.04.037
Yang H., Yuan X., Zhou Y., Mao Y., Zhang T., Liu Y. (2005). Effects of body size and water temperature on food consumption and growth in the sea cucumber Apostichopus japonicus (Selenka) with special reference to aestivation. Aquacult. Res. 36, 1085–1092.
Yin J., Kuebler W. M. (2010). Mechanotransduction by TRP channels: general concepts and specific role in the vasculature. Cell Biochem. Biophys. 56, 1–18. doi: 10.1007/s12013-009-9067-2
Zhan K., Pei-Lin Y., Liu C., Luo J., Yang W. (2016). Detrimental or beneficial: the role of TRPM2 in ischemia/reperfusion injury. Acta Pharmacol. Sin. 37, 4–12. doi: 10.1038/aps.2015.141
Zhao Y., Chen M., Wang T., Sun L., Xu D., Yang H. (2014). Selection of reference genes for qRT-PCR analysis of gene expression in sea cucumber Apostichopus japonicus during aestivation. Chin. J. Oceanology&Limnology. 32, 1248–1256. doi: 10.1007/s00343-015-4004-2
Keywords: TRP channel, Apostichopus japonicus, gene characterization, expression profile, heat stress
Citation: Wang X, Wang Y, Wang Y, Storey KB and Chen M (2023) Characterization and expression profile of transient receptor potential channels in sea cucumber Apostichopus japonicus. Front. Mar. Sci. 10:1142982. doi: 10.3389/fmars.2023.1142982
Received: 12 January 2023; Accepted: 27 March 2023;
Published: 11 April 2023.
Edited by:
Jeremy M. Sullivan, Johns Hopkins University, United StatesReviewed by:
Mohamed Mohsen, Jimei University, ChinaJ. Joe Hull, Agricultural Research Service (USDA), United States
Xuelin Zhao, Ningbo University, China
Yina Shao, Ningbo University, China
Copyright © 2023 Wang, Wang, Wang, Storey and Chen. This is an open-access article distributed under the terms of the Creative Commons Attribution License (CC BY). The use, distribution or reproduction in other forums is permitted, provided the original author(s) and the copyright owner(s) are credited and that the original publication in this journal is cited, in accordance with accepted academic practice. No use, distribution or reproduction is permitted which does not comply with these terms.
*Correspondence: Muyan Chen, Y2hlbm11eWFuQG91Yy5lZHUuY24=