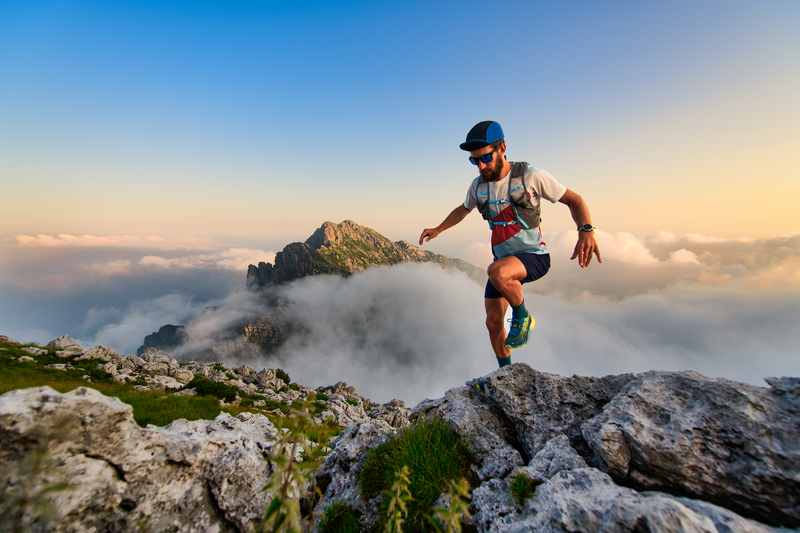
94% of researchers rate our articles as excellent or good
Learn more about the work of our research integrity team to safeguard the quality of each article we publish.
Find out more
ORIGINAL RESEARCH article
Front. Mar. Sci. , 14 February 2023
Sec. Aquatic Physiology
Volume 10 - 2023 | https://doi.org/10.3389/fmars.2023.1141116
This article is part of the Research Topic The defense responses of aquatic animals to the environment View all 7 articles
Freshwater teleosts frequently face the stress of varied ion and pH levels; therefore, they have developed related defense mechanisms to maintain the homeostasis of body-fluid ion and acid-base balance. The different subtypes of ionocytes expressed in the branchial epithelium of adult fish or the skin of larvae are the major sites for fish ion regulation. 1α,25-dihydroxyvitamin D3 (1α,25(OH)2D3), the bioactive form of vitamin D, is a steroid hormone that is involved in the regulation of Ca2+ uptake and acid secretion in teleosts. Our results revealed that 1α,25(OH)2D3 levels were not changed in zebrafish larvae upon exposure to low Na+ freshwater compared to normal freshwater. In contrast, 1α,25(OH)2D3 levels were substantially higher in fish exposed to acidic and low Ca2+ freshwater than in those exposed to normal freshwater. Some hormones regulate ion regulation and acid secretion by modulating ionocyte differentiation and/or proliferation in teleosts; however, the role of vitamin D in this process is unclear. Zebrafish larvae were used as a model in the present study to explore the effect of vitamin D on ionocyte proliferation and/or differentiation. The present study indicated that 1α,25(OH)2D3 treatment increased the number of foxi3a-positive cells, ionocyte progenitors, and mature ionocytes. However, the number of P63-positive epidermal stem cells did not change in the zebrafish larvae treated with 1α,25(OH)2D3. These results revealed that vitamin D exerts a positive effect on the number of ionocytes by increasing the differentiation of ionocytes. Increased ionocyte differentiation by vitamin D is suggested to elevate the capacity of ion regulation and acid secretion in zebrafish to cope with external stress. The present findings indicate the role of vitamin D in the regulation of ionocyte differentiation and provide new insights into the mechanisms of stress adaptation of fish.
Teleosts living in freshwater (FW) environments easily face environmental stresses, such as changeable pH values and ion contents. Ionocytes expressed in the adult gills or skin of larvae are vital sites in fish for ion and acid-base regulation (Evans et al., 2005; Hwang et al., 2011). Previous studies have indicated that the mRNA expression of hormone metabolism genes and hormone levels are modulated in fish upon external changes in ion levels and pH values. Modulated hormone levels are vital for the maintenance of body fluid ions and acid-base homeostasis (Guh et al., 2015; Lin and Hwang, 2016; Yan and Hwang, 2019; Lin et al., 2021). 1α,25-DihydroxyvitaminD3 (1α,25(OH)2D3), a bioactive vitamin D, is a steroid hormone that regulates Ca2+ uptake in vertebrates (Lips, 2006; Lin and Hwang, 2016). In teleosts, the genes for 1α,25(OH)2D3-related metabolic enzymes and receptors have also been identified (Cheng et al., 2006; Goldstone et al., 2010; Lin et al., 2012). As mammals, the fish vitamin D receptor (VDR) can regulate gene expression with 1α,25(OH)2D3 (Howarth et al., 2008). Several studies have revealed the role of 1α,25(OH)2D3 in controlling Ca2+ uptake in fish. In carp (Cyprinus carpiu) and Atlantic cod (Gadus morhua), supplementation with 1α,25(OH)2D3 through feeding or intraperitoneal injection significantly increased plasma Ca2+ level (Swarup et al., 1991; Sundell et al., 1993). In addition, Ca2+ influx was significantly enhanced in zebrafish larvae after immersion in 1α,25(OH)2D3 for three days (Lin et al., 2012). Furthermore, 1α,25(OH)2D3 treatment stimulated gene expression of the epithelial Ca2+ channel (ECaC), a vital transcellular pathway for Ca2+ uptake in ionocytes (Lin et al., 2012). These results revealed that vitamin D can increase the Ca2+ uptake of fish by affecting the gene expression of ECaC.
In addition to controlling Ca2+ uptake, 1α,25(OH)2D3 level were elevated in zebrafish larvae after exposure to acidic freshwater (Lin et al., 2022). When 1α,25(OH)2D3 was used to treat zebrafish larvae, acid secretion increased significantly (Lin et al., 2022). Both types of vitamin D receptor (VDR) have been identified in H+-ATPase rich cells (HRC), the subtype of ionocytes for acid secretion, in zebrafish (Lin et al., 2022). Lin et al. (2022) indicated that two types of VDR are involved in acid secretion. However, some studies have indicated the potential role of vitamin D in the regulation of Na+ uptake. NHE3, which is expressed in a specific ionocyte subtype, is a major transporter for Na+ uptake in fish (Yan and Hwang, 2019). The previous study indicated that 1α,25(OH)2D3 treatment enhanced the gene expression of NHE3 in zebrafish larvae (Lin et al., 2022). In NHE3 knockout mice, the 1α,25(OH)2D3 concentration was significantly higher than that in wild-type mice (Pan et al., 2012). When opossum kidney cells and mouse cortical tubule cells were treated with exogenous 1α,25(OH)2D3, the activity of sodium/hydrogen exchanger 3 (NHE3) was dominantly increased by 35-55% (Binswanger et al., 1993). In Atlantic salmon (Salmo salar), the concentration of body-fluid 1α,25(OH)2D3 was significantly higher in the 50% seawater (SW) smolt than in the FW and SW smolts (Lock et al., 2007). In addition, the mRNA expression of gill VDR was significantly higher in the 50% SW smolt and SW smolt than in the FW smolt (Lock et al., 2007). Ca2+ level is very different between FW and SW; therefore, changes in vitamin D levels and VDR mRNA expression among FW, 50% SW, and SW smolt may be due to variations in external Ca2+ level. However, the Na+ level is different between FW and SW and may also contribute to the changes in vitamin D levels and VDR mRNA expression. Nevertheless, it is still unclear whether the body fluid vitamin D level is regulated by environment Na+ level.
In fish, ionocytes differentiate from epidermal stem cells (Bakkers et al., 2002). The expression of P63, a marker of epidermal stem cells, is induced by bone morphogenetic protein (BMP) signaling and initiates the progression of epidermal stem cell specification (Hsiao et al., 2007). Some P63-positive epidermal cells were then induced to express forkhead box I3a (Foxi3a), a marker of ionocyte progenitor cells (Hsiao et al., 2007; Janicke et al., 2007). Subsequently, ionocyte progenitor cells are further regulated by Foxi3a and differentiate into ionocytes. The differentiating cells later differentiate into different subtypes of ionocytes through the mutual interaction of Foxi3a/-b and Gcm2 (a differentiation marker of acid-secretion ionocytes in zebrafish) (Hsiao et al., 2007; Chang et al., 2009). In fish, some hormones modulate ion and acid-base regulation, mainly by modulating the gene expression of ion transporters and/or the number of ionocytes (Lin and Hwang, 2016; Yan and Hwang, 2019). 1α,25(OH)2D3 level are regulated in zebrafish exposed to externally altered ions and pH values (Lin et al., 2012; Lin et al., 2022). 1α,25(OH)2D3 treatment enhanced the capacity for ion and acid-base regulation by increasing the mRNA expression of ion transporters. 1α,25(OH)2D3 treatment can increase the gene expression of H+-ATPase and ECaC, cell markers for the acid-secreting and Ca2+ uptake ionocytes respectively in zebrafish (Lin et al., 2012; Lin et al., 2022). Nevertheless, the effect of vitamin D on ionocyte proliferation and differentiation in fish remains unclear.
Our previous studies indicated that vitamin D can affect the Ca2+ uptake and acid secretion in zebrafish by influencing the gene expression of ion transporters that are expressed in the ionocytes (Lin et al., 2012; Lin et al., 2022). Some hormones, such as cortisol, isotocin, and stanniocalcin-1 (STC-1), can affect ion regulation and the expression of ion transporters by modulating the ionocyte proliferation and/or differentiation in fish (Yan and Hwang, 2019). To further clarify the potential role and mechanism of vitamin D action in fish ion regulation, we, therefore, designed experiments to explore the effects of externally changing ion concentrations on 1α,25(OH)2D3 level in zebrafish larvae. In addition, the effects of 1α,25(OH)2D3 on ionocyte proliferation and differentiation in zebrafish were examined. Exploring these issues can increase our understanding of the role of vitamin D in fish ion regulation and hence elevate the knowledge of the environmental adaptation mechanisms of fish.
The protocol for animal care and use was approved by the Institutional Animal Care and Utilization Committee of the National Kaohsiung University of Science and Technology (NKUST). The used zebrafish (Danio rerio) were reared in local tap water at 28.5°C under a 14:10-h light-dark photoperiod at the Department of Marine Biotechnology, NKUST, Kaohsiung, Taiwan. Mature zebrafish were paired for breeding in a breeding tank before the experiment, and zebrafish eggs were collected in a Petri dish the next morning.
The zebrafish fertilized eggs at 1-2 cell stage were incubated in normal freshwater (NW, containing 0.5 mM NaCl, 0.2 mM CaSO4, 0.2 mM MgSO4, 0.16 mM KH2PO4, 0.16 mM K2HPO4, and pH value at 7.0), acidic NW (AW, containing 0.5 mM NaCl, 0.2 mM CaSO4, 0.2 mM MgSO4, 0.16 mM KH2PO4, 0.16 mM K2HPO4, and pH value at 4.0), low Na+ NW (LNa, containing 0.05 mM NaCl, 0.2 mM CaSO4, 0.2 mM MgSO4, 0.16 mM KH2PO4, 0.16 mM K2HPO4, and pH value at 7.0), and low Ca2+ NW (LCa, containing 0.5 mM NaCl, 0.02 mM CaSO4, 0.2 mM MgSO4, 0.16 mM KH2PO4, 0.16 mM K2HPO4, and pH value at 7.0) separately. Chemicals purchased from Sigma-Aldrich (St. Louis, MO, USA) were dissolved in ddH2O for each artificial water preparation. The fertilized eggs were incubated until sampling at 2 or 3 days post fertilization (dpf). The medium was changed twice daily.
Whole-body 1α, 25-dihydroxytamin D3 (1α,25(OH)2D3) levels in 3 dpf zebrafish larvae were determined using a 1α, 25(OH)2D3 ELISA kit (Zgenebio Inc., Taipei, Taiwan). 3 dpf zebrafish larvae with NW, AFW, LNa, and LCa treatment were anesthetized by 0.03% MS-222 (Sigma-Aldrich) and then washed several times with 1X phosphate-buffered saline (PBS). Twenty-five larvae were pooled in one vial as a single sample and 6 samples at each treatment group were used for the analysis. Zebrafish larvae in vials were homogenized at 4500 rpm for 1 min (Dynamic homogenizer MS-100, TOMY Digital Biology, Tokyo, Japan). Next, the homogenized homogenates were centrifuged at 15000 rpm at 4°C for 15 min. The supernatant was collected for 1α,25(OH)2D3 and quantified according to the manufacturer’s instructions for the ELISA kit.
Following previous studies (Lin et al., 2012; Lin et al., 2022), fertilized zebrafish eggs were treated with 0 (control) or 20 μg/L 1α,25(OH)2D3 (Sigma-Aldrich). At 2 and 3 dpf, the zebrafish larvae were anesthetized and sampled for subsequent analyses. During the 1α,25(OH)2D3 treatment experiment, neither significant mortality nor abnormal behavior was observed. The incubation medium was changed with a new 1α,25(OH)2D3 solution every day to maintain constant levels of 1α,25(OH)2D3.
3 dpf zebrafish larvae with 0 or 20 μg/L 1α,25(OH)2D3 treatment were anesthetized with 0.03% MS-222 and then briefly rinsed in deionized water. Twenty-five individuals were pooled as 1 sample and 10 samples were analyzed at each group. After the samples were dried at 60°C oven and then HNO3 (13.1 N) was added to samples for digestion at 60°C overnight. Digested solutions were diluted with deionized water, and the total sodium content was measured with a Z-8000 atomic absorption spectrophotometer (Hitachi, Tokyo, Japan). Standard solutions (Merck, Darmstadt, Germany) were used to make the standard curves.
Zebrafish foxi3a, atp6v1a (encoding the α subunit of H+-ATPase), and ecac fragments were obtained by PCR, PCR primer design as previously described (Chou et al., 2011; Lin et al., 2012). The PCR fragment was inserted into the pGEM-T Easy Vector (Promega, Madison, WI, USA). The inserted fragments were amplified with T7 and SP6 primers PCR, and the products were used as templates for in vitro transcription with T7 and SP6 RNA polymerase (Roche) in the presence of digoxigenin (DIG)-UTP (Roche) to synthesize the probes. 2 and 3 dpf zebrafish larvae were anesthetized on ice and fixed with 4% paraformaldehyde (PFA) in a phosphate-buffered saline (PBS; 1.4 mM NaCl, 0.2 mM KCl, 0.1 mM Na2HPO4, and 0.002 mM KH2PO4; pH 7.4) solution at 4°C overnight. Subsequently, we performed in situ hybridization as previously described (Lin et al., 2012). Images were acquired using a Leica M205 microscope (Leica). We follow previous studies (Horng et al., 2009; Chou et al., 2011; Tong et al., 2020) for cell counting. For comparison of cell densities, the staining cells in 8-10 unit areas (100 × 100 μm) from one side of the yolk sac for each larva were counted and averaged. The staining cells in the yolk sac skin of larvae were analyzed using Image J software (Wayne Rasband, NIH). The n value for the cell counting experiments is 9.
For the immunocytochemistry, the collected samples were fixed with 4% PFA at 4°C overnight. Thereafter, the samples were washed with PBS and then transferred to 100% methanol for overnight at −20°C. Next, zebrafish larvae were washed with PBST (PBS with 0.05% Tween 20) and then incubated with 3% bovine serum albumin for 1 h to block non-specific binding at room temperature. Samples were then incubated overnight at 4°C with a polyclonal antibody. The dilution factors for P63 (sc-8431, Santa Cruz Biotechnology, Santa Cruz, CA, USA), α subunit of H+-ATPase, and ECaC (custom production) antibodies were 1:100, 1:100, and 1:1600, respectively. The P63 antibody was used in the present study by referring to the previous studies (Bakkers et al., 2005; Hsiao et al., 2007; Janicke et al., 2007; Chou et al., 2015). After that, the samples were washed with PBST for 10 min three times and further incubated in Alexa Fluor 488 goat anti-mouse or rabbit IgG antibodies (Molecular Probes; diluted 1:400 with PBST) for overnight at 4°C. Images were acquired using a Leica M205 microscope (Leica). The method for cell density counting is described above. The n value for the cell counting experiments is 9
Following a previous study (Lin et al., 2012), zebrafish VDRa MO (5’- AACGGCACTATTTTCCGTAAGCATC-3’), VDRb MO (5’- AACGTTCCGGTCGAACTCATCTGGC-3’), and a standard control MO (5’-CCTCTTACCTCAGTTACAATTTATA-3’) were used for MO knockdown experiments. As in previous studies, a 4 ng/embryo (for MO) dosage was used for injection (Lin et al., 2012; Lin et al., 2022). MOs (4 ng/embryo) were injected into embryos at the 1–2 cell stage using an IM-300 microinjector system (Narishige Scientific Instrument Laboratory, Tokyo, Japan). The MO-injected larvae at 3 dpf were sampled for subsequent analyses.
Values are expressed as mean ± standard error of the mean (SEM). The results were compared using one-way analysis of variance (ANOVA) with Tukey’s pairwise test and Student’s t-test. Statistical significance was set at p < 0.05.
To explore the effects of acidic, low Na+, and low Ca2+ media on whole-body 1α,25(OH)2D3 level in zebrafish larvae, the zebrafish fertilized eggs were exposed to NW (pH 7.0, 0.5 mM [Na+], 0.2 mM [Ca2+]), AW (pH 4.0, 0.5 mM [Na+], 0.2 mM [Ca2+]), LNa (pH 7.0, 0.05 mM [Na+], 0.2 mM [Ca2+]), and LCa (pH 7.0, 0.5 mM [Na+], 0.02 mM [Ca2+]) for 3 days. Exposure to AW and LCa, but not LNa, significantly elevated the 1α,25(OH)2D3 level compared to that in zebrafish larvae exposed to NW (Figure 1).
Figure 1 Effects of normal freshwater (NW, 0.5 mM [Na+], 0.2 mM [Ca2+], pH 7.0), acidic NW (AW, 0.5 mM [Na+], 0.2 mM [Ca2+], pH 4.0), low Na+ NW (LNa, 0.05 mM [Na+], 0.2 mM [Ca2+], pH 7.0), and low Ca2+ NW (LCa, 0.5 mM [Na+], 0.02 mM [Ca2+], pH 7.0) on whole body 1α, 25-dihydroxytamin D3 (1α,25(OH)2D3) level in 3 days post fertilization (dpf) zebrafish larvae. Different letters indicate a significant difference (p<0.05), as determined using one-way ANOVA followed by Tukey’s multiple-comparison test. Values are the mean ± SEM (n=6).
To explore the effect of 1α,25(OH)2D3 on Na+ uptake, zebrafish fertilized eggs were treated with exogenous 1α,25(OH)2D3 (20 μg/L). At 3 dpf, the zebrafish larvae were sampled for measuring the Na+ content. The result indicated the incubation of exogenous 1α,25(OH)2D3 (20 μg/L) caused a significant increase in Na+ level compared to the control group (Figure 2).
Figure 2 The effect of 1α,25(OH)2D3 treatment on the whole-body Na+ content in 3 dpf zebrafish larvae. Values are mean ± SEM (n = 10). Student’s t-test, **p<0.01.
The whole-body 1α,25(OH)2D3 level was significantly increased in zebrafish larvae exposed to acidic and low Ca2+ media. To examine if 1α,25(OH)2D3 affects the cell densities of H+-ATPse-rich (HRC) and ECaC-expressing cells (ECCC), the two subtypes of ionocytes for acid secretion and Ca2+ uptake in zebrafish embryo skin, fertilized zebrafish eggs at the 1-2 cell stage were incubated with 1α,25(OH)2D3 (20 μg/L). Whole mount in situ hybridization was performed to reveal the number of ionocytes in 3 dpf zebrafish larvae. The results showed that 1α,25(OH)2D3 treatment caused an increase in the cell density of atp6v1a, encoding the α subunit of H+-ATPase, and ecac-expressing cells, respectively, in the yolk sac of 3 dpf zebrafish larvae (Figures 3A, C). By whole mount immunocytochemistry, it also showed 1α,25(OH)2D3 treatment caused a dominant increase in the cell density of HRC and ECCC in the yolk sac of zebrafish larvae (Figures 3B, D).
Figure 3 Effects of exogenous 1α,25(OH)2D3 treatment on the density of ionocytes at the embryonic skin of 3 dpf zebrafish larvae. (A) The representative images of whole mount in situ hybridization showing the expression of atp6v1a and ecac-expressing cell (black dots) in the yolk sac of zebrafish larvae treated with 0 (control) and 20 μg/L 1α,25(OH)2D3. (B) The representative images of whole-mount immunocytochemistry showing the expression of H+-ATPase-rich cell (HRC) and ECaC-expressing cell (ECCC) (green dots) in the yolk sac of zebrafish larvae treated with 0 (control) and 20 μg/L 1α,25(OH)2D3. (C, D) The quantitative data for the cell density. Values are the mean ± SEM (n = 9). Student’s t-test, **p<0.01, ***p<0.001. Scale bar: 100 μm.
P63 is a marker of epithelial stem cells (Bakkers et al., 2002). To explore whether 1α,25(OH)2D3 regulates the number of ionocytes by controlling epidermal stem cells, the number of P63-positive cells in the yolk sac area of zebrafish larvae treated with 1α,25(OH)2D3 (20 μg/L) was determined. The results indicated that the density of P63-positive cells in the yolk sac area was not different between the control and 1α,25(OH)2D3 treatment groups in 2 and 3 dpf zebrafish larvae (Figure 4).
Figure 4 Effects of exogenous 1α,25(OH)2D3 treatment on the density of P63-positive cell at the embryonic skin of 2 and 3 dpf zebrafish larvae. (A–D) The representative images of whole mount immunocytochemistry showing the expression of P63-positive cells (green dots) in the yolk sac of zebrafish larvae treated with 0 (control) and 20 μg/L 1α,25(OH)2D3. (a-d) The enlarged images of white squares from (A–D). (E) The quantitative data for the cell density. Values are mean ± SEM (n = 9). Scale bar: 100 μm.
Ionocyte precursor differentiation and epidermal stem cell proliferation can affect ionocyte number (Hsiao et al., 2007; Yan and Hwang, 2019). 1α,25(OH)2D3 treatment increased the number of ionocytes, but did not affect the number of epidermal stem cells in the yolk sac of zebrafish larvae (Figure 4). To examine whether 1α,25(OH)2D3 modulates the number of ionocytes by regulating ionocyte differentiation, the number of foxi3a, a marker of ionocyte progenitor, -expressing cells in the yolk sac of zebrafish larvae treated with 1α,25(OH)2D3 (20 μg/L) was measured. The results indicated that the density of foxi3a-expressing cells was significantly increased in 2 and 3 dpf zebrafish larvae following 1α,25(OH)2D3 treatment (Figure 5). 1α,25(OH)2D3 treatment enhanced the density of foxi3a-expressing cells in 2 and 3 dpf zebrafish larvae, respectively (Figure 5). On the other hand, when the expression of the vitamin D receptor (VDR) was knocked down, the increased number of foxi3a-expressing cells in the yolk sac area by 1α,25(OH)2D3 was suppressed and down to the control level in 3 dpf zebrafish larvae (Figure 6).
Figure 5 Effects of exogenous 1α,25(OH)2D3 treatment on the density of foxi3a-expressing cell at the embryonic skin of 2 and 3 dpf zebrafish larvae. (A) The representative images of whole mount in situ hybridization showing the expression of foxi3a-expressing cell (black dots) in the yolk sac of zebrafish larvae treated with 0 (control) and 20 μg/L 1α,25(OH)2D3. (B) The quantitative data for the cell density. Values are mean ± SEM (n = 9). Student’s t-test, ***p<0.001. Scale bar: 100 μm.
Figure 6 The effect of VDR MOs on the density of foxi3a-expressing cell in 3 dpf zebrafish larvae treated with 1α,25(OH)2D3. (A) The representative images of whole mount in situ hybridization showing the expression of foxi3a-expressing cell (black dots) in the yolk sac of zebrafish larvae treated with 0 (control) and 20 μg/L 1α,25(OH)2D3. (B) The quantitative data for the cell density. Different letters indicate a significant difference (p<0.05), as determined using one-way ANOVA followed by Tukey’s multiple-comparison test. Values are the mean ± SEM (n=9). Scale bar: 100 μm.
Vitamin D is a well-known hypercalcemic hormone and a previous study indicated the 1α,25-DihydroxyvitaminD3 (1α,25(OH)2D3) level was significantly higher in zebrafish larvae exposed to high Ca2+ (2 mM) than in Ca2+ (0.02 mM) water (Lin et al., 2012; Lin and Hwang, 2016). Compared to normal freshwater (NW, [Ca2+] = 0.2 mM), the 1α,25(OH)2D3 level was also higher in zebrafish larvae exposed to low Ca2+ NW (LCa, [Ca2+] = 0.02 mM). 1α,25(OH)2D3 treatment increased the capacity of Ca2+ uptake in zebrafish larvae (Lin et al., 2012). Therefore, the higher 1α,25(OH)2D3 level in zebrafish larvae exposed to LCa is suggested to increase Ca2+ uptake from the environment. In addition, this study showed increased 1α,25(OH)2D3 level in zebrafish larvae exposed to LCa and acidic NW (AW). 1α,25(OH)2D3 treatment increases the gene expression of Na+/H+ exchanger 3b (NHE3b), a vital route for Na+ uptake in ionocytes, in zebrafish larvae (Lin et al., 2022). In addition, the Na+ content was significantly increased in zebrafish larvae treated with 1α,25(OH)2D3. However, the 1α,25(OH)2D3 level was not different between normal freshwater (NW) and low Na+ NW (LNa) ([Na+] is 0.5 and 0.05 mM respectively) in the present study. 1α,25(OH)2D3 may not be involved in regulating Na+ uptake in zebrafish larvae under low Na+ stress.
In zebrafish, H+-ATPase-rich cells (HRCs) are specific subtype ionocytes that are responsible for Na+ uptake and acid secretion, and ECaC-expressing cells (ECCC, also termed as Na+,K+-ATPase cell, NaRC) are the subtype ionocytes for Ca2+ uptake (Yan and Hwang, 2019). 1α,25(OH)2D3 levels were upregulated in zebrafish larvae upon acidic stress, and 1α,25(OH)2D3 treatment increased the capacity for acid secretion at a single HRC level and whole embryonic skin (Lin et al., 2022). In addition, the gene expression of acid secretion-related transporters, such as H+-ATPase and NHE3b, was stimulated in zebrafish larvae with 1α,25(OH)2D3 treatment. The present study found that 1α,25(OH)2D3 treatment increased the number of HRC. The number of ionocytes that express the mRNA of ATP6V1A, the α subunit of H+-ATPase, was also upregulated in zebrafish larvae treated with 1α,25(OH)2D3. Thus, the increased number of HRC by vitamin D treatment may contribute to the increased mRNA expression of acid secretion-related transporters and the acid secretion capacity of the whole embryo. In the present study, we found 1α,25(OH)2D3 levels were not regulated in zebrafish larvae with low and normal Na+ adaptation. However, the Na+ content of zebrafish larvae was upregulated. It has been suggested that NHE3b is expressed in the HRC, and NHE3b and H+-ATPase contribute to Na+ uptake in zebrafish (Shih et al., 2012). Therefore, the increased Na+ content induced by 1α,25(OH)2D3 treatment is suggested by the increased number of HRC. 1α,25(OH)2D3 treatment enhances Ca2+ uptake mainly by upregulating the gene expression of ECaC in zebrafish larvae (Lin et al., 2012). ECaC is a vital cell marker of ECCC, and the present study indicated that the number of ionocytes expressing ECaC mRNA and protein was predominantly upregulated in zebrafish larvae treated with 1α,25(OH)2D3 compared to the untreated group. 1α,25(OH)2D3 may be able to increase Ca2+ uptake by increasing the number of ECCC.
Hormones influence the number of ionocytes in fish by modulating ionocyte precursor differentiation and/or epidermal stem cell proliferation (Yan and Hwang, 2019). P63 is a marker of epidermal stem cells (Bakkers et al., 2002; Hsiao et al., 2007). The previous study reported that isotocin and arginine vasopressin enhanced the number of ionocytes by increasing the proliferation of P63-positive epidermal stem cells in zebrafish (Chou et al., 2011; Tong et al., 2020). The present study showed that vitamin D treatment increased the number of HRC and ECCC in zebrafish. To elucidate the potential regulatory mechanism by which vitamin D affects the number of ionocytes, we examined the effect of 1α,25(OH)2D3 treatment on the number of P63-positive cells. The present results revealed that the number of P63-positive cells was not regulated in zebrafish larvae treated with 1α,25(OH)2D3 treatment. Vitamin D may not be able to stimulate the proliferation of epidermal stem cells to increase the number of HRC and ECCC in zebrafish.
Foxi3a is a helix/forkhead box transcription factor that has been identified as a progenitor marker, and functions as a vital regulator of ionocyte differentiation (Esaki et al., 2007; Hsiao et al., 2007; Janicke et al., 2007). Some hormones, such as cortisol and stanniocalcin-1 (STC-1), also affect the number of ionocytes by increasing foxi3a expression in fish (Cruz et al., 2013; Chou et al., 2015). In the present study, 1α,25(OH)2D3 treatment increased the number of foxi3a-positive cells, and this stimulation was inhibited in zebrafish with vitamin D receptor (VDR) knockdown. Foxi3b is another vital transcription factor involved in ionocyte differentiation (Hsiao et al., 2007). Foxi3b is regulated by Foxi3a in ionocyte progenitors (Hsiao et al., 2007). In terminal ionocyte differentiation, the Foxi3a and Foxi3b network specifically determines HRC and ECCC differentiation specifically (Hsiao et al., 2007). Therefore, the present study revealed that vitamin D can affect foxi3a expression and thereafter regulate the differentiation of HRC and ECCC. In mammals, vitamin D acts with VDR and is vital for skin keratinocyte differentiation by promoting the expression of differentiation markers (Mostafa and Hegazy, 2015). Skin keratinocyte differentiation was reduced in VDR-knockout mice because the expression of keratinocyte markers is defective (Xie et al., 2002). The present study showed that 1α,25(OH)2D3 treatment did not increase the number of foxi3a-positive cell in zebrafish with vitamin D receptor (VDR) knockdown. In addition, previous studies have identified VDR expression in HRC and ECCC (Lin et al., 2012; Lin et al., 2022). Therefore, vitamin D may be via the VDR to regulate ionocyte differentiation. Previous studies indicated that 1α,25(OH)2D3 treatment increased the gene expression of Gcm2, a transcription factor specific for HRC differentiation, in zebrafish larvae (Chang et al., 2009; Lin et al., 2022). Gcm2 regulates ionocyte maturation at a later stage of ionocyte development than Foxi3a in zebrafish (Chang et al., 2009). The increased gcm2 expression in zebrafish larvae treated with 1α,25(OH)2D3 may partially result from the upregulated foxi3a expression.
The present and previous studies revealed that vitamin D levels and gene expression of vitamin D synthesis were increased in fish exposed to low Ca2+ and acidic water (Lin et al., 2012; Lin et al., 2022). In mammals, vitamin D regulates epidermal cell differentiation in fish. To our knowledge, this is the first study to show that vitamin D positively affects ionocyte differentiation in fish. Vitamin D may act via VDR to increase the foxi3a expression to increase ionocyte differentiation. The present study provides new insights into how vitamin D modulates ion regulation and acid secretion in fish under environmental stress. This study increases our knowledge of the stress adaptation mechanisms of fish.
The original contributions presented in the study are included in the article/supplementary material. Further inquiries can be directed to the corresponding author.
The animal study was reviewed and approved by the Institutional Animal Care and Utilization Committee of the National Kaohsiung University of Science and Technology.
C-HL provided the conception and study design. C-HL, H-CC, S-TL, and H-JH carried out the experiments and data collection. C-HL, H-CC, and S-TL performed the data analysis and interpretation. C-HL wrote the manuscript. All the authors reviewed and revised the manuscript and gave final approval for publication.
This work was partly supported by research grants from the Ministry of Science and Technology of Taiwan (MOST109-2313-B-992-003-MY3) and in part by the iEGG and Animal Biotechnology Center from The Feature Area Research Center Program within the framework of the Higher Education Sprout Project by the Ministry of Education (MOE), Taiwan (MOE 109-S-0023-A) to CH L.
The authors declare that the research was conducted in the absence of any commercial or financial relationships that could be construed as a potential conflict of interest.
All claims expressed in this article are solely those of the authors and do not necessarily represent those of their affiliated organizations, or those of the publisher, the editors and the reviewers. Any product that may be evaluated in this article, or claim that may be made by its manufacturer, is not guaranteed or endorsed by the publisher.
1α,25(OH)2D3, 1α, 25-dihydroxyvitamin D3; AW, acidic normal freshwater; ANOVA, analysis of variance; dpf, days post fertilization; ECCC, ECaC-expressing cell; Foxi3a/b, Forkhead box I3a/b; FW, freshwater; HA, H+-ATPase; HRC, H+-ATPase-rich cell; LCa, low Ca2+ normal freshwater; LNa, low Na+ normal freshwater; NHE3, Na+/H+ exchanger 3; NW, normal freshwater; PBS, phosphate-buffered saline; SEM, standard error of the mean; STC, Stanniocalcin; VDR, vitamin D receptor.
Bakkers J., Camacho-Carvajal. M., Nowak. M., Kramer. C., Danger. B., Hammerschmidt M. (2005). Destabilization of DeltaNp63alpha by Nedd4-mediated ubiquitination and Ubc9-mediated sumoylation, and its implications on dorsoventral patterning of the zebrafish embryo. Cell Cycle 4 (6), 790–800. doi: 10.4161/cc.4.6.1694
Bakkers J., Hild M., Kramer C., Furutani-Seiki M., Hammerschmidt M. (2002). Zebrafish DeltaNp63 is a direct target of bmp signaling and encodes a transcriptional repressor blocking neural specification in the ventral ectoderm. Dev. Cell. 2, 617–627. doi: 10.1016/s1534-5807(02)00163-6
Binswanger U., Helmle-Kolb C., Forgo J., Mrkic B., Murer H. (1993). Rapid stimulation of Na+/H+ exchange by 1,25-dihydroxyvitamin D3; interaction with parathyroid-hormone-dependent inhibition. Pflügers Arch. 424, 391–397. doi: 10.1007/BF00374899
Chang W. J., Horng J. L., Yan J. J., Hsiao C. D., Hwang P. P. (2009). The transcription factor, glial cell missing 2, is involved in differentiation and functional regulation of h+-ATPase-rich cells in zebrafish (Danio rerio). Am. J. Physiol. Regul. Integr. Comp. Physiol. 296, R1192–R1201. doi: 10.1152/ajpregu.90973.2008
Cheng W., Guo L., Zhang Z., Soo H. M., Wen C., Wu W., et al. (2006). HNF factors form a network to regulate liver-enriched genes in zebrafish. Dev. Biol. 294 (2), 482–496. doi: 10.1016/j.ydbio.2006.03.018
Chou M. Y., Hung J. C., Wu L. C., Hwang S. P., Hwang P. P. (2011). Isotocin controls ion regulation through regulating ionocyte progenitor differentiation and proliferation. Cell. Mol. Life. Sci. 68, 2797–2809. doi: 10.1007/s00018-010-0593-2
Chou M. Y., Lin C. H., Chao P. L., Hung J. C., Cruz S. A., Hwang P. P. (2015). Stanniocalcin-1 controls ion regulation functions of ion-transporting epithelium other than calcium balance. Int. J. Biol. Sci. 11 (2), 122–132. doi: 10.7150/ijbs.10773
Cruz S. A., Lin C. H., Chao P. L., Hwang P. P. (2013). Glucocorticoid receptor, but not mineralocorticoid receptor, mediates cortisol regulation of epidermal ionocyte development and ion transport in zebrafish (Danio rerio). PloS One 8, e77997. doi: 10.1371/journal.pone.0077997
Esaki M., Hoshijima K., Kobayashi S., Fukuda H., Kawakami K., Hirose. S. (2007). Visualization in zebrafish larvae of na+ uptake in mitochondria-rich cells whose differentiation is dependent on foxi3a. Am. J. Physiol. Regul. Integr. Comp. Physiol. 292, R470–R480. doi: 10.1152/ajpregu.00200.2006
Evans D. H., Piermarini P. M., Choe K. P. (2005). The multifunctional fish gill: Dominant site of gas exchange, osmoregulation, acid-base regulation, andexcretion of nitrogenous waste. Physiol. Rev. 85, 97–177. doi: 10.1152/physrev.00050.2003
Goldstone J. V., McArthur A. G., Kubota A., Zanette J., Parente T., Jönsson M. E., et al. (2010). Identification and developmental expression of the full complement of cytochrome P450 genes in zebrafish. BMC Genomics 11, 643. doi: 10.1186/1471-2164-11-643
Guh Y. J., Lin C. H., Hwang P. P. (2015). Osmoregulation in zebrafish: Ion transport mechanisms and functional regulation. EXCLI. J. 14, 627–659. doi: 10.17179/excli2015-246
Horng J. L., Lin L. Y., Hwang P. P. (2009). Functional regulation of h+- ATPase-rich cells in zebrafish embryos acclimated to an acidic environment. Am. J. Physiol. Cell Physiol. 296, C682–C692. doi: 10.1152/ajpcell.00576.2008
Howarth D. L., Law S. H., Barnes B., Hall J. M., Hinton D. E., Moore L., et al. (2008). Paralogous vitamin d receptors in teleosts: Transition of nuclear receptor function. Endocrinol. 149 (5), 2411–2422. doi: 10.1210/en.2007-1256
Hsiao C. D., You M. S., Guh Y. J., Ma M., Jiang Y. J., Hwang P. P. (2007). A positive regulatory loop between foxi3a and foxi3b is essential for specification and differentiation of zebrafish epidermal ionocytes. PloS One 2, e302. doi: 10.1371/journal.pone.0000302
Hwang P. P., Lee T. H., Lin L. Y. (2011). Ion regulation in fish gills: Recent progress in the cellular and molecular mechanisms. Am. J. Physiol. Regul. Integr. Comp. Physiol. 301, R28–R47. doi: 10.1152/ajpregu.00047.2011
Janicke M., Carney T. J., Hammerschmidt M. (2007). Foxi3 transcription factors and notch signaling control the formation of skin ionocytes from epidermal precursors of the zebrafish embryo. Dev. Biol. 307, 258–271. doi: 10.1016/j.ydbio.2007.04.044
Lin C. H., Hu H. J., Chuang H. J., Tsou Y. L., Hwang P. P. (2021). Cortisol and glucocorticoid receptor 2 regulate acid secretion in medaka (Oryzias latipes) larvae. J. Comp. Physiol. B. 191 (5), 855–864. doi: 10.1007/s00360-021-01390-w
Lin C. H., Hwang P. P. (2016). The control of calcium metabolism in zebrafish (Danio rerio). Int. J. Mol. Sci. 17, 1783. doi: 10.3390/ijms17111783
Lin C. H., Liu S. T., Wang Y. C., Tsou Y. L., Hu H. J. (2022). Vitamin d regulates transepithelial acid secretion in zebrafish (Danio rerio) larvae. Front. Mar. Sci. 9. doi: 10.3389/fmars.2022.990502
Lin C. H., Su C. H., Tseng D. Y., Ding F. C., Hwang P. P. (2012). Action of vitamin d and the receptor, VDRa, in calcium handling in zebrafish (Danio rerio). PloS One 7 (9), e45650. doi: 10.1371/journal.pone.0045650
Lips P. (2006). Vitamin d physiology. Prog. Biophys. Mol. Biol. 92, 4–8. doi: 10.1016/j.pbiomolbio.2006.02.016
Lock E. J., Ornsrud R., Aksnes L., Spanings F. A., Waagbø R., Flik G. (2007). The vitamin d receptor and its ligand 1alpha,25-dihydroxyvitamin D3 in Atlantic salmon (Salmo salar). J. Endocrinol. 193 (3), 459–471. doi: 10.1677/JOE-06-0198
Mostafa W. Z., Hegazy R. A. (2015). Vitamin d and the skin: Focus on a complex relationship: A review. J. Adv. Res. 6 (6), 793–804. doi: 10.1016/j.jare.2014.01.011
Pan W., Borovac J., Spicer Z., Hoenderop J., Bindels R., Shull G., et al. (2012). The epithelial sodium/proton exchanger, NHE3, is necessary for renal and intestinal calcium (re)absorption. Am. J. Physiol. Renal. Physiol. 302 (8), F943–F956. doi: 10.1152/ajprenal.00504.2010
Shih T. H., Horng J. L., Liu S. T., Hwang P. P., Lin L. Y. (2012). Rhcg1 and NHE3b are involved in ammonium-dependent sodium uptake by zebrafish larvae acclimated to low-sodium water. Am. J. Physiol. Regul. Integr. Comp. Physiol. 302, R84–R93. doi: 10.1152/ajpregu.00318.2011
Sundell K., Norman A. W., Björnsson B. T. (1993). 1α,25(OH)2 vitamin D3 increases ionized plasma calcium concentrations in the immature Atlantic cod, Gadus morhua. Gen. Comp. Endocrinol. 91 (3), 344–351. doi: 10.1006/gcen.1993.1135
Swarup K., Das V. K., Norman A. W. (1991). Dose-dependent vitamin D3 and 1 α,25-dihydroxyvitamin D3-induced hypercalcemia and hyperphosphatemia in male cyprinoid Cyprinus carpio. Comp. Biochem. Physiol. A. Physiol. 100 (2), 445–447. doi: 10.1016/0300-9629(91)90497-Z
Tong S. K., Lee H. L., Lee Y. C., Wu L. C., Tsou Y. L., Lu S. W., et al. (2020). Arginine vasopressin modulates ion and acid/base balance by regulating cell numbers of sodium chloride cotransporter and h+-ATPase rich ionocytes. Int. J. Mol. Sci. 21 (11), 3957. doi: 10.3390/ijms21113957
Xie Z., Komuves L., Yu Q. C., Elalieh H., Ng D. C., Leary C., et al. (2002). Lack of the vitamin d receptor is associated with reduced epidermal differentiation and hair follicle growth. J. Invest. Dermatol. 118 (1), 11–16. doi: 10.1046/j.1523-1747.2002.01644.x
Keywords: zebrafish, vitamin D, ion regulation, foxi3a, ionocyte
Citation: Lin C-H, Chang H-C, Liu S-T and Hu H-J (2023) Vitamin D regulates ion regulation by affecting the ionocyte differentiation in zebrafish (Danio rerio) larvae. Front. Mar. Sci. 10:1141116. doi: 10.3389/fmars.2023.1141116
Received: 10 January 2023; Accepted: 02 February 2023;
Published: 14 February 2023.
Edited by:
Xiaodan Wang, East China Normal University, ChinaReviewed by:
James A. Coffman, Mount Desert Island Biological Laboratory, United StatesCopyright © 2023 Lin, Chang, Liu and Hu. This is an open-access article distributed under the terms of the Creative Commons Attribution License (CC BY). The use, distribution or reproduction in other forums is permitted, provided the original author(s) and the copyright owner(s) are credited and that the original publication in this journal is cited, in accordance with accepted academic practice. No use, distribution or reproduction is permitted which does not comply with these terms.
*Correspondence: Chia-Hao Lin, Y2gxMjNAbmt1c3QuZWR1LnR3
†ORCID: Chia-Hao Lin, orcid.org/0000-0002-4416-8599
Disclaimer: All claims expressed in this article are solely those of the authors and do not necessarily represent those of their affiliated organizations, or those of the publisher, the editors and the reviewers. Any product that may be evaluated in this article or claim that may be made by its manufacturer is not guaranteed or endorsed by the publisher.
Research integrity at Frontiers
Learn more about the work of our research integrity team to safeguard the quality of each article we publish.