- 1Marine Biology Laboratory, Earth and Life Institute, Université catholique de Louvain, Louvain-la-Neuve, Belgium
- 2Département Origine et Évolution, UMR7205 ISYEB MNHN-CNRS-UPMC-EPHE, Muséum national d’Histoire naturelle, Paris, France
- 3Invertebrate Department, Museum Victoria, Melbourne, VIC, Australia
Bioluminescence in echinoderms has been known since the early 19th century. Of the four luminous classes known, Crinoidea is the least studied, with only five bioluminescent species reported. The research conducted during the RV Southern Surveyor 2005 “Mapping benthic ecosystems” and the RV Investigator 2017 “Sampling the Abyss” cruises aimed to systematically sample deep benthic habitats along Australia’s eastern and southwestern margins. These cruises allowed us to acquire the first in vivo pictures of light-emitting Crinoidea and luminometric measurements on fresh tissue samples. Four new records of bioluminescence in deep-sea Comatulida from three distinct clades, double the number of known Crinoidea species with bioluminescent capacity. In vivo photography and histology suggest that, in some species, light emission might originate from the sacculi. Pharmacological assays reveal that Thalassometridae light emission is under adrenergic control. Biochemical data indicate the presence of a coelenterazine-based luciferin-luciferase system in Thalassometra cf. gracilis similar to the one described in the ophiuroid Amphiura filiformis. Phylogenetic distribution of bioluminescence among Crinoidea and differences in this trait phenotype could be indicative of multiple acquisitions of luminescent capability in Crinoidea, possibly promoted by the ecological role that bioluminescence might fulfill in the vastness of the deep benthic habitat.
1 Introduction
Bioluminescence designates the emission of cold visible light as a result of a natural biochemical reaction from a living organism (Harvey, 1952; Shimomura and Yampolsky, 2019). Two types of systems exist: (i) the luciferase-luciferin system in which two reagents can be isolated: one enzyme called luciferase and one substrate termed luciferin; and (ii) the photoprotein system consisting of a stabilized luciferase and luciferin complex within a protein. In both systems, light emission occurs after the substrate’s catalytic oxidation by the enzyme (Shimomura and Yampolsky, 2019). Currently, most luminous species come from marine environments with 13 phyla and about 820 genera containing bioluminescent representatives (Herring, 1987; Haddock et al., 2010; Davis et al., 2016; Shimomura and Yampolsky, 2019; Lau and Oakley, 2020). Remarkable video analyses, made on underwater footage from the northeastern Pacific, led to the conclusion that 76% of deep-water pelagic and about 30 to 40% of deep-water benthic organisms can be categorized as being able to emit light (Martini and Haddock, 2017; Martini et al., 2019).
Bioluminescence in Echinodermata has been mentioned since the early 19th century (Viviani, 1805; Harvey, 1952). In the most recent synthesis, a total of 120 bioluminescent species were reported: 20 Asteroidea, 3 Crinoidea, 31 Holothuroidea, 66 Ophiuroidea, and no Echinoidea (Mallefet, 2009). These numbers are still rising slowly (Mallefet and Fujita, 2014; Mallefet, 2022). The work on Ophiuroidea shows the variability in light emission systems (Harvey, 1952; Herring, 1974; Shimomura, 1986; Mallefet et al., 2013; Delroisse et al., 2017b), in response types (Herring, 1983; Grober, 1988; Herring, 1995; Deheyn et al., 1997; Deheyn et al., 2000a; Jones and Mallefet, 2012; Jones and Mallefet, 2013), histology (Harvey, 1952; Buchanan, 1963; Brehm and Morin, 1977; Deheyn et al., 1996; Mallefet, 2009; Delroisse et al., 2017a) and physiology (Harvey, 1952; Buchanan, 1963; Herring, 1974; Brehm and Morin, 1977; Dewael and Mallefet, 2002; Vanderlinden et al., 2003; Dupont et al., 2004; Vanderlinden and Mallefet, 2004; Mallefet, 2009; Vanderlinden et al., 2010; Delroisse et al., 2017b). Only two papers have tried to look more in-depth into the bioluminescence of non-ophiuroid echinoderms (Herring, 1974; Robison, 1992). So far, echinoderms exhibit only intrinsic bioluminescence, meaning these organisms produce their own light (i.e., not via luminescent symbiotic bacteria). Light is mainly produced intracellularly (e.g. Deheyn et al., 1996; Delroisse et al., 2017a) and under nervous control (Dewael and Mallefet, 2002; Vanderlinden et al., 2003; Dupont et al., 2004; Mallefet, 2009; Vanderlinden et al., 2010). To this day, no photophores (i.e., light-emission organs) have been observed in echinoderms (Delroisse et al., 2017a), light emission being produced via photocytes (i.e. light-emission cells) that can be aggregated or not (Harvey, 1952; Buchanan, 1963; Brehm and Morin, 1977; Deheyn et al., 1996; Mallefet, 2009; Delroisse et al., 2017a). Furthermore, the light emission biochemistry in echinoderms remains understudied. Only two systems, both in ophiuroids, have been described in more detail: (i) a photoprotein in Ophiopsila californica (Shimomura, 1986) and (ii) a luciferin-luciferase system in Amphiura filiformis, (Delval and Mallefet, 2010; Shimomura and Yampolsky, 2019). In the latter, biochemical and transcriptomic analyses identified coelenterazine as the luciferin (Mallefet, 2022; Shimomura and Yampolsky, 2019; Mallefet et al., 2020) and, a homologue of the sea pansy Renilla luciferase (RLuc) as the enzyme (Delroisse et al., 2017b). In non-ophiuroids, Herring (1974), detected the presence of a luciferin-luciferase reaction in the crinoid Thaumatocrinus jungerseni and the starfish Hydrasterias ophidion but without identification of the reagents.
Regarding the ecological role of bioluminescence in echinoderms, light emission has been shown to be used for different purposes: (i) for stunning and as aposematic signal i.e. luminescent warning to deter predators (Grober, 1988; Guilford and Cuthill, 1989; Jones and Mallefet, 2010; Jones and Mallefet, 2013), (ii) as burglar alarm i.e. attracting a secondary predator (Robison, 1992; Mallefet, 2009; Jones and Mallefet, 2013), (iii) as sacrificial lure i.e. light distraction in combination with autotomy of a body part (Robison, 1992; Deheyn et al., 2000b; Mallefet, 2009; Jones and Mallefet, 2013). Besides, in some echinoderm species, hypotheses have been made for the possible use of bioluminescence as a tool for communication (Robison, 1992; Deheyn et al., 2000b; Birk et al., 2018) or predation (Morin, 1983; Holland et al., 1991; Jones and Mallefet, 2012).
Crinoidea, commonly known as sea lilies and feather stars, are the most basal of the five classes of Echinodermata. So far, 664 species are recognized in 183 genera and 32 families, yet the whole class is currently undergoing major revisions since the employment of molecular phylogenetic tools (Hemery et al., 2013; Rouse et al., 2013) and the study of their ontogeny (Améziane and Roux, 2005; Améziane et al., 2021) have shown the recurrence of homoplasy in morphology-based phylogenies. For Crinoidea, the only records of bioluminescence date back to the research of (Herring, 1974; Herring, 1983) as well as two anecdotal records by Dilly (1973) and Messing (pers. comm. in Johnsen et al., 2012). Thus, five species have been described as bioluminescent so far: Endoxocrinus wyvillethomsoni (Isselicrinidae, Isocrinida) and Thaumatocrinus jungerseni (Pentametrocrinidae, Comatulida) (Herring, 1974), as well as Thalassometra lusitanica (Thalassometridae, Comatulida) (Herring, 1983), a Ptilocrinus sp. (Hyocrinidae, Hyocrinida) wrongly identified as Pentacrinus by Dilly (1973), and most recently Neocrinus decorus (Isocrinidae, Isocrinida) (Johnsen et al., 2012). In Crinoidea, light emission has been described from the arms, disk, stalk, and cirri. For T. jungerseni a “general pale greenish-blue glow, brightest at the disk” was reported as well as light points visible in the internode regions of the arm segments, the glow spreading along the arms when the animal was handled; no light was observed from the stalk in E. wyvillethomsoni but from arms and, less-brightly, from the cirri (Herring, 1974). In contrast, T. lusitanica does not show light from the main arms but emits from single regions in each cirrus segment (Herring, 1995); N. decorus was described as producing flashes of light along the stalk (Messing pers. comm. in Johnsen et al., 2012). No cell type has been defined as responsible for the light emission in crinoids to date (Herring, 1974).
Participation in deep-sea surveys has given us access to rare crinoid samples. Through the first in vivo photographs, and new pharmacological and luminometric recordings, this paper describes the newly discovered luminescence of four crinoid species belonging to three different families. A special focus was made on Thalassometridae species giving new insights into the luminescence among Crinoidea, with (i) an adrenergic nervous control, (ii) a putative involvement of the saccule in the light emission process, and (iii) the presence of a luciferase-luciferin system based on coelenterazine. These results increase our knowledge of bioluminescence in deep-sea echinoderms as well as update the ever-growing list of bioluminescent species.
2 Materials and methods
2.1 Animal sampling
Organisms were collected during the Southern Surveyor SS10/2005 cruise “Mapping benthic ecosystems on the deep continental shelf and slope in Australia’s Southwestern Region” in 2005 between 200 and 2000 m and during the “Sampling the Abyss’’ cruise (IN2017_V03) in 2017 between 1000 and 4800 m depth using the CSIRO Four Meter Beam Trawl. The depth and place of collected Crinoidea are reported in Supplementary Table 1A. Specimens of Adelatelecrinus cf. vallatus, Monachocrinus cf. aotearoa, Bathycrinus cf. australocrucis, Democrinus cf. japonicus, Thalassometra cf. gracilis, and Stiremetra breviradia were kept in seawater in a dark cold room at 1°C. Stimulation of the light emission, photography and luminometry measurements on specimens were done directly on board the RV Investigator and Southern Surveyor. Photographs were taken with a Canon 1Dx and a Sony Alpha 7SII cameras (Tokyo, Japan) under natural light and then in the dark upon stimulation of the organism. Stimulation of the light emission was either done mechanically with a brush or through immersion of the whole organism (when sufficient specimens were available) in a 200 mM KCl solution (whole depolarization of excitable cells) and/or immersion in with freshwater diluted seawater (50% FW creating osmotic stress).
Whole individuals of S. breviradia and arm pieces with pinnules from M. cf. aotearoa and T. cf. gracilis were stored in a cooled 4% formaldehyde seawater solution. One complete specimen of T. cf. gracilis was kept frozen at -80° C. These samples were transferred to the marine biology laboratory of UCLouvain (Belgium) for further analysis. No tissue samples were taken from D. cf. japonicus and B. cf. australocrucis. The remaining parts of the samples went to the collections of the Victoria Museum (Australia). Furthermore, Antedon bifida, a shallow water crinoid, was collected by SCUBA at about 10 m depth off the Concarneau marine station in 2020 and 2021, specimens were transferred to UCLouvain either alive to test bioluminescence or stored in a 4% formaldehyde seawater solution for histology control. All data regarding the species sampled in this study are summarized in Supplementary Table 1A.
2.2 Light recordings and stimulations
For luminometry, an FB12 single-tube luminometer (Berthold Detection Systems GmbH, Pforzheim, Germany) was used in “single kinetics” mode with the FB12/Sirius software (Berthold Detection Systems Gmbh). Calibration was done with a Tritium light source (Beta Light Source, Saunders-Roe and Nuclear Enterprises, UK). Pieces of the organisms were dissected and put in luminometer tubes. Stimulations were performed using a syringe and an injector coupled to the luminometer. Solutions were either 200 mM KCl, FW, or increasing concentration of adrenaline from 0.27 to 2.73 mmol L-1. All specimens were tested for KCl stimulation except M. cf. aotearoa which presented a high level of spontaneous luminescence. Luminescence recording for this later species was made without any added solution. For S. breviradia and T. cf. gracilis, besides the arms, cirri and calyx were also tested. Light intensity was recorded every 0.2 s for 180 seconds for each measurement. Light emission was standardised by the tissue piece length and characterized as follows: (i) the maximum light intensity (Lmax), expressed in megaquanta per second per cm (Mq s−1 cm−1), and (ii) the total amount of light emitted (Ltot) over 180 seconds, expressed in Mq cm−1. Data of the various species and tests performed are summarized in Supplementary Tables 1B–D.
2.3 Histology of the arms
For histology, decalcification was done on a 1 cm M. cf. aotearoa arm by agitation in a 0.3 M NaCl solution with 2% ascorbic acid that lasted until the tissue was deemed soft (4 h). The piece was then cryoprotected using an increased sucrose concentration series (10% for 1h; 20% for 1h; 30% overnight) in phosphate buffer saline. The tissue was then embedded in Cryomatrix (Thermo Fisher Scientific, Waltham, Massachusetts, U.S.A.) and quickly frozen at -80° C. The 10 µm thin sections were cut with a Leica CM3050 S Research Cryostat (Leica Microsystems GmbH, Wetzlar, Germany) and mounted on Superfrost Plus Microscope slides (Thermo Fisher Scientific). Slices and in toto observations were done under a Leitz Diaplan Fluorescent Microscope (Leica Microsystems Gmbh) with an epifluorescence 100 W mercury arc lamp used as a light source with a blue filter (420 - 490 nm). Pictures were taken with a ToupCam UCMOS Series C-mount USB2.0 CMOS Camera 27 (Hangzhou ToupTek Photonics, Zhejiang, China) associated with an FMAXXX 0,5 x 23,2 mm adapter (ToupTek) and the ToupView software (ToupTek) to measure the objects. Estimation of mean diameters and intervals between dots are expressed as mean ± standard of the mean (SEM) with number of measurements (n). Arms of A. bifida, used as histological control, were subjected to the same treatments.
2.4 Bioluminescent system assays
The frozen T. cf. gracilis stored at -80°C was used to perform coelenterazine and luciferase assays. Each assay was performed ona 1 cm arm piece (weighted beforehand). Luminometric experiments were done according to Mallefet et al. (2020) using the same luminometer. Briefly, for the coelenterazine assay, arm segments were crushed with mortar and pestle in 200 µL of cold argon-saturated methanol. Five µL of the methanolic extract were added to 195 µL Tris buffer (20 mM Tris, 0.5 M NaCl, pH 7.4) in a polystyrene 5 ml tube (Starstedt, Nümbrecht, Germany), vortexed and introduced into the luminometer. Light emission was triggered by injection of 200 µL Tris buffer containing 3 µL Renilla luciferase (RLuc). Obtained Ltot values were used to calculate the coelenterazine amount contained in a gram of arm tissue (ng g-1) as described by Shimomura and Yampolsky (2019). For the luciferase assay, arm segments were crushed with mortar and pestle in 200 µL of Tris Buffer. Then, 10, 20 and 40 µL of the extract were added to 190, 180 and 160 µL Tris solution, respectively. Each diluted T. cf. gracilis luciferase extract was injected into luminometer tubes filled with 220 µl Tris buffer solution containing 5 μL of coelenterazine methanolic stock solution (Prolume Ltd, Pinetop, AZ, USA). Three measures of Lmax were recorded and averaged to calculate the maximal light decay rate corresponding to the luciferase activity, expressed in 109 quanta s−1 g−1 (Shimomura and Yampolsky, 2019). A total of five coelenterazine and seven luciferase activity replicates were performed (see Supplementary Table 1E).
2.5 Crinoidea luminescence phylogenetic distribution
To visualize the phylogenetic distribution of bioluminescence, a phylogeny of extant Crinoidea based on molecular markers was used (Hemery et al., 2013). Assembling both literature information and newly collected data, a new list of luminous and non-luminous species was established.
3 Results
3.1 Crinoidea in vivo photographs
The first in vivo photographs of deep benthic luminous Crinoidea were obtained via the participation in two Australian deep-sea scientific surveys (Figure 1). Natural color of freshly collected S. breviradia appears brownish. Lateral and oral photographs made in the dark after KCl immersion show a greenish luminescence at the level of the calyx and the tegmen (possibly the anal cone), and a more diffuse luminescence with some dots along the arms while cirri do not glow. No proximal to distal luminescent gradient is observed along the arm (Figures 1A, B). Daylight observation of T. cf. gracilis specimens indicates a color pattern variation ranging from brown to more yellowish color. After KCl depolarisation, blue-greenish light only emanates only from the arms with blue emitting dots at the pinnule level; no proximal to distal luminescent gradient is observed along the arm (Figures 1C, D). Examination of the luminescent blue dots size and interval indicates a mean dot diameter of 0.14 ± 0.01 (n=56) with a mean interval of 0.60 ± 0.02 mm (n=60). Based on the daylight photographs, fresh stalked M. cf. aotearoa presents a general beige coloration. Handling of the specimen induced a bioluminescence restricted to blue dots on the arms and pinnules (Figure 1E). The mean dot diameter is 0.16 ± 0.01 mm long (n=81) with a mean interval of 0.89 ± 0.07 mm (n=56). The freshly collected specimen of A. cf. vallatus exhibited a spontaneous luminescence at the arm level (J.M. pers. comm.) that was recorded with the luminometer (see next section), it was not possible to obtain a luminescent photograph for this species. For the two-remaining deep-sea species, B. cf. australocrucis, and D. cf. japonicus, luminous data could not be obtained whatever the used stimulation i.e. mechanical, KCl or FW (see Supplementary Table 1C). Stimulations of arm and calyx from a common shallow water species, A. bifida also failed to induce any luminescence (see Supplementary Table 1D).
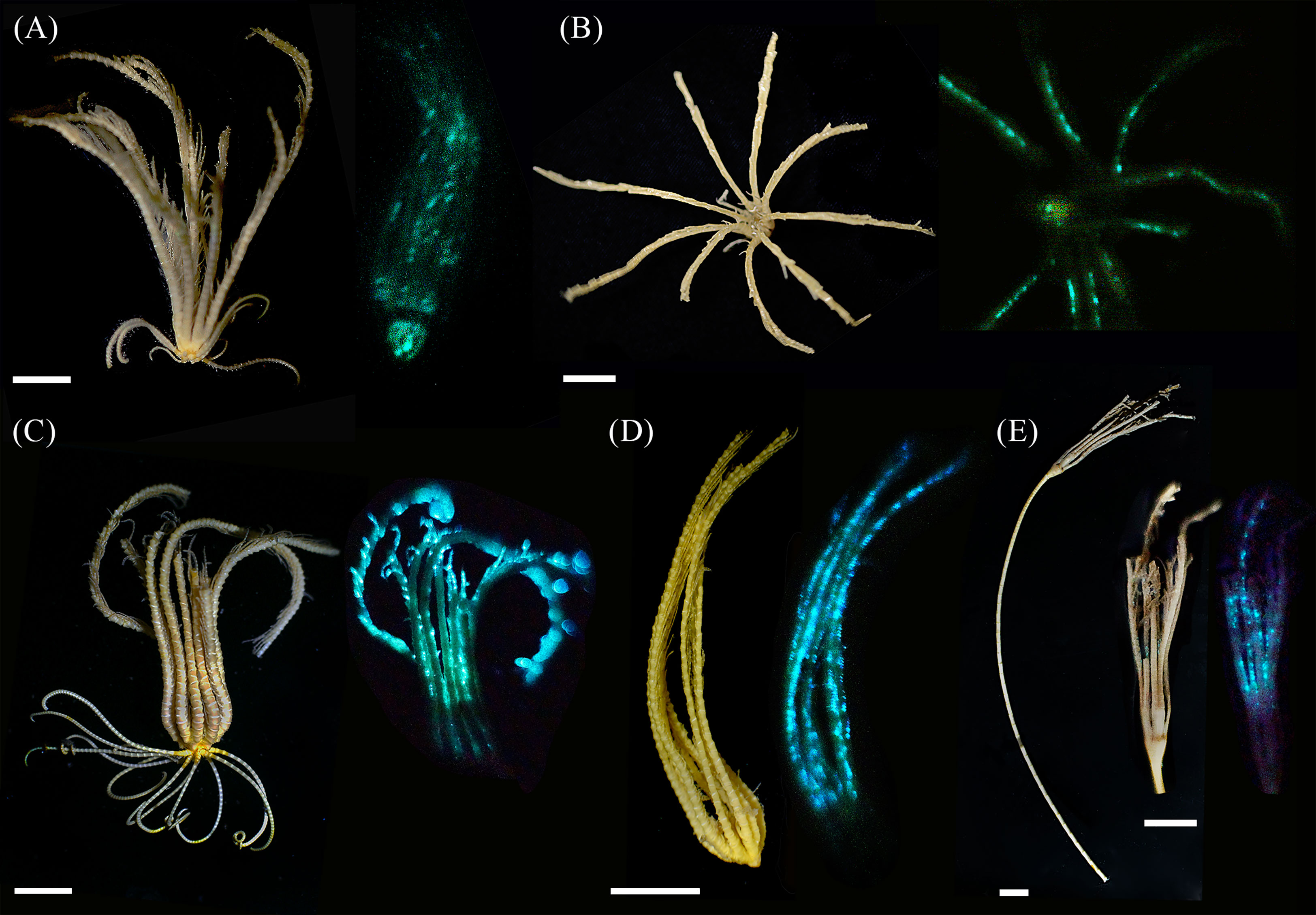
Figure 1 Photography of daylight (left) and light-emitting (right) crinoids. (A) Lateral view of Stiremetra breviradia and (B) oral view of S. breviradia (both KCL stimulation); (C) whole specimen of Thalassometra cf. gracilis and (D) closer view of T.cf gracilis arms (KCL stimulation); (E) specimen of Monachocrinus cf. aotearoa with closer view of calyx and arms (spontaneous bioluminescence after handling the specimen). Scale bars = 1 cm.
3.2 Crinoidea luminescence recordings
Examples of original recordings from 1 cm arm piece of the various species tested during this research are presented in Figure 2. The arm piece of M. cf. aotearoa, spontaneously luminescent, presented a long-lasting glow with a Ltot values of 14202.90 Mq and a Lmax of 85.89 Mq s-1 (Figure 2A). Stimulation with KCl 200 mM of the other species’ arms induces a rapid spike followed by a decrease showing secondary lower glows (Figures 2B–D). For A. cf. vallatus two arm pieces tested reacted to KCl with a highest Lmax value of 20.79 Mq s-1 (Figure 2B). For S. breviradia the arm luminescence intensity (Lmax) is ten-fold lower than the long lasting glow produced by the calyx (Figures 1A, 2C). The arm pieces of T. cf. gracilis specimens respond to a KCl injection with a mean Lmax of 87.21 ± 52.57 Mq s-1. FW applications on arms induce a significant light emission while similar treatments on calyx and cirri only produce a very weak glow. The detailed results obtained with KCl depolarisations and FW osmotic stress on the various tissue are shown in Supplementary Tables 1B, C.
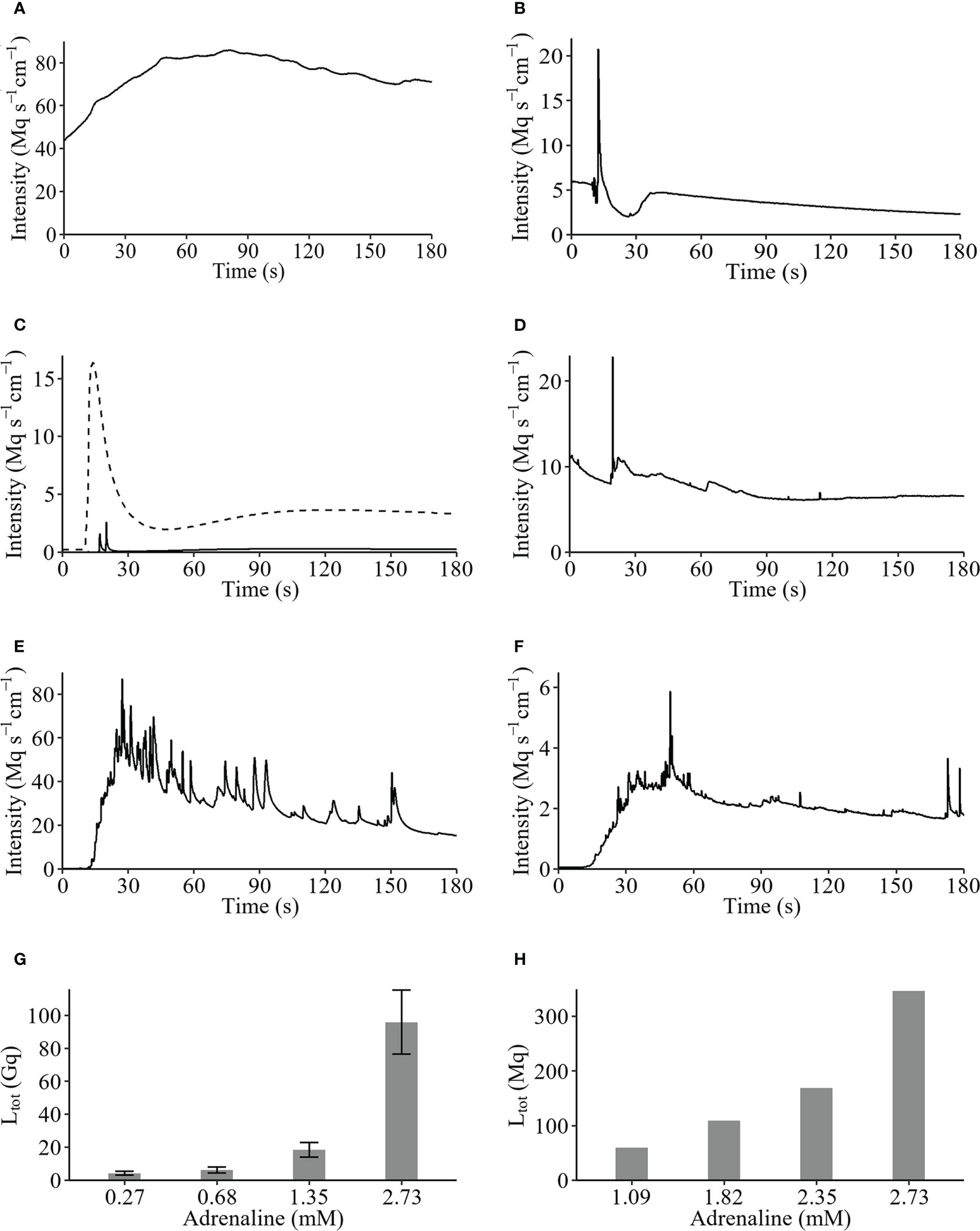
Figure 2 Original recordings of Crinoidea species. (A) Spontaneous luminescence of Monachocrinus cf. aotearoa; KCl-induced luminescences of (B) Adelatelecrinus cf. vallatus arm, (C) Stiremetra breviradia arm (full line) and calyx (dotted line), and (D) Thalassometra cf. gracilis arm; adrenaline 2.7 mM stimulation on (E) S. breviradia and (F) T. cf. gracilis arms; dose-response to adrenaline for (G) S. breviradia and (H) T. cf. gracilis arms expressed as total light (Ltot) emitted during 190 seconds in Gigaquanta and Megaquanta, respectively.
Original recordings of adrenaline 2.7 mM induced-luminescence of S. breviradia and T. cf gracilis show a long lasting glow with numerous fused flashes of light (Figures 2E, F). Adrenaline dose-response show an increase of total light production from 0.27 to 2.73 mM for S. breviradia with a sharp increase from 1.35 to 2.73 mM and a maximal value of 9590 ± 1942 Mq (n=6) (Figure 2G). Considering these results, an adrenaline dose-response range from 1.09 to 2.73 mM was tested on only one T. cf gracilis. A similar pattern is observed with weaker Ltot values peaking at 368.20 Mq for 2.73 mM (Figure 2H). Pharmacological detailed results obtained for adrenaline dose -response on arms from S. breviradia and T. cf gracilis are shown in Supplementary Tables 1B, C respectively. It can be noticed that at the same adrenaline concentration, the luminescence of S. breviradia’s calyx is seven-fold brighter than that of its arm (Supplementary Table 1B).
3.3 Histological analysis
The classical comatulid arm structure (Figure 3A) is found in the studied Crinoidea. Epifluorescence observations on the whole arm and in sections show that the saccules are highly fluorescent, standing out compared to the epifluorescence of surrounding arm tissues in all species, even in the non-luminescent A. bifida. Saccules were observed all along the ventral side of the arms and pinnules in all four species we had tissue from (i.e. S. breviradia, M. cf. aotearoa, T.cf gracilis, A. bifida - Figures 3B–E). Saccules of M. cf. aotearoa measure on average 0.15 ± 0.01 mm long (n=25), with no size difference between arm and pinnule saccules. A closer look at some saccules shows different granulations of their content (Figure 3B). Intervals between saccules are 0.54 ± 0.04 mm (n=22). Sometimes agglomerations of two or three saccules are visible (Figure 3E). The tested T.cf gracilis has smaller saccules on the pinnules of 0.09 ± 0.005 (n=23), with a mean interval of 0.45 ± 0.02 mm (n=19).
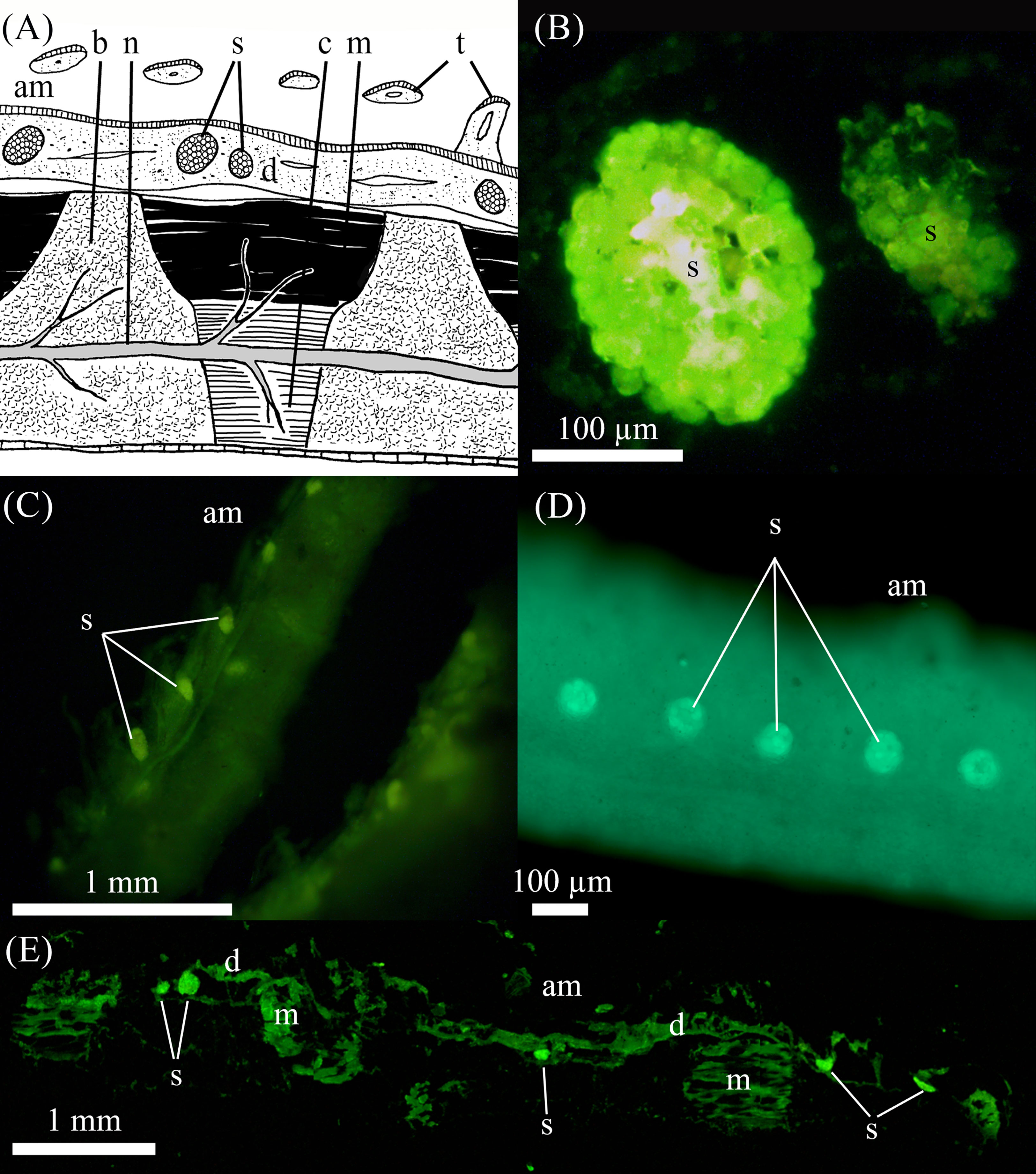
Figure 3 Auto fluorescence of the various arm and pinnules in Crinoidea.(A) Schematic drawing of cross section in Antedon sp. arm (adapted from Candia Carnevali and Saita, 1985) showing the diverse structures; (B) sagittal cryosection into two saccules of Monachocrinus cf. aotearoa observed under epifluorescent blue light stimulation; in toto observation of pinnules under blue light stimulation in (C) Thalassometra cf. gracilis and (D) A bifida; (E) sagittal cryosection into an arm piece of M. cf. aotearoa observed under epifluorescent blue light stimulation. am: ambulacral groove, b: brachial ossicle, c: connective tissue, d: dermis, m: muscle, n: nerve, s: sacculi, t: tentacle.
3.4 Thalassometra cf gracilis luminous system
Both, coelenterazine and luciferase activity assays made with our crushed T. cf gracilis arms were positive with mean values of 4.23 ± 1.16 ng g-1 (n=5) for coelenterazine content and of 4.53 ± 1.49 1012 q s-1 g-1. (n=7) for luciferase activity (Supplementary Table 1E).
3.5 Luminescence distribution among Crinoidea
A simplified phylogeny illustrating the bioluminescence distribution in Crinoidea has been produced using literature and experimental data (Figure 4). Luminescence can be found in three different Crinoidea orders: Hyocrinida, Isocrinida and Comatulida. Notably, inside Comatulida, the expedition IN2017_V03 allowed us to test four different species inside the Bourgueticrinina clade: two proved to be bioluminescent, A. cf. vallatus and M. cf. aotearoa, and another two, B. cf. australocrucis and D. cf. japonicus, can be both considered non-luminous. Moreover, also in Comatulida, our study adds two more bioluminescent species inside the family Thalassometridae. Taking into account the results from Herring (1974) for the pentametrocrinid T. jungerseni we find bioluminescence in three out of the four main Comatulida clades distinguished by Hemery et al. (2013).
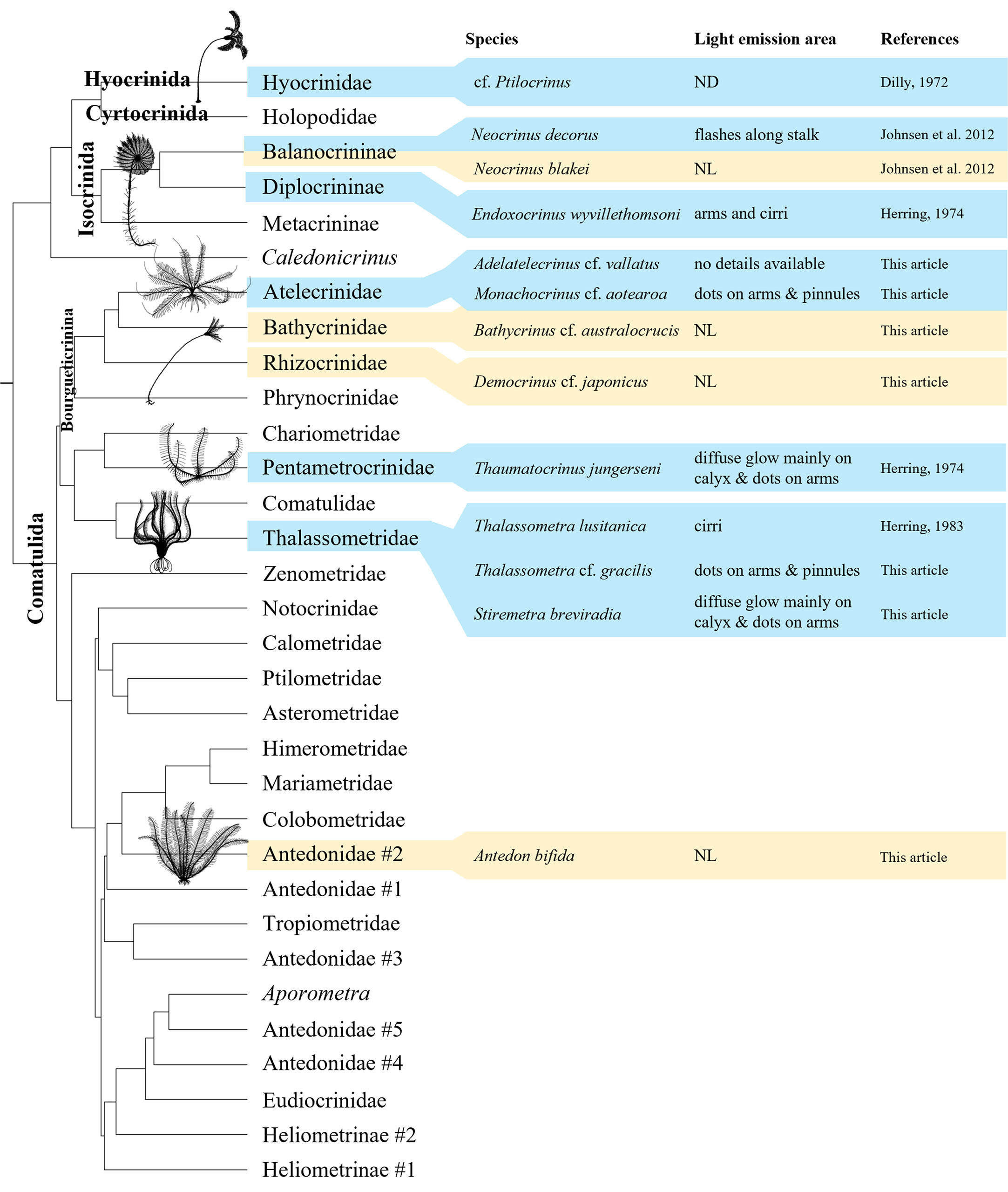
Figure 4 Distribution of luminescence within the class Crinoidea according to the classification based on the latest molecular advancements (Hemery et al., 2013). Clades presenting bioluminescent species are indicated in blue while clades with only non-bioluminescent tested species are in light yellow. Classification of Balanocrininae and Rhizocrinidae follows respectively Améziane et al., 2021 and Roux et al., 2019 (contra Hess and Messing, 2011). Original drawings made by PM-S.
4 Discussion
The major problem with deep-sea research is the challenging access to samples in good conditions. The number of sampling occasions is limited since important logistics and financial means are required (Brökeland and George, 2009). Missions like “Mapping benthic ecosystems on the deep continental shelf and slope in Australia’s Southwestern Region” and “Sampling the abyss” were unique opportunities to collect good quality abyssal samples of fragile organisms enabling the study of their bioluminescence. Thereby, despite the low sample number, interesting insights into deep-sea Crinoidea bioluminescence have been accomplished.
Based on in vivo photographs of the whole organisms, we illustrated the bioluminescence patterns of M. cf. aotearoa, T. cf. gracilis and S. breviradia, corroborated by luminometric measurements. Recording of luminescence induced by KCl depolarization of A. cf. vallatus allows us to confirm the bioluminescent status of this species, even if no photograph of the luminous pattern was obtained. Conversely, for B. cf. australocrucis and D. f. japonicus, the absence of any significant light emission regardless of the stimuli used (mechanical, KCl, or FW) refrained to consider these organisms as luminous. This absence of luminescence needs to be taken with caution as mentioned by Herring (1987): “A negative observation in one situation cannot provide absolute proof that an organism is not bioluminescent in any other circumstance…”. The collection of deep-sea organisms is a very difficult task, problematic for the organisms. Therefore, in situ observations constitute the optimal conditions to confirm or not the bioluminescent status of these species.
In T. cf. gracilis, the absence of a luminous gradient along the arms as well as extremely low values observed from calyx and cirri are consistent with the observations made by Herring (1974) on T. lusitanica. Similarly, S. breviradia’s luminous pattern reveals a stronger glow diffusing from the calyx and luminous dots along the arms, which was also described for T. jungerseni (Pentametrocrinidae) by Herring (1974). Thereby, on one hand the presence of two different emission patterns in Thalassometridae, and on the other hand, the emission pattern convergence observed in two distinct clades, supports the hypothesis that bioluminescence use might be related to different ecological functions.
KCl-induced luminescence supports the depolarization of excitable cells under a physiological nervous control in Crinoidea which is in line with Herring (1974) and what is known from Ophiuroidea (Mallefet, 1999; Mallefet, 2009, Mallefet, 2022). Furthermore, we find that in both tested Thalassometridae, light emission is under extrinsic adrenergic control (indicating the presence of adrenergic receptors), and the response is dose-dependent to adrenaline concentrations. In T. jungerseni (Pentametrocrinidae), Herring (1974), did not obtain any response through local application of adrenaline or noradrenaline. In ophiuroids, catecholamines have been shown to have different effects, acting as (i) positive neuromodulators of light emission in the ophiuroids Ophiopsila californica and Amphiura filiformis, (ii) no effect in Ophionereis fasciata, Ophionereis schayeri and Ophiopsila aranea (Mallefet, 2009), and (iii) neuro inhibitors on acetylcholine-induced luminescence in the ophiuroid Amphipholis squamata (De Bremaeker et al., 2000; Dupont et al., 2004). Little information is available concerning pharmacological studies in Crinoidea except catecholamines presence in Antedon bifida (Pentreath and Cobb, 1972) and the participation of dopamine and glutamate during arm autotomy and regeneration (Candia Carnevali and Bonasoro, 2001; Wilkie et al., 2010). The present results highlight for the first time the involvement of adrenaline in the bioluminescence control of two deep-sea Crinoidea. Herring (1974) found no response when treating T. jungerseni with adrenaline which would suggest possible different control mechanisms in different crinoid clades. However, as no pharmacological protocol or concentration information are available, the inactivity of adrenaline in this pentametrocrinid remains to be confirmed.
Comparing in vivo pictures and histology we hypothesize that, given the position, estimated size and space in between, the glowing dots observed in M. cf. aotearoa and T. cf. gracilis are actually the sacculi in arm and pinnules. We further hypothesize that this might also be the case for the glowing dots observed in T. jungerseni by Herring (1974). The autofluorescence of saccules, in our bioluminescent species, cannot be used as proof for the presence of luminous compound since saccules of A. bifida, a non-luminescent species, show a very bright fluorescent signal as well. Besides, saccules are present in most Comatulida, luminous or not, thus, it is very likely that other functions than bioluminescence are fulfilled by saccules. The latter have been poorly studied and they are generally suggested to be involved in excretion, mucus secretion or limestone production (Prenant, 1928; Holland, 1967; Heinzeller and Welsch, 1994). The use of sacculi for light emission is in line with a physiological nervous control as these organs are innervated (Holland, 1967; Heinzeller and Welsch, 1994).
The ability to emit light has evolved at least 94 times in the tree of life (Lau and Oakley, 2020) and in echinoderms bioluminescence appeared at least twice in Ophiuroidea given the presence of two very distinct light emission systems (Shimomura, 1986; Mallefet et al., 2013; Delroisse et al., 2017b). However, more originations are suggested by the diversity of nervous control mechanisms in this class (Mallefet et al. in prep.). In Crinoidea, the presence of luminous representatives has been established in two orders (Isocrinida and Comatulida) and is anecdotal in a third order (Hyocrinida). Different body parts seem to be able to emit light even inside the same clade and family, and we established the hypothesis of light emission through the sacculi in two very distinct clades inside Comatulida (Thalassometridae and Bourgueticrinina). Considering color, inside Thalassometridae, the green emission of Stiremetra strongly suggests the presence of a green fluorescent protein (GFP) which might not be present in T. cf. gracilis (and M. cf. aotearoa) given its mainly blue emission. Moreover, if Herring (1974) observations on T. jungerseni were to be verified, this would mean possibly two different control mechanisms in different clades. All this diversity suggests that this trait plays an important ecological role for deep sea crinoids.
Recent molecular studies establish Monachocrinus as sister-clade to Atelecrinidae and put the remaining Bathycrinidae as sister-clade to Rhizocrinidae (Eléaume et al. in prep). Future endeavors should therefore test if bioluminescence is an autapomorphy of the Monachocrinus-Atelecrinidae clade or if there might have been a common bioluminescent ancestor to Bourgueticrinina. Given the sporadic appearance of bioluminescence in very distant crinoid clades and the diversity in phenotypes, it is tempting to hypothesize several independent appearances throughout their evolutionary history. However, having only been able to determine the light emission system in one species, given the limited number of species tested and our developing knowledge ofCrinoidea evolutionary, it remains yet to be proven if the ability to luminesce in deep-sea crinoids is due to independent origins or one common bioluminescent ancestor.
Mechanical-induced luminescence of T. jungerseni by Herring (1974) and M. cf. aotearoa in the present study, indicates that crinoid’s bioluminescence can be elicited as a response to contact with other organisms. Herring (1974) also reports an increase of luminescence in T. jungerseni through increased rubbing. Our results similarly highlight an increase of the luminescence in response to raising adrenaline concentrations for the tested Thalassometridae. Moreover, we recorded a wide range of light patterns ranging from strong, long-lasting glows of M. cf. aotearoa under mechanical stimulation, to lower glows with superimposed brief flashes in adrenalin tested Thalassometridae. Crinoids are, therefore, sedentary or sessile organisms able to produce light in response to mechanical stimulation, with different light emission patterns, and which is proportional to the stimulus strength. This would confirm the contact flash predator deterrence function (cfpd) as described by Morin (1983). Cfpd includes startling effects, aposematic signals as well as alarm and mimetic signals (Morin, 1983). However, predator evasion can be done through other multiple non-mutually exclusive ways like sacrificial lures and burglar alarm (Morin, 1983). Most of these functions have been described within the other echinoderm classes. Thus, altough nothing is known about the unpalatability or unprofitability of deep-sea crinoids for their potential predators, it is well documented that some shallow water crinoids produce quinones as defence molecules (Caulier et al., 2022). Light production in the dark could warn the predator of crinoid unpalatability as shown in ophiuroids (Grober, 1988; Jones and Mallefet, 2010). The use of light emission as decoy/sacrificial lure is supported by their capacity to emit long-lasting glows (Morin, 1983). Here, a continuously emitting body part attracts the predator away from essential ones or those more difficult to regrow. As crinoids autotomize readily (Candia Carnevali and Bonasoro, 2001), the use of sacrificial lures in combination with autotomy can also be hypothesized, again as seen in ophiuroids (Mallefet, 2009; Jones and Mallefet, 2013). Let’s note that while crinoids are generally not fast enough to escape highly movable predators like fish (Meyer and Ausich, 1983) this is not necessarily a requirement for the lure to work as long as the rest of the animal is hidden in darkness. Nevertheless, ROV observations show that some crinoids, notably some Atelecrinidae, are capable of rapid escape movements (Eléaume et al., in prep) in which the use of startling effects and sacrificial lures in a more “classic” manner (with the rest of the animal escaping quickly) could be tested. The use of light emission for burglar alarm (i.e. attracting a secondary predator by illuminating the primary predator) can also be hypothesized in Crinoidea given that this defence mechanism can work with flashes and glows, that they easily autotomize (which could allow to mark the primary predator without sacrificing itself) and that this function has been reported for Ophiuroidea (Deheyn et al., 2000b; Mallefet, 2009; Jones and Mallefet, 2013) and is highly possible in Holothuroidea (Robison, 1992).
Finally, an interesting hypothesis by Holland et al. (1991), is the use of bioluminescence in Crinoidea to attract prey. The deep-sea is known as an environment with low food availability hence a role of bioluminescence to attract plankton could be hypothesized. Light lures are widely used in the deep ocean in very different clades from anglerfishes to siphonophores (Johnsen et al., 1999; Haddock et al., 2005; Pietsch, 2009; Haddock et al., 2010). However, we lack proof of direct spontaneous light emission other than weak glows and, furthermore, this seems on first sight rather unlikely in Crinoidea as they are lee-side suspension feeders, putting their aboral side into the current, hence, the light points do not face the water current containing its planktonic prey (Herring, 1974; Morin, 1983; Baumiller, 2008). Nevertheless, a long-lasting glow from the calyx in species clinging to perches could serve to concentrate planktonic prey before passing through the arms, and the dots on the oral side of the arms could attract planktonic prey stuck in the reduced current after crossing the arm-fan. This light emission could be initiated after contact with planktonic prey, reducing the importance of spontaneous light emission for this function. Future in situ observations might further enlighten this discussion.
Our biochemical results reveal for the first time the nature of the luminous system of T. cf. gracilis as a luciferin-luciferase system based on coelenterazine. In 1974, Herring obtained the indication of a luciferin-luciferase system for T. jungerseni, using the hot and cold extracts assay but without being able to identify the nature of the bioluminescent compounds. In other Echinodermata, only two brittlestars have been studied, with O. californica presenting a photoprotein system requiring H2O2 as cofactor to trigger luminescence (Shimomura, 1986) while in A. filiformis a luciferin-luciferase system using coelenterazine and a Renilla-like luciferase is involved (Mallefet et al., 2013; Delroisse et al., 2017b). It is thus, after the ophiuroid A. filiformis, the second time that a coelenterazine-based luminous system is described for Echinodermata and the first time coelenterazine is proven as the luciferin in a non-ophiuroid echinoderm species. The coelenterazine concentration and luciferase activity are close to those observed in other metazoan species (Table 1) (Shimomura and Yampolsky, 2019; Mallefet et al., 2020). The widespread occurrence of coelenterazine within marine fauna is often explained by acquisition through the organism’s diet (Frank et al., 1984; Haddock et al., 2001; Bessho-Uehara et al., 2020; Mallefet et al., 2020). Most recently, dietary acquisition of coelenterazine was demonstrated and monitored for the ophiuroid A. filiformis (Mallefet et al., 2020). Although, deep-sea observations are limited, the hypothesis of a dietary acquisition of coelenterazine in bioluminescent Crinoidea appears plausible considering that they are filter feeders and have been recorded in contact with bioluminescent plankton (Gillibrand et al., 2007).
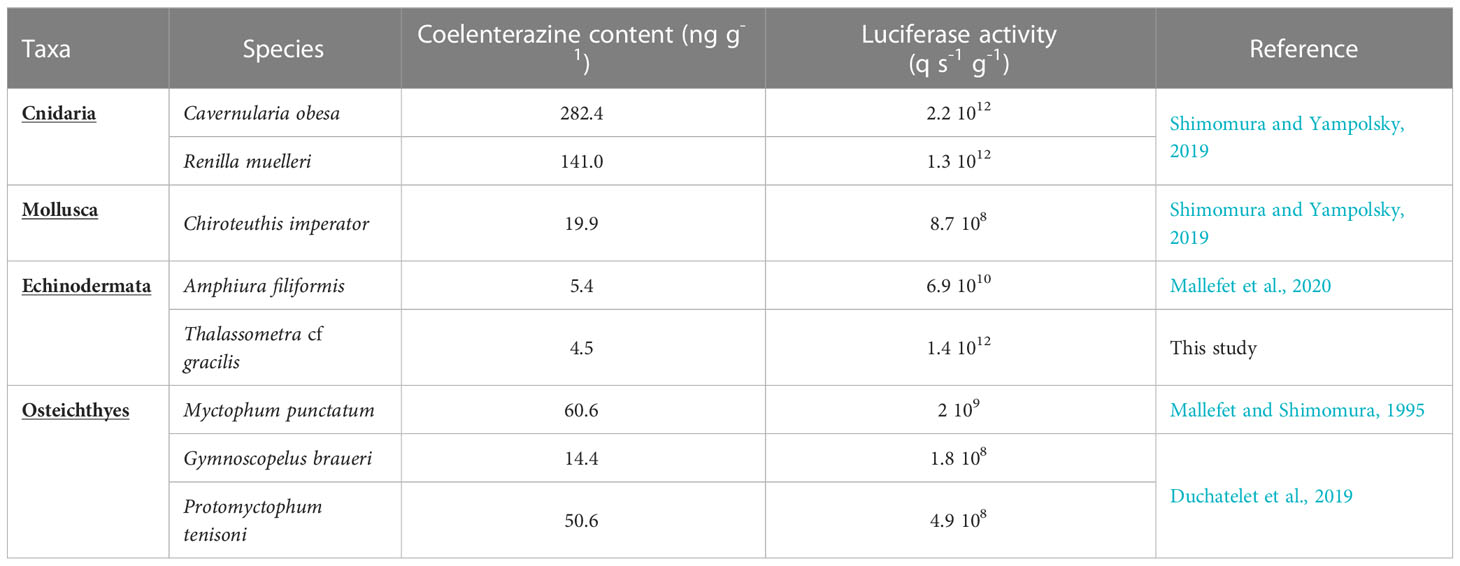
Table 1 Coelenterazine content and luciferase activity for different marine species from different Taxa. Data are normalized per gram of tissue.
In conclusion, this study presents four new records of bioluminescence in deep-sea Comatulida in two distinct phylogenetic clades, doubling the number of known Crinoidea species with this capability. In vivo photography and histology suggest that, in at least two of species, light emission might originate from the sacculi. As of today, this is the best-documented function for these enigmatic organs. Two Thalassometridae species use adrenaline as a neurotransmitter to control their light emission. The first identified luciferin in crinoids is coelenterazine, and the light-emission system of T. cf. gracilis, a coelenterazine-luciferase system appears very similar to the one observed in the Ophiuroidea, A. filiformis. The results, while not definitive, do suggest multiple independent appearances of bioluminescence among Crinoidea species possibly linked to different predator deterrence strategies. The deep-sea remains largely terra incognita. The vastest biome on Earth deserves more attention to apprehend how it is structurated. Increased sampling and studies on rare if not unsuspected inhabitants is a challenge for the future. Further studies on abyssal organisms will help elucidate bioluminescence’s diversity and ecological importance in this realm devoid of non-biological light.
Data availability statement
The original contributions presented in the study are included in the article/Supplementary material. Further inquiries can be directed to the corresponding author.
Author contributions
JM and TH collected the samples. JM collected luminometry data, preserved some tissues and obtained the bioluminescence pictures on board during the surveys. ME identify the species. JM, PM-S performed pharmacological and biochemical studies. PM-S and LD performed the classical histology analyse. ME and PM-S performed the analyse of bioluminescence repartition across Crinoidea. PM-S, LD and JM were major contributors to the initial manuscript. All authors contributed to the article and approved the submitted version.
Funding
This work was supported by an F.R.S.-FNRS FRIA grant to LD. JM was supported by F.R.S.-FNRS travel grants and UCLouvain fund for OAAPC. ME and PM-S were funded by the MNHN grant scheme “Projets fédérateurs du département Origines et Evolution” entitled “Anatomie comparée des crinoïdes pédonculés actuels et fossiles: exploration des relations entre les faunes du Tertiaire et de l’actuel”. PM-S acknowledges support from the Luxembourg National Research Fund (FNR) under project number 17129770.
Acknowledgments
“Mapping benthic ecosystems on the deep continental shelf and slope in Australia’s South west Region” was led by CSIRO under chief scientist Dr. A. Williams; “Sampling the Abyss” was led by Museums Victoria under Chief Scientist Dr. TH and supported by CSIRO Marine National Facility and NESP Marine Biodiversity Hub. The authors acknowledge the scientific and skilledl crews of the RV Southern Surveyor and RV Investigator, the joint efforts of all made these researches possible. The authors thank Dr G. Caulier for collecting A. bifida in Concarneau and C. Coubris for the help during luminometric data analyses. JM is research associate FNRS, PM is a FNR doctoral fellow, ME is Associate professor at MNHN, TO is Researcher at Museums Victoria, and LD is post-doctoral researcher at UCLouvain. This study is the contribution BRC #400 of the Biodiversity Research Center (UCLouvain) from the Earth and Life Institute Biodiversity (ELIV) and the “Centre Interuniversitaire de Biologie Marine” (CIBIM). We thank the reviewers for their interesting input that contributed with certainty to the improvement of this manuscript.
Conflict of interest
The authors declare that the research was conducted in the absence of any commercial or financial relationships that could be construed as a potential conflict of interest.
Publisher’s note
All claims expressed in this article are solely those of the authors and do not necessarily represent those of their affiliated organizations, or those of the publisher, the editors and the reviewers. Any product that may be evaluated in this article, or claim that may be made by its manufacturer, is not guaranteed or endorsed by the publisher.
Supplementary material
The Supplementary Material for this article can be found online at: https://www.frontiersin.org/articles/10.3389/fmars.2023.1136138/full#supplementary-material
References
Améziane N., Eléaume M., Roux M. (2021). Ontogeny of non-muscular brachial articulations in balanocrininae (Echinodermata, crinoidea): iterative trajectories or phylogenetic significance? Zoomorphology 140, 47–67. doi: 10.1007/s00435-020-00508-y
Améziane N., Roux M. (2005). Environmental control versus phylogenic fingerprint in ontogeny: The example of the development of the stalk in the genus Guillecrinus (stalked crinoids, Echinodermata). J. Nat. Hist. 39, 2815–2860. doi: 10.1080/00222930500060595
Baumiller T. K. (2008). Crinoid ecological morphology. Annu. Rev. Earth Planet. Sci. 36, 221–249. doi: 10.1146/annurev.earth.36.031207.124116
Bessho-Uehara M., Yamamoto N., Shigenobu S., Mori H., Kuwata K., Oba Y. (2020). Kleptoprotein bioluminescence: Parapriacanthus fish obtain luciferase from ostracod prey. Sci. Adv. 6 (2), eaax4942. doi: 10.1126/sciadv.aax4942
Birk M. H., Blicher M. E., Garm A. (2018). Deep-sea starfish from the Arctic have well-developed eyes in the dark. Proc. R. Soc B Biol. Sci. 285, 20172743. doi: 10.1098/rspb.2017.2743
Brehm P., Morin J. G. (1977). Localization and characterization of luminescent cells in Ophiopsila californica and Amphipholis squamata (Echinodermata: Ophiuroidea). Biol. Bull. 152, 12–25. doi: 10.2307/1540723
Brökeland W., George K. H. (2009). Editorial: Deep-sea taxonomy — a contribution to our knowledge of biodiversity. Zootaxa 2096, 3. doi: 10.11646/zootaxa.2096.1.2
Buchanan J. B. (1963). Mucus secretion within the spines of ophiuroid echinoderms. Proc. Zool. Soc Lond. 141, 251–259. doi: 10.1111/j.1469-7998.1963.tb01611.x
Candia Carnevali M. D., Bonasoro F. (2001). Microscopic overview of crinoid regeneration. Microsc. Res. Tech. 55, 403–426. doi: 10.1002/jemt.1187
Candia Carnevali M. D., Saita A. (1985). Muscle system organization in the echinoderms: III. fine structure of the contractile apparatus of the arm flexor muscles of the comatulids (Antedon mediterranea). J. Morpho. 185 (1), 75–87. doi: 10.1002/jmor.1051850106
Caulier G., Lourtie A., Brasseur L., Mallefet J., Gerbaux P., Flammang P., et al. (2022). Crinoid anthraquinones as kairomones allowing host selection for the symbiotic snapping shrimp Synalpheus stimpsonii. Chemoecology 32, 95–104. doi: 10.1007/s00049-022-00368-6
Davis M. P., Sparks J. S., Smith W. L. (2016). Repeated and widespread evolution of bioluminescence in marine fishes. PloS One 11 (6), e0155154. doi: 10.1371/journal.pone.0155154
De Bremaeker N., Baguet F., Mallefet J. (2000). Effects of catecholamines and purines on luminescence in the brittlestar Amphipholis squamata (Echinodermata). J. Exp. Biol. 203, 2015–2023. doi: 10.1242/jeb.203.13.2015
Deheyn D., Alva V., Jangoux M. (1996). Fine structure of the photogenous areas in the bioluminescent ophiuroid Amphipholis squamata (Echinodermata, ophiuridea). Zoomorphology 116, 195–204. doi: 10.1007/BF02527159
Deheyn D., Mallefet J., Jangoux M. (1997). Intraspecific variations of bioluminescence in a polychromatic population of Amphipholis squamata (Echinodermata: Ophiuroidea). J. Mar. Biol. Assoc. U. K. 77, 1213–1222. doi: 10.1017/S0025315400038728
Deheyn D., Mallefet J., Jangoux M. (2000a). Evidence of seasonal variation in bioluminescence of Amphipholis squamata (Ophiuroidea, echinodermata): effects of environmental factors. J. Exp. Mar. Biol. Ecol. 245, 245–264. doi: 10.1016/S0022-0981(99)00166-5
Deheyn D., Mallefet J., Jangoux M. (2000b). Expression of bioluminescence in Amphipholis squamata (Ophiuroidea: Echinodermata) in presence of various organisms: a laboratory study. J. Mar. Biol. Assoc. U. K. 80, 179–180. doi: 10.1017/S0025315499001733
Delroisse J., Ullrich-Lüter E., Blaue S., Eeckhaut I., Flammang P., Mallefet J. (2017a). Fine structure of the luminous spines and luciferase detection in the brittle star Amphiura filiformis. Zool. Anz. 269, 1–12. doi: 10.1016/j.jcz.2017.05.001
Delroisse J., Ullrich-Lüter E., Blaue S., Ortega-Martinez O., Eeckhaut I., Flammang P., et al. (2017b). A puzzling homology: a brittle star using a putative cnidarian-type luciferase for bioluminescence. Open Biol. 7, 160300. doi: 10.1098/rsob.160300
Delval S., Mallefet J. (2010). “Proximal to distal gradient of luminescence in the arm of amphiura filiformis (Echinodermata-ophiuroidea),” in Echinoderms: Durham - proceedings of the 12th international echinoderm conference. Ed. Harris L. G., Böttger S. A., Walker C. W., Lesser M. P. (Durham, New Hampshire, USA: CRC Press), 355–357. doi: 10.1201/9780203869543-c55
Dewael Y., Mallefet J. (2002). Luminescence control in ophiuroids (Echinodermata) does not share a common nervous control in all species. J. Exp. Biol. 205, 799–806. doi: 10.1242/jeb.205.6.799
Dilly P. N. (1973). Enigma of coloration and light emission in deep-sea animals. Endeavour 32 (115), 25–29.
Duchatelet L., Hermans C., Duhamel G., Cherel Y., Guinet C., Mallefet J. (2019). “Coelenterazine detection in five myctophid species from the kerguelen plateau,” in Proceedings of the second symposium on kerguelen plateau: marine ecosystem and fisheries. Eds. Welsford D., Dell J., Duhamel G. (Kingston, Tasmania, Australia: Australian Antarctic Division), 31–41.
Dupont S., Mallefet J., Vanderlinden C. (2004). Effect of β-adrenergic antagonists on bioluminescence control in three species of brittlestars (Echinodermata: Ophiuroidea). Comp. Biochem. Physiol. C 138 (1), 59–66. doi: 10.1016/j.cca.2004.04.007
Frank T. M., Widder E. A., Latz M. I. (1984). Dietary maintenance of bioluminescence in a deep-sea mysid. J. Exp. Biol. 108, 385–389. doi: 10.1242/jeb.109.1.385
Gillibrand E. J. V., Bagley P., Jamieson A., Herring P. J., Partridge J. C., Collins M. A., et al. (2007). Deep sea benthic bioluminescence at artificial food falls, 1,000–4,800 m depth, in the porcupine seabight and abyssal plain, north East Atlantic ocean. Mar. Biol. 150, 1053–1060. doi: 10.1007/s00227-006-0407-0
Grober M. S. (1988). Brittle-star bioluminescence functions as an aposematic signal to deter crustacean predators. Anim. Behav. 36, 493–501. doi: 10.1016/S0003-3472(88)80020-4
Guilford T., Cuthill I. (1989). Aposematism and bioluminescence. Anim. Behav. 37, 339–341. doi: 10.1016/0003-3472(89)90126-7
Haddock S. H. D., Casey D. W., Pugh P. R., Schnitzler C. E. (2005). Bioluminescent and red-fluorescent lures in a deep-sea siphonophore. Science 309, 263–263. doi: 10.1126/science.1110441
Haddock S. H. D., Moline M. A., Case J. F. (2010). Bioluminescence in the sea. Annu. Rev. Mar. Sci. 2, 443–493. doi: 10.1146/annurev-marine-120308-081028
Haddock S. H. D., Rivers T. J., Robison B. H. (2001). Can coelenterates make coelenterazine? dietary requirement for luciferin in cnidarian bioluminescence. Proc. Natl. Acad. Sci. 98, 11148–11151. doi: 10.1073/pnas.201329798
Heinzeller T., Welsch U. (1994). “Crinoidea,” in Microscopic anatomy of invertebrates (New York, USA: Wiley-Liss, Inc.), 9–148.
Hemery L. G., Roux M., Améziane N., Eléaume M. (2013). High-resolution crinoid phyletic inter-relationships derived from molecular data. Cah. Biol. Mar. 54, 511–523.
Herring P. J. (1974). New observations on the bioluminescence of echinoderms. J. Zool. 172, 401–418. doi: 10.1111/j.1469-7998.1974.tb04116.x
Herring P. J. (1983). The spectral characteristics of luminous marine organisms. Proc. R. Soc Lond. B Biol. Sci. 220, 183–217. doi: 10.1098/rspb.1983.0095
Herring P. J. (1987). Systematic distribution of bioluminescence in living organisms. J. Biolumin. Chemilumin. 1, 147–163. doi: 10.1002/bio.1170010303
Herring P. J. (1995). “Bioluminescent echinoderms: Unity of function in diversity of expression?,” in Echinoderm research 1995 (Rotterdam: Emson, Smith & Campbell).
Hess H., Messing C. G. (2011). Treatise on invertebrate paleontology, part T, Echinodermata 2 revised, crinoidea 3 (Lawrence: University of Kansas and Paleontological Institute), 216.
Holland N. D. (1967). Some observations on the saccules of Antedon mediterranea (Echinodermata, crinoidea). Pubblicazioni Stazione Zool. Napoli 35, 257–262.
Holland N. D., Grimmer J. C., Wiegmann K. (1991). The structure of the sea lily Calamocrinus diomedae, with special reference to the articulations, skeletal microstructure, symbiotic bacteria, axial organs, and stalk tissues (Crinoida, millericrinida). Zoomorphology 110, 115–132. doi: 10.1007/BF01632868
Johnsen S., Balser E. J., Fisher E. C., Widder E. A. (1999). Bioluminescence in the deep-sea cirrate octopod Stauroteuthis syrtensis verrill (Mollusca: Cephalopoda). Biol. Bull. 197, 26–39. doi: 10.2307/1542994
Johnsen S., Frank T. M., Haddock S. H. D., Widder E. A., Messing C. G. (2012). Light and vision in the deep-sea benthos: I. bioluminescence at 500-1000 m depth in the Bahamian islands. J. Exp. Biol. 215, 3335–3343. doi: 10.1242/jeb.072009
Jones A., Mallefet J. (2010). Aposematic use of bioluminescence in Ophiopsila aranea (Ophiuroidea, Echinodermata). Luminescence 25, 81–216.
Jones A., Mallefet J. (2012). Study of the luminescence in the black brittle-star Ophiocomina nigra: toward a new pattern of light emission in ophiuroids. Zoosymposia 7, 139–145. doi: 10.11646/zoosymposia.7.1.13
Jones A., Mallefet J. (2013). Why do brittle stars emit light? behavioural and evolutionary approaches of bioluminescence. Cah. Biol. Mar. 54, 729–734.
Lau E. S., Oakley T. H. (2020). Multi-level convergence of complex traits and the evolution of bioluminescence. Biol. Rev. 96, 673–691. doi: 10.1111/brv.12672
Mallefet J., Shimomura O. (1995). Presence of coelenterazine in mesopelagic fishes from the Strait of Messina. Marine Biology: International journal on life in oceans and coastal waters 124 (3), 381–385. doi: 10.1007/BF00363911
Mallefet J. (1999). “Physiology of bioluminescence in echinoderms,” in Echinoderms research 1998. Eds. Candia Carnevali M. D., Bonasoro F. (Rotterdam, Netherlands: Balkema), 93–102.
Mallefet J. (2009). “Echinoderm bioluminescence: Where, how and why do so many ophiuroids glow?,” in Bioluminescence in focus: A collection of illuminating essays. Ed. Meyer-Rochow V. B. (Kerala, India: Research Singpost), 67–83.
Mallefet J. (2022). “New lights on echinoderm’s bioluminescence,” in 21st international symposium on bioluminescence & chemiluminescence (Gijon, Spain). Available at: http://hdl.handle.net/2078.1/262023.
Mallefet J., Duchatelet L., Coubris C. (2020). Bioluminescence induction in the ophiuroid Amphiura filiformis luciferase (Ophiuroidea, Echinodermata). J. Exp. Biol. 223 (4), jeb.218719. doi: 10.1242/jeb.218719
Mallefet J., Fujita T. (2014). First luminescence survey of Okinawa brittle stars. Luminescence 29, 29–30. doi: 10.1002/bio.2699_2
Mallefet J., Parmentier B., Mullier X., Shimomura O., Morsomme P. (2013). “Characterisation of amphiura filiformis luciferase (Ophiuroidea, Echinodermata),” in Echinoderms in a changing world. Ed. Johnson C. (London: Taylor & Francis group), 293.
Martini S., Haddock S. H. D. (2017). Quantification of bioluminescence from the surface to the deep sea demonstrates its predominance as an ecological trait. Sci. Rep. 7, 45750. doi: 10.1038/srep45750
Martini S., Kuhnz L., Mallefet J., Haddock S. H. D. (2019). Distribution and quantification of bioluminescence as an ecological trait in the deep sea benthos. Sci. Rep. 9, 14654. doi: 10.1038/s41598-019-50961-z
Meyer D. L., Ausich W. I. (1983). “Biotic interactions among recent and fossil crinoids,” in Biotic interactions in recent and fossil benthic communities, topics in geobiology. Eds. Tevesz M. F. S., McCall P. L. (New York: Springer), 378–420.
Pentreath V. W., Cobb J. L. S. (1972). Neurobiology of Echinodermata. Biol. Rev. 47, 363–392. doi: 10.1111/j.1469-185X.1972.tb00977.x
Pietsch T. W. (2009). “Bioluminescence and luring,” in Oceanic anglerfishes: Extraordinary diversity in the deep Sea. Ed. Pietsch T. W. (Berkeley: University of California Press), 229–251.
Prenant M. (1928). Notes sur les saccules de la comatule Antedon bifida (Pennant). Bull. la Société zoologique France 10, 195–202.
Robison B. H. (1992). Bioluminescence in the benthopelagic holothurian Enypniastes eximia. J. Mar. Biol. Assoc. U. K. 72, 463–472. doi: 10.1017/S0025315400037826
Rouse G. W., Jermiin L. S., Wilson N. G., Eeckhaut I., Lanterbecq D., Oji T., et al. (2013). Fixed, free, and fixed: The fickle phylogeny of extant crinoidea (Echinodermata) and their permian–Triassic origin. Mol. Phylogenet. Evol. 66, 161–181. doi: 10.1016/j.ympev.2012.09.018
Roux M., Eleaume M., Ameziane N. (2019). A revision of the genus conocrinus d'Orbigny 1850 (Echinodermata, crinoidea, rhizocrinidae) and its place among extant and fossil crinoids with a xenomorphic stalk. Zootaxa 4560 (1), 51–84. doi: 10.11646/zootaxa.4560.1.3
Shimomura O. (1986). Bioluminescence in the brittle star Ophiopsila californica. photochem. Photobiol 44, 671–674. doi: 10.1111/j.1751-1097.1986.tb04724.x
Shimomura O., Yampolsky I. V. (2019). Bioluminescence: chemical principles and methods. 3rd edition (Singapore: World Scientific Publishing), 1–556.
Vanderlinden C., Dewael Y., Mallefet J. (2003). Screening of second messengers involved in photocyte bioluminescence control of three ophiuroid species (Ophiuroidea: Echinodermata). J. Exp. Biol. 206 (17), 3007–3014. doi: 10.1242/jeb.00520
Vanderlinden C., Mallefet J. (2004). Synergic effects of tryptamine and octopamine on ophiuroid luminescence (Echinodermata). J. Exp. Biol. 207 (21), 3749–3756. doi: 10.1242/jeb.01209
Vanderlinden C., Mallefet J., Gailly P. (2010). “How do brittle stars control their light emission?,” in Echinoderms: Durham. Eds. Harris L. G., Böttger S. A., Walker C. W., Lesser M. P. (London: Taylor & Francis Group), 419–422.
Viviani D. (1805). Phosphorescencia maris quattuordecim lucescentium animalculorum novis speciebus illustrata (Genova).
Wilkie I. C., Barbaglio A., Maclaren W. M., Carnevali M. C. (2010). Physiological and immunocytochemical evidence that glutamatergic neurotransmission is involved in the activation of arm autotomy in the featherstar Antedon mediterranea (Echinodermata: Crinoidea). J. Exp. Biol. 213 (12), 2104–2115. doi: 10.1242/jeb.039578
Keywords: bioluminescence, Crinoid, echinoderm, pharmacology, luminometry, deep-sea
Citation: Mallefet J, Martinez-Soares P, Eléaume M, O’Hara T and Duchatelet L (2023) New insights on crinoid (Echinodermata; Crinoidea) bioluminescence. Front. Mar. Sci. 10:1136138. doi: 10.3389/fmars.2023.1136138
Received: 02 January 2023; Accepted: 23 February 2023;
Published: 07 March 2023.
Edited by:
Elva G. Escobar-Briones, Instituto de Ciencias del Mar y LimnologíaMacroecología y Biodiversidad, Universidad Nacional Autónoma de México, MéxicoReviewed by:
Matthew Scott Grober, Georgia State University, United StatesPedro Martinez, Universitat de Barcelona, Barcelona, Spain
Copyright © 2023 Mallefet, Martinez-Soares, Eléaume, O’Hara and Duchatelet. This is an open-access article distributed under the terms of the Creative Commons Attribution License (CC BY). The use, distribution or reproduction in other forums is permitted, provided the original author(s) and the copyright owner(s) are credited and that the original publication in this journal is cited, in accordance with accepted academic practice. No use, distribution or reproduction is permitted which does not comply with these terms.
*Correspondence: Jérôme Mallefet, SmVyb21lLm1hbGxlZmV0QHVjbG91dmFpbi5iZQ==
†These authors have contributed equally to this work