- 1Sedimentary Systems-Geology, Department of Geosciences and Natural Resource Management (IGN), University of Copenhagen, Copenhagen, Denmark
- 2Marine Biology Section, Department of Biology, University of Copenhagen, Helsingør, Denmark
- 3Danish Institute for Advanced Study (DIAS)/Nordcee, University of Southern Denmark, Odense, Denmark
- 4NORCE Climate & Environment, Bjerknes Centre for Climate Research, Bergen, Norway
- 5Department of Geological Mapping and Mineral Resources, Geological Survey of Denmark and Greenland (GEUS), Copenhagen, Denmark
- 6Environmental Archaeology and Materials Science, National Museum of Denmark, Kongens Lyngby, Denmark
Microbe-mineral interactions, such as mineral substrate utilization and aggregate formation, have played a key role in the cycling of elements through Earth evolution. In water, soils, and sediment biogeochemistry modulates microbial community composition and mineral formation over spatial and temporal scales. Plastic is a new material that is now widespread in the environment. Both microbial and mineral associations with plastic comprise the Plastisphere, which influences the fate of plastic. This study focuses on how the biogeochemical environment defines microbial and mineral association with polyethylene (PE) and polystyrene (PS) over a 12-month period in a temperate coastal harbor. The coastal harbor environment was separated into 3 conceptual compartments defined by physical and biogeochemical conditions, that allow transfer of electrons between species e.g., light penetration and redox setting. Microbe and mineral association were investigated in the water column, top sediment, and bottom sediment by applying a range of modern analytical techniques to identify changes in the chemical structures of plastics, microbial community development, metal, salt and mineral formation. The epiplastic microbial community was distinct to that of the surrounding environment across changing redox conditions. The type and oxidation state of metallic minerals formed on plastics or entrapped in the biofilm matrix related to the dominant abiotic and biotic processes across redox conditions. FTIR spectroscopy indicated the occurrence of PE and PS oxidation in the various biogeochemical environments. Combined, these findings demonstrate that redox conditions and surrounding biogeochemistry mediate the composition of mineralogical and biological loading of PE and PS in coastal marine environments. This suggests that the biogeochemical setting in which the plastics are stored constrains the development of plastic interfacial biogeochemistry and the potential for plastic degradation and transport over time.
Introduction
Physical, chemical, and biological reactions define the biogeochemical properties of an environment and drive all element cycling and electron exchange between components and across redox settings (Jørgensen, 2000). Plastics have emerged as a ubiquitous pollutant, particularly in the coastal environment where they are found both in the water and sediment across changing redox conditions (Carpenter and Smith, 1972; Colton et al., 1974; Wong et al., 1974; Thompson et al., 2004; Jambeck et al., 2015; Barrett et al., 2020). Macro-, micro- and nanosized plastics enter the marine coastal environment from non-point terrestrial and aerosol sources, fishing and recreation practices, and via, e.g., currents, faecal pellet delivery, to eventually sink to the sediment (e.g., van Sebille et al., 2015; Taylor et al., 2016; Bergmann et al., 2017; Courtene-Jones et al., 2017; Cózar et al., 2017; Horton and Dixon, 2018; Peeken et al., 2018; Amaral-Zettler et al., 2020; Harris et al., 2023). During transport and storage, biogeochemical processes govern the microbial and elemental composition of the environment around the plastic and dictate interactions with the plastic as it moves from source to sink (Rogers et al., 2020). The link between the biogeochemical environments, the governing biotic and abiotic processes influencing the composition of the Plastisphere, and the stability of plastics in the water and sediment, however, is poorly defined.
The plastic surface is the first point of interaction between plastic and its environment. Biofilm on the surface of plastic debris in water and sediment has created a new ecological niche named the Plastisphere (Zettler et al., 2013). The Plastisphere is composed of microorganisms, organic debris, biogenic and abiogenic minerals, metals, and salts. On more developed surfaces, colonization advances to inhabit larger fauna, like worms and bivalves (Zettler et al., 2013). The characteristics of plastic (e.g., polymer type, morphology, size, degradation state) influence e.g., buoyancy, tastiness to biota, cellular uptake, and potential for particle-particle interactions, and in this way constrains interaction within aquatic biogeochemical pathways (Rogers et al., 2020; Wright et al., 2020; Galgani and Loiselle, 2021; Müller et al., 2021). The microbiota in the Plastisphere affect downward transport, food web uptake and degradation of plastic in water as well as in the pore space of soil and sediment (Amaral-Zettler et al., 2015; Jacquin et al., 2019; Amaral-Zettler et al., 2020; Coyle et al., 2020; Fabra et al., 2021; Polhill et al., 2022; Yu et al., 2023). Recent studies have indicated that the mineral, metal and salt component of the Plastisphere is also important for plastic transport in aquatic systems (Kowalski et al., 2016; Leiser et al., 2020; Rogers et al., 2020; Leiser et al., 2021; Müller et al., 2021; Ye et al., 2022). Nonetheless, the elemental composition and crystallinity of these minerals and metals, their abundance, and their distribution on plastic debris across redox environments lacks the characterization required to assess them as templates for microbial attachment and catalysts for microbial reactions associated with plastics. Characterization of microbial community dynamics together with mineral and metal development on plastic is needed to identify and evaluate how biogeochemical processes influence plastic transport or degradation in water and sediment pore water over time.
Besides fueling microbial processes, redox conditions facilitate continuous abiotic reactions in the sediment. Free ions drive abiotic and biotic mineral formation, such as pyrite formation and microbially-mediated iron oxidation (Lowenstam, 1981; Melton et al., 2014; Garber et al., 2021). This mineral formation will take place on or near the buried plastic and may even incorporate microplastics (MP) and nanoplastics (NP) (Leiser et al., 2020). Therefore, key questions for plastics exposed to different biogeochemical environments in water and sediment layers are: 1) which minerals and metals are found at the plastic surface, 2) can degradation be detected, and 3) which associated microorganisms are found linked to different metabolic pathways typically dominant at sediment depth, e.g., sulphur, nitrogen, methane metabolism (e.g., Tagg et al., 2019; Seeley et al., 2020; Galgani and Loiselle, 2021; Sanz-Lázaro et al., 2021; Pinnell et al., 2022).
Here, a long-term experiment was designed to expose polyethylene (PE) and Polystyrene (PS) in a marine coastal environment. The development of microbial community, metal, salt and mineral formation was tracked across biogeochemical environment. Plastic sheets were placed in three different biogeochemical compartments: water column, top sediment and bottom sediment over a period of 18 months and harvested over time. The composition of microbial communities associated with plastic, water and sediment samples were analyzed using 16S rRNA gene sequencing. Biofilm structure, elemental composition, and observable changes to the plastic were probed with Scanning Electron Microscopy – Energy Dispersive Spectroscopy (SEM-EDS). Metal extraction from the test surfaces provided insight to metal sorption or incorporation into the biofilm, while oxidation of the plastic was determined using Fourier Transform Infrared Spectroscopy (FTIR). This multidisciplinary approach was selected to characterize biological and mineralogical loading on plastic across different biogeochemical regimes, defined by light and redox conditions and to investigate the potential effects on plastic over time.
Materials and methods
Site description and experimental design
The experiment was carried out in Svanemøllen Harbor, Copenhagen, Denmark. This is the largest leisure marina in Denmark with a capacity for 1,200 crafts and lies adjacent to the city’s northern industrial harbor that includes a container terminal, ferry berths, and industrial marinas (55.715372°N 12.5876606°E, Figure 1A). Maximum water depth in Svanemøllen Harbor is 2.5 to 3m. In the 8-9 years prior to the experiment, dredging was not carried out in the marina (A. Ostenfeldt, harbor master, pers. comm.). Svanemøllen Harbor opens to the Sound (Danish: Øresund), the narrow water body between Denmark and Sweden that connects the North Sea to the Baltic Sea. Øresund water is brackish, with a salinity gradient from north to south that ranges from 18 to 12 ppt that reflects substantial inflow of freshwater from the Baltic Sea. Sedimentation rates for the Øresund are low, varying between 0.2 – 2.5 g m-2 d-1 (Lumborg, 2005). The sediment at the study site is derived primarily from meltwater sand created by several glacial periods and overlying thick Danian limestone (Frederiksen et al., 2003). Tidal changes are semidiurnal with a maximum height change of 15 cm (Medvedev et al., 2016).
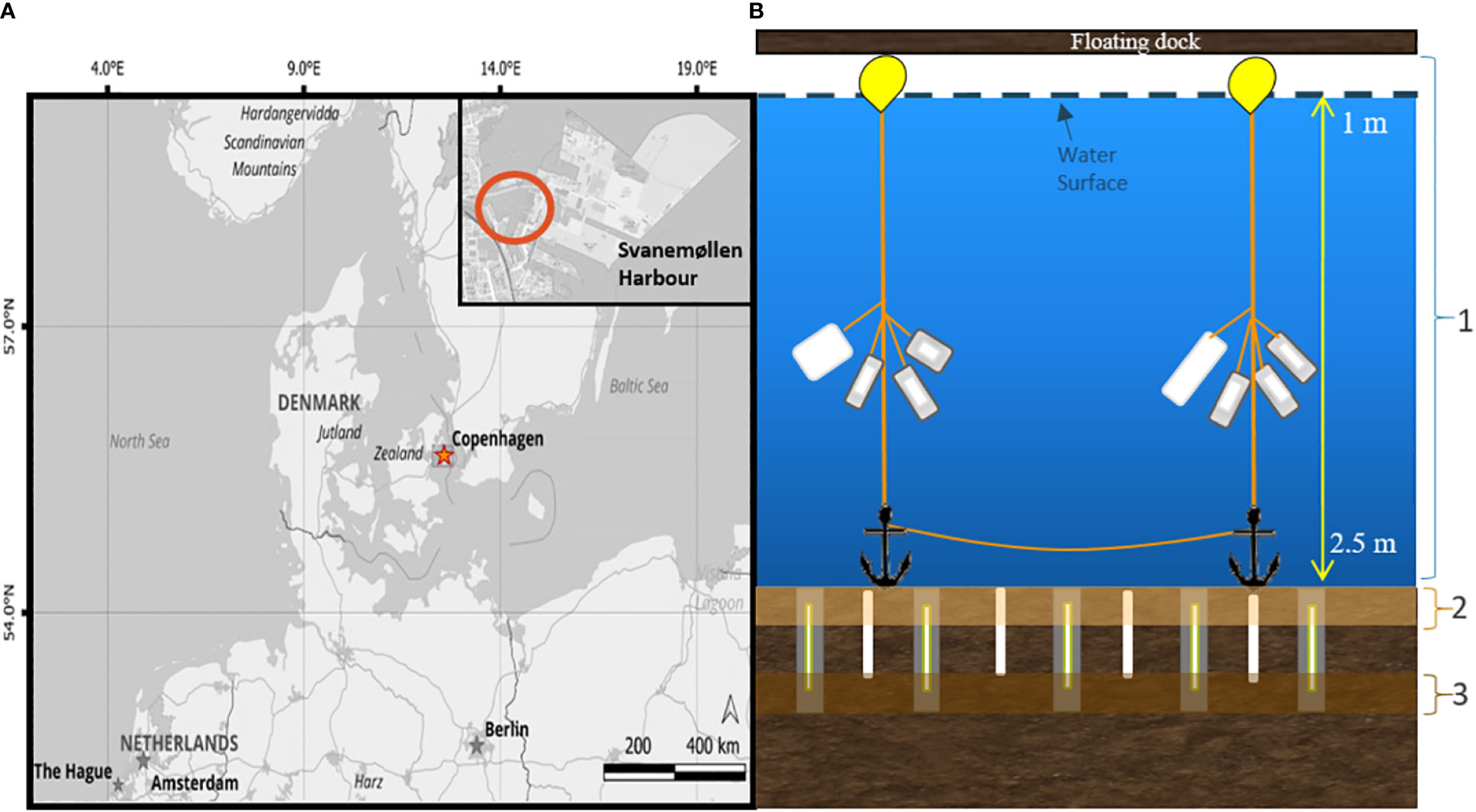
Figure 1 (A) Map of Denmark indicating the location of Svanemøllen Harbor in Copenhagen, along the Øresund Sound. Map data from OpenStreetMap, available at https://www.openstreetmap.org (B) Schematic diagram of the experimental set-up and compartments (marked 1,2,3). Cups with PE, PS, and wood inside were attached to lines with buoys, and anchored at the base. Stakes with PE, PS, and wood sandwiched inside were placed vertically in the sediment (grey), with one set of plastics (either PE or PS) placed in the sediment not in a stake (white). PE and PS were anchored from a floating dock affixed as a flag.
Plastic strips (3.5x22cm) of PE film (Goodfellow, 0.23 mm thickness) and PS film (Goodfellow, 0.05 mm thickness) were placed in the water column and sediment of Svanemøllen Harbor by a diver starting in January 2019 (Figure 1B). PE and PS were chosen due to their abundance in the marine environment (Erni-Cassola et al., 2019) and the differences in their physical properties, particularly stiffness and transparency to light that could distinguish their behavior in the environment. All plastic was exposed to UVA 340 lamps (QUV) for 5 days (flipping halfway through exposure time), equivalent to 11 days under Northern European radiation exposure (Gewert et al., 2018; Romera-Castillo et al., 2018). The UV-pretreatment is a standard procedure to pre-age plastic mimicking weathering in the environment. The PE and PS were set into and exposed to three compartments of the marine environment: 1) water column, 2) top sediment (upper 4 cm, oxic) and 2) bottom sediment (> 4cm, anoxic) (Figure 1B). Untreated wood was deployed as a control material in January 2020 for 6 months. One set of plastics was inserted as strips into the sediment or as flags in the water (PE-no metal and PS-no metal). All samples were placed under or near a floating dock in the middle of the marina for protection from marine traffic over the length of the experiment (Figure 1B). A second set of samples was placed in the water and sediment compartments encased in stainless steel mesh cups and stakes to avoid grazing of microbial biofilm by larger organisms and to provide structure to support vertical profiling in the sediment (Figures 1, SI 1). To this end, PE and PS strips (4 cm x 4 cm) were placed in 316 acid-coated, stainless steel mesh cups with a pore diameter of 200 µm and hung in the water column (PE-cup, PS-cup) (SI 1). For sediment exposure, PE and PS strips (4 cm x 20 cm) were sandwiched in 316 acid-coated stainless steel stakes and inserted into the sediment vertically to capture changing redox processes across sediment depth (PE-stake, PS-stake; SI 1).
Sample collection and processing
The plastic samples were collected by a diver after 6-12 months and kept in a container filled with site water until reaching the laboratory (< 4 km from the marina site). At each sampling time point, water and sediment were collected to provide background biogeochemical data (SI 2) to establish redox transition zones. Water samples were collected and stored in sterile, Duran® glass bottles. Sediment cores were retrieved by a diver, taking care to keep the sediment core vertical, and immediately sealed on the dock with rubber bungs and no headspace to avoid exposure to air.
In the laboratory, the plastics were rinsed with sterile-filtered (0.22 µm) seawater, and aseptically cut into sections using sterile forceps and scissors. Plastic harvested from the water column was cut into 16 (1 cm x 1 cm) pieces and 4 pieces preserved for each analysis type. Plastic harvested from the sediment was cut into 2 cm sections (top and bottom) and further divided into four 1 cm x 1 cm sections. The plastic pieces were then preserved for the various analyses (FTIR, SEM-EDS, metal extractions and 16S rRNA gene analysis as described herein). A detailed overview of sample collection and analyses performed can be found in the Supplementary Information (SI 3).
Analyses with SEM-EDS
For SEM imaging and elemental analyses by EDS, the plastic pieces were fixed in 4% paraformaldehyde for ~18 hours, transferred to 50% ethanol/phosphate buffered saline, and stored at -20°C (Zettler et al., 2013). Thereafter, they were sequentially dehydrated for 10 min in 50%, 70%, 85%, and 95% in ethanol followed by 15 min in 100% ethanol. This was repeated 3 times for a total of 45 min in 100% ethanol and then immediately critically-point dried (Leica-Balzer CPD030, Core Facility for Integrated Microscopy, University of Copenhagen). A subset of plastics were chemically-dried with hexamethyldisilazane for 30 min before a final removal of traces of hexamethyldisilazane by drying under N2 gas stream (Palacios et al., 2019). Following drying, the plastics were coated with 4 to 6 nm platinum and then analyzed by SEM (ZEISS Sigma 300VP Field Emission Scanning Electron Microscope equipped with 2 Bruker Xflash 6|30 129 eV EDS detectors, at the Geological Survey of Denmark and Greenland (GEUS)).
Chemical profiling of plastics
After harvesting, plastic pieces were rinsed with SILEX filtered water, air-dried, and kept at room temperature (20°C) until analysis. A Bruker Alpha P FTIR spectrometer with a single bounce diamond attenuated total reflection (ATR) accessory was used to obtain a chemical profile of the surfaces of all plastic pieces using 25 scans and a resolution of 4 cm-1. All FTIR analyses were conducted at the Environmental Archaeology and Materials Science section of the National Museum of Denmark.
Metal analysis
A diluted nitric acid was used to extract metals on the plastic surface and avoid extraction of metals contained within the polymer matrix. PE, PS and wood were soaked for 2 hours in 2 mL 10% (v/v) HNO3 (high purity acid for trace element analysis) at ambient temperature to extract any attached bio-available metals, following an established protocol (Munier and Bendell, 2018). To minimise contamination, Eppendorf plastic tubes were pre-cleaned with 6M HCl at 80° C for 24 hours before running the metal extraction. Once the extraction was complete, the acid was preserved in pre-acid cleaned Eppendorf tubes and stored at 4°C prior to analysis. The acid extract was then analyzed for aluminium (Al), chromium (Cr), copper (Cu), iron (Fe), manganese (Mn), and zinc (Zn) via an Inductively Coupled Plasma – Optical Emission Spectrometry (ICP-OES (Agilent 720 ICP-OES)) at the NORCE Trace Element Lab (Bergen, Norway). To test if the acid extraction only extracted metals from the surface of the plastics, certified reference materials (CRM) for PE and styrene were analyzed, which had been treated the same as the plastic samples. Currently, there are no available certified reference materials for adsorbed trace metal on the surface of plastics. The acid extractions for CRM have been analyzed using the ICP-OES. Twelve in-house standards and a blank matching the same matrix as the acid extraction to reduce matrix effect were prepared at the NORCE Trace element lab with concentrations between 1 to 5000 µg/g. Samples were diluted with 10% high purity HNO3 if the initial run was above this range. To ensure quality control, a quality check of 60 ppb was analyzed every four samples to correct for instrumental biases and analytical drift, with a precision of the analysis lying between 0.8 to 4.5%. The blank used for the calibration was below the detection limits. All reported metal concentrations were normalized to the mass of the plastic piece. Pristine PE, PS, wood and pre-aged PE and PS were also analyzed for their background metal concentrations.
For bulk sediment analysis, sediment samples were analyzed using x-ray fluorescence (XRF) (Olympus DP6000 XRF, UCPH-IGN). Sediment samples were filled into 20 ml polypropylene scintillation vials (Sarstedt) with more than 1 cm thickness for measurement. A 4.0 µm Prolene® XRF film was placed over the vial containing the sample and inverted. The sample was then tapped to form an even layer without cracks and placed on the measuring window, after which analysis was carried out using XRF.
Sampling of DNA – plastic, wood, water, and sediment
All plastics were cut into 1 x 1 cm rectangles and wood controls were cut into 2 x 0.5 cm pieces using sterile methods, and immediately stored in 1.5 ml Eppendorf tubes at -80°C until DNA extraction. Three 500 ml water samples from the harbor were filtered onto 0.22 µm polycarbonate filters (Whatman® Nucleopore™ Track-Etched Membranes) and frozen at -80°C per sample time point. Sediment cores were cut into 2 cm slices and the sediment transferred to sterile falcon tubes where they were also frozen at -80°C until DNA extraction.
DNA extraction and 16S rRNA gene sequencing
The plastic pieces, polycarbonate filters (water samples), and wood controls were cryogenically ground in 2 ml Eppendorf tubes in liquid nitrogen. Sediment samples were prepared by the addition of 1 g wet weight into 2 mL Eppendorf tubes. DNA was extracted using the DNeasy PowerLyzer PowerSoil Kit, (Qiagen) and quantified with a Quant-iT™ PicoGreen® dsDNA Assay (Invitrogen). The triplicates from water and sediment were pooled and some triplicates were selected to check for variability between them. Plastic pieces and wood controls were processed as single replicates.
The hypervariable regions V4 and V5 were amplified using a fusion primer pair, 515F-Y and 926R, which includes Illumina adaptors (SI 4), covering bacteria and archaea (Parada et al., 2016). Polymerase chain reactions (PCR) were run in triplicate 25 µl reactions using a MyTaq Red Polymerase kit (Nordic BioSite) and 5 ng of DNA templates: 3 min at 94°C followed by 30 cycles of 94°C for 45 s, 50°C for 60 s, 72°C for 90 s, and a final extension of 72°C for 10 min. Negative controls were run in triplicate and included in the downstream protocol. Triplicate reactions were pooled and purified using the GENECLEAN® Turbo kit (MPBiomedicals). For barcoding, 8 fusion specific custom nucleotide indexes were used with a flow cell adaptor sequence (SI 4) in a PCR reaction using a MyTaq Red Polymerase kit containing 3.5 mM MgCl2 and 5 ng of the PCR purified products. The PCR was set at 94°C for 1 min followed by 8 cycles of 94°C for 30 s, 62°C for 30 s, and 72°C for 30 s, and final elongation at 72°C for 5 min. The indexed samples were purified (Agencourt AMPure beads; Beckman Coulter Genomics), quantified, pooled at equimolar concentrations, and paired-end sequenced at the GeoGenetics Sequencing Core using an Illumina MiSeq instrument (UCPH-GLOBE).
Demultiplexed raw fastq files were processed with the Divisive Amplicon Denoising Algorithm 2 package (DADA2, version 1.21.0), (Callahan et al., 2016) using RStudio version 1.4.1717. Read quality profiles were inspected, and primer-free sequences were filtered and trimmed to 250 or 200 bp depending on the forward and reverse sequences, respectively (maxN=0, maxEE=c(2,2), truncQ=2, rm.phix=TRUE), then denoised and merged. Next, chimera sequences were removed. The final amplicon sequence variants (ASVs) were taxonomically assigned using the Silva reference Database version 132 (Quast et al., 2013). Decontamination was carried out by removing ASVs present in negative controls. To do this, ASVs found in negative controls were identified and further removed from all other samples. In total, 38817 ASVs were identified and 38778 ASVs were left after decontamination. Samples were normalized and pruned to remove singletons. Finally, ASV diversity and statistical analyses were performed using the R packages Phyloseq (McMurdie and Holmes, 2013) and FANTAXTIC (Teunisse, 2018). Alpha and beta diversity were investigated and community composition on plastic compared to the surrounding water and sediment. For the 6-month and the 12-month samples, beta diversity was analyzed by non-metric dimensional scaling (NMDS).
Results
SEM imaging of water column and sediment exposed plastics
After 6-month exposure, surfaces of PE and PS exposed in the water column and top sediment were covered with biofilm and minerals (Figures 2A–F). This coverage decreased in general with depth in the sediment for PS (Figures 2A–C) and PE (Figures 2D–F). In the water column, a wide range of cell morphologies were visible, including coccoid, filamentous and bacillus bacteria alongside diatoms (Figures 2, 3, SI 5). Diatoms of various morphologies were found on all plastics in the water column. Plastics in the top sediment, however, were encrusted with more fragmented and broken diatoms, and coccoid cells appeared more abundant on the PS samples than on the PE (Figures 2B, E). Slight differences were visible between the PE and PS samples in the top sediment. Plastics embedded in the bottom sediment appeared sparsely colonized. EDS coupled to SEM images also revealed the presence of metal oxide minerals (chromium, iron, aluminium) at surfaces of PE and PS with crystalline and amorphous morphologies (Figures 4A, B), which were further corroborated through qualitative matching of the chemistry to mineral habit.
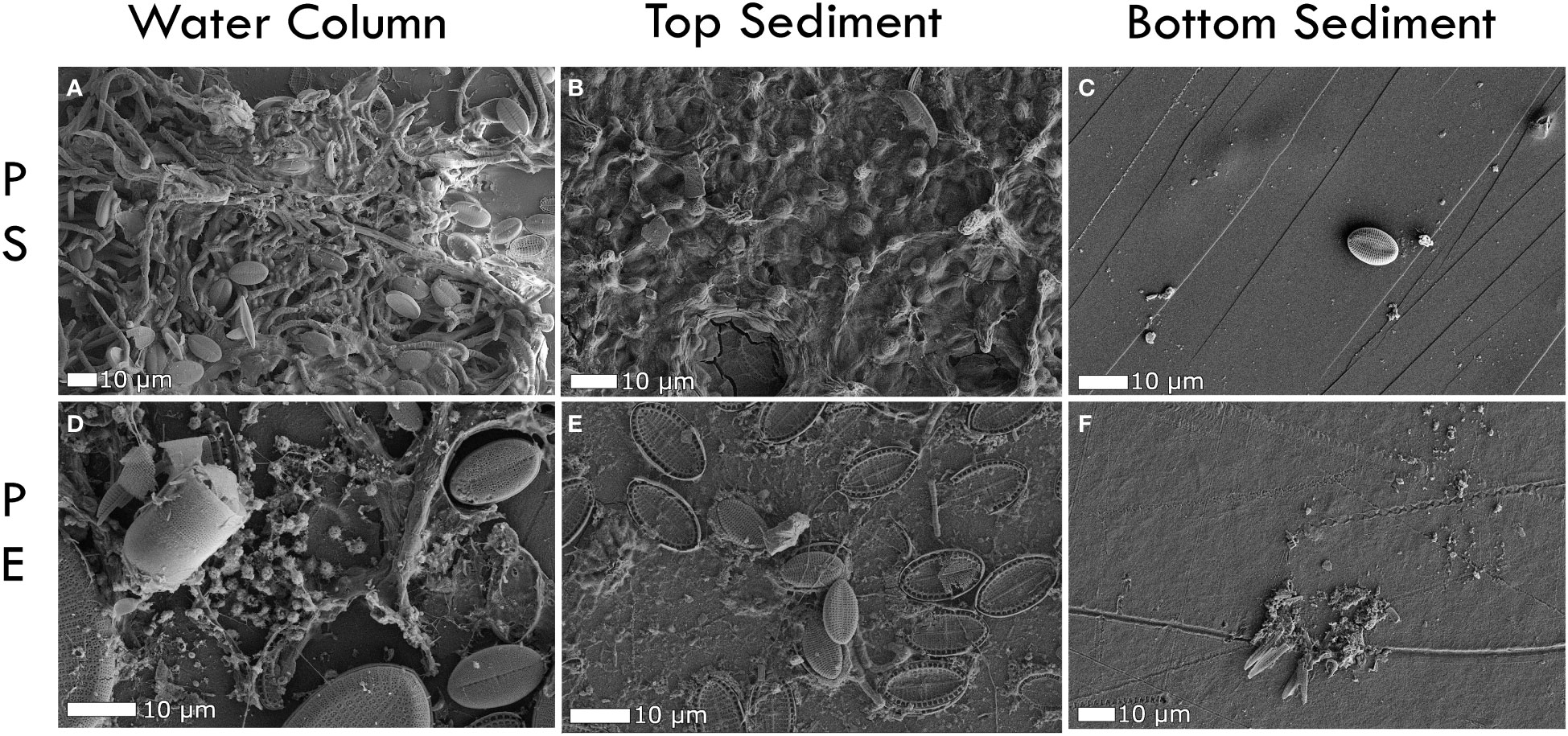
Figure 2 SEM-SE images of PE and PS plastics exposed to the water column and sediment for 6 months without metal encasement. (A) PS in the surface water showing mixed colonization of microorganisms (B) PS in the top sediment, largely dominated by coccoid shaped cells, (C) PS in the deep sediment showing a diatom, but minimal surface coverage apart from some small grains, (D) A mixed community on the surface of a PE sheet exposed to the water column, (E) Fragmented diatoms on the surface of a PE sheet in the top sediment, (F) Some diatom fragments and grains on the PE surface.
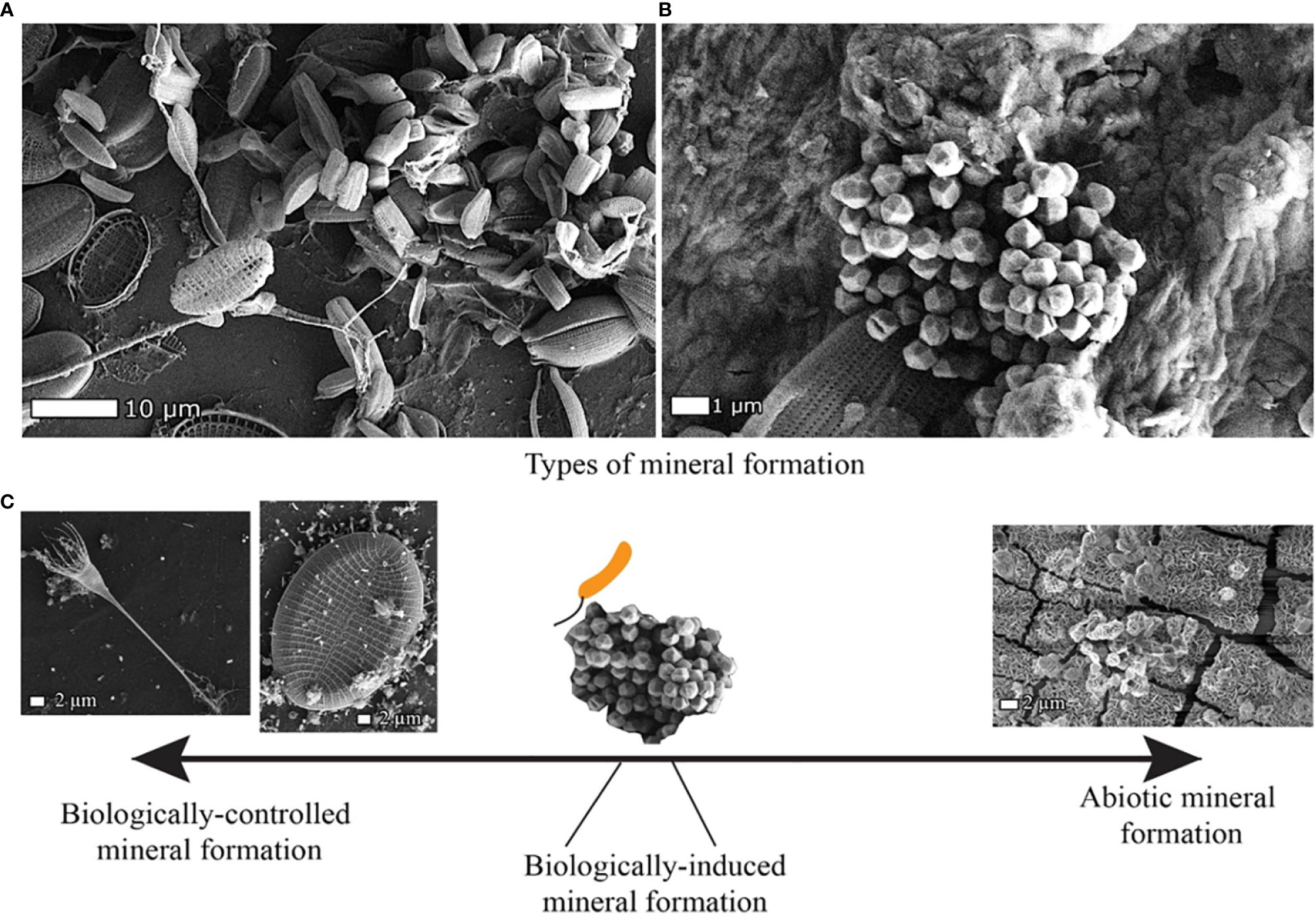
Figure 3 Plastics exposed to water column and sediment of Svanemøllen Harbour, Denmark, show the close association of microorganisms as well as biogenic and abiogenic minerals in SEM-SE micrographs. (A) PS exposed to water column for 3 months colonized by diatoms and (B) PS exposed to top sediment (0-2 cm depth) for 6 months shows incorporation of pyrite framboids into biofilm also consisting of diatoms, bacillus and coccoid encrusted in granular film. (C) Minerals can form through different processes that can be conceptualized along a spectrum from biologically-controlled to fully abiotic processes (Konhauser et al., 2007). Examples of these different types of mineral formation are seen within the microbe-mineral biofilm matrix on plastics.
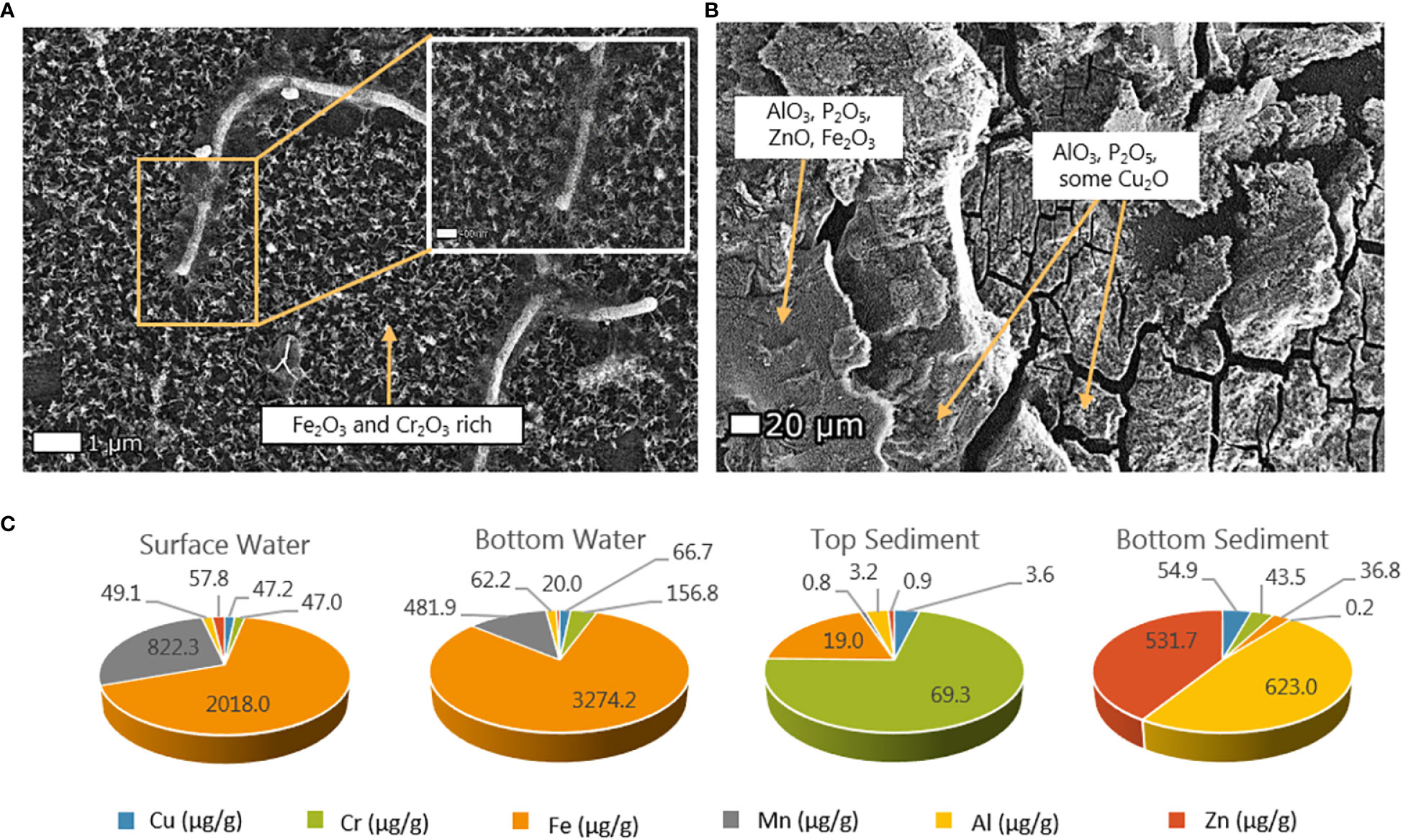
Figure 4 Metal sorption onto polyethylene (PE) exposed in Svanemøllen Harbor to (A) the water column (1 m) and (B) the sediment (12-14 cm depth). Mineral morphology suggests abiotic mineral formation of iron, chromium, alumina, zinc and copper oxides as well as phosphate determined via SEM-EDS. (C) Relative metal concentrations [µg/g] on PS exposed to surface water, top sediment and bottom sediment after 12 months exposure.
Metals analysis
Overall, the largest concentrations of metals were found in the bulk sediment (Table 1). Wood controls made up the second largest metals pool. Generally, higher metal concentrations were found adsorbed to wood than on plastics, but PS had mostly higher concentrations of adsorbed metals than PE after exposure in the harbor (Table 1). Metal-sorption concentrations on plastic varied across the water, top sediment and bottom sediment compartments. Across changing light, redox conditions (water, top and bottom sediment), and time (12-months), Fe and Mn were found in highest concentrations on plastics in the water column with 2018.0 µg/g and 822.3 µg/g respectively (Figure 4C).
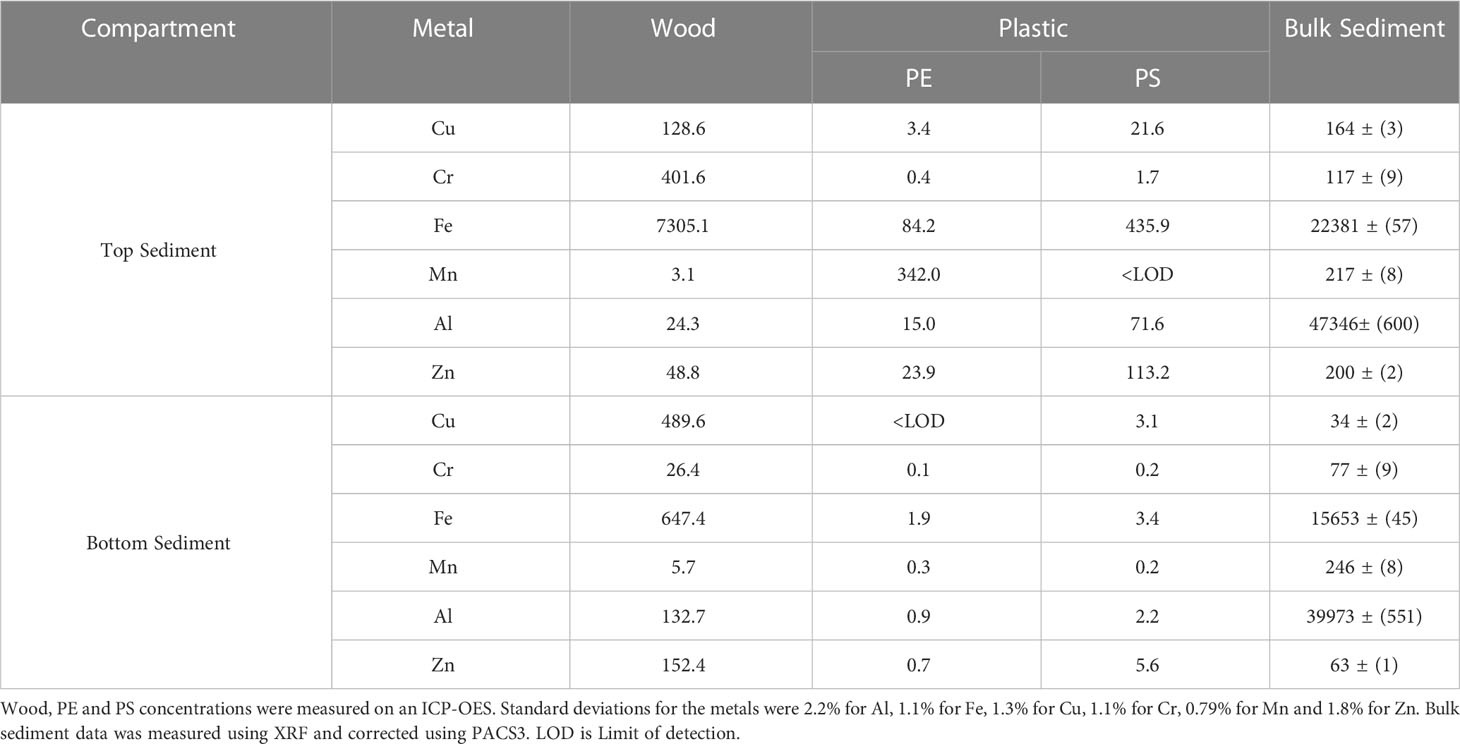
Table 1 Metal concentrations in µg/g extracted from wood controls and plastic (PE and PS) exposed to the top and bottom harbor sediment for 6 months, compared to the bulk sediment.
After 6-month exposure to top sediment, Mn was the most abundant metal adsorbed to PE plastic (342µg/g) and found in higher concentrations than on wood (3.1 µg/g) and bulk sediment (217 µg/g) at that depth. In contrast, Fe was the most abundant metal on PS plastics (435.9 µg/g) but was found in much higher concentrations in bulk sediment (22381 µg/g) and on the wood controls (7305 µg/g) at that depth (Table 1). Zn was also found in high concentrations on PS buried in the top sediment (113.2 µg/g), which was roughly half of the overall bulk sediment zinc concentration (200 µg/g). In the bottom sediment, lower metal concentrations were found adsorbed to PE than PS, and metals were found in lower concentration on the plastics than on the wood samples in general. Of the metals on PE, Fe was found in highest concentration (1.9 µg/g) at these sediment depths and on PS, Zn was found in highest concentration (5.6 µg/g) (Table 1). After 12-month exposure, PS plastics in the top sediment harboured very low concentrations values of all metals, however Cr (69.3µg/g) and Fe (19.0 µg/g) were highest in concentration of the six metals in the top sediment. Whilst on the plastic in the bottom sediment, Al concentrations reached 623.1 µg/g and Zn was adsorbed at concentrations of 531.7 µg/g followed by the other metals (Figure 4C).
Chemical profiling of plastics
Oxidation of PE and PS samples was detected after 12 months in the harbor depending on redox compartment of exposure (Figures 5, SI 6). PS exposed to surface water and top sediment showed more oxidation in absorbance bands between 1300 and 500 cm-1 (Figures 5B, D) than PS buried in the top and bottom sediment (Figures 5C, D). Changes in absorbance were detected in PS exposed to surface waters in comparison to unexposed (pristine) PS (Figure 5A). However, the greatest changes were detected in PS buried in the top sediment layer for 12 months (Figure 5C). PE was oxidized most in the top sediment whereas PS was most oxidized in surface waters where UV levels were highest (SI 6).
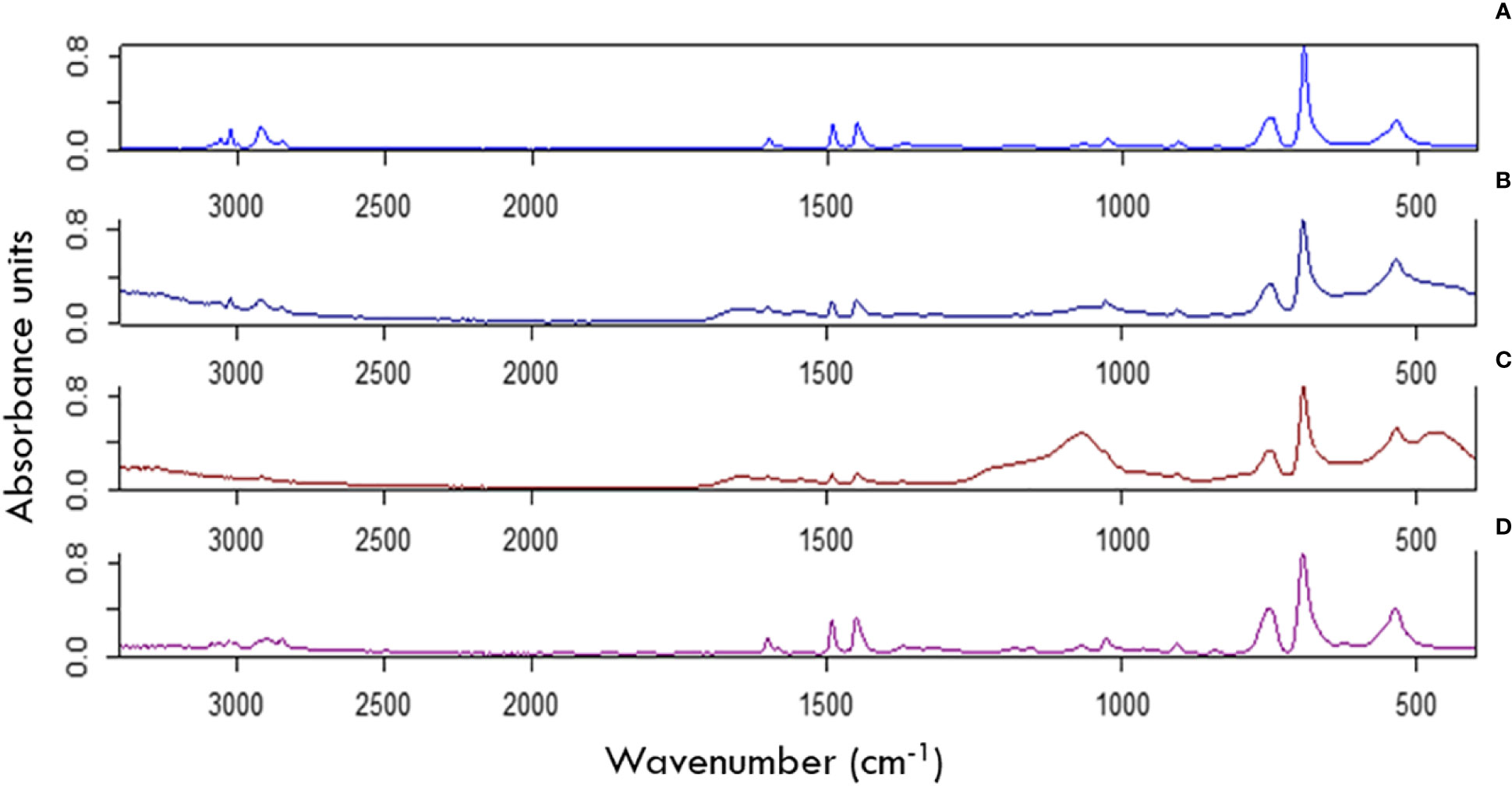
Figure 5 FTIR spectra of pristine (not exposed) PS and PS exposed for 12 months in metal cups and stakes. (A) Not exposed PS sheet showing no oxidation. (B) PS exposed to the surface water compartment for one year, shows some oxidation in the ranges of 1600-500cm-1. (C) PS exposed to the top sediment compartment for one year shows extensive oxidation on the FTIR spectra in the ranges of 1600-500cm-1. (D) PS exposed to the bottom sediment for one year shows no oxidation in the ranges of 1600-500cm-1 but limited changes in absorbance in the ranges between 2700 and 3100 cm-1.
Microbial community composition analyses
NMDS plots for all ASVs were used to depict differences between the epiplastic community composition and the community composition of the surrounding water and sediment after 6-and 12-month exposure. These show that the composition of the epiplastic communities differed from bulk water and sediment communities (Figure 6). Importantly, the stress values for both the 6-month and 12-month NMDS plots were close to and under 0.05, indicating result trustworthiness.
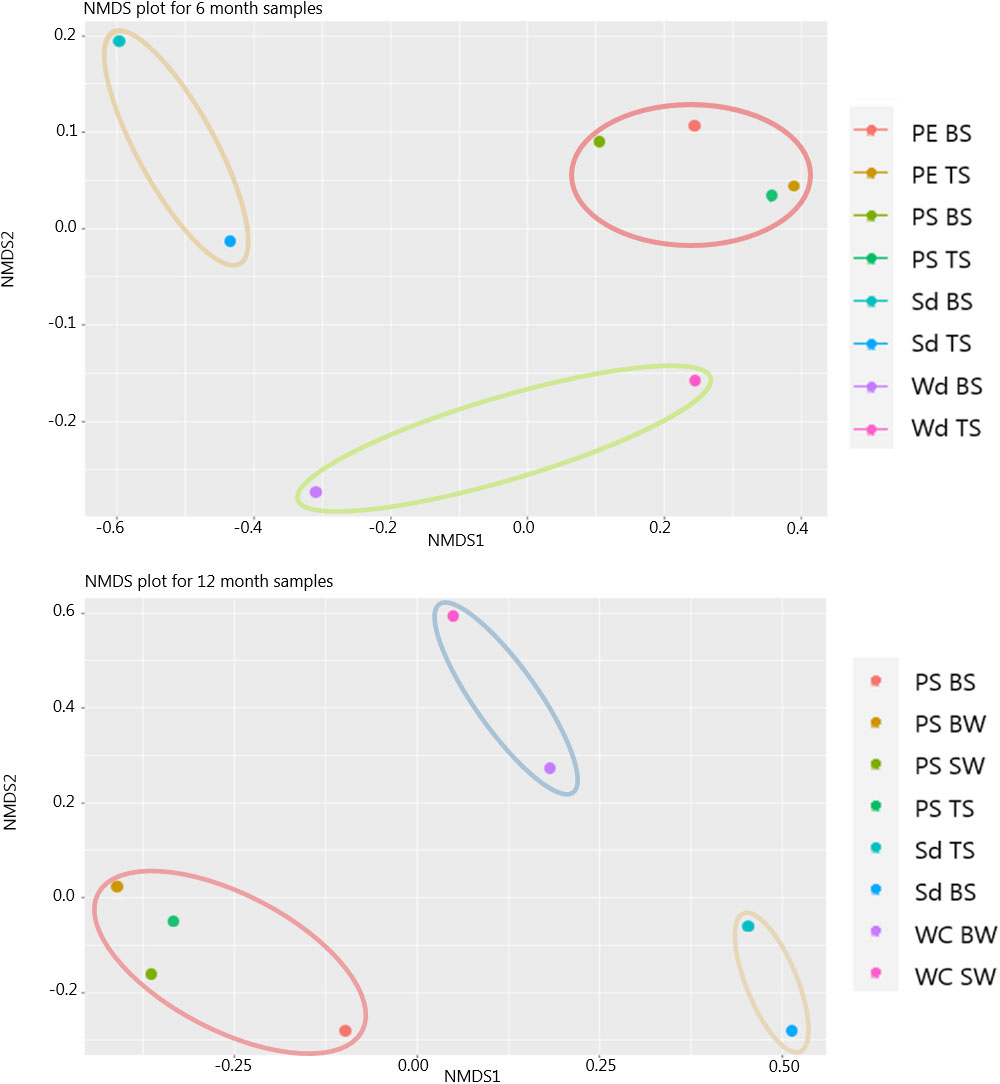
Figure 6 Non-metric dimensionally scaled (NMDS) plot using the Bray-Curtis dissimilarity distance matrix for 6 month and 12 month samples analyzed for 16s rRNA, comparing microbial populations by their abundance and similarity to one another. Acronyms: Surface water (SW), bottom water (BW), oxic top sediment (TS), anoxic bottom sediment (BS), wood (Wd), water column (WC), sediment (Sd) and PE and PS. The 6 month NMDS plot had a stress value of 0.01 in 2 dimensions, and the 12 month NMDS plot had a stress value of 0.06 in 2 dimensions indicating that both plots are a good fit. The ellipses indicate how the samples group together.
Taxonomy and relative abundance of the top 20 ASVs, representing 80% of all obtained reads, associated with plastics, surrounding water, and sediment exposure are shown in Figure 7 (12 months) and Figure 8 (6 months).
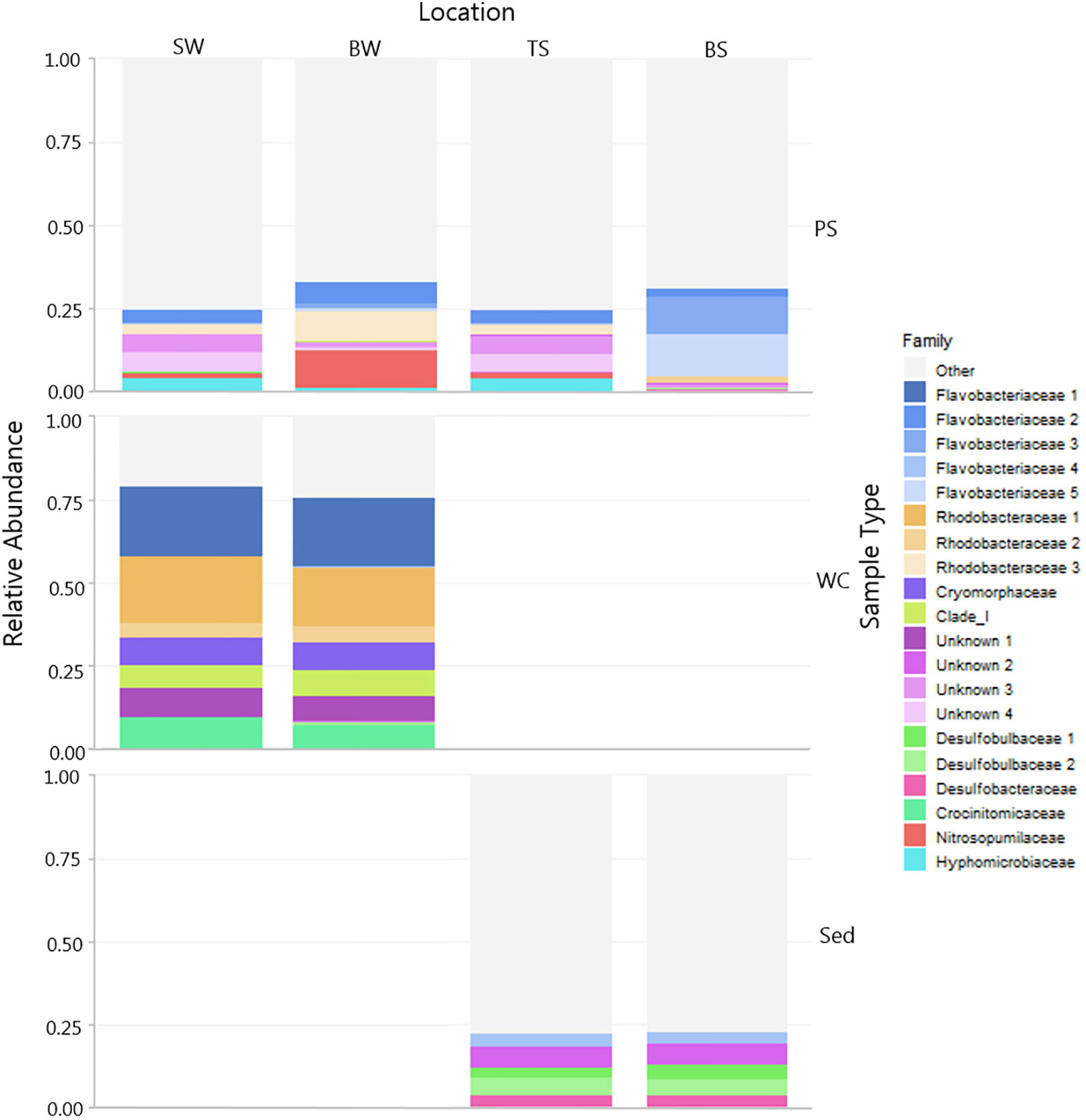
Figure 7 Microbial community associated with PS across different compartments (sediment and water) and background samples (water and sediment). The relative abundance of the top 20 ASVs are shown colored by Family. Note samples are divided by sample type and location. Grey shaded areas are other taxa outside the top 20 abundant ASVs. (PS, polystyrene; WC, water column; Sed, sediment; SW, surface water; BW, bottom water; TS, top sediment'; BS, bottom sediment).
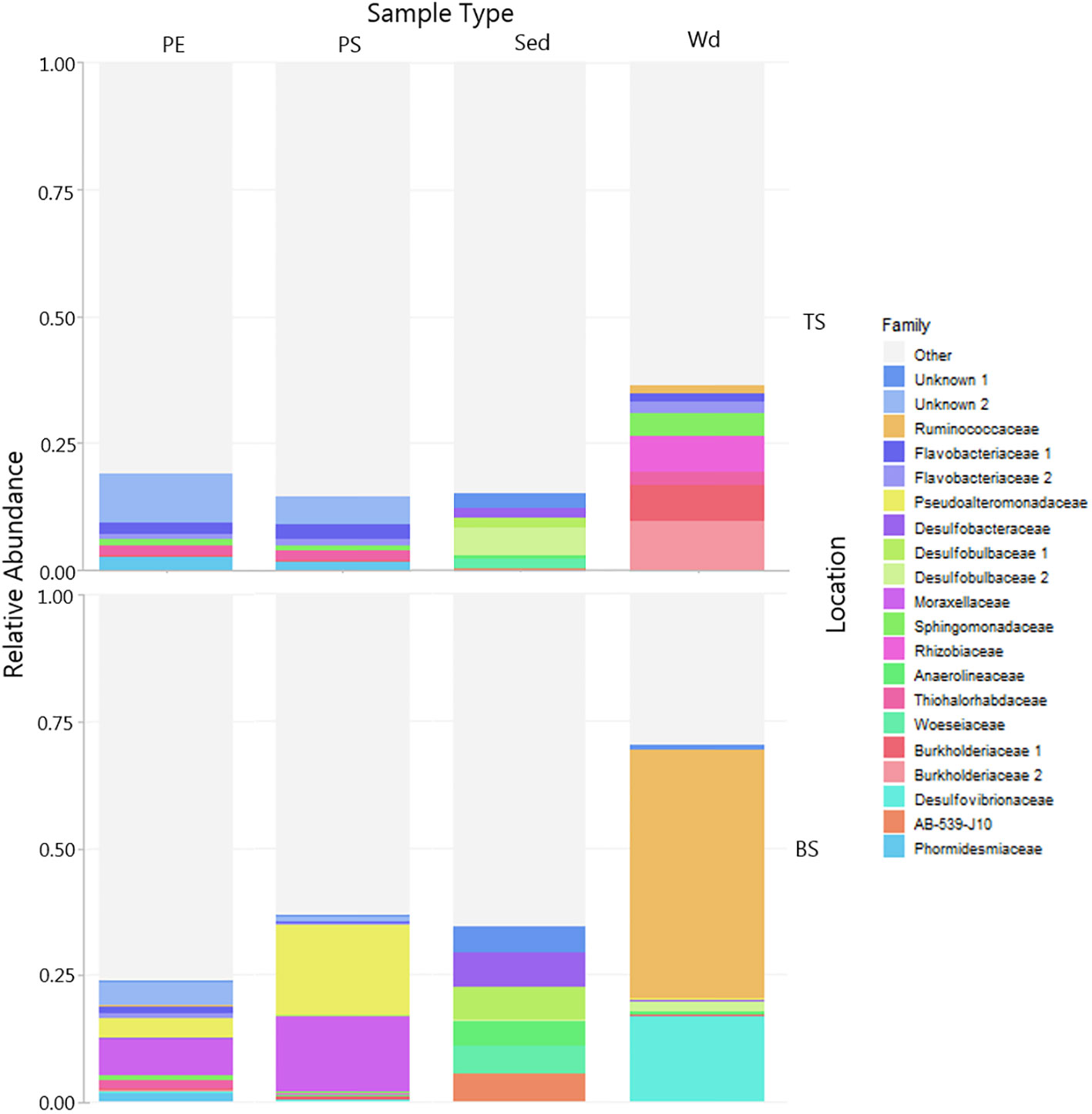
Figure 8 Microbial community associated with PE and PS and wood controls in sediment compared to microbial community in the surrounding bulk sediment (Sd – TS, BS). The relative abundance of the top 20 ASVs colored by family. Plastics and wood controls were exposed for 6 months in top sediment (TS, 0-2cm, oxic) and bottom sediment (BS, >4cm anoxic). Note samples are divided by sample type (PE, PS, Sed, Wd) and location (BS, TS). Grey shaded areas are other taxa outside the top 20 abundant ASVs.
After 6 months, the microbial composition in the sediment was clearly distinct from those on the plastics and the wood control (Figure 8). Furthermore, communities on plastics, wood, and sediment differed from sediment across redox compartments from top to bottom. PE and PS buried in the sediment was colonized by a distinct top sediment community consisting mostly of Proteobacteria and some Cyanobacteria not found in the other samples. Chloroflexi was found in the bulk sediment, but not seen on the plastic or wood at the same location and redox conditions. Wood buried in the bottom sediment is colonized largely by the family Ruminococcaceae. PE and PS diversity differed between the top and bottom sediment compared to the bulk sediment (Figures 7, 8) and in comparison, the bulk water showed a more diverse community compared to the plastic samples.
The PS-associated community in the upper sediment was similar to the bacterial community of the water column. In contrast, there was a change in the community between the epiplastic community extracted from PS exposed to the water and PS exposed to the bottom sediment (Figure 7, 12 months). Analyses were carried out at all levels of phylogeny, and describe a range of families present on PS exposed to water and top sediment. A wide range of families were found on PS exposed to water and top sediment, such as Flavobacteriaceae, Hyphomicrobiaceae, and Rhodobactericaceae, predominantly belonging to the phyla Proteobacteria, Bacteriodetes, and Thaumarchaeota (Figure 7).
Of the top 20 relatively abundant ASVs, PS exposed to the bottom sediment had the largest relative abundance of the Flavobacter genus Maribacter. The Nitrosupumilaceae family was also only found on the PS and largely in plastic exposed to the bottom water (water-sediment interface). Alongside this, the communities found on PS both in the water and the sediment, differed from the background water and sediment communities.
Discussion
This study focuses on the chemical, biological and mineral compositions at the surfaces of polyethylene and polystyrene plastics across changing biogeochemical settings. These comprise the oxic conditions of the water and top sediment and the anoxic bottom sediments. Over the course of a 12-month in situ study plastics exposed to water and sediment were quickly loaded with diverse microorganisms and minerals. These microbial communities and mineral formations were distinct from the surrounding environment and differed across redox regimes.
Microbial community association with coastal plastic debris
The microbial community on plastics floating in the water column differed from those composing the surrounding water (Figure 6) which indicates a specific “Plastisphere” community, as seen in previous studies (Zettler et al., 2013; McCormick et al., 2014; Bryant et al., 2016; Debroas et al., 2017; Jiang et al., 2018; Tagg et al., 2019). In the water samples, community composition was dominated by Bacteriodetes and Proteobacteria, like Flavobactericaceae and Rhodobactericaceae, some of the most common marine bacteria families (Pohlner et al., 2019). The plastic samples from the water column contained various genera of these families, also commonly associated with plastic biofilm communities in other marine environment studies (Zettler et al., 2013; De Tender et al., 2017; Oberbeckmann et al., 2018; Kirstein et al., 2019). Based on the top 20 relatively abundant ASVs, microbial communities sampled from the water column were also more diverse than epiplastic communities (Figure 7), further indicating enrichment of certain organisms on plastic surfaces. The plastic properties themselves, the associated conditions of the plastic eco-corona (Bhagwat et al., 2021), or a preference for an attached rather than a free-living lifestyle among epiplastic organisms may have driven this community selection. It may also be driven by a combination of all of these factors, where the biofilm on the plastic provides an ideal niche for the plastic-specific community.
Plastic sampled from the water column contains the archaeal family Nitrosopumilaceae (Figure 7). These belong to a phylum containing ammonia-oxidizing archaea, which are important ocean nitrifiers (Könneke et al., 2005; Wuchter et al., 2006). The occurrence of Nitrosopumilaceae on plastic indicates a potential increased ammonia availability and a localized nitrogen cycle that may be transported across distance and depth in the water column (Gouin, 2021; Vaksmaa et al., 2021). The increased relative abundance of these archaea in the bottom water column samples (Figure 7) also suggests a larger role for these organisms in the epiplastic biofilm community near the sediment-water interface (Vaksmaa et al., 2021). Plastic particles may host and transport opportunistic and true pathogens, an area of considerable research in the assessment of risk associated with this pollutant. The family Flavobactericaceae is known to host some pathogens (Bernardet and Nakagawa, 2006; Bowley et al., 2021), but whilst these pathogenic bacteria may be attached to plastic particles, the question of whether or not they are viable, whether they can transfer up through the food chain, and whether they can be linked to incidence of disease remains a current point of research.
Enrichment of distinct epiplastic communities holds across the redox regimes from the oxic water column to the anoxic conditions of the sediment (Figures 6–8). Whilst the bulk sediment harbored diverse communities, more specific communities colonized plastics and wood, especially in deeper, anoxic sediment. Distinct community differences were seen between the bulk sediment, and surfaces of the plastic (PE, PS) and wood controls already after 6-month exposure in the sediment (Figure 8). The epiplastic community composition is also dependent on burial depth in the sediment. Plastics buried in oxic, top sediment harbored diatoms (also broken and fragmented), as well as filamentous and coccoid cells within the biofilm (Figures 2, 3, SI 7). In 12-month samples, similarities in microbial community composition were seen between plastic exposed to the water column and plastic buried in the top sediment (Figure 8). Alpha diversity analysis, however, indicates these two communities differed (SI 8). Community composition on PS exposed for 12 months in oxic top sediment is mixed and not dominated by any specific family whilst in the anoxic sediment, the epiplastic community is dominated by the Flavobactericaceae, as was also found colonizing plastics in the water. Indeed, Flavobactericaceae is known for its metabolic flexibility, which would explain its presence on plastics across biogeochemical regimes. The family Flavobactericaceae includes a range of lifestyles from obligate to facultative aerobes, microaerophiles and anaerobes (Bernardet and Nakagawa, 2006). This family is also one of the most documented in many marine plastic-associated bacteria studies (Roager and Sonnenschein, 2019). Members of this family are known to produce enzymes that can degrade nylon oligomers (Negoro, 2000) are some are capable of digesting by-products of nylon degradation (Kinoshita et al., 1975) as well as different types of hydrocarbons such as diesel (Chaudhary et al., 2019) and polyester polycaprolactone (Roager and Sonnenschein, 2019). If these bacteria are able to utilise the leachates of plastic polymers (PE, PS), it could further explain their association to the plastic samples. Some Flavobacteria are also known to colonise diatom detritus (Abell and Bowman, 2005) perhaps selectively attaching to the silica dioxide diatom surface. This metabolic flexibility across redox zones, their preference for specific nutrient sources, and their known colonization of diatom detritus may also explain their dominance in anoxic sediment where diatoms detritus is seen on the buried plastic. Wood controls in the deeper sediment (>4cm) are colonized by Ruminococcaceae not found on plastics, which are highly efficient organic compound degraders that may be attacking the cellulose here (Hahnke et al., 2018; La Reau and Suen, 2018; Nishiwaki-Akine et al., 2020; Ahrens et al., 2021). Plastic buried in this deeper (bottom) sediment instead have higher relative abundances of Pseudoalteromonodaceae and Moraxellaceae families not found on the other samples (plastic in the top sediments, wood controls and background sediment). The PE and PS also show a difference in relative abundance of these two families, with Pseudoalteromonas being more relatively abundant on the PS than the PE, which aligns with observations made in other studies using bacterial strains isolated from Danish waters (Hansen et al., 2021). The Pseudoalteromonas genus has been shown to inhibit the growth of other bacteria and to have antifouling properties (Bernbom et al., 2011), which may explain relative abundance of Pseudoalteromonas and Moraxellaceae and lower presence of other families (Figure 7) on the PS buried at depth in the bottom sediment here. The Moraxellaceae family, represented by the Acinetobacter genus, is also in higher abundance than other groups on plastics buried in anoxic sediment at this site, and with a similar preference for PS. Members of this genus are known to utilise leachates released during plastic degradation (Sheridan et al., 2022), and may explain their occurrence as a preference for the plastic and its leachates (Yin et al., 2020; Akther et al., 2022; Sheridan et al., 2022). These differences in microbial community composition on plastic buried at various depths suggests that environmental conditions and dominating redox processes are controlling factors on plastic colonization, and organisms with metabolic flexibility are found throughout changing redox conditions of the Plastisphere. Here, the top 20 most relatively abundant ASVs are in focus. While identifying some commonly known plastic associated families, it must be noted that this approach may miss other families detected on plastics in other studies. The development of a specific “Plastisphere” community both in coastal harbor water column and sediment is found to align with community profiling on plastics in other marine settings (Zettler et al., 2013; McCormick et al., 2014; Bryant et al., 2016; Debroas et al., 2017; Jiang et al., 2018; Tagg et al., 2019). The wide range of families on the PE and PS plastic here contains a variety of species able to utilise many different metabolic pathways, such as phototrophy, chemoheterotrophy, chemolithotrophy, as well as mixotrophy (Oren and Xu, 2014; Pujalte et al., 2014). Thus, the plastic biofilm houses bacteria consortia that can carry out a number of redox processes making it flexible and dynamic, able to respond to changes over varied redox conditions. However, this study also indicates a community shift dependent on surface, plastic type and biogeochemical environment in which the plastic resides. This has implications for the overall biotic and abiotic composition of the Plastisphere biofilm.
Mineral and metal association with plastic
Biominerals and metal oxides are precipitated, adsorbed, and captured on the plastic surface and within the biofilm matrix associated with both PE and PS after short exposure to the water column (Table 1; Figures 2–4). The precipitation of these minerals, whether formed via biological processes or formed abiotically through chemo-physical reactions (Figure 2), is the result of an intricate interplay of the plastic with the surrounding environment. Mineral association with plastic is rapid and was apparent on the plastic and within the epiplastic biofilm within the first 3 months (SI 5). Even in winter conditions prior to the spring bloom in the Øresund, plastic surfaces become covered with a mixture of biogenic and abiogenic minerals (Figures 2, 3).
Perhaps the most obvious example of biogenic minerals are diatoms, which form a silica dioxide cell wall via biologically controlled mineral formation (Figure 3C). Metal oxides, such as iron oxides (Fe2O3) and chromium oxides (Cr2O3) were also found on plastics floating in the water column (Figure 4). These metal oxides have also been observed in plastic pellets found along the English coastline (Ashton et al., 2010), yet the mechanisms and impact of this attachment are still not fully understood. PE along with polycarbonate is known to sorb iron from marine waters (Fischer et al., 2007). A comparison between biogeochemical regimes in this coastal harbor show that Fe and Mn are sorbed to plastics in significantly higher concentrations in the water column than in the top and bottom sediment. This may reflect the thicker biofilm development on plastics in the water column in comparison to those in the sediment, and selective sorption of Fe and Mn reported for biofilms (Kielemoes et al., 2002; Lee et al., 2014) (Figure 4).
As was found in water, mineral coatings on plastic in the top sediment incorporated biogenic minerals in the form of broken and intact benthic diatoms (Figures 2, 4, SI 7). Metal oxides, such as Fe2O3 and Cr2O3 found on plastics in the water column were also found on plastics in the sediment, albeit in lower concentrations (Figure 4; Table 1). In general, plastics buried deeper in the sediment were less encrusted with minerals and attachment of biofilm was less evident by SEM (Figure 2). In bottom sediment however, wood adsorbed the highest concentrations of Cu and Fe, along with Zn and Al, perhaps unsurprising given the high porosity of this natural material control (Table 1). The bulk sediment contained the highest concentrations of metals, compared to the wood and plastic, likely due to the sediment surface area and porosity (Dobaradaran et al., 2018; Maršić-Lučić et al., 2018). The presence of oxides of Cu, Cr, Al, and Zn, as well as Fe in sediment up to 12-14 cm depth (Figure 4; Table 1) may have its origins in the coastal marina setting and may explain the strong association of these metals on the plastic at the different depths. Boat paint is known to be transported to harbor sediment and is high in zinc and copper for their antifouling properties, but is also made of plastic polymers particles (Song et al., 2014; Turner, 2021; Jian et al., 2022). A greater accumulation of metals are found in antifouling boat paints is seen in sediments near boatyards and boat repair facilities compared to sediments further away from these areas (Lagerström et al., 2016; Soroldoni et al., 2018). Whilst Al may be released from sacrificial anodes used to protect steel structures in harbors (Leleyter et al., 2018). Cr in urban water areas can be sourced from tyres and brake linings or combined sewer overflows (Byrne et al., 2017).
Metals, such as Fe and Mn are important in the functioning of many biological enzymes (Riordan and Vallee, 1974). Mn is required to build reactive centres in manganese peroxidase enzymes. These enzymes are produced by bacteria and fungi commonly cited in plastic studies (Twala et al., 2020), and have been shown to have an affinity for plastics (Enyoh et al., 2022), and are even suggested as potentially degradative towards PE (Twala et al., 2020).
Metal–resistant genes have been found in biofilms found on antifouling boat paints, raising concern that antibiotic resistant genes may also be enriched due to cross-resistance mechanisms within these biofilms (Flach et al., 2017). The distribution of these metals, their sorption onto plastic, and their potential incorporation into minerals associated with plastics is a point of concern for environmental and health risks associated with marine plastic debris. Not only are there health concerns with the plastic associated mineral-microbe biofilm, but this combination of thicker biofilm and increased sorption of metals on plastic in the water column indicates downward transport of the particle due to higher particle mass (Kaiser et al., 2017; Michels et al., 2018; Amaral-Zettler et al., 2021; Schmidtmann et al., 2022).
Mineral phases associated with plastic increase aggregate formation of microplastic and nanoplastic in aqueous environments (Ward and Kach, 2009; de Haan et al., 2019). Whilst the stickiness of biofilm can enhance the aggregation of plastic and mineral particles, increasing particle size and increasing sinking rates (Michels et al., 2018). Such plastic particle aggregation linked to microbes and minerals induces vertical downward transport in the water column (Michels et al., 2018; Leiser et al., 2020; Leiser et al., 2021). The presence of high-density biogenic and abiogenic minerals on plastic, like the silicon dioxide of diatoms, will accentuate this sinking effect further. Laboratory studies have demonstrated this to occur regardless of the amount of biofouling (Leiser et al., 2021), suggesting that mineral association with plastic has a dominant role in mediating downward transport of plastic over biofilm alone.
Pyrite (FeS2) framboids were identified in the biofilm matrix of plastic buried in the top sediment (0-2 cm) (Figure 3B). Interestingly, the morphology of these pyrite framboids suggests that they were not formed directly on the plastic. Rather, it seems more likely that they were adhered to and were incorporated into the plastic biofilm matrix after formation in the sediment porespace. Pyrite (FeS2) typically forms in the anoxic zone through a series of biological and chemical reactions, which precipitate this iron sulphide mineral (Figure 3B) The framboid must then transport upwards in the porewater to the top sediment to adhere to the biofilm at the plastic surface, demonstrating how biogeochemical properties influence the plastic surface. Thus, the association of metals like iron and manganese with the plastic surface and the resulting presence of dissolved and solid mineral phases can be linked to metal cycling as is typical for marine sediment (Thamdrup et al., 1994).
Degradation of plastics in the marine coastal environment
PE and PS exposed to some redox compartments showed indications of oxidation. PE oxidized most after 12-month exposure in the water column while PS was more oxidized in sediment. Possible pathways of PE and PS oxidation in this experimental system include UV photo-oxidation, microbial oxidation and metal-catalyzed oxidation. The initiation steps for oxidation of both PE and PS requires the formation of free-radicals. Propagation of degradation then occurs where the radicals react with oxygen or in more complex reactions eventually leading to autoxidation and ceases when two radicals combine and produce an inert product. Unabated, oxidation leaves the polymer backbone vulnerable to breakdown of stronger, covalent bonds that results in fragmentation of the plastic into smaller particles (Gewert et al., 2015; Chamas et al., 2020).
The coastal environment of Denmark is generally shallow, with maximum depth of 30 m, and Svanemøllen Harbor has a depth of ~3m with little tidal change. In shallow coastal environments, it is possible that light penetrates to the sediment, where it becomes quickly attenuated (Prazeres and Renema, 2019). Plastic anchored in the water column was therefore exposed to both light-penetrated and continual oxygen-rich conditions which fosters the auto-oxidation of plastic (Wei and Zimmermann, 2017; Gewert et al., 2018; Romera-Castillo et al., 2018; Ali et al., 2021). The degradation by-products and leachates from photo-oxidative degradation further interact with the surrounding environment, influencing local biogeochemical processes through the provision of carbon (Gewert et al., 2015; Gewert et al., 2018; Romera-Castillo et al., 2018; Gunaalan et al., 2020; Zhu et al., 2020; Masry et al., 2021). Recent laboratory experiments have demonstrated that these produced carbon compounds can be utilized by microbial communities (Birnstiel et al., 2022; Zadjelovic et al., 2022; Goudriaan et al., 2023).
As plastic sinks, it departs the light-influenced marine environment, and UV-photooxidation can no longer dominate as a leading degradation pathway. Top sediment is characterized by gas exchange with the water column (CO2 and O2) (Lueder et al., 2020), and high labile organic matter accumulation. Microbial processes couple the oxidation of this organic matter to available electron acceptors which leads to the release of nutrients, such as inorganic phosphorous and nitrogen (Bloesch, 2009). Plastic degradation by bacteria and fungi has been documented in the marine water and sediment, which must proceed according to these rules of electron exchange (extensively reviewed in Roager and Sonnenschein, 2019; Rogers et al., 2020).
As plastics become more deeply buried over time, or in the case of MP and NPs, are transported via e.g., pore water flow, sediment reworking or burrowing action, they will enter biogeochemical regimes characterized by more compacted sediment, limited exchange with the water column, no light, and decreasing concentrations of O2. Potential plastic degradation pathways, as they are currently understood, become increasingly limited (Oberbeckmann and Labrenz, 2020). Anaerobic microbial metabolisms dominate. Biogeochemical zones dominated by microbial sulfate reduction, iron reduction and associated mineral formation, e.g., precipitation of Fe-sulfide minerals, as well as the cycling of methane become plastics’ surrounding biogeochemical environment (Figures 3C, SI 2). Iron and sulfate reduction are dominant biogeochemical pathways. Here, biodegradation of plastic would have to proceed via the coupling of dissolved or particulate electron acceptors (Fe and sulphide minerals (FeS, FeS2, FeCO3) to organic carbon or possibly plastics as electron donors.
Transition metals, e.g., Cu(II), Cr(III), Fe(III) Mn(III) and to a certain degree Al and Zn can also serve as electron acceptors, fueling microbial metabolism and element cycling at depth in the sediment, these metals, however, are also effective catalysts (Shashoua, 2008). These metals, present in coastal harbors, may contribute to degradation of plastics in low O2 redox environments of the sediment (SI 2). The role of metals as metabolic fuel for biodegradation in anoxic sediment, their role as catalysts, and the relevant process rates in environmental conditions, however, remain to be explored. Extensive chemical changes to the plastics in water and sediment over 6-12 months is not observed despite the biofilm association, mineral loading, and exposure to UV light. This may be explained by the presence of biofilm (microbial and mineral components) on the plastic. A recent study of the role of biofilm in “shielding” of plastic from UV-degradation found that as biofilm developed, transmittance of visible and UV light decreased and slowed the rate of plastic photochemical degradation (Nelson et al., 2021). The decreased transmission of the visible light spectrum was related to the presence of photosynthetic pigments in the biofilm and attributed to the phototrophic organisms present in the biofilm matrix. Plastics in the water column and top sediment at Svanemøllen Harbor and other coastal settings are similarly colonized by phototrophic organisms (Figures 7, 8) that may carry out the same shielding. Similarly, it is possible that the presence of minerals, such as iron and manganese oxides, as well as calcite, not only alters transport behaviour of plastics in the water column (e.g., Leiser et al., 2020; Leiser et al., 2021), but can also shield plastic from UV light, potential also lies here for diatom silicon dioxide to act in a similar manner. Such UV-shielding has been proposed as a mechanism protecting early life on Earth in times of heightened UV-light radiation (Rambler and Margulis, 1980; Phoenix et al., 2001). Beyond the recalcitrance of plastic itself, such considerations may help explain low to negligible oxidation changes detected by FTIR for the PS water sample compared to that exposed to the top sediment (Figure 5) and other apparent preservation of plastics over time.
Conclusion
A 12-month in situ experiment from a Danish marina showed rapid biofilm and mineral formation (within 3 months) on the surfaces of polystyrene and polyethylene. Microbial communities on plastic in the water and sediment were distinct from the surrounding water and sediment matrix, defining a microbial Plastisphere across redox environments. Members of the microbial community found on the two plastics possess the enzymatic toolbox indicative of involvement in plastic degradation, and element cycling at the plastic surface. The microbe-mineral-rich surfaces represent newly introduced, biogeochemically-active sites. The composition of the minerals associated with plastics include biologically-controlled, biologically induced and abiotic minerals of silicon dioxide, iron and manganese oxides as well as minor metals potentially associated with harbor activities. These metals and minerals may act as templates for microbial attachment to the biofilm, and catalysts for further reactions. These close associations of microorganisms and minerals with plastic potentially influence transport and degradation in the environment, but may also shield surfaces from UV light and thereby inhibit oxidation. Future work must focus on the mechanisms and interactions driven by this biogeochemically-complex surface. Microbe-mineral interactions, such as mineral substrate utilization and aggregate formation, have played a key role in the cycling of elements through Earth evolution. Now the introduction of anthropogenic particles on a global scale, such as plastic, has introduced a new component to these interactions. Longer term studies to better understand the role and impact of microbe-mineral interactions on a variety of plastic types are essential.
Data availability statement
The data presented in the study is deposited in the NCBI SRA repository, accession number PRJNA941828.
Author contributions
KR, JC-C, and NP designed the experiments. KR, JC-C, and MD performed the field work. MD, JC-C, and VF-J carried out the microbial community analysis, advised by CL and LR. AT carried out plastic-associated metal analyses. KR and NK performed SEM-EDS. YS carried out FTIR analysis. All authors contributed to data interpretation and manuscript preparation, led by MD and NP. All authors contributed to the article and approved the submitted version.
Funding
This work was supported by the VILLUM FONDEN (grant number: 15397, Cycling in the plastisphere: the biogeochemical fate of marine microplastics) and Geocenter Danmark (PlasticHotspot project no. 3-2018 and TRACE project no. 2-2020) to NP.
Acknowledgments
We thank Paul Christensen (IGN) for technical assistance and research diving support, Svanemøllen Harbor (A. Ostenfeldt, harbor master) and TJ Andersen-IGN KU for their support.
Conflict of interest
The authors declare that the research was conducted in the absence of any commercial or financial relationships that could be construed as a potential conflict of interest.
Publisher’s note
All claims expressed in this article are solely those of the authors and do not necessarily represent those of their affiliated organizations, or those of the publisher, the editors and the reviewers. Any product that may be evaluated in this article, or claim that may be made by its manufacturer, is not guaranteed or endorsed by the publisher.
Supplementary material
The Supplementary Material for this article can be found online at: https://www.frontiersin.org/articles/10.3389/fmars.2023.1134815/full#supplementary-material
References
Abell G. C. J., Bowman J. P. (2005). Colonization and community dynamics of class flavobacteria on diatom detritus in experimental mesocosms based on southern ocean seawater. FEMS Microbiol. Ecol. 53 (3), 379–391. doi: 10.1016/j.femsec.2005.01.008
Ahrens A. P., Culpepper T., Saldivar B., Anton S., Stoll S., Handberg E. M., et al. (2021). A six-day, lifestyle-based immersion program mitigates cardiovascular risk factors and induces shifts in gut microbiota, specifically lachnospiraceae, ruminococcaceae, faecalibacterium prausnitzii: A pilot study. Nutrients 13, 3459. doi: 10.3390/nu13103459
Akther S., Paul G. K., Mahmud S., Hossain M. S., Saleh M. A., Zaman S., et al. (2022). Comparative polymer biodegradation efficiency of an isolated acinetobacter sp. with bravibacillus sp. and e. coli by resting cells. J. Adv. Biotechnol. Exp. Ther. 5, 487. doi: 10.5455/jabet.2022.d130
Ali S. S., Elsamahy T., Koutra E., Kornaros M., El-Sheekh M., Abdelkarim E. A., et al. (2021). Degradation of conventional plastic wastes in the environment: A review on current status of knowledge and future perspectives of disposal. Sci. Total Environ. 771, 144719. doi: 10.1016/J.SCITOTENV.2020.144719
Amaral-Zettler L. A., Zettler E. R., Mincer T. J. (2020). Ecology of the plastisphere. Nat. Rev. Microbiol. 18, 139–151. doi: 10.1038/s41579-019-0308-0
Amaral-Zettler L. A., Zettler E. R., Mincer T. J., Klaassen M. A., Gallager S. M. (2021). Biofouling impacts on polyethylene density and sinking in coastal waters: A macro/micro tipping point? Water Res. 201, 117289. doi: 10.1016/j.watres.2021.117289
Amaral-Zettler L. A., Zettler E. R., Slikas B., Boyd G. D., Melvin D. W., Morrall C. E., et al. (2015). The biogeography of the plastisphere: Implications for policy. Front. Ecol. Environ. 13 (10), 541–546. doi: 10.1890/150017
Ashton K., Holmes L., Turner A. (2010). Association of metals with plastic production pellets in the marine environment. Mar. pollut. Bull. 60, 2050–2055. doi: 10.1016/J.MARPOLBUL.2010.07.014
Barrett J., Chase Z., Zhang J., Holl M. M. B., Willis K., Williams A., et al. (2020). Microplastic pollution in deep-sea sediments from the great Australian bight. Front. Mar. Sci. 7, 576170. doi: 10.3389/fmars.2020.576170
Bergmann M., Wirzberger V., Krumpen T., Lorenz C., Primpke S., Tekman M. B., et al. (2017). High quantities of microplastic in Arctic deep-Sea sediments from the HAUSGARTEN observatory. Environ. Sci. Technol. 51, 11000–11010. doi: 10.1021/ACS.EST.7B03331
Bernardet J. F., Nakagawa Y. (2006). An Introduction to the Family Flavobacteriaceae. In: Dworkin M., Falkow S., Rosenberg E., Schleifer K. H., Stackebrandt E. (eds) The Prokaryotes. Springer, New York, NY. doi: 10.1007/0-387-30747-8_16
Bernbom N., Ng Y. Y., Kjelleberg S., Harder T., Gram L. (2011). Marine bacteria from Danish coastal waters show antifouling activity against the marine fouling bacterium pseudoalteromonas sp. strain S91 and zoospores of the green alga ulva australis independent of bacteriocidal activity. Appl. Environ. Microbiol. 77 (24), 8557–8567. doi: 10.1128/AEM.06038-11
Bhagwat G., O’Connor W., Grainge I., Palanisami T. (2021). Understanding the fundamental basis for biofilm formation on plastic surfaces: Role of conditioning films. Front. Microbiol. 12. doi: 10.3389/FMICB.2021.687118
Birnstiel S., Sebastián M., Romera-Castillo C. (2022). Structure and activity of marine bacterial communities responding to plastic leachates. Sci. Total Environ. 834, 155264. doi: 10.1016/j.scitotenv.2022.155264
Bloesch J. (2009). Sediments of aquatic ecosystems. In Likens G. E. (Ed.), Encyclopedia Inland Waters, 479–490. doi: 10.1016/B978-012370626-3.00210-6
Bowley J., Baker-Austin C., Porter A., Hartnell R., Lewis C. (2021). Oceanic hitchhikers–assessing pathogen risks from marine microplastic. Trends Microbiol. 29 (2), 107–116. doi: 10.1016/j.tim.2020.06.011
Bryant J. A., Clemente T. M., Viviani D. A., Fong A. A., Thomas K. A., Kemp P., et al. (2016). Diversity and activity of communities inhabiting plastic debris in the north pacific gyre. mSystems 1, e00024–16. doi: 10.1128/mSystems.00024-16
Byrne P., Taylor K. G., Hudson-Edwards K. A., Barrett J. E. (2017). Speciation and potential long-term behaviour of chromium in urban sediment particulates. J. Soils Sediments 17 (11), 2666–2676. doi: 10.1007/s11368-016-1558-3
Callahan B. J., McMurdie P. J., Rosen M. J., Han A. W., Johnson A. J. A., Holmes S. P. (2016). DADA2: High-resolution sample inference from illumina amplicon data. Nat. Methods 13, 581–583. doi: 10.1038/nmeth.3869
Carpenter E. J., Smith K. L. (1972). Plastics on the Sargasso Sea surface. Science 1979) 175, 1240–1241. doi: 10.1126/SCIENCE.175.4027.1240
Chamas A., Moon H., Zheng J., Qiu Y., Tabassum T., Jang J. H., et al. (2020). Degradation rates of plastics in the environment. ACS Sustain. Chem. Eng. 8, 3494–3511. doi: 10.1021/acssuschemeng.9b06635
Chaudhary D. K., Kim D. U., Kim D., Kim J. (2019). Flavobacterium petrolei sp. nov., a novel psychrophilic, diesel-degrading bacterium isolated from oil-contaminated Arctic soil. Sci. Rep. 9 (1), 1–9. doi: 10.1038/s41598-019-40667-7
Colton J. B., Knapp F. D., Burns B. R. (1974). Plastic particles in surface waters of the northwestern atlantic. Science 185, 491–497. doi: 10.1126/SCIENCE.185.4150.491
Courtene-Jones W., Quinn B., Gary S. F., Mogg A. O. M., Narayanaswamy B. E. (2017). Microplastic pollution identified in deep-sea water and ingested by benthic invertebrates in the rockall trough, north Atlantic ocean. Environ. pollut. 231, 271–280. doi: 10.1016/J.ENVPOL.2017.08.026
Coyle R., Hardiman G., Driscoll K. O. (2020). Microplastics in the marine environment: A review of their sources, distribution processes, uptake and exchange in ecosystems. Case Stud. Chem. Environ. Eng. 2, 100010. doi: 10.1016/J.CSCEE.2020.100010
Cózar A., Martí E., Duarte C. M., García-de-Lomas J., van Sebille E., Ballatore T. J., et al. (2017). The Arctic ocean as a dead end for floating plastics in the north Atlantic branch of the thermohaline circulation. Sci. Adv. 3, e1600582. doi: 10.1126/SCIADV.1600582/SUPPL_FILE/1600582_SM.PDF
Debroas D., Mone A., Ter Halle A. (2017). Plastics in the north Atlantic garbage patch: A boat-microbe for hitchhikers and plastic degraders. Sci. total Environ. 599, 1222–1232. doi: 10.1016/j.scitotenv.2017.05.059
de Haan W. P., Sanchez-Vidal A., Canals M., Party N. S. S. (2019). Floating microplastics and aggregate formation in the Western Mediterranean Sea. Mar. pollut. Bull. 140, 523–535. doi: 10.1016/j.marpolbul.2019.01.053
De Tender C., Devriese L. I., Haegeman A., Maes S., Vangeyte J., Cattrijsse A., et al. (2017). Temporal dynamics of bacterial and fungal colonization on plastic debris in the north Sea. Environ. Sci. Technol. 51 (13), 7350–7360. doi: 10.1021/acs.est.7b00697
Dobaradaran S., Schmidt T. C., Nabipour I., Khajeahmadi N., Tajbakhsh S., Saeedi R., et al. (2018). Characterization of plastic debris and association of metals with microplastics in coastline sediment along the Persian gulf. Waste Manage. 78, 649–658. doi: 10.1016/J.WASMAN.2018.06.037
Enyoh C. E., Maduka T. O., Duru C. E., Osigwe S. C., Ikpa C. B. C., Wang Q. (2022). In sillico binding affinity studies of microbial enzymatic degradation of plastics. J. Hazardous Materials Adv. 6, 100076. doi: 10.1016/J.HAZADV.2022.100076
Erni-Cassola G., Zadjelovic V., Gibson M. I., Christie-Oleza J. A. (2019). Distribution of plastic polymer types in the marine environment; A meta-analysis. J. Hazardous Materials 369, 691–698. doi: 10.1016/j.jhazmat.2019.02.067
Fabra M., Williams L., Watts J. E. M., Hale M. S., Couceiro F., Preston J. (2021). The plastic Trojan horse: Biofilms increase microplastic uptake in marine filter feeders impacting microbial transfer and organism health. Sci. Total Environ. 797, 149217. doi: 10.1016/J.SCITOTENV.2021.149217
Fischer A. C., Kroon J. J., Verburg T. G., Teunissen T., Wolterbeek H. T. (2007). On the relevance of iron adsorption to container materials in small-volume experiments on iron marine chemistry: 55Fe-aided assessment of capacity, affinity and kinetics. Mar. Chem. 107, 533–546. doi: 10.1016/J.MARCHEM.2007.08.004
Flach C. F., Pal C., Svensson C. J., Kristiansson E., Östman M., Bengtsson-Palme J., et al. (2017). Does antifouling paint select for antibiotic resistance? Sci. Total Environ. 590–591, 461–468. doi: 10.1016/J.SCITOTENV.2017.01.213
Frederiksen J. K., Brendstrup J., Eriksen F. S., Gordon M. A., Knudsen C., Jørgensen M. E., et al. (2003). Engineering geology of Copenhagen. Bull. Eng. Geol. Environ. 62, 189–206. doi: 10.1007/S10064-003-0189-2/FIGURES/12
Galgani L., Loiselle S. A. (2021). Plastic pollution impacts on marine carbon biogeochemistry. Environ. pollut. 268, 115598. doi: 10.1016/J.ENVPOL.2020.115598
Garber A. I., Cohen A. B., Nealson K. H., Ramírez G. A., Barco R. A., Enzingmüller-Bleyl T. C., et al. (2021). Metagenomic insights into the microbial iron cycle of subseafloor habitats. Front. Microbiol. 12. doi: 10.3389/FMICB.2021.667944
Gewert B., Plassmann M. M., Macleod M. (2015). Pathways for degradation of plastic polymers floating in the marine environment. Environ. Sci.: Processes Impacts 17, 1513–1521. doi: 10.1039/c5em00207a
Gewert B., Plassmann M., Sandblom O., Macleod M. (2018). Identification of chain scission products released to water by plastic exposed to ultraviolet light. Environ. Sci. Technol. Lett. 5, 272–276. doi: 10.1021/acs.estlett.8b00119
Goudriaan M., Morales V. H., van der Meer M. T. J., Mets A., Ndhlovu R. T., van Heerwaarden J., et al. (2023). A stable isotope assay with 13C-labeled polyethylene to investigate plastic mineralization mediated by rhodococcus ruber. Mar. pollut. Bull. 186, 114369. doi: 10.1016/J.MARPOLBUL.2022.114369
Gouin T. (2021). Addressing the importance of microplastic particles as vectors for long-range transport of chemical contaminants: Perspective in relation to prioritizing research and regulatory actions. Microplastics Nanoplastics 1 (1), 1–19. doi: 10.1186/S43591-021-00016-W
Gunaalan K., Fabbri E., Capolupo M. (2020). The hidden threat of plastic leachates: A critical review on their impacts on aquatic organisms. Water Res. 184, 116170. doi: 10.1016/j.watres.2020.116170
Hahnke S., Abendroth C., Langer T., Codoñer F. M., Ramm P., Porcar M., et al. (2018). Complete genome sequence of a new ruminococcaceae bacterium isolated from anaerobic biomass hydrolysis. Genome Announcements 6 (14), e00030–e00018. doi: 10.1128/GENOMEA.00030-18
Hansen J., Melchiorsen J., Ciacotich N., Gram L., Sonnenschein E. C. (2021). Effect of polymer type on the colonization of plastic pellets by marine bacteria. FEMS Microbiol. Lett. 368 (5), fnab026. doi: 10.1093/femsle/fnab026
Harris P. T., Maes T., Raubenheimer K., Walsh J. P. (2023). A marine plastic cloud-global mass balance assessment of plastic pollution in deep ocean sediments. Continental Shelf Res. 255, 104947. doi: 10.1016/j.csr.2023.104947
Horton A. A., Dixon S. J. (2018). Microplastics: An introduction to environmental transport processes. Wiley Interdiscip. Reviews: Water 5, e1268. doi: 10.1002/WAT2.1268
Jørgensen B. B. (2000). “Bacteria and marine biogeochemistry,” in Marine geochemistry. Eds. Schulz H. D., Zabel M. (Berlin, Heidelberg: Springer). doi: 10.1007/978-3-662-04242-7_5
Jacquin J., Cheng J., Odobel C., Pandin C., Conan P., Pujo-Pay M., et al. (2019). Microbial ecotoxicology of marine plastic debris: A review on colonization and biodegradation by the “Plastisphere”. Front. Microbiol. 10. doi: 10.3389/fmicb.2019.00865
Jambeck J. R., Geyer R., Wilcox C., Siegler T. R., Perryman M., Andrady A., et al. (2015). Plastic waste inputs from land into the ocean. Science 347 (6223), 768–771. doi: 10.1126/science.1260352
Jian M., Niu J., Li W., Huang Y., Yu H., Lai Z., et al. (2022). How do microplastics adsorb metals? A preliminary study under simulated wetland conditions. Chemosphere 309, 136547. doi: 10.1016/J.CHEMOSPHERE.2022.136547
Jiang P., Zhao S., Zhu L., Li D. (2018). Microplastic-associated bacterial assemblages in the intertidal zone of the Yangtze estuary. Sci. Total Environ. 624, 48–54. doi: 10.1016/J.SCITOTENV.2017.12.105
Kaiser D., Kowalski N., Waniek J. J. (2017). Effects of biofouling on the sinking behavior of microplastics. Environ. Res. Lett. 12 (12), 124003. doi: 10.1088/1748-9326/AA8E8B
Kielemoes J., Bultinck I., Storms H., Boon N., Verstraete W. (2002). Occurrence of manganese-oxidizing microorganisms and manganese deposition during biofilm formation on stainless steel in a brackish surface water. FEMS Microbiol. Ecol. 39, 41–55. doi: 10.1111/J.1574-6941.2002.TB00905.X
Kinoshita S., Kageyama S., Iba K., Yamada Y., Okada H. (1975). Utilization of a cyclic dimer and linear oligomers of ϵ-aminocaproic acid by achrornobacter guttatus KI 72. Agric. Biol. Chem. 39 (6), 1219–1223. doi: 10.1080/00021369.1975.10861757
Kirstein I. V., Wichels A., Gullans E., Krohne G., Gerdts G. (2019). The plastisphere–uncovering tightly attached plastic “specific” microorganisms. PloS One 14 (4), e0215859. doi: 10.1371/journal.pone.0215859
Konhauser K. O., Amskold L., Lalonde S. v., Posth N. R., Kappler A., Anbar A. (2007). Decoupling photochemical Fe(II) oxidation from shallow-water BIF deposition. Earth Planetary Sci. Lett. 258, 87–100. doi: 10.1016/J.EPSL.2007.03.026
Könneke M., Bernhard A. E., de la Torre J. R., Walker C. B., Waterbury J. B., Stahl D. A. (2005). Isolation of an autotrophic ammonia-oxidizing marine archaeon. Nature 437 (7058), 543–546. doi: 10.1038/nature03911
Kowalski N., Reichardt A. M., Waniek J. J. (2016). Sinking rates of microplastics and potential implications of their alteration by physical, biological, and chemical factors. Mar. pollut. Bull. 109, 310–319. doi: 10.1016/J.MARPOLBUL.2016.05.064
Lagerström M., Norling M., Eklund B. (2016). Metal contamination at recreational boatyards linked to the use of antifouling paints–investigation of soil and sediment with a field portable XRF. Environ. Sci. pollut. Res. 23 (10), 10146–10157. doi: 10.1007/s11356-016-6241-0
La Reau A. J., Suen G. (2018). The ruminococci: Key symbionts of the gut ecosystem. J. Microbiol. 56 (3), 199–208. doi: 10.1007/S12275-018-8024-4
Lee S. W., Parker D. L., Geszvain K., Tebo B. M. (2014). Effects of exogenous pyoverdines on fe availability and their impacts on Mn(II) oxidation by pseudomonas putida GB-1. Front. Microbiol. 5. doi: 10.3389/FMICB.2014.00301/ABSTRACT
Leiser R., Jongsma R., Bakenhus I., Möckel R., Philipp B., Neu T. R., et al. (2021). Interaction of cyanobacteria with calcium facilitates the sedimentation of microplastics in a eutrophic reservoir. Water Res. 189, 116582. doi: 10.1016/J.WATRES.2020.116582
Leiser R., Wu G. M., Neu T. R., Wendt-Potthoff K. (2020). Biofouling, metal sorption and aggregation are related to sinking of microplastics in a stratified reservoir. Water Res. 176, 115748. doi: 10.1016/J.WATRES.2020.115748
Leleyter L., Baraud F., Reinert T., Gouali S., Lemoine M., Gil O. (2018). Fate of aluminium released by sacrificial anodes–contamination of marine sediments by environmentally available compounds. Comptes Rendus Geosci. 350 (5), 195–201. doi: 10.1016/j.crte.2018.05.003
Lowenstam H. A. (1981). Minerals formed by organisms. Science 211, 1126–1131. doi: 10.1126/SCIENCE.7008198
Lueder U., Jørgensen B. B., Kappler A., Schmidt C. (2020). Photochemistry of iron in aquatic environments. Environ. Science: Processes Impacts 22, 12–24. doi: 10.1039/C9EM00415G
Lumborg U. (2005). Modelling the deposition, erosion, and flux of cohesive sediment through Øresund. J. Mar. Syst. 56, 179–193. doi: 10.1016/J.JMARSYS.2004.11.003
Maršić-Lučić J., Lušić J., Tutman P., Bojanić Varezić D., Šiljić J., Pribudić J. (2018). Levels of trace metals on microplastic particles in beach sediments of the island of vis, Adriatic Sea, Croatia. Mar. pollut. Bull. 137, 231–236. doi: 10.1016/J.MARPOLBUL.2018.10.027
Masry M., Rossignol S., Gardette J. L., Therias S., Bussière P. O., Wong-Wah-Chung P. (2021). Characteristics, fate, and impact of marine plastic debris exposed to sunlight: A review. Mar. pollut. Bull. 171, 112701. doi: 10.1016/J.MARPOLBUL.2021.112701
McCormick A., Hoellein T. J., Mason S. A., Schluep J., Kelly J. J. (2014). Microplastic is an abundant and distinct microbial habitat in an urban river. Environ. Sci. Technol. 48, 11863–11871. doi: 10.1021/es503610r
McMurdie P. J., Holmes S. (2013). Phyloseq: An r package for reproducible interactive analysis and graphics of microbiome census data. PLoS ONE 8, 4. doi: 10.1371/journal.pone.0061217
Medvedev I. P., Rabinovich A. B., Kulikov E. A. (2016). Tides in three enclosed basins: The baltic, black, and caspian seas. Front. Mar. Sci. 3. doi: 10.3389/FMARS.2016.00046
Melton E. D., Swanner E. D., Behrens S., Schmidt C., Kappler A. (2014). The interplay of microbially mediated and abiotic reactions in the biogeochemical fe cycle. Nat. Rev. Microbiol. 12 (12), pp.797–808. doi: 10.1038/nrmicro3347
Michels J., Stippkugel A., Lenz M., Wirtz K., Engel A. (2018). Rapid aggregation of biofilm-covered microplastics with marine biogenic particles. Proc. R. Soc. B 285 (1885), 20181203. doi: 10.1098/RSPB.2018.1203
Müller S., Fiutowski J., Rubahn H. G., Posth N. R. (2021). Nanoplastic transport in aqueous environments: The role of chemo-electric properties and hydrodynamic forces for nanoplastic-mineral interaction. ChemRxiv. doi: 10.26434/chemrxiv-2021-zcv78
Munier B., Bendell L. I. (2018). Macro and micro plastics sorb and desorb metals and act as a point source of trace metals to coastal ecosystems. PloS One 13, e0191759. doi: 10.1371/JOURNAL.PONE.0191759
Negoro S. (2000). Biodegradation of nylon oligomers. Appl. Microbiol. Biotechnol. 54 (4), 461–466. doi: 10.1007/s002530000434
Nelson T. F., Reddy C. M., Ward C. P. (2021). Product formulation controls the impact of biofouling on consumer plastic photochemical fate in the ocean. Environ. Sci. Technol. 55, 8898–8907. doi: 10.1021/acs.est.1c02079
Nishiwaki-Akine Y., Kanazawa S., Matsuura N., Yamamoto-Ikemoto R. (2020). Biodegradability of woody film produced by solvent volatilisation of Japanese beech solution. Sci. Rep. 10 (1), 1–9. doi: 10.1038/s41598-019-57228-7
Oberbeckmann S., Kreikemeyer B., Labrenz M. (2018). Environmental factors support the formation of specific bacterial assemblages on microplastics. Front. Microbiol. 8. doi: 10.3389/fmicb.2017.02709
Oberbeckmann S., Labrenz M. (2020). Marine microbial assemblages on microplastics: Diversity, adaptation, and role in degradation. Annu. Rev. Mar. Sci. 12, 209–232. doi: 10.1146/annurev-marine-010419-010633
Oren A., Xu X. W. (2014). The family Hyphomicrobiaceae. In: Rosenberg E., DeLong E. F., Lory S., Stackebrandt E., Thompson F. (eds) The Prokaryotes. Springer, Berlin, Heidelberg. doi: 10.1007/978-3-642-30197-1_257
Palacios P. A., Snoeyenbos-West O., Löscher C. R., Thamdrup B., Rotaru A. E. (2019). Baltic Sea Methanogens compete with acetogens for electrons from metallic iron. ISME J. 13(12), 3011–3023. doi: 10.1038/s41396-019-0490-0
Parada A. E., Needham D. M., Fuhrman J. A. (2016). Every base matters: assessing small subunit rRNA primers for marine microbiomes with mock communities, time series and global field samples. Environ. Microbiol. 18, 1403–1414. doi: 10.1111/1462-2920.13023
Peeken I., Primpke S., Beyer B., Gütermann J., Katlein C., Krumpen T., et al. (2018). Arctic Sea ice is an important temporal sink and means of transport for microplastic. Nat. Commun. 9(1), 1–12. doi: 10.1038/s41467-018-03825-5
Phoenix V. R., Konhauser K. O., Adams D. G., Bottrell S. H. (2001). Role of biomineralization as an ultraviolet shield: Implications for archean life. Geology 823, 823–826. doi: 10.1130/0091-7613(2001)029<0823:ROBAAU>2.0.CO;2
Pinnell L. J., Conkle J., Turner J. W. (2022). Microbial succession during the degradation of bioplastic in coastal marine sediment favors sulfate reducing microorganisms. Front. Mar. Sci. 9, 945822. doi: 10.3389/fmars.2022.945822
Pohlner M., Dlugosch L., Wemheuer B., Mills H., Engelen B., Reese B. K. (2019). The majority of active rhodobacteraceae in marine sediments belong to uncultured genera: A molecular approach to link their distribution to environmental conditions. Front. Microbiol. 10. doi: 10.3389/FMICB.2019.00659
Polhill L., de Bruijn R., Amaral-Zettler L., Praetorius A., van Wezel A. (2022). Daphnia magna's favorite snack: Biofouled plastics. Environ. Toxicol. Chem. 41 (8), 1977–1981. doi: 10.1002/etc.5393
Prazeres M., Renema W. (2019). Evolutionary significance of the microbial assemblages of large benthic foraminifera. Biol. Rev. 94, 828–848. doi: 10.1111/BRV.12482
Pujalte M. J., Lucena T., Ruvira M. A., Arahal D. R., Macián M. C. (2014). The family Rhodobacteraceae. In: Rosenberg E., DeLong E. F., Lory S., Stackebrandt E., Thompson F. (eds) The Prokaryotes. Springer, Berlin, Heidelberg. doi: 10.1007/978-3-642-30197-1_377
Quast C., Pruesse E., Yilmaz P., Gerken J., Schweer T., Yarza P., et al. (2013). The SILVA ribosomal RNA gene database project: Improved data processing and web-based tools. Nucl. Acids Res. 41 (D1), D590–D596. doi: 10.1093/nar/gks1219
Rambler M. B., Margulis L. (1980). Bacterial resistance to ultraviolet irradiation under anaerobiosis: Implications for pre-phanerozoic evolution. Science 210, 638–640. doi: 10.1126/SCIENCE.7001626
Riordan J. F., Vallee B. L. (1974). The functional roles of metals in metalloenzymes. In: Friedman M. (eds) Protein-Metal Interact. Springer, Boston, MA. 48, 33–57. doi: 10.1007/978-1-4684-0943-7_2
Roager L., Sonnenschein E. C. (2019). Bacterial candidates for colonization and degradation of marine plastic debris. Environ. Sci. Technol. 53(20), 11636–11643. doi: 10.1021/acs.est.9b02212
Rogers K. L., Carreres-Calabuig J. A., Gorokhova E., Posth N. R. (2020). SPECIAL ISSUE-CURRENT EVIDENCE micro-by-micro interactions: How microorganisms influence the fate of marine microplastics. Limnology Oceanogr. Lett. 5, 18–36. doi: 10.1002/lol2.10136
Romera-Castillo C., Pinto M., Langer T. M., Álvarez-Salgado X. A., Herndl G. J. (2018). Dissolved organic carbon leaching from plastics stimulates microbial activity in the ocean. Nat. Commun. 9 (1), 1430. doi: 10.1038/s41467-018-03798-5
Sanz-Lázaro C., Casado-Coy N., Beltrán-Sanahuja A. (2021). Biodegradable plastics can alter carbon and nitrogen cycles to a greater extent than conventional plastics in marine sediment. Sci. Total Environ. 756, 143978. doi: 10.1016/J.SCITOTENV.2020.143978
Schmidtmann J., Elagami H., Gilfedder B. S., Fleckenstein J. H., Papastavrou G., Mansfeld U., et al. (2022). Heteroaggregation of PS microplastic with ferrihydrite leads to rapid removal of microplastic particles from the water column. Environ. Science: Processes Impacts 24 (10), 1782–1789. doi: 10.1039/D2EM00207H
Seeley M. E., Song B., Passie R., Hale R. C. (2020). Microplastics affect sedimentary microbial communities and nitrogen cycling. Nat. Commun. 11 (1), 1–10. doi: 10.1038/s41467-020-16235-3
Shashoua Y. (2008). Conservation of plastics: Materials science, degradation and preservation, conservation of plastics: Materials science, degradation and preservation (Routledge: Taylor and Francis). doi: 10.4324/9780080878782
Sheridan E. A., Fonvielle J. A., Cottingham S., Zhang Y., Dittmar T., Aldridge D. C., et al. (2022). Plastic pollution fosters more microbial growth in lakes than natural organic matter. Nat. Commun. 13 (1), 1–9. doi: 10.1038/s41467-022-31691-9
Song Y. K., Hong S. H., Jang M., Kang J. H., Kwon O. Y., Han G. M., et al. (2014). Large Accumulation of micro-sized synthetic polymer particles in the sea surface microlayer. Environ. Sci. Technol. 48, 9014–9021. doi: 10.1021/es501757s
Soroldoni S., Castro Í.B., Abreu F., Duarte F. A., Choueri R. B., Möller O. O., et al. (2018). Antifouling paint particles: Sources, occurrence, composition and dynamics. Water Res. 137, 47–56. doi: 10.1016/j.watres.2018.02.064
Tagg A. S., Oberbeckmann S., Fischer D., Kreikemeyer B., Labrenz M. (2019). Paint particles are a distinct and variable substrate for marine bacteria. Mar. pollut. Bull. 146, 117–124. doi: 10.1016/J.MARPOLBUL.2019.06.013
Taylor M. L., Gwinnett C., Robinson L. F., Woodall L. C. (2016). Plastic microfibre ingestion by deep-sea organisms. Sci. Rep. 6 (1), pp.1–pp.9. doi: 10.1038/srep33997
Teunisse G. M. (2018). Fantaxtic plots for phyloseq data. r package version 4.1.2. Available at: https://github.com/gmteunisse/Fantaxtic.
Thamdrup B., Fossing H., Jørgensen B. B. (1994). Manganese, iron and sulfur cycling in a coastal marine sediment, Aarhus bay, Denmark. Geochim. Cosmochim. Acta 58, 5115–5129. doi: 10.1016/0016-7037(94)90298-4
Thompson R. C., Olsen Y., Mitchell R. P., Davis A., Rowland S. J., John A. W. G., et al. (2004). Lost at Sea: Where is all the plastic? Science New Ser. 304 (5672), 838–838. doi: 10.1126/science.1094559
Turner A. (2021). Paint particles in the marine environment: An overlooked component of microplastics. Water Res. X 12, 100110. doi: 10.1016/J.WROA.2021.100110
Twala P. P., Mitema A., Baburam C., Feto N. A. (2020). Breakthroughs in the discovery and use of different peroxidase isoforms of microbial origin. AIMS Microbiol. 6, 330. doi: 10.3934/MICROBIOL.2020020
Vaksmaa A., Knittel K., Abdala Asbun A., Goudriaan M., Ellrott A., Witte H. J., et al. (2021). Microbial communities on plastic polymers in the Mediterranean Sea. Front. Microbiol. 12. doi: 10.3389/fmicb.2021.673553
van Sebille E., Wilcox C., Lebreton L., Maximenko N., Hardesty B. D., van Franeker J. A., et al. (2015). A global inventory of small floating plastic debris. Environ. Res. Lett. 10, 124006. doi: 10.1088/1748-9326/10/12/124006
Ward J. E., Kach D. J. (2009). Marine aggregates facilitate ingestion of nanoparticles by suspension-feeding bivalves. Mar. Environ. Res. 68 (3), 137–142. doi: 10.1016/j.marenvres.2009.05.002
Wei R., Zimmermann W. (2017). Microbial enzymes for the recycling of recalcitrant petroleum-based plastics: How far are we? Microbial. Biotechnol. 10, 1308–1322. doi: 10.1111/1751-7915.12710
Wong C. S., Green D. R., Cretney W. J. (1974). Quantitative tar and plastic waste distributions in the pacific ocean. Nature 247, 30–32. doi: 10.1038/247030a0
Wright R. J., Bosch R., Gibson M. I., Christie-Oleza J. A. (2020). Plasticizer degradation by marine bacterial isolates: A proteogenomic and metabolomic characterization. Environ. Sci. Technol. 54, 2244–2256. doi: 10.1021/acs.est.9b05228
Wuchter C., Abbas B., Coolen M. J., Herfort L., van Bleijswijk J., Timmers P., et al. (2006). Archaeal nitrification in the ocean. Proc. Natl. Acad. Sci. 103 (33), 12317–12322. doi: 10.1073/pnas.0600756103
Ye X., Cheng Z., Wu M., Hao Y., Lu G., Hu B. X., et al. (2022). Effects of clay minerals on the transport of polystyrene nanoplastic in groundwater. Water Res. 223, 118978. doi: 10.1016/j.watres.2022.118978
Yin C. F., Xu Y., Zhou N. Y. (2020). Biodegradation of polyethylene mulching films by a co-culture of acinetobacter sp. strain NyZ450 and bacillus sp. strain NyZ451 isolated from tenebrio molitor larvae. Int. Biodeterioration Biodegradation 155, 105089. doi: 10.1016/j.ibiod.2020.105089
Yu Y., Miao L., Adyel T. M., Kryss W., Wu J., Hou J. (2023). Aquatic plastisphere: Interactions between plastics and biofilms. Environ. pollut. 322, 121196. doi: 10.1016/j.envpol.2023.121196
Zadjelovic V., Erni-Cassola G., Obrador-Viel T., Lester D., Eley Y., Gibson M. I., et al. (2022). A mechanistic understanding of polyethylene biodegradation by the marine bacterium alcanivorax. J. Hazardous Materials 436, 129278. doi: 10.1016/j.jhazmat.2022.129278
Zettler E. R., Mincer T. J., Amaral-Zettler L. A. (2013). Life in the “Plastisphere”: Microbial communities on plastic marine debris. Environ. Sci. Technol. 47, 7137–7146. doi: 10.1021/es401288x
Keywords: plastic, coastal biogeochemistry, microbe-mineral, plastisphere, plastic degradation
Citation: Dodhia MS, Rogers KL, Fernández-Juárez V, Carreres-Calabuig JA, Löscher CR, Tisserand AA, Keulen N, Riemann L, Shashoua Y and Posth NR (2023) Microbe-mineral interactions in the Plastisphere: Coastal biogeochemistry and consequences for degradation of plastics. Front. Mar. Sci. 10:1134815. doi: 10.3389/fmars.2023.1134815
Received: 30 December 2022; Accepted: 28 February 2023;
Published: 23 March 2023.
Edited by:
Nadia A. Samak, Institute of Process Engineering (CAS), ChinaReviewed by:
Haikun Zhang, Yantai Institute of Coastal Zone Research (CAS), ChinaEl-Sayed Salama, Lanzhou University, China
Copyright © 2023 Dodhia, Rogers, Fernández-Juárez, Carreres-Calabuig, Löscher, Tisserand, Keulen, Riemann, Shashoua and Posth. This is an open-access article distributed under the terms of the Creative Commons Attribution License (CC BY). The use, distribution or reproduction in other forums is permitted, provided the original author(s) and the copyright owner(s) are credited and that the original publication in this journal is cited, in accordance with accepted academic practice. No use, distribution or reproduction is permitted which does not comply with these terms.
*Correspondence: Nicole R. Posth, bnJlcEBpZ24ua3UuZGs=