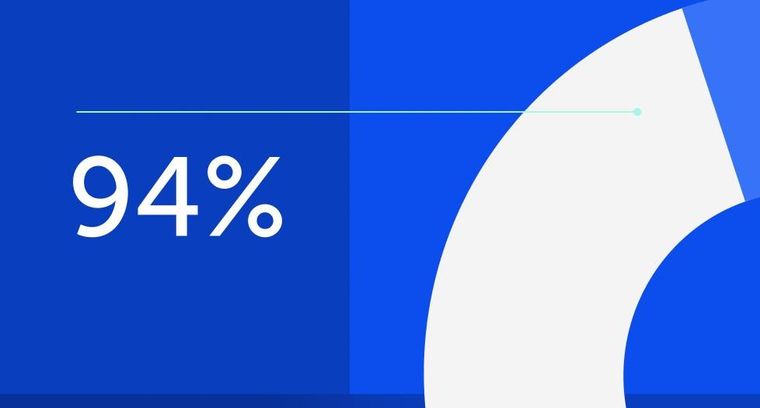
94% of researchers rate our articles as excellent or good
Learn more about the work of our research integrity team to safeguard the quality of each article we publish.
Find out more
SYSTEMATIC REVIEW article
Front. Mar. Sci., 15 March 2023
Sec. Global Change and the Future Ocean
Volume 10 - 2023 | https://doi.org/10.3389/fmars.2023.1134699
This article is part of the Research TopicEcological Consequences of Climate Change in Boreal Marginal SeasView all 11 articles
Plankton respiration is a major process removing oxygen from pelagic environments and constitutes one of the largest oxygen transformations in the sea. Where the O2 supplies due to dissolution, advection and oxygenic photosynthesis are not sufficient, hypoxic, or anoxic waters may result. Coastal waters with limited water exchange are especially prone to have low oxygen levels due to eutrophication and climate change. To support marine environmental management in a period of rapid climate change, we investigated the current knowledge of regulating plankton respiration based on field and experimental studies reported in the literature. Models for regulation of plankton respiration was tested on a three-year field data set. Temperature is the most reported predictor positively influencing plankton respiration (mean r2 = 0.50, n=15). The organic carbon supply driven by primary production has a similar coefficient of determination but fewer reported relationships (mean r2 = 0.52, n=6). Riverine discharges of dissolved organic carbon can override the influence of primary production in estuaries precluding effects of nutrient reductions. The median predictions of respiration regulation produced by current models vary by a factor of 2 from the median of observed values and extreme values varied even more. Predictions by models are therefore still too uncertain for application at regional and local scales. Models with temperature as predictor showed best performance but deviated from measured values in some seasons. The combined dependence of plankton respiration on temperature, phytoplankton production and discharge of riverine organic carbon will probably lead to increased oxygen consumption and reduced oxygen levels with projected climate change. This will be especially pronounced where increased precipitation is expected to enhance riverine discharges of carbon compounds. The biologically mediated transfer of carbon for long-term storage in deeper layers will slow down. Implementation of plankton respiration measurements in long-term ecological monitoring programs at water body and basin scales is advocated, which would enable future multivariate analyses and improvements in model precision across aquatic environments.
The development of large volumes of sea water with low oxygen concentrations (hypoxic) or where oxygen is absent (anoxic) is of increasing concern for society and for the sustainability of marine ecosystem services (Diaz and Rosenberg, 2008; Rabalais et al., 2010; Zhang et al., 2010; Carstensen et al., 2014; Breitburg et al. 2018). Outgassing due to elevated temperatures and enhanced respiration rates of marine organisms are two major processes that decrease oxygen concentrations in aquatic systems (Oschlies et al., 2018). In addition to removing oxygen, respiration also contributes to elevated atmospheric carbon loads by producing CO2 (Figure 1) (Rivkin and Legendre, 2001; Sanders et al., 2016). Current estimates show that less than 1% of carbon assimilated in the sea will end up in deep waters over millennia and thus be removed from biosphere cycling. More than 90% of sedimenting carbon is mineralized back to the atmosphere as CO2 by respiration (Henson et al., 2011; Bach et al., 2019; Fender et al., 2019; Giering et al., 2020). Consequently, the regulation of respiration and associated oxygen consumption will be crucial to accurately model marine and global carbon cycles and to assess the ability of biological and microbial carbon pumps to mitigate CO2 levels in the biosphere (Dang, 2020). This knowledge is also crucial for stakeholders and environmental managers who are responsible for sustaining ecosystem functions, biodiversity, and chemical compositions in the sea.
Figure 1 Schematic view of the role of respiration in the biological and microbial carbon pumps in the sea. Respiration drives oxygen consumption and mineralization of organic carbon pools to CO2 and its emission to the atmosphere. Understanding respiration regulation is therefore crucial for the carbon budget of the atmosphere and the capacity of the ocean to assimilate carbon. Phyto-, protozooplankton and prokaryotic plankton are crucial for operating these processes together with mesozooplankton.
Therefore, we investigated the current understanding of the regulation of plankton respiration using reported general models applied on a coastal multi-year data set of plankton respiration from the well-studied Öre Estuary located in the boreal zone (The Baltic Sea, Vikström and Wikner, 2019; Vikström et al., 2020). The boreal zone constitutes the next largest land carbon reservoir on the planet (Pan et al., 2011), and a substantial load of organic carbon is discharged to the coastal zone (Reader et al., 2014). Coastal waters are important due to high marine biodiversity and productivity, load of terrestrial organic carbon by river discharge and that limited water exchange increases risk of hypoxia in some coastal areas (e.g., the Baltic Sea). Pelagic respiration is dominant in the water column and is the focus of this study, while benthic respiration comprises one-fourth of total marine respiration in estuarine waters (Hopkinson and Smith, 2005). In deeper oceanic environments, pelagic respiration is expected to be even more dominant due to degradation of sedimentary matter by bacterioplankton (Cho and Azam, 1988). Plankton respiration will thereby have a major impact on the marine carbon distribution between the organic fraction and CO2 in both coastal and offshore environments.
The “biological carbon pump” (BCP) is defined as the assimilation of CO2 and synthesis of biomass by autotrophic plankton, followed by sedimentation of dead and aggregated cells as well as faecal pellets produced by grazing zooplankton to deeper water layers (Figure 1). The major transformation of biomass to CO2 occurs in surface waters, and less than 10% is transported to deeper waters (Henson et al., 2011). The potential transformation by prokaryotes of the bioavailable carbon substrates reaching the deep water to recalcitrant compounds is named the “microbial carbon pump” (MCP). Direct evidence for the generation of recalcitrant compounds is methodologically challenging, and only limited experimental evidence has been reported thus far (Jiao et al., 2010). The combined action of BCP and MCP has been proposed as a mechanism of the biosphere to remove CO2 from the atmosphere, a mechanism that is proposed to be bioengineered to speed up CO2 removal.
BCP and MCP are efficiently counteracted by respiration throughout the entire water column (Robinson, 2019). Prokaryotes not only mineralize dissolved and particulate organic matter in the surface layer but also colonize, dissolve and degrade sedimenting aggregates and faecal pellets (Cho and Azam, 1988; Azam and Malfatti, 2007). Determinations of mainly free-living prokaryotic respiration are relevant, as the contribution from respiration by particle-associated prokaryotes seems limited (Belcher et al., 2016), possibly due to the efficient dissolution of aggregates. Microorganisms perform this degradation of organic matter and thereby dominate oxygen consumption in the sea, where phyto- and bacterioplankton account for approximately 33% each, and protozooplankton accounts for 24% (Figure 2, Robinson et al., 2005). The respiration by microbial plankton in the water column is thereby a major cause of the small fraction of carbon reaching the deep ocean, which originally formed in the marine photic zone. Consequently, oxygen consumption contributing to hypoxia and emission of CO2 will mainly be caused by microbial activity in the pelagic realm. For coastal and estuarine environments, it is relevant to point out that planktonic respiration is already a major process that converts organic carbon to CO2 in shallower waters (i.e., > 40 m bottom depth) (Sandberg et al., 2004; Hopkinson and Smith, 2005).
Figure 2 Pie diagram showing the distribution of pelagic respiration among phytoplankton, bacterioplankton, protozooplankton, mesozooplankton and other organisms (e.g., fish) based on Robinson and Williams 2005 (their Table 9.2).
A better understanding of the controlling factors of plankton respiration and how it will be affected by projected climate changes is therefore important. We compile the current understanding of how oxygen consumption is regulated with a particular focus on plankton respiration in the boreal zone. Fish respiration was not investigated, as it constitutes a relatively small part of pelagic respiration (Figure 2, Robinson et al., 2005). Mesozooplankton abundances in Winkler bottle volumes are too low (typically < 150 ml) to contribute to these measurements. Additionally, mesozooplankton respiration constitutes a small share of pelagic respiration (Figure 2, Robinson et al., 2005).
Reports providing empirical evidence for how pelagic oxygen consumption is regulated were compiled from the current literature, reviews, and book sections. Models related to pelagic oxygen consumption were also searched in articles using physical-biogeochemical models to project the effects on oxygen status caused by climate change. Pelagic oxygen consumption consists primarily of plankton respiration, including bacterioplankton (i.e., prokaryotic plankton, Figure 2). Benthic respiration and NH4 oxidation (e.g., nitrification) were not investigated.
The reported mathematical functions with predictors, coefficients of determination (r2) and coefficients of precision were collected. The r2-values were used as a measure of the strengths of relationships. When log transformed data were used to define the statistical relationship those relationships were given lesser rank than relationships obtained with untransformed data to predict plankton respiration on regional or local scales.
Selected equations modelling plankton respiration were tested for their predictive powers using a three-year field dataset (Vikström et al., 2020) and a study of bacterioplankton respiration (Vikström and Wikner, 2019), both collected from a boreal estuary in the northern Baltic Sea.
Reported assessments of the expected effects of current climate change projections on oxygen consumption and oxygen concentrations in the references were compiled.
A comprehensive compilation of the scientific knowledge of aquatic respiration contained in the book by Williams et al. (2005) was used to cover older references. An earlier compilation of bacterioplankton respiration was found in Robinson (2008).
The Web of Science was searched using the following syntaxes: (TS=respiration OR (TS=oxygen AND TS=consumption)) AND (TS=marine OR TS=Sea) AND (TS=climate AND TS=Change) (date of search: 15th September 2022) and (TS=respiration OR (TS=oxygen AND TS=consumption)) AND (TS=marine OR TS=Sea) AND (TS=control AND TS=Regulation) (date of search: 16th September 2022)
References in the authors’ EndNote libraries and paper copy libraries from three decades of research were another source for collecting references.
Articles and chapters were searched for figures that showed relationships between oxygen consumption or respiration versus different predictors. Only marine environments were investigated. The equations for relationships should be reported with at least the coefficient of determination provided. It was also ideal when the statistical significances, number of values and precisions of derived coefficients of the fitted model were reported.
Reports of Q10- or activation energy-values (Ea) for plankton and bacterioplankton respiration were compiled from estuaries and ocean ecosystems.
The texts were screened for titles, subtitles, or conclusions regarding oxygen consumption regulation. In addition, reported assessments of the effect of climate change on oxygen consumption and oxygen status were compiled.
Predictors where significant relationships from several investigations were available were selected for further analysis. Here, the equations reported were compiled and ranked according to their r2 values as indications of model fit. Ease of prediction was seen as key for application to environmental management.
A three-year multiple season dataset of planktonic respiration that was measured with optode sensors (the OPTOCS method, n=191,Vikström et al., 2020) and bacterioplankton respiration data from two seasons (n=42, Vikström and Wikner, 2019), estimated with the same method. The two datasets are from the boreal Öre Estuary (northern Baltic Sea), with high riverine carbon input and encompasses complementary data such as primary production (14CO2 incorporation), bacterioplankton community growth rates (3H-thymidine incorporation), bacterioplankton carbon contents, temperatures, dissolved organic carbon and nutrients. The datasets were used for testing the different models found in the literature in a coastal boreal environment.
One significant relationship was reported as an r2 value by Hopkinson and Smith (2005) but lacked derived equations or associated statistical information. To add this model to the analysis, data were extracted from their Figure 8.9 (WebPlotDigitizer, https://apps.automeris.io/wpd/) and were added to Table 1.
Table 1 Functional relationships and their coefficient of determination (r2) for different quantities of pelagic respiration compiled from the indicated references.
To evaluate the explanatory degrees of models (i.e., Figure 3), the category “plankton respiration” included one “phyto”- and “picoplankton” category each. Additionally, one “planktonic and benthic” category was joined with the plankton category. Discrete values from different depths were used for model testing unless the model was designed for integrated values, where the observed values were integrated in the same manner. Boxplots were used to describe the levels and distributions of values when more than one case occurred. Determinations of model capabilities to predict plankton respiration in the dataset with observations were performed in R Studio (version 2022.07.01 + 554, R_Core_Team, 2021) and packages therein. The conformity between the observed and predicted values of the different models was assessed by boxplots across seasons and residual root mean square error (RMSE) values (Sokal and Rohlf, 1995). Boxplots indicate conformity for both the level and distribution of data, while the RMSEs show residual variances that are not explained by the models. When using temperature as the main driver, the measured temperature at the time of estimation of respiration was used. Complementary data were extracted from the dBotnia database (dBotnia, 1994), which are identical to those reported by Vikström et al. (2020). For the model by Eilola et al. (2009), bioavailable detritus was modelled as 0.75 × [DOC] assuming 50% of marine DOC to be bioavailable and POC constituting 25% of total organic carbon (Forsgren and Jansson, 1992; Sandberg et al., 2004). Bacterioplankton abundances and growth rates were available in the authors’ datasets and are presented in Vikström and Wikner (2019). The measurement of oxygen consumption by optodes sensors (OPTOCS method) enables extraction of initial air saturations and oxygen concentrations from individual runs and was used here for Equation 7 (Table 1). Model fits were evaluated by the root mean square error values calculated for each model (Equation 1) and are presented in Supplementary Table S2.
Figure 3 Boxplots showing the distributions of the coefficient of determination (r2) of environmental predictors for plankton respiration (A) and bacterioplankton respiration (B) reported in the literature. The dashed horizontal black line represents the level for 50% of the explanation. Categories without any boxplot distributions represent values from a single study. The numbers of observations for categories exceeding 1 are shown. The predictors bacterioplankton abundance (BA), beam attenuation (Beam-Att), chlorophyll-a (chl-a), phytoplankton biomass (PhB), phytoplankton production (PhP), particulate organic carbon (POC), riverine total organic carbon (RTOC), temperature (Temp), bacterioplankton growth (BG), dissolved organic carbon (DOC) and dissolved organic nitrogen (DON) are shown.
A total of 36 references were found that included empirical information regarding plankton respiration regulation (Supplementary Table S1). Three of those lacked coefficients of determination and were removed from the data analysis of r2. The publication years of the collected articles covered 1991 to 2020. One-third of those came from books that previously reviewed the subject field. In addition, 4 references reporting different coupled physical-biogeochemical models were reviewed.
As a primary strategy to investigate the current knowledge of plankton respiration regulation, we reviewed some of the models that were applied to simulate the marine carbon cycle. However, in several references the equations used for oxygen consumption were not provided, alternatively based on mass balance calculations or on relationships coupled to nitrogen cycling (Neumann et al., 2002; Savchuk, 2002; Eilola et al., 2009; Meier et al., 2011b). Among reported equations, the Swedish Coastal and Ocean Biogeochemical model (SCOBI) calculate pelagic oxygen consumption based on detritus mineralization and temperature (Eilola et al., 2009). Bendtsen and Hansen (2013) provided a model for oxygen consumption that is included in Table 1, referring to White et al. (1991); Pomeroy and Wiebe (2001) and Lomas et al. (2002) regarding temperature control of pelagic respiration. Their relationship uses a temperature sensitivity-dependence (Q10-value) and half-saturation constant for oxygen concentrations that differs from most other relationships presented in Table S1. They also calculated benthic respiration in a similar manner using different parameter values. The inclusion of oxygen concentration is scientifically motivated but rarely used, as many studies of plankton respiration are performed in well oxygenated environments. However, in environments showing hypoxia, this predictor becomes crucial for proper modelling of oxygen consumption. However, no quality measure of the relationship by Bendtsen and Hansen (2013) is provided, such as the explanation degree or other measures of fit to observed data.
Few studies have directly addressed the regulation of plankton respiration. Research with a focus on respiration has been directed toward the trophic balance between pelagic respiration and carbon fixation (Duarte and Agusti, 1998; Williams and Le, 1998; del Giorgio and Duarte, 2002; Williams et al., 2004) and more recently, climate change effects (Lopez-Urrutia et al., 2006; Vazquez-Dominguez et al., 2007; Sarmento et al., 2010; Panigrahi et al., 2013). One study that directly addressed the regulation of bacterioplankton respiration showed a relationship to DOC (Alonso-Saez et al., 2008), and several studies of respiration in Chesapeake Bay also provide valuable results that are relevant to the regulation of plankton and bacterioplankton respiration (Smith and Kemp, 1995; Smith and Kemp, 2001; Smith and Kemp, 2003). Relationships among respiration and various predictors are also reported in an outstanding review and synthesis of respiration studies in aquatic environments (Williams et al., 2005). For bacterioplankton respiration, a book section by Robinson (2008) provided a comprehensive background. Given the limited community of distinguished researchers in the field who are included in the reference list, earlier reviews of respiration and our own research activity in the field in the past two decades, the risk of having overlooked key reports is assessed to be low.
Studies reporting significant relationships with different predictors were compiled to assess the current knowledge of plankton respiration control (Table S1). The reported coefficient of determination (r2 univariate, R2 multivariate) were used as measures of the explanatory powers of the relationships. A plot of r2 versus predictor provides an overview of the current understanding of plankton and bacterioplankton respiration regulation (Figure 3). Due to varying types of respiration that were measured and distributed as untransformed or transformed values, the number of cases for each combination with predictors become low. The ambient temperature in the sampled environment is the most reported governing predictor (grand mean r2 = 0.50, n=15). Reports using untransformed values for plankton respiration are typically obtained in more homogenous environments and show higher coefficient of determination than those based on logarithm transformed values (i.e., cross-ecosystem studies). Analyses of temperature regulation based on untransformed values alone were performed on aggregated basins or environments that were deemed similar (i.e., estuaries) and resulted in a grand mean r2 of 0.61 (n=9). Cross-system analyses, which are typically based on logarithm transformed values prior to statistical tests, show a lower mean r2- value of 0.32 (n=6) for temperature. Coefficient of determination based on untransformed values are higher (r2 = 0.74, n=6) than when based on logarithm transformed values for plankton respiration, but not so for bacterioplankton respiration. Given the lower coefficient of determination and that back-transformed coefficients are associated with wider confidence intervals, the uncertainties for relationships based on logarithm transformed values are substantial. Increasing imprecision when transforming a predictive function from logarithmic to untransformed measures is of concern when applied to local water bodies or when doing basin scale planning within marine environmental management.
The supply of carbon substrates as a regulator of plankton respiration is also reported, albeit with fewer cases (r2 = 0.52, n=6). Those encompasses different predictors, such as gross primary production (GPP), net primary production (NPP), net plankton community production (NPCP) and riverine DOC (rDOC). The coefficient of determination are comparably high based on the untransformed values for GPP alone (r2 = 0.63, n=2), representing estuarine environments. One report of a significant relationship with phytoplankton biomass (i.e., chlorophyll-a) from the Gulf of Riga had the highest r2 value reported for a predictor but re-analysis resulted in a lower value (r2 = 0.65, year 1995 data) (Olesen et al., 1999). This value was close to one case of a high explanation for chlorophyll-a based on untransformed values reported from the Chesapeake Bay (r2 = 0.70) (Smith and Kemp, 2001). The predictive power of phytoplankton abundance may reflect its importance as a source for carbon substrate supplies via zooplankton exudation, faeces pellet production and dissolution. A lack of effects of mineral nutrients (e.g., NO3 and PO4) on bacterioplankton respiration was reported in a one-year field study (Lemée et al., 2002). The relationship with the supply of organic carbon substrates (including plankton biomass) is directly relevant for assigning eutrophication as a cause of hypoxia development. The high coefficient of determination between plankton respiration and gross primary production from estuaries is the most powerful relationship reported of this type (r2 = 0.77, n=29, untransformed) (Hopkinson and Smith, 2005).
When comparing results and models from different studies both the environmental conditions and methodology applied may influence the results. Still models for regulation of plankton respiration is typically presented as being generally applicable, and ideally should be so, motivating validation in any environment at this stage. Field studies dominated the data set of 37 studies (Table S1) investigated, with only two microcosm studies and one mesocosm and model study each. Spatial and temporal scale of studies vary and could also influence models. Sampling varies from individual stations, via coastal transects to compilation of marine data across sea areas. Temporal scales from days to several years occur. 18 studies were done in estuarine environments, most of them in the boreal zone. Studies representative of oceanic environments amounts to 13. Temperature should have a similar basic effect in most environments, but likely influenced by the level of the ambient water temperature and type of organic matter present. This can be one cause of deviating precision of the models. Estuaries will be directly influenced by input of terrestrial organic matter from rivers with some bioavailable fraction and a large humic component. The influence of riverine discharge is lacking in identified models but shown as a major driver in a Baltic Sea estuary (Vikström et al., 2020). The autochthonous productivity in meso- and eutrophic environments appear to systematically increase plankton respiration, although continued increase (Duarte and Agusti, 1998; Williams and Le, 1998) or levelling off (Hopkinson and Smith, 2005) at higher productivity rates differ between studies.
Distinguishing between the effects of temperature and substrate supply is challenging. An increase in temperature (T) increases the probability of elements and molecules colliding and thereby the chemical reaction rate (k) at a given activation energy (Ea) for a chemical reaction:
where R is the molar gas constant and A is a collision frequency constant (Dickerson and Geis, 1979). Temperature thereby affects both enzyme catalysis of reactions by conformational change of the enzyme, and the chemical reactions of the creation and/or breakage of chemical bonds in or between the substrates. Consequently, organisms, especially bacterioplankton, should also experience elevated temperatures as an extended pool of available substrates. Higher temperature makes many chemical reactions more energetically favorable and thereby more substrates should be possible to use for metabolism. To better approach the issue of confounding predictors, we advocate that future research include multivariate collection of data accompanied by multivariate statistical analyses, including predictor influence measures and correlations. Reports where different predictors have been challenged in multiple stepwise regressions are scarce, and it is therefore not possible to assess whether higher coefficient of determination can be achieved using several predictors. Panigrahi et al. (2013) reported results from a multiple regression with temperature and chlorophyll-a, where only temperature remained once the data were adjusted for samples with oxygen oversaturation (>100%).
Riverine discharge of DOC drives a significant share of plankton respiration in the boreal northern Baltic Sea with high freshwater influence (r2 = 0.38, n=46) (Vikström et al., 2020). Riverine DOC exceeds the carbon supply from estuarine phytoplankton carbon fixation by 4-fold and explains part of the high plankton respiration levels during winter with negligible phytoplankton production (i.e., baseline respiration, del Giorgio and Williams, 2005). Other reports of significant plankton respiration at zero phytoplankton production prevail (Williams and Le, 1998; Du and Shen, 2015), although this was not further discussed by the authors. Thus, riverine DOC can supply coastal respiration with carbon substrates when discharges are sufficient and should be considered together with primary production in coastal areas.
Bacterioplankton respiration shows a coupling to bacterioplankton growth that is recommended as a preferred predictor (Robinson, 2008). This finding reflects that favorable growth conditions for bacterioplankton in general promote their respiration. When using bacterioplankton biomass growth as an environmental indicator, this can provide valuable information for managers on the trophic status and indicate the level and development of oxygen consumption. In this context, the observation that bacterioplankton respiration lacks a relationship to prokaryotic diversity is relevant (Reinthaler and Herndl, 2005) and is supported by other reports of functional redundancy in prokaryotic communities (Fernandez-Gonzalez et al., 2016). However, a recent report in contrary demonstrate significantly different respiration rates between lineages, but still a positive relationship to growth rate (Munson-McGee et al., 2022). For other questions such as climate change effects, external environmental predictors are of more value for projecting net outcomes for bacterioplankton respiration. As bacterioplankton growth estimates also have marked uncertainty and evaluated factors are log10-transformed, the predictive value of those equations for managing the marine environment is limited (Figure 3 and 4). In addition, uncertainty estimates of the derived coefficients are rarely provided in the covered literature regardless of the predictor involved, which precludes assessments of model precision.
Figure 4 Comparison of observed values for plankton respiration in a boreal estuary and modelled values based on indicated functions from the literature (Table 1). Boxplots show the median values, interquartile range (25-75%) and the 0-25% and 75-100% quartiles. Models based in discrete (A) or integrated values (B), respectively, are shown in separate panels.
Complicating the relationship between bacterioplankton respiration and growth, the contribution of maintenance respiration of prokaryotes is probably of a sufficient extent to influence plankton respiration regulation in the field (Carlson et al., 2007; Vikström and Wikner, 2019; Verma et al., 2022; Wikner and Vikström, 2023). This should also apply to other planktonic organisms (e.g., Norberg and DeAngelis, 1997) but is not discussed in a review of zooplankton respiration (Hernández-León and Ikeda, 2005). The influence of maintenance respiration should weaken the relationships with growth-related predictors. Temperature plausibly influences maintenance respiration as well, but other factors in the environment that regulate maintenance activities of organisms should also contribute. Activities such as maintaining osmotic potential inside cells requires energy metabolism via increased ATP synthesis. Nutrients (e.g., nitrogen and phosphorus) are also required for the synthesis of osmolytes, repair of nucleic acids, enzymes, and other cell constituents. However, as elements for maintenance metabolism can be recycled intracellularly and be independent of biomass increase, a share of pelagic respiration should be uncoupled from external nutrient supply. A better understanding of maintenance activities in plankton is recommended for future research to increase our knowledge of maintenance respiration regulation.
The ability of selected relationships to predict a three-year annual dataset of respiration rates from the boreal northern Baltic Sea at the four major seasons were tested (Table 1, Vikström et al., 2020, Figures S1-S13). The power of models to predict respiration were assessed by median and spread of values in boxplots and by root mean square errors between predicted and observed values (Figures 4, 5). Low values of RMSE indicate good predictive power (Sokal and Rohlf, 1995), while match between observed and modelled data sets regarding median and spread were assessed visually. All functions deviated from the observed values for plankton respiration at some season (Figure 4). Temperature and oxygen (Eq. 8) and temperature and carbon (Eq. 9) showed good match on both level and spread with observed values in autumn and summer, while being clearly lower than observed values in winter and spring. Also, net phytoplankton production (Eq. 6) and net primary production (Eq. 7) showed good match regarding the median, but generally larger spread than observed values (c.f., integrated values). Temperature alone (Eq. 3) matched the median well in winter and spring, while being markedly higher at other seasons. Noticeably, the other temperature equation (Eq. 5) was instead closer to observed values in summer and autumn, but lower in winter and spring, showing a correlation to Eq. 3 but on a markedly lower level. For discrete values, 4 of 5 models showed good match during summer while being more scattered other seasons. Gross primary production (Eq. 4) deviated most for integrated values, despite showing a high r2-value to data in the original article.
Figure 5 Same as Figure 4 but for bacterioplankton respiration.
The analysis of root mean square error (RMSE) encompassed all seasons (Table S2). Model Eq. 5, 8, 10, 9, and 3 showed low RMSE values in ascending order (3.3-4.0 µmol dm-3 d-1). All these coincided with models performing well in the box-plot analysis above. However, models with low RMSE were also associated with the highest number of cases tested (n>178 per model). For phytoplankton production, related tests could only include 51 cases, and the difference in n may have contributed to the different RMSE observed.
Even if many predicted distributions of plankton respiration remain within a factor of 2 relative to the observed values, substantial deviations occur that contribute to uncertainty in projecting the effects due to climate change and anthropogenic disturbance. Therefore, none of the tested equations could model the observed respiration rates in the northern Baltic Sea with sufficient accuracy and precision in all seasons. A statistically significant relationship between plankton respiration and temperature has previously been reported for the reference estuary (Panigrahi et al., 2013, Table S4). That relationship was based on a multiple regression using samples from 5 m depth (i.e., volumetric data), where chlorophyll-a, phytoplankton production and dissolved organic carbon was removed from the initial model. In a later study, including influence of riverine discharge of total organic carbon and phytoplankton production integrated over the water column, the sum of those factors (i.e., carbon input) was the only required predictor based on a multiple regression, (Vikström et al., 2020, their Figure 4). Water temperature, chlorophyll-a and dissolved organic carbon were removed from their initial model due to too low influence on plankton respiration. But also those relationships show a moderate predictive ability.
Modelling bacterioplankton respiration showed the best fit to the observed data for the predictors, DOC and bacterioplankton abundance in April but both had a markedly narrower range of values (Figure 5). The exception was bacterioplankton abundance reproducing the spread well in August, albeit at a higher median value. The observed bacterioplankton respiration rates came from another dataset from the same boreal estuary (Vikström and Wikner, 2019). DOC and bacterioplankton abundance were also the predictors showing the lowest RMSE values (Table S2). Significant maintenance respiration at low values of bacterioplankton growth (0.004-0.1 µmol dm-3 d-1) could possibly explain an underestimation by this model in the oligotrophic and comparably cold environment of the boreal estuary with high loads of terrigenous organic matter (Vikström and Wikner, 2019). The analysis of a global data set by (López-Urrutia and Morán, 2007) also find that bacterioplankton respiration is maintained at depleted trophic state while bacterial growth decrease, together reducing the growth efficiency. Taken together, the current models for bacterioplankton respiration also deviates from observed data regarding level, distribution, or both attributes. Consequently, uncertainty in current models precludes precise (< ± 20% CV) prediction of future bacterioplankton respiration in the tested water body.
Given that temperature is a commonly reported and a comparably strong predictor of respiration, current reports of the temperature sensitivity of plankton and bacterioplankton respiration were compiled (Table 2). The Q10 values for plankton respiration showed a median value of 3.2 (range 1.8-26). Variations occur between measurements within the same study and these are to some extent related to season (e.g., Panigrahi et al., 2013). Colder ambient temperatures tend to promote higher values but may also be explained by rates closer to the detection limits of current methods, where comparably small difference in untransformed units may mean multiple increases in rates. High inputs of riverine dissolved organic carbon together with low temperatures are also suggested to explain the high Q10 values in the northern Baltic Sea (Nydahl et al., 2013; Panigrahi et al., 2013). The DOC concentrations in the northern Baltic Sea are 5-fold higher than those in oceanic waters (Voss et al., 2021). Excess bioavailable carbon substrates should promote larger increases in respiration rates with increasing temperature. Plankton respiration seems to be more temperature sensitive than phytoplankton production and bacterioplankton growth (Lefevre et al., 1994; Vazquez-Dominguez et al., 2007; Panigrahi et al., 2013).
Reported assessment of climate change effects on oxygen consumption and concentrations in the current literature were compiled (Table 3). There is a consistent view in these reports that oxygen consumption will increase, and oxygen concentrations will decrease if the current climate projections for temperature are realized. This is in accordance with the evidence that temperature is a major predictor for respiration (Figure 3, Table S1, Figure 6). A literature survey of prokaryotic strains (n=239) and their thermal performance curve support an increase in prokaryotic respiration at higher temperature (Smith et al., 2019), but was mainly related to terrestrial taxa and lacked assessment of changes in substrate supply. Coupled physical-biogeochemical models driven by regionalized meteorological data from global climate simulations also show that oxygen consumption will increase in the Baltic Sea (Meier et al., 2011a). Both direct effect of temperature on plankton respiration and an increased supply of organic matter via higher primary production due to nutrient loads contribute to the increase. A mesocosm study from this sea area provides further support for increased plankton respiration and projects a reduction in the biological pump of organic matter to deeper water layers (Wohlers et al., 2009). However, that projection is based on that primary production increase is an outcome of climate change, while the net outcome of climate change for primary production varies with the marine environment. In contrast to the Baltic Sea scenario, a net 12-26% decrease in global primary production is projected when accounting for changes in nutrient availability (Couespel et al., 2021). Confidence in effect of climate change on primary production also influence reliability in projection of its effect on oxygen consumption.
Table 3 Compilation of the effects of climate change assessed in the literature on oxygen consumption and concentrations.
Figure 6 Conceptual diagram showing how plankton respiration (PR) is mainly controlled by temperature (T, red valve), and supply of bioavailable organic carbon (OC, yellow valve). OC is provided by excretion from grazing zooplankton and fish, phytoplankton and macroalgal exudation and detritus degradation. Coupling between respiration and biomass (BM, green valve, i.e., Chl-a) may reflect its importance for generating OC via zooplankton excretion and viral lysis. Supply of organic matter from riverine DOM discharge (ROC) can be significant in estuaries and contribute to formation of OC. Photolysis increase bioavailability of refractile humic substances. ROC drives basal respiration (PRb). Oxidation of NH4 to NO3 contribute with a smaller share in the marine environment. The significant contribution of maintenance respiration (PRm) to plankton respiration is also indicated.
In the northern Baltic Sea, primary production is also expected to decline but because of elevated precipitation and discharges of organic carbon (Andersson et al., 2015). In environments where precipitation is projected to increase due to climate change, terrestrial organic carbon, nitrogen and phosphorous discharged will also be elevated. Phytoplankton and macroalgae can use these elevated supplies of nitrogen and phosphorous for growth unless counteracted by reduced light penetration or global insolation (i.e., increased cloudiness). Reduced primary production under elevated freshwater discharges has been demonstrated on basin and multiannual scales in the northern Baltic Sea, explained by reduced light irradiance and intensified competition for limiting mineral nutrients (Wikner and Andersson, 2012). Terrestrial organic carbon from boreal watersheds is colored due to humic substances that hamper light penetration in the water column. Part of the terrestrial organic carbon can also be directly bioavailable to aquatic biota (20%, Pettersson et al., 1997) and should become more bioavailable by photolytic cleavage (Bertilsson et al., 1999), promoting bacterioplankton growth and respiration. Increased competition between bacterioplankton and phytoplankton for limited mineral nutrients will therefore further reduce primary production. The projected climate changes with warmer waters and higher precipitation in the northern Baltic Sea are consequently expected to enhance plankton respiration without an increase in primary production (Meier et al., 2011b; Andersson et al., 2015).
The expected effects of climate change in the Baltic Sea are in accordance with assessments conducted on the global scale. López-Urrutia and Morán (2007) conclude that global warming will lead to an increased flux of CO2 from the ocean to the atmosphere. Sarmento and Descy (2008) claim that our knowledge and experimental evidence make it probable that bacterioplankton respiration and losses to grazers will increase, making microorganisms even more dominant in a warmer ocean. Robinson (2008) also concluded that bacterioplankton and thus total respiration will increase with the projected temperature increases. Further support for this view is provided by Oschlies et al. (2018), who projected that warming of waters will not change the integrated oxygen loss but will shift the respiration of organic matter closer to the surface, reducing the oxygen concentration there.
Given the significance of temperature as a predictor, a tentative quantitative estimate of the temperature effect on plankton respiration can be calculated based on the reported temperature sensitivity (i.e., Q10-value, Table 2). Rearranging the Q10-function reported by (Sherr and Sherr, 1996) to
the effect of increased temperature (T2-T1) on increase in plankton respiration (R2/R1) can be calculated. Using the median Q10-value from Table 2 of 3.2 and the Representative Concentration Pathway (RCP) scenario 2.6 with projected 1°C increase in water temperature, a 12% increase in plankton respiration is projected (Table S3, IPCC, 2019). For the RCP8.5 scenario, leading to a 4°C increase in water temperature, the result is 59% increase in R. Those projections assume that the supply of organic carbon for fueling plankton respiration is not limiting this increase. Even a 12% increase in plankton respiration can cause hypoxia to develop over longer periods, or enhance current hypoxic waters to anoxia, assuming similar or reduced ventilation of the water column. Reduced oxygen concentration would influence many biogeochemical processes dependent on the redox potential with potential profound impact on the environmental status.
The authors above, however, simultaneously stress the uncertainties in current models regarding oxygen consumption and respiration. In line with the conclusion in this compilation, Robinson (2008) infers that the uncertainty in bacterioplankton respiration regulation is still too large to make meaningful projections. Additionally, Oschlies et al. (2018) state that the current understanding of oxygen consumption is insufficient for confident modelling of climate change effects. The literature thus concordantly stresses the paucity of knowledge regarding plankton respiration. The long-term projections mentioned above should therefore be viewed as educated guesses.
Ideally, a model for regulation of plankton respiration would apply to all water environments if accounting for major regulating factors properly. This justifies our investigation of models reported up to date on a well-studied boreal estuary. We conclude that current models do not predict plankton respiration sufficiently well but emphasizes that characteristics of the tested environment may influence this conclusion. Similar test with data from other water environments is advocated. To advance our knowledge of respiration in the sea, improved model performance across water environments can increase the confidence in future advice for management. The systematic measurements of oxygen consumption, primary production and temperature should be implemented in long-term ecological and monitoring programs covering several years, also advocated by Breitburg et al. (2018). Collaboration with biogeochemical modelers is advocated for experimental design. Selected representative high-frequency sampling sites should cover all seasons with at least monthly sampling. Here, multivariate statistics and time series analysis, possibly combined with artificial intelligence technique should be employed at local basin or water body scales, while avoiding the application of logarithm-transformed relationships. In estuaries, measurements of river discharge of organic carbon and nutrients is needed. Harmonization of methodology and terminology would facilitate cross-ecosystem syntheses. For future applications by environmental management agencies, simple and precise predictors and clear guidelines are needed and can be achieved by concerted efforts between environmental monitoring and research.
The original contributions presented in the study are included in the article/Supplementary Material. Further inquiries can be directed to the corresponding author.
JW, KV and AV designed the study and contributed to literature search. JW composed introductory and conceptual figures, made Table 1 and S1. AV synthesized degree of explanation and made corresponding figures. KV made modelling of selected equations based on available data sets and completed data into figures and tables. JW was the main author and KV and AV contributed in writing. All authors contributed to the article and approved the submitted version.
We are grateful for the research environment and funding provided by the strategic research program, EcoChange (224-919-09, JW). Financial support was further provided by the Kempe foundations (SMK-1854) to JW. Open access fee was funded by Umeå University within the Sweden Open Access Publishing Framework Agreement.
We acknowledge Marlene Lahti at the Communication Office, Umeå University, for refining figure 1 and 6.
The authors declare that the research was conducted in the absence of any commercial or financial relationships that could be construed as a potential conflict of interest.
All claims expressed in this article are solely those of the authors and do not necessarily represent those of their affiliated organizations, or those of the publisher, the editors and the reviewers. Any product that may be evaluated in this article, or claim that may be made by its manufacturer, is not guaranteed or endorsed by the publisher.
The Supplementary Material for this article can be found online at: https://www.frontiersin.org/articles/10.3389/fmars.2023.1134699/full#supplementary-material
Alonso-Saez L., Vazquez-Dominguez E., Cardelus C., Pinhassi J., Sala M. M., Lekunberri I., et al. (2008). Factors controlling the year-round variability in carbon flux through bacteria in a coastal marine system. Ecosystems 11 (3), 397–409. doi: 10.1007/s10021-008-9129-0
Andersson A., Meier H. E. M., Ripszam M., Rowe O., Wikner J., Haglund P., et al. (2015). Projected future climate change and Baltic Sea ecosystem management. Ambio 44 (3), 345–356. doi: 10.1007/s13280-015-0654-8
Azam F., Malfatti F. (2007). Microbial structure of marine ecosystems. Nat. Rev. 5 (10), 782–791. doi: 10.1038/nrmicro1747
Bach L. T., Stange P., Taucher J., Achterberg E. P., Alguero-Muniz M., Horn H., et al. (2019). The influence of plankton community structure on sinking velocity and remineralization rate of marine aggregates. Global Biogeochemical Cycles 33 (8), 971–994. doi: 10.1029/2019gb006256
Belcher A., Iversen M., Manno C., Henson S. A., Tarling G. A., Sanders R. (2016). The role of particle associated microbes in remineralization of fecal pellets in the upper mesopelagic of the Scotia Sea, Antarctica. Limnology Oceanography 61 (3), 1049–1064. doi: 10.1002/lno.10269
Bendtsen J., Hansen J. L. S. (2013). Effects of global warming on hypoxia in the Baltic Sea-north Sea transition zone. Ecol. Model. 264, 17–26. doi: 10.1016/j.ecolmodel.2012.06.018
Bertilsson S., Stepanauskas R., Cuadros-Hansson R., Graneli W., Wikner J., Tranvik L. (1999). Photochemically induced changes in bioavailable carbon and nitrogen pools in a boreal watershed. Aquat. Microbial Ecol. 19 (1), 47–56. doi: 10.3354/ame019047
Breitburg D., Levin L. A., Oschlies A., Gregoire M., Chavez F. P., Conley D. J., et al. (2018). Declining oxygen in the global ocean and coastal waters. Science 359 (6371), 46–4+. doi: 10.1126/science.aam7240
Carlson C. A., del Giorgio P. A., Herndl G. J. (2007). Microbes and the dissipation of energy and respiration: From cells to ecosystems. Oceanography 20 (2), 89–100. doi: 10.5670/oceanog.2007.52
Carstensen J., Andersen J. H., Gustafsson B. G., Conley D. J. (2014). Deoxygenation of the Baltic Sea during the last century. Proc. Natl. Acad. Sci. United States America 111 (15), 5628–5633. doi: 10.1073/pnas.1323156111
Cho B. C., Azam F. (1988). Major role of bacteria in biogeochemical fluxes in the ocean’s interior. Nature 332, 441–443. doi: 10.1038/332441a0
Couespel D. , Levy M., Bopp L. (2021). Oceanic primary production decline halved in eddy-resolving simulations of global warming. Biogeosciences 18(14), 4321–4349. doi: 10.5194/bg-18-4321-2021
Dang H. Y. (2020). Grand challenges in microbe-driven marine carbon cycling research. Front. Microbiol. 11. doi: 10.3389/fmicb.2020.01039
del Giorgio P. A., Duarte C. M. (2002). Respiration in the open ocean. Nature 420 (6914), 379–384. doi: 10.1038/nature01165
del Giorgio P. A., Williams P. (2005). “The global significance of respiration in aquatic ecosystems: from single cells to the biosphere,” in Respiration in aquatic ecosystems. Eds. del Giorgio P. A., Williams P. J., Le B. (Oxford: Oxford University Press), 267–303.
Diaz R., Rosenberg R. (2008). Spreading dead zones and consequences for marine ecosystems. Science 321), 926–929. doi: 10.1126/science.1156401
Dickerson R. E., Geis I. (1979). Chemistry, matter and the universe (menlo Park, California: Benjamin/Cummings).
Du J. B., Shen J. (2015). Decoupling the influence of biological and physical processes on the dissolved oxygen in the Chesapeake bay. J. Geophysical Research-Oceans 120 (1), 78–93. doi: 10.1002/2014jc010422
Duarte C. M., Agusti S. (1998). The CO2 balance of unproductive aquatic ecosystems. Science 281 (5374), 234–236. doi: 10.1126/science.281.5374.234
Eilola K., Meier H. E. M., Almroth E. (2009). On the dynamics of oxygen, phosphorus and cyanobacteria in the Baltic sea; a model study. J. Mar. Syst. 75 (1), 163–184. doi: 10.1016/j.jmarsys.2008.08.009
Fender C. K., Kelly T. B., Guidi L., Ohman M. D., Smith M. C., Stukel M. R. (2019). Investigating particle size-flux relationships and the biological pump across a range of plankton ecosystem states from coastal to oligotrophic. Front. Mar. Sci. 6. doi: 10.3389/fmars.2019.00603
Fernandez-Gonzalez N., Huber J. A., Vallino J. J. (2016). Microbial communities are well adapted to disturbances in energy input. Msystems 1 (5). doi: 10.1128/mSystems.00117-16
Forsgren G., Jansson M. (1992). The turnover of river-transported iron, phosphorus and organic carbon in the Öre estuary, northern Sweden. Hydrobiologia 235/236, 585–596. doi: 10.1007/BF00026246
Giering S. L. C., Cavan E. L., Basedow S. L., Briggs N., Burd A. B., Darroch L. J., et al. (2020). Sinking organic particles in the ocean-flux estimates from in situ optical devices. Front. Mar. Sci. 6. doi: 10.3389/fmars.2019.00834
Henson S. A., Sanders R., Madsen E., Morris P. J., Le Moigne F., Quartly G. D. (2011). A reduced estimate of the strength of the ocean’s biological carbon pump. Geophysical Res. Lett. 38:1–5. doi: 10.1029/2011gl046735
Hernández-León S., Ikeda T. (2005). “Zooplankton respiration,” in Respiration in aquatic ecosystems. Eds. Giorgio P. A. d., Williams P. J., Le B. (Oxford: Oxford University Press), 57–82.
Hopkinson C. S., Smith E. M. (2005). “Estuarine respiration: an overview of benthic, pelagic and whole system respiration,” in Respiration in aquatic ecosystems. Eds. Giorgio P. A. d., Williams P. J., Le B. (New York: Oxford University Press Inc), 122–146.
Hoppe H.-G., Breithaupt P., Wahlter K., Koppe R., Bleck S., Sommer U., et al. (2008). Climate warming in winter affects the coupling between phytoplankton and bacteria during the spring bloom: a mesocosm study. Aquat. Microbial Ecol. 51, 105–115. doi: 10.3354/ame01198
IPCC (2019). “Summary for policymakers,” in IPCC special report on the ocean and cryosphere in a changing climate. Eds. Pörtner H.-O., Roberts M.-D. D C, Zhai P. V., Tignor M., Poloczanska E., Mintenbeck K., Nicolai M., Okem A., Petzold J., Rama B., Weyer N.(Cambridge, UK and New York, NY, USA: Cambridge University Press).
Jiao N., Herndl G. J., Hansell D. A., Benner R., Kattner G., Wilhelm S. W., et al. (2010). Microbial production of recalcitrant dissolved organic matter: long-term carbon storage in the global ocean. Nat. Rev. Microbiol. 8 (8), 593–599. doi: 10.1038/nrmicro2386
Lefevre D., Bentley T. L., Robinson C., Blight S. P., Williams P. J. L. (1994). The temperature response of gross and net community production and respiration in time-varying assemblages of temperate marine micro-plankton. J. Exp. Mar. Biol. Ecol. 184 (2), 201–215. doi: 10.1016/0022-0981(94)90005-1
Lemée R., Rochelle-Newall E., Van Wambeke F., Pizay M. D., Rinaldi P., Gattuso J. P. (2002). Seasonal variation of bacterial production, respiration and growth efficiency in the open NW Mediterranean Sea. Aquat. Microbial Ecol. 29 (3), 227–237. doi: 10.3354/ame029227
Lomas M. W., Glibert P. M., Shiah F. K., Smith E. M. (2002). Microbial processes and temperature in Chesapeake bay: current relationships and potential impacts of regional warming. Global Change Biol. 8 (1), 51–70. doi: 10.1046/j.1365-2486.2002.00454.x
López-Urrutia A., Morán X. A. G. (2007). Resource limitation of bacterial production distorts the temperature dependence of oceanic carbon cycling. Ecology 88 (4), 817–822. doi: 10.1890/06-1641
Lopez-Urrutia A., San Martin E., Harris R. P., Irigoien X. (2006). Scaling the metabolic balance of the oceans. Proc. Natl. Acad. Sci. United States America 103 (23), 8739–8744. doi: 10.1073/pnas.0601137103
Mazuecos I. P., Aristegui J., Vazquez-Dominguez E., Ortega-Retuerta E., Gasol J. M., Reche I. (2015). Temperature control of microbial respiration and growth efficiency in the mesopelagic zone of the south Atlantic and Indian oceans. Deep-Sea Res. Part I-Oceanographic Res. Papers 95, 131–138. doi: 10.1016/j.dsr.2014.10.014
Meier H. E. M., Andersson H. C., Eilola K., Gustafsson B. G., Kuznetsov I., Muller-Karulis B., et al. (2011a). Hypoxia in future climates: A model ensemble study for the Baltic Sea. Geophysical Res. Lett. 38. doi: 10.1029/2011gl049929
Meier H. E. M., Eilola K., Almroth E. (2011b). Climate-related changes in marine ecosystems simulated with a 3-dimensional coupled physical-biogeochemical model of the Baltic Sea. Climate Res. 48 (1), 31–55. doi: 10.3354/cr00968
Munson-McGee J. H., Lindsay M. R., Sintes E., Brown J. M., D’Angelo T., Brown J., et al. (2022). Decoupling of respiration rates and abundance in marine prokaryoplankton. Nature 612, 764–770. doi: 10.1038/s41586-022-05505-3
Neumann T., Fennel W., Kremp C. (2002). Experimental simulations with an ecosystem model of the Baltic Sea: A nutrient load reduction experiment. Global Biogeochemical Cycles 16 (3). doi: 10.1029/2001gb001450
Norberg J., DeAngelis D. (1997). Temperature effects on stocks and stability of a phytoplankton-zooplankton model and the dependence on light and nutrients. Ecol. Model. 95 (1), 75–86. doi: 10.1016/s0304-3800(96)00033-6
Nydahl A., Panigrahi S., Wikner J. (2013). Increased microbial activity in a warmer and wetter climate enhances the risk of coastal hypoxia. FEMS Microbiol. Ecol. 85 (2), 338–347. doi: 10.1111/1574-6941.12123
Olesen M., Lundsgaard C., Andrushaitis A. (1999). Influence of nutrients and mixing on the primary production and community respiration in the gulf of Riga. J. Mar. Syst. 23 (1-3), 127–143. doi: 10.1016/S0924-7963(99)00054-8
Oschlies A., Brandt P., Stramma L., Schmidtko S. (2018). Drivers and mechanisms of ocean deoxygenation. Nat. Geosci. 11 (7), 467–473. doi: 10.1038/s41561-018-0152-2
Pan Y., Birdsey R. A., Fang J., Houghton R., Kauppi P. E., Kurz W. A., et al. (2011). A Large and persistent carbon sink in the worlds forests. Science 333 (6045), 988–993. doi: 10.1126/science.1201609
Panigrahi S., Nydahl A., Anton P., Wikner J. (2013). Strong seasonal effect of moderate experimental warming on plankton respiration in a temperate estuarine plankton community. Estuar. Coast. Shelf Sci. 135, 269–279. doi: 10.1016/j.ecss.2013.10.029
Pettersson C., Allard B., Boren H. (1997). River discharge of humic substances and humic-bound metals to the gulf of bothnia. Estuar. Coast. Shelf Sci. 44 (5), 533–541. doi: 10.1006/ecss.1996.0159
Pomeroy L. R., Wiebe W. J. (2001). Temperature and substrates as interactive limiting factors for marine heterotrophic bacteria. Aquat. Microbial Ecol. 23 (2), 187–204. doi: 10.3354/ame023187
Pomeroy L. R., Wiebe W. J., Deibel D., Thompson R. J., Rowe G. T., Pakulski J. D. (1991). Bacterial responses to temperature and substrate concentration during the new foundland spring bloom. Mar. Ecology-Progress Ser. 75 (2-3), 143–159. doi: 10.3354/meps075143
R_Core_Team (2021). R: A language and environment for statistical computing (Vienna, Austria: R Foundation for Statistical Computing).
Rabalais N. N., Diaz R. J., Levin L. A., Turner R. E., Gilbert D., Zhang J. (2010). Dynamics and distribution of natural and human-caused hypoxia. Biogeosciences 7 (2), 585–619. doi: 10.5194/bg-7-585-2010
Reader H. E., Stedmon C. A., Kritzberg E. S. (2014). Seasonal contribution of terrestrial organic matter and biological oxygen demand to the Baltic Sea from three contrasting river catchments. Biogeosciences 11 (12), 3409–3419. doi: 10.5194/bg-11-3409-2014
Reinthaler T., Herndl G. J. (2005). Seasonal dynamics of bacterial growth efficiencies in relation to phytoplankton in the southern north Sea. Aquat. Microbial Ecol. 39 (1), 7–16. doi: 10.3354/ame039007
Rivkin R. B., Legendre L. (2001). Biogenic carbon cycling in the upper ocean: Effects of microbial respiration. Science 291 (5512), 2398–2400. doi: 10.1126/science.291.5512.2398
Robinson C. (2000). Plankton gross production and respiration in the shallow water hydrothermal systems of miles, Aegean Sea. J. Plankton Res. 22 (5), 887–906. doi: 10.1093/plankt/22.5.887
Robinson C. (2008). “Heterotrophic bacterial respiration,” in Microbial ecology of the oceans, 2nd ed. Ed. Kirchman D. L. (Hoboken: Johan Wiley & Sons, Inc).
Robinson C. (2019). Microbial respiration, the engine of ocean deoxygenation. Front. Mar. Sci. 5. doi: 10.3389/fmars.2018.00533
Robinson C., Williams P. J. L. (1993). Temperature and antarctic plankton community respiration. J. Plankt. Res. 15 (9), 1035–1051. doi: 10.1093/plankt/15.9.1035
Robinson C., Williams P. J., Le B. (2005). “Respiration and it´s measurement in surface marine waters,” in Respiration in aquatic ecosystems. Eds. del Giorgio P. A., Williams P. J., Le B. (Oxford: Oxford University Press), 147–180.
Sandberg J., Andersson A., Johansson S., Wikner J. (2004). Pelagic food web and carbon budget in the northern Baltic Sea: potential importance of terrigenous carbon. Mar. Ecology-Progress Ser. 268, 13–29. doi: 10.3354/meps268013
Sanders R. J., Henson S. A., Martin A. P., Anderson T. R., Bernardello R., Enderlein P., et al. (2016). Controls over ocean mesopelagic interior carbon storage (COMICS): Fieldwork, synthesis, and modeling efforts. Front. Mar. Sci. 3. doi: 10.3389/fmars.2016.00136
Sarmento H., Descy J. P. (2008). Use of marker pigments and functional groups for assessing the status of phytoplankton assemblages in lakes. J. Appl. Phycology 20 (6), 1001–1011. doi: 10.1007/s10811-007-9294-0
Sarmento H., Montoya J. M., Vazquez-Dominguez E., Vaque D., Gasol J. M. (2010). Warming effects on marine microbial food web processes: how far can we go when it comes to predictions? Philos. Trans. R. Soc. B-Biological Sci. 365 (1549), 2137–2149. doi: 10.1098/rstb.2010.0045
Savchuk O. P. (2002). Nutrient biogeochemical cycles in the gulf of Riga: scaling up field studies with a mathematical model. J. Mar. Syst. 32 (4), 253–280. doi: 10.1016/s0924-7963(02)00039-8
Sherr E. B., Sherr B. F. (1996). Temporal offset in oceanic production and respiration processes implied by seasonal changes in atmospheric oxygen: the role of heterotrophic microbes. Aquat. Microb. Ecol. 11, 91–100. doi: 10.3354/ame011091
Smith E. M., Kemp W. M. (1995). Seasonal and regional variations in plankton community production and respiration for Chesapeake bay. Mar. Ecol. Prog. Ser. 116, 217–231f. doi: 10.3354/meps116217
Smith E. M., Kemp W. M. (2001). Size structure and the production/respiration balance in a coastal plankton community. Limnology Oceanography 46 (3), 473–485. doi: 10.4319/lo.2001.46.3.0473
Smith E. M., Kemp W. M. (2003). Planktonic and bacterial respiration along an estuarine gradient: responses to carbon and nutrient enrichment. Aquat. Microbial Ecol. 30 (3), 251–261. doi: 10.3354/ame030251
Smith T. P., Thomas T. J. H., García-Carreras B., Sal S., Yvon-Durocher G., Bell T., et al. (2019). Community-level respiration of prokaryotic microbes may rise with global warming. Nat. Commun. 10 (1) 5124. doi: 10.1038/s41467-019-13109-1
Vazquez-Dominguez E., Vaque D., Gasol A. M. (2007). Ocean warming enhances respiration and carbon demand of coastal microbial plankton. Global Change Biol. 13 (7), 1327–1334. doi: 10.1111/j.1365-2486.2007.01377.x
Verma A., Amnebrink D., Pinhassi J., Wikner J. (2022). Prokaryotic maintenance respiration and growth efficiency field patterns reproduced by temperature and nutrient control at mesocosm scale. Environ. Microbiol., 1–17. doi: 10.1111/1462-2920.16300
Vikström K., Bartl I., Karlsson J., Wikner J. (2020). Strong influence of baseline respiration in an oligotrophic coastal ecosystem. Front. Mar. Sci. 7 (839). doi: 10.3389/fmars.2020.572070
Vikström K., Wikner J. (2019). Importance of bacterial maintenance respiration in a subarctic estuary: a proof of concept from the field. Microbial Ecol. 77 (3), 574–586. doi: 10.1007/s00248-018-1244-7
Vosjan J. H., Olanczukneyman K. M. (1991). Influence of temperature on respratory ETS-activity of microorganisms from admirality bay, king George island, Antarctica. Netherlands J. Sea Res. 28 (3), 221–225. doi: 10.1016/0077-7579(91)90019-W
Voss M., Asmala E., Bartl I., Carstensen J., Conley D. J., Dippner J. W., et al. (2021). Origin and fate of dissolved organic matter in four shallow Baltic Sea estuaries. Biogeochemistry 154 (2), 385–403. doi: 10.1007/s10533-020-00703-5
White P. A., Kalff J., Rasmussen J. B., Gasol J. M. (1991). The effect of temperature and algal biomass on bacterial production and specific growth rate in freshwater and marine habitats. Microb. Ecol. 21, 99–118. doi: 10.1007/BF02539147
Wikner J., Andersson A. (2012). Increased freshwater discharge shifts the trophic balance in the coastal zone of the northern Baltic Sea. Global Change Biol. 18 (8), 2509–2519. doi: 10.1111/j.1365-2486.2012.02718.x
Wikner J and K., Vikström K. (2023). Extensive prokaryotic maintenance respiration in the sea influenced by osmoregulation. Frontiers in Marine Science, in press.
Williams P. J., Le B. (1998). The balance of plankton respiration and photosynthesis in the open oceans. Nature 394 (6688), 55–57. doi: 10.1038/27878
Williams P. J., Le B., del Giorgio P. A. (2005). Respiration in aquatic ecosystems (Oxford: Oxford University Press).
Williams P. J., Le B., Morris P. J., Karl D. M. (2004). Net community production and metabolic balance at the oligotrophic ocean site: Station ALOHA. Deep-Sea Res. Part I 51 (1), 1563–1578. doi: 10.1016/j.dsr.2004.07.001
Wohlers J., Engel A., Zollner E., Breithaupt P., Jurgens K., Hoppe H. G., et al. (2009). Changes in biogenic carbon flow in response to sea surface warming. Proc. Natl. Acad. Sci. United States America 106 (17), 7067–7072. doi: 10.1073/pnas.0812743106
Keywords: climate, effect, plankton, oxygen, respiration, regulation, temperature, carbon
Citation: Wikner J, Vikström K and Verma A (2023) Regulation of marine plankton respiration: A test of models. Front. Mar. Sci. 10:1134699. doi: 10.3389/fmars.2023.1134699
Received: 30 December 2022; Accepted: 28 February 2023;
Published: 15 March 2023.
Edited by:
Anke Kremp, Leibniz Institute for Baltic Sea Research (LG), GermanyReviewed by:
Liyang Yang, Fuzhou University, ChinaCopyright © 2023 Wikner, Vikström and Verma. This is an open-access article distributed under the terms of the Creative Commons Attribution License (CC BY). The use, distribution or reproduction in other forums is permitted, provided the original author(s) and the copyright owner(s) are credited and that the original publication in this journal is cited, in accordance with accepted academic practice. No use, distribution or reproduction is permitted which does not comply with these terms.
*Correspondence: Johan Wikner, am9oYW4ud2lrbmVyQHVtdS5zZQ==
Disclaimer: All claims expressed in this article are solely those of the authors and do not necessarily represent those of their affiliated organizations, or those of the publisher, the editors and the reviewers. Any product that may be evaluated in this article or claim that may be made by its manufacturer is not guaranteed or endorsed by the publisher.
Research integrity at Frontiers
Learn more about the work of our research integrity team to safeguard the quality of each article we publish.