- 1Hubei Key Laboratory of Regional Development and Environmental Response, Faculty of Resource and Environment, Hubei University, Wuhan, China
- 2Institute of Hydrobiology, Chinese Academy of Sciences (CAS), Wuhan, China
Understanding the link between biodiversity and ecosystem functioning is imperative for ecosystem-based management. The role of multitrophic diversity in sustaining multifunctionality remains unclear, especially in highly diverse aquatic ecosystems. We performed a species-addition experiment consisting of one, two, three, and five species in simulated multitrophic ecosystems to evaluate biodiversity and ecosystem functioning relationships within and across trophic levels. Our results showed that there are positive species richness–productivity relationships within and across trophic levels. We found significant negative correlations between species richness and the cumulative variation of total phosphorus, and between species richness and ecosystem multifunctionality across trophic levels. Also, we found that the relationships between ecosystem multifunctionality and species richness within and across the trophic levels are mediated by a combination of environmental factors, including water temperature, dissolved oxygen, pH, irradiance, and time, rather than by species richness. Our results imply that species richness–ecosystem functioning relationships vary for different ecological functions; the individual ecosystem functions selected and the way multifunctionality calculated are critical when examining links between biodiversity and ecosystem multifunctionality. Our study highlights that multitrophic richness, such as for consumers, is crucial for driving ecosystem multifunctionality. Furthermore, our study implies that management practices for restoring the diversity of aquatic macrophytes in wetlands should consider not only macrophyte richness but also different functional groups and life-forms.
1 Introduction
Biodiversity–ecosystem function (BEF) relationships are a common focus in ecological research programs (Jaillard et al., 2014; van der Plas, 2019). The detrimental effects of human-induced biodiversity loss on ecosystem functions (EFs) have received extensive attention (Jaillard et al., 2014; Schuldt et al., 2018). Early BEF relationship research mostly focused on the relationship between species richness and individual EFs, particularly for productivity and biomass, and revealed that biodiversity–productivity relationships increase monotonically (Zavaleta et al., 2010; Jaillard et al., 2014; Slade et al., 2017). However, other studies have identified other types of biodiversity–productivity relationships, including positive hump-shaped, negative U-shaped, monotonically decreasing, and no apparent relationship (Mittelbach et al., 2001; Hooper et al., 2005; Duffy, 2009; Jaillard et al., 2014). Other researchers concluded that biodiversity effects on EFs seem to be consistent across different groups of organisms, among trophic levels, and across various ecosystems (Cardinale et al., 2012). These inconsistent results therefore suggest that biodiversity–productivity relationships vary depending on the ecosystem (Jaillard et al., 2014).
Most evidence for BEF relationships is from terrestrial systems, like grasslands, forests, and agricultural ecosystems (Bai et al., 2007; Messmer et al., 2014; Gagic et al., 2015; Brooker et al., 2021), whereas freshwater ecosystems have received less attention (Yasuhara et al., 2016). Some studies showed ambivalent evidence of whether BEF relationships attained from terrestrial ecosystems can be extrapolated to aquatic realms (Vaughn, 2010; Duncan et al., 2015; Gamfeldt et al., 2015; Strong et al., 2015; Daam et al., 2019). Freshwater ecosystems are among the most threatened ecosystems in the world (Scheffer, 2004; Davidson et al., 2013; Zhang et al., 2019), therefore, there is an urgent need for assessing BEF relationships in freshwater ecosystems to determine whether they follow the general significance and direction of BEF relationships (Duncan et al., 2015).
Some studies have examined the links between biodiversity and ecosystem functioning (EF) in the freshwater realm, such as the links between macrophyte species richness and biomass and water quality (Engelhardt and Ritchie, 2001; Zhang et al., 2019; Hu et al., 2022), as well as links between benthic macroinvertebrates, the microbial community, and nutrient cycling (Cao et al., 2018; Zhang et al., 2021). These studies showed that the shapes of BEF relationships vary based on the taxonomic group considered, the nature of multi-trophic diversity interactions, and the type of biodiversity indicators selected (Daam et al., 2019). Like previous BEF research in terrestrial ecosystems, studies on BEF in freshwater ecosystems has focused on single trophic levels and individual EFs, but studies indicate that biodiversity affects multiple EFs simultaneously (Jing et al., 2015; Lefcheck et al., 2015; Zhang et al., 2021). Thus, considering an individual EF may underestimate the importance of biodiversity (Lefcheck et al., 2015; Garland et al., 2021), ignore the synergies or trade-offs between different EFs (Hector and Bagchi, 2007; Fu and Yu, 2016), and mask that the functional role of any trophic group may depend on the diversity of others (Jing et al., 2015).
Ecosystems are composed of multiple trophic levels and multitrophic communities are especially important in maintaining multiple EFs (Brose and Hillebrand, 2016; Wang and Brose, 2017; Anujan et al., 2021). Studies of single trophic levels are insufficient to understand the functional consequences of biodiversity decline (Gamfeldt et al., 2015; Lefcheck et al., 2015) because different trophic groups may have complementary (Eisenhauer et al., 2013; Ebeling et al., 2018) or opposite effects on EFs (Duffy et al., 2007). Additionally, some recent studies have shown that the strength of biodiversity effects on EFs can increase over time (Xu et al., 2021). Understanding how biodiversity affects ecosystem multifunctionality (EMF) requires analysis of the diversity within (horizontal diversity) and across trophic levels (vertical diversity) over time (Duffy et al., 2007; Xu et al., 2021). Compared with terrestrial and marine ecosystems, quantitative information about the effect of multitrophic levels and time on biodiversity–ecosystem multifunctionality (BEMF) relationships are largely lacking for freshwater ecosystems (Duffy et al., 2007; Daam et al., 2019). There is thus an urgent need for strengthening this research on BEMF relationships in freshwater ecosystems to fill current research gaps (Guy-Haim et al., 2018).
Due to human activities and global changes, the biodiversity crisis of freshwater ecosystems is deepening, affecting ecosystem services (Zhang et al., 2019). Increasing evidence has shown that freshwater biodiversity loss can cause serious impacts on EFs and may threaten human welfare (Scheffer et al., 2001; Jackson et al., 2016; Xu et al., 2016; Janssen et al., 2021; Losapio et al., 2021). Assessing BEMF relationships within and across trophic levels can allow prediction of the impacts of biodiversity change on the provisioning of ecosystem services (Duncan et al., 2015). We constructed a species-addition microcosm experiment with a diversity gradient (one, two, or three macrophyte species, and three macrophyte species with two aquatic animals) to investigate if (1) the effects of species richness on individual EF and EMF differed significantly among, within, and across trophic levels and, (2) there was a positive diversity–ecosystem stability relationship within and across trophic levels over time.
2 Materials and methods
2.1 Materials and experimental set-up
Three common submerged macrophytes and two common aquatic animals, Hydrilla verticillata, Vallisneria natans, Potamogeton maackianus, Cyprinus carpio, and Cipangopaludina cahayensis were chosen to evaluate the effects of species diversity within and across trophic levels on EMF. H.verticillata, V. natans, and P. maackianus are common macrophytes in the middle-lower reach of the Yangtze River, and they often can grow together and form a stable aquatic plant community. They were collected from Shahu Lake, a eutrophic lake near Hubei University, the proximity facilitating transport. The plants were then washed with running tap water to remove particles and other adjacent organisms. Cyprinus carpio that were 7.78 ± 0.80 cm (n = 24) in length and 4.93 ± 1.95 g (n = 24) in weight, and Cipangopaludina cahayensis were 5.68 ± 0.40 g (n = 12) in weight, were brought from Chongqing Yongchuan Yuxi aquaculture base. The protocols involving animals were reviewed and approved by the Animal Ethics and Welfare Committee of Hubei University. Before the experiment, the three macrophytes and the two aquatic animals were cultivated in aquariums with tap water in the greenhouse; the temperature varied from 12.5 °C to 30.4 °C and the average temperature was 23.1 ± 6.1 °C during the experiment.
A species-addition experiment, consisting of twelve 110 L polyethylene buckets (diameter = 0.4 m, height = 0.65 m), was carried out from August 20 to November 20, 2021. The buckets were placed in the experimental greenhouse of Hubei University and filled with tap water which had total nitrogen (TN) and total phosphorus (TP) concentrations of 1.8 and 0.02 mg/L, respectively, and a water depth of 47 cm. The buckets were randomly divided into four treatments, each replicated three times, including H. verticillata monoculture (Mon), H. verticillata + V. natans mixed culture (Mixtwo), H. verticillata + V. natans + P. maackianus mixed culture (Mixthree), and H. verticillata + V. natans + P. maackianus + C. carpio + C. cahayensis mixed culture (Mixfive). During the experiment, the water temperature varied from 10.7 °C to 28.6 °C, the average water temperature was 21.3 ± 6.0 °C, and the pH of the water varied from 8.3 to 9.9.
Before the experiment, H. verticillata, V. natans, and P. maackianus were washed thoroughly with tap water followed by deionized water, and the lateral branches or stolons of the plants were removed. Then, complete clean plants of V. natans (10 cm length) and apices of H. verticillata and P. maackianus cut at 10 cm below the top were prepared and planted in small plastic pots (length 18 cm and width 12 cm), which were filled with 5 cm fine sand. Fourteen plants were planted in each plastic pot. The density of H. verticillata was 14 in monoculture, the densities of H. verticillata and V. natans were eight and six in Mixtwo, and the densities of H. verticillata, V. natans, and P. maackianus were eight, three, and three in Mixthree in each pot, respectively. In total, 168 young seedlings of similar size were transplanted into 12 small plastic pots, which included three H. verticillata monoculture pots, three H. verticillata + V. natans mixed culture pots, and six H. verticillata + V. natans + P. maackianus mixed culture pots. Then, 12 small plastic pots were placed randomly into the 12 polyethylene buckets. At the same time, three polyethylene buckets of the H. verticillata + V. natans + P. maackianus mixed cultures and two C. carpio and three C. cahayensis were randomly added to each polyethylene bucket. We used a line to lift and lower the pots, which allowed gentle and nondestructive sampling. During the experiment, deionized water was replenished every 2 days to compensate for evaporation losses and assure that the water levels were similar in all 12 polyethylene buckets. Also, dead C. carpio were replaced with live ones of approximately similar size.
2.2 Sampling and chemical analyses
At the beginning of the experiment, the height/length and fresh weight of all 168 macrophytes were measured with a ruler and an analysis balance. Macrophytes were measured on days 14, 28, 42, 56, and 70 after the start of the experiment. This was done by pulling up the tray on which the plant pots were standing, making handling and measuring easy and non-destructive. The height and length of each branch were measured with a ruler. After each measurement (except the last time), we put the macrophytes back into the corresponding polyethylene bucket. At the end of the experiment, all macrophytes were harvested and weighed, and the biomass of phytoplankton in the water column was proxied by total chlorophyll. Chlorophyll content was measured according to the method described by Jampeetong and Brix (2009). Filamentous macroalgae growing on the macrophytes or at the water surface were collected by hand, and epiphyton growing on the bucket wall and on the sand surface was collected by brush and then weighed.
The macrophyte biomasses of different sampling times were estimated by the fitted linear relationship between plant height and biomass using the data of height and biomass measured at the beginning of the experiment and at 70 days. The linear fitting equations for the three tested macrophytes were as follows:
where x is the plant height in cm and y is the plant biomass in g.
The water temperature, dissolved oxygen content and pH, total nitrogen (TN), and total phosphorus (TP) concentration of each polyethylene bucket were measured at the beginning of the experiment and on days 14, 28, 42, 56, and 70. The water temperature, dissolved oxygen content, and pH of the water bodies were measured at 30 cm depth between 10:30 and 12:00 using a YSI (YSI 6600V2). The irradiance was measured by an illuminance meter (MODEL ZDS-10W-2D). TP content was measured using a colorimeter, an AutoAnalyzer (Bran+Luebbe GmbH, Inc., Germany), following sulfuric acid/hydrogen peroxide digestion and the ammonium molybdate ascorbic acid method. TN content was measured using an IL-500N nitrogen analyzer (Hach Company, Loveland, USA). During the experiment, different volumes of NH4CL and KH2PO4 were added every 14 days to maintain 1.8 mg/L TN and 0.02 mg/L TP concentration in the water column in each bucket.
2.3 Statistical analysis
Three ecological functions, including total net primary productivity (the total biomass of macrophyte, phytoplankton, filamentous macroalgae, and epiphyton) and cumulative variation of TN and TP, at the end of the experiment in each polyethylene bucket, were used to quantify EMF.
The cumulative variation of TN and TP was calculated as:
where CTN(TP) represents the cumulative variation of TN or TP, Ci represents the TN or TP content of the water measured at different sampling periods, and C0 represents the value of the TN or TP content at the beginning of the experiment.
According to Maestre et al. (2012), we used the mean value method to quantify EMF because it simply and intuitively reflects the ability of communities to maintain EMF (Jing et al., 2015). We calculated the average of standardized data of the total net primary productivity and TN and TP cumulative variation. The multifunctionality index (M) for each polyethylene bucket was the average of the three evaluated functions M can be calculated according to the method used by Xu et al. (2016):
where F represents the number of functions being measured, fi represents the measures of function i, ri represents a mathematical function that converts fi to a positive value, and g represents standardizing of all index values (the top 5% of the measured values of each function are averaged as the maximum value of the function, and the ratio of each measured value to the maximum value is calculated).
The mean value method cannot distinguish between one function being provided at a high level and another being provided at a low level vs. two functions being provided at an intermediate level (Byrnes et al., 2014). As such, we calculated MFt using 20%, 30%, 40%, 50%, 60%, and 70% of our threshold values. Thus, we supplemented the mean value method with a threshold analysis method for further analysis of the relationship between species richness and EMF.
Community temporal stability of macrophyte productivity was calculated for each treatment using the ratio of μ to σ:
where μ represents mean net macrophyte primary productivity and σ represents net macrophyte primary productivity standard deviation.
Before statistical analysis, all data were tested for normality and were subjected to Levene’s tests. Non-normal data were transformed (log10) to obtain normality. Repeated-measures ANOVA was used to test the effects of species richness, time, and their interaction on the total primary productivity, CTN(TP), and temporal stability of macrophyte productivity. A one-way ANOVA was applied to determine the statistical significance (P< 0.05) of the differences in the total primary productivity and the productivity of macrophytes, filamentous macroalgae and epiphyton, and phytoplankton at the end of the experiment, as well as CTN(TP), EMF, and temporal stability of macrophyte productivity among different treatments. The relationships between species richness and individual EFs, EMF, and temporal stability of macrophyte productivity were evaluated using simple OLS regressions within and across trophic levels. Variation partitioning analysis was used to analyze the degree of explanation of three indicator systems (environmental factors including water temperature, dissolved oxygen, pH, irradiance, time, and species richness) for the variation in EMF within and across trophic levels.
All the statistical analyses were performed with SPSS 20.0 for Windows (IBM Inc., Chicago, IL, USA) and R version 4.2.1 (https://www.r-project.org/), and figures were generated in Origin 2021 for Windows (OriginLab, Inc., USA).
3 Results
3.1 Effects of experimental treatments on individual EFs, EMF, and temporal stability of macrophyte productivity
For the three tested individual EFs (total primary productivity and the cumulative variation of TN and TP) and the temporal stability of macrophyte productivity, repeated-measures ANOVA showed that experimental treatments only significantly influenced total primary productivity (P< 0.001, Table 1, Figure 1). Time significantly influenced total primary productivity and the cumulative variation of TN (Table 1, Figure 2). The interactions of experimental treatments and time affect the three tested individual EFs (Table 1).
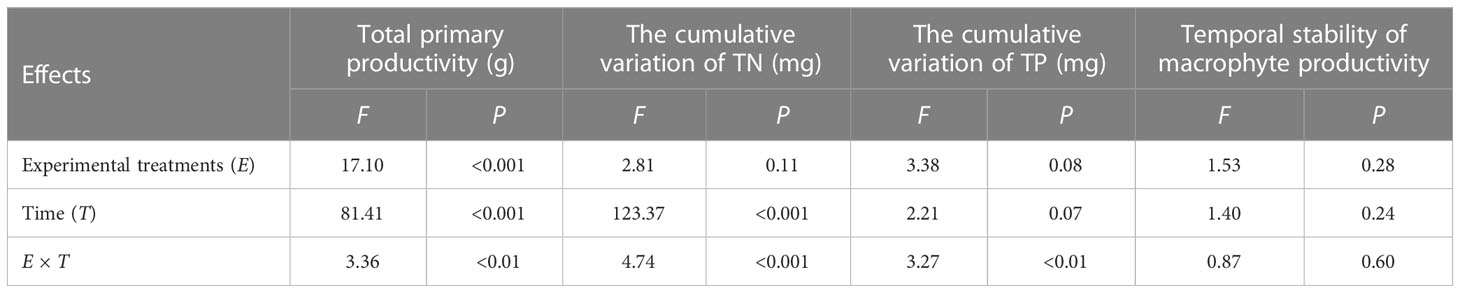
Table 1 Results from the repeated-measures ANOVA testing the effects of experimental treatments, time, and their interaction on the total primary productivity, the cumulative variation of TN and TP, and temporal stability of macrophyte productivity.
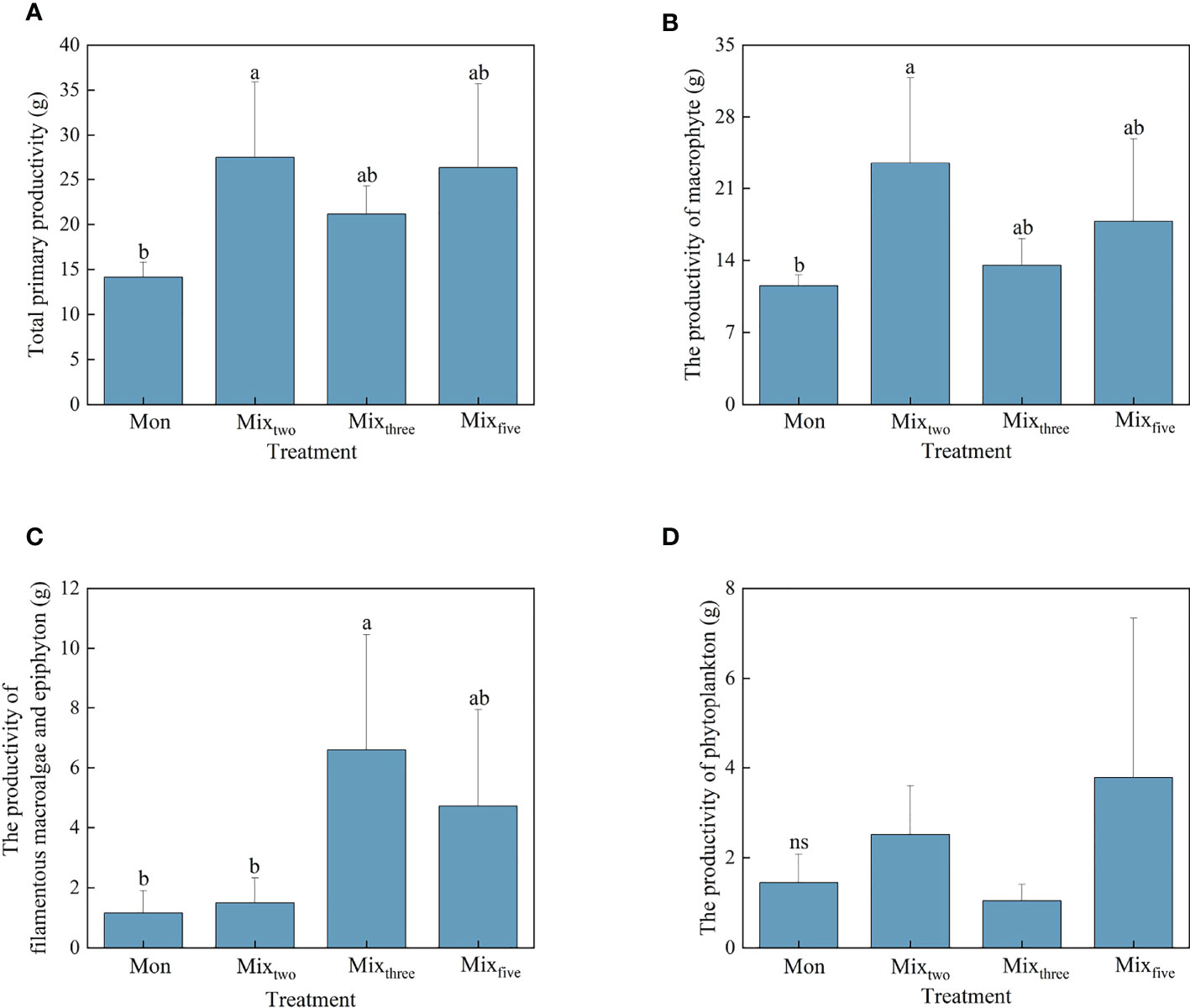
Figure 1 The total primary productivity (A) and the productivity of macrophyte (B), filamentous macroalgae and epiphyton (C), and phytoplankton (D) under different treatments at the end of the experiment. Bars represent mean values ± SD (n = 3), and different letters indicate significant differences (P< 0.05) between the treatments. (Mon: H. verticillata monoculture, Mixtwo: H. verticillata + V. natans mixed culture, Mixthree: H. verticillata + V. natans + P. maackianus mixed culture, and Mixfive: H. verticillata + V. natans + P. maackianus + C. carpio + C. cahayensis mixed culture).
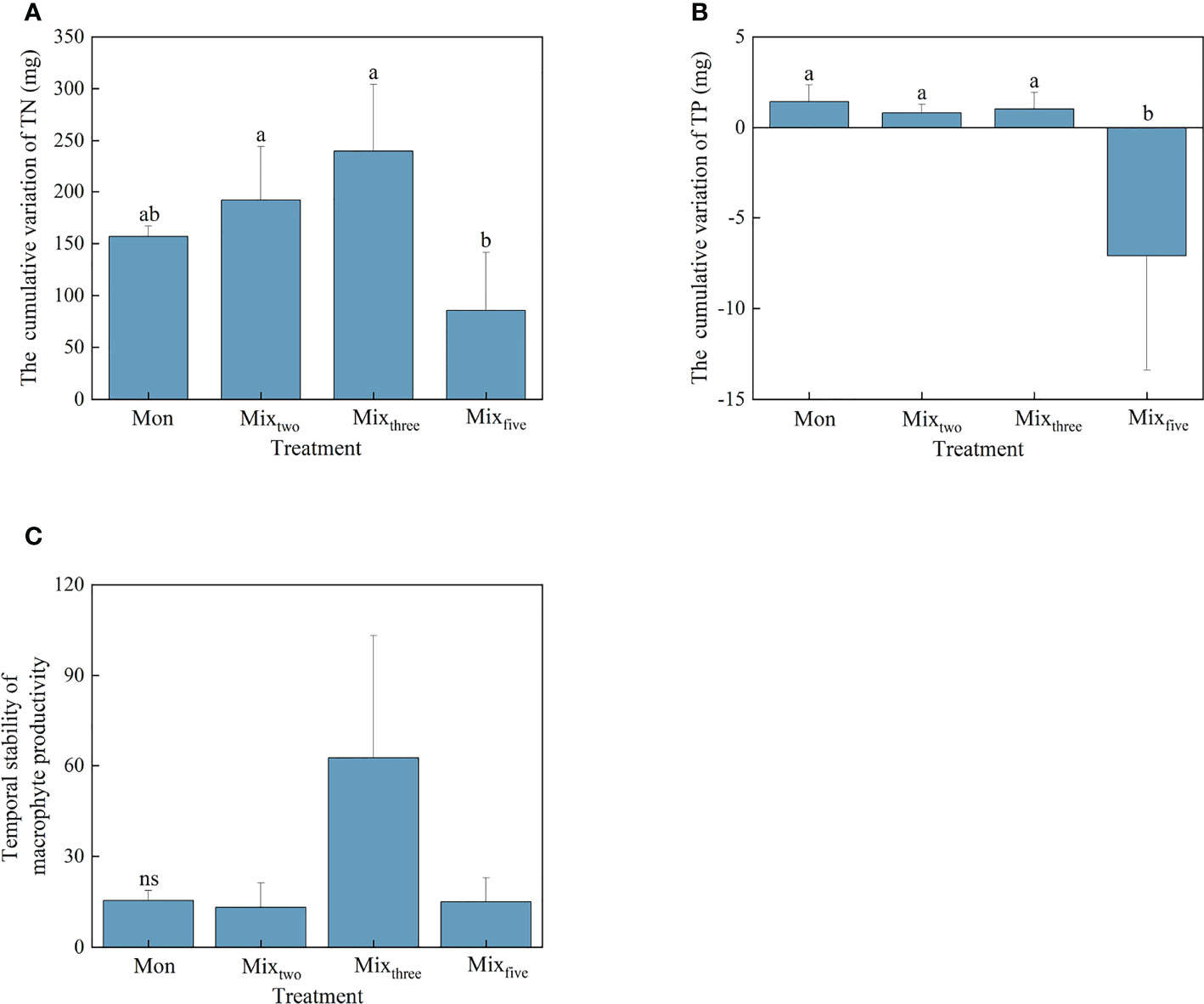
Figure 2 The cumulative variation of TN (A) and TP (B) and the temporal stability of macrophyte productivity (C) under different treatments at the end of the experiment. Bars represent mean values ± SD (n = 3), and different letters indicate significant differences (P< 0.05) between the treatments.
The response patterns of the total primary productivity and the macrophyte productivity for experimental treatments were similar. At the end of the experiment, the highest total primary productivity and macrophyte productivity both occurred in the Mixtwo treatment, but significant differences in total primary productivity and macrophyte productivity were only found between the Mon and Mixtwo treatments (P< 0.05, Figures 1A, B). For other primary producers, the productivity of phytoplankton showed no significant difference among the four experimental treatments, and the productivity of filamentous macroalgae and epiphyton under the Mixthree treatment was significantly higher than Mon and Mixtwo treatments (P< 0.05, Figures 1C, D).
For the cumulative variation of TN and TP, the values of the two tested individual EFs in the Mixfive treatment were significantly lower than that under other experimental treatments at the end of the experiment (P< 0.05). However, no significant differences were found in the amount of cumulative variation of the two aforementioned individual EFs among the three experimental treatments within a trophic level (Figures 2A, B). For the temporal stability of macrophyte productivity, there was no significant difference among the four experimental treatments (Figure 2C).
A one-way ANOVA showed that trophic complexity had a significant effect on EMF (P< 0.05, Figure 3). At the end of the experiment, the value of EMF across trophic levels was significantly lower than that within a trophic level (P< 0.05), while there was no significant difference among different macrophyte species richness treatments within a trophic level (Figure 3).
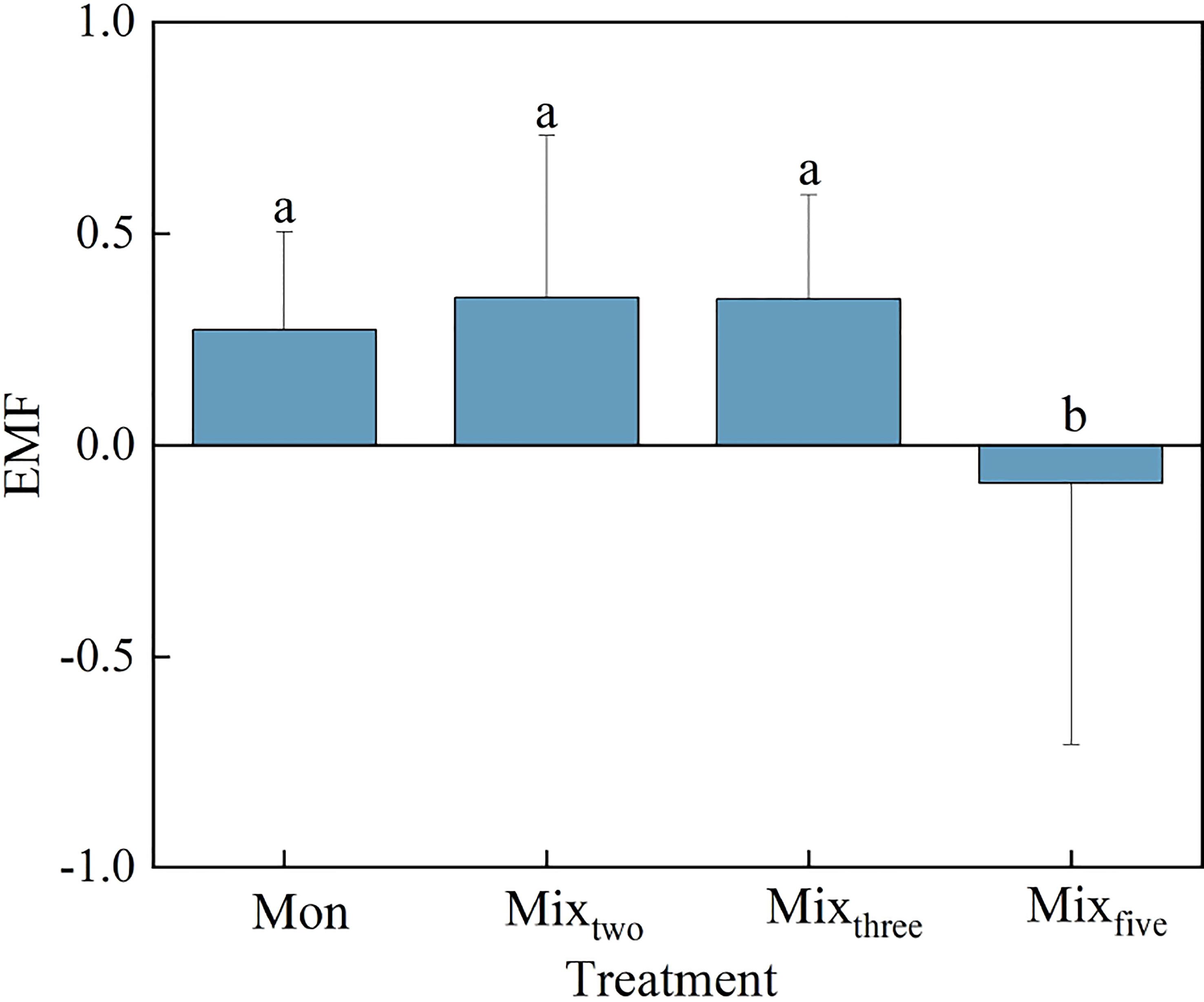
Figure 3 The EMF in different treatments at the end of the experiment. Bars represent mean values ± SD (n = 3), and different letters indicate significant differences (P< 0.05) between the treatments.
3.2 Relationship between species richness and individual EFs, EMF, and temporal stability of macrophyte productivity
For individual EFs, simple OLS regression analyses showed that species richness positively correlated with the total primary productivity within and across the trophic levels (Figure 4A). Across the trophic levels, there was a significant negative relationship between species richness and the cumulative variation of TP and EMF (P< 0.05, Figures 4C, D). However, there were no significant correlations between species richness and the cumulative variation of TN and temporal stability of macrophyte productivity within and across the trophic levels (Figures 4B–E).
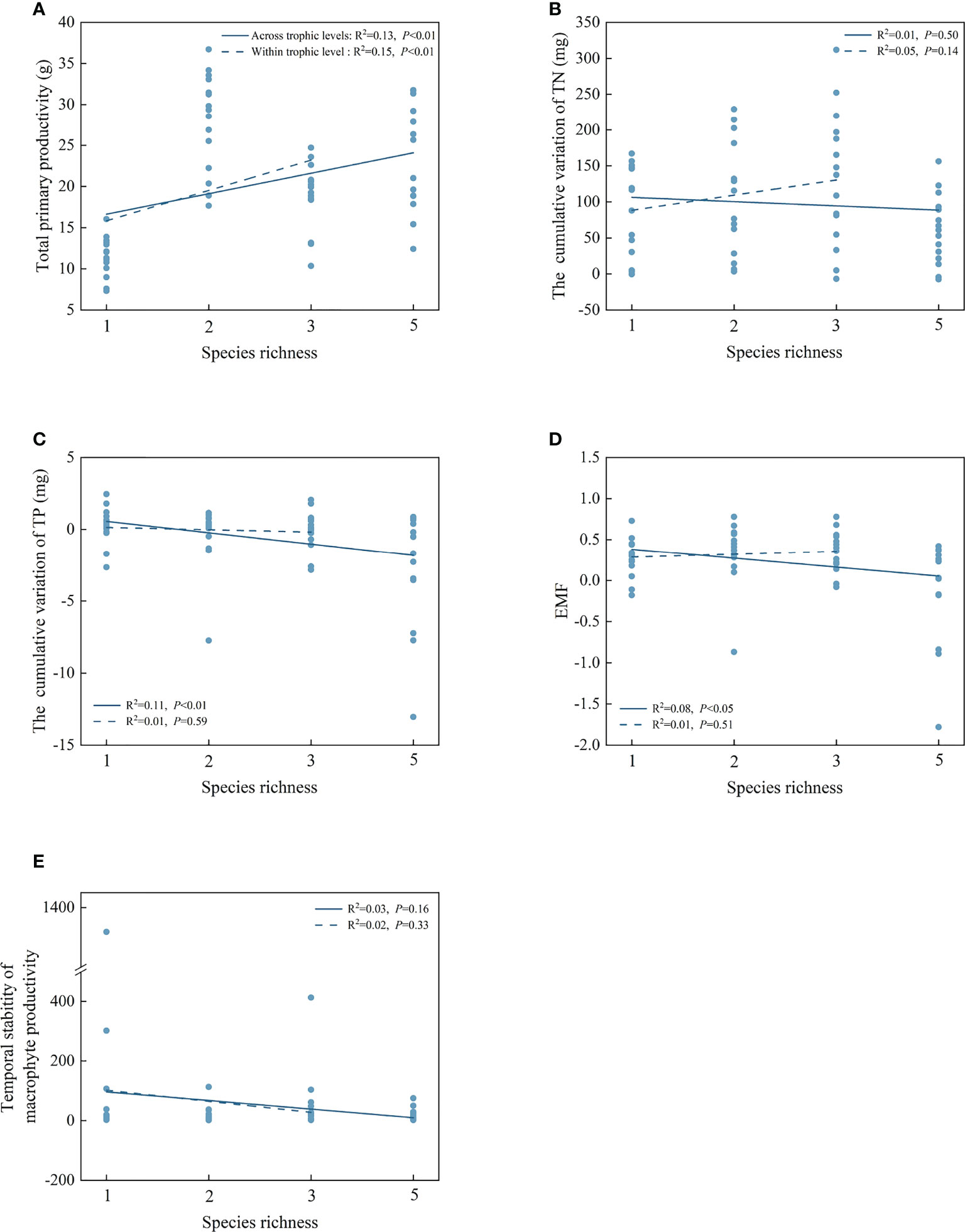
Figure 4 The correlations of species richness on total primary productivity (A), the cumulative variation of TN (B), the cumulative variation of TP (C), EMF (D), and temporal stability of macrophyte productivity (E) within and across trophic levels.
A threshold analysis showed that species richness positively affected the number of functions exceeding the threshold at values of 40% and 50%, and the relationships between species richness and the number of functions became flatter at higher and lower threshold values of 70% and 30%, respectively. By contrast, when the threshold value was 20%, species richness negatively affected the number of functions exceeded (Figure 5).
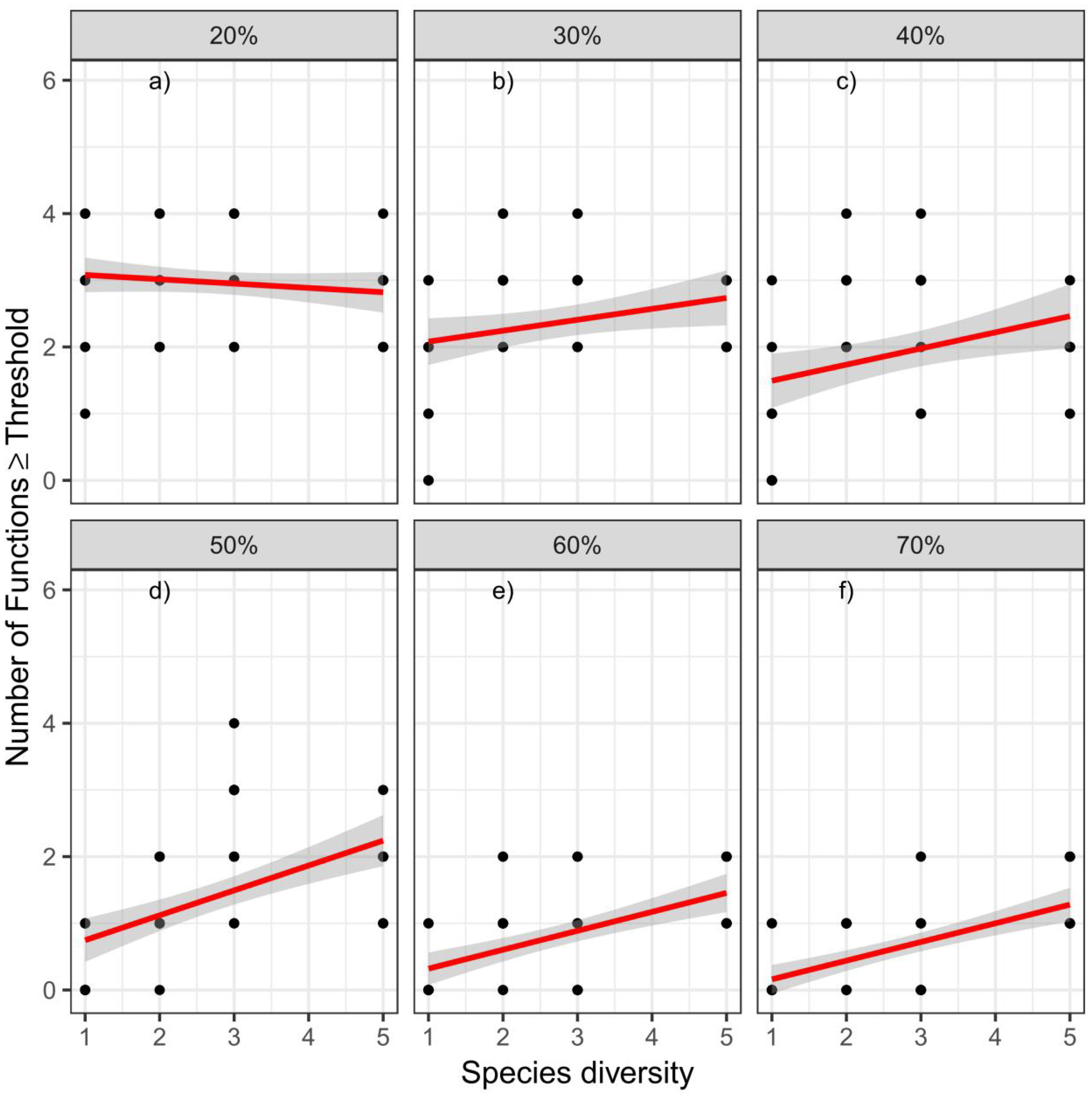
Figure 5 The relationships between species richness and multifunctionality. Panels show the relationships for six different thresholds (20%, 30%, 40%, 50%, 60%, and 70%).
3.3 Estimation of the relative contribution of the driving factors of EMF
Variation partitioning analyses showed that the number of variances of EMF explained by environmental factors, time, and species richness were similar among, within, and across the trophic levels, and the total explanations for EMF within and across trophic levels both were 99.60%, although the independent effects of the three driving factors were weak (Figure 6). Variation partitioning showed that the shared effects of environmental factors and time accounted for a high percentage, with their combined effects for EMF within and across trophic levels 94.10% and 89.00%, respectively (Figure 6). Furthermore, our results showed that time has the strongest independent effects on EMF within and across trophic levels among the three tested factors.
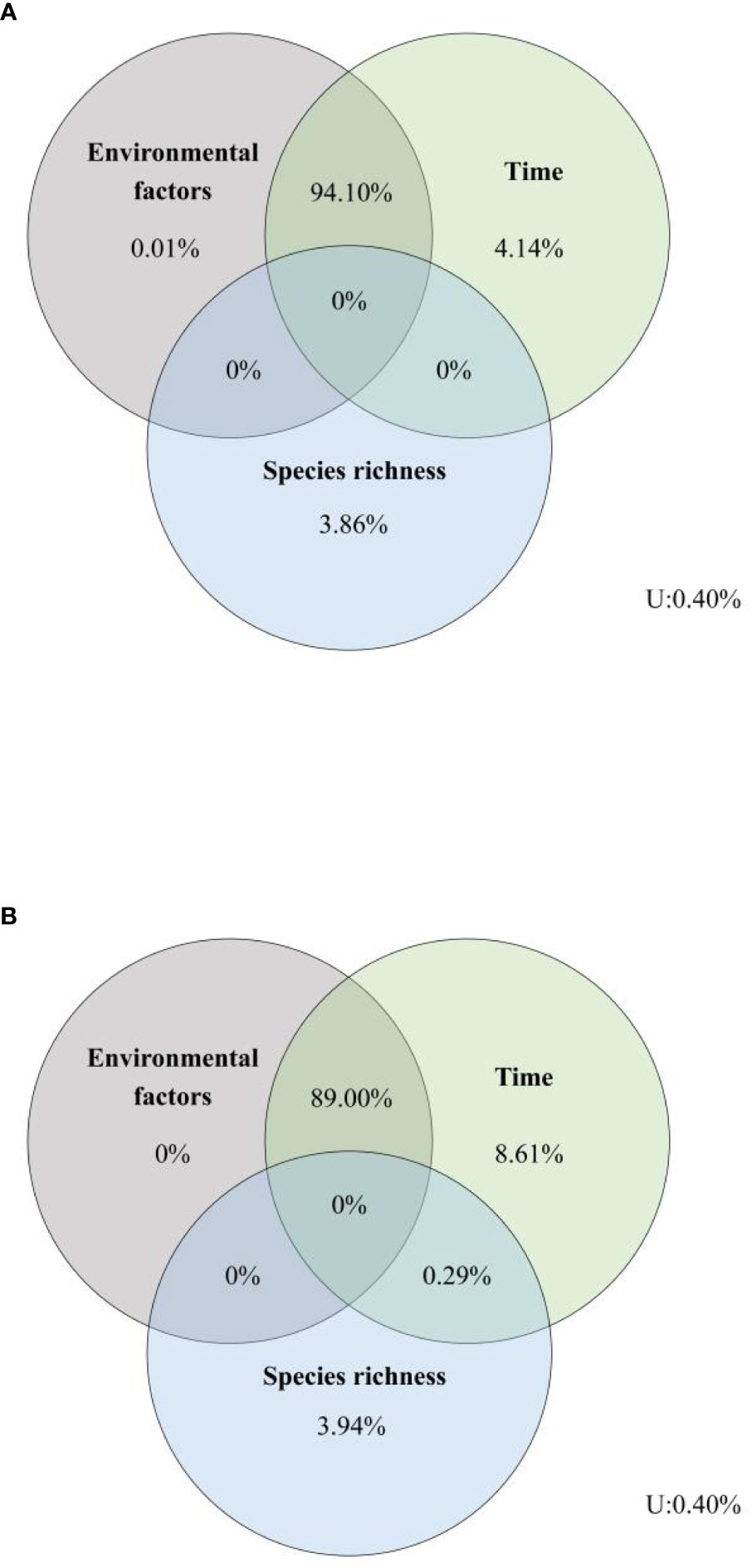
Figure 6 Relative effects of environmental factors, time, and species richness on EMF (A) within a trophic level, (B) across trophic levels. U, unexplained.
4 Discussion
4.1 Individual EFs within and across trophic levels
For individual EFs, as found in several other studies (Tilman et al., 1997; Engelhardt and Ritchie, 2001; Isbell et al., 2011; Chang et al., 2022), we showed that species richness–productivity relationships are positive both within and across trophic species diversity levels. Previous studies showed that the two main mechanisms responsible for the positive species richness–productivity relationship are the niche complementarity effect hypothesis and the selection effect hypothesis (Loreau and Hector, 2001). In this study, our results indicated that positive richness–productivity relationships may be largely due to the selection effect. Some studies demonstrated that higher productivity from greater species richness sometimes arises from the greater chance that superior competitors with high productivity are present in species mixtures (Tilman et al., 1997; Engelhardt and Ritchie, 2001). Our results indicated that H. verticillata became dominant due to environmental selection and competition and provided higher productivity within a community. Similar life-forms of submerged macrophyte species involved in our study may reduce the niche complementarity effect on system productivity. This may be because complementarity among submerged macrophyte species, especially for species of a similar type, might be rather weak, resulting in species diversity having no significant effect on vegetative production (Zhang et al., 2019). Thus, it is important to note that the types of species used in such experiments may lead to different outcomes (Zhang et al., 2019). Besides macrophyte productivity, our results showed that there were higher filamentous macroalgae and epiphyton productivity in communities with greater species richness. The most plausible explanation may be through a niche complementarity effect because various macrophytes can access different and more overall resources (Vanderstukken et al., 2011; Zhang et al., 2019). Therefore, our results suggest that the underlying mechanisms of species richness–productivity relationships were primary producer taxonomic group-specific.
Different from the positive correlation between species richness and productivity, our findings showed that species richness did not significantly relate to the cumulative variation of TN and TP within a trophic level. Previous studies demonstrated that BEF relationships for different EFs are not always congruent, and there are many different BEF relationships with positive, negative, nonlinear, and non-significant correlations (Mittelbach et al., 2001; Hooper et al., 2005; Duffy, 2009; Jaillard et al., 2014; Brose and Hillebrand, 2016). The observed non-significant correlations between species richness and the cumulative variation of TN and TP can be explained by other important processes besides nutrient uptake by plants and algae, including nutrient recycling by microorganisms or nutrients retained by sediment or surface structures, playing vital roles in nitrogen and phosphorus removal (Engelhardt and Ritchie, 2001; Zhang et al., 2019). We found a significant negative relationship between species richness and the cumulative variation of TP across trophic levels, but there were no significant correlations between species richness and the cumulative variation of TN. The main reason may be due to the different removal mechanisms of TN and TP in the water column, e.g., TN loss is mainly via denitrification by microorganisms; however, TP loss is mainly by plant absorption (Kyambadde et al., 2004; Paerl et al., 2011; Ma et al., 2017; Xing et al., 2020). Another reason may be that consumer excretion and decomposition of dead bodies release nutrients to the water column, resulting in increased phosphorus concentrations in the water column (Northcote, 1988). Our results further suggest that consumers play an important role in the EFs of freshwater systems (Duffy, 2002; Thébault and Loreau, 2003; Duffy et al., 2005) and that multitrophic species diversity has a greater impact on EMF than macrophyte diversity (Lefcheck et al., 2015).
4.2 EMF and temporal stability of macrophyte productivity within and across trophic levels
Quite different from most research results that biodiversity enhances EMF (Hector and Bagchi, 2007; Lefcheck et al., 2015; Perkins et al., 2015; Moi et al., 2021), our results showed a significant negative correlation between species richness and EMF across trophic levels. Early research about the relationship between biodiversity and EF showed similar negative species richness–EMF relationships and suggested that greater diversity caused by poor competitors results in reduced productivity (Loreau, 1998; Lasky et al., 2014). In our study, the negative species richness–EMF relationships were not due to primary productivity because there were non-significant differences in total primary productivity. Two reasons account for this phenomenon. First, it may be due to selected individual EFs and the functions used to calculate EMF (Edlinger et al., 2020; Garland et al., 2021; Jing and He, 2021), because different individual EFs would influence EMF through different trade-offs or synergies (Zavaleta et al., 2010; Byrnes et al., 2014). Second, the average approach used to calculate EMF in our study may affect the objective weight and general weight of the three selected individual EFs, which can lead to higher contribution rates of TN and TP cumulative variation for EMF (Byrnes et al., 2014). The effects of nutrients released via consumer excretion and the decomposition of dead bodies may be additive (or synergistic). Thus, our results emphasize the importance of selecting individual EFs, as well as the fact that the calculation of EMF can result in different outcomes for BEMF relationships.
Increased diversity facilitates the temporal stability of community productivity (Cottingham et al., 2001; Valone and Hoffman, 2003; Tilman et al., 2006). Although we hypothesized that there were positive diversity–ecosystem stability relationships within and across trophic levels, the lack of consistent differences in temporal stability of productivity among the four experimental treatments did not support this hypothesis. In agreement with some other studies (Jiang and Pu, 2009; Sasaki and Lauenroth, 2011; Ma et al., 2017), our results also showed that increased macrophyte species richness does not affect the temporal stability of macrophyte productivity within a trophic level. The most probable explanation is that productivity was controlled by dominant macrophyte species, rather than species richness, which results in no effect of species richness on macrophyte productivity (Sasaki and Lauenroth, 2011; Ma et al., 2017). Another possible reason may be lower species richness and lower trophic level complexity, as the relationship between species richness and temporal stability of community productivity was critically dependent (Jiang and Pu, 2009). Our study uses low species richness and fewer trophic levels and, therefore, almost certainly underestimates the levels of diversity needed for EMF.
4.3 Effect of species richness, time and environmental factors on EMF
Our results showed that the links between EMF and biodiversity within and across trophic levels are mediated by the combination of environmental factors and time, more so than by species richness. Many studies have proved that environmental factors and time can affect the interactions between the various elements of an ecosystem which results in effects on the EMF (Bai et al., 2022). Changing environmental factors can affect EF both directly via altering rates of ecosystem processes (Spaak et al., 2017) and indirectly via altering species dynamics and interactions (Dornelas et al., 2014; Blowes et al., 2019); environmental factors could also interact with biodiversity in regulating EF (Eisenhauer et al., 2019; Benkwitt et al., 2020). For example, individual responses to environmental variations cause changes in population abundance, generating interactions between populations, which in turn affect community structure and composition that extend to entire ecosystems (Hofmann and Todgham, 2010). Some studies indicated the effects of environmental factors on EMF may change over time (Tilman et al., 2001; Xu et al., 2021; Guo et al., 2022; Hong et al., 2022), and the response rate of EF to environmental change depends on the resilience of ecosystem functions over time (Wu et al., 2018; Qu et al., 2019). Long-term experiments are revealing that the slope of the BEF relationship can vary with environmental context across sites and through time (Isbell et al., 2011; Qiu and Cardinale, 2020). Thus, some researchers strongly emphasize that the dimension of time should be taken into consideration in experiments and observational studies which can predict whether, when, and to what extent ecosystems will be affected by altered conditions (Edlinger et al., 2020).
We found a weak influence of species richness on EMF within and across trophic levels. This also may be due to selected individual EFs and the diversity of the taxonomic group considered. In our study, we only took macrophytes, snails, and fish as representatives for biodiversity. Other groups of organisms, such as microbes, can drive many ecosystem processes, especially nutrient cycling, but our study does not directly address the functional role played by these and other important groups. Obviously, our results underestimated the effects of some organisms on EMF, especially for microbes which can significantly influence the nitrogen cycle in our study. Therefore, our results further highlight the significance of selecting individual EFs and considering the diversity of the taxonomic groups for BEMF relationship research (Vaughn, 2010; Zhang et al., 2019).
5 Conclusion
In this study, we examined how biodiversity affects the individual EFs and EMF within and across trophic levels over time in freshwater ecosystems. Although our study used low levels of species richness and fewer trophic levels, we found the following: 1) positive diversity–productivity relationships within and across trophic levels, 2) significant negative correlations between species richness and the cumulative variation of TP and EMF across trophic levels, and 3) the links between EMF and biodiversity within and across the trophic levels are mediated by the combination of environmental factors and time, rather than by species richness. Together with other research, these results indicate that BEF relationships for different EFs are not always the same, and multitrophic species diversity has a greater impact on EF than plant diversity.
Beyond experimental limitations, our study provides important insights and implications for the conservation and management of shallow lakes. Species richness of multiple trophic groups, such as consumers and primary producers, are crucial moderators of EMF. Similar submerged macrophyte species involved in this study may have influenced the experimental outcomes because individual species with unique traits can be as important to ecosystem function as high species richness. Furthermore, species within functional groups are not necessarily ecological equivalents. The composition of aquatic plant functional groups is directly related to community productivity (Fu et al., 2014; 2019). Some studies have shown that differences in ecosystem function among different life forms due to plant phylogeny or plant adaptations to the environment, and the complex interactions between species of different functional groups in natural ecosystems can have significant effects on the structure and function of ecosystems (Thébault and Loreau, 2003; Ives et al., 2005). Thus, our results imply that management practices that restore the diversity of aquatic macrophytes in wetlands should consider not only species diversity but also different functional groups and life forms. Future research should study these relationships between different functional groups and life forms in the context of global change to more comprehensively study the functional dynamics of freshwater ecosystems to achieve better predictive power for conservation and restoration initiatives.
Data availability statement
The raw data supporting the conclusions of this article will be made available by the authors, without undue reservation.
Ethics statement
The studies involving animals were reviewed and approved by Animal Ethics and Welfare Committee of Hubei University.
Author contributions
ZL and ZX designed the study. ZX, HY, HM, QP and SY conducted the experiment. ZX, QC, YY, and LW performed chemical analyses and statistical analyses. ZX wrote the paper, and other co-authors reviewed the paper. All authors contributed to the article and approved the submitted version.
Funding
The study was financed by National Natural Science Foundation of China (32271612), the Key Project of the National Natural Science Foundation of Hubei Province (2020CFA005), and the Young and Middle-aged Science & Technology Innovation Team Project of Hubei Provincial Department of Education (T201701).
Conflict of interest
The authors declare that the research was conducted in the absence of any commercial or financial relationships that could be construed as a potential conflict of interest.
Publisher’s note
All claims expressed in this article are solely those of the authors and do not necessarily represent those of their affiliated organizations, or those of the publisher, the editors and the reviewers. Any product that may be evaluated in this article, or claim that may be made by its manufacturer, is not guaranteed or endorsed by the publisher.
References
Anujan K., Heipern S. A., Prager C. M., Weeks B. C., Naeem S. (2021). Trophic complexity alters the diversity multifunctionality relationship in experimental grassland mesocosms. Ecol. Evolution. 11, 6471–6479. doi: 10.1002/ece3.7498
Bai Y., Wu J., Pan Q., Huang J., Wang Q., Li F., et al. (2007). Positive linear relationship between productivity and diversity: Evidence from the Eurasian steppe. J. Appl. Ecol. 44, 1023–1034. doi: 10.1111/j.1365-2664.2007.01351.x
Bai X. H., Zhao W. W., Yin C. C. (2022). Change process and interaction mechanism of ecosystem services from the perspective of regime shift. Acta Ecologica Sin. 42 (15), 6054–6065. doi: 10.5846/stxb202103280808
Benkwitt C. E., Wilson S. K., Graham N. A. (2020). Biodiversity increases ecosystem functions despite multiple stressors on coral reefs. Nat. Ecol. Evol. 4 (7), 919–926. doi: 10.1038/s41559-020-1203-9
Blowes S. A., Supp S. R., Antão L. H., Bates A., Bruelheide H., Chase J. M., et al. (2019). The geography of biodiversity change in marine and terrestrial assemblages. Science 366 (6463), 339–345. doi: 10.1126/science.aaw1620
Brooker R. W., George T. S., Homulle Z., Karley A. J., Newton A. C., Pakeman R. J., et al. (2021). Facilitation and biodiversity-ecosystem function relationships in crop production systems and their role in sustainable farming. J. Ecol. 109 (5), 2054–2067. doi: 10.1111/1365-2745.13592
Brose U., Hillebrand H. (2016). Biodiversity and ecosystem functioning in dynamic landscapes. Philos. Trans. R. Soc. Lond. B. Biol. Sci. 371 (1694), 20150267. doi: 10.1098/rstb.2015.0267
Byrnes J. E., Gamfeldt L., Isbell F., Lefcheck J. S., Griffin J. N., Hector A., et al. (2014). Investigating the relationship between biodiversity and ecosystem multifunctionality: challenges and solutions. Methods Ecol. Evol. 5 (2), 111–124. doi: 10.1111/2041-210X.12143
Cao X., Chai L., Jiang D., Wang J., Liu Y., Huang Y. (2018). Loss of biodiversity alters ecosystem function in freshwater streams: potential evidence from benthic macroinvertebrates. Ecosphere 9 (10), e02445. doi: 10.1002/ecs2.2445
Cardinale B. J., Duffy J. E., Gonzalez A., Hooper D. U., Perrings C., Venail P., et al. (2012). Biodiversity loss and its impact on humanity. Nature 486 (7401), 59–67. doi: 10.1038/nature11148
Chang C. W., Miki T., Ye H., Souissi S., Adrian R., Anneville O., et al. (2022). Causal networks of phytoplankton diversity and biomass are modulated by environmental context. Nat. Commun. 13 (1), 1140. doi: 10.1038/s41467-022-28761-3
Cottingham K. L., Brown B. L., Lennon J. T. (2001). Biodiversity may regulate the temporal variability of ecological systems. Ecol. Lett. 4 (1), 72–85. doi: 10.1046/j.1461-0248.2001.00189.x
Daam M. A., Teixeira H., Lillebø A. I., Nogueira A. J. A. (2019). Establishing causal links between aquatic biodiversity and ecosystem functioning: Status and research needs. Sci. Total Environ. 656, 1145–1156. doi: 10.1016/j.scitotenv.2018.11.413
Davidson T. A., Reid M. A., Sayer C. D., Chilcott S. (2013). Palaeolimnological records of shallow lake biodiversity change: exploring the merits of single versus multi-proxy approaches. J. Paleolimnology 49, 431–446. doi: 10.1007/s10933-013-9696-8
Dornelas M., Gotelli N. J., McGill B., Shimadzu H., Moyes F., Sievers C., et al. (2014). Assemblage time series reveal biodiversity change but not systematic loss. Science 344 (6181), 296–299. doi: 10.1126/science.1248484
Duffy J. E. (2002). Biodiversity and ecosystem function: the consumer connection. Oikos 99 (2), 201–219. doi: 10.1034/j.1600-0706.2002.990201.x
Duffy J. E. (2009). Why biodiversity is important to the functioning of real-world ecosystems. Front. Ecol. Environ. 7 (8), 437–444. doi: 10.1890/070195
Duffy J. E., Cardinale B. J., France K. E., McIntyre P. B., Thébault E., Loreau M. (2007). The functional role of biodiversity in ecosystems: incorporating trophic complexity. Ecol. Lett. 10 (6), 522–538. doi: 10.1111/j.1461-0248.2007.01037.x
Duffy J. E., Paul Richardson J., France K. E. (2005). Ecosystem consequences of diversity depend on food chain length in estuarine vegetation. Ecol. Lett. 8 (3), 301–309. doi: 10.1111/j.1461-0248.2005.00725.x
Duncan C., Thompson J. R., Pettorelli N. (2015). The quest for a mechanistic understanding of biodiversity-ecosystem services relationships. Proc. R. Soc. B: Biol. Sci. 282 (1817), 20151348. doi: 10.1098/rspb.2015.1348
Ebeling A., Rzanny M., Lange M., Eisenhauer N., Hertzog L. R., Meyer S. T., et al. (2018). Plant diversity induces shifts in the functional structure and diversity across trophic levels. Oikos 127 (2), 208–219. doi: 10.1111/oik.04210
Edlinger A., Saghaï A., Herzog C., Degrune F., Garland G. (2020). Towards a multidimensional view of biodiversity and ecosystem functioning in a changing world. New Phytol. 228, 820–822. doi: 10.1111/nph.16881
Eisenhauer N., Dobies T., Cesarz S., Hobbie S. E., Meyer R. J., Worm K., et al. (2013). Plant diversity effects on soil food webs are stronger than those of elevated CO2 and n deposition in a long-term grassland experiment. Proc. Natl. Acad. Sci. 110 (17), 6889–6894. doi: 10.1073/pnas.1217382110
Eisenhauer N., Schielzeth H., Barnes A. D., Barry K., Brose U., Bruelheide H., et al. (2019). A multitrophic perspective on biodiversity–ecosystem functioning research. Adv. Ecol. Res. 61, 1–48. doi: 10.1016/bs.aecr.2019.06.001
Engelhardt K. A., Ritchie M. E. (2001). Effects of macrophyte species richness on wetland ecosystem functioning and services. Nature 411 (6838), 687–689. doi: 10.1038/35079573
Fu H., Yuan G., Li W., Ge D., Zou D., Huang Z. (2019). Environmental effects on community productivity of aquatic macrophytes are mediated by species and functional composition. Ecohydrology 12 (8), e2147. doi: 10.1002/eco.2147
Fu H., Zhong J., Yuan G., Ni L., Xie P., Cao T. (2014). Functional traits composition predict macrophytes community productivity along a water depth gradient in a freshwater lake. Ecol. Evol. 4 (9), 1516–1523. doi: 10.1002/ece3.1022
Fu B. J., Yu D. D. (2016). Trade-off analyses and synthetic integrated method of multiple ecosystem services. Resour. Sci. 38 (1), 1–9. doi: 10.18402/resci.2016.01.01
Gagic V., Bartomeus I., Jonsson T., Taylor A., Winqvist C., Fischer C., et al. (2015). Functional identity and diversity of animals predict ecosystem functioning better than species-based indices. Proc. R. Soc. B: Biol. Sci. 282 (1801), 20142620. doi: 10.1098/rspb.2014.2620
Gamfeldt L., Lefcheck J. S., Byrnes J. E., Cardinale B. J., Duffy J. E., Griffin J. N. (2015). Marine biodiversity and ecosystem functioning: what's known and what's next? Oikos 124 (3), 252–265. doi: 10.1111/oik.01549
Garland G., Banerjee S., Edlinger A., Miranda Oliveira E., Herzog C., Wittwer R., et al. (2021). A closer look at the functions behind ecosystem multifunctionality: A review. J. Ecol. 109 (2), 600–613. doi: 10.1111/1365-2745.13511
Guo C., Zhu M., Xu H., Zhang Y., Qin B., Zhu G., et al. (2022). Spatiotemporal dependency of resource use efficiency on phytoplankton diversity in lake taihu. Limnology Oceanography 67 (4), 830–842. doi: 10.1002/lno.12038
Guy-Haim T., Lyons D. A., Kotta J., Ojaveer H., Queirós A. M., Chatzinikolaou E., et al. (2018). Diverse effects of invasive ecosystem engineers on marine biodiversity and ecosystem functions: A global review and meta-analysis. Global Change Biol. 24 (3), 906–924. doi: 10.1111/gcb.14007
Hector A., Bagchi R. (2007). Biodiversity and ecosystem multifunctionality. Nature 448 (7150), 188–190. doi: 10.1038/nature05947
Hofmann G. E., Todgham A. E. (2010). Living in the now: physiological mechanisms to tolerate a rapidly changing environment. Annu. Rev. Physiol. 72, 127–145. doi: 10.1146/annurev-physiol-021909-135900
Hong P., Schmid B., De Laender F., Eisenhauer N., Zhang X., Chen H., et al. (2022). Biodiversity promotes ecosystem functioning despite environmental change. Ecol. Lett. 25 (2), 555–569. doi: 10.1111/ele.13936
Hooper D. U., Chapin F. S. III, Ewel J. J., Hector A., Inchausti P., Lavorel S., et al. (2005). Effects of biodiversity on ecosystem functioning: a consensus of current knowledge. Ecol. Monogr. 75 (1), 3–35. doi: 10.1890/04-0922
Hu A., Li Y., Yang Y., Peng Q., Li Z. (2022). Effects of different growth form submerged macrophyte assemblages on biomass accumulation and water purification. Lake Sci. 34 (5), 1484–1492. doi: 10.18307/2022.0527
Isbell F., Calcagno V., Hector A., Connolly J., Harpole W. S., Reich P. B., et al. (2011). High plant diversity is needed to maintain ecosystem services. Nature 477 (7363), 199–202. doi: 10.1038/nature10282
Ives A. R., Cardinale B. J., Snyder W. E. (2005). A synthesis of subdisciplines: predator-prey interactions, and biodiversity and ecosystem functioning. Ecol. Lett. 8 (1), 102–116. doi: 10.1111/j.1461-0248.2004.00698.x
Jackson M. C., Weyl O. L. F., Altermatt F., Durance I., Friberg N., Dumbrell A. J., et al. (2016). “Recommendations for the next generation of global freshwater biological monitoring tools,” in Advances in ecological research, vol. 55 . Eds. Dumbrell A. J., Kordas R. L., Woodward G. (Academic Press), 615–636. doi: 10.1016/bs.aecr.2016.08.008
Jaillard B., Rapaport A., Harmand J., Brauman A., Nunan N. (2014). Community assembly effects shape the biodiversity-ecosystem functioning relationships. Funct. Ecol. 28 (6), 1523–1533. doi: 10.1111/1365-2435.12267
Jampeetong A., Brix H. (2009). Effects of NaCl salinity on growth, morphology, photosynthesis and proline accumulation of salvinia natans. Aquat. Bot. 91 (3), 181–186. doi: 10.1016/j.aquabot.2009.05.003
Janssen A. B. G., Hilt S., Kosten S., de Klein J. J., Paerl H. W., Van de Waal D. B. (2021). Shifting states, shifting services: Linking regime shifts to changes in ecosystem services of shallow lakes. Freshw. Biol. 66, 1–12. doi: 10.1111/fwb.13582
Jiang L., Pu Z. (2009). Different effects of species diversity on temporal stability in single-trophic and multitrophic communities. Am. Nat. 174 (5), 651–659. doi: 10.1086/605961
Jing X., Sanders N. J., Shi Y., Chu H., Classen A. T., Zhao K., et al. (2015). The links between ecosystem multifunctionality and above-and belowground biodiversity are mediated by climate. Nat. Commun. 6 (1), 8159. doi: 10.1038/ncomms9159
Jing X., He J. (2021). Relationship between biodiversity, ecosystem multifunctionality and multiserviceability: literature overview and research advances. Chin. J. Plant Ecology. 45 (10), 1094–1111. doi: 10.17521/cjpe.2020.0154
Kyambadde J., Kansiime F., Gumaelius L., Dalhammar G. (2004). A comparative study of cyperus papyrus and miscanthidium violaceum-based constructed wetlands for wastewater treatment in a tropical climate. Water Res. 38 (2), 475–485. doi: 10.1016/j.watres.2003.10.008
Lasky J. R., Uriarte M., Boukili V. K., Erickson D. L., John Kress W., Chazdon R. L. (2014). The relationship between tree biodiversity and biomass dynamics changes with tropical forest succession. Ecol. Lett. 17 (9), 1158–1167. doi: 10.1111/ele.12322
Lefcheck J. S., Byrnes J. E., Isbell F., Gamfeldt L., Griffin J. N., Eisenhauer N., et al. (2015). Biodiversity enhances ecosystem multifunctionality across trophic levels and habitats. Nat. Commun. 6 (1), 1–7. doi: 10.1038/ncomms7936
Loreau M. (1998). Ecosystem development explained by competition within and between material cycles. Proc. R. Soc. London. Ser. B: Biol. Sci. 265 (1390), 33–38. doi: 10.1098/rspb.1998.0260
Loreau M., Hector A. (2001). Partitioning selection and complementarity in biodiversity experiments. Nature 412 (6842), 72–76. doi: 10.1038/35083573
Losapio G., Schmid B., Bascompte J., Michalet R., Cerretti P., Germann C., et al. (2021). An experimental approach to assessing the impact of ecosystem engineers on biodiversity and ecosystem functions. Ecology 102 (2), e03243. doi: 10.1002/ecy.3243
Ma Z., Liu H., Mi Z., Zhang Z., Wang Y., Xu W., et al. (2017). Climate warming reduces the temporal stability of plant community biomass production. Nat. Commun. 8 (1), 15378. doi: 10.1038/ncomms15378
Maestre F. T., Quero J. L., Gotelli N. J., Escudero A., Ochoa V., Delgado-Baquerizo M., et al. (2012). Plant species richness and ecosystem multifunctionality in global drylands. Science 335 (6065), 214–218. doi: 10.1126/science.1215442
Messmer V., Blowes S. A., Jones G. P., Munday P. L. (2014). Experimental evaluation of diversity-productivity relationships in a coral reef fish assemblage. Oecologia 176, 237–249. doi: 10.1007/s00442-014-2992-9
Mittelbach G. G., Steiner C. F., Scheiner S. M., Gross K. L., Reynolds H. L., Waide R. B., et al. (2001). What is the observed relationship between species richness and productivity? Ecology 82 (9), 2381–2396. doi: 10.1890/0012-9658(2001)082[2381:WITORB]2.0.CO;2
Moi D. A., Romero G. Q., Antiqueira P. A., Mormul R. P., Teixeira de Mello F., Bonecker C. C. (2021). Multitrophic richness enhances ecosystem multifunctionality of tropical shallow lakes. Funct. Ecol. 35 (4), 942–954. doi: 10.1111/1365-2435.13758
Northcote T. G. (1988). Fish in the structure and function of freshwater ecosystems: a “top-down” view. Can. J. Fisheries Aquat. Sci. 45 (2), 361–379. doi: 10.1139/f88-044
Paerl H. W., Xu H., McCarthy M. J., Zhu G., Qin B., Li Y., et al. (2011). Controlling harmful cyanobacterial blooms in a hyper-eutrophic lake (Lake Taihu, China): the need for a dual nutrient (N & P) management strategy. Water Res. 45 (5), 1973–1983. doi: 10.1016/j.watres.2010.09.018
Perkins D. M., Bailey R. A., Dossena M., Gamfeldt L., Reiss J., Trimmer M., et al. (2015). Higher biodiversity is required to sustain multiple ecosystem processes across temperature regimes. Global Change Biol. 21 (1), 396–406. doi: 10.1111/gcb.12688
Qiu J., Cardinale B. J. (2020). Scaling up biodiversity-ecosystem function relationships across space and over time. Ecology 101 (11), e03166. doi: 10.1002/ecy.3166
Qu Y., Wu N., Guse B., Makarevičiūtė K., Sun X., Fohrer N. (2019). Riverine phytoplankton functional groups response to multiple stressors variously depending on hydrological periods. Ecol. Indic. 101, 41–49. doi: 10.1016/j.ecolind.2018.12.049
Sasaki T., Lauenroth W. K. (2011). Dominant species, rather than diversity, regulates temporal stability of plant communities. Oecologia 166 (3), 761–768. doi: 10.1007/s00442-011-1916-1
Scheffer M. (2004). “The story of some shallow lakes,” in Ecology of shallow lakes. Eds. DeAngelis D. L., Manly B. F. J. (Dordrecht, the Netherlands: Kluwer Academic Publishers), 1–19.
Scheffer M., Carpenter S., Foley J. A., Folke C., Walker B. (2001). Catastrophic shifts in ecosystems. Nature 413 (6856), 591–596. doi: 10.1038/35098000
Schuldt A., Assmann T., Brezzi M., Buscot F., Eichenberg D., Gutknecht J., et al. (2018). Biodiversity across trophic levels drives multifunctionality in highly diverse forests. Nat. Commun. 9 (1), 2989. doi: 10.1038/s41467-018-05421-z
Slade E. M., Kirwan L., Bell T., Philipson C. D., Lewis O. T., Roslin T. (2017). The importance of species identity and interactions for multifunctionality depends on how ecosystem functions are valued. Ecology 98 (10), 2626–2639. doi: 10.1002/ecy.1954
Spaak J. W., Baert J. M., Baird D. J., Eisenhauer N., Maltby L., Pomati F., et al. (2017). Shifts of community composition and population density substantially affect ecosystem function despite invariant richness. Ecol. Lett. 20 (10), 1315–1324. doi: 10.1111/ele.12828
Strong J. A., Andonegi E., Bizsel K. C., Danovaro R., Elliott M., Franco A., et al. (2015). Marine biodiversity and ecosystem function relationships: the potential for practical monitoring applications. Estuarine Coast. Shelf Sci. 161, 46–64. doi: 10.1016/j.ecss.2015.04.008
Thébault E., Loreau M. (2003). Food-web constraints on biodiversity–ecosystem functioning relationships. Proc. Natl. Acad. Sci. 100 (25), 14949–14954. doi: 10.1073/pnas.2434847100
Tilman D., Lehman C. L., Thomson K. T. (1997). Plant diversity and ecosystem productivity: theoretical considerations. Proc. Natl. Acad. Sci. 94 (5), 1857–1861. doi: 10.1073/pnas.94.5.1857
Tilman D., Reich P. B., Knops J. M. (2006). Biodiversity and ecosystem stability in a decade-long grassland experiment. Nature 441 (7093), 629–632. doi: 10.1038/nature04742
Tilman D., Reich P. B., Knops J., Wedin D., Mielke T., Lehman C. (2001). Diversity and productivity in a long-term grassland experiment. Science 294 (5543), 843–845. doi: 10.1126/science.1060391
Valone T. J., Hoffman C. D. (2003). A mechanistic examination of diversity-stability relationships in annual plant communities. Oikos 103 (3), 519–527. doi: 10.1034/j.1600-0706.2003.12279.x
van der Plas F. (2019). Biodiversity and ecosystem functioning in naturally assembled communities. Biol. Rev. 94 (4), 1220–1245. doi: 10.1111/brv.12499
Vanderstukken M., Mazzeo N., Van Colen W., Declerck S. A., Muylaert K. (2011). Biological control of phytoplankton by the subtropical submerged macrophytes Egeria densa and Potamogeton illinoensis: a mesocosm study. Freshw. Biol. 56 (9), 1837–1849. doi: 10.1111/j.1365-2427.2011.02624.x
Vaughn C. C. (2010). Biodiversity losses and ecosystem function in freshwaters: emerging conclusions and research directions. BioScience 60 (1), 25–35. doi: 10.1525/bio.2010.60.1.7
Wang S., Brose U. (2017). Biodiversity and ecosystem functioning in food webs: the vertical diversity hypothesis. Ecol. Lett. 21, 9–20. doi: 10.1111/ele.12865Wu
Wu N., Qu Y., Guse B., Makarevičiūtė K., To S., Riis T., et al. (2018). Hydrological and environmental variables outperform spatial factors in structuring species, trait composition, and beta diversity of pelagic algae. Ecol. Evol. 8 (5), 2947–2961. doi: 10.1002/ece3.3903
Xing P., Li B., Han Y., Gu Q., Wan H. (2020). Responses of freshwater ecosystems to global change: research progress and outlook. Chin. J. Plant Ecol. 44 (5), 565–574. doi: 10.17521/cjpe.2020.0009
Xu W., Jing X., Ma Z., He J. S. (2016). A review on the measurement of ecosystem multifunctionality. Biodiversity Sci. 24 (1), 72–84. doi: 10.17520/biods.2015170
Xu Q., Yang X., Yan Y., Wang S., Loreau M., Jiang L. (2021). Consistently positive effect of species diversity on ecosystem, but not population, temporal stability. Ecol. Lett. 24 (10), 2256–2266. doi: 10.1111/ele.13777
Yasuhara M., Doi H., Wei C. L., Danovaro R., Myhre S. E. (2016). Biodiversity-ecosystem functioning relationships in long-term time series and palaeoecological records: deep sea as a test bed. Philos. Trans. R. Soc. B: Biol. Sci. 371 (1694), 20150282. doi: 10.1098/rstb.2015.0282
Zavaleta E. S., Pasari J. R., Hulvey K. B., Tilman G. D. (2010). Sustaining multiple ecosystem functions in grassland communities requires higher biodiversity. Proc. Natl. Acad. Sci. 107 (4), 1443–1446. doi: 10.1073/pnas.0906829107
Zhang Q., Liu Y. P., Luo F. L., Dong B. C., Yu F. H. (2019). Does species richness affect the growth and water quality of submerged macrophyte assemblages? Aquat. Bot. 153, 51–57. doi: 10.1016/j.aquabot.2018.11.006
Keywords: ecosystem functioning, multitrophic richness, freshwater ecosystem, temporal stability, multifunctionality
Citation: Xu Z, Yang H, Mao H, Peng Q, Yang S, Chou Q, Yang Y, Li Z and Wei L (2023) Environment and time drive the links between the species richness and ecosystem multifunctionality from multitrophic freshwater mesocosms. Front. Mar. Sci. 10:1125705. doi: 10.3389/fmars.2023.1125705
Received: 16 December 2022; Accepted: 29 March 2023;
Published: 20 April 2023.
Edited by:
Stelios Katsanevakis, University of the Aegean, GreeceReviewed by:
Yongjiu Cai, Nanjing Institute of Geography and Limnology, Chinese Academy of Sciences (CAS), ChinaChunhua Liu, Wuhan University, China
Copyright © 2023 Xu, Yang, Mao, Peng, Yang, Chou, Yang, Li and Wei. This is an open-access article distributed under the terms of the Creative Commons Attribution License (CC BY). The use, distribution or reproduction in other forums is permitted, provided the original author(s) and the copyright owner(s) are credited and that the original publication in this journal is cited, in accordance with accepted academic practice. No use, distribution or reproduction is permitted which does not comply with these terms.
*Correspondence: Zhongqiang Li, bGl6aHFAaHVidS5lZHUuY24=; Lifei Wei, d2VpbGlmZWkyNTA4QDE2My5jb20=