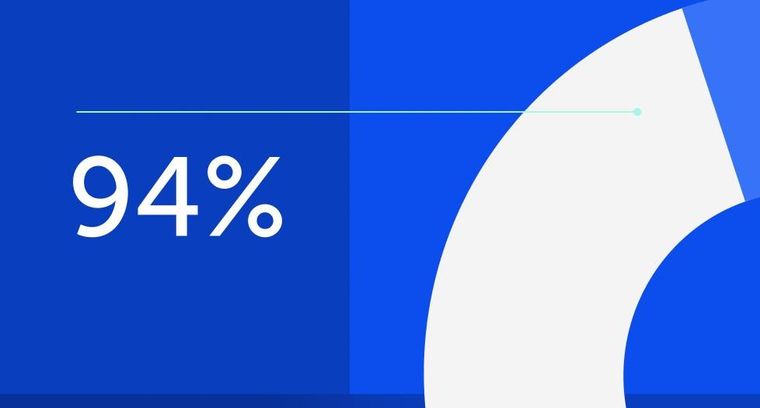
94% of researchers rate our articles as excellent or good
Learn more about the work of our research integrity team to safeguard the quality of each article we publish.
Find out more
ORIGINAL RESEARCH article
Front. Mar. Sci., 03 August 2023
Sec. Marine Biology
Volume 10 - 2023 | https://doi.org/10.3389/fmars.2023.1120695
This article is part of the Research TopicBiological Rhythms of Aquatic Organisms in a Changing ClimateView all 9 articles
The intertidal sea mussel Mytilus californianus inhabits the Pacific coastline of North America. As a sessile organism it must cope with daily fluctuations of the marine and terrestrial environments. Organisms in stressful environments are commonly faced with energetic trade-offs between somatic and reproductive growth and stress management. Although, this energetic theory is generally accepted for mussels as well, the spectrum of mechanisms underlying this framework have not been widely investigated. In the current study we hypothesized that mussels acclimated to a cyclical moderately warm aerial environment would display enhanced transcript abundance of genes related to metabolism and exhibit resilient digestive enzyme activity (energy acquisition). Following acclimation to simulated tidal regimes in the laboratory we observed higher gene-expression of citrate synthase (CS), citrate lyase (ACLY), and mammalian target of rapamycin (MTOR) in heat stressed mussels. The expression of CS and MTOR was not elevated under acute thermal stress, suggestive that repeated stress is required for robust expression of these genes given that all other environmental variables are constant. We also observed reduced activity of the digestive enzyme, amylase in heat-shocked acclimated mussels (a proxy for energy acquisition). Our results suggest that mussels that settle high on shore not only face the challenge of thermal stress repair and limited access to food but may also be compromised by reduced digestive performance. Mussels may have adapted to cyclical energetic stress by overexpressing particular energy-related genes that can mitigate the disturbance to energy balance once the abundant transcripts are translated into functional proteins.
The intertidal sea mussel Mytilus californianus form reef-like structures along the coastline of eastern Pacific shores from Baja California to Aleutian Islands of Alaska. These ubiquitous structures harbor hundreds of associated species and play an important role within coastal food webs of this region. As a sessile eurythermal marine ectotherm, M. californianus is exposed to the aerial environment during low-tide periods making it susceptible to thermal perturbations of physiological processes (Hofmann and Somero, 1995; Yao and Somero, 2012). The risk of environmental perturbation leading to injury or death of individuals and population decline has risen in recent years due to increasing average air-temperatures and more frequent extreme events resulting from global climate change (Helmuth et al., 2011). The 6th assessment from the Intergovernmental Panel on Climate Change stated that marine ecosystems have been negatively impacted by global climate change and environmental stress on animals will increase in the future. Toward this end, heat waves have decimated populations of mussels and associated invertebrates along specific regions of shore, such as high-shore intertidal zones lining the northwest coast of North America (Harley, 2008). Entire mussel beds have disappeared (observed in Southern California Bight), but extreme temperatures that occur acutely may not be the sole reason for such losses (Smith et al., 2006). Therefore, in order to finely predict how M. californianus will adjust to a changing environment, it is imperative to understand how mussels perform physiologically under aerial heat stress that causes sub-optimal performance while still allowing for compensatory adjustments to alleviate its impact (i.e. moderate versus extreme air-temperatures)—based upon concepts in (Pörtner and Farrell, 2008).
Physiological performance of M. californianus under acute or extreme temperature conditions in field and laboratory settings is well documented in the context of cellular physiology, metabolism, growth, and survival (Evans and Somero, 2010; Lockwood et al., 2010; Tomanek and Zuzow, 2010; Wang et al., 2018). Evolutionary processes allow organisms to respond to environmental change over long timescales, while physiological plasticity is a mechanism that yields performance over short-term fluctuations. Mussels and other intertidal bivalves that are acclimatized, acclimated, or acutely subjected to extreme temperatures display plastic phenotypes such as valve closure, aerobic metabolic depression, heart failure, and reduced feeding (Braby and Somero, 2006; Anestis et al., 2010; Dowd and Somero, 2013; He et al., 2022). At the cellular level, Mytilus spp. and other intertidal bivalves activate a molecular heat-shock response (HSR) following the onset of extreme aerial thermal stress—a function of temperature and time (Lockwood et al., 2010; Tomanek and Zuzow, 2010). In this context, gene-expression profiling is a tractable tool to monitor HSR temporally in bivalves (Gracey et al., 2008; Connor and Gracey, 2020). Genes expressed during extreme thermal conditions include those that synthesize heat-shock proteins, chaperonins, anti-apoptosis proteins, and more (Gracey et al., 2008; Payton et al., 2017; Connor and Gracey, 2020). The spectrum of heat-shock genes function together to stabilize proteins during protein-denaturing events and participate in refolding processes during onset stress and/or recovery when optimal environmental conditions return, known as proteostasis.
While some enzymes can resist damage at high temperatures (e.g. at or above lethal temperatures) (Lockwood and Somero, 2012), critical enzymes negatively affected by extreme thermal regimes either by destabilization or reduced gene-expression may include those related to digestion of food particles in the gut —digestive enzymes. For example, digestive enzyme activity in M. edulis increases in vitro with temperature to some level of denaturation then declines (Brock et al., 1986). This is considered a critical enzymatic condition that can be partially predicted for proteins through thermal deactivation experiments (Aymard and Belarbi, 2000). Observations of digestive enzyme activity allows for perception of energy acquisition strategies in facing environmental fluctuations (German et al., 2015). Despite its importance for optimal organismal performance, there are few studies focused on the effects of moderate aerial warming on digestive enzyme activity. To this point, a recent laboratory study showed lower carbohydrase activity (amylase) in mussels acclimated four weeks to low-tide moderate heat exposure (+9 °C) within a 12hr:12hr low-tide/high-tide cycle (Pham et al., 2023). However, a field study revealed that amylase activity in M. californianus was similar across microhabitats that differed in temperature and food availability (Connor et al., 2016). Therefore, further studies on environmental modulation of digestive enzymes in intertidal mussels will enhance our understanding of the impacts of environmental change on their long-term ecological success, especially when studied in conjunction with other phenotypes such as gene-expression.
While the behavior of HSR (protection) and the functional performance of digestive enzymes (energy acquisition) under extreme stress is somewhat predictable in mussels (i.e. robust HSR gene activation and lower digestive enzyme performance) (Khan et al., 2020), less is known about how these characteristics vary in intensity and time, to maintain homeostasis under daily moderate fluctuating environmental conditions (Sokolova et al., 2012). In this context, populations of M. californianus that reside high on the shore are subjected to twice-daily tides for weeks at a time throughout the year. As facultative anaerobes, Mytilus spp. close their valves for protection from desiccation and predation leading to reduced tissue oxygen concentrations and activation of anaerobic metabolic pathways—a low energy production state (De Zwaan, 1977; Shick et al., 1988; Connor and Gracey, 2012). Hence, aerial exposure is a complex multi-stressor environmental event that subjects mussels to functional-hypoxia and thermal perturbation simultaneously (Helmuth and Hofmann, 2001). To this end, we previously acclimated mussels to a twice-daily tidal sequence followed by a low-tide heat-shock (+7 °C), and tissues were analyzed for respiration metabolites in the current study. This analysis revealed an upregulation of the anaerobic metabolism marker, succinate, after low-tide heat-shock while the aerobic metabolism marker, citrate, was muted (Supplementary Figure 1). Under mixed semidiurnal tides, mussels can face aerial periods upwards of 20 hours and experience temperature differences of 20 degrees between water and air temperatures (Zetler and Flick, 1985; Helmuth and Hofmann, 2001). However, protracted aerial exposure periods and extremely elevated air temperatures do not typically occur simultaneously (Helmuth et al., 2002). Therefore, in this study we investigated the temporal expression of genes related to energy maintenance and digestive enzyme activity in mussels subjected to moderate conditions that occur more readily in nature than extreme conditions.
During periods of moderately warm air conditions, ectotherms are predicted to re-direct energy for growth (somatic and reproductive) toward thermal protection because ATP is required for both processes (Lindquist and Craig, 1988; Hawkins, 1991; Feder et al., 1992). For example, reduced growth rates in mussels acclimated/acclimatized to hot temperatures in laboratory and field settings have been reported (Fitzgerald-Dehoog et al., 2012; Connor and Robles, 2015). However, more recent biological theory suggests that the impact of energy trade-offs can be mitigated by plastic physiological adjustments (Thaler et al., 2012). In this context, Mytilus spp. exposed to air during low-tide close their shell-valves leading to functional hypoxia in the face of elevated air temperatures (Miller and Dowd, 2019). Within this framework, a recent microarray gene profiling study showed activated TCA-cycle related genes in M. californianus acclimated to an extensive day-time low-tide period combined with a moderate +8 °C heat-shock (Connor and Gracey, 2020). In a separate study, mussels exposed to twice-daily tides and constant cool temperature, did not show robust TCA-cycle expression suggestive that genes related to energy generation may be triggered by elevated temperatures—versus exclusively responding to energy demand (Connor and Gracey, 2011). Analyzed together, these studies revealed that temperature-cycles modulate metabolic genes temporally. However, differences in air-exposure periods and acclimation duration between studies, likely play a role in perceived thermal effects. Therefore, temporal studies designed to compare control and treatment groups can provide additional insight into thermal sensitivity of gene-expression in M. californianus.
In the current study we took a focused control versus treatment approach to test the ability of heat to trigger metabolic genes and modulate the digestion of starch by acclimating mussels to two tidal regimes that differed in temperature during low-tide (+10 °C) followed by temporal measurements of TCA-cycle related genes (citrate synthase, mitochondrial (CS) and citrate lyase (ACLY)) (Zaidi et al., 2012), mammalian target of rapamycin (MTOR), and heat-shock chaperone genes. Enzyme activity of the carbohydrase, amylase forms within the digestive gland. Citrate synthase, mitochondrial (CS) is the first enzyme in the TCA-cycle and serves as an important marker for aerobic metabolism. Citrate lyase (ACLY) is a key enzyme that links the mitochondria to fatty-acid synthesis. Mammalian target of rapamycin (MTOR) has a broad spectrum of functions that act to regulate metabolism. Amylase digests starch to glucose and responds more sensitively to temperature variation than other digestive enzymes in acclimated M. californianus (Pham et al., 2023). To evaluate differences between acclimation and acute effects, we also measured gene-expression of citrate synthase (CS), mTOR (MTOR) and digestive enzyme activity of amylase in mussels acutely warmed in air. We predicted that mussels acclimated to a moderate heat + tidal (8hr:16hr low-tide/high-tide cycle) regime for two weeks would trigger the expression of TCA-cycle related genes during low-tide versus acclimation to a cool but otherwise identical tidal regime. Furthermore, we hypothesized that digestive enzyme activity would not be negatively impacted by the cyclical thermal regime because its resilience is required to offset the energy demands of thermal stress recovery, at least within the short-term (see Pham et al. (2023) for the effects of long-term acclimation). Lastly, we hypothesized that repeated heat exposure rather than acute perturbations is essential for enhanced gene-expression of citrate synthase and homeostasis of amylase enzyme activity.
Mussels 63.03 ± 0.81 mm in length, 28.54 ± 0.32 mm in width, and 27.27 ± 0.95 g were collected from a mid-shore microhabitat within San Buenaventure State Beach in Ventura, CA during low-tide on 28 January, 2021. Individuals were brought into a laboratory space at the University of California, Irvine where epibionts were removed, and left to depurate in a 189L holding tank of 60:40 artificial: gravel filtered seawater within an environmental room set to 15°C for 24 hrs in constant light conditions. Mussels were then moved into an intertidal simulation system within the cold room.
The intertidal simulation system consisted of two replicate 3L tanks and a 178L sump. Water level within the replicate tanks was controlled by water pumps. Pumps within each tank transferred water to the sump to create low-tide conditions in the replicate tanks once per day. A sump-pump filled tanks to commence high-tide. The sump-pump remained on during high-tide conditions and the overflow of water supplied to each replicate tank circulated back to the sump [as described in Pham et al. (2023)]. The replicate tanks and the sump contained several turbulence water-pumps and air-stones to maintain gas exchange. The sump included, mechanical filtration, a protein skimmer, CO2 scrubber, and biological filtration to control for excess algae, protein abundance, low pH, and high nitrite and ammonia. The timing of tank component operations was controlled by a small computer (Neptune Systems; Morgan Hill, CA). Mussels were fed by adding 3 ml of liquid algae (Live Algae: Filter Feeder Formula; Algae Research Supply; Carlsbad, CA) to the sump at the onset of high-tide. For each replicate tank, low-tide began at 9am and lasted until a 5pm high-tide start. The control tank temperature did not vary and matched the cold room temperature of 15°C. Heated tanks were equipped with ceramic lamps that activated during each low-tide event using a temperature controller (Omega; Norwalk, CT). The heating event included a ramp period of 1.5°C/hour and a constant interval at 25°C until high-tide occurred (Figure 1). Mussels were acclimated to this sequence for 14 days in constant light rather than a L:D cycle as a means to isolate the effects of temperature. Following acclimation, mussels were sacrificed to collect gill, and digestive gland tissues every three hours beginning and ending at 9am and 9pm, respectively. The harvested tissues were stored at -80°C for later use. This design created ten groups: five groups from the cold (C) tank (9amC, 12pmC, 3pmC, 6pmC, 9pmC) and five groups from the hot (H) tank (9amH, 12pmH, 3pmH, 6pmH, 9pmH). A total of six mussels were sacrificed at each time point from each tank (Supplementary Figure 2). Gills and digestive glands were later used for gene-expression and digestive enzyme activity analyses respectively.
Figure 1 Simulated temperature regime. Mussels were placed into either a cold or hot tank. Temperatures were maintained at 15 °C in the cold tank. Air temps were raised to 25 °C at 1.5 °C/hr during low-tide in the hot tank. Low-tide occurred at 9am and high-tide returned at 5pm.
Following acclimation (final high-tide), mussels were transplanted from the control to the heated tank (N=6) and vice versa (N=5) while some were left in their original tank (cold N=6, hot N=4). These mussels were sacrificed after 22 hours (3pm on the next day) and gill tissues were removed and stored at -80°C. The gills were used for gene-expression analysis.
A subset of mussels (N=15) was moved directly from the holding tank into low-tide heat-exposure conditions. Mussels were equally distributed between three incubators set at 15°C, 18°C, and 22°C, respectively. Mussels were sacrificed after 2 hours, and gill and digestive gland tissues were removed and stored at -80°C. Gills and digestive glands were later used for gene-expression and digestive enzyme activity analyses, respectively.
Gill tissue was homogenized in Trizol (Qaigen; Germantown, MD) and RNA extracted using glass-fiber filters. A total of 1 ug RNA was used in a reverse transcription reaction (iScript cDNA Synthesis Kit; BioRad, Richmond, CA) to synthesize cDNA. A 1 ul volume of cDNA was used in a qPCR reaction with SYBR green (iTaq; BioRad) and a Bio-Rad CFX thermocycler. The PCR program used performed 40 cycles of denaturing (95°C), annealling (60°C), and extension (72°C). For qPCR reactions, 1 ul of 5 uM primers for citrate synthase (CS), citrate lyase (ACLY), mTOR (MTOR), Hsp73 (HSPA8), t-complex protein 1 (TCP1), and t-complex protein 1 subunit eta (CCT7) genes were used. Tubulin-alpha (TUBA1A) was used as the reference gene. Primers were designed using M. californianus annotated expressed sequence tags (ESTs) (Gracey et al., 2008) (Table 1). The EST sequences were annotated by alignment with orthologues using BLAST. We reported the top hit for model organisms (Table 1). A phylogenetic analysis between M. californianus and the model organisms was performed (Supplementary Figure 3). Gene-expression was calculated using the relative quantity method (2ΔCt) (target-reference) with bootstrapping (Pfaffl, 2007). For comparison, we also performed (2ΔCt-ΔCt) fold-change method without bootstrapping but included outlier removal of delta scores by boxplot (>1.5 IQR) followed by standardization (see methods of mean-centering and autoscaling in Willems et al. (2008)). Rescaling was performed with mean standard deviations of low-tide or high-tide autoscaled expression values (Willems et al., 2008). The 9am cold group was used as the control. Finally, an effort to measure gene expression in the digestive gland using homogenate or pelleted tissue was deployed. However, the preliminary results revealed discrepancies between the two forms and the effort was cancelled (Supplementary Figure 4).
Amylase protocols followed the Somoygi-Nelson method, as described by German and Bittong (2009). Briefly, digestive gland tissue was diluted in assay buffer (25 mol maleate and 1 mmol CaCl2 at pH 6.5) and homogenized on ice for 1 min. After centrifugation, the supernatant was aliquoted and stored in -80°C for later assay use. Sample tubes were made in duplicate by mixing 10 ul of homogenate with 50 ul assay buffer and 40 ul of 1:10 starch solution (Sigma Potato Starch) made fresh every time with assay buffer. Blank samples were made with 90 ul assay buffer and 10 ul homogenate. Samples were incubated at 20°C (low-tide temperature between cold and warm experimental tanks) for 30 minutes and the reaction stopped with 20 ul 1 N NaOH. A 200 ul volume of Reagent A was added and then the samples were boiled for 10 minutes and cooled on ice. Following incubation, 200 ul of Reagent B was added and then the samples were vortexed twice after a 5-min increment [Somoygi-Nelson reagent ingredients are described in German et al. (2004)]. A total 100 ul of sample was diluted in 300 ul NanoPure H2O and centrifuged at 8000 rpm for 5 minutes then plated in duplicate in clear-bottom 96 well plates. Absorbance was read at 650 nm in a microplate reader (BioTek Synergy H1 Hybrid Reader).
We deployed bootstrapping and randomization techniques to overcome the lack of a normal distribution in qPCR data and account for human, run-to-run, and reagent variation (Pfaffl et al., 2002). Bootstrapping (50 permutations) was performed for all genes and the randomized Cqs of the target genes were matched with randomized Cqs of the reference gene per group, and RQ was subsequently determined for each pair. The number of permutations used allowed for pairs to match at least twice in a group. Tubulin-alpha (TUBA1A) and target gene Cq scores were extrapolated to a final data set of n=500. A one-way ANOVA and Tukey’s (WSD) test was used to compare control vs. heated tanks between each other and at every time point. Tukey (WSD) test is a balance between Tukey (HSD) and Newman-Keuls tests. A one-way ANOVA with Tukey’s (HSD) was used to analyze amylase activity. For each test, a p-value <0.05 was considered significant.
All of the genes revealed differential expression resulting from a p<0.05 in each full factorial ANOVA. Similar trends were discovered using the fold-change method however significant expression of ACLY was not observed (Supplementary Table 1). Tukey’s test of citrate synthase (CS) expression revealed that mussels in the 6 pm hot-group had the highest expression (Figure 2A) and was significantly different than the 6 pm cold group. It was also distinct from 9am (C,H), 12pm (C,H), and 3pmC (Figure 2A; Supplemental Table 1). There was a >2-fold expression difference between the 9amC and 6pmH groups. Gene-expression was suppressed between 9am and 12pm in both tanks but began to rise subsequently. Tukey’s test revealed that the rise in expression during low-tide in the hot tank was marginally greater than expression in the cold tank. The mean expression at 3pm for the cold and hot tanks was 1.91 and 3.31 units, respectively. The area under curve by trapezoidal method for heat and cold treated mussels was 14.98 and 10.80 respectively. Citrate lyase (ACLY) was also differentially expressed (p<0.05) and showed an upward trend during warming that peaked after high-tide (Figure 2B). The area under curve by trapezoidal method for hot and cold treated mussels was 4.53 and 1.59 respectively. There was a significant difference between hot and cold treatments at 6pm only (≈3-fold) (p<0.05). The gene-expression of HSPA8 was flat during low-tide in both tanks, however it was induced >30-fold from 6pm to 9pm in the hot tank, while expression in the cold tank remained low (Figure 3A). Transcript abundance of chaperonin genes were also robustly expressed after high-tide (p<0.05) (Figure 3B). Both genes were expressed ≈10-fold at 6pm. The expression of t-complex protein 1 subunit eta (CCT7) was sustained at 9pm and only dropped 1% while t-complex protein 1 (TCP1) dropped 46%. Similar to the trend of high-tide activation, mTOR (MTOR) was muted during low-tide warming and robustly expressed (>5-fold) following high-tide, which was also significantly higher than expression in the cold tank at that time (Figure 4).
Figure 2 Tidal gene-expression in acclimated mussels. Mussels were acclimated for 15 days in an intertidal system in either a hot or cold regime. (A) Relative transcript abundance of citrate synthase (CS), and (B) citrate lyase (ACLY) in gill tissue. Values represent means ± 1SE. N=50 permuted values from original groups containing 6 animals each. Asterisks represent significant differences (p-value<0.05) between the hot and cold acclimated mussels at the associated time-point.
Figure 3 Tidal gene-expression in acclimated mussels. Mussels were acclimated for 15 days in an intertidal system in either a hot or cold regime. (A) Relative transcript abundance of heat-shock protein 73 (HSPA8) and (B) t-complex protein 1 (TCP1) and t-complex protein 1 subunit eta (CCT7) in gill tissue. Values represent means ± 1SE. N=50 permuted values from original groups containing 6 animals each. Asterisks represent significant differences (p-value<0.05) between the hot and cold acclimated mussels at the associated time-point.
Figure 4 Tidal gene-expression of mTOR (MTOR) in gill of acclimated mussels. Mussels were acclimated for 15 days in an intertidal system in either a hot or cold regime. Values represent means ± 1SE. N=50 permuted values from original groups containing 6 animals each. Asterisks represent significant differences (p-value<0.05) between the hot and cold acclimated mussels at the associated time-point.
Mussels subjected to acute heat stress or transplanted, did not show mean differences in expression of citrate synthase (CS) or mTOR (MTOR) between the experimental groups p>0.05 (Figures 5A, B). Similar results were found in the analysis of hsp73 (HSPA8) p>0.05 (Figures 6A, B).
Figure 5 Acute-heat gene-expression and effects of transplanting in gill. (A) Gene expression of citrate synthase (CS) and mTOR (MTOR); mussels from a benign subtidal laboratory environment were subjected to acute thermal stress. N=50 permuted values from original groups containing 5 animals each. (B) Mussels were transplanted back to their original treatment or across treatments. N=50 permuted values from original groups containing 4-6 animals each (see materials and methods). Boxplots represent the interquartile range and the whiskers represent 1.5* the interquartile range.
Figure 6 Acute-heat gene-expression and effects of transplanting in gill. (A) Gene expression of heat-shock protein 73 (HSPA8); mussels from a benign subtidal laboratory environment were subjected to acute thermal stress. N=50 permuted values from original groups containing 5 animals each. (B) Mussels were transplanted back to their original treatment or across treatments. N=50 permuted values from original groups containing 6 animals each. Boxplots represent the interquartile range, and the whiskers represent 1.5* the interquartile range.
One outlier was removed from the 3pm acclimated hot-group (identified by boxplot; >1.5 IQR). ANOVA revealed that amylase activity in at least one group was the significantly lower than all other groups (Figure 7A) p<0.05. The 9am hot-group was significantly lower than the 3pm cold-group. In general, amylase activity was lower in the hot acclimated group. Excluding the 3pm hot-group outlier, the mean value of amylase activity in the hot-group was 46% lower than that in the cold-group. No variation in amylase activity occurred between acute heat-shocked groups following the removal of outliers (Figure 7B).
Figure 7 Amylase activity in acclimated or acute heat-shocked mussels. (A) Mussels were acclimated for 15 days within an intertidal simulation system. Digestive glands were removed following acclimation and enzyme activity measured (N=6). (B) Mussels submerged in a cool environment (15 °C) were subjected to acute thermal shocks (N=5). Digestive gland was collected after the shocks and enzyme activity measured. Letters represent homogenized groups revealed by Tukey’s test. Boxplots represent the interquartile range, and the whiskers represent 1.5*the interquartile range.
In this study we hypothesized that mussels acclimated to cycles of a combined moderate aerial-exposure and +10 °C heat-shock (in constant light) would promote elevated expression of energy-production and regulation genes in M. californianus. Mytilus californianus closes its shell valves for protection during low-tide, leading to hypoxic conditions within its shell-cavity—functional hypoxia (Bayne et al., 1976). Interestingly, we observed citrate synthase (CS) transcripts increasingly rose during low-tide heat-stress, however this pattern was less dramatic in mussels subjected to an isothermal tidal condition. Citrate synthase catalyzes the first step of the TCA-cycle under aerobic oxidation of acetyl-CoA in mammalian model systems. As facultative anaerobes, Mytilus spp. turn on anaerobic metabolic pathways during low-tide functional-hypoxia (De Zwaan, 1977; Connor and Gracey, 2012) including when faced with moderate levels of aerial heat simultaneously (Supplementary Figure 1) (Kluytmans et al., 1978). Hence, it appears that there is an environmental-physiological mismatch between shell cavity environmental condition (hypoxic) and the core function of citrate synthase (CS)—promotion of oxidative respiration. Therefore, the initiation of citrate synthase (CS) during low-tide functional-hypoxia may prime mussels for aerobic respiration during high-tide. Although it is generally assumed that interactions between environment and gene-expression are characterized as stimuli-response coupling (i.e. symmetrical response), a study in yeast first-revealed asymmetrical responses in fluctuating environments—stimulation of physiological processes in one environment enhances the ability to cope with a subsequent prevailing condition (Mitchell et al., 2009). To the best of our knowledge no study has directly tested asymmetrical gene-expression responses in mussels. Here, we observed a trend of increasing expression of citrate synthase (CS) that initiated during low-tide warming until one hour into high-tide after which it decreased. It is possible that the rate of translation increased, or that gene-expression decreased during high-tide in response to citrate synthase protein concentration meeting the energetic demands of stress-recovery. Similarly, Connor and Gracey (2020) observed that several TCA-cycle genes (aconitate hydratase, malate dehydrogenase, succinyl-CoA ligase, isocitrate dehydrogenase) were upregulated following four weeks of daily warming (+8 °C) combined with extreme aerial exposure (20 hrs). Although, citrate synthase (CS) was not rhythmically expressed in that study, the range of its expression was similar to the other differentially expressed TCA-cycle genes which were identified as rhythmic rather than stochastic (unpublished data).
It is difficult to compare our findings to other bivalve temporal-based studies due to the multivariable nature of simulated tides. Some of the variables that should be considered for comparison include respiratory state, acclimation time, air-exposure duration, water-temperature, aerial heat-rate, max-air temperature, sampling resolution, sampling time-point, L:D cycle, and nutrients. Within this framework Andrade et al. (2019) subjected M. galloprovincialis to cycles of air exposure at two isothermal conditions (18 °C and 21°C) and revealed lower Electron Transport System activity in warmer acclimated mussels. Nancollas and Todgham, (2022) acclimated M. californianus to cycles of heat (6 hr:6 hr, emersion:immersion; +7 °C heat-shock every daytime low-tide) and found evidence of lowered anaerobic metabolism in warm versus cold acclimated mussels. These studies show the complexities of identifying the molecular underpinnings of energetic homeostasis in Mytilus spp. through comparative tidal simulations. Nevertheless, elevated temperature and hypoxia affect the function of mitochondria; therefore, there may be a link between citrate synthase gene-expression and environmentally perturbed mitochondria (Sokolova, 2018). It is important to consider that the regulation of citrate synthase (CS) gene-expression is not well understood in animal models (reviewed in Gaster et al., 2012).
In the current study, the observation of elevated expression of citrate synthase (CS) following acclimation to a more moderate tidal environment versus extreme conditions, highlights the environmental sensitivity of this phenotype in mussels. In support of the notion that acclimation to temperature cycles is required for elevated citrate synthase (CS) gene-expression, we found that acute heat-shocks of +3 °C or +7 °C did not enhance expression within the first two hours post heat-shock, compared to the control group. In line with our findings, Lesser (2016) revealed elevated citrate synthase enzyme activity in M. edulis acclimated to daily cycles of aerial heat (+10 °C). Alternatively, Dowd and Somero (2013) showed no change in citrate synthase enzyme activity in M. californianus over an acute tidal regime that also subjected individuals to aerial heat energy (+8 to +23 °C). Similarly, a previous report also showed that citrate synthase enzyme activity in M. galloprovincialis was not affected by acute moderate heat-shock (Collins et al., 2020). Further support for acclimation driven expression is shown through our finding that, cold acclimated mussels did not display enhanced citrate synthase (CS) gene-expression at 3pm following warming that initiated at 9am. However, the warm acclimated transplanted mussels also did not show differential expression at 3pm. This could be correspondent to more variation in the strength of the response between the beginning of acclimation versus the later responses. An alternative explanation is that mussels may have responded (up or down-regulation) to a slight change in the heating regime due to tank handling. Also, the sample size in the hot-to-hot group was smaller than all other groups due to animal loss in the hot tank, possibly limiting the observation of variation in that group.
The citrate lyase (ACLY) gene-expression pattern observed was similar to that of citrate synthase (CS). Transcript abundance in warm acclimated mussels appeared to elevate during low-tide versus no response in mussels subjected to cool conditions. Additionally, transcript abundance peaked after onset of submergence suggestive that heat may have induced the stronger response. In agreement with this finding, Connor and Gracey (2020) revealed an upregulation of citrate lyase (ACLY) in aerial-heat acclimated mussels (see a description of the study above). Citrate lyase is a key step in de novo fatty-acid synthesis, which is a reductive set of processes that can occur during hypoxic or normoxic conditions in mammals (Beckner et al., 2010). Regardless, a generous supply of electrons and ATP is required for fatty-acid biosynthesis, both of which may not be accessible during low-tide aerial-exposure. This model aligns with our finding that peak expression of citrate lyase (ACLY) occurred during submergence in coordination with active oxidative phosphorylation. A possible explanation for initiation of expression during low-tide is that citrate lyase enzymatically generates oxaloacetate which may participate in cytosolic steps of the glucose-succinate anaerobic pathway (Tielens et al., 2002); however, this pathway has not been experimentally demonstrated in mussels. An additional possibility is that the acetyl-coA generated from citrate lyase activity can participate in acetylation of histones (Chypre et al., 2012) in line with previous observations that the expression of transcription regulatory genes (JUN, FOSL2, and CEBPE) are upregulated during low-tide periods and further enhanced when low-tide aerial temperatures rise (Evans and Somero, 2010; Connor and Gracey, 2011). Importantly, Dunphy et al. (2015) revealed a marked abundance of palmitic acid, the first product of fatty-acid synthesis, in mussel Perna canaliculus following heat-stress. In this context, elevated heat can disrupt the integrity of phospholipid containing cellular membranes in bivalves requiring reestablishment though fatty-acid synthesis during recovery (Zhang and Dong, 2021; Zhang et al., 2023).
This study also investigated the effects of the simulated tidal environment on HSR. As expected, chaperone gene-expression was robustly elevated under warm conditions, similarly to previous studies of heat-shock responses in intertidal bivalves exposed to warm air or water in laboratory settings (Lockwood et al., 2010; Connor and Gracey, 2011; Zhang et al., 2012). Unique to the current study, is the observation that peak abundance of heat-shock protein 73 (HSPA8) occurred hours after maximum aerial temperature was reached and during submergence. It is important to note that Connor and Gracey (2020) observed non-rhythmic expression of HSP-70B (reannotated here as HSPA8) in mussels acclimated to lengthy low-tide periods combined with a daily +8 °C daytime heat-shock. Although the authors did not observe rhythmicity in HSPA8 gene-expression, transcript abundance oscillated over the two-day time course (unpublished data). In line with the current study, Gracey et al. (2008) observed that HSPA8 was higher in field mussels residing in a warmer high-shore environment compared to mussels situated low on the shore. The initiation of expression occurred on day two of a three-day time course in response to increasingly longer aerial exposure times as semidiurnal tides transitioned to mixed semidiurnal tides coinciding with shifting air temperatures. Heat-shock protein 73 (HSPA8) also responds to acute temperature stress, however peak expression may occur hours after exposure to heat-shock (Lockwood et al., 2010; Connor and Gracey, 2011; O’Brien et al., 2021). In line with this reasoning, we did not find differential expression of (HSPA8) in acutely shocked mussels. These previous studies suggest that its expression is triggered by a range of complex environmental variables. Interestingly, the expression of chaperonins t-complex protein 1 (TCP1) and t-complex protein 1 subunit eta (CCT7) was relatively maintained over the course of low-tide heat-stress but peaked during high-tide. A possible explanation for this finding is that chaperonins play an important role in folding newly synthesized proteins requiring its constant expression across stressful environmental conditions that may induce protein turnover. These findings are in line with the general understanding that some components of HSR are pronounced during stress, while others are more important for heat-shock recovery (Connor and Gracey, 2020).
Mammalian target of rapamycin (MTOR) is a regulator of a compendium of molecular processes within cells, including stress management. Autophagy is a conserved molecular pathway that leads to the degradation of damaged organelles and proteins within lysosomes during stress. Phosphorylated mTOR complexes halt autophagy and promotes growth. In line with the inference that heat stress can lead to cellular damage and a possible change in the balance between anabolic and catabolic pathways, we observed higher expression of MTOR in warm acclimated mussels. The mTOR stress response is not well studied in molluscs, however it is important to highlight findings in Sforzini et al. (2018) that revealed dephosphorylation and inactivation of mTOR complexes in chemically stressed mussels leading to increased autophagy and reduced growth activity. While no previous study has focused on the gene-expression of mTOR in M. californianus under aerial-warming, a field-based microarray study of acclimatized mussels revealed expression of autophagy and protein turnover-related genes during thermal stress (Gracey et al., 2008). However, it is important to note that MTOR was not a target gene on the microarray platform used in that study. Finally, previous studies showed upregulation of MTOR orthologues under heat stress in aerial and submerged conditions in oyster (Clark et al., 2013; He et al., 2023) and shrimp (Liang et al., 2020), highlighting the importance of its role in regulation of metabolism in marine invertebrate models.
In this study we asked whether mussels possess strategies to mitigate energetic stress related to ATP demands, generated from heat recovery, through resilience of digestive enzyme activity. Interestingly, we observed lower amylase activity in mussels challenged to daily but not acute heat stress. Therefore, chronic moderate temperatures may negatively impact mussels’ ability to digest starch and disrupt their energetic balance. There is an energetic trade-off between growth (somatic and reproductive) and protection in most organisms (Zera and Harshman, 2001). In line with this concept, mussels subjected to elevated temperatures grow slower than mussels in cool habitats (Fitzgerald-Dehoog et al., 2012; Connor and Robles, 2015). However, empirical evidence for the mechanisms supporting these findings are minimal (Petes et al., 2007). Assuming the existence of functional trade-offs in mussels, it is logical to assume that reduced ability to take advantage of ingested food particles due to lowered digestion performance may impact trade-offs in a variety of ways during heat-stress and lead to reduce growth. Within this prism, Albentosa et al. (2012) showed a positive correlation between growth and digestive enzyme activity, including amylase. Also, Fitzgerald-Dehoog et al. (2012) found that growth can be recovered/mitigated by increased food presentation in heat-stressed mussels (Fitzgerald-Dehoog et al., 2012). Importantly, Petes et al. (2007), reported a trade-off between protection of gametes from heat related oxidative stress and the impairment of energy available for gonadal growth. These results are in line with a recent report which showed a decrease in amylase activity in M. californianus subjected to (12hr:12hr tides and +9 °C), supporting the inference that elevated temperature has a negative effect on the acquisition of fuel that modulates total available energy.
There is a range of possibilities for the observed reduced digestive enzyme activity in warm acclimated mussels. Gene-expression of amylase may have been reduced during aerial warming. To this end, amylase protein levels are associated with gene-expression in oysters (Huvet et al., 2003) and bivalves subjected to elevated heat show reduced total mRNA levels (Lesser, 2016; Feidantsis et al., 2020). Elevated heat may have initiated denaturing of amylase; however, a thermal activation study for M. californianus has not been conducted at this time. Rather, Brock et al. (1986) found that the breakpoint temperature of amylase in M. edulis is 24°C. Furthermore, Mytilus spp. digest extra- and intracellularly, and possess an extensive endolysosomal system comprised of heterolysosomes and residual bodies to digest food within the digestive gland. Previous studies revealed that membrane stability of lysosomes within the digestive gland is disturbed following heat-stress (Moore, 1976; Tremblay and Pellerin-Massicotte, 1997). It is also noteworthy that there is a relationship between lysosomal stability and growth (Bayne et al., 1979). Heat exposure can modulate valve gape in mussels which in turn can affect feeding and digestion (Anestis et al., 2007). However, we did not assess valve-gape in the current study. Finally, filtration rate is positively correlated with intake, and May et al. (2021) showed a decrease of filtration rates in M. californianus following acute heat-shock in mussels acclimated to combined high-food concentrations and extreme aerial heat (30 °C).
Mytilus californianus is an ecological engineer established along the western coasts of North America. Understanding its physiology is crucial for predicting its future success under a changing climate. As a sessile species, its energetics is modulated by the prevailing environment. The intertidal environment is energetically stressful, due to bouts of aerial heat, functional hypoxia, and limited periods of feeding. Thus, energy acquired from the environment must be distributed in a manner that firstly promotes maintenance and survival, and secondarily committed to somatic and reproductive growth. It is possible that M. californianus may ameliorate these heat trade-offs by upregulating genes that provide energy and minimize the negative effects of heat stress on overall energy balance. Simultaneously, energy balance may be challenged due to reduced digestive enzyme activity and the ability to acquire nutrients (e.g., glucose). We do not know what mechanisms drive the thermally responsive genes in mussels though evidence from eukaryotic studies suggest temperature sensitive transcription factors bind to DNA binding motifs upstream of gene promoter regions. Transcription factors known to integrate environmental fluctuations and gene-expression in mammals include HIF1, heat-shock factors and clock proteins. Gene and protein expression studies revealed that these transcription factors are modulated or adapted to cyclic heat and tides in bivalves (Buckley et al., 2001; Kawabe and Yokoyama, 2012; Chapman et al., 2020) and other invertebrates (O’Neill et al., 2015; Schnytzer et al., 2018). Chromatin immunoprecipitation studies are required to identify which genes are targets of these transcription factors. Finally, further work is needed to explore whether protein abundance of energy-generating genes is elevated following aerial warming acclimation. Also, measurements of these markers under several variations of the acute heat shock regime could help to better understand differences between acute and acclimation physiology in bivalves.
The raw data supporting the conclusions of this article will be made available by the authors, without undue reservation.
KC is the principal investigator and performed manuscript prep., study design, and provided funding. AG formulated inferences, edited the manuscript, and partially funded the project. GC performed all of the laboratory experiments, analyses, graphs, and manuscript prep. ES performed phylogenetic analysis. BW performed gene-expression analysis. All authors contributed to the article and approved the submitted version.
This project was funded by the University of California, Irvine as a part of start up funds (to KC). A very small portion was funded by the National Science Foundation IOS 0745451 (to AG).
We thank Helen H. Hong for laboratory support.
The authors declare that the research was conducted in the absence of any commercial or financial relationships that could be construed as a potential conflict of interest.
All claims expressed in this article are solely those of the authors and do not necessarily represent those of their affiliated organizations, or those of the publisher, the editors and the reviewers. Any product that may be evaluated in this article, or claim that may be made by its manufacturer, is not guaranteed or endorsed by the publisher.
The Supplementary Material for this article can be found online at: https://www.frontiersin.org/articles/10.3389/fmars.2023.1120695/full#supplementary-material
Albentosa M., Sánchez-Hernández M., Campillo J. A., Moyano F. J. (2012). Relationship between physiological measurements (SFG-scope for growth-) and the functionality of the digestive gland in mytilus galloprovincialis. Comp. Biochem. Physiol. Part A Mol. Integr. Physiol. 163, 286–295. doi: 10.1016/j.cbpa.2012.07.019
Andrade M., De Marchi L., Soares A. M. V. M., Rocha R. J. M., Figueira E., Freitas R. (2019). Are the effects induced by increased temperature enhanced in mytilus galloprovincialis submitted to air exposure? Sci. total Environ. 647, 431–440.
Anestis A., Lazou A., Pörtner H. O., Michaelidis B. (2007). Behavioral, metabolic, and molecular stress responses of marine bivalve mytilus galloprovincialis during long-term acclimation at increasing ambient temperature. Am. J. Physiol. Integr. Comp. Physiol. 293, R911–R921. doi: 10.1152/ajpregu.00124.2007
Anestis A., Pörtner H. O., Michaelidis B. (2010). Anaerobic metabolic patterns related to stress responses in hypoxia exposed mussels mytilus galloprovincialis. J. Exp. Mar. Bio. Ecol. 394, 123–133. doi: 10.1016/j.jembe.2010.08.008
Aymard C., Belarbi A. (2000). Kinetics of thermal deactivation of enzymes: a simple three parameters phenomenological model can describe the decay of enzyme activity, irrespectively of the mechanism. Enzyme Microb. Technol. 27, 612–618. doi: 10.1016/S0141-0229(00)00258-1
Bayne B. L., Bayne C. J., Carefoot T. C., Thompson R. J. (1976). The physiological ecology of mytilus californianus Conrad. Oecologia 22, 229–250. doi: 10.1007/BF00344794
Bayne B. L., Moore M. N., Widdows J., Livingstone D. R., Salkeld P. (1979). Measurement of the responses of individuals to environmental stress and pollution: studies with bivalve molluscs. Philos. Trans. R. Soc London. B Biol. Sci. 286, 563–581. doi: 10.1098/rstb.1979.0046
Beckner M. E., Fellows-Mayle W., Zhang Z., Agostino N. R., Kant J. A., Day B. W., et al. (2010). Identification of ATP citrate lyase as a positive regulator of glycolytic function in glioblastomas. Int. J. Cancer 126, 2282–2295. doi: 10.1002/ijc.24918
Braby C. E., Somero G. N. (2006). Following the heart: temperature and salinity effects on heart rate in native and invasive species of blue mussels (genus mytilus). J. Exp. Biol. 209, 2554–2566. doi: 10.1242/jeb.02259
Brock V., Kennedy V. S., Brock A. (1986). Temperature dependency of carbohydrase activity in the hepatopancreas of thirteen estuarine and coastal bivalve species from the north American east coast. J. Exp. Mar. Bio. Ecol. 103, 87–101. doi: 10.1016/0022-0981(86)90134-6
Buckley B. A., Owen M.-E., Hofmann G. E. (2001). Adjusting the thermostat: the threshold induction temperature for the heat-shock response in intertidal mussels (genus mytilus) changes as a function of thermal history. J. Exp. Biol. 204, 3571–3579. doi: 10.1242/jeb.204.20.3571
Chapman E. C., Bonsor B. J., Parsons D. R., Rotchell J. M. (2020). Influence of light and temperature cycles on the expression of circadian clock genes in the mussel mytilus edulis. Mar. Environ. Res. 159, 104960. doi: 10.1016/j.marenvres.2020.104960
Chypre M., Zaidi N., Smans K. (2012). ATP-citrate lyase: a mini-review. Biochem. Biophys. Res. Commun. 422, 1–4. doi: 10.1016/j.bbrc.2012.04.144
Clark M. S., Thorne M. A. S., Amaral A., Vieira F., Batista F. M., Reis J., et al. (2013). Identification of molecular and physiological responses to chronic environmental challenge in an invasive species: the p acific oyster, c rassostrea gigas. Ecol. Evol. 3, 3283–3297. doi: 10.1002/ece3.719
Collins C. L., Burnett N. P., Ramsey M. J., Wagner K., Zippay M. L. (2020). Physiological responses to heat stress in an invasive mussel mytilus galloprovincialis depend on tidal habitat. Mar. Environ. Res. 154, 104849. doi: 10.1016/j.marenvres.2019.104849
Connor K. M., Gracey A. Y. (2011). Circadian cycles are the dominant transcriptional rhythm in the intertidal mussel mytilus californianus. Proc. Natl. Acad. Sci. 108, 16110–16115. doi: 10.1073/pnas.1111076108
Connor K. M., Gracey A. Y. (2012). High-resolution analysis of metabolic cycles in the intertidal mussel mytilus californianus. Am. J. Physiol. Integr. Comp. Physiol. 302, R103–R111. doi: 10.1152/ajpregu.00453.2011
Connor K., Gracey A. Y. (2020). Cycles of heat and aerial-exposure induce changes in the transcriptome related to cell regulation and metabolism in mytilus californianus. Mar. Biol. 167.9, 1–12. doi: 10.1007/s00227-020-03750-6
Connor K. M., Robles C. D. (2015). Within-site variation of growth rates and terminal sizes in mytilus californianus along wave exposure and tidal gradients. Biol. Bull. 228, 39–51. doi: 10.1086/BBLv228n1p39
Connor K. M., Sung A., Garcia N. S., Gracey A. Y., German D. P. (2016). Modulation of digestive physiology and biochemistry in mytilus californianus in response to feeding level acclimation and microhabitat. Biol. Open 5, 1200–1210. doi: 10.1242/bio.019430
De Zwaan A. (1977). Anaerobic energy metabolism in bivalve molluscs. Ocean. Mar. Biol. Annu. Rev. 15, 103–187.
Dowd W. W., Somero G. N. (2013). Behavior and survival of mytilus congeners following episodes of elevated body temperature in air and seawater. J. Exp. Biol. 216, 502–514. doi: 10.1242/jeb.076620
Dunphy B. J., Watts E., Ragg N. L. C. (2015). Identifying thermally-stressed adult green-lipped mussels (Perna canaliculus gmeli) via metabolomic profiling. Am. Malacological Bull. 33.1, 127–135.
Evans T. G., Somero G. N. (2010). Phosphorylation events catalyzed by major cell signaling proteins differ in response to thermal and osmotic stress among native (Mytilus californianus and mytilus trossulus) and invasive (Mytilus galloprovincialis) species of mussels. Physiol. Biochem. Zool. 83, 984–996. doi: 10.1086/656192
Feder J. H., Rossi J. M., Solomon J., Solomon N., Lindquist S. (1992). The consequences of expressing hsp70 in drosophila cells at normal temperatures. Genes Dev. 6, 1402–1413. doi: 10.1101/gad.6.8.1402
Feidantsis K., Giantsis I. A., Vratsistas A., Makri S., Pappa A.-Z., Drosopoulou E., et al. (2020). Correlation between intermediary metabolism, hsp gene expression, and oxidative stress-related proteins in long-term thermal-stressed mytilus galloprovincialis. Am. J. Physiol. Integr. Comp. Physiol. 319, R264–R281. doi: 10.1152/ajpregu.00066.2020
Fitzgerald-Dehoog L., Browning J., Allen B. J. (2012). Food and heat stress in the California mussel: evidence for an energetic trade-off between survival and growth. Biol. Bull. 223, 205–216. doi: 10.1086/BBLv223n2p205
Gaster M., Nehlin J. O., Minet A. D. (2012). Impaired TCA cycle flux in mitochondria in skeletal muscle from type 2 diabetic subjects: marker or maker of the diabetic phenotype? Arch. Physiol. Biochem. 118, 156–189. doi: 10.3109/13813455.2012.656653
German D. P., Bittong R. A. (2009). Digestive enzyme activities and gastrointestinal fermentation in wood-eating catfishes. J. Comp. Physiol. B 179, 1025–1042. doi: 10.1007/s00360-009-0383-z
German D. P., Horn M. H., Gawlicka A. (2004). Digestive enzyme activities in herbivorous and carnivorous prickleback fishes (Teleostei: stichaeidae): ontogenetic, dietary, and phylogenetic effects. Physiol. Biochem. Zool. 77, 789–804. doi: 10.1086/422228
German D. P., Sung A., Jhaveri P., Agnihotri R. (2015). More than one way to be an herbivore: convergent evolution of herbivory using different digestive strategies in prickleback fishes (Stichaeidae). Zoology 118, 161–170. doi: 10.1016/j.zool.2014.12.002
Gracey A. Y., Chaney M. L., Boomhower J. P., Tyburczy W. R., Connor K., Somero G. N. (2008). Rhythms of gene expression in a fluctuating intertidal environment. Curr. Biol. 18, 1501–1507. doi: 10.1016/j.cub.2008.08.049
Harley C. D. G. (2008). Tidal dynamics, topographic orientation, and temperature-mediated mass mortalities on rocky shores. Mar. Ecol. Prog. Ser. 371, 37–46. doi: 10.3354/meps07711
Hawkins A. J. S. (1991). Protein turnover: a functional appraisal. Funct. Ecol. 5 (2), 222–233. doi: 10.2307/2389260
He G., Xiong X., Peng Y., Yang C., Xu Y., Liu X., et al. (2023). Transcriptomic responses reveal impaired physiological performance of the pearl oyster following repeated exposure to marine heatwaves. Sci. Total Environ. 854, 158726. doi: 10.1016/j.scitotenv.2022.158726
He G., Zou J., Liu X., Liang F., Liang J., Yang K., et al. (2022). Assessing the impact of atmospheric heatwaves on intertidal clams. Sci. Total Environ. 841, 156744. doi: 10.1016/j.scitotenv.2022.156744
Helmuth B., Harley C. D. G., Halpin P. M., O’Donnell M., Hofmann G. E., Blanchette C. A. (2002). Climate change and latitudinal patterns of intertidal thermal stress. Science 80). 298, 1015–1017. doi: 10.1126/science.1076814
Helmuth B. S. T., Hofmann G. E. (2001). Microhabitats, thermal heterogeneity, and patterns of physiological stress in the rocky intertidal zone. Biol. Bull. 201, 374–384. doi: 10.2307/1543615
Helmuth B., Yamane L., Lalwani S., Matzelle A., Tockstein A., Gao N. (2011). Hidden signals of climate change in intertidal ecosystems: what (not) to expect when you are expecting. J. Exp. Mar. Bio. Ecol. 400, 191–199. doi: 10.1016/j.jembe.2011.02.004
Hofmann G., Somero G. (1995). Evidence for protein damage at environmental temperatures: seasonal changes in levels of ubiquitin conjugates and hsp70 in the intertidal mussel mytilus trossulus. J. Exp. Biol. 198, 1509–1518. doi: 10.1242/jeb.198.7.1509
Huvet A., Daniel J.-Y., Quere C., Dubois S., Prudence M., Van Wormhoudt A., et al. (2003). Tissue expression of two α-amylase genes in the pacific oyster crassostrea gigas. Effects two different Food rations. Aquacult. 228, 321–333. doi: 10.1016/S0044-8486(03)00323-5
Kawabe S., Yokoyama Y. (2012). Role of hypoxia-inducible factor α in response to hypoxia and heat shock in the pacific oyster crassostrea gigas. Mar. Biotechnol. 14, 106–119. doi: 10.1007/s10126-011-9394-3
Khan F. U., Hu M., Kong H., Shang Y., Wang T., Wang X., et al. (2020). Ocean acidification, hypoxia and warming impair digestive parameters of marine mussels. Chemosphere 256, 127096. doi: 10.1016/j.chemosphere.2020.127096
Kluytmans J. H., Van Graft M., Janus J., Pieters H. (1978). Production and excretion of volatile fatty acids in the sea musselMytilus edulis l. J. Comp. Physiol. 123, 163–167. doi: 10.1007/BF00687845
Lesser M. P. (2016). Climate change stressors cause metabolic depression in the blue mussel, mytilus edulis, from the gulf of Maine. Limnol. Oceanogr. 61, 1705–1717. doi: 10.1002/lno.10326
Liang Q., Ou M., Li Z., Ren Y., Wei W., Qiao X., et al. (2020). Functional analysis target of rapamycin (TOR) on the penaeus vannamei in response to acute low temperature stress. Fish Shell. Immunol. 96, 53–61. doi: 10.1016/j.fsi.2019.11.070
Lindquist S., Craig E. A. (1988). The heat-shock proteins. Annu. Rev. Genet. 22, 631–677. doi: 10.1146/annurev.ge.22.120188.003215
Lockwood B. L., Sanders J. G., Somero G. N. (2010). Transcriptomic responses to heat stress in invasive and native blue mussels (genus mytilus): molecular correlates of invasive success. J. Exp. Biol. 213, 3548–3558. doi: 10.1242/jeb.046094
Lockwood B. L., Somero G. N. (2012). Functional determinants of temperature adaptation in enzymes of cold-versus warm-adapted mussels (Genus mytilus). Mol. Biol. Evol. 29, 3061–3070. doi: 10.1093/molbev/mss111
May M. A., Feezell M. K., Gonzalez S. J., Vasquez M. C., Todgham A. E., Tomanek L. (2021). Sirtuin-dependent recovery from aerial heat shock: The effects of food ration, thermal history, and sirtuin inhibition on clearance rates and valve gape activity of the california mussel, mytilus californianus (Conrad). J. Exp. Mar. Biol. Ecol. 536, 151510.
Miller L. P., Dowd W. W. (2019). Repeatable patterns of small-scale spatial variation in intertidal mussel beds and their implications for responses to climate change. Comp. Biochem. Physiol. Part A Mol. Integr. Physiol. 236, 110516. doi: 10.1016/j.cbpa.2019.06.016
Mitchell A., Romano G. H., Groisman B., Yona A., Dekel E., Kupiec M., et al. (2009). Adaptive prediction of environmental changes by microorganisms. Nature 460, 220. doi: 10.1038/nature08112
Moore M. N. (1976). Cytochemical demonstration of latency of lysosomal hydrolases in digestive cells of the common mussel, mytilus edulis, and changes induced by thermal stress. Cell Tissue Res. 175, 279–287. doi: 10.1007/BF00218706
Nancollas S. J., Todgham A. E. (2022). The influence of stochastic temperature fluctuations in shaping the physiological performance of the california mussel, mytilus californianus. J. Exp. Biol. 225, 14jeb243729.
O’Brien C. J., Hong H. C., Bryant E. E., Connor K. M. (2021). The observation of starch digestion in blue mussel mytilus galloprovincialis exposed to microplastic particles under varied food conditions. PloS One 16, e0253802. doi: 10.1371/journal.pone.0253802
O’Neill J. S., Lee K. D., Zhang L., Feeney K., Webster S. G., Blades M. J., et al. (2015). Metabolic molecular markers of the tidal clock in the marine crustacean Eurydice pulchra. Curr. Biol. 25, R326–R327. doi: 10.1016/j.cub.2015.02.052
Payton L., Perrigault M., Hoede C., Massabuau J.-C., Sow M., Huvet A., et al. (2017). Remodeling of the cycling transcriptome of the oyster crassostrea gigas by the harmful algae alexandrium minutum. Sci. Rep. 7, 3480. doi: 10.1038/s41598-017-03797-4
Petes L. E., Menge B. A., Murphy G. D. (2007). Environmental stress decreases survival, growth, and reproduction in new Zealand mussels. J. Exp. Mar. Bio. Ecol. 351, 83–91. doi: 10.1016/j.jembe.2007.06.025
Pfaffl M. W., Horgan G. W., Dempfle L. (2002). Relative expression software tool (REST©) for group-wise comparison and statistical analysis of relative expression results in real-time PCR. Nucleic Acids Res. 30, e36–e36. doi: 10.1093/nar/30.9.e36
Pham T., Hong H. C., Swig B., German D. P., Connor K. M. (2023). Elevated aerial temperature modulates digestive enzyme activities in mytilus californianus. Comp. Biochem. Physiol. Part B Biochem. Mol. Biol. 265, 110825. doi: 10.1016/j.cbpb.2022.110825
Pörtner H. O., Farrell A. P. (2008). Physiology and climate change. Science 322, 690–692. doi: 10.1126/science.1163156
Schnytzer Y., Simon-Blecher N., Li J., Waldman Ben-Asher H., Salmon-Divon M., Achituv Y., et al. (2018). Tidal and diel orchestration of behaviour and gene expression in an intertidal mollusc. Sci. Rep. 8, 4917. doi: 10.1038/s41598-018-23167-y
Sforzini S., Moore M. N., Oliveri C., Volta A., Jha A., Banni M., et al. (2018). Role of mTOR in autophagic and lysosomal reactions to environmental stressors in molluscs. Aquat. Toxicol. 195, 114–128. doi: 10.1016/j.aquatox.2017.12.014
Shick J. M., Widdows J., Gnaiger E. (1988). Calorimetric studies of behavior, metabolism and energetics of sessile intertidal animals. Am. Zool. 28, 161–181. doi: 10.1093/icb/28.1.161
Smith J. R., Fong P., Ambrose R. F. (2006). Dramatic declines in mussel bed community diversity: response to climate change? Ecology 87, 1153–1161. doi: 10.1890/0012-9658(2006)87[1153:DDIMBC]2.0.CO;2
Sokolova I. (2018). Mitochondrial adaptations to variable environments and their role in animals’ stress tolerance. Integr. Comp. Biol. 58, 519–531. doi: 10.1093/icb/icy017
Sokolova I. M., Frederich M., Bagwe R., Lannig G., Sukhotin A. A. (2012). Energy homeostasis as an integrative tool for assessing limits of environmental stress tolerance in aquatic invertebrates. Mar. Environ. Res. 79, 1–15. doi: 10.1016/j.marenvres.2012.04.003
Thaler J. S., McArt S. H., Kaplan I. (2012). Compensatory mechanisms for ameliorating the fundamental trade-off between predator avoidance and foraging. Proc. Natl. Acad. Sci. 109, 12075–12080. doi: 10.1073/pnas.1208070109
Tielens A. G. M., Rotte C., van Hellemond J. J., Martin W. (2002). Mitochondria as we don’t know them. Trends Biochem. Sci. 27, 564–572. doi: 10.1016/S0968-0004(02)02193-X
Tomanek L., Zuzow M. J. (2010). The proteomic response of the mussel congeners mytilus galloprovincialis and m. trossulus to acute heat stress: implications for thermal tolerance limits and metabolic costs of thermal stress. J. Exp. Biol. 213, 3559–3574. doi: 10.1242/jeb.041228
Tremblay R., Pellerin-Massicotte J. (1997). Effect of the tidal cycle on lysosomal membrane stability in the digestive gland of mya arenaria and mytilus edulis l. Comp. Biochem. Physiol. Part A Physiol. 117, 99–104. doi: 10.1016/S0300-9629(96)00232-0
Wang J., Ren R., Yao C.-L. (2018). Oxidative stress responses of mytilus galloprovincialis to acute cold and heat during air exposure. J. Molluscan Stud. 84, 285–292. doi: 10.1093/mollus/eyy027
Willems E., Leyns L., Vandesompele J. (2008). Standardization of real-time PCR gene expression data from independent biological replicates. Anal. Biochem. 379, 127–129. doi: 10.1016/j.ab.2008.04.036
Yao C.-L., Somero G. N. (2012). The impact of acute temperature stress on hemocytes of invasive and native mussels (Mytilus galloprovincialis and mytilus californianus): DNA damage, membrane integrity, apoptosis and signaling pathways. J. Exp. Biol. 215, 4267–4277. doi: 10.1242/jeb.073577
Zaidi N., Swinnen J. V., Smans K. (2012). ATP-citrate lyase: a key player in cancer metabolism. Cancer Res. 72, 3709–3714. doi: 10.1158/0008-5472.CAN-11-4112
Zera A. J., Harshman L. G. (2001). The physiology of life history trade-offs in animals. Annu. Rev. Ecol. Syst. 32, 95–126. doi: 10.1146/annurev.ecolsys.32.081501.114006
Zetler B. D., Flick R. E. (1985). Predicted extreme high tides for California: 1983–2000. J. Waterw. Port Coastal Ocean Eng. 111, 758–765. doi: 10.1061/(ASCE)0733-950X(1985)111:4(758)
Zhang W., Dong Y. (2021). Membrane lipid metabolism, heat shock response and energy costs mediate the interaction between acclimatization and heat-hardening response in the razor clam sinonovacula constricta. J. Exp. Biol. 224, jeb243031. doi: 10.1242/jeb.243031
Zhang G., Fang X., Guo X., Li L., Luo R., Xu F., et al. (2012). The oyster genome reveals stress adaptation and complexity of shell formation. Nature 490, 49–54. doi: 10.1038/nature11413
Keywords: mussels, gene-expression, amylase activity, heat stress, entrainment
Citation: Chan G, Gracey AY, Solares E, Wehrle BA and Connor KM (2023) Cycles of heat exposure elevate metabolic enzyme genes and alters digestion in mussels. Front. Mar. Sci. 10:1120695. doi: 10.3389/fmars.2023.1120695
Received: 10 December 2022; Accepted: 14 June 2023;
Published: 03 August 2023.
Edited by:
Xiaolong Gao, Xiamen University, ChinaReviewed by:
Liqiang Zhao, Guangdong Ocean University, ChinaCopyright © 2023 Chan, Gracey, Solares, Wehrle and Connor. This is an open-access article distributed under the terms of the Creative Commons Attribution License (CC BY). The use, distribution or reproduction in other forums is permitted, provided the original author(s) and the copyright owner(s) are credited and that the original publication in this journal is cited, in accordance with accepted academic practice. No use, distribution or reproduction is permitted which does not comply with these terms.
*Correspondence: Kwasi M. Connor, a21jb25ub3JAdWNpLmVkdQ==
Disclaimer: All claims expressed in this article are solely those of the authors and do not necessarily represent those of their affiliated organizations, or those of the publisher, the editors and the reviewers. Any product that may be evaluated in this article or claim that may be made by its manufacturer is not guaranteed or endorsed by the publisher.
Research integrity at Frontiers
Learn more about the work of our research integrity team to safeguard the quality of each article we publish.