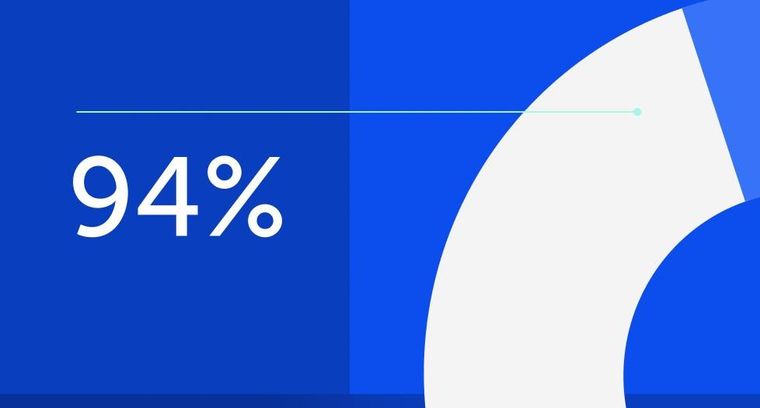
94% of researchers rate our articles as excellent or good
Learn more about the work of our research integrity team to safeguard the quality of each article we publish.
Find out more
HYPOTHESIS AND THEORY article
Front. Mar. Sci., 05 June 2023
Sec. Marine Megafauna
Volume 10 - 2023 | https://doi.org/10.3389/fmars.2023.1117409
This article is part of the Research TopicWhales and ClimateView all 14 articles
Whales have been titled climate savers in the media with their recovery welcomed as a potential carbon solution. However, only a few studies were performed to date providing data or model outputs to support the hypothesis. Following an outline of the primary mechanisms by which baleen whales remove carbon from the atmosphere for eventual sequestration at regional and global scales, we conclude that the amount of carbon whales are potentially sequestering might be too little to meaningfully alter the course of climate change. This is in contrast to media perpetuating whales as climate engineers. Creating false hope in the ability of charismatic species to be climate engineers may act to further delay the urgent behavioral change needed to avert catastrophic climate change impacts, which can in turn have indirect consequences for the recovery of whale populations. Nevertheless, whales are important components of marine ecosystems, and any further investigation on existing gaps in their ecology will contribute to clarifying their contribution to the ocean carbon cycle, a major driver of the world’s climate. While whales are vital to the healthy functioning of marine ecosystems, overstating their ability to prevent or counterbalance anthropogenically induced changes in global carbon budget may unintentionally redirect attention from known, well-established methods of reducing greenhouse gases. Large scale protection of marine environments including the habitats of whales will build resilience and assist with natural carbon capture.
Baleen whales (mysticetes) are present in all oceans and are among the largest marine animals to have ever existed. From studies of terrestrial megafauna, evidence has emerged that various species can modify their environment and indirectly influence landscape carbon dynamics. For example, the presence of forest elephants (Loxodonta cyclotis) favors the emergence of fewer and larger trees with higher wood density (Berzaghi et al., 2019). White rhinos (Ceratotherium simum simum) help maintain short grass communities which result in smaller more patchy fires (Waldram et al., 2008), and dugongs (Dugong dugong), large aquatic grazers, can alter seagrass communities affecting carbon sequestration and storage (Scott et al., 2018). Theories have emerged that baleen whales may also act as ecosystem engineers by influencing the ocean carbon cycle on regional and large basin scales as part of the marine food web (Willis, 2014) even bringing the idea of ‘carbon credits’ into the debate (Hagger et al., 2022; Pearson et al., 2023).
Whales amongst other marine life contribute to the biological carbon pump providing a secondary transfer pathway (Bowen, 1997; Mariani et al., 2020; National Academies of Sciences and Medicine, 2022). The biological carbon pump involves a number of processes through which inorganic carbon such as CO2 is fixed into organic matter via photosynthesis and then transported into deeper ocean away from the atmosphere (Claustre et al., 2021). Whales mediate transfer of carbon from inorganic to organic forms through marine biota activity and its export into the deeper ocean. Carbon export may eventually add to the carbon pool of the global ocean circulation or sequestration into marine sediments, which implies much longer recycling time scales (millennia) of inorganic carbon back to the atmosphere (Strand and Benford, 2009; Legge et al., 2020). Marine organic carbon travels through nested cycles operating on temporal scales of orders of magnitude difference and are subsystems of the global ocean carbon cycle (Figure 1).
Figure 1 The nested cycles of marine organic carbon (Corg), including (1) net primary production and heterotrophic respiration in the surface ocean, (2) export production from the surface ocean and respiration in the deep sea followed by upwelling or mixing of the respired CO2 back to the surface ocean (the soft-tissue component of the biological pump); and (3) the burial of sedimentary organic carbon. Also shown are the indicative residence times (r). Note that carbon reservoirs shown refer solely to the fraction affected by organic carbon cycling; for instance, the deep-ocean value shown is of carbon dioxide produced by respiration, not the carbon released from CaCO3 dissolution. Adapted from Hain et al. (2014). *Southern Ocean estimates, Savoca et al., 2021 **All oceans estimate, Pershing et al., 2010 and Pearson et al., 2023 ***Southern Ocean estimates, Pershing et al., 2010.
The following examples from the literature can give indications of carbon volumes but need to be understood within a context of complex systems and are provided to give a better understanding of how some theories were derived. The global ocean net primary productivity has been estimated at 53 Pg carbon annually (Johnson and Bif, 2021). Ocean export production ratios (i.e., export from the surface ocean to deep ocean as a fraction of primary production) have been estimated to range from 0.02–0.96 (Jo et al., 2021). This organic matter decomposes at depth and drives the biological pump. However, of the organic matter raining out of the surface ocean, only 1% (0.05 Pg carbon out of 4 Pg carbon annually; Hain et al., 2014) is incorporated into the surficial sediments and potentially sequestered for scales longer than the global ocean circulation. Latest estimates put the ocean carbon sink at 9-11% higher than previously estimated (Terhaar et al., 2022). A carbon sink is defined as any form of carbon accumulation and storage for long periods of time (millennia) that removes CO2 from the atmosphere (Alexandrov, 2008). The carbonate pump is estimated to be larger than the soft-tissue pump; some organisms construct inorganic hard parts from CaCO3 of which ~25% (~0.25 Pg C out of 1 Pg C annually) sinks to the seafloor and is preserved and buried in the sediments. The total amount of carbon permanently sequestered from the atmosphere is therefore approximately 0.3 Pg carbon annually (Honjo, 2004; Hain et al., 2014).
To summarize the main aspects, about 20-32% of anthropogenic CO2 is transferred from the atmosphere to the ocean through the biological, carbonate and solubility pumps (Sabine Christopher et al., 2004; Khatiwala et al., 2009; Hauck et al., 2020; Kim and Kim, 2021). For instance, in the period 2009-2018, 48 ± 3% of anthropogenic emissions (18.0 ± 0.07 Pg CO2/yr) remained in the atmosphere, 29 ± 6% (11.7 ± 2.2 Pg CO2) were taken up by terrestrial ecosystems, and 23 ± 5% (9.1 ± 2.2 Pg CO2) were taken up by the ocean (Friedlingstein et al., 2019), of which approximately 40% were absorbed in the Southern Ocean (Terhaar et al., 2021).
For whales to play a role in reducing atmospheric CO2 concentrations, they need to influence the biological pump such that there is an increase in (i) the export of organic carbon from the surface to the deep ocean and/or (ii) the amount removed from the ocean and entering the slower sediment circuit (Figure 1). In the following section, we outline the contrast between available data on whales and carbon export and coverage of the topic by media, often portrait as climate savers.
We then present an overview of the role of baleen whales relative to the global ocean carbon cycle, and frame their potential effects in the light of existing literature. We further review the knowledge of whale ecology in the context of the ocean carbon cycle, to provide a better understanding on baleen whales’ claimed role as climate engineers and propose a range of fundamental research requiring further investigation.
The topic of whales as carbon sinks has received much media attention combining two popular subjects: whales and climate change. A non-representative search in ProQuest using the terms “whales” and “carbon” revealed 352 newspaper articles from over 45 countries between 2012 and 2022, with a strong increase of interest in the topic in the past three years (Figure 2). An online search in Google Scholar and ProQuest on peer-reviewed studies in international journals over the same 10-year period resulted in six studies providing observations or modelling studies on whales as carbon sinks mostly focusing on the Southern Ocean (Figure 2, Supplementary Table 1). A report that triggered a strong outreach in media was published by the World Monetary Fund in 2019 by Chami et al. (2019) (e.g., “Protecting whales to protect the planet” UNEP, 2019; “Restoring whales to their pre-hunted numbers could capture 1.7 billion tons of CO2 a year”, EuroNews, 2021). The report used data from research publications analyzed in the following sections (Lavery et al., 2010; Pershing et al., 2010; Roman and McCarthy, 2010) to calculate a potential carbon uptake by whales. An opinion published by (Pearson et al., 2023) further underlined the gap between available scientific evidence on this topic and also triggered a strong media response (e.g., “Whales can have an important but overlooked role in tackling the climate crisis, researchers say” CNN News 15/12/2022) with many news stories continuing to claim whales as climate savers or climate engineers. The phenomenon of simplifying complex relationships to gain readers attention is particularly common in the “post-truth” era (Gobo and Marcheselli, 2022) amplified by the increased use of social media.
Figure 2 (A) Number of published newspaper articles in English and number of peer reviewed scientific studies based on a ProQuest online database search for entries between 01/01/2010 and 01/01/2021. Search term “whales and carbon” and (B) Results from a search for the keywords “whales” and “carbon” in social media posts over a 10-days period showing the increased posting on this topic for “World Whale Day” on the 20th February 2022. Engagements refer to number of interactions with a post e.g., comments and reactions such as likes.
A search of social media posts on varies platforms that contained the key words “whales” and “carbon” using the analyst tool keyhole (https://keyhole.co) showed that this topic has a high retention within the social media domain (Figure 2). Over 10 days between 18th-27th February 2022, 677 posts were reported, showing a sharp increase of posts during and after World Whale Day on the 20th February. However, it is important to notice that 88% of these were reposts and only 10% had original content related to whales and carbon further underlining the high retention of this topic from original posts. Retention of popular topics is high across social media platforms even if the original content is not updated (Kapoor et al., 2018). The sharing of content is often driven by believes over accuracy (Pennycook et al., 2021). The implications of a believe-driven process can result in a diversion of attention towards well-established methods of carbon sequestration. It can result in attention and resources drawn away from proven, effective nature-based solutions to climate change.
There are five pathways identified by Roman et al. (2014) and further outlined by Pearson et al. (2023) in which whales can potentially enhance the removal of carbon from the atmosphere into the deep ocean and/or deposition. They are summarized in Figure 3 and discussed in the next paragraphs in the context and order of contribution to global carbon export. There are difficulties and limitations in quantifying the impact of whales on carbon sequestrations with the estimated contribution of each pathway being hypothetical. Estimates are presented to provide a guidance and support the theory that whales have a limited contribution to global carbon export. Processes in the euphotic zone are generally faster and short lived (days to weeks), whereas processes in deeper parts of the ocean take much longer (years to millennia).
Figure 3 Illustration of five different ways in which whales can contribute to an increase in oceanic removal of atmospheric carbon: whale pump, whale conveyor belt, whale biomass and known long life span, whale falls, and whale bioturbation (artist impression based on Roman et al., 2014).
Primary production is generally limited in macro-nutrient depleted oligotrophic oceans. Despite macro-nutrients (nitrate and phosphate) are abundant in the in the Southern Ocean (Marinov et al., 2008), micro-nutrients, prevalently iron (Fe), are scarce in the so-called high-nutrient-low-chlorophyll (HNLC) regions of the Southern Ocean, and in the equatorial and northern Pacific. This results in a limited primary production in vast region of the global oceans and associated CO2 drawdown from the atmosphere.
In theory, the whale conveyor belt, whale pump, and whale bioturbation are linked to the biological pump by increasing the availability of nutrients and enhancing phytoplankton primary production, thereby driving a positive feedback loop in the oceanic carbon pump (Smetacek and Naqvi, 2008; Roman et al., 2014; Pearson et al., 2022). Primary production in the sunlit surface ocean is limited by nutrient availability. Macro-nutrients (nitrate and phosphate) are abundant in the deep ocean and in the Southern Ocean (Marinov et al., 2008), while micro-nutrient, prevalently iron (Fe), are scarce in the so-called high-nutrient-low-chlorophyll (HNLC) regions of the Southern Ocean, and in the equatorial and northern Pacific. For example, Roman et al. (2014) suggested that blue whales (Balaenoptera musculus) in the Southern Ocean transported an estimated 88 t of nitrogen per year (estimated for 2001) from Antarctic feeding grounds to tropical breeding grounds via nitrogen-rich urea released through catabolism of lipids and proteins during fasting. Although it is difficult to demonstrate the excess production in lieu of the large natural variability, this flux can be considered new production and could lead to a carbon flux of up to 2,100 tons of CO2 per year (equivalent to 572 tons of carbon) by phytoplankton (Martin et al., 2021). However, this quantity is then released in the upper ocean where respiration processes may rapidly release it back to the atmosphere (Bolaños et al., 2020). Respiration of whales during their migration also add to release of CO2 back to the atmosphere (Lavigne et al., 1986; Huntley et al., 1991). While nutrients directly recycled by whales within the upper water column from distant regions support primary production, they do not necessarily stimulate downward particle flux, which is required for carbon sequestration (Lavery et al., 2014; Martin et al., 2021).
The key micro-nutrient iron that is translocated via whales is generally scarce in open oceans, especially in the Southern Ocean (de Baar et al., 2005; Tagliabue et al., 2017). Whale-dependent recycling and relocation of iron is suggested to play a key role in increasing primary productivity, and in turn enhancing the biological pump and possibly carbon export (Smith et al., 2013; Ratnarajah et al., 2016). Generally, the contribution of baleen whales to the iron flux is through the spatial redistribution of iron in surface waters rather than through the recycling of iron. Given the extent of ocean where primary production occurs, internal cycling of iron by phytoplankton degradation is much larger than whale contribution (Maldonado et al., 2016). However, whale feces do supply and distribute both macro- and micro-nutrients to areas where external sources are limited (Roman and McCarthy, 2010), for instance when crossing the Southern Ocean HNLC region in the meridional migration. Whale feces are known to have high iron concentration (146 ± 134 μg/g) (Nicol et al., 2010; Ratnarajah et al., 2014) compared to Antarctic krill (65 ± 41 μg/g, Supplementary Tables 2, 3). Whales provide biomagnification because they preferentially store carbon in their muscles, but do not need all the Fe found in the ingested krill biomass (e.g., 147 mg Fe/kg dry weight of krill; Nicol et al., 2010). It is suggested that foraging whales concentrate carbon in their muscles and release a majority of ingested iron in a more bioavailable fraction through feces. Ideally bioavailable iron stays at +2 stage, which is soluble. In contrast, iron +3 is insoluble. Inside the whale stomachs digestion is an acidic (HCl) and oxygen depleted environment, which would favor the reduction reaction, i.e. Fe+3 to Fe +2 (Ratnarajah et al., 2014; Ratnarajah et al., 2017; Ratnarajah et al., 2018), however, further evidence on the bioavailability of whale feces is required. The iron concentration in whale feces reaches ten million times that of the Southern Ocean surface waters (Nicol et al., 2010) and can contribute 3 to 5 orders of magnitude higher iron concentrations than that required for phytoplankton growth (Wing et al., 2014). For instance, compared to surface seawater dissolved iron concentration of ~ 0.1 – 0.5 nmol/L in the Southern Ocean (Tagliabue et al., 2012), humpback whale (Megaptera novaeangliae) feces iron concentration ranges from 186 - 754 nmol/L and from 5,026 – 22,526 nmol L in dissolved (<0.2 μ) and particulate (>0.2 μ) phases, respectively (Ratnarajah et al., 2017). Other factors, such as the differences between soluble and colloidal iron, fraction of lithogenic iron, and available organic matter, may also influence bioavailability and ocean primary production (Ratnarajah et al., 2016; Ratnarajah et al., 2018). Fin (B. physalus) and blue whale species are likely the largest contributors to iron supply, due to their higher prey consumption, and thus having a greater potential for enhanced carbon fluxes (Durfort et al., 2020). Whether this contribution to the natural ocean iron cycle would lead to enhanced sequestration of carbon is however not demonstrated. Iron relocation is one of the most likely components of the biological pump that can be enhanced by whales’ activity in open ocean that otherwise lie barren. Modelling based on the latest baleen whale prey consumption estimates suggested that in some areas such as the Scotia Sea, iron from whale feces may have stimulated more than 20% of net primary production prior whaling (Savoca et al., 2021). Given that phytoplankton uptakes one quarter of iron released by whales (Ratnarajah et al., 2016), for four whale species (blue, fin, humpback and Antarctic minke whales - B. bonaerensis) in the Southern Ocean could capture up to 0.215 Pg carbon annually during pre-whaling period (Savoca et al., 2021) However, whale feces driven carbon capture is likely to vary among the global oceans based on the nutrient availability in surface ocean and bioavailable fraction of nutrients in whale feces. For instance, unlike the Southern Ocean, the Southern Benguela upwelling system in the Southeast Atlantic is a nutrient rich oceanic sector in a low latitude feeding area. Hence, the carbon capture potential through the whale pump in the Southern Benguela feeding ground is likely lower compared to the estimations made in the Southern Ocean.
Similar considerations can be made for the whale pump, which describes the process of whales moving up and down the water column for feeding, and transferring nutrients to surface waters in the process (Nicol et al., 2010; Pearson et al., 2022). Spatial and temporal scales are much smaller in this case than for regional migrations, and there is no quantification in the literature whether this process may have an annual effect on the duration of the blooms or on net ecosystem production. For instance, there is no evidence of how more effective whales could be in relocating iron from the deeper Southern Ocean to the surface when compared to the typical mixing rates. It has been reported that sperm whales (Physeter macrocephalus) feeding in depth of 1,000 m can make nutrients available when defaecating at the surface, coinciding with phytoplankton blooms (Roman et al., 2014). Based on a population estimation from the year 2000, Lavery et al. (2010) estimated that an additional 240,000 t of CO2 (0.000066 Pg carbon) annually were exported into the Southern Ocean through the promotion of phytoplankton growth (of which one quarter is recycled by phytoplankton) from the nutrients provided by sperm whale feces. These estimates are indicative of the contribution exerted by whales to ecosystem functioning, but do not give a comparative view of their role with respect to the background physical-biogeochemical dynamics and also exclude the respiration of whales during this process.
Whale bioturbation (Figure 4) is the process by which whales resuspend bed sediments into the water column, when feeding on mollusks and other organisms on the ocean floor. It is conceptually similar to the whale pump, but involves a pool of nutrients that are assumed to be less readily available to marine degradation. This process is commonly observed in feeding of demersal fish, the main difference is their smaller size compared to whales (Mariani et al., 2020). For example, gray whales (Eschrichtius robustus) have been shown to remobilize nutrients into the Bering Sea when foraging for amphipods (Nelson and Johnson, 1987; Alter et al., 2007). However, this is non-selective bioturbation, and the same process could release organic matter previously buried in the sediments, thus enhancing the recycling of carbon back to CO2. The overall whale bioturbation contribution to carbon export or sequestration has not been quantified to date and may prove difficult to be disentangled from other bottom biogeochemical processes but its contribution to carbon export if any is likely smaller than the whale pump contribution. Bottom feeding is only known for a few whale species such as gray whales restricted to small areas.
Figure 4 Main research areas, gaps and possible case studies that fall under modelling, quantification and validation themes to better define the role of whales as carbon sinks.
The long-life span and large body size of whales can contribute to carbon storage over decades. Using whale populations of eight baleen whale species from the year 2001, the living biomass stock of carbon was estimated to be about 0.002 Pg (Pearson et al., 2023). Commercial whaling has reduced the size of baleen whale populations by an average of 70% since 1900 (Tulloch et al., 2019). Prior to whaling, the carbon in living biomass was estimated to be five times higher (0.01 Pg). Other marine organisms like fish also accumulate carbon but over shorter times scales with the removal of fish from the ocean causing an estimated 0.00034 Pg of carbon release per year since 1950 (Mariani et al., 2020). The overall contribution of whales living tissue to carbon storage is limited by their lifespan (at least several decades) and their own respiration as for all animals. However, the value of animals to carbon sequestration in general also needs to be assessed in the context of their ecosystem function ((Schmitz et al., 2023).
Whales can fall into the deep sea after death, where their carcasses can take hundreds of years to decompose, or millennia if buried in sediments, which depends on sedimentation rate and available oxygen (Pershing et al., 2010). However, the overall contribution of whale falls to carbon sequestration could be relatively small and might be in the range of only 2% of estimated carbon export by whales (Durfort et al., 2020). The amount of biogenic carbon is species specific and depending on the region. Whale species vary in size and some species, such as southern right whales (Eubalaena australis), have a tendency to float after death and are less likely to sink. The effect would be more marked in regions supporting larger whale populations e.g. on the west coast of North America where a study from marine sanctuaries off San Francisco claimed that whale falls represent roughly 60% of the estimated total annual carbon removal of the area equivalent to about 10 890 tons of carbon or 0.00001 Pg (Hutto et al., 2021). Should the population recover to pre-whaling numbers, Pearson et al. (2023) estimated for baleen whale species (blue, fin, humpback, southern right, gray and Antarctic minke whales) a carbon sequestration potential through sinking carcasses of 0.000062 Pg carbon annually. Respiration rates of whales to atmospheric CO2 would need to be subtracted from this calculation as well as any CO2 release during decomposition.
Carbon buried under marine sediment within the deep sea is generally a long-term (millennia) removal of carbon from the atmosphere (Teng and Zhang, 2018). However, the burial rate of organic carbon is a function of sedimentation rates (Betts and Holland, 1991), which is less than 0.01 cm k per year for major ocean basins and the Southern Ocean; (Restreppo et al., 2020). Scavengers remove whale soft tissue at high rates (40–60 kg per day), and then the exposed bones are colonized by dense assemblages of polychaetas and crustaceans (Smith and Baco, 2003). Anerobic bacteria decomposes bone lipids. The scavenging process can last up to 50 years (Smith and Baco, 2003), which prevents whale carcasses from being buried under falling sediment. Therefore, burial of organic carbon (sequestration) associated with whale falls is expected to be the least effective pathway of carbon sequestration by whales will make only a small contribution to carbon sequestration.
Based on the literature presented in the previous sections, there is a common understanding that baleen whales contribute to carbon uptake, as done by other marine life in the marine ecosystem. The challenge remains to quantify whether this uptake at the whale’s population level translates into an effective enhancement of carbon export and subsequent sequestration that is comparable with the known scales of the global ocean. A lack of comparable global data for different regions and species, has led to generalized estimations based on few observations with extrapolations based on numerical models. Our analysis has demonstrated that only relatively few studies so far have provided direct observations on the baleen whales contribution to the ocean carbon cycle on a locally limited scale, and that the majority of the carbon sequestration potential estimates are obtained through model parameterizations. Currently there is a lack of evidence showing that whales make a significant contribution to global carbon export to alter climate change. We looked at whale carbon sequestration pathways and available knowledge for each pathway suggests that the influence of whales on carbon flux is small compared to the global carbon flux. Nevertheless, public interest on this topic has steadily increased over the past decade underlining the need for more research in this field. The gap between media and available science to support the theory of whales influencing global climate may distract falsely portraying the role of whales as important to increase carbon sequestration from the atmosphere may distract policy from other important issues.
Whales could enhance primary production through a range of processes (Savoca et al., 2021). If such processes and the precise mechanisms involved can be established, and the mitigation contribution quantified, then additional benefits as ecosystem services be identified, they could be related to some form of ‘carbon credits’ (Costello et al., 2012), and the corresponding funds be used for marine ecosystem restoration (Hagger et al., 2022).
Enhancing primary production through supply of iron appears to be the most professed contributor to carbon sequestration by whales (Savoca et al., 2021). In situ experiments with whale feces have shown diatom growth (Smith et al., 2013) but field conditions are likely highly variable. In the past, iron fertilization experiments have been undertaken with mixed results and also triggering a controversial debate as it has long been criticized for its large-scale impacts (Chisholm Sallie et al., 2001; Martin et al., 2013). Similar experiments are once again underway partially attempting to simulate the effect of whale feces on marine productivity. In late 2021, a group of researchers released a mix of nitrogen, phosphorus and trace elements off the coast of Sydney, Australia simulating the effect of whale feces in the marine environment (The Guardian, 21/12/2021). As part of a similar experiment, led by the Centre for Climate Repair at Cambridge, United Kingdom, researchers are releasing a mix of iron, phosphors, nitrates and silica in the Indian Ocean (New Scientist 22/02/2022). Results from these experiments have not been presented to our knowledge so far.
Smetacek et al. (2012) found a peak of a large diatom bloom in the fourth week after fertilization in an experiment in the Antarctic Circumpolar Current. Half of the diatoms sank deeper than 1,000 m, potentially contributing to carbon removal from the atmosphere. However, significant diatom increase only occurs in HNLC waters when sufficient light conditions occur, which encompass only one-third of the ocean (Boyd et al., 2007). Even the most successful experiment managed to export just 900 t of carbon to the marine environment (Pershing et al., 2010). A larger experiment releasing 100 t of iron sulphate in Canada triggered a debate about the effectiveness and grey areas of ocean fertilisation (Tollefson, 2012). Such experiments are providing us with valuable information about the biological pump but so far have not shown to be effective for climate-relevant CO2 carbon removal. Achieving this requires long time removal of carbon from the atmosphere with phytoplankton or other forms of carbon stored in sediment (Figure 1). Large-scale fertilisation of marine environments will provide short term removal (100 – 1000-year cycle) of CO2 but will not provide long-term solutions. Furthermore, it would take hundreds of such events to match the export potential of fully restored whale populations (Pershing et al., 2010).
Efforts to model how whales influence the ocean’s CO2 sink capacity have revealed a number of data gaps. The majority of research in this field has concentrated on the Southern Ocean despite the lack of oceanographic data (nutrient transport, circulation) for this region that is required to understand carbon sequestration. Case studies are lacking from most regions, which is particularly relevant given the northern/southern hemisphere dichotomy in micronutrient limitation (Wing et al., 2014). This includes the lack of emerging research on the carbon cycle of all marine vertebrates such as fish and seabirds (Martin et al., 2021; Rhodes-Reese et al., 2021) and the nutrient uptake by bacteria and viruses (Ratnarajah et al., 2018). Viruses are widely distributed in the marine environment, accumulating carbon at an equivalent to over 75 million blue whales (Suttle, 2005).
Also, for precise estimates, it should be considered that the retention of nutrients varies in the whale body with age and reproductive status (Tynan, 1997). The variation in food intake by age and sex also drives iron retention. There are relevant weight variations between breeding and feeding season but some studies assumed defecation of whales in similar amounts between feeding and breeding grounds (Roman and McCarthy, 2010; Roman et al., 2014). Respiration rates of whales would also vary with different behavioral stages (feeding, migrating, socializing or breeding) and regions (tropical versus arctic). For example, Tynan (1997) estimated that humpback whales can contribute up to 34,254 Mt CO2 (equivalent to 9-71 Mt carbon) to the atmosphere through respiration.
Removal of fixed carbon through whale falls depends on different aspects, such as species, location of death, and season for instance. Also, the carbon levels in different types of tissues (bone, muscle, blubber, visceras) for each species are currently unknown. Although previously it was assumed that 50% of dead whales reach the deep sea (Baco and Smith, 2003) and that biomass-carbon conversion does not change with carcass degradation, now it is clear that all these factors need to be taken into consideration for the evaluation of the contribution of whales falls to deep ocean and sediment carbon stocks.
The whale conveyor belt, whale pump and whale bioturbation potentially increase iron availability for phytoplankton growth. Data on the arrival and departure times of individual whales and their migratory path are crucial to refining bioenergetics models and predictions of iron flux. It is one of the key factors involved in enhancing phytoplankton growth. The amount of biogenic iron (and other elements at the surface waters) released by whales in relation to region, species, prey and time has not been studied in much detail. For example, Ratnarajah et al. (2017) estimated the fractions of lithogenic and biogenic iron in the feces of baleen whales, which showed up to possible 80% biogenic iron for Southern Ocean samples.
The size and nature of iron particles, their reduction or complexation of certain molecular forms allows different phytoplankton classes to access the iron (Ratnarajah et al., 2018; Sutak et al., 2020). There is limited knowledge about whale feces particle size and iron content (Supplementary Table 2) (Ratnarajah et al., 2016; Schlosser et al., 2018). The iron-retention and release rates by baleen whales have also not been measured directly (Savoca et al., 2021). Thus, the complex physico-chemical nature of whale feces influencing phytoplankton blooms on spatial and temporal scales are largely unknown.
Predicting the impact on the carbon sink capacity of oceans from recovering whale populations is being further complicated by the increasing impacts of climate change. Climate change could delay or weaken the carbon sequestration processes (Sigman and Hain, 2012) by whales as their populations are facing food shortages under changing ocean conditions (Seyboth et al., 2016). Some generalist whale species such as humpback whales have the ability to forage on multiple prey types with similar net energy gain and thus can buffer against increased ocean variability under climate change, at least in some periods and regions with potential alternative prey available (Fleming et al., 2016) but other species such as gray whales are more susceptible to the fluctuations in the abundance of specific prey items (Torres et al., 2022). However, this will alter the iron export and carbon capture abilities. There is emerging evidence of altered feeding behavior from humpback whales in the Southern Hemisphere (e.g., supergroups in South Africa) (Findlay et al., 2017). This may result in reduced bioavailable iron content of humpback whale feces in these regions and the large HNLC regions along their migratory paths. Also related to the uncertainties in estimating whales’ contribution to carbon uptake from the atmosphere are the confidence intervals associated with abundance estimates of the different species, as for example even for the well-studied Antarctic minke whale (Branch, 2011; Galletti Vernazzani et al., 2017).
Most calculation of carbon export from whales have not included the CO2 loss to the atmosphere via respiration, therefore not providing whales´ net capacity for carbon removal. Huntley et al. (1991) estimated that marine top predators may transfer as much as 20-25% of photosynthetically fixed carbon back to the atmosphere. The respiration rate of whales can be estimated using the following equation, as previously used by Lavigne et al. (1986): R = 140m^a where R represents respiration rate (kcal/day), m states the average mass of the whales and a being a fixed value of 0.75 for whales (Brown and Gillooly, 2003). The respired carbon is calculated on the assumption that 1 kcal of metabolism produces 0.38 g CO2 (or 0.10 g C). For an estimated 1.1 million baleen whales (Pearson et al., 2023) of different species and age classes an average weight of 15 ton per whale would result in 0.0079 Pg respired carbon annually, equivalent to 50% carbon stored in whale biomass (Lavigne et al., 1986). A hypothetical number that would also largely fluctuate over time and there is no consensus if respiration rate doubles in marine mammals compared to terrestrial mammals (Sims, 2000). The respired carbon stored in whale biomass could therefore also be much less than 50%. Most of the phytoplankton biomass is respired through consumers and only <1% reaches the sediments (Hain et al., 2014), the whales’ contribution to atmospheric CO2 via respiration likely plays a smaller role in the calculation of their net capacity for carbon removal. However, a thorough understanding of respiration rates and the overall contribution of whales to atmospheric CO2 is fundamental for the ongoing discussion about whales and carbon export.
Derived from the above discussion, we identified 12 fundamental research areas related to the contribution of whales to atmospheric carbon sink (Figure 4). An overarching topic that applies to all these research fields is the impact of whaling on ecosystem function and future population growth or decline. These areas fall under the overarching themes of modelling, quantification and validation. Future ecosystem models require the inclusion of nutrient flux from whales. There are different aspects of whale nutrient flux that require further investigations including the role of whale mediated nutrient distribution in ocean processes, the role of horizontal movement in nutrient distribution, and the relevance of background nutrient and trace metal content in seawater.
Quantification and with-it validation of ecosystem models, firstly requires standardization for validation and best practice guidelines given the large spatial and temporal scale to be covered with many case studies from different regions. Areas in which further quantification is required are the phytoplankton carbon flux (and intake of recycled nutrients from whale faeces), spatial and temporal variation of whale faeces (nutrient content, iron, trace elements) in relation to prey and estimation of refined consumption rates of whales (according to time on the feeding grounds and prey composition), carbon flux from cetaceans to the atmosphere.
The above research areas should be targeted with case studies on bioavailability of whale-derived nutrients and case studies in particular in the Northern Hemisphere and in areas with contrasting foraging species.
Whales can substantially influence their marine environment and play a part on the global carbon cycle with importance varying regionally depending on the location and species type. However, there is a need for careful evaluation of their impact in the context of climate mitigation, which at present is far from certain and efforts in that direction are somewhat misguided for the lack of corroborative scientific data. Detailed evaluation is needed for different oceanic regimes, and within a comparative context that also accounts for the role of whales in CO2 mitigation as opposed to other organisms and ecosystems that also contribute substantially to ecosystem functioning.
Combining behavioural observations with bioenergetics, biotic, chemical, and physical features of the marine environment, oceanographic modeling, and nutrient modeling promise to be rewarding. Further exploring the potential contribution of whales to carbon and nutrient cycles and other ecosystem functions if the populations were to recover can add to the conservation value of whales. While some are claiming that baleen whale populations may meet criteria of carbon sequestration to be considered as a natural climate solution (Chami et al., 2020) this should not define the overarching value. Carbon fluxes observed in other marine ecosystems such as mangroves (0.031-0.034 Pg per year) or salt marshes (up to 0.087 Pg per year) are significantly higher than those of baleen whales (Duarte et al., 2005). Competition over carbon credits between whales and marine ecosystems would be counterproductive. Given the uncertainties and lack of data, increased focus on whales and carbon in the public domain bear the risk of inflating the value of whales as carbon sinks. Management measures that help protect marine habitats functioning as carbon sinks including deep see environments will make a significant contribution to mitigate climate change (Simard et al., 2016). Large scale protection of marine environments including the habitats of whales will build resilience and assist with natural carbon capture.
The presence of whales is associated with many other benefits for the marine environment. Whales have economic value through whale-watching (O’Connor et al., 2009), intrinsic cultural value to many societies, they host other species and are an essential source of food for abyssal ecosystems (Baco and Smith, 2003; Sumida et al., 2016). Carbon sequestration may not be the most important contributor to this value, and a further investigation of this role remains a global task.
The original contributions presented in the study are included in the article/Supplementary Material. Further inquiries can be directed to the corresponding author.
Ethical review and approval was not required for the animal study because no data was collected as part of this research. Peer reviewed studies are being used.
All authors significantly contributed toward development of the manuscripts. J-OM, SS, JB, ES, SP, GF MV, KF AR and BM contributed to conception and design of study. J-OM, SS, MV, and AR contributed to assessment and organization. J-OM, SS, and AR contributed to model development. SS, JB, ES, SP, GF, MV, KF, BM, and AR contributed to interpretation of results. J-OM wrote the manuscript. All authors contributed to the article and approved the submitted version.
This work was supported by a grant to Griffith University from a private charitable trust as part of the Whales & Climate Research Program.
The authors declare that the research was conducted in the absence of any commercial or financial relationships that could be construed as a potential conflict of interest.
All claims expressed in this article are solely those of the authors and do not necessarily represent those of their affiliated organizations, or those of the publisher, the editors and the reviewers. Any product that may be evaluated in this article, or claim that may be made by its manufacturer, is not guaranteed or endorsed by the publisher.
The Supplementary Material for this article can be found online at: https://www.frontiersin.org/articles/10.3389/fmars.2023.1117409/full#supplementary-material
Supplementary Table 1 | Overview of some scientific studies assessing whales and carbon between 1991-2022.
Supplementary Table 2 | Iron concentration from whale feces as reported in current literature.
Supplementary Table 3 | Estimated iron concentration from different Antarctic krill samples.
Alexandrov G. (2008). “Climate change 1: short-term dynamics,” in Encyclopedia of ecology. Eds. Jørgensen S. E., Fath B. D. (Oxford: Academic Press), 588–592.
Alter S. E., Rynes E., Palumbi S. R. (2007). DNA Evidence for historic population size and past ecosystem impacts of gray whales. Proc. Natl. Acad. Sci. 104, 15162–15167. doi: 10.1073/pnas.0706056104
Baco A. R., Smith C. R. (2003). High species richness in deep-sea chemoautotrophic whale skeleton communities. Mar. Ecol. Prog. Ser. 260, 109–114. doi: 10.3354/meps260109
Berzaghi F., Longo M., Ciais P., Blake S., Bretagnolle F., Vieira S., et al. (2019). Carbon stocks in central African forests enhanced by elephant disturbance. Nat. Geosci. 12, 725–729. doi: 10.1038/s41561-019-0395-6
Betts J. N., Holland H. D. (1991). The oxygen content of ocean bottom waters, the burial efficiency of organic carbon, and the regulation of atmospheric oxygen. Global Planetary Change 5, 5–18. doi: 10.1016/0921-8181(91)90123-E
Bolaños L. M., Karp-Boss L., Choi C. J., Worden A. Z., Graff J. R., Haëntjens N., et al. (2020). Small phytoplankton dominate western north Atlantic biomass. ISME J. 14, 1663–1674. doi: 10.1038/s41396-020-0636-0
Bowen W. (1997). Role of marine mammals in aquatic ecosystems. Mar. Ecol. Prog. Ser. 158, 267–274. doi: 10.3354/meps158267
Boyd P. W., Jickells T., Law C. S., Blain S., Boyle E. A., Buesseler K. O., et al. (2007). Mesoscale iron enrichment experiments 1993-2005: synthesis and future directions. Science 315, 612–617. doi: 10.1126/science.1131669
Branch T. A. (2011). Humpback whale abundance south of 60°S from three complete circumpolar sets of surveys. J. Cetacean Res. Manage. 3, 53–69. doi: 10.47536/jcrm.vi.305
Brown J. H., Gillooly J. F. (2003). Ecological food webs: high-quality data facilitate theoretical unification. Proc. Natl. Acad. Sci. 100, 1467–1468. doi: 10.1073/pnas.0630310100
Chami R., Cosimano T. F., Fullenkamp C., Oztosun S. (2019). Nature’s solution to climate change: a strategy to protect whales can limit greenhouse gases and global warming. Finance Dev. 56, 34–38.
Chami R., Fullenkamp C., Berzaghi F., Español-Jiménez S., Marcondes M., Palazzo J. (2020). On valuing nature-based solutions to climate change: a framework with application to elephants and whales. doi: 10.2139/ssrn.3686168
Chisholm Sallie W., Falkowski Paul G., Cullen John J. (2001). Dis-crediting ocean fertilization. Science 294, 309–310. doi: 10.1126/science.1065349
Claustre H., Legendre L., Boyd P. W., Levy M. (2021). The oceans’ biological carbon pumps: framework for a research observational community approach. Front. Mar. Sci. 8. doi: 10.3389/fmars.2021.780052
Costello C., Gaines S., Gerber L. R. (2012). A market approach to saving the whales. Nature 481, 139–140. doi: 10.1038/481139a
de Baar H. J. W., Boyd P. W., Coale K. H., Landry M. R., Tsuda A., Assmy P., et al. (2005). Synthesis of iron fertilization experiments: from the iron age in the age of enlightenment. J. Geophysical Research: Oceans 110. doi: 10.1029/2004jc002601
Duarte C. M., Middelburg J. J., Caraco N. (2005). Major role of marine vegetation on the oceanic carbon cycle. Biogeosciences 2, 1–8. doi: 10.5194/bg-2-1-2005
Durfort A., Mariani G., Troussellier M., Tulloch V., Mouillot D. (2020). The collapse and recovery potential of carbon sequestration by baleen whales in the southern ocean. Research Square. Available at: https://assets.researchsquare.com/files/rs-92037/v1_covered.pdf?c=1631844426.
EuroNews (2021). Restoring whales to their pre-hunted numbers could capture 1.7 billion tonnes of CO2 a year. Euronews. Available at: https://www.euronews.com/green/2021/10/27/restoring-whales-to-their-pre-hunted-numbers-could-capture-1-7-billion-tonnes-of-co2-a-year.
Findlay K. P., Seakamela S. M., Meÿer M. A., Kirkman S. P., Barendse J., Cade D. E., et al. (2017). Humpback whale “super-groups” – a novel low-latitude feeding behaviour of southern hemisphere humpback whales (Megaptera novaeangliae) in the benguela upwelling system. PLoS One 12, e0172002. doi: 10.1371/journal.pone.0172002
Fleming A. H., Clark C. T., Calambokidis J., Barlow J. (2016). Humpback whale diets respond to variance in ocean climate and ecosystem conditions in the California current. Global Change Biol. 22, 1214–1224. doi: 10.1111/gcb.13171
Friedlingstein P., Jones M. W., O'sullivan M., Andrew R. M., Hauck J., Peters G. P., et al. (2019). Global carbon budget 2019. Earth Syst. Sci. Data 11, 1783–1838. doi: 10.5194/essd-11-1783-2019
Galletti Vernazzani B., Jackson J. A., Cabrera E., Carlson C. A., Brownell R. L. Jr. (2017). Estimates of abundance and trend of Chilean blue whales off isla de chiloé, Chile. PLoS One 12, e0168646. doi: 10.1371/journal.pone.0168646
Gobo G., Marcheselli V. (2022). “Scientists, experts and public opinion,” in Science, technology and society: an introduction. Eds. Gobo G., Marcheselli V. (Cham: Springer International Publishing), 163–178.
Hagger V., Waltham N. J., Lovelock C. E. (2022). Opportunities for coastal wetland restoration for blue carbon with co-benefits for biodiversity, coastal fisheries, and water quality. Ecosystem Serv. 55, 101423. doi: 10.1016/j.ecoser.2022.101423
Hain M. P., Sigman D. M., Haug G. H. (2014). “The biological pump in the past,” in Treatise on geochemistry, 2nd ed. (Amsterdam, Netherlands: Elsevier), 485–517.
Hauck J., Zeising M., Le Quéré C., Gruber N., Bakker D. C. E., Bopp L., et al. (2020). Consistency and challenges in the ocean carbon sink estimate for the global carbon budget. Front. Mar. Sci. 7. doi: 10.3389/fmars.2020.571720
Honjo S. (2004). Particle export and the biological pump in the southern ocean. Antarctic Sci. 16, 501–516. doi: 10.1017/S0954102004002287
Huntley M. E., Lopez M. D., Karl D. M. (1991). Top predators in the southern ocean: a major leak in the biological carbon pump. Science 253, 64–66. doi: 10.1126/science.1905841
Hutto S. H., Brown M., Francis E. (2021). Blue carbon in marine protected areas: part 2; a blue carbon assessment of greater farallones national marine sanctuary. national marine sanctuaries conservation science series ONMS-21-07 (Washington, DC: U.S. Department of Commerce, National Oceanic and Atmospheric Administration, Office of National Marine Sanctuaries).
Jo C. O., Ryu J., Woo S.-Y., Lee W.-J., Kim H. W., Choi Y.-S. (2021). Estimation of ocean export production ratios based on mixed layer depth and satellite chlorophyll observations. Regional Stud. Mar. Sci. 45, 101816. doi: 10.1016/j.rsma.2021.101816
Johnson K. S., Bif M. B. (2021). Constraint on net primary productivity of the global ocean by argo oxygen measurements. Nat. Geosci. 14, 769–774. doi: 10.1038/s41561-021-00807-z
Kapoor K. K., Tamilmani K., Rana N. P., Patil P., Dwivedi Y. K., Nerur S. (2018). Advances in social media research: past, present and future. Inf. Syst. Front. 20, 531–558. doi: 10.1007/s10796-017-9810-y
Khatiwala S., Primeau F., Hall T. (2009). Reconstruction of the history of anthropogenic CO2 concentrations in the ocean. Nature 462, 346–349. doi: 10.1038/nature08526
Kim S.-U., Kim K.-Y. (2021). Impact of climate change on the primary production and related biogeochemical cycles in the coastal and sea ice zone of the southern ocean. Sci. Total Environ. 751, 141678. doi: 10.1016/j.scitotenv.2020.141678
Lavery T. J., Roudnew B., Gill P., Seymour J., Seuront L., Johnson G., et al. (2010). Iron defecation by sperm whales stimulates carbon export in the southern ocean. Proc. R. Soc. B: Biol. Sci. 277, 3527–3531. doi: 10.1098/rspb.2010.0863
Lavery T. J., Roudnew B., Seymour J., Mitchell J. G., Smetacek V., Nicol S. (2014). Whales sustain fisheries: blue whales stimulate primary production in the southern ocean. Mar. Mammal Sci. 30, 888–904. doi: 10.1111/mms.12108
Lavigne D. M., Innes S., Worthy G., Kovacs K. M., Schmitz O. J., Hickie J. P. (1986). Metabolic rates of seals and whales. Can. J. Zoology 64, 279–284. doi: 10.1139/z86-047
Legge O., Johnson M., Hicks N., Jickells T., Diesing M., Aldridge J., et al. (2020). Carbon on the Northwest European shelf: contemporary budget and future influences. Front. Mar. Sci. 7. doi: 10.3389/fmars.2020.00143
Maldonado M. T., Surma S., Pakhomov E. A. (2016). Southern ocean biological iron cycling in the pre-whaling and present ecosystems. Philos. Trans. R. Soc. A: Mathematical Phys. Eng. Sci. 374, 20150292. doi.: 10.1098/rsta.2015.0292
Mariani G., Cheung W. W. L., Lyet A., Sala E., Mayorga J., Velez L., et al. (2020). Let more big fish sink: fisheries prevent blue carbon sequestration–half in unprofitable areas. Sci. Adv. 6, eabb4848. doi: 10.1126/sciadv.abb4848
Marinov I., Follows M., Gnanadesikan A., Sarmiento J. L., Slater R. D. (2008). How does ocean biology affect atmospheric pCO2? theory and models. J. Geophysical Research: Oceans 113. doi: 10.1029/2007jc004598
Martin A. H., Pearson H. C., Saba G. K., Olsen E. M. (2021). Integral functions of marine vertebrates in the ocean carbon cycle and climate change mitigation. One Earth 4, 680–693. doi: 10.1016/j.oneear.2021.04.019
Martin P., van der Loeff M. R., Cassar N., Vandromme P., D'ovidio F., Stemmann L., et al. (2013). Iron fertilization enhanced net community production but not downward particle flux during the southern ocean iron fertilization experiment LOHAFEX. Global Biogeochem. Cycles 27, 871–881. doi: 10.1002/gbc.20077
National Academies of Sciences and Medicine (2022). A research strategy for ocean-based carbon dioxide removal and sequestration (Washington, DC: The National Academies Press).
Nelson C. H., Johnson K. R. (1987). Whales and walruses as tillers of the sea floor. Sci. Am. 256, 112–117. doi: 10.1038/scientificamerican0287-112
Nicol S., Bowie A., Jarman S., Lannuzel D., Meiners K. M., van der Merwe P. (2010). Southern ocean iron fertilization by baleen whales and Antarctic krill. Fish Fisheries 11, 203–209. doi: 10.1111/j.1467-2979.2010.00356.x
O’Connor S., Campbell R., Cortez H., Knowles T. (2009). Whale Watching Worldwide: tourism numbers, expenditures and expanding economic benefits, a special report from the International Fund for Animal Welfare). Yarmouth MA, USA: Economists at Large) 21, 38–46.
Pearson H. C., Savoca M. S., Costa D. P., Lomas M. W., Molina R., Pershing A. J., et al. (2023). Whales in the carbon cycle: can recovery remove carbon dioxide? Trends Ecol. Evol. 38 (3), 238–249. doi: 10.1016/j.tree.2022.10.012
Pennycook G., Epstein Z., Mosleh M., Arechar A. A., Eckles D., Rand D. G. (2021). Shifting attention to accuracy can reduce misinformation online. Nature 592, 590–595. doi: 10.1038/s41586-021-03344-2
Pershing A. J., Christensen L. B., Record N. R., Sherwood G. D., Stetson P. B. (2010). The impact of whaling on the ocean carbon cycle: why bigger was better. PLoS One 5, e12444. doi: 10.1371/journal.pone.0012444
Ratnarajah L., Bowie A. R., Lannuzel D., Meiners K. M., Nicol S. (2014). The biogeochemical role of baleen whales and krill in southern ocean nutrient cycling. PLoS One 9, e114067. doi: 10.1371/journal.pone.0114067
Ratnarajah L., Lannuzel D., Townsend A. T., Meiners K. M., Nicol S., Friedlaender A. S., et al. (2017). Physical speciation and solubility of iron from baleen whale faecal material. Mar. Chem. 194, 79–88. doi: 10.1016/j.marchem.2017.05.004
Ratnarajah L., Melbourne-Thomas J., Marzloff M. P., Lannuzel D., Meiners K. M., Chever F., et al. (2016). A preliminary model of iron fertilisation by baleen whales and Antarctic krill in the southern ocean: sensitivity of primary productivity estimates to parameter uncertainty. Ecol. Model. 320, 203–212. doi: 10.1016/j.ecolmodel.2015.10.007
Ratnarajah L., Nicol S., Bowie A. R. (2018). Pelagic iron recycling in the southern ocean: exploring the contribution of marine animals. Front. Mar. Sci. 5. doi: 10.3389/fmars.2018.00109
Restreppo G. A., Wood W. T., Phrampus B. J. (2020). Oceanic sediment accumulation rates predicted via machine learning algorithm: towards sediment characterization on a global scale. Geo-Marine Lett. 40, 755–763. doi: 10.1007/s00367-020-00669-1
Rhodes-Reese M., Clay D., Cunningham C., Moriles-Miller J., Reese C., Roman J., et al. (2021). Examining the role of marine mammals and seabirds in southeast alaska’s marine ecosystem dynamics. Front. Mar. Sci. 8. doi: 10.3389/fmars.2021.720277
Roman J., Estes J. A., Morissette L., Smith C., Costa D., Mccarthy J., et al. (2014). Whales as marine ecosystem engineers. Front. Ecol. Environ. 12, 377–385. doi: 10.1890/130220
Roman J., McCarthy J. J. (2010). The whale pump: marine mammals enhance primary productivity in a coastal basin. PLoS One 5, e13255. doi: 10.1371/journal.pone.0013255
Sabine Christopher L., Feely Richard A., Gruber N., Key Robert M., Lee K., Bullister John L., et al. (2004). The oceanic sink for anthropogenic CO2. Science 305, 367–371. doi: 10.1126/science.1097403
Savoca M. S., Czapanskiy M. F., Kahane-Rapport S. R., Gough W. T., Fahlbusch J. A., Bierlich K. C., et al. (2021). Baleen whale prey consumption based on high-resolution foraging measurements. Nature 599, 85–90. doi: 10.1038/s41586-021-03991-5
Schlosser C., Schmidt K., Aquilina A., Homoky W. B., Castrillejo M., Mills R. A., et al. (2018). Mechanisms of dissolved and labile particulate iron supply to shelf waters and phytoplankton blooms off south Georgia, southern ocean. Biogeosciences 15, 4973–4993. doi: 10.5194/bg-15-4973-2018
Schmitz O. J., Sylvén M., Atwood T. B., Bakker E. S., Berzaghi F., Brodie J. F., et al. (2023). Trophic rewilding can expand natural climate solutions. Nat. Climate Change, 1–10. doi: 10.1038/s41558-023-01631-6
Scott A. L., York P. H., Duncan C., Macreadie P. I., Connolly R. M., Ellis M. T., et al. (2018). The role of herbivory in structuring tropical seagrass ecosystem service delivery. Front. Plant Sci. 9. doi: 10.3389/fpls.2018.00127
Seyboth E., Groch K. R., Dalla Rosa L., Reid K., Flores P., Secchi E. R. (2016). Southern right whale (Eubalaena australis) reproductive success is influenced by krill (Euphausia superba) density and climate. Sci. Rep. 6, 28205. doi: 10.1038/srep28205
Simard F., Laffoley D., Baxter J. M. (Eds.) (2016). Marine protected areas and climate change: adaptation and mitigation synergies, opportunities and challenges (Gland, Switzerland: IUCN).
Sims D. W. (2000). Putting marine mammals back in the mainstream. Nature 405, 14–14. doi: 10.1038/35011156
Smetacek V., Klaas C., Strass V. H., Assmy P., Montresor M., Cisewski B., et al. (2012). Deep carbon export from a southern ocean iron-fertilized diatom bloom. Nature 487, 313–319. doi: 10.1038/nature11229
Smetacek V., Naqvi S. W. A. (2008). The next generation of iron fertilization experiments in the southern ocean. Philos. Trans. R. Soc. A: Mathematical Phys. Eng. Sci. 366, 3947–3967. doi: 10.1098/rsta.2008.0144
Smith C. R., Baco A. R. (2003). Ecology of whale falls at the deep-Sea floor. Oceanogr. Mar. Biol. an Annu. Rev. 41, 311–354. doi: 10.1201/9780203180570-33
Smith L. V., Mcminn A., Martin A., Nicol S., Bowie A. R., Lannuzel D., et al. (2013). Preliminary investigation into the stimulation of phytoplankton photophysiology and growth by whale faeces. J. Exp. Mar. Biol. Ecol. 446, 1–9. doi: 10.1016/j.jembe.2013.04.010
Strand S. E., Benford G. (2009). Ocean sequestration of crop residue carbon: recycling fossil fuel carbon back to deep sediments. Environ. Sci. Technol. 43, 1000–1007. doi: 10.1021/es8015556
Sumida P. Y. G., Alfaro-Lucas J. M., Shimabukuro M., Kitazato H., Perez J., Soares-Gomes A., et al. (2016). Deep-sea whale fall fauna from the Atlantic resembles that of the pacific ocean. Sci. Rep. 6, 22139. doi: 10.1038/srep22139
Sutak R., Camadro J.-M., Lesuisse E. (2020). Iron uptake mechanisms in marine phytoplankton. Front. Microbiol. 11. doi: 10.3389/fmicb.2020.566691
Tagliabue A., Bowie A. R., Boyd P. W., Buck K. N., Johnson K. S., Saito M. A. (2017). The integral role of iron in ocean biogeochemistry. Nature 543, 51–59. doi: 10.1038/nature21058
Tagliabue A., Mtshali T., Aumont O., Bowie A. R., Klunder M. B., Roychoudhury A. N., et al. (2012). A global compilation of dissolved iron measurements: focus on distributions and processes in the southern ocean. Biogeosciences 9, 2333–2349. doi: 10.5194/bg-9-2333-2012
Teng Y., Zhang D. (2018). Long-term viability of carbon sequestration in deep-sea sediments. Sci. Adv. 4, eaao6588. doi: 10.1126/sciadv.aao6588
Terhaar J., Frölicher T. L., Joos F. (2021). Southern ocean anthropogenic carbon sink constrained by sea surface salinity. Sci. Adv. 7, eabd5964. doi: 10.1126/sciadv.abd5964
Terhaar J., Frölicher T. L., Joos F. (2022). Observation-constrained estimates of the global ocean carbon sink from earth system models. Biogeosciences 19, 4431–4457. doi: 10.5194/bg-19-4431-2022
Tollefson J. (2012). Ocean-fertilization project off Canada sparks furore. Nature 490, 458–459. doi: 10.1038/490458a
Torres L. G., Bird C. N., Rodríguez-González F., Christiansen F., Bejder L., Lemos L., et al. (2022). Range-wide comparison of Gray whale body condition reveals contrasting Sub-population health characteristics and vulnerability to environmental change. Front. Mar. Sci. 9. doi: 10.3389/fmars.2022.867258
Tulloch V. J. D., Plagányi É.E., Brown C., Richardson A. J., Matear R. (2019). Future recovery of baleen whales is imperiled by climate change. Global Change Biol. 25, 1263–1281. doi: 10.1111/gcb.14573
Tynan C. T. (1997). Cetacean distributions and oceanographic features near the Kerguelen Plateau. Geophys. Res. Lett. 24, 2793–2796. doi: 10.1029/97GL02860
Unep (2019). Protecting whales to protect the planet. United Nations Environment Program. Available at: https://www.unep.org/news-and-stories/story/protecting-whales-protect-planet.
Waldram M. S., Bond W. J., Stock W. D. (2008). Ecological engineering by a mega-grazer: white rhino impacts on a south African savanna. Ecosystems 11, 101–112. doi: 10.1007/s10021-007-9109-9
Willis J. (2014). Whales maintained a high abundance of krill; both are ecosystem engineers in the southern ocean. Mar. Ecol. Prog. Ser. 513, 51–69. doi: 10.3354/meps10922
Keywords: blue carbon, whales, carbon export, ocean carbon cycle, climate change
Citation: Meynecke J-O, Samanta S, de Bie J, Seyboth E, Prakash Dey S, Fearon G, Vichi M, Findlay K, Roychoudhury A and Mackey B (2023) Do whales really increase the oceanic removal of atmospheric carbon? Front. Mar. Sci. 10:1117409. doi: 10.3389/fmars.2023.1117409
Received: 06 December 2022; Accepted: 24 April 2023;
Published: 05 June 2023.
Edited by:
Francesco Ferretti, Virginia Tech, United StatesReviewed by:
Matthew Savoca, Stanford University, United StatesCopyright © 2023 Meynecke, Samanta, de Bie, Seyboth, Prakash Dey, Fearon, Vichi, Findlay, Roychoudhury and Mackey. This is an open-access article distributed under the terms of the Creative Commons Attribution License (CC BY). The use, distribution or reproduction in other forums is permitted, provided the original author(s) and the copyright owner(s) are credited and that the original publication in this journal is cited, in accordance with accepted academic practice. No use, distribution or reproduction is permitted which does not comply with these terms.
*Correspondence: Jan-Olaf Meynecke, by5tZXluZWNrZUBncmlmZml0aC5lZHUuYXU=
Disclaimer: All claims expressed in this article are solely those of the authors and do not necessarily represent those of their affiliated organizations, or those of the publisher, the editors and the reviewers. Any product that may be evaluated in this article or claim that may be made by its manufacturer is not guaranteed or endorsed by the publisher.
Research integrity at Frontiers
Learn more about the work of our research integrity team to safeguard the quality of each article we publish.