- 1College of Life and Environmental Sciences, University of Exeter, Exeter, United Kingdom
- 2School of Biological Sciences, University of Bristol, Bristol, United Kingdom
- 3Horniman Museum and Gardens, London, United Kingdom
- 4CARMABI Foundation, Piscaderabaai z/n, Willemstad, Curaçao
Recruitment of coral larvae on reefs is crucial for individual survival and ecosystem integrity alike. Coral larvae can detect and respond to a wide range of biotic and abiotic cues, including acoustic cues, to locate suitable sites for settlement and metamorphosis. However, the acoustic ecology of coral larvae, including how they perceive auditory cues, remains poorly understood. In this mini-review we consider both ex situ physiology and behavior, and in situ ecological and behavioral studies, to first provide an updated overview of the abiotic and biotic cues used by coral larvae to guide settlement. We then explore in detail the use of acoustic cues and the current literature on behavioral responses to acoustic stimuli. Finally, we discuss gaps in our understanding of the mechanisms by which coral larvae detect acoustic cues, highlighting a novel application of technology to explore these sensory capabilities. We also address how larval phonotaxis, i.e., the ability to orient to a sound cue, can be applied to coral reef conservation. Current research suggests that acoustic cues are likely used at small spatial scales, and that coral larvae may have directional acoustic sensitivity enabling phonotactic behavior. Recruitment of coral larvae on reefs is significantly influenced by habitat-specific soundscape variation and likely affected by anthropogenic disturbance. We propose a novel application of the remote sensing technology, micro-scanning laser Doppler vibrometry (LDV), to quantify the micromechanical responses of putative acoustically sensitive epidermal microstructures. We then highlight the potential for incorporation of acoustic enrichment techniques in coral reef conservation and restoration interventions.
1 Introduction
Marine invertebrate larvae were once considered passive particles lacking the ability to detect or respond to their environment (G. Thorson, 1950; Chia et al., 1984) but it is now widely accepted that interactions between both environmental conditions and biologically-generated cues affect larval behavior and physiology across many marine invertebrate taxa, including corals (Rodriguez et al., 1993; Shanks, 2009; Gleason and Hofmann, 2011) (Table 1). The ocean was once described as ‘The Silent World’ by Cousteau and Dumas in 1953, but we now know that coral reefs are bioacoustically rich. Many reef inhabitants produce sound during a wide array of behaviors which together contribute to the ambient soundscape of the “choral” reef (Schmitz, 2002; Lobel et al., 2010; Lobel, 2013; Radford et al., 2014a). This ambient soundscape has been shown to act as an orientation cue for the pelagic larvae of many fish, decapod crustaceans and reef-building corals, assisting their orientation towards suitable settlement sites (Tolimieri et al., 2000; Tolimieri et al., 2002; Jeffs et al., 2003; Simpson et al., 2004; Leis and Lockett, 2005; Simpson et al., 2005; Montgomery et al., 2006; Vermeij et al., 2010; Radford et al., 2011).
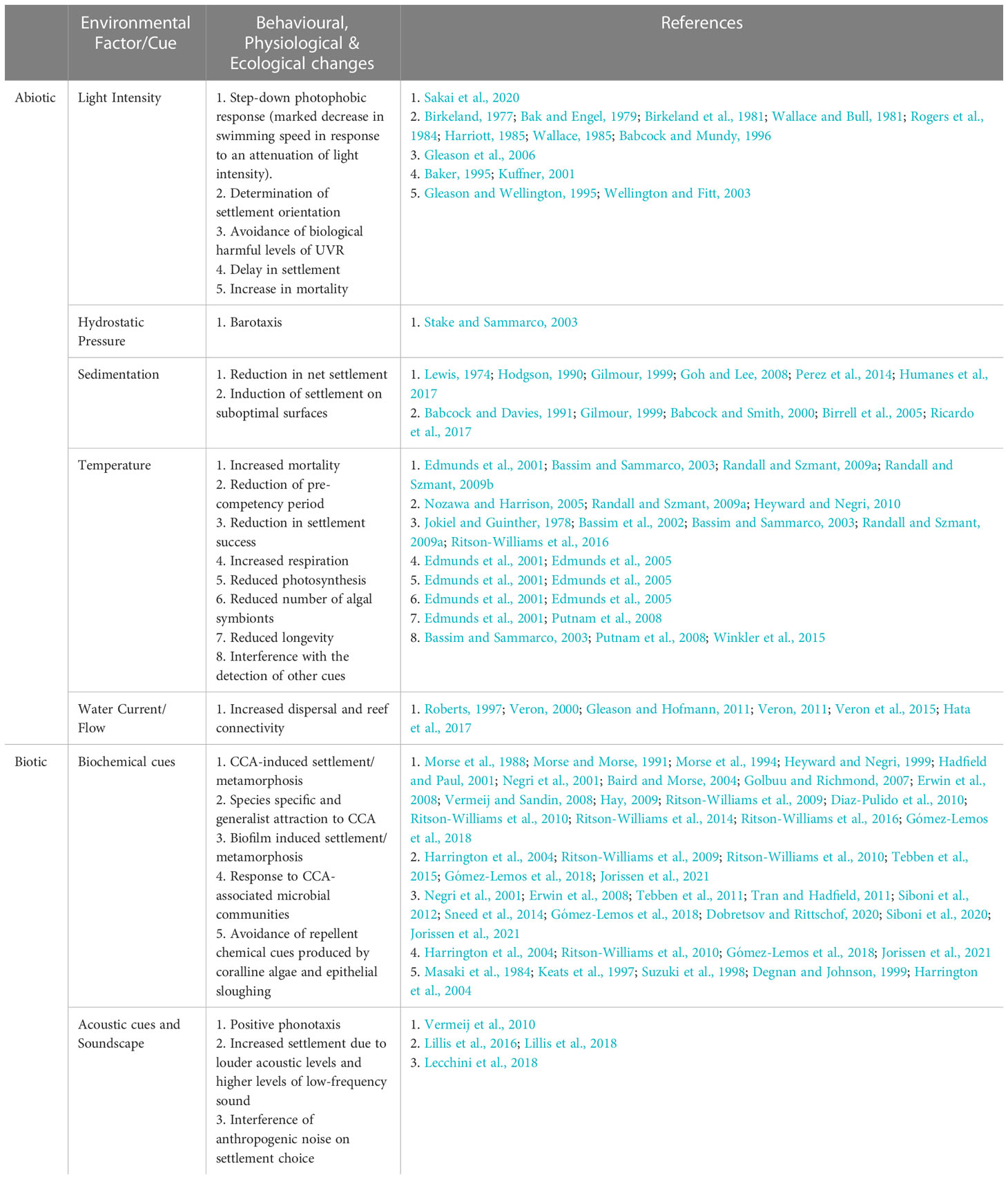
Table 1 Collated research outlining the abiotic and biotic environmental factors and cues that induce behavioral, physiological and ecological changes associated with enhanced or disrupted settlement in coral larvae.
Reef-building corals represent keystone species in coral reef ecosystems, providing valuable ecosystem goods and services to 100s of millions of people (Woodhead et al., 2019). However, the behavioral responses of coral larvae (planulae) to acoustic stimuli and the sensory mechanisms by which they detect acoustic cues remain poorly understood. Yet, these are of increasing importance, especially in the context of growing anthropogenic pressures on coral reefs, including climate change, overfishing, sewage and fertilizer runoff and noise pollution (Lecchini et al., 2018; Richmond et al., 2018; Jones, 2019; Duarte et al., 2021). Coral larvae can respond to an array of environmental cues that guide their settlement. We review these, with a particular emphasis on acoustics and soundscapes, the importance of which is just recently coming to light.
1.1 Environmental cues influencing coral larval settlement
1.1.1 Water flow and local currents
Local water currents play an extremely important role in the connectivity between coral reefs, influencing species diversity, dispersal and recruitment of coral larvae across local to regional spatial scales (Roberts, 1997; Veron, 2000; Veron, 2011; Veron et al., 2015; Hata et al., 2017). Currents connecting reefs seldom fall below 100 mms-1 (Baird and Morse, 2014). As coral larvae swim at speeds of <5 mms-1 (Szmant and Meadows, 2006; Gleason et al., 2009; Hata et al., 2017), directed swimming from the open ocean to reefs is limited. Nevertheless, modelling using data obtained from fish has shown that vertical migration of larvae during ontogeny reduces interactions with ocean currents, thus altering recruitment and connectivity among reefs (Paris et al., 2007). Wave action has also been shown to accelerate development in purple sea urchin (Strongylocentrotus purpuratus) and Pacific sand dollar (Dendraster excentricus), where increased turbulence, associated with shallower coastal waters, induced larval competence and enhanced larval settlement (Gaylord et al., 2013; Hodin et al., 2018).
1.1.2 Light intensity
Corals need sufficient levels of solar radiation to support the photosynthetic requirements of their symbionts (Chalker et al., 1988). Ambient light levels, spectral quality and substratum color significantly influence larval settlement across many species of coral larvae (Babcock and Mundy, 1996; Mundy and Babcock, 1998; Mason et al., 2011; Strader et al., 2015; Foster and Gilmour, 2016; Sakai et al., 2020). However, the strength and directionality of larval phototaxis varies with species, age, water temperature, light intensity and wavelength of light (Lewis, 1974; Bassim and Sammarco, 2003; Brooke and Young, 2005; Gleason et al., 2006; Sakai et al., 2020; Mulla et al., 2021).
During settlement experiments, coral larvae of many species preferentially settle onto the undersides of substrates in shallower water, altering their settlement preferences to vertical and upward facing surfaces at greater depths (Birkeland, 1977; Bak and Engel, 1979; Birkeland et al., 1981; Wallace and Bull, 1981; Rogers et al., 1984; Harriott, 1985; Wallace, 1985; Babcock and Mundy, 1996; Strader et al., 2015). Several species, however, aggregate in darker regions, representing a trade-off between required photosynthetically active radiation (PAR) and intensified levels of ultraviolet radiation (UVR). At irradiance levels found in near-surface waters, light has been shown to increase avoidance behaviour (Gleason et al., 2006), prolong settlement (Baker, 1995; Kuffner, 2001) and cause higher levels of mortality of larvae (Gleason and Wellington, 1995; Wellington and Fitt, 2003).
1.1.3 Hydrostatic pressure
Hydrostatic pressure causes directional changes in swimming orientation (barotaxis) in a range of aquatic invertebrate taxa (Forward, 1990; Kingsford et al., 2002; Goldsteins and Butler, 2009). However, to our knowledge, only one study on the brooding coral Porites astreoides (Stake and Sammarco, 2003) has examined barotaxis in cnidarians. In this study, booded larvae were exposed to pressures ranging from surface conditions (103.4 kPa) to those at ~40 m below the surface. When exposed to surface pressure, larvae displayed positive barotaxis and swam downwards, but at greater pressures, larvae swam upwards (Stake and Sammarco, 2003). Although evidence of barotaxis in coral larvae is limited, these findings reflect those demonstrated by other zooplankton (Morgan, 1984; Forward, 1989; Forward, 1990; Kingsford et al., 2002). Furthermore, barotaxis enables corals to sense and settle in their species-specific optimal irradiance environments, even when irradiance information is lacking, e.g., during diurnal/nocturnal shifts or periods of shading (Stake and Sammarco, 2003; Gleason and Hofmann, 2011).
1.1.4 Temperature variation
Stressful sublethal temperatures interfere with normal settlement behavior in coral larvae. In studies on two broadcast-spawning corals, warmer water temperatures negatively affected larval physiology, dispersal and settlement via increased larval mortality (Bassim and Sammarco, 2003; Randall and Szmant, 2009a), increased swimming/searching behaviors (Bassim and Sammarco, 2003), reduced pre-competency period (Nozawa and Harrison, 2005; Heyward and Negri, 2010) and reduced settlement success (Jokiel and Guinther, 1978; Bassim et al., 2002; Bassim and Sammarco, 2003). Similarly, in studies on brooding corals, as water temperatures dropped below or exceeded the ambient temperatures from where they were collected, planulae exhibited increased mortality (Edmunds et al., 2001; Randall and Szmant, 2009b; Ritson-Williams et al., 2016), reduced longevity (Edmunds et al., 2001; Putnam et al., 2008), reduced net settlement (Hartmann et al., 2013; Ritson-Williams et al., 2016), increased metamorphosis, reduced photosynthesis and diminished algal symbiont density (Edmunds et al., 2001; Edmunds et al., 2005).
1.1.5 Suspended and deposited sediment
Sedimentation has negative effects on both adult and larval-stage coral (Reviewed in Jones et al., 2015; Tuttle and Donahue, 2022). In observational studies of the brooding species Favia fragum (Lewis, 1974) and Pocillopora damicornis (Hodgson, 1990; Goh and Lee, 2008; Perez et al., 2014), net larval settlement was significantly reduced when suspended sedimentation was higher. Likewise, in field and laboratory studies, both high (~100 mg l-1) and low (~50 mg l-1) levels of suspended sediment adversely affected larval settlement and survival in the broadcast-spawning species Acropora digitifera and A. tenuis (Gilmour, 1999; Humanes et al., 2017). In both in situ and aquaria studies using larvae of the broadcast-spawning A. millepora, increased deposited sedimentation both reduced larval settlement and prevented larval settlement on upward facing substrates, with larvae settling only on vertical surfaces and the undersides of substrates (Babcock and Davies, 1991; Gilmour, 1999; Babcock and Smith, 2000; Birrell et al., 2005; Ricardo et al., 2017). Sedimentation most likely interferes with larval settlement by disrupting other sensory mechanisms, e.g., by masking chemical cues and impairing phototaxis (Ricardo et al., 2017). However, because it is difficult to track sediment dynamics on reef surfaces through time, it remains difficult to predict how the effects of sedimentation on short-term settlement will affect longer-term recruitment and survival.
1.1.6 Biochemical cues
In numerous studies of both brooding and broadcast-spawning coral species, crustose coralline algae (CCA) and its cell wall-associated compounds have been widely found to attract coral larvae and induce coral larval attachment (Morse et al., 1988; Morse and Morse, 1991; Morse et al., 1994; Morse et al., 1996; Heyward and Negri, 1999; Hadfield and Paul, 2001; Negri et al., 2001; Baird and Morse, 2004; Harrington et al., 2004; Golbuu and Richmond, 2007; Erwin et al., 2008; Vermeij and Sandin, 2008; Hay, 2009; Ritson-Williams et al., 2009; Diaz-Pulido et al., 2010; Ritson-Williams et al., 2010; Ritson-Williams et al., 2014; Tebben et al., 2015; Ritson-Williams et al., 2016; Gómez-Lemos et al., 2018; Jorissen et al., 2021). While CCA has also been found to induce settlement and metamorphosis across many different invertebrate taxa (Pawlik, 1992; Hadfield and Paul, 2001; Whalan et al., 2012; Sneed et al., 2015), the inducing capacity of CCA is highly variable, with complex interspecific interactions between corals and CCA. In two critically endangered species of broadcast-spawning Caribbean Acroporids (A. palmata & A. cervicornis), different species of CCA each induce varied amounts of larval settlement, with two relatively rare species of CCA being the most effective (Ritson-Williams et al., 2010). Interestingly, the cosmopolitan encrusting coralline algae Titanoderma prototypumm, found across both Caribbean and Pacific reefs, appears to be more attractive to larvae of reef-building Acroporids, inducing greater rates of settlement compared with other, more common, co-inhabiting CCA species (Harrington et al., 2004; Ritson-Williams et al., 2010; Gómez-Lemos et al., 2018). Furthermore, T. prototypumm significantly promoted settlement on the CCA surface compared with neighboring dead coral or plastic surfaces (Jorissen et al., 2021). In addition, some studies have found that specific microbial biofilms can also induce larval settlement in the absence of the CCA (Negri et al., 2001; Erwin et al., 2008; Tebben et al., 2011; Sneed et al., 2014; Gómez-Lemos et al., 2018; Dobretsov and Rittschof, 2020; Jorissen et al., 2021). Marine microbial biofilms are composed of many species of bacteria, unicellular algae (including diatoms) and protozoa. These produce an array of extracellular polymeric substances and signaling proteins shown to impact larval settlement and metamorphosis (reviewed in Dobretsov & Rittschof, 2020). Several studies have identified Pseudoalteromonas spp., a marine bacterium found in both Caribbean and Pacific CCA species, as a strong inducer of metamorphosis in larvae from both brooding and broadcast-spawning corals, including the important reef-building families Acroporidae and Pocilloporidae (Negri et al., 2001; Tebben et al., 2011; Siboni et al., 2012; Tebben et al., 2015) as well as an inducer of complete settlement (i.e., attachment to the substrate and metamorphosis) (Tran and Hadfield, 2011; Sneed et al., 2014; Tebben et al., 2015). It is worth noting that many CCA species have also evolved strategies to deter or prevent larval settlement, such as allelopathy (Suzuki et al., 1998; Degnan and Johnson, 1999) and sloughing (shedding of upper epithelial layers) (Masaki et al., 1984; Keats et al., 1997).
Thus, it is likely that CCA-induced coral settlement results from cues produced both by the CCA itself and by the associated microbial biofilm (Webster et al., 2004; Gómez-Lemos et al., 2018; Jorissen et al., 2021).
2 Acoustic cues and soundscapes
The grinding and popping of foraging echinoids, grazing scarids, vocalizing fish and snapping shrimp all contribute to the biophony of coral reefs (Simpson et al., 2004; Simpson et al., 2008; Lobel et al., 2010; Lobel, 2013; McWilliam et al., 2017). Thus, higher quality, healthy coral reefs are significantly louder, richer in acoustic events and more acoustically complex than degraded reefs (Piercy et al., 2014; Bertucci et al., 2016; Freeman and Freeman, 2016; Gordon et al., 2018). Acoustic cues are particularly useful for aquatic animals as sound travels faster and further underwater relative to other sensory cues, irrespective of directional currents (Urick, 1983; Ainslie, 2010; Duarte et al., 2021). Many marine invertebrates, therefore, have evolved the ability to detect and respond to acoustic cues, most likely by using specialized receptors (Salmon and Horch, 1973; Popper et al., 2001; Schmitz, 2002; Kaifu et al., 2008; Mooney et al., 2010; Vermeij et al., 2010; Wilkens et al., 2012; Lillis et al., 2013; Edmonds et al., 2016; Lillis et al., 2016; Solé et al., 2016; Vazzana et al., 2016; Charifi et al., 2017; Wale, 2017; Jézéquel et al., 2018; Lillis et al., 2018), and many taxa demonstrate increased rates of larval settlement in the presence of acoustic cues and during louder levels of acoustic cues (Jeffs et al., 2003; Simpson et al., 2004; Simpson et al., 2005; Stanley et al., 2010; Simpson et al., 2011; Stocks, 2012; Stanley et al., 2012a; Stanley et al., 2012b; Lillis et al., 2013; Lillis et al., 2015; Hinojosa et al., 2016).
Acoustic cues can also influence the swimming orientation and settlement behavior of coral larvae. In an in situ settlement chamber experiment, larvae of the Caribbean scleractinian coral Orbicella faveolata (previously Montastraea faveolata) exhibited directed phonotaxis, with larvae moving towards the source of a broadcasted coral reef soundscape irrespective of chamber orientation (Vermeij et al., 2010). In a separate study, O. faveolata larvae exhibited higher settlement rates when exposed to soundscapes from louder, more diverse coral reefs when compared to soundscapes from two quieter reefs characterized by either sponges and coral rubble or industrial debris and algal growth. (Lillis et al., 2016). These findings imply that the elevated acoustic power associated with more diverse habitats, or the absence or presence of specific frequencies within healthier habitats, may lead to increased larval settlement. The same authors found that settlement rates in larvae of the reef-building coral Porites astreoides doubled in an acoustic environment with higher levels of low-frequency sound, which are typical of a healthier reef with higher coral cover and higher densities of fish (Lillis et al., 2018). This suggests that low-frequency sounds are the predominant drivers of response in this species, and that the absence of these low frequencies may reduce settlement.
High-frequency sounds attenuate more rapidly underwater, but lower-frequency sounds emanating from reefs are theoretically detectable to invertebrates within 500 m from the source (Rogers and Cox, 1988; Anderson et al., 2021). However, currents and fluid flows may limit the ability of larvae to successfully navigate to cues 500 m away; therefore in practice, the range of detection and successful response may be closer still to 10 – 100 m (Gleason and Hofmann, 2011). Although O. faveolata larvae exhibit directional phonotaxis in situ (Vermeij et al., 2010), the experimental confinement to an acrylic chamber likely restricted fluid flow, allowing larvae to move unimpeded by currents. Therefore, our understanding of the spatial scale at which coral larvae are able to detect acoustic stimuli in their natural environment is still limited. The difficulties associated with in situ settlement experiments in complex topographical and hydrodynamic environments both highlights the challenge of interpreting the ecological significance and restoration utility of experimental results (Hata et al., 2017; Mayorga-Adame et al., 2017; Randall et al., 2020; Levenstein et al., 2022) as well as the many considerations that must be made when deisgning future acoustic larval settlement experiments.
To date, most studies of phonotaxis in coral planulae have been conducted with larvae from broadcast spawners (but see Lillis et al., 2018), therefore larvae from brooding corals are relatively understudied. However, it is proposed that mechanosensory epidermal cilia are responsible for auditory perception in coral (Vermeij et al., 2010). Therefore, given the abundance of dense cilia found on their surface, brooded larvae are also expected to possess the sensory mechanisms to detect and respond to acoustic stimuli (Gleason and Hofmann, 2011). This hypothesis requires further testing.
3 Mechanisms for acoustic detection in coral larvae
Sonic vibrations in water have both pressure and particle motion components (Reviewed in Nedelec et al., 2016). In their adult stages, most aquatic invertebrates can detect the particle motion component of sound, using specialized organs such as mechanosensory setae, chordotonal stretch receptors between the joints of appendages and statocyst and statolith receptor systems (Popper and Fay, 1999; Popper and Lu, 2000; Popper et al., 2001; Bleckmann, 2004; Nedelec et al., 2016). Many invertebrate larvae, including those of cnidarians, have a diversity of cilia-based mechanosensory systems that function during feeding, locomotion, tactic response, predator–prey interactions and settlement (Chia and Crawford, 1977; Chia and Koss, 1979; Freeman and Ridgway, 1990; Marlow et al., 2009; Bezares-Calderón et al., 2020), with many of these systems sensitive to acoustic particle motion (Tranter et al., 1982; Rogers and Cox, 1988; Budelmann, 1992; Kennedy et al., 1996; Zhadan, 2005; Tran and Hadfield, 2013; Lillis et al., 2015).
The sensory mechanisms employed by coral larvae to detect acoustic stimuli, however, remain unknown. Early studies of the temperate reef-building coral-species Balanophyllia regia and the tropical coral species Pocillopora damicornis demonstrated that the larval ectoderm is primarily composed of flagellated collar cells - a single flagellum surrounded by a ring of microvilli (Lyons, 1973; Vandermeulen, 1975). While the main function of these cells are primarily thought to be calcification, phagocytosis of food particles and motility, it has been suggested that these cells may also have a sensory function. This assumption was based on their similarities with statocyst systems used in the detection of acoustic cues in other invertebrate taxa (Lyons, 1973).
The laser Doppler vibrometry (LDV) method relies on the detection of the Doppler frequency shift that occurs when light is dispersed by a moving surface (Rothberg et al., 2017). In a study exploring particle motion detection in marine invertebrates, LDV was used to measure whole body vibrations (displacement, velocity and acceleration) as a putative stimulus of statocyst organs in cuttlefish (Family Sepiidae) and scallops (Family Pectinidae) (André et al., 2016). This experiment piloted the use of LDV techniques in an underwater bioacoustics study and highlights its potential value for use across other marine invertebrate taxa. LDV has also been successfully used to measure the mechanical response of microstructures such as antennae and sensory hairs to electrical and sound stimuli in several terrestrial invertebrates (Göpfert et al., 1999; Göpfert and Robert, 2002; Sutton et al., 2016). Although it is evident that coral larvae both respond to acoustic cues and possess the mechanosensory structures capable of detecting particle motion (Vermeij et al., 2010; Lillis et al., 2016; 2018) (Figures 1C, D), to date there have not been any attempts to measure the mechanical responses of their exterior cilia-based sensory systems to acoustic cues in a bioacoustics study, nor has this been done for the larvae of any marine invertebrate. We propose that laser Doppler vibrometry could be broadly applied to investigate the mechanosensory ability of coral larvae epidermal cilia, including quantifying both cilia beat dynamics and frequency-specific sensitivity to incident particle velocity. Using analytical signals capturing the spectral diversity of samples from coral reef sound recordings, and playbacks of the recordings themselves, it will be possible to determine the auditory sensitivity and bandwidth of coral larvae, offering a mechanistic basis for their phonotactic behavior (Figures 1A, B).
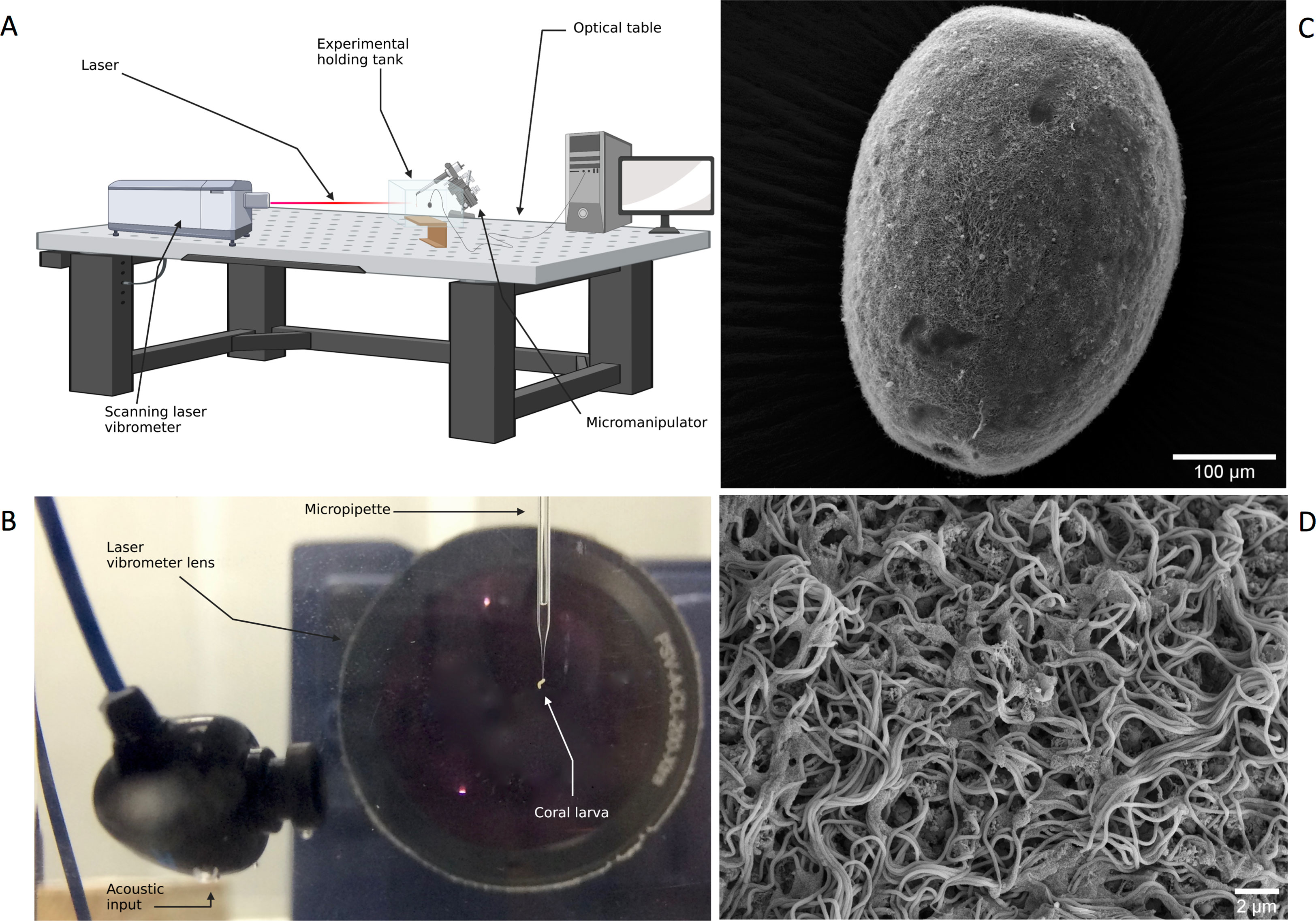
Figure 1 (A) Proposed set-up for coral larvae laser Doppler vibrometry experiment. (B) Close-up of set-up for tethering Acropora millepora larvae in laser Doppler vibrometry experiment (C) Scanning Electron Microscopy (SEM) image of an Acropora millepora planula larva. (D) Magnified larval epiderm highlighting cilia. SEM images: Emelie Brodrick. Laser Doppler Vibrometry schematic (A) created with BioRender.com.
4 Ecological significance and applying acoustic enrichment to reef conservation and restoration
Coral reef soundscapes play an important role in coral larval orientation, habitat location, settlement and recruitment, ultimately affecting reef growth and resilience (Vermeij et al., 2010; Lillis et al., 2016; Lillis et al., 2018). However, with many coral reefs subject to degradation through climate change, overfishing and pollution, reef soundscapes are changing (Spalding and Brown, 2015; Hughes et al., 2017; Hughes et al., 2018; Duarte et al., 2021). For example, between 2012 and 2016, cyclones and intense bleaching meant the Great Barrier Reef experienced the most severe degradation period in recorded history (Hughes et al., 2017). As a result, soundscapes were negatively impacted across four complementary ecoacoustic indices; they were on average 15 dB SPL re 1 µPa quieter and had significantly reduced acoustic complexity, richness and rates of snapping shrimp (Family Alpheidae) snaps (Gordon et al., 2018).
In light of the ecological crisis on coral reefs, novel restoration techniques are becoming increasingly important in the conservation and restoration of these ecosystems. One promising new tool is acoustic enrichment, whereby recordings from relatively healthy coral reefs are played back through underwater speakers (Gordon et al., 2019). This approach has been demonstrated to improve metrics of fish community health in degraded coral reef habitat on an experimental scale (Gordon et al., 2019). Over the natural fish breeding season on the Great Barrier Reef (November-December), this study showed that reefs with acoustic enrichment had increases in fish recruitment across multiple trophic guilds, a doubling in overall fish abundance, and a 50% increase in species richness (Gordon et al., 2019). A subsequent study found that successful management and restoration of coral reefs leads to the recovery of the natural soundscape; maturing restoration projects in Sulawesi exhibited similar levels of acoustic richness to healthy reefs (Lamont et al., 2021).
Recent coral reef restoration efforts have focused on increasing population sizes, genetic diversity and the natural adaptive capacity of corals, for example, through fragment rescue, asexual propagation, in situ and ex situ coral nurseries and sexual propagation in order to mitigate reef degradation caused by climate change and local stressors (Heyward et al., 2002; Cruz and Harrison, 2017, dela Cruz and Harrison, 2020; Suzuki et al., 2020; Randall et al., 2020; Vardi et al., 2021; Harrison et al., 2021; Baums et al., 2019, 2022). In addition, coral breeding efforts in land-based facilities continue to scale up (Craggs et al., 2017; Craggs et al., 2020; O’Neil et al., 2021) while virtually all coral propagation programs seek more efficient ways to induce coral settlement in large numbers without introducing potentially detrimental competing organisms (Randall et al., 2020). Acoustic enrichment can be used in conjunction with all of these newer, breeding-based restoration techniques to help increase settlement rates, population growth and species diversity. By boosting coral settlement at restoration sites, short term acoustic enrichment will also help to restore natural acoustic complexity and phonic richness, thus further accelerating and reinforcing reef recovery.
Current examples of acoustic enhancement in reef restoration include 'The Reef Song Project', an Australian Coral Reef Resilience Initiative (ACRRI) undertaken in association with the Australian Institute of Marine Science (AIMS). This project is the first to investigate the efficacy of acoustic enrichment in situ. Using healthy reef recordings to attract fish communities to sixty patch reefs made of coral rubble and live fragments at Ningaloo Reef and the Great Barrier Reef in Australia, this five-year initiative is primarily exploring the roles of fish husbandry and herbivory on coral growth and reef recovery. Using photogrammetry, coral growth will be monitored over time (Australian Institute of Marine Science, 2023). Additionally, the Woods Hole Oceanographic Institute (WHOI) have developed the ‘Reef Solutions Initiative’. Following the discovery by WHOI scientists that coral larvae are attracted to the soundscapes of healthy reefs (Lillis et al., 2016; Lillis et al., 2018), this initiative seeks to incorporate acoustic enrichment into intervention strategies to help corals repopulate degraded reefs (Woods Hole Oceanographic Institute, 2023). To improve our understanding of the reef recovery process and the impact of reef restoration, the application of low-cost, low specification passive acoustic monitoring in combination with machine-learning analysis may be applied to improve the analysis of ecoacoustic indices and successfully track coral reef restoration (Lamont et al., 2022; Williams et al., 2022).
In sum, acoustic enrichment is a promising tool for coral reef restoration due to its demonstrated efficacy across multiple taxa, yet its potential is still largely untested. Restoring keystone species and re-establishing complex interspecific interactions can promote successful management and restoration of coral reef ecosystems. Reef-building scleractinian corals are keystone species and it is their three-dimensional structure on which all coral reef life forms depend for food, sanctuary and survival. In order to fully assess the potential of acoustic enrichment and effectively apply this method as a reef restoration tool, we must continue to explore how different coral taxa respond to acoustic cues while gaining a better understanding of the mechanisms by which coral larvae sense their acoustic environment. This will also allow us to effectively place acoustics within the hierarchy of sensory cues that coral larvae integrate to locate an optimal site for settlement and recruitment to the reef.
Author contributions
JP, EW and SDS conceived the idea for this mini-review. JP wrote the manuscript. All authors listed made substantial contribution to the discussion of ideas outlined in the work and the development of the manuscript. All authors contributed to the article and approved the submitted version.
Funding
This material is based in part on work supported by NSF grants IOS-1848671 and CBET2133675 (to KLM). EWA is supported by a BBSRC David Phillips Fellowship BB/T00990X/1. SDS is supported by NERC Grant NE/P001572/1.
Acknowledgments
We/The authors would like to thank Prof. Andrew Radford and the extended University of Exeter and University of Bristol Bioacoustics Research Group for their ongoing stimulating discussions, support and insight.
Conflict of interest
The authors declare that the research was conducted in the absence of any commercial or financial relationships that could be construed as a potential conflict of interest.
Publisher’s note
All claims expressed in this article are solely those of the authors and do not necessarily represent those of their affiliated organizations, or those of the publisher, the editors and the reviewers. Any product that may be evaluated in this article, or claim that may be made by its manufacturer, is not guaranteed or endorsed by the publisher.
References
Ainslie M. (2010). Principles of sonar performance modelling. Berlin: Springer. doi: 10.1007/978-3-540-87662-5
Anderson E. R., Butler J., Butler M. J. (2021). Response of fish and invertebrate larvae to backreef sounds at varying distances: Implications for habitat restoration. Front. Mar. Sci. 8, 697. doi: 10.3389/FMARS.2021.663887/BIBTEX
André M., Kaifu K., Solé M., van der Schaar M., Akamatsu T., Balastegui A., et al. (2016). Contribution to the understanding of particle motion perception in marine invertebrates. Adv. Exp. Med. Biol. 875, 47–55. doi: 10.1007/978-1-4939-2981-8_6
Australian Institute of Marine Science (AIMS). (2023). Australian Coral Reef Resilience Initiative. Available at: https://www.aims.gov.au/research-topics/environmental-issues/climate-change/australian-coral-reef-resilience-initiative. (Accessed 21 February 2023).
Babcock R., Davies P. (1991). Effects of sedimentation on settlement of Acropora millepora. Coral Reefs 9, 205–208. doi: 10.1007/BF00290423
Babcock R., Mundy C. (1996). Coral recruitment: Consequences of settlement choice for early growth and survivorship in two scleractinians. J. Exp. Mar. Biol. Ecol. 206, 179–201 doi: 10.1016/S0022-0981(96)02622-6
Babcock R., Smith L. (2000). Effects of sedimentation on coral settlement and survivorship. Mar. Biol. 137 (4), 663–676. doi: 10.1007/s002270000374
Baird A. H., Morse A. N. C. (2004). Induction of metamorphosis in larvae of the brooding corals Acropora palifera and Stylophora pistillata. Mar. Freshw. Res. 55, 469–472. doi: 10.1071/MF03121
Bak R. P. M., Engel M. S. (1979). Distribution, abundance and survival of juvenile hermatypic corals (Scleractinia) and the importance of life history strategies in the parent coral community. Mar. Biol. 54, 341–352. doi: 10.1007/BF00395440
Baker A. C. (1995). “Solar UV-a inhibition of planula larvae in the reef-building coral Pocillopora damicornis. In: Gulko D., Jokiel P. (Eds.), Ultraviolet radiation and coral reefs, (Hawaii: Hawaii Institute of Marine Biology Tech Rep) 41, 149–163.
Baums I. B., Baker A. C., Davies S. W., Grottoli A. G., Kenkel C. D., Kitchen S. A., et al. (2019). Considerations for maximizing the adaptive potential of restored coral populations in the western Atlantic. Ecological Applications 29 (8), e01978.
Baums I. B., Chamberland V. F., Locatelli N. S., Conn T. (2022). Maximizing Genetic Diversity in Coral Restoration Projects. In Coral Reef Conservation and Restoration in the Omics Age (pp. 35–53). Cham: Springer International Publishing.
Bassim K. M., Sammarco P. W. (2003). Effects of temperature and ammonium on larval development and survivorship in a scleractinian coral (Diploria strigosa). Mar. Biol. 142, 241–252. doi: 10.1007/s00227-002-0953-z
Bassim K. M., Sammarco P. W., Snell T. L. (2002). Effects of temperature on success of (self and non-self) fertilization and embryogenesis in Diploria strigosa (Cnidaria, scleractinia). Mar. Biol. 140, 79–88. doi: 10.1007/s00227-001-0722-4
Bertucci F., Parmentier E., Lecellier G., Hawkins A. D., Lecchini D. (2016). Acoustic indices provide information on the status of coral reefs: An example from moorea island in the south pacific. Sci. Rep. 6, 33326. doi: 10.1038/srep33326
Bezares-Calderón L. A., Berger J., Jékely G. (2020). Diversity of cilia-based mechanosensory systems and their functions in marine animal behaviour. Philos. Trans. R. Soc. B: Biol. Sci. 375, 20190137. doi: 10.1098/rstb.2019.0376
Birkeland C. (1977). “The importance of rate of biomass accumulation in early succession stages of benthic communities to the survival of coral recruits,” in Proc. 3rd Int. Coral Reef Symp. 16–21.
Birkeland C., Rowley D., Randall R. H. (1981). Coral recruitment patterns at Guam. In Proc. 4th Int. Coral Reef Symp. 2, 399–344.
Birrell C. L., McCook L. J., Willis B. L. (2005). Effects of algal turfs and sediment on coral settlement. Mar. pollut. Bull. 51, 408–414. doi: 10.1016/j.marpolbul.2004.10.022
Bleckmann H. (2004). 3-d-orientation with the octavolateralis system. J. Physiol. Paris 98, 149–171. doi: 10.1016/j.jphysparis.2004.03.015
Brooke S., Young C. M. (2005). Embryogenesis and larval biology of the ahermatypic scleractinian Oculina varicosa. Mar. Biol. 146, 735–747. doi: 10.1007/s00227-004-1481-9
Budelmann B. U. (1992). Hearing in nonarthropod invertebrates. The Evolutionary Biology of Hearing. 141–155. doi: 10.1007/978-1-4612-2784-7_10
Chalker B. E., Barnes D. J., Dunlap W. C., Jokiel P. L. (1988). Light and reef-building corals. Interdiscip. Sci. Rev. 13, 146–154. doi: 10.1179/isr.1988.13.3.222
Charifi M., Sow M., Ciret P., Benomar S., Massabuau J. C. (2017). The sense of hearing in the pacific oyster, Magallana gigas. PloS One 12 (9), e0185353. doi: 10.1371/journal.pone.0185353
Chia F. S., Buckland-Nicks J., Young C. M. (1984). Locomotion of marine invertebrate larvae: a review. Can. J. Zool. 62, 1205–1222. doi: 10.1139/z84-176
Chia F.-S., Crawford B. (1977). Comparative fine structural studies of planulae and primary polyps of identical age of the sea pen, Ptilosarcus gurneyl. J. Morphol. 151, 321–342. doi: 10.1002/jmor.1051510108
Chia F.-S., Koss R. (1979). Fine structural studies of the nervous system and the apical organ in the planula larva of the sea anemone Anthopleura elegantissima. J. Morphol. 160, 179–196. doi: 10.1002/jmor.1051600303
Crabbe J., Rodriguez-Martinez R., Villamizar E., Goergen L., Croquer A., Banaszak A. (2022). Acropora cervicornis. The IUCN Red List of Threatened Species 2022. Available at: https://www.iucnredlist.org/species/133381/165860142 (Accessed 21 February 2023).
Craggs J., Guest J. R., Davis M., Simmons J., Dashti E., Sweet M. (2017). Inducing broadcast coral spawning ex situ: Closed system mesocosm design and husbandry protocol. Ecol. Evol. 7, 189–198. doi: 10.1002/ece3.3538
Craggs J., Guest J., Davis M., Sweet M. (2020). Completing the life cycle of a broadcast spawning coral in a closed mesocosm. Invertebr. Reprod. Dev. 64, 189–198. doi: 10.1080/07924259.2020.1759704
Cruz D. W. D., Harrison P. L. (2017). Enhanced larval supply and recruitment can replenish reef corals on degraded reefs. Sci. Rep. 17 (1), 1–13. doi: 10.1038/s41598-017-14546-y
Degnan B. M., Johnson C. R. (1999). Inhibition of settlement and metamorphosis of the ascidian Herdmania curvata by non-geniculate coralline algae. Biol. Bull. 197, 298–307. doi: 10.2307/1542787
dela Cruz D. W., Harrison P. L. (2020). Enhancing coral recruitment through assisted mass settlement of cultured coral larvae. PloS One 15 (12), e0242847. doi: 10.1371/JOURNAL.PONE.0242847
Diaz-Pulido G., Harii S., McCook L. J., Hoegh-Guldberg O. (2010). The impact of benthic algae on the settlement of a reef-building coral. Coral Reefs 29, 203–208. doi: 10.1007/s00338-009-0573-x
Dobretsov S., Rittschof D. (2020). Love at first taste: Induction of larval settlement by marine microbes. Int. J. Mol. Sci. 21, 731. doi: 10.3390/ijms21030731
Duarte C. M., Chapuis L., Collin S. P., Costa D. P., Devassy R. P., Eguiluz V. M., et al. (2021). The soundscape of the anthropocene ocean. Science 371 (6529), eaba4658. doi: 10.1126/science.aba4658
Edmonds N. J., Firmin C. J., Goldsmith D., Faulkner R. C., Wood D. T. (2016). A review of crustacean sensitivity to high amplitude underwater noise: Data needs for effective risk assessment in relation to UK commercial species. Mar. pollut. Bull. 108, 5–13. doi: 10.1016/j.marpolbul.2016.05.006
Edmunds P., Gates R., Gleason D. (2001). The biology of larvae from the reef coral Porites astreoides, and their response to temperature disturbances. Mar. Biol. 139, 981–989. doi: 10.1007/s002270100634
Edmunds P. J., Gates R. D., Leggat W., Hoegh-Guldberg O., Allen-Requa L. (2005). The effect of temperature on the size and population density of dinoflagellates in larvae of the reef coral Porites astreoides. Invertebrate Biol. 124, 366–373. doi: 10.1111/j.1744-7410.2005.00018.x
Erwin P. M., Song B., Szmant A. M. (2008). “Settlement behavior of Acropora palmata planulae: Effects of biofilm age and crustose coralline algal cover,” in Proceedings of the 11th International Coral Reef Symposium, Florida, 1219–1223.
Forward R. B. (1989). Depth regulation of larval marine decapod crustaceans: test of an hypothesis. Mar. Biol. 102, 397–404. doi: 10.1007/BF00428280
Forward R. B. (1990). Responses of crustacean larvae to hydrostatic pressure: Behavioral basis of high barokinesis. Mar. Behav. Physiol. 17, 1–17. doi: 10.1080/10236249009378773
Foster T., Gilmour J. P. (2016). Seeing red: Coral larvae are attracted to healthy-looking reefs. Mar. Ecol. Prog. Ser. 559, 141–153. doi: 10.3354/meps11902
Freeman L. A., Freeman S. E. (2016). Rapidly obtained ecosystem indicators from coral reef soundscapes. Mar. Ecol. Prog. Ser. 561, 1–14. doi: 10.3354/meps11938
Freeman G., Ridgway E. B. (1990). Cellular and intracellular pathways mediating the metamorphic stimulus in hydrozoan planulae. Roux’s Arch. Dev. Biol. 199, 357–365. doi: 10.1007/BF02029553
Gaylord B., Hodin J., Ferner M. C. (2013). Turbulent shear spurs settlement in larval sea urchins. Proc. Natl. Acad. Sci. U.S.A. 110, 6901–6906. doi: 10.1073/pnas.1220680110
Gilmour J. (1999). Experimental investigation into the effects of suspended sediment on fertilisation, larval survival and settlement in a scleractinian coral. Mar. Biol. 135, 217–225. doi: 10.1007/s002270050645
Gleason D. F., Danilowicz B. S., Nolan C. J. (2009). Reef waters stimulate substratum exploration in planulae from brooding Caribbean corals. Coral Reefs 28, 391–398. doi: 10.1007/s00338-009-0480-1
Gleason D. F., Edmunds P. J., Gates R. D. (2006). Ultraviolet radiation effects on the behavior and recruitment of larvae from the reef coral Porites astreoides. Mar. Biol. 148, 503–512. doi: 10.1007/s00227-005-0098-y
Gleason D. F., Hofmann D. K. (2011). Coral larvae: From gametes to recruits. J. Exp. Mar. Biol. Ecol. 408, 42–57. doi: 10.1016/j.jembe.2011.07.025
Gleason D. F., Wellington G. M. (1995). Variation in UVB sensitivity of planula larvae of the coral Agaricia agaricites along a depth gradient. Mar. Biol. 123, 693–703. doi: 10.1007/BF00349112
Goh B. P. L., Lee C. S. (2008). “A study of the effect of sediment accumulation on the settlement of coral larvae using conditioned tiles,” in Proceedings of the 11th International Coral Reef Symposium. (Florida: Ft. Lauderdale) 7–11.
Golbuu Y., Richmond R. H. (2007). Substratum preferences in planula larvae of two species of scleractinian corals, Goniastrea retiformis and Stylaraea punctata. Mar. Biol. 152, 565–572. doi: 10.1007/s00227-007-0717-x
Goldsteins J. S., Butler I. V. M. J. (2009). Behavioral enhancement of onshore transport by postlarval Caribbean spiny lobster (Panulirus argus). Limnol. Oceanogr. 54, 1669–1674. doi: 10.4319/lo.2009.54.5.1669
Gómez-Lemos L. A., Doropoulos C., Bayraktarov E., Diaz-Pulido G. (2018). Coralline algal metabolites induce settlement and mediate the inductive effect of epiphytic microbes on coral larvae. Sci. Rep. 8, 16590. doi: 10.1038/s41598-018-35206-9
Göpfert M. C., Briegel H., Robert D. (1999). Mosquito hearing: Sound-induced antennal vibrations in male and female Aedes aegypti. J. Exp. Biol. 202, 2727–2738. doi: 10.1242/jeb.202.20.2727
Göpfert M. C., Robert D. (2002). The mechanical basis of drosophila audition. J. Exp. Biol. 205, 1199–1208. doi: 10.1242/jeb.205.9.1199
Gordon T. A. C., Harding H. R., Wong K. E., Merchant N. D., Meekan M. G., McCormick M. I., et al. (2018). Habitat degradation negatively affects auditory settlement behavior of coral reef fishes. Proc. Natl. Acad. Sci. U.S.A. 115, 5193–5198. doi: 10.1073/pnas.1719291115
Gordon T. A. C., Radford A. N., Davidson I. K., Barnes K., McCloskey K., Nedelec S. L., et al. (2019). Acoustic enrichment can enhance fish community development on degraded coral reef habitat. Nat. Commun. 10, 5413. doi: 10.1038/s41467-019-13186-2
Hadfield M. G., Paul V. J. (2001). “Natural chemical cues for settlement and metamorphosis of marine-invertebrate larvae,” in Marine Chemical Ecology, ed. McClintock J. B., Baker B. J. (Boca Raton, FL: CRC Press).
Harrington L., Fabricius K., De’Ath G., Negri A. (2004). Recognition and selection of settlement substrata determine post-settlement survival in corals. Ecology 85, 3428–3437. doi: 10.1890/04-0298
Harriott V. J. (1985). “Recruitment patterns of scleractinian corals at Lizard Island, Great Barrier Reef,” in Proceedings Of The Fifth International Coral Reef Congress. Tahiti, 27 May -1 June 1985. Symposia and Seminars (B), ed. Richmond R. H. (Miami, FL: International Society for Reef Studies) 4, 367–372.
Harrison P. L., dela Cruz D. W., Cameron K. A., Cabaitan P. C. (2021). Increased coral larval supply enhances recruitment for coral and fish habitat restoration. Front. Mar. Sci. 8, 1786. doi: 10.3389/FMARS.2021.750210/BIBTEX
Hartmann A. C., Marhaver K. L., Chamberland V. F., Sandin S. A., Vermeij M. J. A. (2013). Large Birth size does not reduce negative latent effects of harsh environments across life stages in two coral species. Ecology 94, 1826–1837. doi: 10.1890/13-0161.1
Hata T., Madin J. S., Cumbo V. R., Denny M., Figueiredo J., Harii S., et al. (2017). Coral larvae are poor swimmers and require fine-scale reef structure to settle. Sci. Rep. 7, 1–10. doi: 10.1038/s41598-017-02402-y
Hay M. E. (2009). Marine chemical ecology: Chemical signals and cues structure marine populations, communities, and ecosystems. Ann. Rev. Mar. Sci. 1, 193–212. doi: 10.1146/annurev.marine.010908.163708
Heyward A. J., Negri A. P. (1999). Natural inducers for coral larval metamorphosis. Coral Reefs 18, 273–279. doi: 10.1007/s003380050193
Heyward A. J., Negri A. P. (2010). Plasticity of larval pre-competency in response to temperature: Observations on multiple broadcast spawning coral species. Coral Reefs 29, 631–636. doi: 10.1007/s00338-009-0578-5
Heyward A. J., Smith L. D., Rees M., Field S. N. (2002). Enhancement of coral recruitment by in situ mass culture of coral larvae. Mar. Ecol. Prog. Ser. 230, 113–118. doi: 10.3354/MEPS230113
Hinojosa I. A., Green B. S., Gardner C., Hesse J., Stanley J. A., Jeffs A. G. (2016). Reef sound as an orientation cue for shoreward migration by pueruli of the rock lobster, Jasus edwardsii. PloS One 11, e0157862. doi: 10.1371/journal.pone.0157862
Hodgson G. (1990). Sediment and the settlement of larvae of the reef coral Pocillopora damicornis. Coral Reefs 9, 41–43. doi: 10.1007/BF00686720
Hodin J., Ferner M. C., Ng G., Gaylord B. (2018). Sand dollar larvae show within-population variation in their settlement induction by turbulence. Biol. Bull. 235, 116–127. doi: 10.1086/699827
Hughes T. P., Kerry J. T., Álvarez-Noriega M., Álvarez-Romero J. G., Anderson K. D., Baird A. H., et al. (2017). Global warming and recurrent mass bleaching of corals. Nature 543, 373–377. doi: 10.1038/nature21707
Hughes T. P., Kerry J. T., Baird A. H., Connolly S. R., Dietzel A., Eakin C. M., et al. (2018). Global warming transforms coral reef assemblages. Nature 556, 492–496. doi: 10.1038/s41586-018-0041-2
Humanes R., Ricardo G. F., Willis B. L., Fabricius K. E., Negri A. P. (2017). Cumulative effects of suspended sediments, organic nutrients and temperature stress on early life history stages of the coral Acropora tenuis. Sci. Rep. 7, 44101. doi: 10.1038/srep44101
Jeffs A., Tolimieri N., Montgomery J. C. (2003). Crabs on cue for the coast: The use of underwater sound for orientation by pelagic crab stages. Mar. Freshw. Res. 54, 953–956. doi: 10.1071/MF03007
Jézéquel Y., Bonnel J., Coston-Guarini J., Guarini J. M., Chauvaud L. (2018). Sound characterization of the European lobster Homarus gammarus in tanks. Aquat. Biol. 27, 195–202. doi: 10.3354/ab00692
Jokiel P., Guinther E. (1978). Effects of temperature on reproduction in the hermatypic coral Pocillopora damicornis. Bull. Mar. Sci. 28, 728–734. doi: 10.1007/978-3-030-16663-7_68
Jones R., Ricardo G. F., Negri A. P. (2015). Effects of sediments on the reproductive cycle of corals. Mar. pollut. Bull. 100, 13–33. doi: 10.1016/j.marpolbul.2015.08.021
Jorissen H., Galand P. E., Bonnard I., Meiling S., Raviglione D., Meistertzheim A. L., et al. (2021). Coral larval settlement preferences linked to crustose coralline algae with distinct chemical and microbial signatures. Sci. Rep. 11, 20931. doi: 10.1038/s41598-021-94096-6
Kaifu K., Akamatsu T., Segawa S. (2008). Underwater sound detection by cephalopod statocyst. Fisheries Sci. 74, 1261–1268. doi: 10.1111/j.1444-2906.2008.01589.x
Keats D. W., Knight M. A., Pueschel C. M. (1997). Antifouling effects of epithallial shedding in three crustose coralline algae (Rhodophyta, coralinales) on a coral reef. J. Exp. Mar. Biol. Ecol. 213, 281–294. doi: 10.1016/S0022-0981(96)02771-2
Kennedy V. S., Newell R. I. E., Eble A. F. (1996). The eastern oyster: Crassostrea virginica (Maryland Sea Grant: College Park), 467, p.513.
Kingsford M. J., Leis J. M., Shanks A., Lindeman K. C., Morgan S. G., Pineda J. (2002). Sensory environments, larval abilities and local self-recruitment. Bull. Mar. Sci. 70, 309–340. doi: 10.5343/bms.2002.1076
Kuffner I. B. (2001). Effects of ultraviolet (UV) radiation on larval settlement of the reef coral Pocillopora damicornis. Mar. Ecol. Prog. Ser. 217, 251–261. doi: 10.3354/meps217251
Lamont T. A. C., Chapuis L., Williams B., Dines S., Gridley T., Frainer G., et al. (2022). HydroMoth: Testing a prototype low-cost acoustic recorder for aquatic environments. Remote Sens Ecol. Conserv. 8, 91–102. doi: 10.1002/rse2.249
Lamont T. A. C., Williams B., Chapuis L., Prasetya M. E., Seraphim M. J., Harding H. R., et al. (2021). The sound of recovery: Coral reef restoration success is detectable in the soundscape. J. Appl. Ecol. 58 (3), 742–756. doi: 10.1111/1365-2664.14089
Lecchini D., Bertucci F., Gache C., Khalife A., Besson M., Roux N., et al. (2018). Boat noise prevents soundscape-based habitat selection by coral planulae. Sci. Rep. 8, 10552. doi: 10.1038/s41598-018-27674-w
Leis J. M., Lockett M. M. (2005). Localization of reef sounds by settlement-stage larvae of coral-reef fishes (Pomacentridae). Bull. Mar. Sci. 76, 59–74. doi: 10.4319/2005.76.3.12
Levenstein M. A., Gysbers D. J., Marhaver K. L., Kattom S., Tichy L., Quinlan Z., et al. (2022). Millimeter-scale topography facilitates coral larval settlement in wave-driven oscillatory flow. PloS One 17 (9), p.e0274088. doi: 10.1371/journal.pone.0274088
Lewis J. B. (1974). The settlement behaviour of planulae larvae of the hermatypic coral Favia fragum (Esper). J. Exp. Mar. Biol. Ecol. 15, 191–203. doi: 10.1016/0022-0981(74)90042-2
Lillis A., Apprill A., Suca J. J., Becker C., Llopiz J. K., Mooney T. A. (2018). Soundscapes influence the settlement of the common Caribbean coral Porites astreoides irrespective of light conditions. R Soc. Open Sci. 5 (12), 181358. doi: 10.1098/rsos.181358
Lillis A., Bohnenstiehl D. W. R., Eggleston D. B. (2015). Soundscape manipulation enhances larval recruitment of a reef-building mollusk. PeerJ 3, e999. doi: 10.7717/peerj.999
Lillis A., Bohnenstiehl D. W., Peters J. W., Eggleston D. (2016). Variation in habitat soundscape characteristics influences settlement of a reef-building coral. PeerJ 4, e2557. doi: 10.7717/peerj.2557
Lillis A., Eggleston D. B., Bohnenstiehl D. R. (2013). Oyster larvae settle in response to habitat-associated underwater sounds. PloS One 8, e79337. doi: 10.1371/journal.pone.0079337
Lobel P. S. (2013). The “Choral Reef”: The ecology of underwater sounds. In Joint International Scientific Diving Symposium. 179–185.
Lobel P. S., Kaatz I. M., Rice A. N. (2010). “Acoustical behavior of coral reef fishes,” in Reproduction and sexuality in marine fishes: Patterns and processes. 131–150. doi: 10.1525/california/9780520264335.003.0010
Lyons K. M. (1973). Collar cells in planula and adult tentacle ectoderm of the solitary coral Balanophyllia regia (anthozoa eupsammiidae). Z. für Zellforschung und Mikroskopische Anatomie 145, 491–503. doi: 10.1007/BF00307189
Marlow H. Q., Srivastava M., Matus D. Q., Rokhsar D., Martindale M. Q. (2009). Anatomy and development of the nervous system of Nematostella vectensis, an anthozoan cnidarian. Dev. Neurobiol. 69, 235–254. doi: 10.1002/dneu.20698
Masaki T., Fujita D., Hagen N. T. (1984). The surface ultrastructure and epithallium shedding of crustose coralline algae in an “Isoyake” area of southwestern Hokkaido, Japan. Hydrobiologia, 116–117, 43–49. doi: 10.1007/BF00027669
Mason B., Beard M., Miller M. W. (2011). Coral larvae settle at a higher frequency on red surfaces. Coral Reefs 30, 183–191. doi: 10.1007/s00338-011-0739-1
Mayorga-Adame C. G., Batchelder H. P., Spitz Y. H. (2017). Modeling larval connectivity of coral reef organisms in the Kenya-Tanzania region. Front. Mar. Sci.. 4, 92. doi: 10.3389/fmars.2017.00092
McWilliam J. N., McCauley R. D., Erbe C., Parsons M. J. G. (2017). Soundscape diversity in the great barrier reef: Lizard island, a case study. Int. J. Anim. Sound its Recording 27, 1–18. doi: 10.1080/09524622.2017.1344930
Montgomery J. C., Jeffs A., Simpson S. D., Meekan M., Tindle C. (2006). Sound as an orientation cue for the pelagic larvae of reef fishes and decapod crustaceans. Adv. Mar. Biol. 51, 143–196. doi: 10.1016/S0065-2881(06)51003-X
Mooney T. A., Hanlon R. T., Christensen-Dalsgaard J., Madsen P. T., Ketten D. R., Nachtigall P. E. (2010). Sound detection by the longfin squid (Loligo pealeii) studied with auditory evoked potentials: Sensitivity to low-frequency particle motion and not pressure. J. Exp. Biol. 213, 3748–3759. doi: 10.1242/jeb.048348
Morgan E. (1984). The pressure-responses of marine invertebrates: a psychophysical perspective. Zool. J. Linn. Soc. 80, 209–230. doi: 10.1111/J.1096-3642.1984.TB01974.X
Morse D. E., Hooker N., Morse A. N. C., Jensen R. A. (1988). Control of larval metamorphosis and recruitment in sympatric agariciid corals. J. Exp. Mar. Biol. Ecol. 116, 193–217. doi: 10.1016/0022-0981(88)90027-5
Morse A. N. C., Iwao K., Baba M., Shimoike K., Hayashibara T., Omori M. (1996). An ancient chemosensory mechanism brings new life to coral reefs. Biol. Bull. 191, 149–154. doi: 10.2307/1542917
Morse D. E., Morse A. N. C. (1991). Enzymatic characterization of the morphogen recognized by Agaricia humilis (Scleractinian coral) larvae. Biol. Bull. 181, 363–373. doi: 10.2307/1542493
Morse D. E., Morse A. N. C., Raimondi P. T., Hooker N. (1994). Morphogen-based chemical flypaper for Agaricia humilis coral larvae. Biol. Bull. 186, 72–81. doi: 10.2307/1542051
Mulla A. J., Lin C. H., Takahashi S., Nozawa Y. (2021). Photo-movement of coral larvae influences vertical positioning in the ocean. Coral Reefs 40, 1297–1306. doi: 10.1007/S00338-021-02141-7/FIGURES/5
Mundy C. N., Babcock R. C. (1998). Role of light intensity and spectral quality in coral settlement: Implications for depth-dependent settlement? J. Exp. Mar. Biol. Ecol. 223, 235–255. doi: 10.1016/S0022-0981(97)00167-6
Nedelec S. L., Campbell J., Radford A. N., Simpson S. D., Merchant N. D. (2016). Particle motion: the missing link in underwater acoustic ecology. Methods Ecol. Evol. 7, 836–842. doi: 10.1111/2041-210X.12544
Negri A. P., Webster N. S., Hill R. T., Heyward A. J. (2001). Metamorphosis of broadcast spawning corals in response to bacteria isolated from crustose algae. Mar. Ecol. Prog. Ser. 223, 121–131. doi: 10.3354/meps223121
Nozawa Y., Harrison P. L. (2005). Temporal settlement patterns of larvae of the broadcast spawning reef coral Favites chinensis and the broadcast spawning and brooding reef coral Goniastrea aspera from Okinawa, Japan. Coral Reefs 24, 324–332. doi: 10.1007/s00338-005-0476-4
O’Neil K. L., Serafin R. M., Patterson J. T., Craggs J. R. K. (2021). Repeated ex situ spawning in two highly disease susceptible corals in the family meandrinidae. Front. Mar. Sci. 8, 669976. doi: 10.3389/fmars.2021.669976
Paris C. B., Chérubin L. M., Cowen R. K. (2007). Surfing, spinning, or diving from reef to reef: Effects on population connectivity. Mar. Ecol. Prog. Ser. 347, 285–300. doi: 10.3354/meps06985
Pawlik J. R. (1992). Chemical ecology of the settlement of benthic marine invertebrates. Oceanography and Marine Biology: An Annual Review, 30, 273–335.
Perez K. III, Rodgers K. S., Jokiel P. L., Lager C. V., Lager D. J. (2014). Effects of terrigenous sediment on settlement and survival of the reef coral Pocillopora damicornis. Peer J. 2, e387. doi: 10.7717/peerj.387
Piercy J. J. B., Codling E. A., Hill A. J., Smith D. J., Simpson S. D. (2014). Habitat quality affects sound production and likely distance of detection on coral reefs. Mar. Ecol. Prog. Ser. 516, 31–44. doi: 10.3354/meps10986
Popper A. N., Fay R. R. (1999). The auditory periphery in fishes. Comparative hearing: fish and amphibians. 43–100. doi: 10.1007/978-1-4612-0533-3_3
Popper A. N., Lu Z. (2000). Structure-function relationships in fish otolith organs. Fisheries research. 46 (1-3), 15–25. doi: 10.1016/S0165-7836(00)00129-6
Popper A. N., Salmon M., Horch K. W. (2001). Acoustic detection and communication by decapod crustaceans. J. Comp. Physiol. A: Sensory, Neural, and Behavioral Physiology 187 (2), 83–89. doi: 10.1007/s003590100184
Putnam H. M., Edmunds P. J., Fan T. Y. (2008). Effect of temperature on the settlement choice and photophysiology of larvae from the reef coral Stylophora pistillata. Biol. Bull. 215 (2), 135–142. doi: 10.2307/25470694
Radford A. N., Kerridge E., Simpson S. D. (2014). Acoustic communication in a noisy world: Can fish compete with anthropogenic noise? Behav. Ecol. 25 (5), 1022–1030. doi: 10.1093/beheco/aru029
Radford C. A., Stanley J. A., Simpson S. D., Jeffs A. G. (2011). Juvenile coral reef fish use sound to locate habitats. Coral Reefs 30 (2), 295–305. doi: 10.1007/s00338-010-0710-6
Randall C. J., Negri A. P., Quigley K. M., Foster T., Ricardo G. F., Webster N. S., et al. (2020). Sexual production of corals for reef restoration in the anthropocene. Mar. Ecol. Prog. Ser. 635, 1–19. doi: 10.3354/MEPS13206
Randall C. J., Szmant A. M. (2009a). Elevated temperature affects development, survivorship, and settlement of the elkhorn coral, Acropora palmata (Lamarck 1816). Biol. Bull. 217 (3), 269–282. doi: 10.1086/BBLv217n3p269
Randall C. J., Szmant A. M. (2009b). Elevated temperature reduces survivorship and settlement of the larvae of the Caribbean scleractinian coral, Favia fragum (Esper). Coral Reefs 28 (4), 917–925. doi: 10.1007/s00338-009-0482-z
Ricardo G. F., Jones R. J., Nordborg M., Negri A. P. (2017). Settlement patterns of the coral Acropora millepora on sediment-laden surfaces. Sci. Total Environ. 609, 1367–1378. doi: 10.1016/j.scitotenv.2017.07.153
Richmond R. H., Tisthammer K. H., Spies N. P. (2018). The effects of anthropogenic stressors on reproduction and recruitment of corals and reef organisms. Front. Mar. Sci. 5, 226. doi: 10.3389/fmars.2018.00226
Ritson-Williams R., Arnold S., Fogarty N., Steneck R. S., Vermeij M., Paul V. J. (2009). New perspectives on ecological mechanisms affecting coral recruitment on reefs. Smithson Contrib. Mar. Sci. 38, 437–457. doi: 10.5479/si.01960768.38.437
Ritson-Williams R., Arnold S. N., Paul V. J. (2016). Patterns of larval settlement preferences and post-settlement survival for seven Caribbean corals. Mar. Ecol. Prog. Ser. 548, 127–138. doi: 10.3354/meps11688
Ritson-Williams R., Arnold S. N., Paul V. J., Steneck R. S. (2014). Larval settlement preferences of Acropora palmata and Montastraea faveolata in response to diverse red algae. Coral Reefs 33, 1119–1124. doi: 10.1007/s00338-013-1113-2
Ritson-Williams R., Paul V. J., Arnold S. N., Steneck R. S. (2010). Larval settlement preferences and post-settlement survival of the threatened Caribbean corals Acropora palmata and A. cervicornis. Coral Reefs 29, 71–79. doi: 10.1007/s00338-009-0555-z
Roberts C. M. (1997). Connectivity and management of caribbean coral reefs. Science 278 (5342), 1454–1457. doi: 10.1126/SCIENCE.278.5342.1454
Rodriguez S. R., Ojeda F. P., Inestrosa N. C. (1993). Settlement of benthic marine invertebrates. Mar. Ecol. Prog. Ser. 97, 135–148. doi: 10.3354/meps097193
Rogers P. H., Cox M. (1988). Underwater sound as a biological stimulus. Sensory Biol. Aquat. Anim., 131–149. doi: 10.1007/978-1-4612-3714-3_5
Rogers C. S., Fitz H. C., Gilnack M., Beets J., Hardin J. (1984). Scleractinian coral recruitment patterns at salt river submarine canyon, st. Croix, U.S. virgin islands. Coral Reefs 3 (2), 69–76. doi: 10.1007/BF00263756
Rothberg A. J., Allen M. S., Di Maio D., Dirckx J. J. J., Ewins D. J., Halkon B. J., et al. (2017). An international review of laser Doppler vibrometry: Making light work of vibration measurements. Optics Lasers Eng. 99, 120–126. doi: 10.1016/j.optlaseng.2016.10.023
Sakai Y., Kato K., Koyama H., Kuba A., Takahashi H., Fujimori T., et al. (2020). A step-down photophobic response in coral larvae: implications for the light-dependent distribution of the common reef coral, Acropora tenuis. Sci. Rep. 10 (1), 1–11. doi: 10.1038/s41598-020-74649-x
Salmon M., Horch K. (1973). Vibration reception by the fiddler crab, Uca minax. Comp. Biochem. Physiol. A Physiol. 44 (2), 485–493. doi: 10.1016/0300-9629(73)90506-9
Schmitz B. (2002). Sound production in Crustacea with special reference to the alpheidae. In The Crustacean Nervous System. 269–282. Springer Berlin Heidelberg.
Shanks A. L. (2009). Pelagic larval duration and dispersal distance revisited. Biol. Bull. 216, 373–385. doi: 10.1086/bblv216n3p373
Siboni N., Abrego D., Puill-Stephan E., King W. L., Bourne D. G., Raina J. B., et al. (2020). Crustose coralline algae that promote coral larval settlement harbor distinct surface bacterial communities. Coral Reefs 39, 1703–1713. doi: 10.1007/S00338-020-01997-5
Siboni N., Abrego D., Seneca F., Motti C. A., Andreakis N., Tebben J., et al. (2012). Using bacterial extract along with differential gene expression in Acropora millepora larvae to decouple the processes of attachment and metamorphosis. PloS One 7, e37774. doi: 10.1371/journal.pone.0037774
Simpson S. D., Meekan M. G., Jeffs A., Montgomery J. C., McCauley R. D. (2008). Settlement-stage coral reef fish prefer the higher-frequency invertebrate-generated audible component of reef noise. Anim. Behav. 75, 1831–1838. doi: 10.1016/j.anbehav.2007.11.004
Simpson S. D., Meekan M. G., McCauley R. D., Jeffs A. (2004). Attraction of settlement-stage coral reef fishes to reef noise. Mar. Ecol. Prog. Ser. 276, 263–268. doi: 10.3354/meps276263
Simpson S. D., Meekan M., Montgomery J., McCauley R., Jeffs A. (2005). Homeward sound. Sci. (1979) 308, 221. doi: 10.1126/science.1107406
Simpson S. D., Radford A. N., Tickle E. J., Meekan M. G., Jeffs A. G. (2011). Adaptive avoidance of reef noise. PloS One 6, e16625. doi: 10.1371/journal.pone.0016625
Sneed J. M., Riston-Williams R., Paul. V. J. (2015). Crustose coralline algal species host distinct bacterial assemblages on their surfaces. ISME J. 9, 2527–2536. doi: 10.1038/ismej.2015.67
Sneed J. M., Sharp K. H., Ritchie K. B., Paul V. J. (2014). The chemical cue tetrabromopyrrole from a biofilm bacterium induces settlement of multiple Caribbean corals. Proc. R. Soc. B: Biol. Sci. 281, 20133086. doi: 10.1098/rspb.2013.3086
Solé M., Lenoir M., Fontuño J. M., Durfort M., van der Schaar M., André M. (2016). Evidence of cnidarians sensitivity to sound after exposure to low frequency noise underwater sources. Sci. Rep. 6, 37979. doi: 10.1038/srep37979
Spalding M. D., Brown B. E. (2015). Warm-water coral reefs and climate change. Sci. (1979) 350, 769–771. doi: 10.1126/science.aad0349
Stake J. L., Sammarco P. W. (2003). Effects of pressure on swimming behavior in planula larvae of the coral Porites astreoides (Cnidaria, scleractinia). J. Exp. Mar. Biol. Ecol. 288, 223–238. doi: 10.1016/S0022-0981(03)00018-2
Stanley J. A., Radford C. A., Jeffs A. G. (2010). Induction of settlement in crab megalopae by ambient underwater reef sound. Behav. Ecol. 21, 113–120. doi: 10.1093/beheco/arp159
Stanley J. A., Radford C. A., Jeffs A. G. (2012a). Effects of underwater noise on larval settlement. Adv. Exp. Med. Biol. 730, 371–374. doi: 10.1007/978-1-4419-7311-5_84
Stanley J. A., Radford C. A., Jeffs A. G. (2012b). Location, location, location: Finding a suitable home among the noise. Proc. R. Soc. B: Biol. Sci. 279, 1050–1056. doi: 10.1098/rspb.2012.0697
Stocks J. R. (2012). Response of marine invertebrate larvae to natural and anthropogenic sound: A pilot study. Open Mar. Biol. J. 6. doi: 10.2174/1874450801206010057
Strader M. E., Davies S. W., Matz M. V. (2015). Differential responses of coral larvae to the color of ambient light guide them to suitable settlement microhabitat. R Soc. Open Sci. 2, 150358. doi: 10.1098/rsos.150358
Sutton G. P., Clarke D., Morley E. L., Robert D. (2016). Mechanosensory hairs in bumblebees (Bombus terrestris) detect weak electric fields. Proc. Natl. Acad. Sci. U.S.A. 113, 7261–7265. doi: 10.1073/pnas.1601624113
Suzuki G., Okada W., Yasutake Y., Yamamoto H., Tanita I., Yamashita H., et al. (2020). Enhancing coral larval supply and seedling production using a special bundle collection system “coral larval cradle” for large-scale coral restoration. Restor. Ecol. 28, 1172–1182. doi: 10.1111/REC.13178
Suzuki Y., Takabayashi T., Kawaguchi T., Matsunaga K. (1998). Isolation of an allelopathic substance from the crustose coralline algae, Lithophyllum spp., and its effect on the brown alga, Laminaria religiosa miyabe (Phaeophyta). J. Exp. Mar. Biol. Ecol. 225, 69–77. doi: 10.1016/S0022-0981(97)00208-6
Szmant A. M., Meadows M. G. (2006). “Developmental changes in coral larval buoyancy and vertical swimming behavior: Implications for dispersal and connectivity,” in Proceedings of the 10th International Coral Reef Symposium, Vol. 1, 431–437.
Tebben J., Motti C. A., Siboni N., Tapiolas D. M., Negri A. P., Schupp P. J., et al. (2015). Chemical mediation of coral larval settlement by crustose coralline algae. Sci. Rep. 5, 10803. doi: 10.1038/srep10803
Tebben J., Tapiolas D. M., Motti C. A., Abrego D., Negri A. P., Blackall L. L., et al. (2011). Induction of larval metamorphosis of the coral Acropora millepora by tetrabromopyrrole isolated from a Pseudoalteromonas bacterium. PloS One 6, e19082. doi: 10.1371/journal.pone.0019082
Thorson G. (1950). Reproductive and larval ecology of marine bottom invertebrates. Biol. Rev. 25, 1–45. doi: 10.1111/j.1469-185X.1950.tb00585.x
Tolimieri N., Haine O., Montgomery J. C., Jeffs A. (2002). Ambient sound as a navigational cue for larval reef fish. Bioacoustics 12, 283–284. doi: 10.1080/09524622.2002.9753700
Tolimieri N., Jeffs A., Montgomery J. C. (2000). Ambient sound as a cue for navigation by the pelagic larvae of reel fishes. Mar. Ecol. Prog. Ser. 207, 219–224. doi: 10.3354/meps207219
Tran C., Hadfield M. G. (2011). Larvae of Pocillopora damicornis (Anthozoa) settle and metamorphose in response to surface-biofilm bacteria. Mar. Ecol. Prog. Ser. 433, 85–96. doi: 10.3354/meps09192
Tran C., Hadfield M. G. (2013). Localization of sensory mechanisms utilized by coral planulae to detect settlement cues. Invertebrate Biol. 132, 113–121. doi: 10.1111/ivb.12025
Tranter P. R. G., Nicholson D. N., Kinchington D. (1982). A description of spawning and post-gastrula development of the cool temperate coral, Caryophyllia smithi. J. Mar. Biol. Assoc. United Kingdom 62, 343–359. doi: 10.1017/s0025315400044106
Tuttle L. J., Donahue M. J. (2022). Effects of sediment exposure on corals: a systematic review of experimental studies. Environ. Evid. 11, 2. doi: 10.1186/s13750-022-00256-0
Urick R. J. (1983). Principles of underwater sound, 3rd Edition. Peninsula Publising Los Atlos, California, 22, pp.23–24.
Vandermeulen J. H. (1975). Studies on reef corals. III. fine structural changes of calicoblast cells in Pocillopora damicornis during settling and calcification. Mar. Biol. 31, 283–288. doi: 10.1007/BF00390649
Vardi T., Hoot W. C., Levy J., Shaver E., Winters R. S., Banaszak A. T., et al. (2021). Six priorities to advance the science and practice of coral reef restoration worldwide. Restoration Ecology 29 (8), e13498.
Vazzana M., Celi M., Maricchiolo G., Genovese L., Corrias V., Quinci E. M., et al. (2016). Are mussels able to distinguish underwater sounds? assessment of the reactions of Mytilus galloprovincialis after exposure to lab-generated acoustic signals. Comp. Biochem. Physiol. A Mol. Integr. Physiol. 201, 75–82. doi: 10.1016/j.cbpa.2016.06.029
Vermeij M. J. A., Marhaver K. L., Huijbers C. M., Nagelkerken I., Simpson S. D. (2010). Coral larvae move toward reef sounds. PloS One 5, e10660. doi: 10.1371/journal.pone.0010660
Vermeij M. J. A., Sandin S. A. (2008). Density-dependent settlement and mortality structure the earliest life phases of a coral population. Ecology 89, 1997–2002. doi: 10.1890/07-1296.1
Veron J. E. N. (2000). Corals of the world. 1st ed. Ed. Stafford-Smith Townsville M. (Mc, Queensland: Australian Institute of Marine Science and CRR Ald Pty Ltd). 138–139.
Veron J. E. N. (2011). “Corals: Biology, skeletal deposition, and reef-building,” in Encyclopedia of modern coral reefs: Structure, form and process. 275–281. doi: 10.1007/978-90-481-2639-2_9
Veron J., Stafford-Smith M., DeVantier L., Turak E. (2015). Overview of distribution patterns of zooxanthellate scleractinia. Front. Mar. Sci. 2, 81. doi: 10.3389/fmars.2014.00081
Wale M. A. (2017). The effects of anthropogenic noise playbacks on marine invertebrates (Edinburgh Napier University).
Wallace C. (1985). Seasonal peaks and annual fluctuations in recruitment of juvenile scleractinian corals. Mar. Ecol. Prog. Ser. 21, 289. doi: 10.3354/meps021289
Wallace C. C., Bull G. D. (1981). “Patterns of juvenile coral recruitment on a reef front during a spring-summer spawning period,” in Proceedings of the 4th International Coral Reef Symposium, Manila. 2, pp. 345–350.
Webster N. S., Smith L. D., Heyward A. J., Watts J. E., Webb R. I., Blackall L. L., et al. (2004). Metamorphisis of a Scleractinian Coral in Response to Microbial Biofilms. Appl. Environ. Microbiol. 70 (2), 1213–1221.
Wellington G. M., Fitt W. K. (2003). Influence of UV radiation on the survival of larvae from broadcast-spawning reef corals. Mar. Biol. 143, 1075–1082. doi: 10.1007/s00227-003-1150-4
Whalan S., Webster N. S., Negri A. P. (2012). Crustose coralline algae and a cnidarian neuropeptide trigger larval settlement in two coral reef sponges. PloS One 7 (7), e30386. doi: 10.1371/journal.pone.0030386
Wilkens S. L., Stanley J. A., Jeffs A. G. (2012). Induction of settlement in mussel (Perna canaliculus) larvae by vessel noise. Biofouling 28 (3), 301–310. doi: 10.1080/08927014.2011.651717
Williams B., Lamont T. A. C., Chapuis L., Harding H. R., May E. B., Prasetya M. E., et al. (2022). Enhancing automated analysis of marine soundscapes using ecoacoustic indices and machine learning. Ecol. Indic. 140, 108986. doi: 10.1016/J.ECOLIND.2022.108986
Winkler N. S., Pandolfi J. M., Sampayo E. M. (2015). Symbiodinium identity alters the temperature-dependent settlement behaviour of Acropora millepora coral larvae before the onset of symbiosis. Proc. R. Soc. B: Biol. Sci. 282, 20142260. doi: 10.1098/rspb.2014.2260
Woodhead A. J., Hicks C. C., Norström A. V., Williams G. J., Graham N. A. J. (2019). Coral reef ecosystem services in the anthropocene. Funct. Ecol. 33 (9), 1700–1710. doi: 10.1111/1365-2435.13331
Woods Hole Oceanographic Institution (WHOI). (2023). Reef Solutions. Available at: https://reefsolutions.whoi.edu/. (Accessed on 21 February 2023).
Keywords: coral reefs, bioacoustics, phonotaxis, laser doppler vibrometry, restoration, acoustic enrichment, larvae
Citation: Pysanczyn JW, Williams EA, Brodrick E, Robert D, Craggs J, Marhaver KL and Simpson SD (2023) The role of acoustics within the sensory landscape of coral larval settlement. Front. Mar. Sci. 10:1111599. doi: 10.3389/fmars.2023.1111599
Received: 29 November 2022; Accepted: 20 February 2023;
Published: 30 May 2023.
Edited by:
Marta Solé, BarcelonaTech (UPC), SpainReviewed by:
Steve Whalan, Southern Cross University, AustraliaCarly J. Randall, Australian Institute of Marine Science (AIMS), Australia
Copyright © 2023 Pysanczyn, Williams, Brodrick, Robert, Craggs, Marhaver and Simpson. This is an open-access article distributed under the terms of the Creative Commons Attribution License (CC BY). The use, distribution or reproduction in other forums is permitted, provided the original author(s) and the copyright owner(s) are credited and that the original publication in this journal is cited, in accordance with accepted academic practice. No use, distribution or reproduction is permitted which does not comply with these terms.
*Correspondence: Josh W. Pysanczyn, ai5weXNhbmN6eW5AZXhldGVyLmFjLnVr
†ORCID: Kristen L. Marchaver, orcid.org/0000-0002-8953-1902