- 1Chinese Academy of Sciences (CAS) Key Laboratory of Marine Ecology and Environmental Sciences, Institute of Oceanology, Chinese Academy of Sciences, Qingdao, China
- 2Laboratory for Marine Ecology and Environmental Science, Qingdao National Laboratory for Marine Science and Technology, Qingdao, China
- 3CAS Engineering Laboratory for Marine Ranching, Institute of Oceanology, Chinese Academy of Sciences, Qingdao, China
- 4Shandong Province Key Laboratory of Experimental Marine Biology, Qingdao, China
- 5Fishery Resources and Ecology Research Department, Zhejiang Marine Fisheries Research Institute, Zhoushan, China
- 6Horn Point Laboratory, University of Maryland Center for Environmental Science, Cambridge, MD, United States
- 7College of Fisheries, Ocean University of China, Qingdao, China
- 8North China Sea Marine Forecasting Center of State Oceanic Administration, Qingdao, China
- 9Department of Marine Organism Taxonomy & Phylogeny, Institute of Oceanology, Chinese Academy of Sciences, Qingdao, China
- 10Guangxi Institute of Oceanography, Nanning, China
Reef habitat in coastal ecosystems is increasingly being augmented with artificial reefs (ARs) and is simultaneously experiencing increasing hypoxia due to eutrophication and climate change. Relatively little is known about the effects of hypoxia on organisms that use complex habitat arrangements and how the presence of highly preferred AR habitat can affect the exposure of organisms to low dissolved oxygen (DO). We performed two laboratory experiments that used video recording of behavioral movement to explore 1) habitat usage and staying duration of individuals continuously exposed to 3, 5, and 7 mg/L dissolved oxygen (DO) in a complex of multiple preferred and avoided habitat types, and 2) the impact of ARs on exposure to different DO concentrations under a series of two-way replicated choice experiments with or without AR placement on the low-oxygen side. Six common reef-dependent species found in the northeastern sea areas of China were used (i.e., rockfish Sebastes schlegelii and Hexagrammos otakii, filefish Thamnaconus modestus, flatfish Pseudopleuronectes yokohamae, sea cucumber Stichopus japonicus, and crab Charybdis japonica). Results showed that lower DO levels decreased the usage of preferred habitats of the sea cucumber and the habitat-generalist filefish but increased the habitat affinity to preferred habitat types for the two habitat-specific rockfishes. Low DO had no effect on the crab’s habitat usage. In the choice experiment, all three fish species avoided 1 mg/L, and the rockfish S. schlegelii continued to avoid the lower DO when given choices involving pairs of 3, 5, and 7 mg/L, while H. otakii and the flatfish showed less avoidance. The availability of ARs affected exposure to low DO for the habitat-preferring rockfishes but was not significant for the flatfish. This study provides information for assessing the ecological effects and potential for adaptation through behavioral movement for key reef-dependent species under the increasing overlap of ARs and hypoxia anticipated in the future.
Introduction
The frequency and extent of hypoxia, which is typically defined as the dissolved oxygen (DO) concentration of less than 2 mg/L, are rapidly increasing in coastal areas around the world (Diaz and Rosenberg, 2008; Breitburg et al., 2018), and this trend is predicted to continue and likely accelerate into the future under climate change (Weiland et al., 2012; Rabalais and Turner, 2019). Hypoxia in river-influenced coastal areas typically results from eutrophication (increasing nitrogen and phosphorous) generated by point and non-point sources that accompany the human development of watersheds (Caddy, 1993; Steckbauer et al., 2011; Watson, 2016; Domenici et al., 2017). Nutrients stimulate primary and secondary production in surface waters that subsequently sink and decompose, with the associated decline in oxygen, in the bottom layer that is isolated from oxygen inputs due to vertical stratification of the water column (Steckbauer et al., 2011). Hypoxia often occurs during the summer months (warm conditions) when primary production is high from the spring influx of nutrients and vertical stratification has been established. DO increases again in the fall as production decreases and increasing mixing of the water column breaks down or weakens the stratification (Wei et al., 2021b).
Hypoxia causes direct effects on individuals that can translate into localized and population-level responses (Rose et al., 2009). Direct effects from exposure to low DO concentrations result in increased mortality (including massive mortality events), reduced growth, lowered reproduction (Diaz and Rosenberg, 2008), and altered behavior (Wannamaker and Rice, 2000; Vaquer-Sunyer and Duarte, 2008; Rasmuson et al., 2021). Mortality generally occurs with hypoxia, while sublethal effects can occur at DO concentrations up to 4 mg/L (Hrycik et al., 2017).
Hypoxia also causes indirect effects on individuals. Low DO can affect the prey and predators of the species of interest, which then, even without direct exposure, affect growth and mortality (David and David, 2000). Major indirect effects occur from the avoidance movement in response to hypoxia exhibited by many mobile species to reduce their exposure to low DO (Craig, 2012; Lagos et al., 2015; Jorissen and Nugues, 2021; LaBone et al., 2021). Avoidance results in indirect effects because it is not the exposure to DO that causes physiological effects but rather the consequences of individuals being forced to move to less preferred locations and habitats where mortality, growth, and reproduction would be different than if they were able to inhabit the avoided areas (Eby and Crowder, 2002; LaBone et al., 2021).
Reef-dependent species are especially susceptible to hypoxia because they show an affinity for specific habitats that are not uniformly available and, to various degrees, may be reluctant to simply move. Many reef-oriented species therefore exhibit complex ecological responses that emerge from specific differences in behavior and how the spatial arrangement of the reefs allows for connectivity and intersects with the spatially and temporally variable patterns of hypoxia (Rasmuson et al., 2021).
The increasing use of artificial reefs to augment fisheries and the increasing frequency and extent of hypoxia will lead to more situations of overlap between artificial reefs and hypoxia. For example, the summer hypoxic zones in the northern Gulf of Mexico are well-known, and the use of oil and gas platforms that are converted to artificial reefs after they no longer are operational often overlaps with the hypoxic areas (Bianchi et al., 2010). Artificial reef habitats are commonly deployed to restore some of the functioning of degraded habitats in coastal areas (Lemoine et al., 2019). Engineering devices have been proposed with artificial reefs to alleviate local hypoxia, like generating artificial downwelling flow and regionally changing hydrodynamic conditions (Fan et al., 2019).
Laboratory experiments have been extensively used to quantify the effects of low DO on growth, mortality, reproduction, avoidance, and other (e.g., foraging) behaviors (Wu, 2002; Domenici et al., 2007; Portner and Peck, 2010). These experiments have focused on varying DO, sometimes also varying other environmental variables (e.g., temperature, salinity, acidification) in multi-factor designs (Gunderson et al., 2016; Breitburg et al., 2019). What has not been explored in detail is how hypoxia affects the behavior and exposure of individuals in complex habitats and how this differs among reef-dependent species that exhibit highly specific habitat affinities. Habitat assessment based on a field survey of reef-dependent species demonstrated species-specific preferences under normoxic conditions (Yu et al., 2020). Whether hypoxia would cause relatively simple shifts of individuals among reefs that are predictable from species information on preferences and swimming behavior is not known (e.g., generalist good swimming species move to nearby reefs). Such information is difficult to infer from field studies where individuals are affected by multiple factors and hypoxia is often temporally and spatially dynamic.
In this paper, we used a laboratory experiment approach to explore the behavioral responses of reef-dependent fish and invertebrate species to independently manipulated habitat complexity and DO. We performed two laboratory experiments and analyzed the video-recorded movement behavior of representative reef-dependent species in tanks with complex scaled-reduced habitats under different DO concentrations. AR habitat was included as part of the complex habitat and was a factor in the experiments. Behavioral responses to habitat usage, staying duration, and the influence of AR were quantified to assess how species differences in affinities for habitat types and avoidance behavior interact with low DO to affect their exposure. Specifically, Experiment I examined how DO concentration affected the use of preferred habitat types. Experiment II explored how the presence of AR habitat affected avoidance and thereby exposure to low DO. We discuss the implications of the results on the ability of the species to adapt via movement behavior to conditions when habitat use overlaps with hypoxia.
Materials and methods
Experiments were conducted between 24 September and 30 October 2020, at the laboratory of the Tianyuan aquaculture company, Shandong, China. Our study adheres to the policies relating to animal experiments and welfare (e.g., EU Directive 2010/63/EU for animal experiments; ASAB/ABS guidelines), and all experiments are approved by the Institutional Animal Care and Use Committee of Ocean University of China.
Animal selection and description
Yu et al. (2022) grouped the epibenthic reef-dependent species found in a reef area located in Bohai, China, into six archetypes based on their shared responses to the environmental variables of temperature, DO, substrate type, and distance to the nearest reef. The six archetypes simplified the spatial distribution responses of multiple species to environmental conditions and ARs and offered representative reef-dependent species as indicators of community response. We used the member species in the three reef-associated archetypes to select six species (juvenile-stage) to conduct our experiments: rockfish Sebastes schlegelii and Hexagrammos otakii and crab Charybdis japonica from the dominant reef-dependent species archetype; sea cucumber Stichopus japonicus from the reef-neighbor archetype; and filefish Thamnaconus modestus and flatfish Pseudopleuronectes yokohamae from the DO-independent reef-neighbor archetype. The average length and weight of individuals of the test species were typical for their juvenile life stages (Supplementary Table S1). Five of the six species (not P. yokohamae) that represented all three reef-associated archetypes were used in Experiment I. Two species (S. schlegelii and H. otakii) from the reef-dependent species archetype and P. yokohamae from the DO-independent archetype were used in Experiment II.
Except for the crab C. japonica that was purchased from Penglai Fisheries Wholesale Market (Shandong, China), the other species were obtained from organisms bred in the facilities of the Tianyuan Aquaculture Company. All species were kept separate for 7 days. Fish and sea cucumber were fed a commercial diet (Shandong Oriental Ocean Sci-Tech Co. Ltd., Yantai, China) and the crab was fed fresh shellfish twice daily. Feeding of the experimental organisms was stopped 48 h before the experiments began. Experimental seawater was extracted from a coastal underground source and filtered by sand filtration for the experiments. The water quality of the experimental water was nearly identical to the water quality of their culturing water (pH 7.88 ± 0.1; temperature 18.61 ± 0.44°C; DO 7.73 ± 0.2 mg/L; salinity 31.13 ± 0.17).
Experimental set-up and procedure
Different experimental tanks were utilized for two experiments (Figure 1). Details of the experiments are provided in Supplementary—Section 1.2.1. We designed a 120 × 120 × 50 cm regular hexagon uncovered apparatus as the experimental tank for Experiment I (Figure 1A). The tank was constructed from polymethyl methacrylate (PMMA) and divided into six triangle sections using a transparent PMMA bottom clapboard (2.5 cm in height and 0.5 cm in width). We placed five artificially-simulated habitats (i.e., artificial reef [AR], boulder, rubble, gravel, and sand) in sections of the tank and left one section empty (blank).
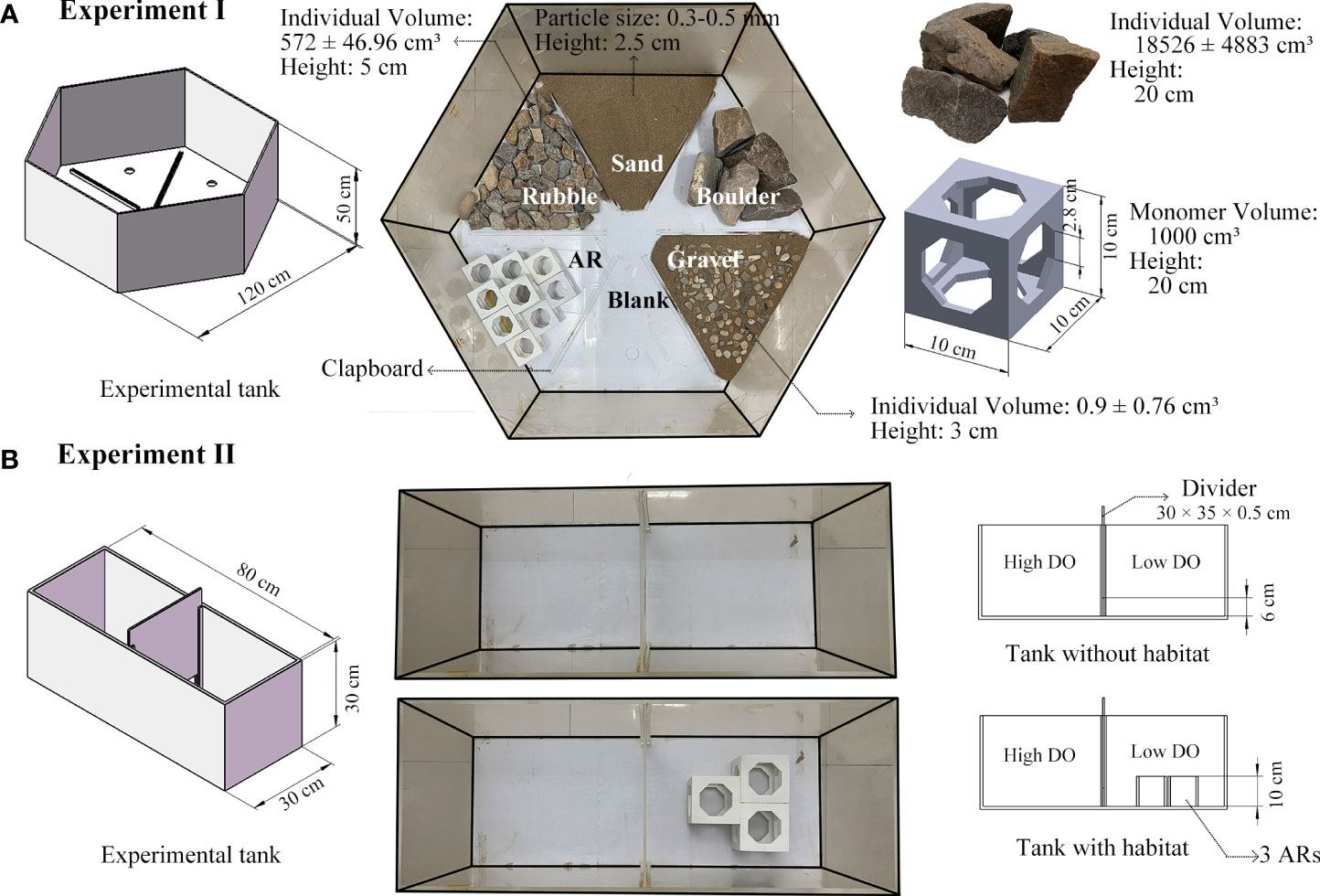
Figure 1 Design of the tanks used in the laboratory experiments. (A), regular hexagon apparatus of Experiment I with artificially-configured versions of the five habitats and one blank area; (B), rectangle channel apparatus of Experiment II with and without artificial reefs placed in the low-oxygen side.
The experimental tank for Experiment II was an 80 × 30 × 30 cm PMMA uncovered rectangular chamber with a 0.5 cm-thick PMMA plate inserted in the middle to establish two sections that were maintained at different DO levels (Figure 1B). We set the height off the bottom of the liftable bottom plate to 6 cm to ensure all species could easily swim back and forth between the sections. In addition, three AR monomers, identical to Experiment I, were selected to be place horizontally in the lower oxygen section to enable detection of any interference of AR on the hypoxia-avoidance capacity of the species.
Lighting was provided by a fixed incandescent light bulb (100 lx, 2 m in height), and a Huawei XiaoTun Camera (1080P, 1.8 m in height) mounted above each experimental tank was used to record the locations of everyone within the treatment sections of the tanks. Water depth was maintained at 30 cm, and nitrogen and air were bubbled to the bottom of the tank to control the target DO concentration of ±0.4 mg/L (Wannamaker and Rice, 2000). DO concentration in the tanks was monitored by Taiwan Hengxin AZ-8403 dissolved oxygen meters at the start and end of each of the experiments. DO readings were taken in each section (habitat area) in Experiment I and along the side at 5 cm from the bottom and water surface in Experiment II.
Experiment I—Habitat usage
Experiment I (left side of Figure 2) was conducted under three DO levels (7, 5, and 3 mg/L) and repeated eight times (replicates) for sea cucumber S. japonicus and crab C. japonica and four times for each of the three fish species (Table 1). Thirty individuals were used in each trial (defined as a specific DO treatment in a replicate), except for 20 individuals in each trial used for H. otakii. Once the DO concentration reached the target level, 20 or 30 individuals were released into the central area of the tanks. Organisms were allowed to acclimatize for 30 min, and then video recording went for 1 to 12 h, depending on the species. A new central area within a 15 cm radius of the center of the apparatus was added for sea cucumber and crab to accommodate their slower-swimming behavior compared to fish. Details of the difference in the number of species and experimental design are provided in Supplementary—Section 1.2.2.
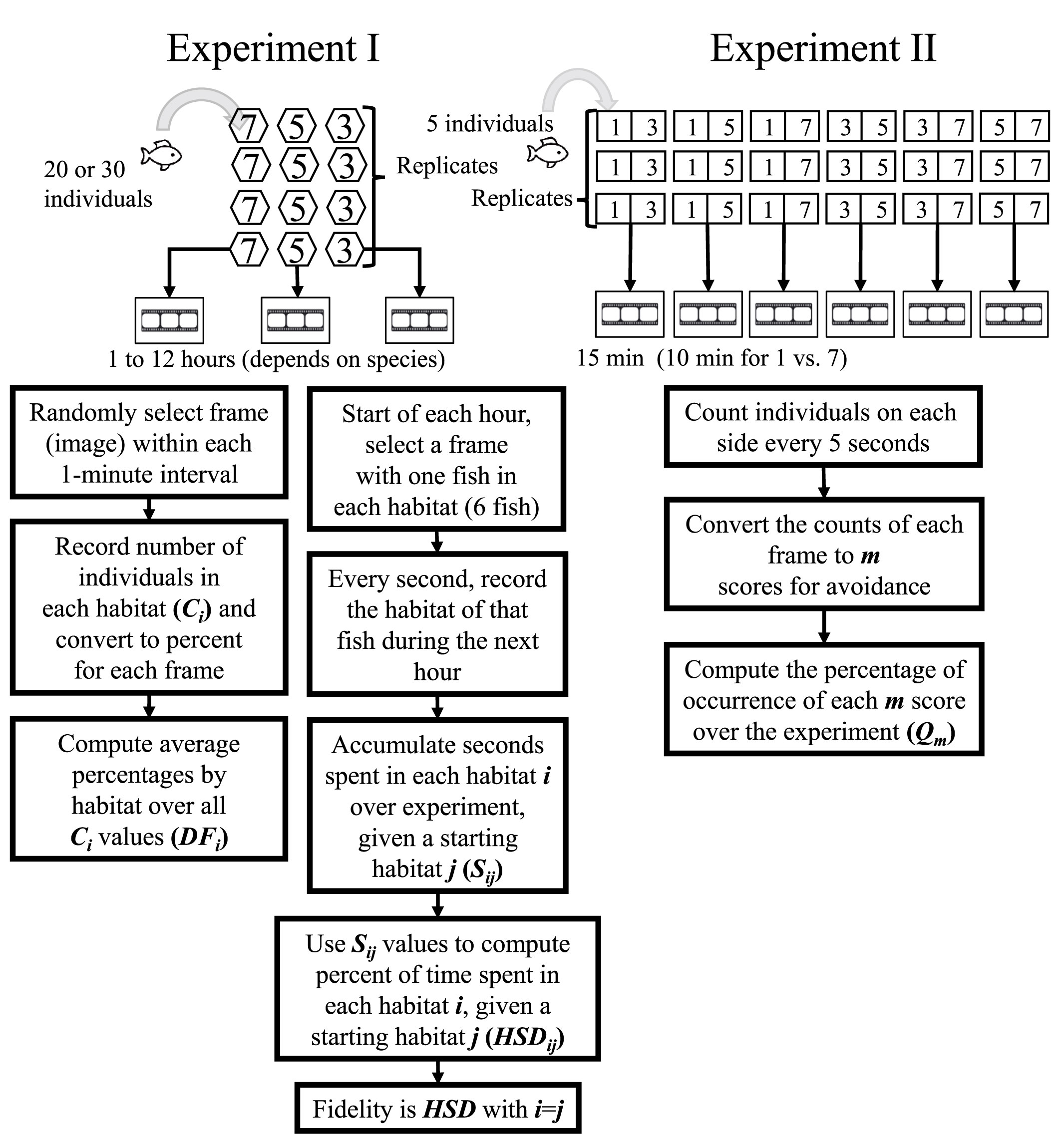
Figure 2 Flow chart of the design and analysis for Experiment I (left side) and Experiment II (right side).
A parallel set of control experiments was designed and implemented to assess the spatial preferences of each species for areas within the experimental tank. All habitats were removed from the tank, and the oxygen level was set at the normoxia (7 mg/L) condition (Table 1). The five species demonstrated no significant differences in sections when all were blank habitats (Supplementary Tables S2, S3).
Experiment II—Hypoxic avoidance
In Experiment II (right side of Figure 2), we set up various pair-wise combinations of different DO concentrations between the two sides of the rectangle chamber (1 vs. 3, 1 vs. 5, 1 vs. 7, 3 vs. 5, 3 vs. 7, and 5 vs. 7 mg/L, Table 2). Experiment II was divided into two parts: one part did not include AR habitat and was used to measure the hypoxia-avoidance capacity of each species when only DO vary, and the second part then included placing three AR monomers on the lower-oxygen side to explore how having a preferred habitat type (AR) affected the hypoxia-avoidance capacity (Table 2). Both parts (with and without ARs) were replicated six times. Individuals were held for 30 minutes in the same-design apparatus with normoxic conditions on both sides before their introduction to the testing tank.
Once the target DO concentrations were reached on each side of the tank and the 30-minute acclimatization was completed, the central divider was lifted to create the bottom channel for passage. The five acclimatized fish were then gently introduced into the bottom channel with a small diddle net. Recording started 5 min after fish introduction, and recording was for 15 min (10 min for the 1 vs. 7 mg/L DO combination). The relatively short duration ensured that starting and ending DO concentrations varied less than 0.4 mg/L from the target DO levels.
Behavior observations and parameter calculations
For both experiments and each species, we analyzed the video recording of each trial and accumulated the results over replicates. The recordings had 24 frames (images) every second and we sampled images from those frames for analyses.
Experiment I—Habitat usage
Two metrics were calculated for the experiment with habitat types present and the control experiment with empty sections. For convenience, we refer to the sections in the control experiment by the habitat types of the version of the experiment that used habitat. This is even though no habitat was present.
The first metric was the percent of individuals by habitat type, denoted DFi, i = 1, 2, 3, 4, 5, 6. This refers to AR, boulder, rubble, gravel, sand, and blank. First, we randomly selected an image (frame) from each 1-minute interval. The number of individuals in each habitat (section) was counted (Ci) for each selected frame and converted to a percentage based on the total number of individuals present. The number of C values for a species in a specific treatment was therefore the duration of the treatment (minutes) × number of replicates. Distribution frequency (DFi, expressed as a percent) was then computed for each of the six habitat types in a treatment as the average of the Ci values for that habitat type (Figure S1).
The second metric of habitat staying duration (HSDij) was calculated from the same recordings as the first metric and for the fish species only. HSD reflected the movement trajectory of individuals among the habitats. At the start of an hour, we selected a frame that had at least one individual in each of the six sections and treated that as their starting habitat. j = 1, 2, 3, 4, 5, 6 refers to the starting habitat as AR, boulder, rubble, gravel, sand, and blank. We then followed that individual in frames every second and recorded what habitat it was in for the rest of that hour. This was then repeated (one individual in each of the six starting habitats) for every hour of the experiment. The cumulative seconds spent by each individual over replicates (Sij) was determined. The number of S values for a species that individuals starting in each habitat spent in each of the six habitats was the duration of the one-hour treatment (12 h) × four replicates or 48 values, and Sij values were then used to calculate the percent of total seconds (HSDij, Figure S2). Habitat fidelity was the special case of the habitat staying duration computed for when the starting habitat used the cumulative seconds spent in that same habitat (i.e., HSDii).
Experiment II—Hypoxic avoidance
A metric that categorized the degree of avoidance for the lower DO choice (Qm) was used in the experiment with AR present and the control version that had no ARs. The number of individuals on each side of the tank was recorded from a frame selected every 5 s and categorized into the behavior codes (denoted m) that used how the five individuals were split between the sections (Table 3). Thus, there were 15 min × 12 images per minute × six replicates, or 1,080 values of m for each species in each treatment. These values of m were then converted to the percent of the values (Qm) accounted for by each of the six possible values of m (Figure S3). For statistical analysis and labeling of figures, we then further categorized the six behavior codes into three hypoxia-avoidance capacity categories: strong (m = 1, 2), weak (m = 3), and no capacity (m = 4, 5, 6).
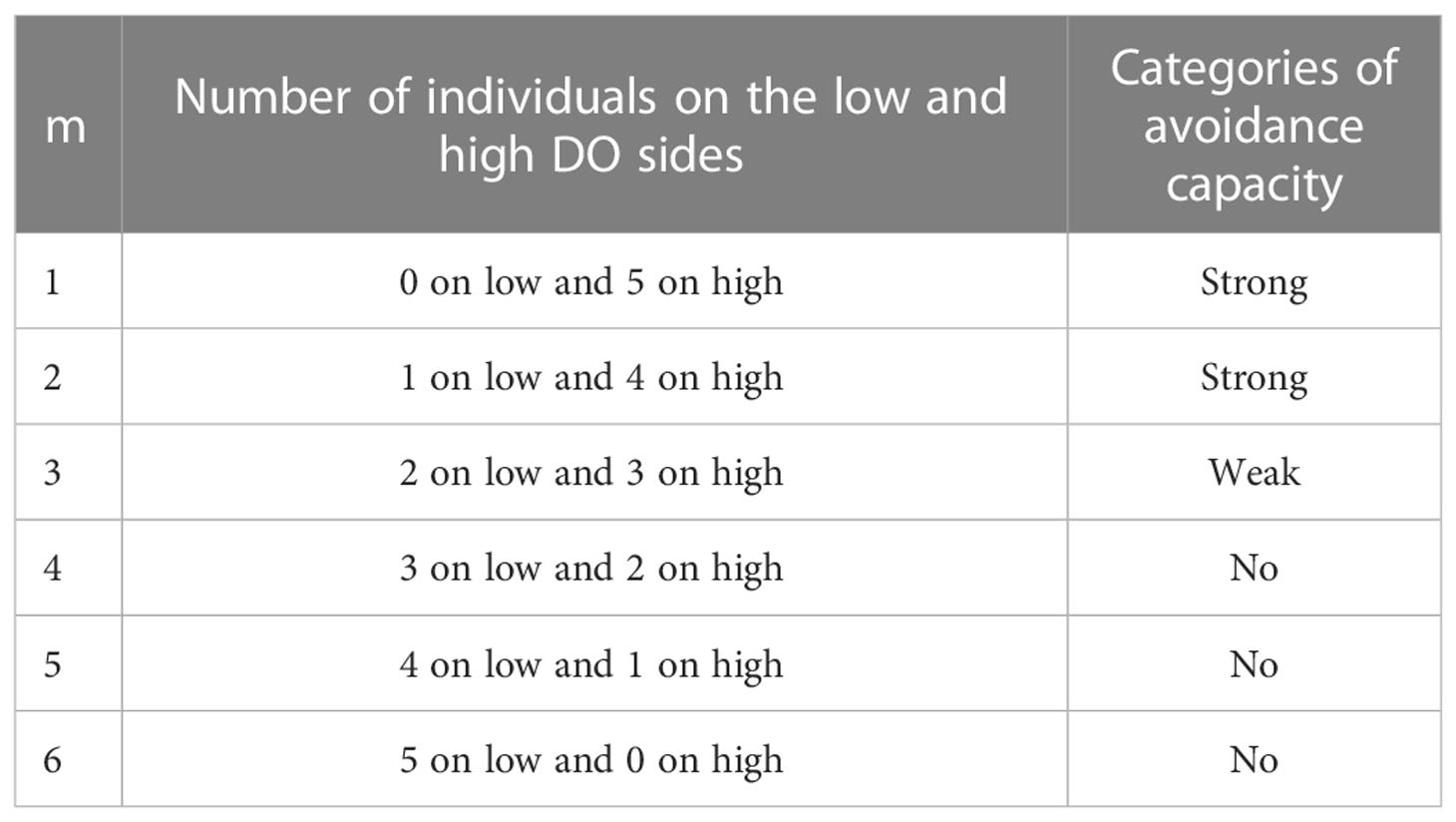
Table 3 Use of the number of individuals on each side of the tank in each frame to assign a degree of avoidance category (m) to the frame and categories of avoidance capacity.
Statistical analysis
The behavior metrics of DFi and HSDii (fidelity only, I = j) from Experiment I and the 3-category version of degree of avoidance from Experiment II were analyzed using the Kruskal–Wallis test (α = 0.05) followed by a Nemenyi post-hoc pairwise comparison (Table 4). For Experiment I, the tests were to assess the effect of the three DO concentrations (on usage DFi and fidelity HSDii) for each habitat type and were done separately for the experiment with three DO levels and for the control for each species. Also, we tested DFi across habitats given the same DO levels for the experiment with three DO levels and for the control for each species (see Supplementary Tables S2–S8). For Experiment II, the tests were to compare the “strong,” “weak,” and “no” avoidance capacity categories for each pair-wise combination of lower and higher DO and were done separately for when ARs are present and for when they are absent (control) for each species. Significant differences were reported using letters on the figures to denote differences (a, b, c; A, B, C for the control results of Experiment II). All analyses were conducted using the R program (v. 4.1.0) with the “PMCMRplus,” “tidycerse,” and “circlize” packages.
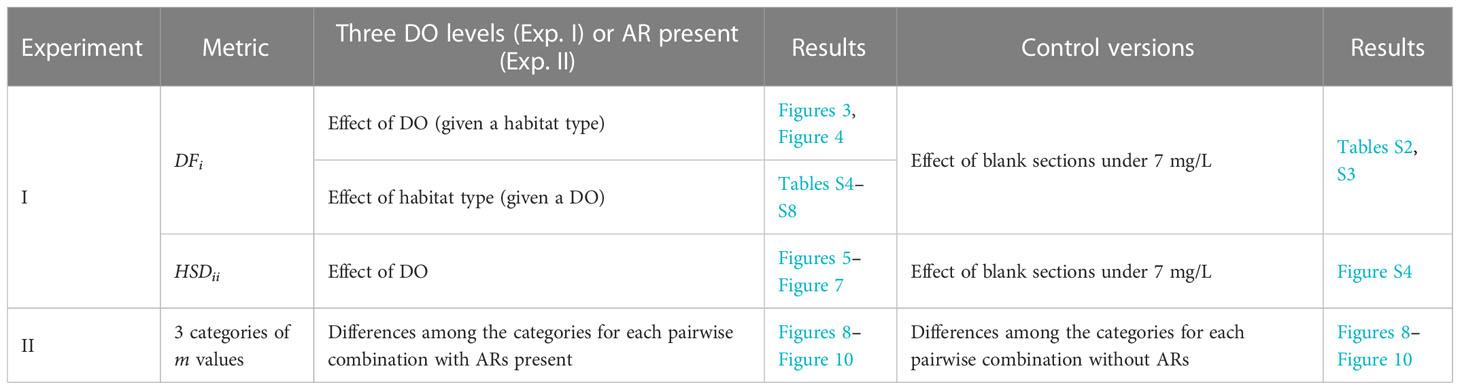
Table 4 Testing metrics from Experiments I and II using the Kruskal–Wallis test followed by Nemenyi pairwise comparison.
Results
Preferred habitats under normoxia and no ARs
Frequency percentages from Experiment I under the normoxic condition (7 mg/L DO) indicated the preferred habitats and movement characteristics for each species. Four species (i.e., sea cucumber S. japonicus, crab C. japonica, and two rockfishes H. otakii and S. schlegelii) similarly preferred boulder, AR, and rubble habitats, while the filefish T. modestus had a strong preference for the blank area followed by AR (black bars in Figures 3, 4). More detailed results of habitat preferences are documented in Supplementary—Section 2.1. In the control of Experiment I (no ARs and other habitats), rockfish H. otakii indicated the highest dependence on its starting position, followed by S. schlegelii, while T. modestus showed relatively equal constant staying durations among all habitats (Supplementary—Section 2.2 and Figure S4).
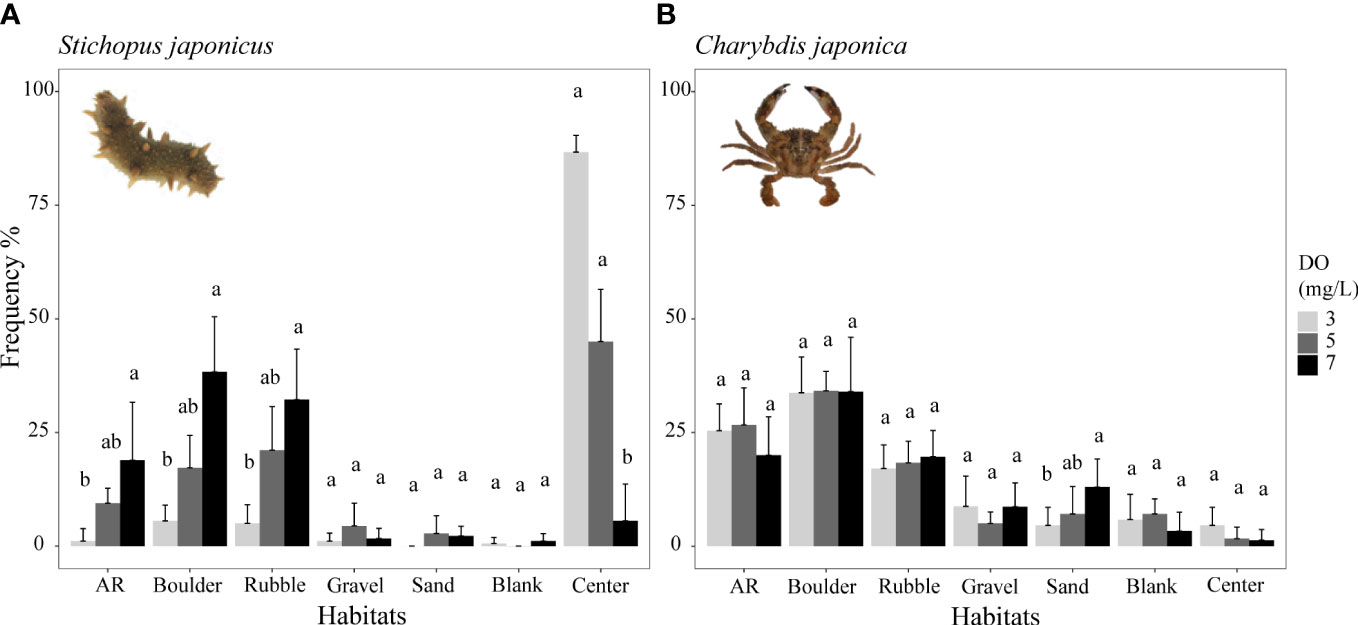
Figure 3 Mean distribution frequency percentage of sea cucumber Stichopus japonicus from reef-neighbor archetype (A) and crab Charybdis japonica from dominant reef-dependent archetype (B) in each habitat under three DO levels of Experiment I. Data are expressed as the mean ± SEs and analyzed by the Kruskal–Wallis test, followed by a post-hoc multiple comparison test between three DO levels in the same habitat (α = 0.05). Significant differences are indicated by different letters.
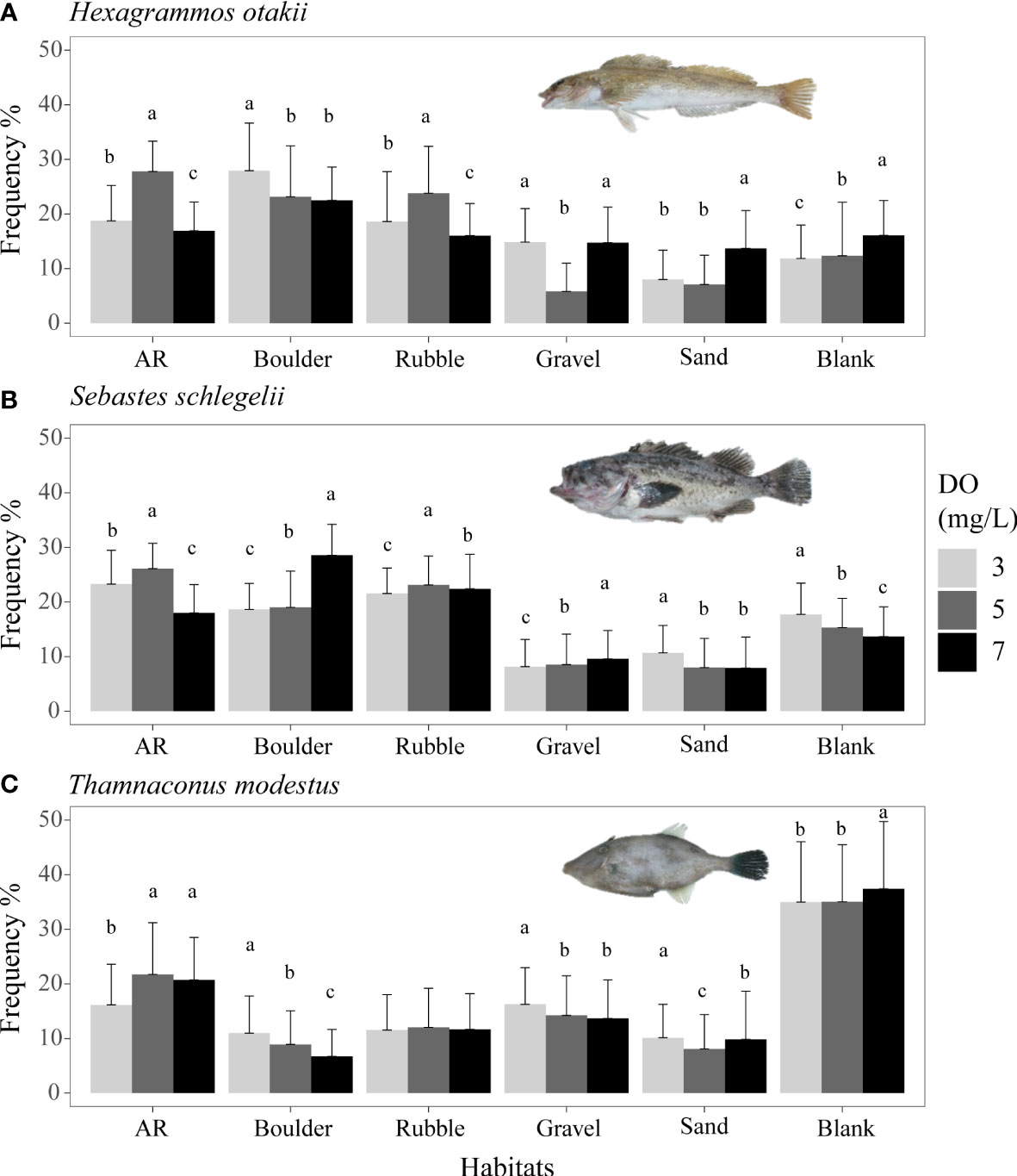
Figure 4 Mean distribution frequency of rockfish Hexagrammos otakii (A) and Sebastes schlegelii (B) both from dominant reef-dependent archetype, and filefish Thamnaconus modestus from DO-independent reef-neighbor archetype (C) for each habitat under three DO concentrations of Experiment I. These results are from Experiment I. Data were analyzed by the Kruskal–Wallis test, followed by a post-hoc multiple comparison test comparing the three DO levels in each habitat (α = 0.05). Significant differences are indicated by different letters.
Low DO effects on habitat usage
Distribution frequency
Overall, lower DO concentrations decreased the use of preferred habitats (AR, boulder, and rubble areas) by the sea cucumber S. japonicus (Figure 3A) and the preferred habitats (blank and AR areas) of the filefish T. modestus (Figure 4C). In contrast, lower DO increased the dependence on complex habitats (AR, boulders, rubble) of the two rockfish species S. schlegelii and H. otakii (Figures 4A, B). DO concentration had no significant influence on how the crab C. japonica used its preferred habitats (Figure 3B).
Examining the results in more detail, the distribution frequency of sea cucumber decreased in the AR, boulder, and rubble habitats with decreasing DO concentration (Figure 3A). The highest percent frequencies were observed under the normoxic condition (7 mg/L), while the lowest frequencies occurred under the lowest (3 mg/L) DO concentration. Percent frequencies for 7 mg/L and 3 mg/L were: 38 versus 5.5% in boulders, 32 versus 5% in rubble, and 19 versus 1.1% in ARs. These were reduced below 5 mg/L, and especially below 3 mg/L. Most individuals congregated in the center of the tank under low oxygen conditions, which resulted in no differences in distribution frequency related to DO for the less preferred habitats (p >0.05, Supplementary Table S4).
Crabs showed the same habitat preferences as the sea cucumber, but crab usage of habitat types was not significantly affected by low DO conditions (Figure 3B; Supplementary Table S5). Lowered DO concentrations did not significantly influence the distribution frequencies of their preferred usage levels (p >0.05, Figure 3B), with the exception of sand.
Both rockfish species (Figures 4A, B) showed the highest use of ARs and rubble at 5 mg/L, with use being lower for 3 and 7 mg/L (p <0.05, Supplementary Tables S6, S7). This resulted in a dome shape for the bars in Figures 4A, B. However, for the remaining habitats, including the preferred habitat of boulders, the two rockfish species showed different or even opposite responses. Use of boulder increased from 22 to 28% with decreasing DO (from 7 to 3 mg/L) for H. otakii, while the use of boulder decreased from 29 to 20% with S. schlegelii. Among the less preferred habitats, a mix of patterns of dome-shaped, no differences, and increasing or decreasing usage was observed.
The final species, T. modestus, showed a habitat usage response to low oxygen that differed from the other species (Figure 4C). T. modestus showed the weakest preferences for the habitat types, with the blank option getting the most use for all DO concentrations. Habitat use of blank was similar (although p <0.05) at about 35% for 7 and 3 mg/L (Supplementary Table S8). While use was generally low for other habitats, decreasing DO (7 mg/L versus 3 mg/L) caused a decrease in usage of ARs (from 21 to 16%) and an increased use of boulders (from 6.7 to 11%). Other habitats showed little dependence on DO concentration.
Staying duration
In general, low DO concentrations affected the staying duration of habitats for the two rockfish species that showed a high degree of preference for specific habitats. This was compared to a small DO effect observed for the generalist filefish T. modestus.
The staying duration in the preferred habitats of both rockfish species was increased by lower DO concentrations, but with some differences. Both species showed the same increase in staying duration with low DO in a preferred habitat. Staying duration from 7 to 3 mg/L increased in AR (77 to 88%) and boulder for H. otakii (p <0.05, Figures 5A, B). Similarly, staying duration increased moderately in AR and in rubble for S. schlegelii (Figure 6C). However, duration in rubble showed no differences for H. otakii (Figure 5C), and S. schlegelii showed reduced duration in boulders (Figure 6B).
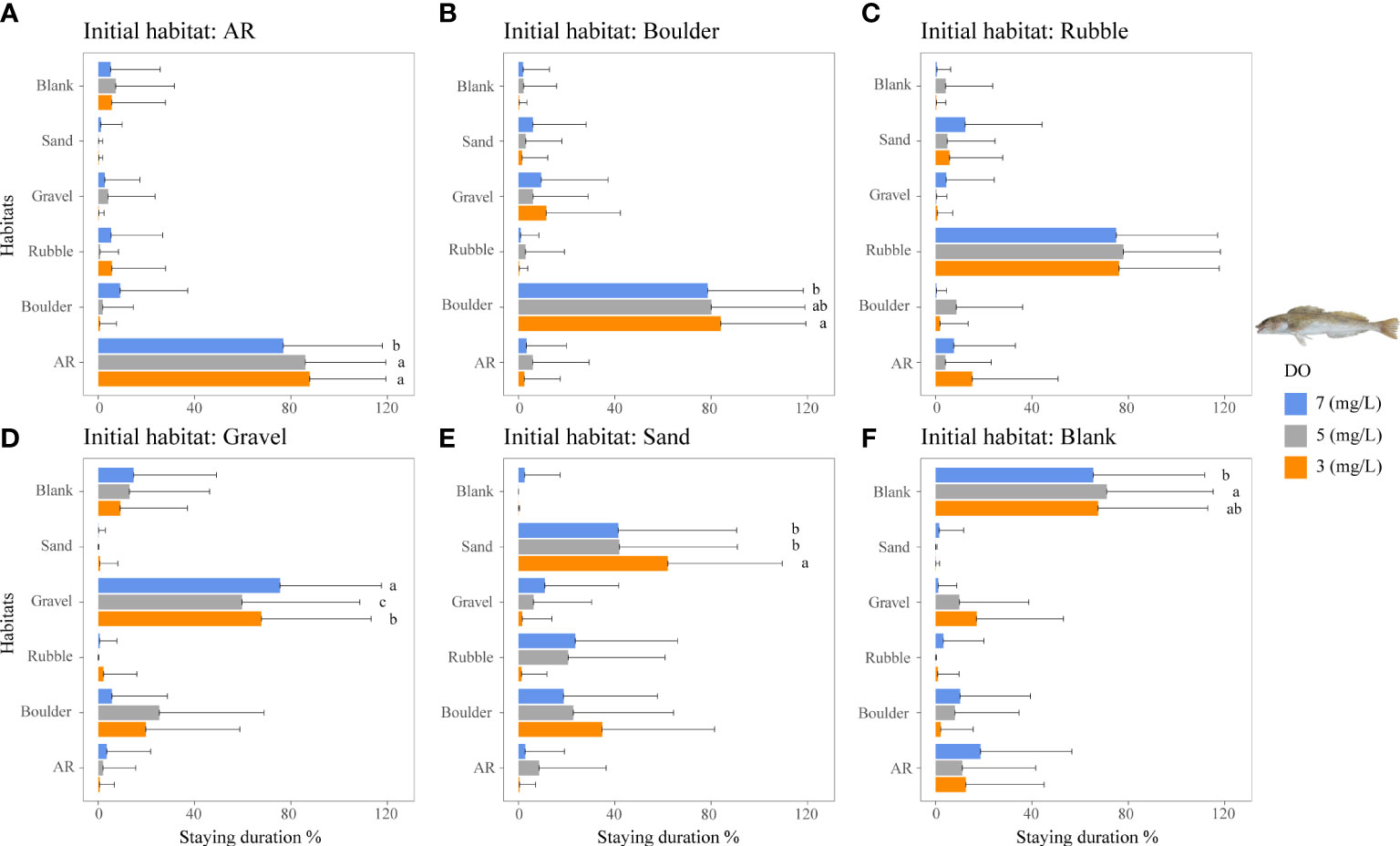
Figure 5 Mean staying duration percentage of the rockfish H. otakii from dominant reef-dependent archetype under the three DO concentrations of Experiment I. Data for the special case of staying duration that used the time spent in the same habitat type as the individual was started from (i.e., fidelity) were analyzed by the Kruskal–Wallis test, followed by a post-hoc multiple comparison test comparing the three DO levels (α = 0.05). Significant differences are indicated by different letters. (A–F) Initial starting habitat is from AR, boulder, rubble, gravel, sand, and blank, respectively.
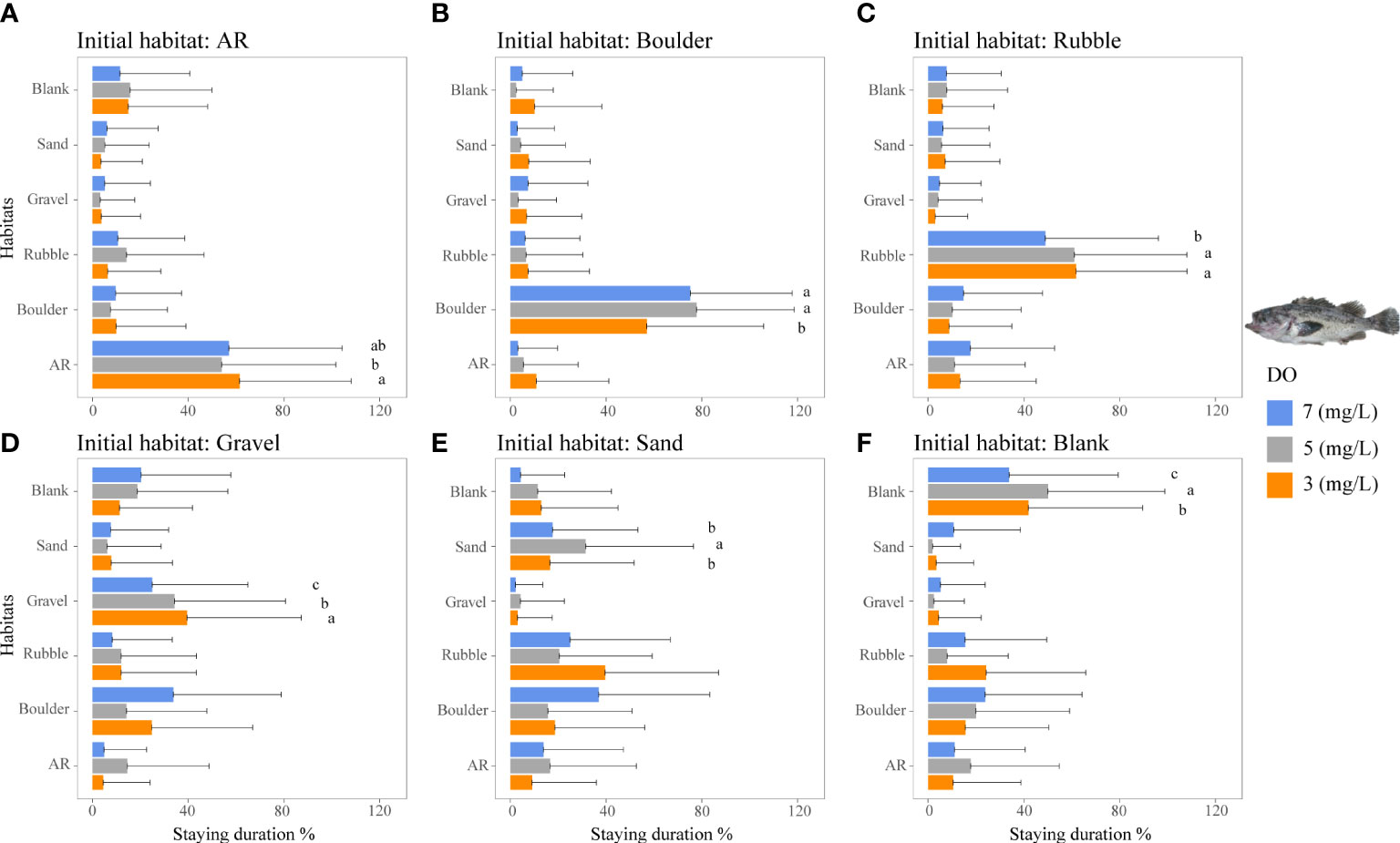
Figure 6 Mean staying duration percentage of the rockfish S. schlegelii from dominant reef-dependent archetype under the three DO concentrations of Experiment I. Data for the special case of staying duration that used the time spent in the same habitat type as the individual was started from (i.e., fidelity) were analyzed by the Kruskal–Wallis test, followed by a post-hoc multiple comparison test comparing the three DO levels (α = 0.05). Significant differences are indicated by different letters. (A–F) Initial starting habitat is from AR, boulder, rubble, gravel, sand, and blank, respectively.
For the filefish T. modestus, lower DO concentrations showed only slight effects on staying duration, with the highest staying duration in the blank area under all DO conditions, followed by the second-most usage in its other preferred habitat of AR (Figure 7). The staying duration in the AR area was higher under 3 mg/L (34%) than under 5 and 7 mg/L DO (20% and 28%, p <0.05, Figure 7A).
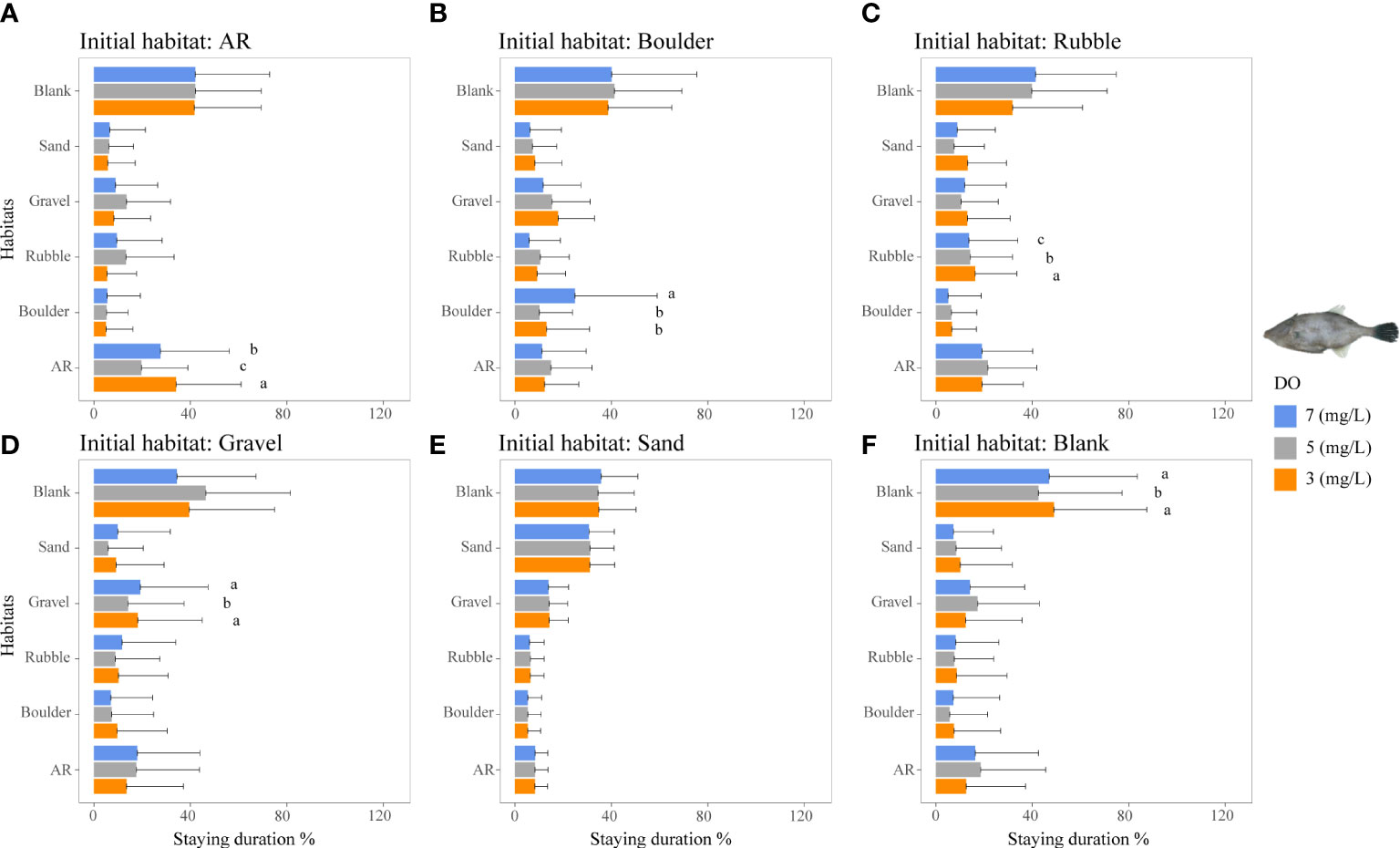
Figure 7 Mean staying duration percentage of the filefish T. modestus from DO-independent reef-neighbor archetype for the three DO concentrations in Experiment I. Data for the special case of staying duration that used the time spent in the same habitat type as the individual was started from (i.e., fidelity) were analyzed by the Kruskal–Wallis test, followed by a post-hoc multiple comparison test comparing the three DO levels (α = 0.05). Significant differences are indicated by different letters. (A–F) Initial starting habitat is from AR, boulder, rubble, gravel, sand, and blank, respectively.
Exposure to low DO concentrations
Avoidance without ARs
All three species avoided 1 mg/L, as shown by the gray bars (indicating no AR present) being to the left in the three top panels (i.e., all panels that included 1 mg/L in Figures 8–10). When given choices involving pairs of 3, 5, and 7 mg/L, the rockfish S. schlegelii continued to avoid the lower DO (gray bars remain to the left in Figure 9), while H. otakii and the flatfish P. yokohamae showed less avoidance (gray bars shifted to the right in the three bottom panels of Figures 8, 10). S. schlegelii showed a significantly strong capacity to avoid low oxygen in all DO combinations (p <0.05), with the frequency of the strong-capacity groups (behavior groups m = 1 and 2) being 79% to 100% (Figure 9). H. otakii showed a significantly higher frequency of strong avoidance (m = 1 and 2) in certain combinations: 78% (1 versus 3 mg/L), 56% (1 versus 5), and 83% (1 versus 7) (Figure 8), but showed no significant difference in avoidance between 3 versus 5, 3 versus 7, and 5 versus 7 mg/L DO combinations. This is seen as the gray bars being centered and spread out over the behavior groups in the lower three panels of Figure 8. The flatfish P. yokohamae also demonstrated avoidance like H. otakii: strong capacity to avoid 1 mg/L but “weak” or “no” capacity to avoid low oxygen in the trials including 3, 5, and 7 mg/L. As with H. otakii, the avoidance of P. yokohamae also showed gray bars to the left for the top 3 panels and centered and spread out for the lower three panels (Figure 10).
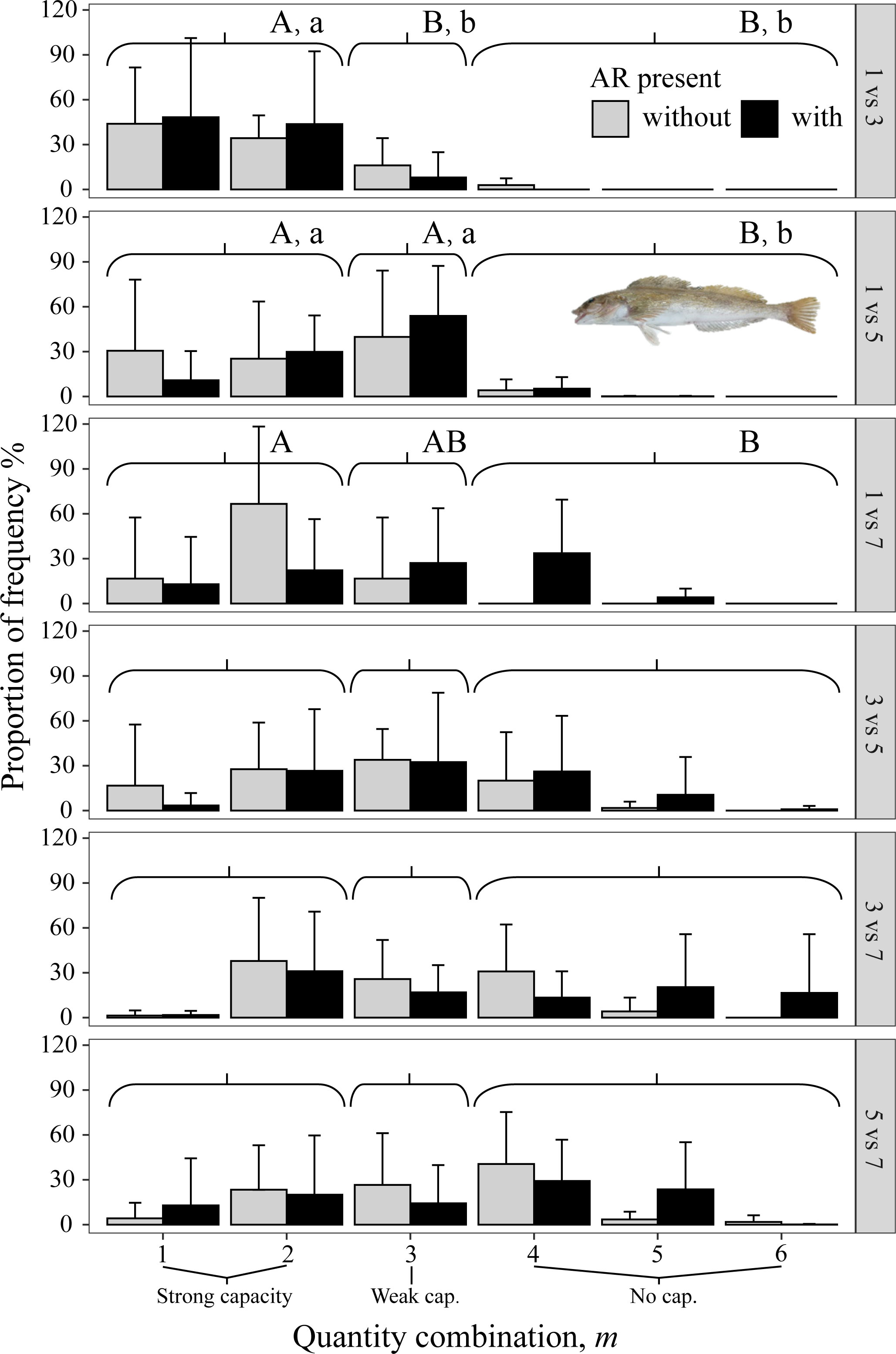
Figure 8 Mean percent of each of the six possible values for m that codes the degree of avoidance of the lower DO side for rockfish H. otakii from dominant reef-dependent archetype in Experiment II, with ARs present in the lower DO side (black bars) and without ARs (gray bars). Each panel shows the results for each pairwise combination of low DO in one side and higher DO on the other side of the tank. Data were analyzed by the Kruskal–Wallis test, followed by a post-hoc multiple comparison test comparing the three categories of avoidance capacity: strong (m = 1 and 2), weak (m = 3), and none (m = 4, 5, and 6) across DO levels for each pairwise DO combination (α = 0.05). Significant differences are indicated by different letters (lower case for ARs present and upper case for control).
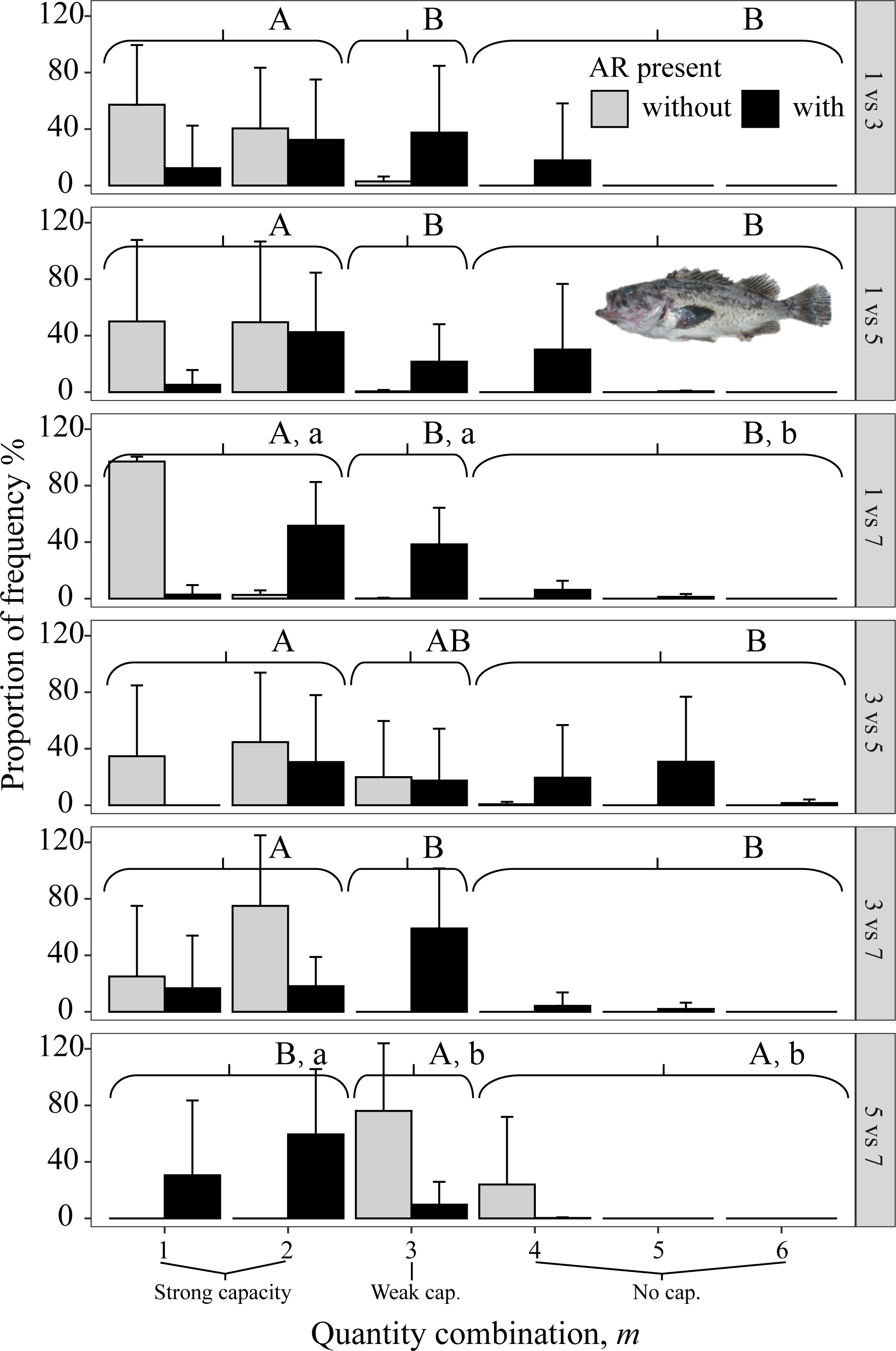
Figure 9 Mean percent of each of the six possible values for m that codes the degree of avoidance of the lower DO side for rockfish S. schlegelii from dominant reef-dependent archetype in Experiment II, with ARs present in the lower DO side (black bars) and without ARs (gray bars). Each panel shows the results for each pairwise combination of low DO in one side and higher DO on the other side of the tank. Data were analyzed by the Kruskal–Wallis test, followed by a post-hoc multiple comparison test comparing the three categories of avoidance capacity: strong (m = 1 and 2), weak (m = 3), and none (m = 4, 5, and 6) across DO levels for each pairwise DO combination (α = 0.05). Significant differences are indicated by different letters (lower case for ARs present and upper case for control).
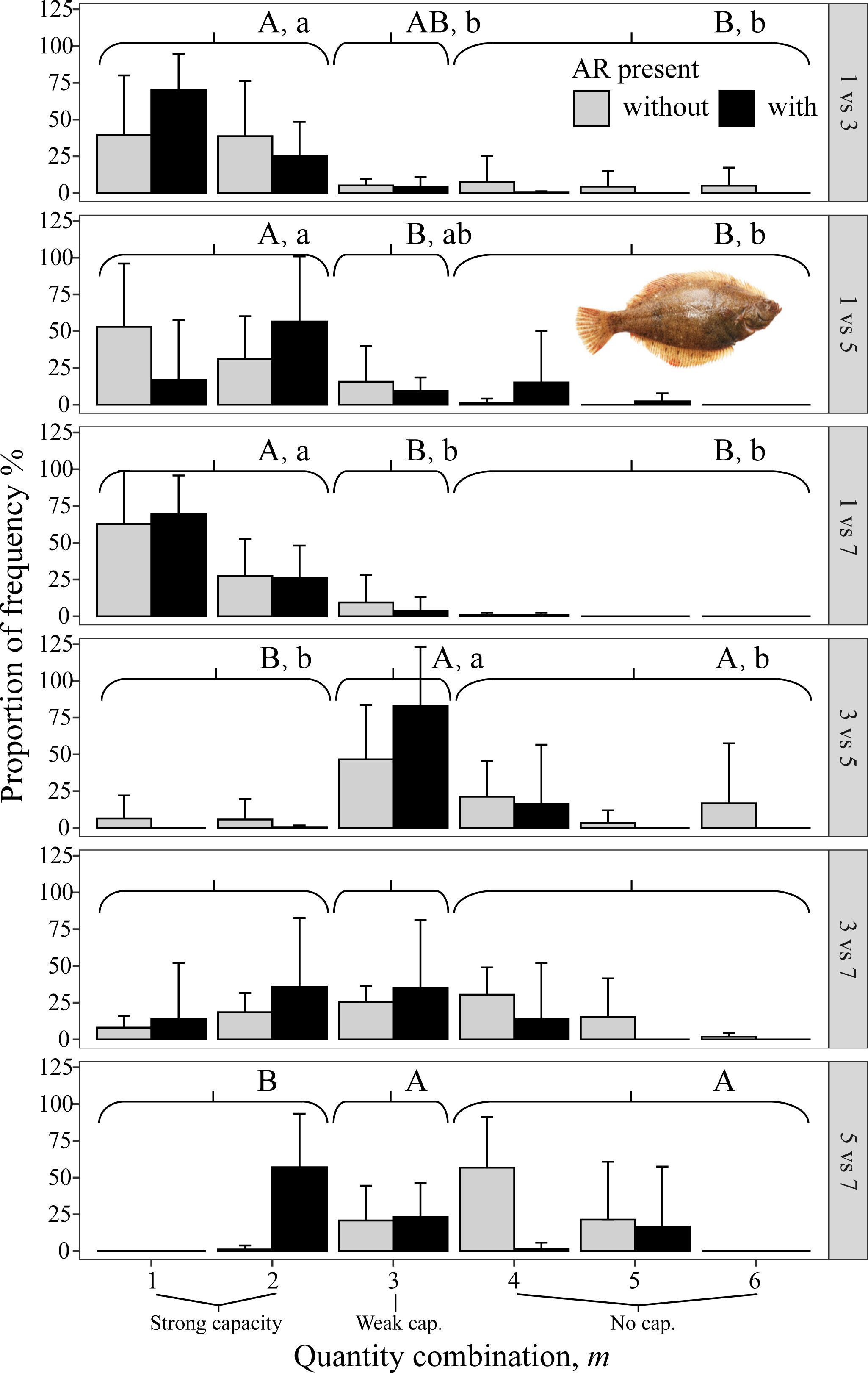
Figure 10 Mean percent of each of the six possible values for m that codes the degree of avoidance of the lower DO side for the flatfish P. yokohamae from DO-independent reef-neighbor archetype in Experiment II, with ARs present in the lower DO side (black bars) and without ARs (gray bars). Each panel shows the results for each pairwise combination of low DO in one side and higher DO on the other side of the tank. Data were analyzed by the Kruskal–Wallis test, followed by a post-hoc multiple comparison test comparing the three categories of avoidance capacity: strong (m = 1 and 2), weak (m = 3), and none (m = 4, 5, and 6) across DO levels for each pairwise DO combination (α = 0.05). Significant differences are indicated by different letters (lower case for ARs present and upper case for control).
Avoidance with ARs
Overall, the availability of ARs affected avoidance, and therefore exposure to low DO concentrations, for the habitat-preferring rockfishes but was not a significant factor affecting exposure for the flatfish.
The presence of ARs caused H. otakii and S. schlegelii to reduce their avoidance capacity. However, H. otakii continued avoidance in the presence of ARs at 1 versus 3 mg/L and 1 versus 5 mg/L, but increased its exposure when the lowest DO was 3 or 5 (black bars in Figure 8). The decrease in avoidance at 1 versus 7 is inconsistent with the general pattern of H. otakii continuing to avoid very low DO.
S. schlegelii increased its exposure to low DO with the presence of ARs at all levels of DO, even when the lowest DO was 1 mg/L (black bars to the right of gray bars in all panels, Figure 9). There was also an exception with S. schlegelii with ARs causing increased avoidance capacity at 5 versus 7. In this case, as opposed to the exception with 1 versus 7 for H. otakii, the consequences of higher exposure to lower DO concentrations are small because 5 mg/L is still high enough for minimal physiological effects. Finally, the avoidance of the flatfish P. yokohamae was unaffected by the presence of ARs (gray and black bars showed the same pattern from left to right in each panel, Figure 10).
Discussion
Effects of low DO on fish have focused on growth, mortality, reproduction, and behavioral responses of individuals in laboratory experiments (Shimps et al., 2005; Portner and Peck, 2010; Wang et al., 2016; French and Wahl, 2018). Some exploration of interactions between DO and temperature (Roman et al., 2019) using a variety of endpoints such as growth and mortality (McNeill and Perry, 2006; McBryan et al., 2013; Marcek et al., 2020). There are a few examples of how habitat usage and affinity can be altered by low DO and how habitat can influence avoidance of low DO. In the field, both cases involve how habitat and avoidance behavior combine to determine how individuals use their preferred habitats and their exposure to low DO concentrations. Our experiments were designed to directly address movement behavior as the interplay between habitat usage and avoidance of low DO.
Our results showed several important species differences in how reef-dependent species behaviorally respond and use preferred habitats under low DO. In Experiment I, lower DO concentrations decreased the usage of preferred habitats by the sea cucumber (AR, boulders, and rubble areas) and by the filefish (blank and AR areas) but increased the dependence on preferred habitats (AR, boulders, and rubble) for the two rockfish species. Low DO did not affect how the crab species used their habitats. The low oxygen effect was much stronger for the two rockfishes that showed a high degree of preference for specific habitats compared to the relatively small DO effect for the generalist filefish. Experiment II showed that all three species examined avoided hypoxic water (1 mg/L DO). Differences among species arose with choices between moderate (3 and 5 mg/L) and high DO concentrations (7 mg/L). The rockfish S. schlegelii continued to avoid lower DO when given choices involving pairs of 3, 5, and 7 mg/L. In contrast, H. otakii and the flatfish showed weaker avoidance of lower DO concentrations. As with habitat usage in Experiment I, the effects of low DO on avoidance in Experiment II were stronger for the two rockfish species with strong habitat preferences than for the more generalist flatfish.
Effects of hypoxia on habitat usage
Hypoxia had the most significant impact on habitat usage for the sea cucumber, S. japonicus. When the DO was decreased to 3 mg/L, many sea cucumbers lost their moving ability and gathered in the initial introduced position in the tank. This phenomenon is consistent with Zhou et al. (2018), who explored how hypoxia affects the behavior of the sea cucumber, Apostichopus japonicus. At the other extreme, the habitat usage by the crab C. japonica was not influenced by low DO, which may be related to its strong hypoxia tolerance, flexible usage of various habitat types like rock and sand (Yatsuya and Matsumoto, 2021), and its territorial nature (Fowler and McLay, 2013; Yu et al., 2020). Zhu et al. (2022) examined how complex habitats can reduce the territorial behavior of crabs. After the crabs were introduced into the tank, we observed some transitory fighting and competitive behavior for territory in the gravel, sand, and blank habitat sections; some individuals would hide in the crevices of the boulders or bury themselves in the sand. All or some combination of these traits would lead to the crab being unresponsive to low DO.
Fish may exhibit a range of physiological responses as adaptations to hypoxic conditions (Domenici et al., 2017). For example, some species exposed to low DO accelerate oxygen uptake by the gills to facilitate systemic blood transport (Randall, 1982; Richards, 2009). While these adaptive measures provide short-term relief from the effects of hypoxia, if hypoxia persists, fish may decrease their metabolic rate by reducing aerobic activities (Claireaux and Lefrançois, 2007; Pörtner and Farrell, 2008). Studies have demonstrated the limiting effects of hypoxia on fish swimming behavior, such as decreased swim speed and reduced caudal fin swing frequency (Domenici et al., 2017; Messina-Henríquez et al., 2022). This reduction in mobility and physiology (Jones, 1971; Jourdan-Pineau et al., 2010) may have contributed to the increased stay duration of the reef fish species observed in Experiment I. The two rockfish species displayed strong preferences for their preferred habitats. The staying duration of the less mobile H. otakii in AR habitat (highly preferred) increased with lower DO concentrations. Approximately 5 mg/L DO showed the largest use values for ARs, but 3 mg/L DO had the longest staying duration. The persistence to use AR under 3 mg/L could be due to the relatively large H. otakii occupying sufficient spaces to impede other individuals from swimming into the AR section. While low DO also increased the staying duration of the more mobile S. schlegelii, they showed a range of responses in other preferred habitats.
The behavioral responses of fish to hypoxia may include a trade-off between reducing swimming activity to reduce oxygen demand and increasing swimming activity to move to more oxygenated waters (Domenici et al., 2000; Lefrançois et al., 2009; Zhang et al., 2010). How reducing activity to lower metabolism combines with avoidance behavior that involves increasing activity is not well documented and is likely dependent on species and conditions (Diaz and Rosenberg, 1996; Domenici et al., 2007; Herbert et al., 2011). Our experimental results confirmed inter-specific differences in movement responses to hypoxia. In contrast to the rockfish species, the habitat generalist filefish T. modestus was curious about the AR area in the tank. Many filefish individuals first touched the AR with their rostral side, swam around the reef two or three times, and then left that section and entered more-open sections like blank, sand, or gravel habitats. After seemingly traversing all habitat types, they sometimes returned to the AR section and repeated without ever entering the interior structure of the AR. Under the 3 mg/L DO condition, only filefish individuals frequently swam to the water surface for breathing, which is consistent with the behavioral characteristics of fish with strong swimming capacity under hypoxic stress (Domenici et al., 2013); the two rockfishes were rarely observed swimming near the water surface.
Effects of artificial reef on exposure to low oxygen
In large-scale sea areas, hypoxic conditions may change gradually and can be distributed in patches (LaBone et al., 2021). A relatively stationary and stable low-oxygen location allows most organisms, especially fish, to avoid this area. In contrast, an uneven spatial distribution of local hypoxic areas or local areas that vary in intensity over time would result in individuals having to constantly respond to changing DO conditions as they swim. Our study examined the effects of the presence of AR, a preferred habitat for many species, on avoidance when faced with two DO conditions. This implies how ARs affect the exposure of individuals to low DO. We used a range of DO values representative of the values observed in the field.
Many studies have confirmed the hypoxia avoidance behavior of organisms relative to high DO values (Domenici et al., 2007). Less definitive is when individuals are given choices between hypoxia and moderate DO and moderate DO and high DO conditions. Meyer-Gutbrod et al. (2021) investigated the shifts in the vertical distributions of rock reef fish species in the Southern California Channel Islands in response to hypoxia and showed that 19 of 23 species of fish shifted to a shallower depth. Craig (2012) detected aggregation patterns of many species in nearby oxygenated refuge habitats when hypoxia was most severe. Campbell and Rice (2014) also summarized that fish densities may increase in nearshore oxygenated refuges when hypoxic conditions expand.
However, the effects of the presence of preferred habitats on the capacity for hypoxia avoidance are still relatively unknown for many habitat-dependent species. Mitamura et al. (2021) tracked tagged marbled flatfish and found that 36% of the individuals were still distributed in shallow water around an AR despite warm waters. They suggested this phenomenon was related to the high prey conditions associated with ARs. Rasmuson et al. (2021) used an acoustic telemetry array to monitor the movement trajectories of the deacon rockfish (Sebastes diaconus) for 11 months at a nearshore rocky reef area. They found that the resident deacon rockfish were resistant to moving away from the local reef area and that hypoxia resulted in individuals using more rugose habitats during the daytime. In our study, we detected that AR influenced the capacity for hypoxia avoidance of the two rockfish species, although to different degrees. The presence of ARs caused H. otakii and S. schlegelii to reduce their avoidance capacity, with H. otakii maintaining strong avoidance at 1 mg/L and diminished avoidance when the lower DO was 3 or 5 mg/L, while the more uniformly reduced avoidance of S. schlegelii would generally result in increased exposure. Our results agreed with Rasmuson et al. (2021) and showed that hypoxia directly increased the staying duration of our rockfish species when in AR, boulders, and rubble sections under the light condition, and the capacity of hypoxia avoidance of the rockfishes was influenced (generally reduced) by the presence of ARs. Because we did not use baits in the experimental apparatus, the effect of AR can be viewed as potentially indicative of habitat dependence and avoidance relevant to field conditions.
Responses of reef-dependent species to changing ocean conditions
Documenting how hypoxia affects habitat usage and how the presence of preferred habitat (e.g., ARs) can influence avoidance and therefore exposure can inform future studies and management. Examining the effects of low DO levels on habitat usage allows us to make a precautionary estimate of how low DO may reduce the availability of preferred habitats. Anthropogenic climate change is predicted to increase the frequency and extent of hypoxia (Hughes et al., 2020). Thus, this information can be used to assess changes in habitat, as preferred habitats may decline simultaneously with increasing hypoxia. ARs were preferred for several species in our experiments and are a management tool for enhancing stocks. The presence of ARs weakened the hypoxia-avoidance capacity of the strong habitat-affinity rockfish species, and in different directions for the two species with moderate DO concentrations (i.e., 3 and 5 mg/L). The low oxygen effect was much stronger for the two rockfishes that showed a high degree of preference for specific habitats compared to the relatively small DO effect for the generalist filefish. Experiment II showed that all three fish species examined avoided hypoxic water (1 mg/L DO). Differences among species arose with choices between moderate (3 and 5 mg/L) and high DO concentrations (7 mg/L). Further, crab movement behavior showed little sensitivity to low DO, and some relationships of habitat usage (two rockfish species) were dome-shaped as a function of DO. Anticipating where low DO will occur can ensure the placement of ARs in locations likely to be of high habitat quality in the future. Similarly, knowing how ARs will affect avoidance and exposure to low DO is also useful to select locations where species are productive. Simultaneous examination of complex habitats and low oxygen is essential to consider the tradeoff between placing AR in the best habitat that may also experience hypoxia versus less optimal habitats with little hypoxia. It is especially important to consider such tradeoffs under likely future climate conditions.
Species may be able to cope with hypoxic stress associated with their habitats through phenotypic plasticity, and extreme types (not the average) of individuals can show more capacity for avoidance and higher tolerance (McBryan et al., 2013). Such variation occurs because of among-individual differences in phenotypes that affect their physiology, behavior, and ability to acclimate. Many teleost fish species associated with coral reefs have been investigated for their variability in physiological tolerance to hypoxia (Nilsson and Östlund-Nilsson, 2004; Nilsson et al., 2010; Wong et al., 2018). Zhu et al. (2013) reviewed the hypoxia adaptation of fish through examination of variation in proteins and signaling pathways. Our results can directly inform the adaptation potential of behavioral movements related to the usage of preferred habitats under hypoxia and avoidance of low DO, so reef managers can consider how hypoxia could affect reef-dependent species distribution and productivity.
Design, location selection, and management of reefs may increasingly have to consider hypoxia as a factor. The behavior of target species, as well as unwanted species (e.g., sea stars) in some cases (Hayes and Sliwa, 2003), can be used with other information to infer the performance of ARs in the planning stages and after deployment. In our experiments, the dependence of the rockfish species on ARs was strengthened under low DO with likely increased exposure to low DO concentrations, while the dependence of the sea cucumber was weakened, but individuals changed their behavior to gather in the center of the tank. Experiment II showed that the presence of ARs reduced the hypoxia-avoidance capacity of the rockfish species for low DO (1 mg/L). but with an important difference at higher DO concentrations: H. otakii avoidance was relatively unaffected compared to no AR present, whereas the avoidance of S. schlegelii continued to be diminished. ARs to enhance fisheries have been deployed in areas that experience hypoxia. Examples include the northern Gulf of Mexico continental shelf adjacent to the Mississippi River (Rabalais and Turner, 2019), the Gulf of Finland and deep basins of the Baltic Sea (Kõuts et al., 2021), the western Bohai Sea in China (Wei et al., 2019), and the northeastern area off the Changjiang Estuary (Wei et al., 2021a). The ARs in these systems can affect the local trophic dynamics on or in the vicinity of the ARs (Reeves et al., 2018; Plumlee et al., 2020; Wang et al., 2022), and this may also need to be incorporated into assessments of hypoxia effects on reef layouts, locations, and performance. While hypoxic conditions are a major consideration, sublethal DO concentrations are also important. Many laboratory studies document that DO concentrations between 2 and 5 often result in less avoidance (i.e., significant exposure), and individuals show reduced growth and fecundity (Uphoff et al., 2011; LaBone et al., 2021). Our study showed that the presence of ARs would likely increase the exposure of the two rockfish species to 3 mg/L. Therefore, both hypoxia and higher (moderate) DO concentrations should be part of the design and assessment of performance for ARs.
In addition to how AR locations relate to low DO areas, the connectedness among ARs should also be considered. Based on our results (Experiment I) showing the prolonged staying duration by rock fishes in their preferred habitats, we propose that reef managers consider ways to increase the connectivity of ARs between low and high-oxygen areas. Highly connected AR layouts may help rockfish avoid low DO concentrations while maintaining the overall performance of the ARs. The effective distances between AR monomers are determined by the overall ecological connectivity provided by reef monomers, natural reefs, and the spatial distribution of other habitat types (Logan and Lowe, 2018; Reeds et al., 2018). If the effective distances were considered and adjusted at the design of the ARs or by adding strategic new ARs after operation, corridors that encourage movement among ARs under hypoxia could minimize the low DO effects. Additional experiments like Experiment II that have ARs with both low and high DO conditions, combined with ecological modeling (e.g., Campbell et al., 2011), would further aid in designing AR-centric escape corridors.
Conclusion
Continued eutrophication of coastal waters and global climate change will increase the frequency and scope of hypoxic events (Rabalais et al., 2010). Reef-dependent species typically utilize multiple preferred habitat types that are patchily distributed and form a complex seascape of habitats (Rilov et al., 2007; Huntington et al., 2010). Our study confirmed previous results on the habitat preferences of multiple species that use reef habitats to various degrees. Two laboratory experiments explored the effect of low oxygen on habitat utilization when organisms are faced with multiple habitat types and the effect of the presence of ARs on movement behavior related to avoidance capacity. Taken together, this study indicated that hypoxia would increase the habitat dependence of the sea cucumber S. japonicus, two rockfishes S. schlegelii and H. otakii, and the habitat use of the filefish T. modestus in more expansive waters but have no significant impact on the crab C. japonica. The presence of ARs on the low-oxygen side enhanced the exposure to low DO for the rockfish S. schlegelii, followed by H. otakii, and slightly influenced the flatfish P. yokohamae. Our study adds novel knowledge and evidence to the behavioral responses to hypoxia of reef-dependent species that use complex habitats. The results provide information for assessing the ecological effects and potential for adaptation through behavioral movement for key reef-dependent species under the increasing overlap of ARs and hypoxia anticipated in the future with more ARs being deployed and climate change amplifying hypoxia.
Data availability statement
The original contributions presented in the study are included in the article/Supplementary Material. Further inquiries can be directed to the corresponding authors.
Ethics statement
The animal study was reviewed and approved by Institutional Animal Care and Use Committee of Ocean University of China.
Author contributions
HY came up with the main ideas, conducted all experiments, and wrote the manuscript. GF conducted experiments, offer proposal for the experiment design, and made biological measurements of species. KR offered constructive comments and ideas, instructed to tackle with problems of analysis, revised and edited this manuscript. JL conducted the experiments and look after the experimental species. JF and HW gave constructive ideas and revised the structure and contents of this article. QC offered valuable references and examined statistics test. YT and TZ applied for the research project, offered funds of this study, offered valuable suggestion, and revised this article. All authors listed have made a substantial, direct, and intellectual contribution to the work and approved it for publication.
Funding
This research was supported by the National Key R&D Program of China (2019YFD0901301), the National Natural Science Foundation of China (Grant No. 42106102), the Primary Research and Development Plan of Guangxi Province (Grant No. 2021AB34014), and the Qingdao Postdoctoral Application Research Project (Grant No. QDBSH20220201045). The funders had no role in study design, data collection and analysis, decision to publish, or preparation of the manuscript.
Acknowledgments
We thank all scientific staffs and crew members of the Lab of Marine Fisheries Technology and Yantai Tianyuan Aquaculture Company for their assistance in experiments.
Conflict of interest
The authors declare that the research was conducted in the absence of any commercial or financial relationships that could be construed as a potential conflict of interest.
Publisher’s note
All claims expressed in this article are solely those of the authors and do not necessarily represent those of their affiliated organizations, or those of the publisher, the editors and the reviewers. Any product that may be evaluated in this article, or claim that may be made by its manufacturer, is not guaranteed or endorsed by the publisher.
Supplementary material
The Supplementary Material for this article can be found online at: https://www.frontiersin.org/articles/10.3389/fmars.2023.1109523/full#supplementary-material
References
Bianchi T., DiMarco S., Cowan J., Hetland R., Chapman P., Day J., et al. (2010). The science of hypoxia in the northern gulf of Mexico: A review. Sci. Total Environ. 408, 1471–1484. doi: 10.1016/j.scitotenv.2009.11.047
Breitburg D. L., Baumann H., Sokolova I. M., Frieder C. A. (2019). “Multiple stressors -forces that combine to worsen deoxygenation and its effects,” in Ocean deoxygenation: Everyone’s problem. causes, impacts, consequences and solutions. Eds. Laffoley D. D. A., Baxter J. M. (Gland: IUCN), 225–247.
Breitburg D. L., Levin L. A., Oschlies A., Grégoire M., Chavez F. P., Conley D. J., et al. (2018). Declining oxygen in the global ocean and coastal waters. Science 359, eaam7240. doi: 10.1126/science.aam7240
Caddy J. F. (1993). Toward a comparative evaluation of human impacts on fishery ecosystems of enclosed and semi-enclosed seas. Rev. Fish. Sci. 1, 57–95. doi: 10.1080/10641269309388535
Campbell L. A., Rice J. A. (2014). Effects of hypoxia-induced habitat compression on growth of juvenile fish in the neuse river estuary, north Carolina, USA. Mar. Ecol. Prog. Ser. 497, 199–213. doi: 10.3354/meps10607
Campbell M. D., Rose K. A., Boswell K., Cowan J. (2011). Individual-based modeling of an artificial reef fish community: Effects of habitat quantity and degree of refuge. Ecol. Model. 222, 3895–3909. doi: 10.1016/j.ecolmodel.2011.10.009
Claireaux G., Lefrançois C. (2007). Linking environmental variability and fish performance: integration through the concept of scope for activity. Philos. Trans. R. Soc B-Biol. Sci. 362, 2031–2041. doi: 10.1098/rstb.2007.2099
Craig J. K. (2012). Aggregation on the edge: Effects of hypoxia avoidance on the spatial distribution of brown shrimp and demersal fishes in the northern gulf of Mexico. Mar. Ecol. Prog. Ser. 445, 75–95. doi: 10.3354/meps09437
David L. T., David B. E. (2000). Effects of hypoxia on an estuarine predator-prey interaction: Foraging behavior and mutual interference in the blue crab callinectes sapidus and the infaunal clam prey mya arenaria. Mar. Ecol. Prog. Ser. 196, 221–237. doi: 10.3354/meps196221
Diaz R. J., Rosenberg R. (1996). Marine benthic hypoxia: A review of its ecological effects and the behavioural responses of benthic macrofauna. Oceanographic literature Rev. 12, 1250.
Diaz R. J., Rosenberg R. (2008). Spreading dead zones and consequences for marine ecosystems. Science 321, 926–929. doi: 10.1126/science.1156401
Domenici P., Herbert N. A., Lefrançois C., Steffensen J. F., McKenzie D. J. (2013). “The effect of hypoxia on fish swimming performance and behaviour,” in Swimming physiology of fish: Towards using exercise to farm a fit fish in sustainable aquaculture. Eds. Palstra A. P., Planas J. V. (Berlin, Heidelberg: Springer Berlin Heidelberg Press) 129–159. doi: 10.1007/978-3-642-31049-2_6
Domenici P., Lefrançois C., Shingles A. (2007). Hypoxia and the antipredator behaviours of fishes. Philos. Trans. R. Soc B-Biol. Sci. 362, 2105–2121. doi: 10.1098/rstb.2007.2103
Domenici P., Steffensen J. F., Batty R. S. (2000). The effect of progressive hypoxia on swimming activity and schooling in Atlantic herring. J. Fish Biol. 57, 1526–1538. doi: 10.1111/j.1095-8649.2000.tb02229.x
Domenici P., Steffensen J. F., Marras S. (2017). The effect of hypoxia on fish schooling. Philos. Trans. R. Soc B-Biol. Sci. 372, 236–249. doi: 10.1098/rstb.2016.0236
Eby L., Crowder L. (2002). Hypoxia-based habitat compression in the neuse river estuary: context-dependent shifts in behavioral avoidance thresholds. Can. J. Fish. Aquat. Sci. 59, 952–965. doi: 10.1139/F02-067
Fan W., Pan D., Xiao C., Lin T., Pan Y., Chen Y. (2019). Experimental study on the performance of an innovative tide-induced device for artificial downwelling. Sustainability 11 (19), 1–23. doi: 10.3390/su11195268
Fowler A. E., McLay C. L. (2013). Early stages of a new Zealand invasion by charybdis japonica (A. Milne-Edwards 1861) (Brachyura: Portunidae) from Asia: Population demography. J. Crustac. Biol. 33, 224–234. doi: 10.1163/1937240X-00002127
French C. G., Wahl D. H. (2018). Influences of dissolved oxygen on juvenile largemouth bass foraging behaviour. Ecol. Freshw. Fish 27, 559–569. doi: 10.1111/eff.12370
Gunderson A. R., Armstrong E. J., Stillman J. H. (2016). Multiple stressors in a changing world: the need for an improved perspective on physiological responses to the dynamic marine environment. Annu. Rev. Mar. Sci. 8, 357–378. doi: 10.1146/annurev-marine-122414-033953
Hayes K. R., Sliwa C. (2003). Identifying potential marine pests–a deductive approach applied to Australia. Mar. pollut. Bull. 46, 91–98. doi: 10.1016/S0025-326X(02)00321-1
Herbert N. A., Skjæraasen J. E., Nilsen T., Salvanes A. G. V., Steffensen J. F. (2011). The hypoxia avoidance behaviour of juvenile Atlantic cod (Gadus morhua l.) depends on the provision and pressure level of an O2 refuge. Mar. Biol. 158, 737–746. doi: 10.1007/s00227-010-1601-7
Hrycik A. R., Almeida L. Z., Höök T. O. (2017). Sub-Lethal effects on fish provide insight into a biologically-relevant threshold of hypoxia. Oikos 126, 307–317. doi: 10.1111/oik.03678
Hughes D., Alderdice R., Cooney C., Kuhl M., Pernice M., Voolstra C., et al. (2020). Coral reef survival under accelerating ocean deoxygenation. Nat. Clim. Change 10, 296–307. doi: 10.1038/s41558-020-0737-9
Huntington B. E., Karnauskas M., Babcock E. A., Lirman D. (2010). Untangling natural seascape variation from marine reserve effects using a landscape approach. PloS One 5, e12327. doi: 10.1371/journal.pone.0012327
Jones D. R. (1971). The effect of hypoxia and anaemia on the swimming performance of rainbow trout (Salmo gairdneri). J. Exp. Biol. 55, 541–551. doi: 10.1242/jeb.55.2.541
Jorissen H., Nugues M. M. (2021). Coral larvae avoid substratum exploration and settlement in low-oxygen environments. Coral Reefs. 40, 31–39. doi: 10.1007/s00338-020-02013-6
Jourdan-Pineau H., Dupont-Prinet A., Claireaux G., McKenzie D. J. (2010). An investigation of metabolic prioritization in the European Sea bass, dicentrarchus labrax. Physiol. Biochem. Zool. 83, 68–77. doi: 10.1086/648485
Kõuts M., Maljutenko I., Elken J., Liu Y., Hansson M., Viktorsson L., et al. (2021). Recent regime of persistent hypoxia in the Baltic Sea. Environ. Res. Commun. 3, 075004. doi: 10.1088/2515-7620/ac0cc4
LaBone E. D., Rose K. A., Justic D., Huang H., Wang L. (2021). Effects of spatial variability on the exposure of fish to hypoxia: A modeling analysis for the gulf of Mexico. Biogeosciences 18, 487–507. doi: 10.5194/bg-18-487-2021
Lagos M. E., White C. R., Marshall D. J. (2015). Avoiding low-oxygen environments: oxytaxis as a mechanism of habitat selection in a marine invertebrate. Mar. Ecol. Prog. Ser. 540, 99–107. doi: 10.3354/meps11509
Lefrançois C., Ferrari R. S., Moreira da Silva J., Domenici P. (2009). The effect of progressive hypoxia on spontaneous activity in single and shoaling golden grey mullet liza aurata. J. Fish Biol. 75, 1615–1625. doi: 10.1111/j.1095-8649.2009.02387.x
Lemoine H. R., Paxton A. B., Anisfeld S. C., Rosemond R. C., Peterson C. H. (2019). Selecting the optimal artificial reefs to achieve fish habitat enhancement goals. Biol. Conserv. 238, 108200. doi: 10.1016/j.biocon.2019.108200
Logan R. K., Lowe C. G. (2018). Residency and inter-reef connectivity of three gamefishes between natural reefs and a large mitigation artificial reef. Mar. Ecol. Prog. Ser. 593, 111–126. doi: 10.3354/meps12527
Marcek B. J., Burbacher E. A., Dabrowski K., Winslow K. P., Ludsin S. A. (2020). Interactive effects of hypoxia and temperature on consumption, growth, and condition of juvenile hybrid striped bass. Trans. Am. Fish. Soc 149, 71–83. doi: 10.1002/tafs.10210
McBryan T. L., Anttila K., Healy T. M., Schulte P. M. (2013). Responses to temperature and hypoxia as interacting stressors in fish: Implications for adaptation to environmental change. Integr. Comp. Biol. 53, 648–659. doi: 10.1093/icb/ict066
McNeill B., Perry S. F. (2006). The interactive effects of hypoxia and nitric oxide on catecholamine secretion in rainbow trout (Oncorhynchus mykiss). J. Exp. Biol. 209, 4214–4223. doi: 10.1242/jeb.02519
Messina-Henríquez S., Aguirre Á., Brokordt K., Flores H., Oliva M., Allen P. J., et al. (2022). Swimming performance and physiological responses of juvenile cojinoba seriolella violacea in hypoxic conditions. Aquaculture 548, 737560. doi: 10.1016/j.aquaculture.2021.737560
Meyer-Gutbrod E., Kui L., Miller R., Nishimoto M., Snook L., Love M. (2021). Moving on up: Vertical distribution shifts in rocky reef fish species during climate-driven decline in dissolved oxygen from 1995 to 2009. Glob. Change Biol. 27, 6280–6293. doi: 10.1111/gcb.15821
Mitamura H., Nishizawa H., Mitsunaga Y., Tanaka K., Takagi J., Noda T., et al. (2021). Attraction of an artificial reef: A migratory demersal flounder remains in shallow water under high temperature conditions in summer. Environ. Biol. Fishes 105, 1953–1962. doi: 10.1007/s10641-021-01153-0
Nilsson G. E., Östlund-Nilsson S., Munday P. L. (2010). Effects of elevated temperature on coral reef fishes: Loss of hypoxia tolerance and inability to acclimate. Comp. Biochem. Physiol. A-Mol. Integr. Physiol. 156, 389–393. doi: 10.1016/j.cbpa.2010.03.009
Plumlee J. D., Dance K. M., Dance M. A., Rooker J. R., TinHan T. C., Shipley J. B., et al. (2020). Fish assemblages associated with artificial reefs assessed using multiple gear types in the northwest gulf of Mexico. Bull. Mar. Sci. 96, 655–678. doi: 10.5343/bms.2019.0091
Pörtner H. O., Farrell A. P. (2008). Physiology and climate change. Science 322, 690–692. doi: 10.1126/science.1163156
Portner H. O., Peck M. (2010). Climate change effects on fishes and fisheries: Towards a cause-and-effect understanding. J. Fish Biol. 77, 1745–1779. doi: 10.1111/j.1095-8649.2010.02783.x
Rabalais N. N., Díaz R. J., Levin L. A., Turner R. E., Gilbert D., Zhang J. (2010). Dynamics and distribution of natural and human-caused hypoxia. Biogeosciences 7, 585–619. doi: 10.5194/bg-7-585-2010
Rabalais N. N., Turner R. E. (2019). Gulf of Mexico hypoxia: Past, present, and future. Limnol. Oceanogr. Bull. 28, 117–124. doi: 10.1002/lob.10351
Randall D. (1982). The control of respiration and circulation in fish during exercise and hypoxia. J. Exp. Biol. 100, 275–288. doi: 10.1242/jeb.100.1.275
Rasmuson L. K., Blume M. T. O., Rankin P. S. (2021). Habitat use and activity patterns of female deacon rockfish (Sebastes diaconus) at seasonal scales and in response to episodic hypoxia. Environ. Biol. Fishes 104, 535–553. doi: 10.1007/s10641-021-01092-w
Reeds K. A., Smith J. A., Suthers I. M., Johnston E. L. (2018). An ecological halo surrounding a large offshore artificial reef: Sediments, infauna, and fish foraging. Mar. Environ. Res. 141, 30–38. doi: 10.1016/j.marenvres.2018.07.011
Reeves D. B., Chesney E. J., Munnelly R. T., Baltz D. M., Marx B. D. (2018). Abundance and distribution of reef-associated fishes around small oil and gas platforms in the northern gulf of mexico’s hypoxic zone. Estuaries Coasts 41, 1835–1847. doi: 10.1007/s12237-017-0349-4
Richards J. G. (2009). “Chapter 10 metabolic and molecular responses of fish to hypoxia,” in Fish physiology. Eds. Richards J. G., Farrell A. P., Brauner C. J. (Amsterdam, The Netherlands: Academic Press), 443–485. doi: 10.1016/S1546-5098(08)00010-1
Rilov G., Figueira W. F., Lyman S. J., Crowder L. B. (2007). Complex habitats may not always benefit prey: Linking visual field with reef fish behavior and distribution. Mar. Ecol. Prog. Ser. 329, 225–238. doi: 10.3354/meps329225
Roman M. R., Brandt S. B., Houde E. D., Pierson J. J. (2019). Interactive effects of hypoxia and temperature on coastal pelagic zooplankton and fish. Front. Mar. Sci. 6. doi: 10.3389/fmars.2019.00139
Rose K. A., Adamack A. T., Murphy C. A., Sable S. E., Kolesar S. E., Craig J. K., et al. (2009). Does hypoxia have population-level effects on coastal fish? musings from the virtual world. J. Exp. Mar. Biol. Ecol. 381, S188–S203. doi: 10.1016/j.jembe.2009.07.022
Shimps E. L., Rice J. A., Osborne J. A. (2005). Hypoxia tolerance in two juvenile estuary-dependent fishes. J. Exp. Mar. Biol. Ecol. 325, 146–162. doi: 10.1016/j.jembe.2005.04.026
Steckbauer A., Duarte C., Carstensen J., Vaquer-Sunyer R., Conley D. (2011). Ecosystem impacts of hypoxia: Thresholds of hypoxia and pathways to recovery. Environ. Res. Lett. 6, 025003. doi: 10.1088/1748-9326/6/2/025003
Uphoff J. H., McGinty M., Lukacovic R., Mowrer J., Pyle B. (2011). Impervious surface, summer dissolved oxygen, and fish distribution in Chesapeake bay subestuaries: Linking watershed development, habitat conditions, and fisheries management. North Am. J. Fish Manage. 31, 554–566. doi: 10.1080/02755947.2011.598384
Vaquer-Sunyer R., Duarte C. M. (2008). Thresholds of hypoxia for marine biodiversity. Proc. Natl. Acad. Sci. 105, 15452–15457. doi: 10.1073/pnas.0803833105
Wang X., Feng J., Lin C., Liu H., Chen M., Zhang Y. (2022). Structural and functional improvements of coastal ecosystem based on artificial oyster reef construction in the bohai Sea, China. Front. Mar. Sci. 9. doi: 10.3389/fmars.2022.829557
Wang S. Y., Lau K., Lai K.-P., Zhang J.-W., Tse A. C.-K., Li J.-W., et al. (2016). Hypoxia causes transgenerational impairments in reproduction of fish. Nat. Commun. 7, 12114. doi: 10.1038/ncomms12114
Wannamaker C. M., Rice J. A. (2000). Effects of hypoxia on movements and behavior of selected estuarine organisms from the southeastern united states. J. Exp. Mar. Biol. Ecol. 249, 145–163. doi: 10.1016/S0022-0981(00)00160-X
Watson A. (2016). Oceans on the edge of anoxia. Science 354, 1529–1530. doi: 10.1126/science.aaj2321
Wei Q., Wang B., Yao Q., Xue L., Sun J., Xin M., et al. (2019). Spatiotemporal variations in the summer hypoxia in the bohai Sea (China) and controlling mechanisms. Mar. pollut. Bull. 138, 125–134. doi: 10.1016/j.marpolbul.2018.11.041
Wei Q., Wang B., Zhang X., Ran X., Fu M., Sun X., et al. (2021a). Contribution of the offshore detached changjiang (Yangtze river) diluted water to the formation of hypoxia in summer. Sci. Total Environ. 764, 142838. doi: 10.1016/j.scitotenv.2020.142838
Wei Q., Xue L., Yao Q., Wang B., Yu Z. (2021b). Oxygen decline in a temperate marginal sea: Contribution of warming and eutrophication. Sci. Total Environ. 757, 143227. doi: 10.1016/j.scitotenv.2020.143227
Weiland F., van Beek L., Kwadijk J., Bierkens M. (2012). Global patterns of change in discharge regimes for 2100. Hydrol. Earth Syst. Sci. 16, 1047–1062. doi: 10.5194/hess-16-1047-2012
Wong C. C., Drazen J. C., Callan C. K., Korsmeyer K. E. (2018). Hypoxia tolerance in coral-reef triggerfishes (Balistidae). Coral Reefs 37, 215–225. doi: 10.1007/s00338-017-1649-7
Wu R. (2002). Hypoxia: From molecular responses to ecosystem responses. Mar. pollut. Bull. 45, 35–45. doi: 10.1016/S0025-326X(02)00061-9
Yatsuya K., Matsumoto Y. (2021). Effects of the swimming crab charybdis japonica on sea urchin mesocentrotus nudus grazing: Cage experiments in barren ground and land-based tanks. Fish. Sci. 87, 817–826. doi: 10.1007/s12562-021-01555-0
Yu H., Fang G., Rose K. A., Tang Y., Song X. (2022). Examining epibenthic assemblages associated with artificial reefs using a species archetype approach. Mar. Coast. Fish. 14, e10206. doi: 10.1002/mcf2.10206
Yu H., Yang W., Liu C., Tang Y., Song X., Fang G. (2020). Relationships between community structure and environmental factors in xixiakou artificial reef area. J. Ocean Univ. 19, 883–894. doi: 10.1007/s11802-020-4298-3
Zhang J., Gilbert D., Gooday A. J., Levin L., Naqvi S. W. A., Middelburg J. J., et al. (2010). Natural and human-induced hypoxia and consequences for coastal areas: Synthesis and future development. Biogeosciences 7, 1443–1467. doi: 10.5194/bg-7-1443-2010
Zhou X., Zhang X., Li W. (2018). Effects of temperature and dissolved oxygen on the survival, activity and the adaptation strategy of metabolism in sea cucumber (Apostichopus japonicus). J. Fisheries China 42 (08), 1209–1219.
Zhu C. D., Wang Z. H., Yan B. (2013). Strategies for hypoxia adaptation in fish species: A review. J. Comp. Physiol. B 183, 1005–1013. doi: 10.1007/s00360-013-0762-3
Keywords: habitat usage, hypoxia, behavioral response, reef-dependent species, hypoxia avoidance, exposure to low oxygen
Citation: Yu H, Fang G, Rose KA, Lin J, Feng J, Wang H, Cao Q, Tang Y and Zhang T (2023) Effects of habitat usage on hypoxia avoidance behavior and exposure in reef-dependent marine coastal species. Front. Mar. Sci. 10:1109523. doi: 10.3389/fmars.2023.1109523
Received: 27 November 2022; Accepted: 02 February 2023;
Published: 24 February 2023.
Edited by:
Juan D. Gaitan-Espitia, The University of Hong Kong, Hong Kong SAR, ChinaReviewed by:
Marcelo Lagos, The University of Hong Kong, Hong Kong SAR, ChinaChuanxin Qin, South China Sea Fisheries Research Institute (CAFS), China
Copyright © 2023 Yu, Fang, Rose, Lin, Feng, Wang, Cao, Tang and Zhang. This is an open-access article distributed under the terms of the Creative Commons Attribution License (CC BY). The use, distribution or reproduction in other forums is permitted, provided the original author(s) and the copyright owner(s) are credited and that the original publication in this journal is cited, in accordance with accepted academic practice. No use, distribution or reproduction is permitted which does not comply with these terms.
*Correspondence: Yanli Tang, dGFuZ3lhbmxpQG91Yy5lZHUuY24=; Tao Zhang, dHpoYW5nQHFkaW8uYWMuY24=