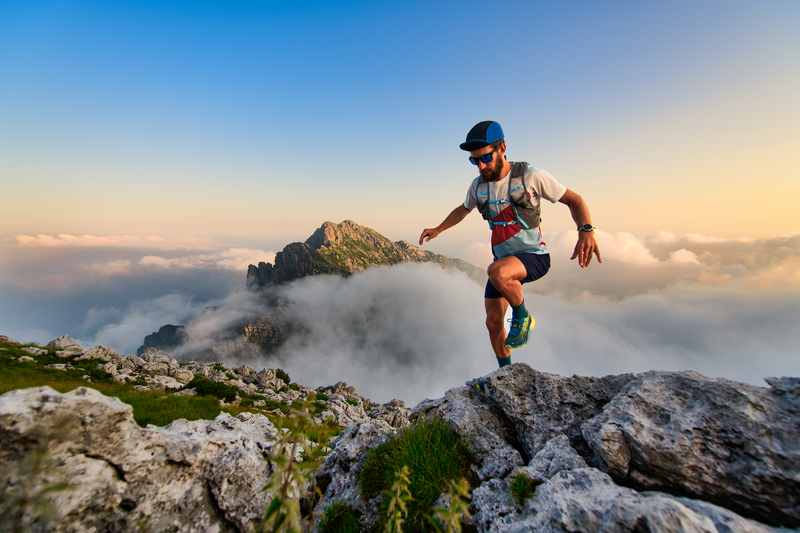
95% of researchers rate our articles as excellent or good
Learn more about the work of our research integrity team to safeguard the quality of each article we publish.
Find out more
ORIGINAL RESEARCH article
Front. Mar. Sci. , 16 March 2023
Sec. Marine Biology
Volume 10 - 2023 | https://doi.org/10.3389/fmars.2023.1104526
This article is part of the Research Topic Marine Invertebrates and Sound View all 12 articles
Introduction: Noise pollution is a major stressor in the marine environment; however, responses of economically and ecologically important invertebrates, such as oysters, are largely unknown.
Methods: Under laboratory conditions, we measured acute behavioral and physiological responses of eastern oysters (Crassostrea virginica) to sound treatments mimicking human activity in the environment.
Results: Oysters immediately reduced their valve gape under simulated pile driving sound, but not drilling or boating sound. Pile-driving sound also reduced adductor muscle glycogen, but not triglyceride. None of the sound treatments affected longer-term (12 hours) valve activity levels after the administration of sounds. Interestingly, neither acute nor longer-term valve gaping responses were correlated with glycogen content on the individual level, suggesting that the observed behavioral responses to sound were not mechanistically driven by energetic physiology.
Discussion: Our results suggest that C. virginica responds to some, but not all, anthropogenic sounds. Future studies assessing downstream effects on growth, reproduction, and survival in the wild are needed to better understand the effects of anthropogenic sounds on oyster populations and the biological communities they support.
In recent years, the ecological consequences of sound caused by human activity in the marine environment have become a topic of contemporary interest (Williams et al., 2015; Popper and Hawkins, 2016; Wale et al., 2019; Duarte et al., 2021). With increasing nautical activities in coastal areas (e.g., pile driving, cargo shipping, drilling, recreational activities), marine organisms are increasingly exposed to anthropogenic noise pollution. Anthropogenic noise is expected to have wide ranging effects on marine organisms, including both lethal and sub-lethal impacts (Tyack, 2008; Johansson, 2011; Popper and Hawkins, 2016). At present, studies regarding the impact of noise on marine organisms have focused largely on fish and mammals (Peng et al., 2015). Yet, despite representing >90% of marine organisms, there is a lack of information regarding the effects of sound on invertebrates (Nedelec et al., 2014). Further studies are thus urgently required to better understand the impact of noise pollution on these marine organisms (Solé et al., 2023). Among invertebrates, bivalves are some of the most commercially and ecologically valuable. In 2018 bivalve aquaculture yielded a global production of 17.7 million metric tons, more than doubling the production of marine and coastal finfish aquaculture (FAO, 2020). Bivalves are increasingly recognized not only for their substantial ecological value, but for their economic importance as well (Clements and Comeau, 2019a; Van der Schatte Olivier et al., 2020). While shellfish aquaculture and fisheries are important economic activities for current and expanding coastal communities, these activities expose bivalves to various sounds (e.g., boat engines, mechanical sorting), the impact of which is still poorly understood. Although bivalves can tolerate a wide range of environmental stressors (Pourmozaffar et al., 2019), little is known of their susceptibility to anthropogenic noise pollution (Firestone and Jarvis, 2007; Bittencourt et al., 2014; Peng et al., 2015; Williams et al., 2015; Jolivet et al., 2016; Bonnel et al., 2022). One indicator of stress in bivalves is valve gaping behavior (Clements and Comeau, 2019b). A wide valve opening in bivalves can be indicative of an unstressed animal (Tran et al., 2011; Tran et al., 2016), while partial or complete valve closure can be considered as a protective response when threatened or stressed (Charifi et al., 2017; Charifi et al., 2018). Behaviorally, some cockles (Cardium edule) are known to close their valves in response to vibrations (Kastelein, 2008). Valve closures in response to sound are also reported for mussels (Mytilus edulis) (Roberts et al., 2015). Pacific oysters, Magallana gigas, were reported to engage in transient valve closures in response to sound in a frequency-dependent manner, responding to sound frequencies of 10 to <1000 Hz, with maximum responses occurring between 10 to 200 Hz (Charifi et al., 2017). In a recent field experiment, Doyle et al. (2020) reported that giant clams, Tridacna maxima, responded behaviorally to sound by increasing the frequency of mantle retractions, and may become “distracted” (i.e., alter predator avoidance behaviors) by sound in areas of water flow. Stress responses, however, usually involve adjustments to all levels of animal organization, and physiological and molecular impacts may therefore accompany behavioral responses to stressors. For example, stress can impact energetic physiology and reduce the amount of energy available for growth and reproduction (Calow, 1985). With respect to sound, Peng et al. (2016) reported that marginal effects of sound on digging behavior in Asian razor clams (Sinonovacula constricta) were accompanied by increased O:N ratios (oxygen consumed versus nitrogen excreted), although metabolic and excretion rates were unaffected. Likewise, Charifi et al. (2018) reported that Pacific oysters exhibited reduced valve gaping and gill function in response to noise pollution, which positively resulted in less metal accumulation, but negatively drove reductions in feeding and growth. Reductions in physiological energetic parameters such as glycogen or lipids (i.e., triglycerides) can also be used as indicators of stress in bivalves (Widdows, 1985). Overall, however, few studies have assessed the effects of sound on bivalves, and those consolidating behavior and physiology are lacking. Notably, studies have yet to consolidate valve gaping behavior and physiological energetics in the context of noise pollution. As part of the National Ecosystem Stressors Program, the Department of Fisheries and Oceans Canada (DFO) recently designated acoustic disturbance as a priority stressor of national importance. Likewise, stressor effects on ecological and economically valuable bivalves are of significant interest and importance under DFO’s mandate to protect Canada’s aquatic ecosystems from negative impacts. The eastern oyster (C. virginica) is a valuable commercial species with a wide geographic distribution occupying an important place in the marine ecosystem (Lacoste et al., 2016). In Atlantic Canada, C. virginica supports the local economy through commercial fisheries and aquaculture activities and is of significance for the ecosystem services it provides (Clements and Comeau, 2019a). Given the paucity of information regarding the effect of sound on bivalves, coupled with the importance of noise pollution and bivalves to DFO’s mandate, the goal of this study was to experimentally determine whether anthropogenic noise could affect the valve gaping behavior and energetic physiology of adult Eastern oysters (Crassostrea virginica) under a laboratory setting. Based on previous observations of bivalve molluscs in response to noise pollution (e.g., Kastelein, 2008; Roberts et al., 2015; Charifi et al., 2017), we hypothesized that exposure to sound would affect the behavior of the Eastern oyster by reducing the opening of the valves and that exposure to sound would result in lower concentration of energy reserves in the form of glycogen and triglycerides – physiological responses that have yet to be documented in response to noise pollution in bivalves (to the best of our knowledge).
In October 2019, 72 adult oysters (C. virginica; mean ± SD shell length: 59.2 ± 3.9 mm) were collected from an oyster aquaculture site in Lamèque Bay, New Brunswick, Canada (64° 40’ 6” W, 47° 47’14.7” N). The oysters were then transported to the Institute of Marine Sciences in Rimouski (ISMER) where they were each connected to a non-invasive valvometry system (DC-204R, Tokyo Sokki Kenkyujo Co., Japan) described in Nagai et al. (2006) (see 2.4 Behavioral measurements section below for details). The oysters were then placed in a recirculating seawater system (Multi-stressor units, Aquabiotech System) and were acclimatized to laboratory conditions for seven days prior to behavioral experiments. The seawater temperature was initially kept the same as the field conditions from which the oysters came (≈12 °C) and was gradually increased to 18 °C over the seven-day acclimatization period, where they remained for approximately two days; temperature during the experimental period was held constant at 18 °C. Salinity was held constant at approximately 27 PSU during both the acclimatization and experimental periods. During acclimatization, oysters were fed 1% of their dry mass daily with a tri-species microalgal mixture (1:1:1 ratio of Isochrysis galbana, Pavlova lutheri, and Chaetoceros gracilis).
Alongside a control treatment (i.e., no sound), three sound treatments were selected for the experiment: 1) Fishing boat; 2) Drilling; and 3) Pile driving (Figure 1). Sound treatments were recorded in each individual experimental unit with a Loggerhead LS1 underwater acoustic recorder (sampling frequency of 44.1 kHz) equipped with a HTI-96-MIN hydrophone (sensibility -170 dB re 1VµPa-1). Sound levels were adjusted to match field realistic situations. The boat sound used was the same and at similar levels, as described in Jolivet et al. (2016) and displayed a mussel culture boat of 11 meters long equipped with a diesel motor (300 hp). Drilling and pile driving sounds were recorded during the offshore wind farm installation in the bay of Saint-Brieuc (France) with a calibrated hydrophone (High Tech, Inc., HTI-99-HF: sensitivity −169.7 dB re 1 V/μ Pa; frequency range 2 Hz to 125 kHz). Spectral composition and source sound level were determined using the MATLAB (The MathWorks, Inc.) software to select a 30 s sequence that was repeated during emission. We specifically sought to test the three sounds without interference from other natural sounds in the aquatic environment (and any interpolation of our results to natural systems should therefore be made with caution). Sounds were administered in the Larvosonic system (Figure 1) previously developed for studying the impacts of anthropogenic noises on the early stages of benthic marine invertebrates. Olivier et al. (2023) provide a comprehensive description of the Larvosonic system and its acoustic characteristics. In summary, integrated acoustic panels (diffuser and bass trap components) effectively dampen the reflection of the whole frequency bandwidth, and as already detailed in Olivier et al. (2023), when the source level increases by N dB, both Pressure Energy and Kinetic Energy increase by N dB even if impedance (ratio between KE/PE) i) evolves nonlinearly as a function of the source–receiver distance and ii) for a given source–receiver configuration, the impedance evolves nonlinearly as a function of frequency. All oysters were placed at the bottom of each cylinder so that the impedance ratio is similar for a fixed frequency for the four external cylinders but slightly varies from the internal ones (lower speaker distance). As described in Figure 1, our study design incorporated one Larvosonic system per sound condition, which each system consisting of a large tank (120 cm length × 68 cm width × 68 cm depth) filled with freshwater and supporting 6 semi-submerged experimental cylinder units that constitute 6 replicates. An underwater loudspeaker (Clark Synthesis AQ339, Diluvio, 8Ohms/20-17 000Hz) was positioned in the center of each tank to diffuse the sound. Speakers were connected to a Denon amplifier (DN-300Z/16–bit/20-20 000Hz/44.1KHz), then to a matrix mixer with a signal processor (Yamaha 26x8 MTX3, Buena Park, CA, USA). In each tank corresponding to a specific sound, comparative SPL00-pk measurements were obtained between each experimental unit, as less than~ 6dB were measured between central and external cylinders (see Table 1 in Olivier et al., 2023 for additional information). There was a weak contamination of the control sound treatment (no added sound) by sound emissions of other tanks estimated to + 9 dB re 1 µPa (compared to the sound level of the experimental room without any sound emission).
Figure 1 Experimental design used to test for effects of anthropogenic sounds on behavioral and physiological responses in Eastern oyster, C. virginica, including sound treatment conditions. The bottom panel (in dashed red box) is a 3D representation of the experimental tanks developed at the Institut des Sciences de la Mer à Rimouski – ISMER (Larvosonic system; Olivier et al., 2023). Note that the experiment was repeated two times and that some oysters were removed prior to analysis due to technical and/or logistical issues (see 2.3 Experimental design section for details). Ambient SPL peak to peak in the room (Local) was 114.50 ± 0.10 dB re 1µPa.
Each tank contained six semi-submerged cylinder units (n = 1 oyster cylinder-1, 6 oysters tank-1), each filled with eight liters of filtered seawater (10 and 1 μm filters). Once the oysters were placed at the bottom of each individual cylinder, they acclimatized for nine hours after which they were continuously exposed to their respective sound treatment for 12 hours; oysters were not fed during this time. Valve gaping behavior was continuously measured throughout the acclimatization and experimental periods, and individual tissue samples (adductor muscle and digestive gland) were collected at the end of the sound exposure period. The experiment was repeated twice (n = 12 oysters treatment -1 total) over a period of three days. Technical issues (i.e., malfunctioning sensors) with some of the valvometry systems resulted in the loss of data for some individuals, resulting in final sample sizes of 7, 8, 6, and 10 oysters for the Boat, Drilling, Pile Driving, and Control treatments, respectively.
Each individual oyster was connected to a non-invasive valvometry system (DC-204R, Tokyo Sokki Kenkyujo Co., Japan) described in Nagai et al. (2006). A Hall element sensor (HW-300a) was attached to the external ventral margin of one valve with UV resin (Solarez, Wahoo International, Vista, CA, USA) and a small magnet was attached to the external ventral margin of the opposite valve. Functionally, the Hall sensor measures the magnetic flux (flux density) between it and the magnet, which is proportional to the distance between the sensor and the magnet. This flux density was then translated to a microvoltage (μV) via Dynamic Strain Recorders (DC 204R) and recorded on a SD card. For the purposes of this experiment, data were recorded at a frequency of one measurement per second. Upon completion of each experiment, the linear relationship between μV and valve opening (i.e., the μV value at a range of known mm distances between the ventral margins of the two valves) was derived for each individual oyster to calculate the valve opening for each point μV measurement. We then computed the relative change (%) in the oyster valve opening in response to each sound using the following equation:
where VOb and VOa represent the mean valve opening (in mm) 5 mins before (VOb) and 5 mins after (VOa) the application of sound. Herein, a negative number indicates a valve closure (avoidance) in response to sound, while a positive value indicates an opening in response to sound. Alongside the relative change (%) in oyster valve opening, we also computed the longer-term valve activity levels by adding up the total distance moved (in mm) for each oyster after the administration of sounds. Herein, the absolute (+ sign) distances of each measured valve opening, and closure (in mm) were summed for each individual oyster to compute the “total distance moved” over the 12 hours observation period following the administration of the sound treatment.
To document physiological energetics and relate them back to any observable behavioral effects, glycogen and triglyceride concentrations were measured in each oyster. Glycogen concentration was determined in the adductor muscle using a slight modification of the method described in Keppler and Decker (1974). Briefly, 30 mg of adductor muscle was homogenized in 5 volumes of 6% perchloric acid using a sonicator (Q55 Sonicator). The homogenate was then neutralized with 1.5 volumes of 2M KHCO3. Then, 50 µl of the slurry was transferred to a clean tube and the glycogen was then hydrolyzed by adding 100 µl of amyloglucosidase (56 U ml-1) in a 0.4 M sodium acetate buffer (pH 4.8). Following a 120-minute incubation at 40°CC, the hydrolysis was stopped by adding 50 µl of 6% PCA and the acid was neutralized by adding 50 µl of 2M KHCO3. The sample was then centrifuged at 2000 g for 5 mins and the supernatant was kept. Each sample was also processed without hydrolysis by adding PCA before amyloglucosidase to determine and remove the concentration of free glucose from that of hydrolyzed glycogen. The glucose concentration was then measured using a coupled enzyme test as described by Williams et al. (2019). The glycogen content is reported as μmoles glycosyl units · g of tissue-1. Triglyceride concentration was determined in the digestive gland. Triglycerides were extracted according to Bligh and Dyer (1959) with slight modifications. Approximately 45 mg of digestive gland tissue was homogenized in 1 ml of methanol using a sonicator followed by the addition of 2 ml of chloroform. The solution was incubated at room temperature for 120 mins and mixed by inversion every 15 minutes. Then, 0.6 ml of distilled water was added to the mixture to generate phase separation. Following a centrifugation at 1000 g for 5 mins, the organic phase was removed and transferred to a new tube and the chloroform was completely evaporated under a fume hood. The extracted lipids were resuspended in 200 μl of ethanol and the triglyceride concentration was measured using a commercial kit according to the supplier’s instructions (InfinityTM Triglycerides Liquid Stable Reagent, Thermo Scientific Inc.). The triglyceride concentration is reported as μmoles · g of tissue-1.
To test for sound effects on behavioral (relative change in valve opening and valve activity levels) and physiological (glycogen and triglyceride content) responses, we built mixed linear effects (LME) models and used ANOVA to test for the effect of treatment on each response variable (significance level of p ≤ 0.05). Models included sound treatment as a fixed categorical factor with four levels (boat, drilling, pile driving, and control) and experiment as a categorical random variable. Linear regression was used to determine whether physiological energetics (glycogen and triglyceride content) were related to changes in both valve gaping responses to sound. Assumptions of homoscedasticity and normality of the residuals were verified using Levene’s tests and Shapiro-Wilk tests, respectively. A logarithm transformation was applied to the glycogen content variable. Statistical analysis was performed with R software (RStudio version 3.6.2; R Core Team, 2020). Linear mixed models were built using the lmer() function from the lmerTest package (Kuznetsova et al., 2017), and the Anova() function from the car package (Fox and Weisberg, 2019; with Type 3 sum of squares) was used to obtain fixed effect significance. Where significant overall effects of sound were detected, Tukey HSD post hoc comparisons were used to determine pairwise group differences using the glht() function in the multcomp package (Hothorn et al., 2008).
Examples of typical individual valve gaping responses in each sound treatment are depicted in Figure 2. Linear mixed effects results indicated a significant overall effect of sound treatment on the relative change in valve opening (LME ANOVA: X23 = 10.47, p = 0.015). Tukey HSD pairwise comparisons revealed that valve opening was significantly decreased in oysters from the Pile Driving treatment as compared to the Control (p = 0.0086), while valve gaping was unaffected by Boat (p = 0.8541) and Drilling (p = 0.8503) sounds (Figure 3A). In contrast to the relative change in valve opening, valve activity levels (total distance moved (in mm) after the administration of sounds) were not affected by any of the sound treatments (LME ANOVA: X23 = 4.22, p = 0.2385; Figure 3B).
Figure 2 Recordings of valve gaping activity of a single representative oyster responding to the (A) Control, (B) Boat, (C) Drilling, and (D) Pile driving sound treatments over a period of 21 hours. The vertical red line indicates the start of sound exposure. Note the immediate and sustained decrease in valve gaping after sound exposure in panel (D).
Figure 3 (A) Relative change (%) in oyster valve opening between five minutes before and after the start of sound treatments. Letters above the bars represent pairwise differences according to Tukey HSD tests. (B) Valve activity levels (total distance moved, in mm) by oysters after the administration of sound treatments. Each point represents an individual oyster.
Oyster glycogen reserves were significantly affected by sound (LME ANOVA: X23 = 11.4, p = 0.0098). Tukey HSD pairwise comparisons indicated that oyster glycogen concentrations were significantly lower in the Pile Driving treatment as compared to the Control (p = 0.0062), while the other two sound treatments were statistically similar to the Control (Figure 4A). In contrast to glycogen, triglyceride content was unaffected by any of the sound treatments (LME ANOVA: X23 = 0.44, p = 0.9308; Figure 4B). Although sound treatments appeared to affect valve opening and glycogen in similar ways, linear regression revealed no relationship between individual changes in valve opening and individual glycogen content (F1,29 = 2.34, p = 0.137, R2 = 0.07). Likewise, triglyceride content was not related to relative change in valve gaping (Linear regression: F1,29 = 0.25, p = 0.621, R2 = 0.009). Linear regression also revealed no relationship between individual valve activity levels and individual glycogen content (F1,29 = 0.000012, p = 0.997, R2 = -0.03), nor triglyceride content (F1,29 = 0.56, p = 0.462, R2 = -0.02).
Figure 4 Concentration of glycogen in the adductor muscle (A) and triglyceride in the digestive gland (B) of oysters from each of the four sound treatments. Each point represents an individual oyster. The letters above the bars indicate the results of the multiple comparison test (Tukey) for the significant effect of sound treatments on the amount of glycogen.
To our knowledge, this is the first study to link behavioral and physiological responses to anthropogenic noise in bivalves. Our results suggest that certain sounds such as pile driving can affect oyster behavior and physiology by reducing valve gaping amplitudes and glycogen concentration in the adductor muscle, while other sounds such as boating and drilling into the sea floor appear to have a negligible effect. These results indicate that bivalve behavior and physiology may be sensitive to some, but not all, anthropogenic noises when applied acutely. Based on these experimental observations, the noise created by prolonged periods such as pile driving sound near oyster beds has the potential to exert population and community level impacts where oysters are present in high abundance; however, more direct research is necessary. In this experiment, pile driving sounds were characterized by short pulses of high-level soundwaves, while the boating and drilling sounds were characterized by more sustained levels of lower-level sound. Bivalves thus appear to be sensitive to anthropogenic sounds commonly occurring in coastal regions. Varied responses to the different treatments could be attributed to both the frequency and amplitude of certain sounds. Indeed, Charifi et al. (2017) reported that the valve gaping responses of Pacific oysters, Magallana gigas, to sound were frequency dependent. Such sound-specific responses have been observed in other studies as well. For example, behavioral responses of coral reef fishes to boat noise depend on engine type, which is likely a result of different types of sounds produced by different types of engines (McCormick et al., 2018; McCormick et al., 2019). The magnitude of behavioral responses in squid (Sepioteuthis australis) are also reported to increase incrementally as sound levels from air guns increased (Fewtrell and McCauley, 2012). In the context of other studies, our results ultimately suggest that responses of marine organisms to sound are likely complex, appearing both species and sound specific. Given the paucity of information on the responses of bivalves and other invertebrates to anthropogenic noise, additional research is strongly warranted.
One indicator of stress in bivalves is valve gaping behavior (Clements and Comeau, 2019b). Valve gaping has been used to monitor bivalve stress in experiments involving chemical and nutritional stressors (Di Fiori et al., 2012; Cordeiro et al., 2017), environmental fluctuations (Palais et al., 2011; Dowd and Somero, 2013), and other stressors such as oxygen and salinity (Tang and Riisgård, 2016; Woodin et al., 2020). In many circumstances, bivalves tend to completely or partially close their valves to avoid stressful conditions. For example, bivalves tend to partially close their valves in response to the threat of predation, perhaps to ‘hide’ from predators (Smee and Weissburg, 2006; Carroll and Clements, 2019; Clements et al., 2020; Clements et al., 2021). Likewise, oysters tend to close in response to stressful low oxygen conditions (Porter and Breitburg, 2016; Coffin et al., 2021). Shell closure and the restriction of filtration are behavioral responses by which oysters can also limit soft tissue exposure to noxious or stressful agents (Hegaret et al., 2007). In our experiments, we observed that oysters in simulated pile driving noise exhibited rapid valve closures (almost completely in some circumstances; Figure 2D) in the first seconds-minutes following exposure to noise, followed by a gradual reopening of valves. While drastically understudied, valve closure responses to sound in bivalves have also been reported in blue mussels (Roberts et al., 2015) and Pacific oysters (Charifi et al., 2017). As such, pile driving sounds (or at least sounds with similar characteristics to our pile driving treatment) appear to represent an acute anthropogenic stressor for eastern oysters. Given this species’ remarkable latitudinal distribution range (4,000 km) along North America’s coastline (Carriker and Gaffney, 1996), and the requirement of pile driving for bridge and wharf construction, it is possible that numerous oyster populations have been impacted over time. In contrast, however, there were no significant differences in valve activity levels during the 12 hours following sound exposure, suggesting no long-term behavioral impacts.
Alongside behavioral responses to sound intensities mimicking pile driving, we also observed significant reductions in glycogen content in oysters exposed to simulated pile driving sound. Glycogen content in bivalves is known to decrease in the presence of various other stressors as well. For example, Encomio and Chu (2000) reported that glycogen content was reduced in the adductor muscle of C. virginica with increased exposure to polycholobiphenyl (PCBs). Similarly, acute exposures to heavy metals such as HgCl2 and CdCl2 can reduce glycogen content in freshwater bivalves, Lamellidens marginalis (Sonawane and Sonawane, 2018). Increases in water temperature are also widely reported to affect glycogen content in bivalves (Andrade et al., 2018; Clements et al., 2018; Weber et al., 2020). Such reductions in glycogen content likely reflect the need for energy utilization to avoid stressful conditions. The reduction in glycogen may be associated with a “ flight” response (McCarty, 2016) where oysters mobilized glucose molecules via glycogenolysis (Wright et al., 2008), perhaps in attempt to avoid physical stress caused by pile driving sound (Hegaret et al., 2007; Sampaio and Freire, 2016). While it may thus be tempting to associate the observed reductions in glycogen content with changes in valve gaping, we did not observe any correlation between individual glycogen content and either of our valve gaping responses (acute valve closures nor longer-tern valve activity levels). This lack of correlation is not totally unexpected given that bivalve adductor muscles are comprised of both smooth and striated muscle fibers, allowing for rapid and prolonged valve closures (either full or partial) without expending additional energy (i.e., “catch contractions”; Galler et al., 2010). It thus seems that the utilization of glycogen supplied energy to some other process involved in stress avoidance that we, unfortunately, did not measure. As such, a mechanistic understanding of sound-related changes in glycogen content awaits further research.
Although we observed significant reductions in glycogen content in response to pile driving sound, triglyceride content remained unaffected. This lack of effect on triglycerides is probably related to the acute nature of our experiments. For example, Vinagre et al. (2012), showed that it takes exposure to a stressor for more than 15 days in order to observe effects on triglyceride stores. In our study, the period of exposure to anthropogenic noise was twelve hours. Likewise, Plaistow et al. (2001) reported that physiological stresses of shorter duration will deplete glycogen reserves while prolonged stresses will draw on triglyceride reserves. While the lack of effect on triglycerides is not surprising, experiments with longer exposure times are needed to understand the chronic impacts of anthropogenic noise on coastal bivalves. Interestingly, the degree of valve opening following sound was only a fraction of the pre-sound valve opening, and this reduced valve gaping was evident for many minutes following exposure to simulated pile driving noise (e.g., Figure 2D). Coupled with the significant reduction in glycogen content, these results suggest that anthropogenic noise associated with pile driving sound may have broad-reaching effects on coastal bivalves. In oysters, stress can reduce the energy available for growth and reproduction. For example, Bøhle (1972) reported reduced filtration activity under stressful salinity conditions, which has been linked to reduced valve gape amplitudes under low salinity (Casas et al., 2018; but see Dodd et al., 2018 for contrasting results whereby stress does not reduce filtration). As such, behavioral and physiological responses to the pile driving sound herein have the potential to impact oyster growth rates and thus have implications for bivalve fisheries and aquaculture production. Bivalves also provide important ecosystem services such as water filtration, which could be affected in areas where pile driving, or exposure to various sounds are prevalent. Indeed, noise-driven changes in valve gaping behavior and gill function have been linked to depressed feeding and growth in Pacific oysters, Magallana (Crassostrea) gigas, during 14-day exposures (Charifi et al., 2018). However, it is important to note here that such effects remain speculative, as our experiment measured acute (<12 hours) responses to sound, and that of Charifi et al. (2018) was also short-term (14 days). Indeed, recent evidence suggests that bivalves may be able to adapt to repeated exposures to stress (Clements et al., 2021) and it is certainly possible that oysters are able to habituate to repeated sound for longer exposures, particularly under natural conditions. Indeed, we observed no effects of any sound treatment on activity levels 12 hours following the sound, suggesting that sound impacts on bivalve behavior may be restricted to acute responses. Furthermore, as previously mentioned, exposure to anthropogenic sounds in our experiments was administered in the absence of natural coastal soundscapes, further complicating direct inferences to natural systems. Recent laboratory and field studies revealed that habitat-related sound can affect settlement in oyster larvae (Lillis et al., 2013). Field experiments testing effects of prolonged sound intensities similar to our pile driving treatment on oyster behavior, physiology, feeding, growth, and survival are ultimately needed to determine if the anthropogenic noises tested herein can have population- and community-level effects on oyster-associated systems. As with any experiment, our study is subject to limitations. Of particular note is that our sample size is low (6−10 individuals per sound treatment), and our results should be interpreted with some caution. To overcome this limitation, future studies should include more individuals. Nonetheless, the trends in the data are relevant and align with the results of other studies on anthropogenic noise and bivalves (Roberts et al., 2015; Vazzana et al., 2016; Charifi et al., 2017). Additionally, we are unable to determine if it is the intensity of sound, the frequency of sound, or both that resulted in valve gaping behavior changes with our data. Other studies have documented that blue mussels (Roberts et al., 2015) and Pacific oysters (Charifi et al., 2017) are sensitive to a wide range of sound frequencies. To the best of our knowledge, studies testing the effects of anthropogenic noise on bivalves have not mechanistically determined which attribute of sound (e.g., intensity vs. frequency) results in animal responses. Indeed, it is possible that different attributes of sound may drive responses of different biological traits. For example, it may be that the intensity of sound affects gaping behavior while the frequency or duration of sound affects physiology. While this mechanistic understanding is not possible from our data, future studies would thus benefit from teasing out which sound attributes drive bivalve responses to sound. On top of that, we only used one sound recording per sound treatment. In order to generalize these recordings to various noises with similar intensities and frequencies, it would require using multiple recordings from multiple sources for each treatment (e.g., multiple recordings from multiple boats). Future studies should try to use multiple recordings from multiple sources of the same sound treatment.
Over the past decade, noise pollution has been a topic of contemporary importance in the marine environment, including coastal areas habited by bivalves. Coupled with previous studies, the behavioral and physiological responses to sounds detected in this study suggest that Eastern oysters (C. virginica) may be sensitive to some, but not all, sounds created acutely by anthropogenic activity in coastal systems. As oysters play important economic and ecological roles in nearshore coastal communities, more studies regarding the effects of various noises on oysters in their natural environment are warranted. Studies including chronic effects on ecologically and economically critical traits such as growth, reproduction, and survival are needed to better understand the potential effects of anthropogenic noise on bivalve fisheries and aquaculture production, as well as the ecosystem services that bivalves provide.
The original contributions presented in the study are included in the article/Supplementary Material. Further inquiries can be directed to the corresponding author.
Ethical review and approval were not required for the study on animals (bivalve molluscs) in accordance with the legislation and requirements of the Canadian Council of Animal Care.
TL conceptualized, designed, and setup the experiments, analyzed data, and wrote and revised the manuscript. JC assisted with analyzing data and writing/revising the manuscript. LAC provided financial and supervisory support, assisted with conceptualizing and designing the experiment, experimental setup, data analysis, and revising the manuscript. GC realized and analyzed implementation of sound profiles. RT provided in kind support, assisted with conceptualizing and designing the experiment, set up and conducted the experiment, and revised the manuscript. FO and LC assisted with the design and implementation of sound profiles, and revised the manuscript. RB provided financial and supervisory support, and revised the manuscript. SL provided financial and supervisory support, assisted with conceptualizing and designing the experiment, assisted in data collection, and revised the manuscript. All authors contributed to the article and approved the submitted version.
This work was supported by the Ecosystem Stressors Program’s Operational Funds (Fisheries and Oceans Canada, Gulf Region) and a NSERC Discovery grant to S.G.L. (RGPIN-2019-05751).
We want to thank Michelle Maillet and Erica Watson at Fisheries and Oceans Canada, along with Viviane Baldwin, Loïck Ducros, and Chloé Melanson in Dr. Simon Lamarre’s lab at Université de Moncton, for assistance with laboratory procedures. Thanks also to the team at ISMER for technical assistance with animal husbandry and sample processing. We thank Delphine Mathias (SOMME consulting company, Brest, France) for conducting the acoustic analysis. A special thanks to Dr. Rémi Sonier and Dr. Luke Poirier at Fisheries and Oceans Canada, for constructive feedback on earlier versions of this manuscript.
The authors declare that the research was conducted in the absence of any commercial or financial relationships that could be construed as a potential conflict of interest.
All claims expressed in this article are solely those of the authors and do not necessarily represent those of their affiliated organizations, or those of the publisher, the editors and the reviewers. Any product that may be evaluated in this article, or claim that may be made by its manufacturer, is not guaranteed or endorsed by the publisher.
The Supplementary Material for this article can be found online at: https://www.frontiersin.org/articles/10.3389/fmars.2023.1104526/full#supplementary-material
Andrade J. T., Cordeiro N. I., Montresor L. C., Luz D. M., Luz R. C., Martinez C. B., et al. (2018). Effect of temperature on behavior, glycogen content, and mortality in Limnoperna fortunei (Dunker 1857)(Bivalvia: Mytilidae). J. Limnol. 77 (2) , 189–98. doi: 10.4081/jlimnol.2017.1658
Bøhle B. (1972). Effects of adaptation to reduced salinity on filtration activity and growth of mussels (Mytilus edulis l.). J. Exp. Mar. Biol. Ecol. 10 (1), 41–47. doi: 10.1016/0022-0981(72)90091-3
Bittencourt L., Carvalho R. R., Lailson-Brito J., Azevedo A. F. (2014). Underwater noise pollution in a coastal tropical environment. Mar. pollut. Bull. 83 (1), 331–336. doi: 10.1016/j.marpolbul.2014.04.026
Bligh E. G., Dyer W. J. (1959). A rapid method of total lipid extraction and purification. Can. J. Biochem. Physiol. 37 (8), 911–917. doi: 10.1139/y59-099
Bonnel J., Chauvaud S., Chauvaud L., Mars J., Mathias D., Olivier F. (2022). The effect of anthropogenic sounds on marine life; the example of offshore wind projects. Éditions Quae, 168.
Calow P. (1985). “Adaptive aspects of energy allocation,” in Fish energetics (Dordrecht: Springer), 13–31.
Carriker M. R., Gaffney P. M. (1996). “A catalogue of selected species of living oysters (Ostreacea) of the world,” in The eastern oyster crassostrea virginica. Eds. Kennedy V. S., Newell R. I. E., Eble A. F. (Maryland Sea Grant College, College Park), 1–18.
Carroll J. M., Clements J. C. (2019). Scaredy-oysters: in situ documentation of an oyster behavioural response to predators. Southeastern Nat. 18 (3), N21–N26. doi: 10.1656/058.018.0303
Casas S. M., Filgueira R., Lavaud R., Comeau L. A., La Peyre M. K., La Peyre J. F. (2018). Combined effects of temperature and salinity on the physiology of two geographically-distant eastern oyster populations. J. Exp. Mar. Biol. Ecol. 506, 82–90. doi: 10.1016/j.jembe.2018.06.001
Charifi M., Miserazzi A., Sow M., Perrigault M., Gonzalez P., Ciret P., et al. (2018). Noise pollution limits metal bioaccumulation and growth rate in a filter feeder, the pacific oyster Magallana gigas. PloS One 13 (4), e0194174. doi: 10.1371/journal.pone.0194174
Charifi M., Sow M., Ciret P., Benomar S., Massabuau J. C. (2017). The sense of hearing in the pacific oyster, Magallana gigas. PloS One 12 (10), e0185353. doi: 10.1371/journal.pone.0185353
Clements J. C., Comeau L. A. (2019a). Nitrogen removal potential of shellfish aquaculture harvests in eastern Canada: A comparison of culture methods. Aquaculture Rep. 13, 100183. doi: 10.1016/j.aqrep.2019.100183
Clements J. C., Comeau L. A. (2019b). Use of high-frequency noninvasive electromagnetic biosensors to detect ocean acidification effects on shellfish behavior. J. Shellfish Res. 38, 811–818. doi: 10.2983/035.038.0330
Clements J. C., Hicks C., Tremblay R., Comeau L. A. (2018). Elevated seawater temperature, not pCO2, negatively affects post-spawning adult mussels (Mytilus edulis) under food limitation. Conserv. Physiol. 6 (1), cox078. doi: 10.1093/conphys/cox078
Clements J. C., Poirier L. A., Pérez F. F., Comeau L. A., Babarro J. M. (2020). Behavioural responses to predators in Mediterranean mussels (Mytilus galloprovincialis) are unaffected by elevated pCO2. Mar. Environ. Res. 161, 105148. doi: 10.1016/j.marenvres.2020.105148
Clements J. C., Ramesh K., Nysveen J., Dupont S., Jutfelt F. (2021). Animal size and sea water temperature, but not pH, influence a repeatable startle response behaviour in a wide-ranging marine mollusc. Anim. Behav. 173, 191–205. doi: 10.1016/j.anbehav.2020.12.008
Coffin M. R., Clements J. C., Comeau L. A., Guyondet T., Maillet M., Steeves L., et al. (2021). The killer within: Endogenous bacteria accelerate oyster mortality during sustained anoxia. Limnol. Oceanogr. 66 (7), 2885–2900. doi: 10.1002/lno.11798
Cordeiro N. I. S., Andrade J. T. M., Montresor L. C., Luz D. M. R., Araújo J. M., Martinez C. B., et al. (2017). Physiological response of invasive mussel Limnoperna fortunei (Dunke) (Bivalvia: Mytilidae) submitted to transport and experimental conditions. Braz. J. Biol. 77 (1), 191–198. doi: 10.1590/1519-6984.15315
Di Fiori E., Pizarro H., dos Santos Afonso M., Cataldo D. (2012). Impact of the invasive mussel Limnoperna fortunei on glyphosate concentration in water. Ecotoxicol. Environ. Saf. 81, 106–113. doi: 10.1016/j.ecoenv.2012.04.024
Dodd L. F., Caracappa J. C., Fegley S. R., Grabowski J. H., Piehler M. F. (2018). Threat of predation does not affect Crassostrea virginica filtration. Estuaries Coasts 41 (1), 293–298. doi: 10.1007/s12237-017-0269-3
Dowd W. W., Somero G. N. (2013). Behavior and survival of Mytilus congeners following episodes of elevated body temperature in air and seawater. J. Exp. Biol. 216 (3), 502–514. doi: 10.1242/jeb.076620
Doyle R., Kim J., Pe A., Blumstein D. T. (2020). Are giant clams (Tridacna maxima) distractible? a multi-modal study. PeerJ 8, e10050. doi: 10.7717/peerj.10050
Duarte C. M., Chapuis L., Collin S. P., Costa D. P., Devassy R. P., Eguiluz V. M., et al. (2021). The soundscape of the anthropocene ocean. Science 371 (6529), eaba4658. doi: 10.1126/science.aba4658
Encomio V., Chu F. L. E. (2000). The effect of PCBs on glycogen reserves in the eastern oyster Crassostrea virginica. Mar. Environ. Res. 50 (1-5), 45–49. doi: 10.1016/S0141-1136(00)00044-1
FAO (2020). The state of world fisheries and aquaculture 2020. sustainability in action (Rome: Food and Agriculture Organization of the United Nations).
Fewtrell J. L., McCauley R. D. (2012). Impact of air gun noise on the behaviour of marine fish and squid. Mar. pollut. Bull. 64 (5), 984–993. doi: 10.1016/j.marpolbul.2012.02.009
Firestone J., Jarvis C. (2007). Response and responsibility: Regulating noise pollution in the marine environment. J. Int. Wildlife Law Policy 10 (2), 109–152. doi: 10.1080/13880290701347408
Fox J., Weisberg S. (2019). An {R} companion to applied regression. 3rd ed. (Thousand Oaks, California: Sage publications).
Galler S., Litzlbauer J., Kröss M., Grassberger H. (2010). The highly efficient holding function of the mollusc “catch”muscle is not based on decelerated myosin head cross-bridge cycles. Proc. R. Soc. B: Biol. Sci. 277 (1682), 803–808. doi: 10.1098/rspb.2009.1618
Hegaret H., Wikfors G. H., Shumway S. E. (2007). Diverse feeding responses of five species of bivalve mollusc when exposed to three species of harmful algae. J. Shellfish Res. 26 (2), 549–559. doi: 10.2983/0730-8000(2007)26[549:DFROFS]2.0.CO;2
Hothorn T., Bretz F., Westfall P. (2008). Simultaneous inference in general parametric models. Biometrical J. 50, 346–363. doi: 10.1002/bimj.200810425
Johansson K. (2011). Impact of anthropogenic noise on fish behaviour and ecology. (Umeå Sweden: Swedish University of Agricultural Sciences. [research essay].
Jolivet A., Tremblay R., Olivier F., Gervaise C., Sonier R., Genard G., et al. (2016). Validation of trophic and anthropic underwater noise as settlement trigger in blue mussels. Sci. Rep. 6, 33829. doi: 10.1038/srep33829
Kastelein R. A. (2008). Effects of vibrations on the behaviour of cockles (bivalve molluscs). Bioacoustics 17, 1-3, 74–75. doi: 10.1080/09524622.2008.9753770
Keppler D., Decker K. (1974). “Glycogen – determination with amyloglucosidase,” in Methods of enzymatic analysis. Ed. Bergmeyer H. U. (New York, NY: Academic Press), 1127–1131.
Kuznetsova A., Brockhoff P. B., Christensen R. H. B. (2017). lmerTest package:Tests in linear mixed effects models. J. Stat. Software 82, 1e26. doi: 10.18637/jss.v082.i13
Lacoste É., Raimbault P., Harmelin-Vivien M., Gaertner-Mazouni N. (2016). Trophic relationships between the farmed pearl oyster Pinctada margaritifera and its epibionts revealed by stable isotopes and feeding experiments. Aquaculture Environ. Interact. 8, 55–66. doi: 10.3354/aei00157
Lillis A., Eggleston D. B., Bohnenstiehl D. R. (2013). Oyster larvae settle in response to habitat-associated underwater sounds. PloS One 8 (10), e79337. doi: 10.1371/journal.pone.0079337
McCarty R. (2016). “The fight-or-flight response: A cornerstone of stress research,” in Stress: Concepts, cognition, emotion, and behavior (San Diego: Academic Press), 33–37.
McCormick M. I., Allan B. J., Harding H., Simpson S. D. (2018). Boat noise impacts risk assessment in a coral reef fish but effects depend on engine type. Sci. Rep. 8 (1), 3847.
McCormick M. I., Fakan E. P., Nedelec S. L., Allan B. J. (2019). Effects of boat noise on fish fast-start escape response depend on engine type. Sci. Rep. 9 (1), 1–10. doi: 10.1038/s41598-019-43099-5
Nagai K., Honjo T., Go J., Yamashita H., Oh S. J. (2006). Detecting the shellfish killer Heterocapsa circularisquama (Dinophyceae) by measuring bivalve valve activity with a hall element sensor. Aquaculture 255 (1-4), 395–401. doi: 10.1016/j.aquaculture.2005.12.018
Nedelec S. L., Radford A. N., Simpson S. D., Nedelec B., Lecchini D., Mills S. C. (2014). Anthropogenic noise playback impairs embryonic development and increases mortality in a marine invertebrate. Sci. Rep. 4, 5891. doi: 10.1038/srep05891
Olivier F., Gigot M., Mathias D., Jezequel Y., Meziane T., L'Her C., et al. (2023). Assessing the impacts of anthropogenic sounds on early stages of benthic invertebrates: The “Larvosonic system”. Limnol. Oceanogr.: Methods 21, 53–68. doi: 10.1002/lom3.10527
Palais F., Mouneyrac C., Dedourge-Geffard O., Giamberini L., Biagianti-Risbourg S., Geffard A. (2011). One-year monitoring of reproductive and energy reserve cycles in transplanted zebra mussels (Dreissena polymorpha). Chemosphere 83, 1062–1073. doi: 10.1016/j.chemosphere.2011.01.060
Peng C., Xinguo Z., Guangxu L. (2015). Noise in the sea and its impacts on marine organisms. Int. J. Environ. Res. Public Health 12.10, 12304–12323. doi: 10.3390/ijerph121012304
Peng C., Zhao X., Liu S., Shi W., Guo C., Jiang J., et al. (2016). Effects of anthropogenic sound on digging behavior, metabolism, Ca2+/Mg2+ ATPase activity, and metabolism-related gene expression of the bivalve Sinonovacula constricta. Sci. Rep. 6, 24266. doi: 10.1038/srep24266
Plaistow S. J., Troussard J. P., Cézilly F. (2001). The effect of the acanthocephalan parasite Pomphorhynchus laevis on the lipid and glycogen content of its intermediate host Gammarus pulex. Int. J. Parasitol. 31 (4), 346–351. doi: 10.1016/S0020-7519(01)00115-1
Popper A. N., Hawkins A. (Eds.) (2016). The effects of noise on aquatic life II (New York, NY: Springer), 1292.
Porter E. T., Breitburg D. L. (2016). Eastern Oyster, Crassostrea virginica, valve gape behavior under diel-cycling hypoxia. Mar. Biol. 163 (10), 218. doi: 10.1007/s00227-016-2980-1
Pourmozaffar S., Tamadoni Jahromi S., Rameshi H., Sadeghi A., Bagheri T., Behzadi S., et al. (2019). The role of salinity in physiological responses of bivalves. Rev. Aquaculture 12 (3), 1548–1566. doi: 10.1111/raq.12397
R Core Team (2020). R: A language and environment for statistical computing. R Foundation Stat. Computing.
Roberts L., Cheesman S., Breithaupt T., Elliott M. (2015). Sensitivity of the mussel Mytilus edulis to substrate-borne vibration in relation to anthropogenically generated noise. Mar. Ecol. Prog. Ser. 538, 185–195. doi: 10.3354/meps11468
Sampaio F. D., Freire C. A. (2016). An overview of stress physiology of fish transport: Changes in water quality as a function of transport duration. Fish Fisheries 17 (4), 1055–1072. doi: 10.1111/faf.12158
Smee D. L., Weissburg M. J. (2006). Clamming up: Environmental forces diminish the perceptive ability of bivalve prey. Ecology 87 (6), 1587–1598. doi: 10.1890/0012-9658(2006)87[1587:CUEFDT]2.0.CO;2
Solé M., Kaifu K., Mooney T. A., Nedelec S. L., Olivier F. (2023). Marine invertebrates and noise. Front. Mar. Sci. 10:1129057. doi: 10.3389/fmars.2023.1129057
Sonawane S. M., Sonawane M. (2018). Effect of heavy metals Hgcl2 and Cdcl2 on glycogen activity of bivalve Lamellidens marginalis. IOSR J. Pharm. 8 (8), 28–35.
Tang B., Riisgård H. U. (2016). Physiological regulation of valve-opening degree enables mussels Mytilus edulis to overcome starvation periods by reducing the oxygen uptake. Open J. Mar. Sci. 6 (03), 341. doi: 10.4236/ojms.2016.63029
Tran D., Nadau A., Durrieu G., Ciret P., Parisot J. P., Massabuau J. C. (2011). Field chronobiology of a molluscan bivalve: How the moon and sun cycles interact to drive oyster activity rhythms. Chronobiol. Int. 28 (4), 307–317. doi: 10.3109/07420528.2011.565897
Tran D., Sow M., Camus L., Ciret P., Berge J., Massabuau J. C. (2016). In the darkness of the polar night, scallops keep on a steady rhythm. Sci. Rep. 6 (1), 1–9. doi: 10.1038/srep32435
Tyack P. L. (2008). Implications for marine mammals of large-scale changes in the marine acoustic environment. J. Mammalogy 89 (3), 549–558. doi: 10.1644/07-MAMM-S-307R.1
Van der Schatte Olivier A., Jones L., Le Vay L., Christie M., Wilson J., Malham S. K. (2020). A global review of the ecosystem services provided by bivalve aquaculture. reviews in. Aquaculture 12, 3–25. doi: 10.1111/raq.12301
Vazzana M., Celi M., Maricchiolo G., Genovese L., Corrias V., Quinci E. M., et al. (2016). Are mussels able to distinguish underwater sounds? assessment of the reactions of Mytilus galloprovincialis after exposure to lab-generated acoustic signals. Comp. Biochem. Physiol. Part A: Mol. Integr. Physiol. 201, 61–70. doi: 10.1016/j.cbpa.2016.06.029
Vinagre C., Madeira D., Narciso L., Cabral H. N., Diniz M. (2012). Effect of temperature on oxidative stress in fish: lipid peroxidation and catalase activity in the muscle of juvenile seabass, Dicentrarchus labrax. Ecol. Indic. 23, 274–279. doi: 10.1016/j.ecolind.2012.04.009
Wale M. A., Briers R. A., Hartl M. G., Bryson D., Diele K. (2019). From DNA to ecological performance: Effects of anthropogenic noise on a reef-building mussel. Sci. Total Environ. 689, 126–132. doi: 10.1016/j.scitotenv.2019.06.380
Weber A., von Randow M., Voigt A. L., von der Au M., Fischer E., Meermann B., et al. (2020). Ingestion and toxicity of microplastics in the freshwater gastropod Lymnaea stagnalis: no microplastic-induced effects alone or in combination with copper. Chemosphere 263, 128040. doi: 10.1016/j.chemosphere.2020.128040
Widdows J. (1985). Physiological procedures. In Bayne B. L., editor. The effects of stress and pollution on marine animals. (New York, NY: Praeger Press), pp. 161–178.
Williams K. J., Cassidy A. A., Verhille C. E., Lamarre S. G., MacCormack T. J. (2019). Diel cycling hypoxia enhances hypoxia tolerance in rainbow trout (Oncorhynchus mykiss): evidence of physiological and metabolic plasticity. J. Exp. Biol. 222 (14), jeb206045. doi: 10.1242/jeb.206045
Williams R., Wright A. J., Ashe E., Blight L. K., Bruintjes R., Canessa R., et al. (2015). Impacts of anthropogenic noise on marine life: Publication patterns, new discoveries, and future directions in research and management. Ocean Coast. Manage. 115, 17–24. doi: 10.1016/j.ocecoaman.2015.05.021
Woodin S. A., Wethey D. S., Olabarria C., Vazquez E., Dominguez R., Macho G., et al. (2020). Behavioral responses of three venerid bivalves to fluctuating salinity stress. J. Exp. Mar. Biol. Ecol. 522, 151256. doi: 10.1016/j.jembe.2019.151256
Keywords: animal behavior, coastal ecosystem, energetic physiology, environmental stressors, global change biology, noise pollution
Citation: Ledoux T, Clements JC, Comeau LA, Cervello G, Tremblay R, Olivier F, Chauvaud L, Bernier RY and Lamarre SG (2023) Effects of anthropogenic sounds on the behavior and physiology of the Eastern oyster (Crassostrea virginica). Front. Mar. Sci. 10:1104526. doi: 10.3389/fmars.2023.1104526
Received: 21 November 2022; Accepted: 01 March 2023;
Published: 16 March 2023.
Edited by:
Marta Solé, Universitat Politècnica de Catalunya, BarcelonaTech (UPC), SpainReviewed by:
John Carroll, Georgia Southern University, United StatesCopyright © 2023 Ledoux, Clements, Comeau, Cervello, Tremblay, Olivier, Chauvaud, Bernier and Lamarre. This is an open-access article distributed under the terms of the Creative Commons Attribution License (CC BY). The use, distribution or reproduction in other forums is permitted, provided the original author(s) and the copyright owner(s) are credited and that the original publication in this journal is cited, in accordance with accepted academic practice. No use, distribution or reproduction is permitted which does not comply with these terms.
*Correspondence: Tamara Ledoux, ZXRsMDkyNEB1bW9uY3Rvbi5jYQ==
Disclaimer: All claims expressed in this article are solely those of the authors and do not necessarily represent those of their affiliated organizations, or those of the publisher, the editors and the reviewers. Any product that may be evaluated in this article or claim that may be made by its manufacturer is not guaranteed or endorsed by the publisher.
Research integrity at Frontiers
Learn more about the work of our research integrity team to safeguard the quality of each article we publish.