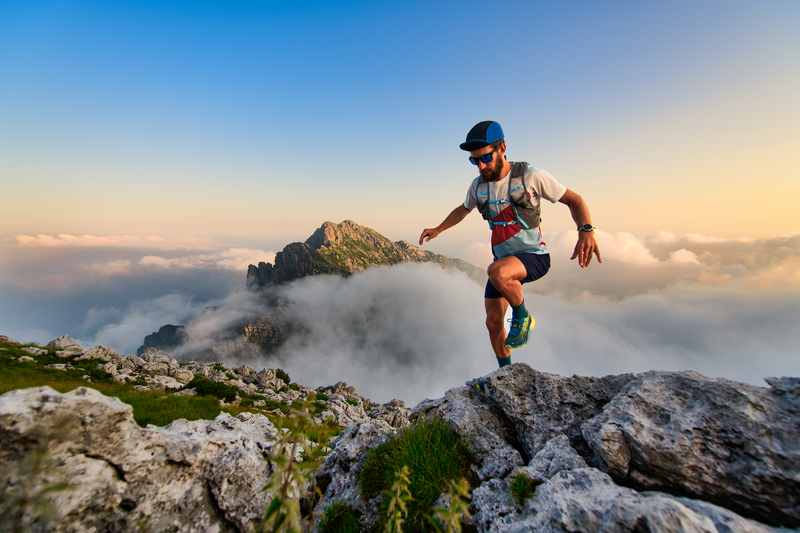
95% of researchers rate our articles as excellent or good
Learn more about the work of our research integrity team to safeguard the quality of each article we publish.
Find out more
ORIGINAL RESEARCH article
Front. Mar. Sci. , 17 February 2023
Sec. Marine Biology
Volume 10 - 2023 | https://doi.org/10.3389/fmars.2023.1095762
In the Seto Inland Sea, the largest semi-enclosed sea in Japan, the most dominant diatom in the past, Skeletonema spp., has been replaced by another diatom Chaetoceros spp. since the 1980s, and this shift is often explained as the result of oligotrophication. Based on previous observations of a shift from Skeletonema spp. to Chaetoceros spp. under prolonged sunny conditions, the recent increase in solar insolation over the last 30 years might have also accelerated the replacement of Skeletonema by Chaetoceros, especially during the summer when nutrient levels are relatively low and solar insolation is high. In our experiments, culture strains of Skeletonema costatum and Chaetoceros lorenzianus under severely nitrogen-limited conditions exhibited less non-photochemical quenching (NPQ) under prolonged exposure (1 h) to high light (800 µmol-photons m-2 s-1) and a decrease in photochemical quenching (qP) which was especially notable in S. costatum. Conversely, marked increases in NPQ were observed under severely phosphorus-limited conditions, even under short time exposure (30 s) to high light, even though the increase in NPQ could not relieve the decrease in qP, which was more apparent in S. costatum. These trends in NPQ and qP were attributed to the limited nutrients because replenishment of the nutrients led to a decrease in NPQ and an increase in qP. Interestingly, this recovery was faster in C. lorenzianus than S. costatum. The results showed that phosphorus depletion caused severe photoinhibition especially in S. costatum, irrespective of active NPQ induction. Further, given the severe phosphorus-limited conditions in the Seto Inland Sea for an extended period, we conducted competition experiments using continuous coculture of both species to simulate the typical summer environment where severe phosphorus limitation and high light occur. The results showed that the shift from S. costatum to C. lorenzianus was accelerated by continuous exposure to high light, which could explain the recent shift in the dominant species in the summer in the study area.
Phytoplankton, or single-cell algae, are primary producers in aquatic food webs and form the foundation of the marine ecosystem. Among them, diatoms are commonly found groups that are most important primary producers in the sea (Sumper and Brunner, 2006). Since the mid-1980s, the dominant diatom species in the Seto Inland Sea, which is the largest semi-enclosed sea in Japan, has shifted from Skeletonema spp. to Chaetoceros spp. (Itakura and Yamaguchi, 2007; Nishikawa et al., 2010; Imai et al., 2015). It is commonly considered that Skeletonema spp. generally prefer nutrient-rich coastal areas while Chaetoceros spp. are more common in areas with relatively low nutrient levels (Yamada et al., 1980a; Yamada et al., 1980b; Yamada et al., 1982). The shift from Skeletonema spp. to Chaetoceros spp. in the Seto Inland Sea is considered to be due to oligotrophication of the sea (Itakura and Yamaguchi, 2007; Nishikawa et al., 2010; Tada et al., 2014; Imai et al., 2015); indeed, levels of total phosphorus (P) and total nitrogen (N) decreased markedly in the early 1980s due to the promulgation of a strict sewage law in the late 1970s (Abo and Yamamoto, 2019).
However, the nutrient requirements of relatively small phytoplankton species (e.g., Skeletonema spp.) are generally lower than those of larger species (e.g., Chaetoceros spp.) (Harrison et al., 1977; Bienfang et al., 1982; Pan et al., 2010). Due to its low nutrient requirements, S. costatum can grow relatively fast under nutrient deficient conditions (Nishijima and Fukami, 1993), which is advantageous in oligotrophic environments. There is a marked discrepancy between field observations, in which senescence of Skeletonema spp. has been observed in response to oligotrophication, and experimental results in which fast growth has been observed under nutrient deficient conditions. These findings imply that environmental factors other than oligotrophication and nutrient levels negatively affect the growth of Skeletonema and the shift between species.
One of these factors might be a change in the photic conditions in the sea. Field observations by Ohara et al. (2020) showed that, while Chaetoceros spp. were typically more abundant than Skeletonema spp. in the center of the sea in summer, Skeletonema spp. occasionally formed blooms when sea surface light levels were less than the annual average of the sampling period (2014–2017). The amount of solar radiation reaching the ground has increased in western Japan over the last 30 years due to domestic vehicle emission controls (Chirifu, 2012) and/or anthropogenic emission reductions (almost 10%/y), such as the reduction in PM2.5 in northern China (Uno et al., 2017). In addition, a recent increase in the transparency of sea water (Tarutani, 2007; Abo et al., 2018) may have produced a situation in which light can penetrate deeper into the sea.
Excess light is harmful to any plant as it can decrease photosynthesis (Demmig-Adams and Adams III, 1992) and can accelerate photoinhibition under nutrient stressed conditions (Lu and Zhang, 2000; Huang et al., 2004). If the photosynthesis of Skeletonema spp. is adversely affected by the combination of oligotrophic and high light conditions, both of which are characteristics of the Seto Inland Sea in summer, then Skeletonema spp. may be outcompeted by Chaetoceros spp. To test this hypothesis, we compared photoinhibition tolerances under nutrient-limited conditions in Skeletonema costatum and Chaetoceros lorenzianus, with a focus on non-photochemical quenching (NPQ).
Of the various photoprotective mechanisms employed by plants and algae, NPQ is considered to be one of the most important (Horton and Ruban, 2005; Jahns and Holzwarth, 2012; Niyogi and Truong, 2013; Goss and Lepetit, 2015). In studies on the photoinhibition and photoprotective mechanisms of plants, NPQ increased to protect the photosystems at high levels of nutrient stress and irradiance (Khamis et al., 1990; Lu and Zhang, 2000; Cheng, 2003). Similar increases in NPQ under nutrient-limited conditions have been reported in the diatoms Phaeodactylum tricornutum (Taddei et al., 2016; Wagner et al., 2016; Huang et al., 2019), Thalassiosira pseudonana, T. weissflogii (Liu et al., 2011; Liefer et al., 2018) and T. punctigera (Li et al., 2021); however, no information is currently available for the genera investigated in this study. Based on the assumption that the recent shift from Skeletonema spp. to Chaetoceros spp. may be linked to oligotrophication and increased insolation, we investigated the photosynthetic responses of both genera under the following N- and P-limited conditions: 1) monitoring quenching parameters under different levels of N or P limitation in chemostat cultures (continuous cultures) was performed to assess taxon-specific responses to various levels of nutrient limitation; 2) S. costatum and C. lorenzianus were cocultured in chemostat cultures to simulate the typical summer environment where severe P limitation and high light occur, and transient changes in the composition of both species were monitored.
Clonal strains of Skeletonema costatum sensu stricto (Greville) Cleve and Chaetoceros lorenzianus Grunow, originally isolated from the Seto Inland Sea of Japan were used. The strains were maintained in f/2 medium (Guillard, 1975), with Na2SeO3 (final 1.0 × 10-8 M) and hydroxymethyl aminomethane (final 2 mM) additions. The strains were incubated at 20°C under a photon flux density (PFD) of 150 µmol-photons m-2 s-1 (L:D=12:12 h) provided by a cool-white tube-type light-emitting diode (LED) light source.
Continuous cultures were used to obtain cells in which only one of the target nutrients (N or P) was depleted. In continuous culture, new medium is supplied continuously to a culture vessel, and the same amount of culture medium is removed (chemostat culture, Ukeles, 1973). The dilution rate in the culture vessel can be calculated as the daily outflow volume relative to the total volume of the culture vessel. For this purpose, 1 L custom-made cylindrical vessels with a side drainage port were fabricated (Supplementary Figure 1). Teflon tubes (inner diameter = 2 mm) were inserted into a silicon plug that was placed in the vessel mouth, and one of these tubes was connected to a tubing pump (SMP-23AS, AS ONE, Japan) to supply medium. Another Teflon tube was used to obtain samples for the experiment. Air was supplied from the conical bottom of the vessel to mix the culture. A side drainage port was used to remove excess medium and pressurized air. For each diatom species, four continuous cultures (i.e., N- and P-limited conditions at two different dilution rates), were established; each diatom species was subjected to continuous culture at a high dilution rate (HDR: 0.44–0.54 day-1) and a low dilution rate (LDR: 0.13–0.16 day-1) under N- and P-limited conditions. Experiments were performed at 20°C under a PFD of 150 µmol-photons m-2 s-1 (L:D=12:12 h).
In the principle of our continuous culture experiment, a culture was maintained initially as batch culture until when the target nutrient was depleted (approx. for 2 weeks), then the continuous culture experiment was started by supplying new N- or P-limited f/2 medium. For the initial batch cultures, N-limited f/2 medium with a 1/10 NaNO3 concentration (final 88.3 µM) or P-limited f/2 medium with a 1/10 NaH2PO4 concentration (final 3.6 µM) was initially placed in the culture vessels, which were then inoculated with S. costatum or C. lorenzianus to give initial cell densities of 1,000 cells mL-1 and 500 cells mL-1, respectively. This density ratio in the precultures was determined based on a previous study in nutrient uptake ratios and cell volumes of S. costatum and Chaetoceros gracile (Bienfang et al., 1982); nutrient uptake of C. gracile, whose cell volume was about three times larger than S. costatum, was 1.3–1.5 times faster than S. costatum. The mean cell volume of the C. lorenzianus culture strain in this study was about four times larger than the S. costatum culture strain (234.25 ± 74.99 µm3 (n = 32) and 946.56 ± 471.78 µm3 (n = 32), respectively), suggesting that nutrient uptake of C. lorenzianus was faster than C. gracile. The cultures were maintained as batch cultures for 2 weeks without supplying new medium. Upon entering the beginning of the stationary growth phase when the target nutrient was assumed to be totally depleted, the continuous culture experiments were started by supplying new N- or P-limited f/2 medium continuously at the two dilution rates mentioned above. An aliquot of the culture was retrieved from the Teflon tube using a syringe, and cell density, residual inorganic nutrients and photosynthetic parameters were measured daily. The cell densities were measured by counting under a microscope using a plankton counting chamber (MPC-200, Matsunami Glass, Japan). To avoid counting dead cells, live cell staining was conducted by adding neutral red solution (Sukisaki and Umino, 2013). Residual nitrogen (dissolved inorganic nitrogen (DIN: NO3 + NO2 + NH4-N)) and phosphorus (dissolved inorganic phosphorus (DIP: PO4-P)) were analyzed using a nutrient autoanalyzer (SWAAT, BLTEC, Japan). To prevent nutrient contamination, all instruments made of glass or plastic were soaked overnight in 1 N hydrochloric acid and then washed with MilliQ before use. Based on the constant cell densities and complete depletions of residual nutrients, cultures were assumed to have reached a steady state (balanced growth phase) at day 12, when the specimens were subjected to the experiments described below.
Before subjecting the continuous cultures to the prolonged high light exposure experiments, the photosynthetic parameters, Fv/Fm (i.e., maximum quantum yield of dark-adapted photosystem II) and quenching parameters were initially measured during rapid light curve measurements (Ralph and Gademann, 2005) using cells retrieved at day 12 using a pulse amplitude modulation (PAM) fluorometer (WATER-PAM, Heinz Walz, Germany). The measurements were performed in triplicate. Then, 1.2 mL of the culture that had been dark-adapted for more than 15 min was transferred to a cuvette and illuminated with eight increasing intensities of actinic lights (i.e., PFD = 158, 241, 356, 553, 825, 1179, 1648, 2743 µmol-photons m-2 s-1) for 30 s. The quenching parameters qP and NPQ were obtained at each PFD level using software (WinControl-3, Heinz Walz), and Fv/Fm, qP and NPQ (Stern-Volmer NPQ) were estimated using the following equations (Kitajima and Butler, 1975; Schreiber et al., 1986; Bilger and Björkman, 1990):
where, Fm is maximum fluorescence yield after dark acclimation; Fo is minimum fluorescence yield after dark acclimation; Fm’ is maximum fluorescence yield in light-acclimated state; F’ is variable basal fluorescence under actinic light; Fo’ is minimum fluorescence yield in the light-acclimated state. After measuring the parameters mentioned above, each 80 mL aliquot was separately dispensed into six 270-mL tissue culture flasks (Canted Neck, IWAKI, Japan) for eight different specimen materials (i.e., N- and P-depleted samples × two dilution rates × two species) (Supplementary Figure 2). Among the six flasks, three were for observing the recovery responses after each nutrient was replenished, i.e., an NH4Cl solution (final 100 µM) or a NaH2PO4 solution (final 10 µM) was added to the three flasks for the N- or P-depleted specimens, respectively. The other three flasks were used as controls. All of the flasks were incubated in the dark for 15 min to measure Fo and Fm. The samples were then transferred to a water bath and incubated at 20°C. Half of the bath was illuminated from the bottom with low light (LL: 50 µmol-photons m-2 s-1), and the remaining half was illuminated with high light (HL: 800 µmol-photons m-2 s-1). This high light intensity was determined based on field data obtained at the sea surface at noon on a sunny day (approx. 750 µmol-photons m-2 s-1 on average). To determine the initial values of qP and NPQ before HL exposure, the flasks were initially maintained under LL exposure for 60 min, followed by HL exposure for 60 min, and then under LL exposure for another 60 min to observe the recovery from HL-induced stress. To measure qP and NPQ, sample aliquots of approximately 5 mL were retrieved from the flask at 30 min and 60 min during the initial LL incubation step, and then at 15-min intervals during the HL and the final LL exposure steps. A 1.2 mL aliquot was subjected to PAM fluorometry analysis.
To simulate the summer season in our study area, S. costatum and C. lorenzianus were mixed in a P-limited continuous culture, and shifts in the relative abundance of the two species under two different light levels was performed in triplicate. The two diatom species were initially precultured separately in batch culture for 2 weeks at 25°C under a PFD of 150 µmol-photons m-2 s-1 (L:D=12:12 h); the initial cell densities were the same as in the above experiment, i.e., 1,000 cells mL-1 for S. costatum and 500 cells mL-1 for C. lorenzianus, respectively. After the 2 weeks, the cultures were subjected to semicontinuous culture for 1 week; 10% (volume) of the culture was replaced daily with new f/2 medium in order to minimize the likelihood of differences in nutrient stress, possibly due to differences in growth and/or nutrient consumption rates between species. Then, 150 mL of the precultured diatoms were mixed and transferred to a total of six continuous culture vessels. The vessels were filled to 1 L with P-limited f/2 medium, and new medium was supplied at a dilution rate of 0.21 day-1. Then, three of the six vessels were illuminated with moderate light (ML: PFD = 150 µmol-photons m-2 s-1), and the other three were illuminated with high light (HL: PFD = 800 µmol-photons m-2 s-1). Residual inorganic nutrients, i.e., DIP and DIN were measured daily for the first 5 days and then once every two days thereafter. Cell densities and photosynthetic parameters were measured at day 1, 2, 4, 7, 9, 11, 13 and 15. Cell density measurements were performed as described in the “2.2 Continuous culture” section. To measure the photosynthetic parameters Fv/Fm and NPQ for each species, the cells from the cultures were placed on a glass slide with a cover slip and measurements were conducted by a microscopy-type PAM fluorometer (Micro-FluorCam FC-2000, Photon Systems Instruments, Czech Republic) as described in Higo et al. (2017). To obtain NPQ, light levels corresponding to ML or HL were supplied using the transmission light of the microscope for 1 min. Under this illumination, a fluorescence-induction curve was plotted with saturation pulses (five times) using the Quenching Analysis Wizard of the PAM software (FluorCam 7 ver. 1.2.5.24, Photon Systems Instruments). At least 15 colonies of each species were measured.
In this study, the prolonged high light exposure experiment and the competition experiment were conducted in triplicate. The average parameters were obtained from the prolonged high light exposure experiments, i.e., qP and NPQ, and the competition experiments i.e., cell density, Fv/Fm and NPQ. The above data were subjected to statistical analysis using Student’s t-test in R 4.2.2 (R Development Core Team, 2022). In this study, a p-value less than 0.01 was considered statistically significant.
Concentrations of the target nutrients decreased over time in the continuous cultures and reached almost zero. Excess amounts of a nontarget nutrient, DIP for N-limited conditions and DIN for P-limited conditions, were observed, even after the target nutrients had been depleted (Figure 1). According to the principle of continuous culture, cells grow in accordance with the nutrient supply, (i.e., dilution rate) and typically maintain a constant density (Ukeles, 1973), which is called a balanced growth phase. Although fluctuations in cell densities were observed, those in S. costatum under N-limited conditions appeared to be constant after 5 days at a HDR (0.52 day-1) and after 7 days at a LDR (0.13 day-1) (Figure 1A). In the case of C. lorenzianus, the cell densities under N-limited conditions were constant after 8 days at a HDR (0.44 day-1) and a LDR (0.16 day-1) (Figure 1B). Under P-limited conditions, cell densities of S. costatum were constant after 7 days at a HDR (0.54 day-1) and a LDR (0.14 day-1) (Figure 1C). Likewise, cell densities of C. lorenzianus under P-limited conditions at 11 days at a HDR (0.46 day-1) and 9 days at a LDR (0.13 day-1) were also constant (Figure 1D). To supply acclimated cells for given nutrient-limited conditions to the next experiment, cultures at day 12 were considered suitable because those at day 12 assumed to be under a balanced growth phase. At day 12, under both N- and P-limited conditions, the cell densities of S. costatum at both dilution rates were nearly similar (Figures 1A, C); c.a. 4.7 × 105 at the HDR versus c.a. 3.1 × 105 at the LDR for N limitation and c.a. 2.0 × 105 at the HDR versus c.a. 2.1 × 105 at the LDR for P limitation. On the other hand, the cell densities of C. lorenzianus in the LDR treatments were one order of magnitude lower than those in the HDR treatments (Figures 1B, D); c.a. 1.5 × 105 at the HDR versus c.a. 5.2 × 104 at the LDR for N limitation and c.a. 3.3 × 104 at the HDR versus c.a. 5.7 × 103 at the LDR for P limitation. The cell densities of both species under the P-limited conditions were lower than those under the N-limited conditions. Significantly lower Fv/Fm values – less than 0.5 in the LDR treatment – were observed in both N- and P-limited S. costatum cultures (0.47 for N limitation and 0.39 for P limitation, respectively) and P-limited C. lorenzianus cultures (0.42). In the case of N-limited C. lorenzianus cultures, Fv/Fm values were as high as 0.65 in the LDR treatment. Compared to the LDR treatments, the Fv/Fm values in the HDR treatments in both species were higher than 0.55 under both N- and P-limited conditions.
Figure 1 Residual nutrients (DIN: dissolved inorganic nitrogen, DIP: dissolved inorganic phosphorus) and cell density during continuous culture in an N-limited S. costatum culture (A), N-limited C. lorenzianus culture (B), P-limited S. costatum culture (C) and P-limited C. lorenzianus culture (D). HDR, high dilution rate; LDR, low dilution rate.
From the rapid light curve measurements on day 12, the values of qP and NPQ after exposure to moderate light intensity (ML: 158 µmol-photons m-2 s-1) and high light intensity (HL: 825 µmol-photons m-2 s-1) were estimated and are shown in Figure 2. Under N limitation, both species exhibited higher qP values under ML exposure, even in the LDR treatment (Figure 2A, 0.84 for S. costatum and 0.88 for C. lorenzianus, respectively). Relatively higher qP values were observed in C. lorenzianus in the LDR treatment, even under HL exposure (0.71), while those in S. costatum decreased to 0.56. Under such N-limited conditions, the NPQ values of S. costatum increased to almost the same levels for each light intensity, regardless of the dilution rate (Figure 2C). Unlike in S. costatum, the NPQ values observed in C. lorenzianus showed almost no increase under ML exposure at either dilution rate. Indeed, the values under HL even remained as low as 0.09 in the HDR treatment and 0.08 in the LDR treatment. Under P limitation, even under the ML, lower qP values were observed at a LDR compared with a HDR in both species (Figure 2B; 0.73 to 0.56 for S. costatum and 0.82 to 0.66 for C. lorenzianus, respectively). Decreases in qP were more obvious under HL exposure, with values decreasing from the HDR treatment to the LDR treatment; specifically, from 0.46 to 0.11 in S. costatum and from 0.66 to 0.33 in C. lorenzianus. As in N-limited conditions, increases were also observed in NPQ in S. costatum, although the changes were more marked (Figure 2D). In both species, the NPQ values were high in the LDR treatment, especially under HL exposure. For example, NPQ values were 1.38 in S. costatum and 0.35 in C. lorenzianus.
Figure 2 Estimated values of qP (A, B) and NPQ (C, D) inferred from rapid light curve measurements. Pale bars indicate parameters under moderate light intensity (ML: 158 µmol-photons m-2 s-1) and dark bars indicate the parameters at a high light intensity (HL: 825 µmol-photons m-2 s-1). For each species, paired bar graphs show the results of dilution rate experiments, with the two bars on the left showing the results obtained for the high dilution rate (HDR: 0.44–0.54 day-1) and the two bars on the right showing the results of the low dilution rate (LDR: 0.13–0.16 day-1).
Figure 3 shows the combined effect of nutrient depletion and prolonged exposure (60 min) to high light (HL: 800 µmol-photons m-2 s-1), and with reintroduction of the depleted nutrients. The qP values observed in this experiment were higher than those in Figure 2, probably due to the recovery of qP after NPQ induction until the first measurement (15 min after starting HL exposure), implying the existence of some form of feedback after NPQ induction.
Figure 3 Transients of qP (upper) and NPQ (lower) in N-limited S. costatum (A, B), N-limited C. lorenzianus (C, D), P-limited S. costatum (E, F) and P-limited C. lorenzianus (G, H). Low light exposure is represented in gray bars (LL: 50 µmol-photons m-2 s-1) and high light exposure is represented in white bars (HL: 800 µmol-photons m-2 s-1). HDR, high dilution rate; LDR, low dilution rate. Values are averages ± standard division (SD) for triplicated experiments (average ± SD, n = 3).
Under N-limited conditions, S. costatum showed a decrease in qP values under HL, and in the controls, the lowest qP value in the LDR treatment (0.83 ± 0.12) was slightly lower than that in the HDR treatment (0.87 ± 0.01) (Figures 3A, B). During these phases, the maximum value of NPQ under HL exposure in the HDR treatment (0.96 ± 0.05) was twice that in the LDR treatment (0.54 ± 0.04). The addition of NH4Cl to these N-depleted cultures did not cause a drastic improvement in NPQ; for example, the maximum NPQ value under HL exposure was not significantly different between the control and samples supplemented with NH4Cl at both dilution rates (p = 0.12 in the HDR treatment and p = 0.54 in the LDR treatment, respectively). These findings, i.e., lower NPQ induction in the LDR treatments, were also observed in C. lorenzianus where the maximum NPQ value at the HDR (Figure 3C, 0.88 ± 0.12) was twice that at the LDR (Figure 3D, 0.47 ± 0.02). At that time, relatively higher qP values were maintained during HL exposure (Figures 3C, D). While a marked increase in NPQ was not observed in C. lorenzianus in Figure 2, NPQ was significantly induced in C. lorenzianus under prolonged HL exposure. In contrast to S. costatum, the addition of NH4Cl caused a significant decreased in the NPQ in C. lorenzianus; the maximum NPQ values in samples supplemented with NH4Cl decreased significantly and compared to the NPQ values in the controls in the HDR treatment (0.88 ± 0.12 to 0.47 ± 0.02, p = 0.005). In the LDR treatment, the maximum NPQ values in samples supplemented with NH4Cl were also lower than those in the controls, although statistical support was weak (0.47 ± 0.10 to 0.26 ± 0.07, p = 0.04).
Under P-limited conditions, qP decreased markedly under HL exposure in both species (Figures 3E–H); these findings are similar to the results shown in Figure 2B. In the case of S. costatum, the lowest qP value in the controls of the LDR treatment (0.70 ± 0.09) was lower than that in the HDR treatment (0.89 ± 0.09). In C. lorenzianus, the decrease in qP values was less marked between treatments; while the lowest qP value in the controls of the HDR treatment was 0.83 ± 0.02, that in the LDR treatment was 0.74 ± 0.13. Similar to the results obtained for the rapid light curve measurements (Figure 2D), under HL exposure, NPQ values increased more in the LDR treatments in both species compared with those in the HDR treatments; the maximum NPQ in the LDR (Figure 3F, 0.79 ± 0.07) was 3.3 times that at the HDR (Figure 3E, 0.24 ± 0.02) for S. costatum, and the NPQ value at the LDR (Figure 3H, 1.52 ± 0.03) under HL exposure was 4.9 times that at the HDR (Figure 3G, 0.31 ± 0.08) for C. lorenzianus. As in the case of N limitation, while the addition of NaH2PO4 did not significantly alter NPQ induction in S. costatum at either dilution rate, the addition of NaH2PO4 resulted in a marked recovery in qP levels and a decrease in NPQ values, especially at the LDR treatments in C. lorenzianus (Figure 3H); while there was no statistical difference, the minimum qP values under HL recovered compared with those in the control (0.74 ± 0.13 in the control, 0.92 ± 0.06 with the addition of NaH2PO4, p = 0.09) and the maximum NPQ values with the addition of NaH2PO4 were significantly lower than those of the control (1.52 ± 0.03 in the control, 0.60 ± 0.08 with the addition of NaH2PO4, p = 4.2 × 10-5). In both species, NPQ induction in the LDR treatments was still observed under LL exposure after the HL exposure, even with the addition of NaH2PO4 (Figures 3F, H).
To simulate the combination of P limitation and high light conditions, which appears to affect S. costatum negatively and has a limited effect on C. lorenzianus, an experiment consisting of a co-mixed culture of both species in a single continuous culture vessel was conducted in triplicate. As in the single-species continuous experiments above, the levels of the target nutrient, i.e., DIP, were totally depleted after day 5 or 3 under moderate light (ML: 150 µmol-photons m-2 s-1) (Figure 4A) or high light (HL: 800 µmol-photons m-2 s-1) (Figure 4B), respectively, while the DIN levels remained constant. Under ML conditions, both species showed gradual increases in cell density until day 4, when the cell density of S. costatum was higher than that of C. lorenzianus (Figure 4C). Similar trends were observed under HL conditions (Figure 4D). While C. lorenzianus maintained almost constant densities from day 4 to day 15 under both light conditions, the cell densities of S. costatum decreased 10-fold under ML conditions and decreased suddenly by 100-fold under HL conditions on day 7 from the values on day 4. Under ML conditions, S. costatum showed a gradual decrease in cell density and finally disappeared on day 15. Under HL conditions, S. costatum disappeared at day 9.
Figure 4 Residual nutrients and cell density under each light condition in competition experiments. Dissolved inorganic nitrogen (DIN) and dissolved inorganic phosphorus (DIP) are shown in the top graphs (A, B) and cell densities were shown in the bottom graphs (C, D). Values are averages ± standard division (SD) of three treatments.
Because stationary-phase cultures were used prior to the start of the continuous culture experiments, the cellular Fv/Fm values on day 1 for both species were relatively low, with the values of S. costatum being higher than those of C. lorenzianus (Figures 5A, B). On day 2, under both light conditions, Fv/Fm values increased gradually as the cell density increased at the start of the continuous culture. While there was no significant difference between in the Fv/Fm values obtained for each species on day 2 under both ML (p = 0.09) and HL (p = 0.05) conditions, the cellular Fv/Fm values of C. lorenzianus were significantly higher than those of S. costatum on day 4 under both light conditions (p = 0.001 under ML conditions and p = 2.8 × 10-14 under HL conditions, respectively). After day 7, Fo was too low to determine Fv/Fm and thus the values for S. costatum were treated as being below the detection limit. However, C. lorenzianus exhibited relatively higher Fv/Fm values on day 7 (0.57 ± 0.06 under ML conditions and 0.55 ± 0.09 under HL conditions, respectively), which then decreased slightly at day 15 (0.51 ± 0.60 under ML condition and 0.45 ± 0.60 under HL condition). NPQ values in S. costatum were higher under both light levels, and were higher than those of C. lorenzianus on day 1 (Figures 5C, D). On day 2, under ML conditions, NPQ values in C. lorenzianus increased while those in S. costatum remained similar to those on day 1 (0.49 ± 0.34 on day1 and 0.45 ± 0.34 day 2, respectively). Conversely, the NPQ values of both species increased significantly under HL conditions, although the increase was more obvious and significantly higher in S. costatum (1.18 ± 0.44 for S. costatum and 0.83 ± 0.60 for C. lorenzianus, p = 1.6 × 10-5). At day 4, which was the turning point of the decrease in S. costatum, the NPQ values of both species decreased suddenly. After day 7, since the fluorescence levels of S. costatum were under the detection limits, NPQ could not be estimated. This was similar to the case in Fv/Fm values, but C. lorenzianus exhibited gradual increases of NPQ.
Figure 5 Fv/Fm and NPQ under each light condition during the competition experiments. (A) and (B) show the Fv/Fm value of each species, while (C) and (D) show the NPQ of each species. Values are averages ± standard division (SD) of more than 15 colony measurements for each of the three treatments. Asterisks (*) indicate significant differences between species (p< 0.01, Student’s t-test). DL, detection limit; ND, no data.
In the continuous culture experiments, similar cell densities were observed in S. costatum at either dilution rate under both N- and P-limited conditions on day 12 when the cells were in a balanced growth phase. However, in the C. lorenzianus cultures, the cell densities under LDR conditions were one order of magnitude lower than those under HDR conditions under both N- and P-limited conditions. Therefore, S. costatum appears to maintain constant cell densities irrespective of nutrient levels, while the cell densities of C. lorenzianus changed depending on nutrient availability. Nutrient uptake in phytoplankton increases as cell size increases (Marañón et al., 2013). Compared to C. lorenzianus, the smaller cells of S. costatum may have affected their lower nutrient uptake and helped to maintain higher cell densities; however, C. lorenzianus might possess another strategy to decrease cell density, which would enable this species to allocate more nutrients to each cell. This assumption is supported by the observation that C. lorenzianus maintained higher Fv/Fm values than S. costatum, even under the LDR conditions. Therefore, the following discussion on N-limited conditions should be interpreted after considering such differences between both species. In the case of P-limited conditions, the above assumed strategies in both species might be negligible because they had comparable Fv/Fm values under the same dilution rates.
From the results of the rapid light curve measurements, under N-limited conditions, both species exhibited relatively higher qP values (> 0.8) regardless of their N deficiency status (i.e., dilution rate) under ML exposure (Figure 2A). However, under HL exposure, the qP values in S. costatum decreased to 0.64 in the HDR treatment and more extensively to 0.56 in the LDR treatment, while NPQ reached almost 1 in both dilution rate treatments. In contrast, such a decline in qP and an increase in NPQ were not observed in C. lorenzianus, even under the same conditions. Li et al. (2021) reported that a higher NPQ was observed in the diatom Thalassiosira punctigera under a combination of high light and N-limited conditions. They proposed that the increase in NPQ was a passive response to a decrease in PSII performance caused by inhibition of the de novo synthesis of D1 protein and resulting imbalance of the repair-damage cycle of PSII under N deficiency. In another diatom, Phaeodactylum tricornutum, N deficiency has been reported to decrease the D1 protein (PsbA) (Levitan et al., 2015). Our results showed similar increases in NPQ values under N-limited and HL conditions in S. costatum, but no such increases were observed in C. lorenzianus. These findings imply that S. costatum was more susceptible than C. lorenzianus to the combination of N limitation and HL conditions and consequently, that NPQ should be induced as a photoprotective strategy. Alternatively, as mentioned previously, the lower densities of C. lorenzianus may have meant that more nitrogen could be allocated to each cell, obviating the need to induce NPQ. However, the high NPQ induction observed in S. costatum might not have been sufficient to mitigate against photoinhibition under N-deficient and HL conditions because qP decreased, as shown in Figure 2A.
Conversely, under prolonged light exposure (Figure 3), C. lorenzianus induced NPQ to a similar level as that observed in S. costatum. NPQ induction is known to vary between diatom species and with light intensity and duration (Lavaud et al., 2004). Therefore, while the response of S. costatum occurred quickly, that of C. lorenzianus may have been slower. NPQ induction in N-limited C. lorenzianus was found only under prolonged HL exposure, implying that NPQ induction under continuous HL exposure may be required even in this species. By inducing NPQ, C. lorenzianus effectively maintained high qP levels under HL exposure (Figure 3C). Conversely, S. costatum appeared to be unable to maintain qP levels, irrespective of high NPQ induction (Figure 3A). These findings reinforce the above interpretation of the rapid light curve measurements in which the high NPQ induction in S. costatum may not have been sufficient for mitigating against photoinhibition under N-deficient and HL exposure. The reasons for the decrease in NPQ values observed in both species in the LDR treatments were unclear and remain speculative (see discussion below). Nevertheless, insufficient NPQ induction under LDR treatments caused a further decrease in qP values during HL exposure in S. costatum, but such a decrease was less apparent in C. lorenzianus.
Interestingly, decreases in NPQ values were also observed with N addition, but only in C. lorenzianus (Figure 3C). Such decreases in NPQ values, which were caused by N addition and not by N deficiency, were assumed to have occurred in response to NPQ relief due to a decrease in the need for the photoprotective function of C. lorenzianus. Indeed, the reintroduction of nitrogen has been shown to relieve NPQ in several microalgae due to the rapid recovery of photochemical functions (Liefer et al., 2018; Zhang et al., 2019). Such a quick recovery of the photochemical functions in C. lorenzianus would enable it to use higher light after reintroducing nitrogen, which further suggests the advantage that this species has over S. costatum under the combination of N limitation and HL conditions.
Our experiments showed that, under severely P-limited conditions, i.e., under LDR conditions, even ML exposure caused significant decreases in qP in both species (Figure 2B), indicating that even the ML intensity might be excessive and/or cause damage to PSII downstream. In rice plants, qP was also reduced under P-deficient conditions (Xu et al., 2007), implying that the proportion of the reduced state of quinone QA was elevated (Schreiber et al., 1986). Decreases in qP were more apparent under HL exposure, especially in S. costatum, suggesting that additional high light might lead to downstream deterioration and/or a marked reduction in QA. In addition, the residual DIP concentrations in S. costatum under N-limited condition were relatively lower than those in C. lorenzianus (shown in Figure 1A, LDR), suggesting S. costatum might have a higher demand for phosphorus than C. lorenzianus and this might lead to lower qP values in S. costatum than C. lorenzianus under the P-limited conditions. As with N limitation, both species induced NPQ passively under a combination of P limitation and HL exposure (Figure 2D), with the highest NPQ values observed in the LDR treatment. These findings suggest that P deficiency induced more severe photoinhibition than N deficiency, and that more photoprotection was necessary. Similar NPQ increases have been observed under P-limited conditions in various microalgae (Cui et al., 2017; Guo et al., 2018; Sun et al., 2019; Rocha et al., 2021). In these studies, NPQ activity was enhanced under P deficiency in order to dissipate excess light energy, protect against potential photooxidative damage and maintain the fluency of the energy flow (Cui et al., 2017). In Figure 3, these NPQ-based photoprotection mechanisms were observed in both species under HL exposure, although they were more apparent in C. lorenzianus under prolonged HL exposure (the maximum NPQ under HL exposure in the LDR conditions was 0.79 for S. costatum and 1.52 for C. lorenzianus). As mentioned previously, C. lorenzianus changed cell allocation responses based on nutrient loads. Higher levels of NPQ induction in C. lorenzianus compared to S. costatum, even under P-limited conditions, might indicate a higher potential for NPQ induction in C. lorenzianus.
Such active NPQ induction in P-starved cells is unique and differs from the case of N deficiency, in which NPQ impairment was observed. It is known that NPQ induction in diatoms relies mainly on qE (Goss and Lepetit, 2015), a quenching mechanism that is controlled by the build-up of the trans-thylakoid proton gradient, the conversion of xanthophylls diadinoxanthin (Dd) into diatoxanthin (Dt), and the presence of Lhcx proteins. Dt needs to be bound to LHC proteins, most likely to Lhcx proteins, to enhance NPQ (Lepetit et al., 2013), so the production of Dt and Lhcx proteins could control NPQ. Elevated expression of specific Lhcx genes under N deficiency has been reported in the diatom Phaeodactylum tricornutum (Taddei et al., 2016). Although there have been no reports of elevated Lhcx expression under P-limited conditions in diatoms, Dt was reported to be generally high under P limitation in the coccolithophorid Emiliania huxleyi (Stolte et al., 2000). This may be due to a malfunction in ATP synthase, which would be unable to drain protons out of the lumen, resulting in an excessive accumulation of protons and an increase in the trans-thylakoid proton gradient (ΔpH), which would in turn activate pH-dependent diadinoxanthin de-epoxidase (Huang et al., 2019). While further investigations are needed, the increase and decrease in NPQ under P- and N-limited conditions could be affected by the size or status of the Dd/Dt pools and Lhcx expression, which may show species-specific variations.
Similar to the case of N limitation, relief of NPQ induction with P addition was observed only in C. lorenzianus (Figure 3H). The reintroduction of phosphate also improved qP in this species, which facilitated the quick recovery of this species (compared to S. costatum).
The above experiments revealed characteristic responses in both species. Especially under P deficiency, S. costatum was more susceptible to light stress and actively induced NPQ to protect itself against excessive light; however, as indicated by the decrease in qP, NPQ might be insufficient. In contrast, C. lorenzianus could maintain relatively higher qP levels without NPQ after exposure to HL for a short time. Indeed, even under prolonged exposure to HL, C. lorenzianus actively induced NPQ, which resulted in the maintenance of higher qP levels than in S. costatum. Based on recent reports that have described a decreasing trend in phosphorus in the Seto Inland Sea (Hayashi et al., 2000; Ohara et al., 2020), we employed continuous cocultures of S. costatum and C. lorenzianus under P-limited condition to simulate the typical summer environment where severe P deficiency and high light occur. The results showed a population shift from S. costatum to C. lorenzianus under both ML and HL conditions, which could support several of the observed phenomena of Chaetoceros succession from Skeletonema in the field, presumably due to ocean oligotrophication (Tada et al., 2014). Succession of Chaetoceros over Skeletonema under P-limited conditions was also reported by Roden and O'Mahony (1984). In their experiment, a field-collected phytoplankton assemblage containing S. costatum and Chaetoceros sp. was supplied with a P-limited medium (N:P = 34) in a semicontinuous culture. Their findings showed that S. costatum became dominant. under a higher dilution rate condition when P limitation was moderate; conversely, S. costatum was outcompeted by Chaetoceros sp. under a lower dilution rate condition. Given this background and the findings of this study, the rapid succession of C. lorenzianus under HL conditions in our experiment suggests the existence of a synergetic relationship between HL conditions and P limitation. In addition, the findings that C. lorenzianus seemed to be able to allocate more nutrients to cells and recover faster upon nutrient replenishment may also explain the observed succession dynamics in this species. These assumptions might be supported by the photosynthetic responses of C. lorenzianus and S. costatum, in which the former exhibited relatively higher Fv/Fm values than the latter at day 4, likely because of the better physiological status of C. lorenzianus compared to S. costatum. The higher NPQ in S. costatum at the beginning of culture under both ML and HL conditions in the single-species culture experiments was previously attributed to active photoprotection of the photosystem damaged by P deficiency. This defense mechanism, however, eventually deteriorated after day 4 and resulted in a rapid decline in the S. costatum population. Although NPQ also decreased in C. lorenzianus on day 4, unlike the decrease observed in S. costatum, this decrease may have been ascribed to relaxation of NPQ, as shown in Figure 3H, probably because C. lorenzianus could use all of the phosphorus due to the decrease in S. costatum. Recent severe P limitation and increased solar insolation in summer in the Seto Inland Sea is definitely disadvantageous for S. costatum. This could be one of the reasons for the recent replacement of Skeletonema spp. by Chaetoceros spp. in the Seto Inland Sea and might be applicable for other bays in western Japan where a shift from Skeletonema spp. to Chaetoceros spp. has also been observed (Tada et al., 2014; Satomichi et al., 2019).
We investigated photosynthetic reaction parameters, especially NPQ, under HL exposure using culture strains of the diatoms S. costatum and C. lorenzianus, which were maintained in continuous cultures under N- or P- limited conditions. Under severe N deficiency, NPQ was lower in both species, and this decrease was associated with a decline in qP in S. costatum. In contrast to N deficiency, P deficiency caused a marked increase in NPQ in both species, presumably to protect the photosystems from excess light under P deficiency. Regardless of this protective function, the decrease in qP, which was more obvious in S. costatum, suggested that the species was unable to protect its photosystem. The reintroduction of nitrogen or phosphorus led to a relaxation of NPQ and an increase in qP, both of which recovered faster in C. lorenzianus. The above results indicated that S. costatum is more susceptible to high light under nutrient deficient conditions, particularly under P deficiency, and is less able to recover photosystem functioning after nutrients were reintroduced. These results support previous assumptions that Skeletonema spp. are at a disadvantage over Chaetoceros spp. under oligotrophic conditions, especially P limitation, and further indicate that high light works synergistically against the former. In the results of coculture under P-limited continuous culture conditions, S. costatum was replaced by C. lorenzianus at 15 days, and this was accelerated by high light exposure, resulting replacement by 9 days. This observation appears to mimic the current situation in the Seto Inland Sea of Japan, where the historically dominant Skeletonema has been replaced by Chaetoceros, possibly in response to a recent decrease in phosphorus and an increase in solar insolation at the sea.
The original contributions presented in the study are included in the article/Supplementary Material. Further inquiries can be directed to the corresponding author.
RY designed the study, had full access to all data, and takes responsibility for the validity thereof. SO contributed to data analysis and reviewed the manuscript; KK contributed to the discussion and reviewed/edited the manuscript. RY wrote the manuscript with contributions from SO and KK. All authors contributed to the article and approved the submitted version.
This study was financially supported by the Research Fellowship for Young Scientists (Grant Number 21J22797 to RY) and by KAKENHI (Grant number 22K14937 to SO), Japan Society for the Promotion of Science (JSPS).
We would like to thank members of the glass workshop of Hiroshima University for fabricating the cylindrical glass vessels, and Shotaro Naruse and Mayuna Nakagawa of the Aquatic Ecology Laboratory of Hiroshima University for assistance with our experiments.
The authors declare that the research was conducted in the absence of any commercial or financial relationships that could be construed as a potential conflict of interest.
All claims expressed in this article are solely those of the authors and do not necessarily represent those of their affiliated organizations, or those of the publisher, the editors and the reviewers. Any product that may be evaluated in this article, or claim that may be made by its manufacturer, is not guaranteed or endorsed by the publisher.
The Supplementary Material for this article can be found online at: https://www.frontiersin.org/articles/10.3389/fmars.2023.1095762/full#supplementary-material
Abo K., Akiyama S., Harada K., Nakaji Y., Hayashi H., Murata K., et al. (2018). Long-term variations in water quality and causal factors in the seto inland Sea, Japan. Bull. Coast. Oceanography 55.2, 101–111. doi: 10.32142/engankaiyo.55.2_101
Abo K., Yamamoto T. (2019). Oligotrophication and its measures in the seto inland Sea, Japan. Bull. Jap. Fish. Res. Edu. Agen. 49, 21–26.
Bienfang P. K., Harrison P. J., Quarmby L. M. (1982). Sinking rate response to depletion of nitrate, phosphate and silicate in four marine diatoms. Mar. Biol. 67, 295–302. doi: 10.1007/BF00397670
Bilger W., Björkman O. (1990). Role of the xanthophyll cycle in photoprotection elucidated by measurements of light-induced absorbance changes, fluorescence and photosynthesis in leaves of Hedera canariensis. Photosynth. Res. 25.3, 173–185. doi: 10.1007/BF00033159
Cheng L. (2003). Xanthophyll cycle pool size and composition in relation to the nitrogen content of apple leaves. J. Exp. Bot. 54 (381), 385–393. doi: 10.1093/jxb/erg011
Cui Y., Zhang H., Lin S. (2017). Enhancement of non-photochemical quenching as an adaptive strategy under phosphorus deprivation in the dinoflagellate Karlodinium veneficum. Front. Microbiol. 8. doi: 10.3389/fmicb.2017.00404
Demmig-Adams B., Adams W. W. I. (1992). Photoprotection and other responses of plants to high light stress. Annu. Rev. Plant Phys. 43.1, 599–626. doi: 10.1146/annurev.pp.43.060192.003123
Goss R., Lepetit B. (2015). Biodiversity of NPQ. J. Plant Physiol. 172, 13–32. doi: 10.1016/j.jplph.2014.03.004
Guillard R. R. (1975). “Culture of phytoplankton for feeding marine invertebrates,” in Culture of marine invertebrate animals. Eds. Smith W. L., Chanley M. H. (Boston, MA: Springer), 29–60. doi: 10.1007/978-1-4615-8714-9_3
Guo J., Wilken S., Jimenez V., Choi C. J., Ansong C., Dannebaum R., et al. (2018). Specialized proteomic responses and an ancient photoprotection mechanism sustain marine green algal growth during phosphate limitation. Nat. Microbiol. 3 (7), 781–790. doi: 10.1038/s41564-018-0178-7
Harrison P. J., Conway H. L., Holmes R. W., Davis C. O. (1977). Marine diatoms grown in chemostats under silicate or ammonium limitation. III. cellular chemical composition and morphology of Chaetoceros debilis, skeletonema costatum, and Thalassiosira gravida. Mar. Biol. 43 (1), 19–31. doi: 10.1007/BF00392568
Hayashi M., Yanagi T., Hashimoto T. (2000). Standing stock ratios nitrogen and phosphorus in the seto inland Sea. Oceanography Japan 9.2, 83–89. doi: 10.5928/kaiyou.9.83
Higo S., Maung-Saw-Htoo-Thaw, Yamatogi T., Ishida N., Hirae S., Koike K. (2017). Application of a pulse-amplitude-modulation (PAM) fluorometer reveals its usefulness and robustness in the prediction of Karenia mikimotoi blooms: A case study in sasebo bay, Nagasaki, Japan. Harmful Algae 61, 63–70. doi: 10.1016/j.hal.2016.11.013
Horton P., Ruban A. (2005). Molecular design of the photosystem II light-harvesting antenna: Photosynthesis and photoprotection. J. Exp. Bot. 56.411, 365–373. doi: 10.1093/jxb/eri023
Huang Z. A., Jiang D. A., Yang Y., Sun J. W., Jin S. H. (2004). Effects of nitrogen deficiency on gas exchange, chlorophyll fluorescence, and antioxidant enzymes in leaves of rice plants. Photosynthetica 42.3, 357–364. doi: 10.1023/B:PHOT.0000046153.08935.4c
Huang B., Marchand J., Blanckaert V., Lukomska E., Ulmann L., Wielgosz-Collin G., et al. (2019). Nitrogen and phosphorus limitations induce carbon partitioning and membrane lipid remodelling in the marine diatom Phaeodactylum tricornutum. Eur. J. Phycol. 54.3, 342–358. doi: 10.1080/09670262.2019.1567823
Imai I., Ishida T., Itakura S., Yamaguchi M. (2015). Abundance, spatial distribution and composition of resting stage cells of diatoms in bottom sediments of harima Nada and Osaka bay, Eastern seto inland Sea, Japan. Bull. Fisheries Sciences Hokkaido Univ. 65.1, 31–38. doi: 10.14943/bull.fish.65.1.31
Itakura S., Yamaguchi M. (2007). Environmental change and occurrence mechanism of harmful algal blooms in the seto inland Sea. Japanese J. Benthology 62, 57–61. doi: 10.5179/benthos.62.57
Jahns P., Holzwarth A. R. (2012). The role of the xanthophyll cycle and of lutein in photoprotection of photosystem II. Biochim. Biophys. Acta (BBA)-Bioenergetics 1817.1, 182–193. doi: 10.1016/j.bbabio.2011.04.012
Khamis S., Lamaze T., Lemoine Y., Foyer C. (1990). Adaptation of the photosynthetic apparatus in maize leaves as a result of nitrogen limitation: relationships between electron transport and carbon assimilation. Plant Physiol. 94.3, 1436–1443. doi: 10.1104/pp.94.3.1436
Kitajima M. B. W. L., Butler W. L. (1975). Quenching of chlorophyll fluorescence and primary photochemistry in chloroplasts by dibromothymoquinone. Biochim. Biophys. Acta (BBA)-Bioenergetics 376.1, 105–115. doi: 10.1016/0005-2728(75)90209-1
Lavaud J., Rousseau B., Etienne A. L. (2004). General features of photoprotection by energy dissipation in planktonic diatoms (Bacillariophyceae). J. Phycol. 40.1, 130–137. doi: 10.1046/j.1529-8817.2004.03026.x
Lepetit B., Sturm S., Rogato A., Gruber A., Sachse M., Falciatore A., et al. (2013). High light acclimation in the secondary plastids containing diatom Phaeodactylum tricornutum is triggered by the redox state of the plastoquinone pool. Plant Physiol. 161.2, 853–865. doi: 10.1104/pp.112.207811
Levitan O., Dinamarca J., Zelzion E., Lun D. S., Guerra L. T., Kim M. K., et al. (2015). Remodeling of intermediate metabolism in the diatom Phaeodactylum tricornutum under nitrogen stress. P. Natl. Acad. Sci. U.S.A. 112.2, 412–417. doi: 10.1073/pnas.1419818112
Li Z., Lan T., Zhang J., Gao K., Beardall J., Wu Y. (2021). Nitrogen limitation decreases the repair capacity and enhances photoinhibition of photosystem II in a diatom. Photochem. Photobiol. 97.4, 745–752. doi: 10.1371/journal.pone.0195705org/10.1111/php.13386
Liefer J. D., Garg A., Campbell D. A., Irwin A. J., Finkel Z. V. (2018). Nitrogen starvation induces distinct photosynthetic responses and recovery dynamics in diatoms and prasinophytes. PloS One 13.4, e0195705. doi: 10.1371/journal.pone.0195705
Liu S., Guo Z., Li T., Huang H., Lin S. (2011). Photosynthetic efficiency, cell volume, and elemental stoichiometric ratios in Thalassirosira weissflogii under phosphorus limitation. Chin. J. Oceanol. Limnol. 29.5, 1048–1056. doi: 10.1007/s00343-011-0224-2
Lu C., Zhang J. (2000). Photosynthetic CO2 assimilation, chlorophyll fluorescence and photoinhibition as affected by nitrogen deficiency in maize plants. Plant Sci. 151.2, 135–143. doi: 10.1016/S0168-9452(99)00207-1
Marañón E., Cermeño P., López-Sandoval D. C., Rodríguez-Ramos T., Sobrino C., Huete-Ortega M., et al. (2013). Unimodal size scaling of phytoplankton growth and the size dependence of nutrient uptake and use. Ecol. Lett. 16.3, 371–379. doi: 10.1111/ele.12052
Nishijima T., Fukami K. (1993). “Raphidophyceae and bacillariophyceae,” in Effect of N:P ratio in water on aquatic organisms. Ed. Yoshida Y. (Tokyo: Kouseisha Kouseikaku), 20–28.
Nishikawa T., Hori Y., Nagai S., Miyahara K., Nakamura Y., Harada K., et al. (2010). Nutrient and phytoplankton dynamics in harima-Nada, Eastern seto inland Sea, Japan during a 35-year period from 1973 to 2007. Estuaries Coasts 33.2, 417–427. doi: 10.1007/s12237-009-9198-0
Niyogi K. K., Truong T. B. (2013). Evolution of flexible non-photochemical quenching mechanisms that regulate light harvesting in oxygenic photosynthesis. Curr. Opin. Plant Biol. 16.3, 307–314. doi: 10.1016/j.pbi.2013.03.011
Ohara S., Yano R., Hagiwara E., Yoneyama H., Koike K. (2020). Environmental and seasonal dynamics altering the primary productivity in bingo-Nada (Bingo sound) of the seto inland Sea, Japan. Plankt. Benthos Res. 15, 78–96. doi: 10.3800/pbr.15.78
Pan S., Shen Z., Liu W., Han X., Miao H., Ma H. (2010). Nutrient compositions of cultured Skeletonema costatum, Chaetoceros curvisetus, and Thalassiosira nordenskiöldii. Chin. J. Oceanology Limnology 28.6, 1131–1138. doi: 10.1007/s00343-010-9909-1
R Development Core Team. (2022). R: A language and Environment for Statistical Computing. R Foundation for Statistical Computing. Available at: https://www.r-project.org/.
Ralph P. J., Gademann R. (2005). Rapid light curves: a powerful tool to assess photosynthetic activity. Aquat. Bot. 82.3, 222–237. doi: 10.1016/j.aquabot.2005.02.006
Rocha G. S., Lombardi A. T., Espíndola E. L. G. (2021). Combination of p-limitation and cadmium in photosynthetic responses of the freshwater microalga Ankistrodesmus densus (Chlorophyceae). Environ. pollut. 275, 116673. doi: 10.1016/j.envpol.2021.116673
Roden C. M., O'Mahony K. W. (1984). Competition as a mechanism of adaptation to environmental stress in outdoor cultures of marine diatoms. Mar. Ecol. Prog. series. Oldendorf 16.3, 219–227.
Satomichi N., Esaki Y., Tada K. (2019). Changes in Nutrient Concentrations and Species Composition of Phytoplankton in Fukuoka Bay, Japan during an 18-year Period from 1993 to 2010. Bulletin on Coastal Oceanography 52.6, 133–141. doi: 10.32142/engankaiyo.56.2_133
Schreiber U., Schliwa U., Bilger W. (1986). Continuous recording of photochemical and non-photochemical chlorophyll fluorescence quenching with a new type of modulation fluorometer. Photosynth. Res. 10.1, 51–62. doi: 10.1007/BF00024185
Stolte W., Kraay G. W., Noordeloos A. A. M., Riegman R. (2000). Genetic and physiological variation in pigment composition of Emiliania huxleyi (Prymnesiophyceae) and the potential use of its pigment ratios as a quantitative physiological marker. J. Phycol. 36.3, 529–539. doi: 10.1046/j.1529-8817.2000.99158.x
Sukisaki S., Umino K. (2013). A technical method for assessing the viability of phytoplankton to examine the performance of a ballast water management system. Bull. Plankton Soc Japan 60.1, 35–40. doi: 10.24763/bpsj.60.1_35
Sumper M., Brunner E. (2006). Learning from diatoms: nature's tools for the production of nanostructured silica. Advanced Funct. Materials 16.1, 17–26. doi: 10.1002/adfm.200500616
Sun K. M., Xin M., Sun P., Li Y., Li R., Tang X., et al. (2019). Photosynthetic activity of Prorocentrum donghaiense Lu acclimated to phosphorus limitation and its photosynthetic responses to nutrient depletion. J. Appl. Phycol. 31.3, 1721–1732. doi: 10.1007/s10811-018-1701-1
Tada K., Nishikawa T., Tarutani K., Yamamoto K., Ichimi K., Yamaguchi H., et al. (2014). Nutrient decrease in the Eastern part of the seto inland Sea and its influence on the ecosystem’s lower trophic levels. Bull. Coast. Oceanogr. 52.1, 39–47. doi: 10.32142/engankaiyo.52.1_39
Taddei L., Stella G. R., Rogato A., Bailleul B., Fortunato A. E., Annunziata R., et al. (2016). Multisignal control of expression of the LHCX protein family in the marine diatom Phaeodactylum tricornutum. J. Exp. Bot. 67.13, 3939–3951. doi: 10.1093/jxb/erw198
Tarutani K. (2007). Long-term variations in water environments in the seto inland Sea of Japan during 1973 to 2002 based on data from the fisheries monitoring program. Japan. Plankt. Benthos Res. 62, 52–56. doi: 10.5179/benthos.62.52
Ukeles R. (1973). “Continuous culture–a method for the production of unicellular algal foods,” in Handbook of phycological methods: culture methods and growth measurements. Ed. Stein J. R. (Cambridge: Cambridge University Press), 233–255.
Uno I., Wang Z., Yumimoto K., Itahashi S., Osada K., Irie H., et al. (2017). Is PM2.5 trans-boundary environmental problem in Japan dramatically improving? Japan Soc. Atmospheric Environ. 52.6, 177–184. doi: 10.11298/taiki.52.177
Wagner H., Jakob T., Lavaud J., Wilhelm C. (2016). Photosystem II cycle activity and alternative electron transport in the diatom Phaeodactylum tricornutum under dynamic light conditions and nitrogen limitation. Photosynth. Res. 128.2, 151–161. doi: 10.1007/s11120-015-0209-7
Xu H. X., Weng X. Y., Yang Y. (2007). Effect of phosphorus deficiency on the photosynthetic characteristics of rice plants. Russ. J. Plant Physiol. 54.6, 741–748. doi: 10.1134/S1021443707060040
Yamada M., Tsuruta A., Yoshida Y. (1980a). A list of phytoplankton as eutrophic level indicator. Bull. Japanese Soc. Sci. Fisheries 46.12, 1435–1438. doi: 10.2331/suisan.46.1435
Yamada M., Tsuruta A., Yoshida Y. (1980b). Classification of eutrophic level in several regions. Bull. Japanese Soc. Sci. Fisheries 46.12, 1439–1444. doi: 10.2331/suisan.46.1439
Yamada M., Tsuruta A., Yoshida Y. (1982). Map illustrating biological classification of eutrophic level in several areas in the inland Sea of seto. Bull. Japanese Soc. Sci. Fisheries 48.8, 1129–1132. doi: 10.2331/suisan.48.1129
Keywords: diatom, non-photochemical quenching (NPQ), photoinhibition, pulse amplitude modulation (PAM) fluorometry, oligotrophication, Seto Inland Sea of Japan
Citation: Yano R, Ohara S and Koike K (2023) High light stress under phosphorus limitation in summer may accelerate diatom shift from Skeletonema to Chaetoceros in an oligotrophic coastal area of Japan. Front. Mar. Sci. 10:1095762. doi: 10.3389/fmars.2023.1095762
Received: 11 November 2022; Accepted: 06 February 2023;
Published: 17 February 2023.
Edited by:
Jun Sun, China University of Geosciences Wuhan, ChinaReviewed by:
Zhengke Li, Shaanxi University of Technology, ChinaCopyright © 2023 Yano, Ohara and Koike. This is an open-access article distributed under the terms of the Creative Commons Attribution License (CC BY). The use, distribution or reproduction in other forums is permitted, provided the original author(s) and the copyright owner(s) are credited and that the original publication in this journal is cited, in accordance with accepted academic practice. No use, distribution or reproduction is permitted which does not comply with these terms.
*Correspondence: Ryoko Yano, cnlva295YW5vQGhpcm9zaGltYS11LmFjLmpw
Disclaimer: All claims expressed in this article are solely those of the authors and do not necessarily represent those of their affiliated organizations, or those of the publisher, the editors and the reviewers. Any product that may be evaluated in this article or claim that may be made by its manufacturer is not guaranteed or endorsed by the publisher.
Research integrity at Frontiers
Learn more about the work of our research integrity team to safeguard the quality of each article we publish.