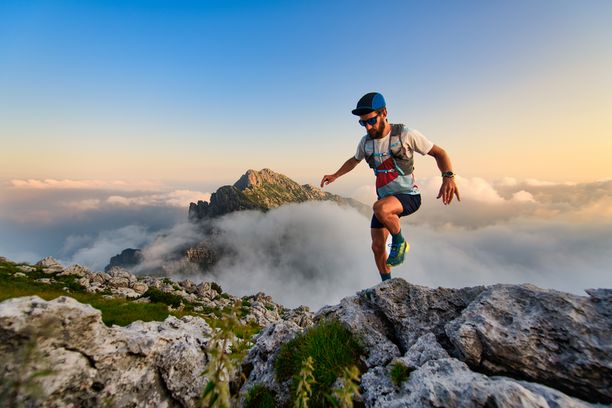
95% of researchers rate our articles as excellent or good
Learn more about the work of our research integrity team to safeguard the quality of each article we publish.
Find out more
ORIGINAL RESEARCH article
Front. Mar. Sci., 10 May 2023
Sec. Coral Reef Research
Volume 10 - 2023 | https://doi.org/10.3389/fmars.2023.1089746
This article is part of the Research TopicInnovative Approaches to Coral Reef Science by Early Career ResearchersView all 15 articles
Mesophotic corals live at ~30-150 m depth and can sustain metabolic processes under light-limited conditions by enhancing autotrophy through specialized photoadaptations or increasing heterotrophic nutrient acquisition. These acclimatory processes are often species-specific, however mesophotic ecosystems are largely unexplored and acclimation limits for most species are unknown. This study examined mesophotic coral ecosystems using a remotely operated vehicle (Ashmore Reef, Western Australia at 40–75m depth) to investigate the trophic ecology of five species of scleractinian coral (from genera Leptoseris, Pachyseris, and Craterastrea) using stable isotope analyses (δ13C and δ15N) of host and symbiont tissues and protein concentration. Trophic strategies were analyzed between species and between overall corals sampled above and below the end-photic point, where light is only 1% of surface irradiance. Results showed species-specific differences in resource use. Leptoseris hawaiiensis, L. scabra, and P. speciosa had similar Δ13C values (δ13C host - δ13C symbiont) approaching zero (< 0.5 ‰) which indicated greater dependence on symbiont autotrophy. In contrast, Leptoseris glabra and Craterastrea levis had higher Δ13C values (1.4 to 3.5 ‰) which indicated a greater reliance on external carbon sources. The latter two species also demonstrated tight nitrogen recycling within the holobiont, exhibiting low Δ15N values (host δ15N - symbiont δ15N =< 0.5 ‰), compared to more autotrophic species (Δ15N = >1.2 ‰). Some species demonstrated the ability to maintain metabolic processes despite substantially reduced light availability (0.5 – 2% of surface irradiance). This research challenges our knowledge of acclimation limits for many scleractinian corals and contributes novel information for Ashmore Reef, the Western Australia region and mesophotic ecosystems in general, and critically examines common methods used to interpretate trophic ecology with bulk stable isotopes δ13C and δ15N.
Mesophotic coral reef ecosystems (MCEs) harbor scleractinian corals between ~30 to 150 m depth where light availability is reduced compared to shallow water. Many scleractinian corals rely on photoautotrophic nutrient input via symbiotic intracellular algae (Symbiodiniaceae) to meet metabolic demands in oligotrophic waters (Baker, 2003; Muller-Parker et al., 2015). Thus, light availability is the primary factor driving vertical coral ecology (Mass et al., 2010; Kahng et al., 2019; Tamir et al., 2019) and biodiversity changes over depth (López-Londoño et al., 2022). As corals are mixotrophic they can also acquire organic nutrients through heterotrophy by the active or passive capture of plankton or particulate organic matter (Lewis and Price, 1975; Sebens et al., 1996). Some coral species are depth-generalists, surviving from shallow water down to upper mesophotic depths (to ~60 m). In contrast, depth-specialist corals typically inhabit low-light, mesophotic environments (below ~60 m) (Bongaerts et al., 2015; Lesser et al., 2019) and have been recorded at 172 m depth (Leptoseris hawaiiensis, French Polynesia) (Rouzé et al., 2021). Increasing heterotrophic nutrient acquisition can compensate for potential photosynthetic deficiencies under low light availability (e.g., turbid waters, seasonal environmental changes, and depth) (Anthony andFabricius, 2000; Ferrier-Pagès et al., 2011; Kahng et al., 2019), provisioning longer-term energy reserves (Seemann et al., 2012; Tremblay et al., 2015) compared to rapidly metabolised photosynthates (Tremblay et al., 2015). However, the understanding of metabolic trade-offs at depth remains unclear and investigations into the trophic plasticity and photoadaptations of mesophotic Scleractinia under various environmental conditions are still in their infancy.
Interest in MCEs has accelerated over the last decade (reviewed in Turner et al., 2017) due to technical advances (e.g., remotely operated vehicles (ROVs), autonomous underwater vehicles (AUVs) and imaging technology) (Muir et al., 2015; Armstrong et al., 2019; Pinheiro et al., 2019) and interest in the Deep Reef Refuge Hypothesis (DRRH), which suggests MCEs may function as short-term ecological refuges, long term refugia or deep areas of resilience for threatened corals (Glynn, 1996; Riegl and Piller, 2003; Bongaerts and Smith, 2019). Despite this, mesophotic corals remain poorly studied when compared to shallow reefs (< 30 m) (Kahng et al., 2014; Turner et al., 2017; Eyal et al., 2021), and available literature is geographically biased towards the northern hemisphere regions (i.e., Atlantic, Red Sea, and Caribbean) (Turner et al., 2017; Muir and Pichon, 2019). Little is known about mesophotic ecosystems in the Indo-Pacific and northwest Australian regions, despite hosting some of the world’s most extensive reef systems with the highest coral species richness globally (Laverick et al., 2018; Muir and Pichon, 2019). Furthermore, physiological studies predominantly use common species from shallower waters such as Stylophora pistillata (Reynaud et al., 2002; Alamaru et al., 2009; Einbinder et al., 2009; Houlbrèque and Ferrier-Pagès, 2009; Mass et al., 2010) and Montastraea cavernosa (Vize, 2006; Lesser et al., 2007; Lesser et al., 2010; Serrano et al., 2014), hence there is a dearth of literature for the majority of mesophotic scleractinian corals (Loya et al., 2019). Understanding how mesophotic corals can survive in only a fraction of shallow reef irradiance (Englebert et al., 2017; Hoeksema et al., 2017) is an essential part of understanding their ecophysiology and limitations (Loya et al., 2016). Symbiodiniaceae can translocate up to 95% of their photosynthates to coral hosts (Sutton and Hoegh-Guldberg, 1990), yet corals can also increase heterotrophic reliance to supplement for metabolic deficits (Muscatine et al., 1989; Lesser et al., 2010; Sturaro et al., 2021) as photosynthate production slows over depth (Mass et al., 2010; Kahng et al., 2019).
Whole tissue (bulk) stable isotope analysis (SIA) of carbon (δ13C) and nitrogen (δ15N) provides information about the trophic ecology and dietary contributions of corals (Muscatine et al., 1989; Reynaud et al., 2002; Lesser et al., 2010; Tremblay et al., 2015). The δ13C values of coral host tissues typically reflect those of key nutrient sources, such as photosynthates from symbionts or external carbon sources obtained heterotrophically from the water column (i.e., plankton and particulate organic matter) (Peterson and Fry, 1987; Muscatine et al., 1989; Kahng et al., 2019). Therefore, larger deviations in δ13C between the coral host and symbionts indicate the incorporation of external nutrients. The process of photosynthesis preferentially fixes lighter carbon isotopes (12C) (Muscatine et al., 1989), thus symbionts with higher photosynthetic rates have higher δ13C values (i.e., in shallow waters) (Muscatine et al., 1989; Reynaud et al., 2002; Lesser et al., 2010). Subsequently, δ13C values of symbionts (and the overall holobiont) typically decrease gradually with increasing depth in response to light reductions that lower photosynthetic fractionation (Heikoop et al., 1998; Lesser et al., 2010). Low host δ13C values can also result from heterotrophic feeding and may reflect the δ13C values of particulate resources such as phytoplankton and zooplankton (-17 ‰ to -21 ‰) (Land et al., 1975; Fontugne and Claude Duplessy, 1978; Rodrigues and Grottoli, 2006).
In typical food webs, an estimated average δ15N enrichment of approximately +2 to 3.4 ‰ occurs in consumers with each trophic level (Minagawa and Wada, 1984; Lesser et al., 2010) and has previously been used to assess levels of heterotrophy in corals. This enrichment estimation, however, incorporates averages from various taxa and environments (including terrestrial and freshwater) (Minagawa and Wada, 1984; Zanden and Rasmussen, 2001). In addition to this, there are no overall trends between δ15N and heterotrophy in mesophotic corals (Lesser et al., 2022), likely due to multiple nitrogen inputs (Mullin et al., 1984) and nitrogen recycling (Reynaud et al., 2009; Tremblay et al., 2015; Rangel et al., 2019), however this relationship has been largely unstudied. Complementary physiological/metabolic measurements (e.g., dietary biomarkers, protein analysis, photosynthetic proxies) can strengthen the interpretation of SIA and trophic strategies (Houlbrèque et al., 2003; Ferrier-Pagès et al., 2011; Crandall et al., 2016; Radice et al., 2019a), yet species-specific physiological adaptations and metabolic trade-offs at depth should also be considered (Houlbrèque and Ferrier-Pagès, 2009; Cooper et al., 2011a; Cooper et al., 2011b; Seemann, 2013; Brunner, 2015).
Western Australia includes a vast marine realm spanning over 30 degrees of latitude where important coral biodiversity hotspots have been identified in shallow waters (< 30 m) of the north-west region (e.g., Scott Reef, Ashmore Reef) (Richards et al., 2014). To date, few studies have analyzed mesophotic coral physiology in the extended Western Australian region (Cooper et al., 2011a; Cooper et al., 2011b) and, to our knowledge, the physiology of mesophotic corals at Ashmore Reef has never been examined. This study aimed to determine the levels of autotrophy and heterotrophy in mesophotic coral species using SIA of carbon (δ13C) and nitrogen (δ15N) and protein analyses. As the sampling range extends into the lower mesophotic depths, it is hypothesized that in general, coral species will require the input of external nutrient sources to sustain their metabolic budget at depth necessitated by low light levels. It is also hypothesized that P. speciosa, a known obligate autotroph (Veron, 2000), will differ to the depth-specialist species of the genera Leptoseris and Craterastrea. Here, we provide a first assessment of in-situ trophic ecology in depth-specialists Craterastrea levis, Leptoseris glabra, Leptoseris hawaiiensis, Leptoseris scabra and depth-generalist Pachyseris speciosa for Western Australia. This research also provides valuable information on the limits of acclimation of Indo-Pacific mesophotic corals and the trophic capabilities of zooxanthellate corals at depth.
Ashmore Reef is an exposed, oceanic reef situated in the Timor Sea along Australia’s north-west shelf, 460 km north of the Dampier Peninsula on Australia’s mainland (Supplementary Figure 1A). Sample collections were conducted at Ashmore Reef, Western Australia (12°13’45°S 123°02’15°E) on the R/V FALKOR between 12 and 24 April 2021, as part of the Mesophotic Coral Examination hosted by Schmidt Ocean Institute in collaboration with the Australian Institute of Marine Science, University of Western Australia, Western Australian Museum and Curtin University. Coral sampling was conducted at six sites around the reef between 41 – 75 m (Supplementary Figure 1B) (Permit Number AU-COM2021-508). Sites were chosen to encompass habitats around as much of the reef as possible to align with shallow reef monitoring sites (Gilmour et al., 2019), however the most eastern side of the reef was not accessible for sampling due to strong currents. Leptoseris spp. and Pachyseris sp. were target taxa based on their known presence at mesophotic depths in NW Australia (Cooper et al., 2011a; Cooper et al., 2011b; Turner et al., 2018; Heyward and Radford, 2019; Muir and Pichon, 2019). April marks the beginning of the dry season, and conditions during all sampling days were calm with no cloud cover.
Coral fragments (~5 cm length) were sampled using the Remotely Operated Vehicle (ROV) SUBASTIAN and stored in solid quivers, in seawater from the collection site (i.e., same water temperature), for the duration of the dive (~1.5 - 7 hours), where no light reached the samples. Few samples were alternatively stored in transparent boxes on the ROV. Subsequently, all samples were immediately transferred to the lab and only samples stored in quivers (where there was no light interaction) were measured for dark-adapted quantum yield (Fv/Fm). Fragments for SIA and protein analysis were subsampled and rinsed with filtered seawater (FSW) (0.45 µm Millipore filters through an EZ-STREAM® vacuum pump) to remove any extraneous particles or debris and frozen at -80°C.
The species were identified based on skeletal morphology as Leptoseris hawaiiensis, Leptoseris glabra, Leptoseris scabra, Craterastrea levis and Pachyseris speciosa. All sample information (e.g., species, depth, site) is detailed in Supplementary Tables 1, 5.
CTD casts (Biospherical Quantum Sensor, LI-COR) recorded downwelling profiles of solar irradiance (photosynthetically active radiation (PAR) µmol m-2 s-1) (McCree, 1981), temperature (°C) (ITS-90), and chlorophyll-a (mg-1 m-3) (WET Labs ECO-AFL/FL). An overall mean PAR by depth was calculated from midday (11:30am - 12:30pm) from two CTD casts (sites 2W and 3NW) and when solar irradiance was highest (Mass et al., 2010; Kirk, 2011; Tamir et al., 2019). This informed mean mid-photic and end-photic points that occur at 10% and 1% of surface PAR, respectively (Lesser et al., 2010; Kirk, 2011). To further describe the light environment at Ashmore Reef, a mean vertical diffuse attenuation coefficient of downwelling light (PARKd) was calculated according to Lambert-Beer law (Iz = I0 eKdz) defined by Kirk (2011). PARKd is primarily influenced by solar incidence (Brakel, 1979; Lesser et al., 2019), scattering, and absorption of light in the water column (Kirk, 2011; Muir and Pichon, 2019). Due to logistical issues and challenging current conditions, not all environmental variables were obtained from each site (Supplementary Table 2). It is acknowledged that temporal differences in cast times could influence readings.
Skeletal vouchers were taxonomically examined to identify species based on the accepted names in the World Register of Marine Species (https://www.marinespecies.org - 12.08.2021). Identifications were made after viewing macro and microstructural features and with reference to the following literature (Dinesen, 1980; Veron, 2000; Benzoni et al., 2012; Arrigoni et al., 2019). Whole skeletal images were taken with a Cannon EOS80D DSLR Camera, and macro images with a Leticia LAS X camera. Scanning electron microscopy (SEM) was undertaken on select specimens using the Vega3 Tescan Variable Pressure microscope (HV: 20kV, BI 10.00) in the John de Laeter Centre at Curtin University, Western Australia.
Dark-adapted quantum yield of photosystem II (Fv/Fm) of each colony was measured to determine coral health at the time of sampling. This was carried out using a Dive Pulse-Amplitude-Modulated (PAM) Fluorometer (Diving-PAM, Walz Gmbh, Germany) with the saturation pulse method (Genty et al., 1989; Schreiber, 2004) within 45 minutes after reaching the surface. Corals were only taken out of the quivers briefly (~30 seconds) to perform PAM measurements under a low artificial light (~1 – 2 μmol photons m-1 s-1). Average values were taken from three measurements of the outer, central, and inner sections of each colony (>20 mm apart) to capture intracolonial variation in chlorophyll distribution (Ralph et al., 2005). The fiberoptic cable was held directly onto the coral coenosarc (the most planar surface) as a standardization of optical distance (0 mm), in order to minimize potential variation in fluorescence (Ralph et al., 2005).
The methodology used for SIA was an adaptation of Einbinder et al. (2009) and Reynaud et al. (2002). Samples were thawed from -80°C and holobiont material comprising the animal host tissue (host) and algal symbionts (symbiont) was removed by gently airbrushing (OZITO 3 L 105 W airbrush) with a reservoir of 10 - 15 mL FSW (0.45 µm) at a maximum of 4 psi. The holobiont slurry was initially centrifuged (Heraeus Multifuge X1R Centrifuge, Thermo Scientific) at 4°C at 3000 g for 5 minutes to separate the host tissue from the symbiont pellet. Mucousy samples that did not separate on initial centrifugation were homogenized using a handheld tissue homogenizer (D-9, MICCRA) for 30 - 60 seconds on 11,000 rpm and centrifuged again at 3000 g for 5 minutes (Sturaro et al., 2019). The host supernatant was centrifuged again (3 times) at 4°C at 3000 g for 5 minutes and purity was checked under a compound microscope. The symbiont pellet was resuspended in 2 mL of FSW and centrifuged again at 3000 g for 5 minutes. The supernatant was discarded, and this process was repeated 2 - 3 times before checking for purity.
The symbiont components were acidified with 1 N HCl to remove any residual carbonates (e.g., skeletal fragments). The acid was added dropwise with a pipette until bubbling ceased (Sturaro et al., 2019). Acidified samples were sonicated for 10 minutes before being repeatedly washed with FSW and centrifuged at 3000 g for 5 minutes until supernatant pH = 7 (neutral). Any white top layer that may comprise host lipids and excess liquid was removed with a pipette. Samples were freeze-dried (Alpha 1-2 LD plus Freeze-Dryer, Martin Christ) for >24 hours.
Triplicates of the dried host and symbiont material (~0.2 mg for symbiont and ~0.2 - 0.6 mg for host tissue) were encapsulated in tin cups (3.5 × 5 mm, Eurovector) and combusted to CO2 and N2 in an Elemental Analyzer (Flash 2000 HT, Thermo Scientific) coupled to an Isotope Ratio Mass Spectrometer (DELTA V Advantage Mass Spectrometer, Thermo Scientific) via a ConFlow IV to measure stable isotope ratios of carbon (13C/12C) and nitrogen (15N/14N) as δ13C and δ15N in per mil (Western Australian Organic & Isotope Geochemistry Centre labs, Curtin University, Australia) for host (n = 30) and symbiont (n = 30) samples. The instrument was calibrated by running triplicate blanks and duplicates of certified reference materials USGS40 (L-glutamic acid), USGS41 (L-glutamic acid) (Qi et al., 2003), and IAEA-600 (caffeine) (Coplen et al., 2006), before and after each full sample batch. Instrumental analytical precision for carbon and nitrogen was 0.1 ± 0.08 ‰ and 0.1 ± 0.09 ‰, respectively. SIA values for individual samples were averaged from triplicates and conventionally expressed as delta (δ) values in parts per mil (‰) as deviations relative to the Vienna Peedee Belemnite Limestone Standard (V-PDB) for C and atmospheric nitrogen gas (N2) for N (Peterson and Fry, 1987; Coplen, 2011; Ben-David and Flaherty, 2012).
Aliquots for protein analysis were obtained from final host supernatant samples by pipetting 0.5 mL of host tissue into a 20 mL glass vial, diluted with 2 μL of FSW and stored at -20°C prior to analysis. The absorbance of samples was measured in triplicate by adding 2 μL of tissue to a Nanodrop 1000 Spectrophotometer (software V3.8) to obtain average absorbances at λ 235 nm and 280 nm (setting: ProteinA280; sample type: universal mg/mL). The instrument was repeatedly calibrated to Milli-Q® water and FSW was used as a control for the possible presence of proteins in the dilutant. Calculations to determine concentrations followed those by (Whitaker and Granum, 1980) to account for interference of other nucleic and amino acids.
Data were normalized to the surface area of the coral airbrushed. Surface area measurements were taken using the foil method as in (Marsh, 1970). Four 100 cm2 (10 cm x 10 cm) squares of foil were cut and measured to obtain a mean weight for 100 cm2, then the area for 1 cm2 calculated. Final protein concentration (mg/mL) per cm2 of skeletal surface area was calculated as:
The foil method has come under critique for overestimating the surface area of branching and rounded morphologies due to significant foil overlapping (Hoegh-Guldberg, 1988; Veal et al., 2010), yet all corals in this study exhibited flat, simple morphologies which avoided overlapping.
All analyses were conducted in R (v1.4.1717). Data were inspected visually to check normality and homogeneity using histogram charts and sample quantile plots (RStudio Team, 2020). Linear Mixed Effects (LME) models (‘nlme’) were used to test for effects of species, species and tissue type, or depth and species with site included as a random effect.
Sampling was not fully replicated across species and depths due to the opportunistic sampling method, challenges with environmental conditions and patchy habitat distribution in Ashmore’s mesophotic reef. Subsequently, analyses were performed for overall means for either species or irradiance level. Corals were separated into two irradiance levels: above 1% of surface irradiance (above 1% SI), which included corals from 45 – 54 m depths (n = 11), and below 1% of surface irradiance (below 1% SI), which included corals from 55 – 75 m depths (n = 19). These groups represent corals living above and below the mean end-photic point, which occurs when light levels reach just 1% of surface irradiance.
One outlier was removed from the analysis of protein (L. hawaiiensis < 55 m depth). The difference in stable isotopes between individual paired host and symbiont samples (Δ13C and Δ15N) were also tested (e.g., host δ13C – symbiont δ13C). Significance levels were based on α = 0.05. Post-hoc Tukey pairwise tests were analyzed for significant results (‘emmeans’). Means are ± 1 Standard Deviation. Pearson’s correlation tests were used to test relationships between depth vs. symbiont δ13C, symbiont δ15N and protein. Ellipse plots of isotopic niches (δ13C vs. δ15N) were plotted based on a 40% confidence interval representing the maximum likelihood standard ellipse area (Jackson et al., 2011). All raw data are contained in Supplementary Table 5.
Solar irradiance (PAR) followed an exponential decline over depth. Mean surface PAR was 2245 µmol m-2 s-1 (at 1 m) with an average diffuse attenuation coefficient of PARKd = 0.069 m-1. Mean mid- (10%) and end-photic (1%) points were realized at 27 m and 54 m, respectively (Supplementary Figure 2). This represents the optical properties of water at Ashmore Reef at time of sampling. Mean sea surface temperature was 29.51 ± 0.12°C (± SE) and gradually decreased over depth by 1.69 ± 0.17°C to 80 m depth (Supplementary Figure 2). This indicated relatively consistent temperatures across the sampled depth range (41 – 75 m). Chlorophyll-a fluorescence readings for chlorophyll-a density in the water column represented quantities of phytoplankton across depth. These quantities were highest at mesophotic depths, with all sites exhibiting low phytoplankton concentrations at the surface (< 1 mg m-3) until 25 – 35 m or deeper before peaking at depths approximate to the upper mesophotic zone (30 – 68 m).
Dark-adapted quantum yields (Fv/Fm) across species ranged from 0.69 - 0.71, which indicated that corals were in healthy condition at the time of sampling (Ralph et al., 2015, e.g., Padilla-Gamiño et al., 2019; Bhagooli et al., 2021). There were species differences detected in Fv/Fm among species (F1,23 = 5.69, p = 0.002). Pairwise Tukey post-hoc test showed Leptoseris hawaiiensis (0.71 ± 0.01) had significantly higher Fv/Fm than P. speciosa (0.67 ± 0.03) (t19 = 3.411, p = 0.022) and L. glabra (0.67 ± 0.01) (t,19 = -3.589 p = 0.015), yet overall mean values in corals remained constant between depths above and below 1% SI (F1,23 = 0.610 p = 0.444).
The coral host and symbiont fractions were analyzed for δ13C (‰). Mean coral host δ13C trends showed higher values in L. glabra (-19.1 ± 1.5 ‰) and C. levis (-19.5 ± 0.7 ‰) compared to L. hawaiiensis (-21.3 ± 1.4 ‰), L. scabra (-20.1 ± 2.0 ‰), and P. speciosa (-20.2 ± 1.2 ‰) (Table 1; Figure 1A), yet these differences were not significant (F4,22 = 2.394, p = 0.081) (Supplementary Table 4). In contrast, symbiont δ13C values were lowest in L. glabra (-22.6 ± 0.8 ‰) and C. levis (-21.0 ± 1.4 ‰) and highest in L. scabra (-20.6 ± 1.5 ‰) and P. speciosa (-20.7 ± 0.7 ‰) (Table 1; Figure 1B). Linear mixed effects models indicated a significant effect of species (F4,22 = 3.565, p = 0.022), with Tukey pairwise tests revealing significantly lower symbiont δ13C in L. glabra (-22.6 ± 0.8 ‰) compared to both L. hawaiiensis (-20.9 ± 0.7 ‰) (t22 = -3.502, p= 0.015) and P. speciosa (-20.7 ± 0.7 ‰) (t22 = -3.535, p = 0.014) (Supplementary Table 4).
Figure 1 (A) Mesophotic coral host (pink) and symbiont (green) δ13C values, (B) δ15N values, and (C) isotopic niches of hosts (pink solid line) and symbionts (green dashed line) from five coral species collected between 44 m and 75 m depth from Ashmore Reef, Western Australia. Dots in (C) represent individual data points and ellipses are based on a 40% confidence interval that represent the maximum likelihood standard ellipse area. The sample size of Leptoseris scabra (n = 3) was insufficient to calculate an ellipse, however trends in its isotopic niche can be observed.
Table 1 Overall means of stable isotope variables (Host δ13C and δ15N, Symbiont δ13C and δ15N, host-symbiont δ13C (Δ13C), and host-symbiont δ15N (Δ15N) and protein concentrations in mesophotic corals collected in April 2021 from Ashmore Reef, Western Australia, across species and irradiance levels (over 1% surface irradiance (SI) and under 1% SI).
Differences in host δ13C were not detected between depths above and below 1% SI (F1,25 = 0.013, p = 0.909), however overall symbiont δ13C had a significant relationship with depth and became more negative as depth increased and light availability diminished (correlation= -0.545, t28 = -3.435, p = 0.002) (Figure 2). Accordingly, corals from depths above 1% SI (-20.3 ± 0.8 ‰) had significantly higher symbiont δ13C values than corals below 1% SI (-21.4 ± 1.0 ‰) (F1,25 = 7.277, p = 0.012).
Figure 2 Trends over depth of Symbiont δ13C (44 m to 75 m) recorded in mesophotic coral species in April at Ashmore Reef, Western Australia.
Linear mixed effects models used to test for differences between coral host vs. symbiont δ13C among species found no significant effect of species (F4,47 = 1.286, p = 0.289), however there was a significant effect of host vs. symbiont tissues (F1,47 = 6.417, p = 0.015) and an interaction between species and tissue type (F4,47 = 4.401, p = 0.004) (Supplementary Table 4). Tukey post-hoc tests showed that L. glabra had higher host δ13C values (-19.1 ± 1.5 ‰) compared to its symbionts (-22.6 ± 0.8 ‰) (t47 = 4.363, p = 0.003) (Figure 1A).
The Δ13C value is the pairwise difference between individual coral host and symbiont δ13C values and was also tested for differences among species and between irradiance levels. Leptoseris glabra (3.5 ± 1.7 ‰) and C. levis (1.4 ± 1.0 ‰) had relatively higher Δ13C compared to all other species (L. hawaiiensis, P. speciosa and L. scabra) that had Δ13C values below 0.5 ‰. (Table 1; Figure 3A). Linear mixed effects models revealed an effect of species (F4,21 = 4.086, p = 0.013), with significant differences between in L. glabra (Δ13C = 3.5 ± 1.7 ‰) and L. hawaiiensis (-0.4 ± 1.7 ‰) (t21 = 3.928, p = 0.006) (Supplementary Table 4). Mean coral Δ13C was also analyzed between irradiance levels, with corals above 1% SI exhibiting extremely low mean Δ13C values (0.1 ± 1.6 ‰), compared to corals below 1% (1.1 ± 2.2 ‰), yet there was a lot of variation within the data and differences between these irradiance levels were not significant (F1,21 = 2.328, p = 0.142).
Figure 3 (A) Differences between paired δ13C values from individual coral hosts and symbionts (Δ13C) and (B) paired δ15N values from individual coral hosts and symbionts (Δ15N) in five mesophotic coral species (44 m to 75 m depth) from Ashmore Reef, Western Australia.
Mean coral host δ15N values of corals ranged from 5.3 ± 0.6 ‰ (L. glabra) to 6.3 ± 0.7 ‰ (L. scabra) (Table 1), yet no significant differences occurred among species (F4,22 = 0.347, p = 0.844) (Table 1; Figure 1B; Supplementary Table 4). Conversely, there was a significant effect of species on symbiont δ15N (F4,22 = 3.476, p = 0.024). Craterastrea levis and L. glabra shared the lowest mean symbiont δ15N (5.0 ± 0.6 ‰ and 5.0 ± 0.8 ‰, respectively) while L. hawaiiensis had the highest (3.6 ± 1.3 ‰), however differences were only significant between L. hawaiiensis and L. glabra (t22 = 3.216, p = 0.029) (Figure 1B; Table 1; Supplementary Table 4). Irradiance level did not affect host δ15N (F1,25 = 1.166, p = 0.290) or symbiont δ15N (F1,25 = 0.959, p = 0.337). (Table 1; Figure 1B; Supplementary Table 4). In contrast to symbiont δ13C data, no significant trends were found between symbiont δ15N and depth (correlation = -0.137, t28 = -0.734, p = 0.469).
Linear mixed effects models were used to test for differences in δ15N between coral hosts and symbionts among species. Results showed no significant effect of species (F4,47 = 1.024, p = 0.405), however there was a significant effect of tissue type (F1,47 = 34.803, p<0.001). Tukey post-hoc tests showed that L. hawaiiensis host had significantly higher host δ15N (5.8 ± 1.4 ‰) compared to its symbionts (3.6 ± 1.3 ‰) (t47 = 5.181, p<0.001) (Table 1; Figures 1B, C; Supplementary Table 4).
The Δ15N value reflects the difference between individual host and symbiont δ15N values and was assessed among species and depth ranges. Coral Δ15N was highest in L. hawaiiensis (2.3 ± 1.2 ‰) and L. scabra (2.1 ± 0.6 ‰) and lowest in L. glabra (0.3 ± 1.1 ‰) and C. levis (0.5 ± 0.4 ‰) (Table 1; Figure 3B). The coral species had a significant effect on Δ15N (F4,21 = 3.490, p = 0.025) with Tukey post-hoc tests revealing that L. hawaiiensis had significantly higher mean Δ15N than L. glabra (+2 ‰) (t21 = -3.077, p = 0.041). There was no significant effect of irradiance level, with Δ15N values remaining constant between corals from depths above and below 1% SI (1.4 – 1.5 ‰) (F1,21 = 0.086, p = 0.773) (Table 1; Supplementary Table 4).
The isotopic niches of L. glabra and L. hawaiiensis hosts and symbionts did not overlap, with δ13C values differentiating L. glabra isotopic niches while δ15N values differentiated L. hawaiiensis niches. The isotopic niches of P. speciosa and C. levis showed slight overlap between hosts and symbionts. While there was insufficient data to plot isotopic niches of L. scabra, hosts and symbionts were also differentiated by δ15N values (Figure 1C).
Isotopic niches of coral hosts and symbionts did not overlap at any irradiance level, with δ15N values driving niche in corals at depths above 1% of surface irradiance, and 13C and 15N influencing differentiation in corals at depths below 1% of surface irradiance (Figure 4).
Figure 4 Coral isotopic niches of host and symbiont carbon (δ13C) and nitrogen (δ15N) stable isotopes from colonies sampled between 44 m and 75 m depth in April at Ashmore Reef, Western Australia. Depth ranges are relative to the recorded depth of the end-photic point (1% of surface irradiance at 45 m depth) and categorized into light environments above and below 1% of surface irradiance (SI). Triangle and dot points represent individual data from host (circle) and symbiont (tringle) fractions. Ellipses are based on a 40% confidence interval that represents the maximum likelihood standard ellipse area.
There was significant variance in protein among species (F4,20 = 4.635, p = 0.008), with L. scabra (2.26 ± 0.3 mg mL-1 cm-2) and C. levis (1.9 ± 0.5 mg mL-1 cm-1) having ~2 - 2.3 times higher protein concentrations than P. speciosa (0.97 ± 0.5 mg mL-1 cm-2) (L. scabra vs. P. speciosa, t20 = 3.277, p = 0.028, and C. levis vs. P. speciosa, t20 = 3.515, p = 0.016) (Table 1; Supplementary Table 4), while L. hawaiiensis and L. glabra had similar protein concentrations of ~1.5 mg mL-1 cm-2 (Table 1). There were no correlations detected between protein concentration and depth (correlation = -0.094, t28 = 1.519, p = 0.140), and no statistical differences were detected in corals from depth above or below the end photic point (1% SI) (F1,20 = 3.398, p = 0.080) (Table 1; Supplementary Table 4).
To gain insight into the limits of acclimation of mesophotic corals, this study assessed autotrophy and heterotrophy through the analysis of SIA and protein concentrations of several mesophotic species at Ashmore Reef, Western Australia, across a 31 m depth range (44 to 75 m) from light environments above and below the end-photic point (at 1% of surface irradiance). We found that, despite extremely low light conditions, L. hawaiiensis, L. scabra, and P. speciosa were able to maintain metabolic processes predominantly through photosynthetic carbon, whereas L. glabra and C. levis showed higher dependencies on heterotrophy. No significant differences between autotrophy and heterotrophy were observed between corals from depths above versus below 1% of surface irradiance and thus, the end-photic point does not represent an environmental threshold for nutrient acquisition in this study. This research presents the first physiological data for C. levis (observed 44 – 75 m), an understudied and apparently uncommon depth-specialist species.
The lowest host δ13C value was -23.0 ‰ in our study (L. hawaiiensis), with an even lower symbiont δ13C value of -23.7 ‰ (different colony of L. hawaiiensis) that may be the lowest reported for a symbiotic scleractinian coral, thus expanding recorded δ13C ranges. Similarly low host and symbiont δ13C values were reported for mesophotic coral in Hawaii (-22.7 ‰), Red Sea (-22.7 ‰), and Jamaica (-22.4 ‰) (Muscatine et al., 1989; Einbinder et al., 2009; Padilla-Gamiño et al., 2019), providing insight into the metabolic limits of corals. In general, symbiont δ13C values tended to be more negative than host δ13C in our study. It is likely that metabolic CO2 derived from mesophotic hosts with low δ13C may drive the particularly low symbiont δ13C values observed, in addition to greater isotopic discrimination due to reduced photosynthesis (Muscatine et al., 1989). This trend could also be attributed to a higher concentration of 13C-depleted fatty tissues (e.g., lipids) (Alamaru et al., 2009; Sturaro et al., 2019), which can be considerably elevated in symbiont cells compared to their host (Radice et al., 2019a).
Increasingly negative symbiont δ13C over depth indicated that photosynthetic activity was reduced as light availability decreased (Farquhar et al., 1989; Muscatine et al., 1989; Lesser et al., 2022). Despite this, L. hawaiiensis, P. speciosa and L. scabra had Δ13C values close to zero, suggesting reliance on symbiont-derived carbon and efficient carbon cycling (Muscatine et al., 1989). Pachyseris speciosa is primarily more autotrophic (Veron, 2000) and does not increase heterotrophy over depth in another Indian Ocean location (Radice et al., 2019a; Radice et al., 2019b; Radice et al., 2022), thus this species provides good indication that δ13C interpretations of autotrophy in this study are robust.
In contrast, L. glabra and C. levis had higher distinctions between their host and symbiont δ13C (Δ13C = 1.4 ± 1 ‰ and 3.5 ± 1.7 ‰, respectively), indicating greater assimilations of externally-derived nutrients, likely in compensation for low photosynthetic carbon assimilation (Swart, 1983; Reynaud et al., 2002; Palardy et al., 2008; Lesser et al., 2010). Host δ13C of these species were also within the ranges of small plankton (-17 ‰ to -21 ‰) (Land et al., 1975; Fontugne and Claude Duplessy, 1978; Rodrigues and Grottoli, 2006). The capacity for heterotrophic prey uptake is species-specific and subject to food availability (Ferrier-Pagès et al., 2003; Tremblay et al., 2015). High chlorophyll-a concentrations at the sampling depths (~1.5 mg m-3) suggest rich availability of particulate matter in the mesophotic at Ashmore Reef (i.e., phytoplankton) that would support zooplankton (Kiørboe, 2011), particularly compared to other mesophotic ecosystems (e.g., 0.6 - 1 mg m-3) (Bridge et al., 2011; Ziegler et al., 2015). Thus, it is likely that corals in this oceanic reef are acquiring higher levels of plankton to meet metabolic demands. The mixotrophic abilities of scleractinian zooxanthellate corals, however, comprise a range across a spectrum. Clear separation of L. glabra isotopic niches driven by δ13C suggest the reliance for heterotrophy in this species exceeds that of C. levis, which shows slight overlap.
Nitrogen is essential for maintaining symbiont growth, photosynthesis and overall holobiont health in corals (Falkowski et al., 1993; Radecker et al., 2015). Lower Δ15N values were recorded in corals exhibiting greater heterotrophic inputs of carbon (L. glabra, C. levis) compared to corals assimilating more photosynthates (L. hawaiiensis, P. speciosa, L. scabra). In typical food webs, consumers exhibit a stepwise enrichment of δ15N and as such, can be used to determine trophic position (Minagawa and Wada, 1984; Lesser et al., 2010). If applying this method to corals, we would expect the host tissues to have significantly higher δ15N than its symbiont. Yet, the effects of acquiring nitrogen from multiple sources (Mullin et al., 1984) and effective nitrogen recycling within the holobiont (Reynaud et al., 2009; Tremblay et al., 2015; Rangel et al., 2019) complicates the application of a coral trophic enrichment factor that is likely lower (0 - 2 ‰) than in other animals (Radice et al., 2022).
In addition to particulate matter capture, corals obtain nitrogen through the uptake of dissolved inorganic nitrogen (DIN) in the forms of ammonium and nitrate (Mullin et al., 1984; Grover et al., 2002). Ammonium is preferred by corals (Bythell, 1990), however, nitrate often increases in availability from shallow to mesophotic depths (Mullin et al., 1984; Morrow et al., 2016; Radice et al., 2021). Ammonium is depleted in 15N (Miyake and Wada, 1967), thus it would be expected that its acquisition decreases coral δ15N. The δ15N of nitrate is generally correlated to its concentration levels (Erler et al., 2015; Isaji et al., 2022), but deep-water nitrate typically fall between ~5 – 7 ‰ at subsurface depths (< 200 m) because of the deep-water oceanic pool (Sigman et al., 2009; Sigman and Fripiat, 2019; Isaji et al., 2022). When considering that (1) DIN is mostly acquired and used by the symbionts (Muscatine and D’Elia, 1978; Pernice et al., 2012), and (2) the uptake rates of ammonium can be significantly higher in more autotrophic vs. more heterotrophic corals (Grover et al., 2002) - the acquisition of both dissolved inorganic nitrate and ammonium can explain higher host vs. symbiont δ15N values in photosynthate-dependent corals (L. hawaiiensis, P. speciosa and L. scabra) (Δ15N =1.2 to 2.3 ‰).
By contrast, the very similar host and symbiont δ15N values in L. glabra and C. levis demonstrate tight holobiont nitrogen recycling (Δ15N = 0.3 - 0.5 ‰) (Reynaud et al., 2009; Rangel et al., 2019), with Δ15N differences being significant between L. glabra (more heterotrophic) and L. hawaiiensis (more autotrophic). Nitrogen can be translocated between the host and symbiont within hours (Tremblay et al., 2015), which may become particularly important if light limitation affects symbiont nitrogen uptake. As plankton ingestion can result in the synthesis of over three times more nitrogen than DIN (Hoegh-Guldberg and Williamson, 1999; Grover et al., 2002), it is hypothesized that heterotrophic nitrogen acquisition from plankton or particulate organic matter could provide a metabolic advantage to the uptake of dissolved nitrogen. If symbiont density decreases over depth (Ziegler et al., 2015) with lower photosynthate reliance, this may in turn reduce overall nitrogen needs, allowing the recycling process of heterotrophically-derived nitrogen (Ferrier-Pagès et al., 2021) to meet overall holobiont demands (as seen in L. glabra and C. levis in this study). Interestingly, L. hawaiiensis and L. scabra may increase symbiont density over depth (Padilla-Gamiño et al., 2019). This could further explain why the more autotrophic-dependent species in our study exhibited less efficient nitrogen recycling likely due to reliance on DIN to meet higher nitrogen demands, which has a high turnover in coral cells (Krueger et al., 2018), and may be less effective metabolically (Hoegh-Guldberg and Williamson, 1999; Grover et al., 2002).
Our analysis has highlighted some constraints in understanding trophic ecology in mesophotic corals using common techniques used for interpreting δ13C and δ15N. The correlations seen in this study between host and symbiont Δ13C and Δ15N were not analogous. In other studies, corals that have exhibited very similar host and symbiont δ13C (i.e., reliant on photosynthates - Peterson and Fry, 1987; Muscatine et al., 1989), have also displayed differentiated δ15N values. For example, Porites evermanni (Δ13C = -0.13 ± 0.47 ‰ and Δ15N = -1.79 ± 0.68 ‰) (Price et al., 2021), Platygyra spp., (Δ13C = -0.70 ± 0.46 ‰ and Δ15N = 2.50 ± 1.20 ‰), Favites spp., (Δ13C = -0.50 ± 0.52 ‰ and Δ15N = 2.50 ± 1.39 ‰) (Conti-Jerpe et al., 2020), and Montastraea cavernosa that has an isotopic niche differentiation driven by Δ15N but not Δ13C at mesophotic depths (Lesser et al., 2022). Yet, these particular metrics (Δ13C values close to 0, and higher Δ15N values) are generally considered evidence of increasing heterotrophy (e.g., Conti-Jerpe et al., 2020; Price et al., 2021).
Furthermore, Δ15N values close to zero have also been recorded in species with larger Δ13C (i.e., Δ13C values that indicate external carbon acquisition), such as Montipora capitata where Δ13C = 1.53 ± 0.63 and Δ15N = 0.09 ± 0.50 ‰ (Price et al., 2021), and Stylophora pistillata whereby its isotopic niche differentiation was driven by Δ13C but not by Δ15N at mesophotic depths (Lesser et al., 2022). Yet, these metrics (higher Δ13C with lower Δ15N) are commonly assessed to be primarily autotrophic (e.g., Price et al., 2021).
The methods used to interpret stable isotope data in the context of coral trophic ecology include (1) calculating the differences between host and symbiont δ13C (Δ13C or δ13Chost-symbionts), whereby a more negative value is said to indicate greater heterotrophic contribution (Muscatine et al., 1989; Price et al., 2021), (2) calculating the differences between host and symbiont δ15N (Δ15N or δ15Nhost-symbionts), where by higher values denote heterotrophy) (e.g., Conti-Jerpe et al., 2020; Price et al., 2021; Lesser et al., 2022) and (3) SIBER Bayesian models, whereby a greater differentiation in isotopic niches of δ13C and δ15N indicate greater heterotrophic reliance (Jackson et al., 2011; Conti-Jerpe et al., 2020).
The Δ13C metric does not appear to work the same for corals in low light environments such as in our study. This metric relies on the coral host becoming more depleted in δ13C due to external carbon sources being more depleted in δ13C than the coral host and symbiont. This is typically true for corals in shallow light environments and even many mesophotic environments. Yet, under a more extreme light condition, symbiont δ13C can be extremely negative (e.g., this study, -22 to -23 ‰), and external food sources, such as plankton and particulate matter, could likely be more enriched than the photosynthetic carbon source (i.e., the symbiont) (Land et al., 1975; Fontugne and Claude Duplessy, 1978; Rodrigues and Grottoli, 2006) although plankton was not assessed in our study. Alternatively, for mesophotic corals, Δ13C values that deviate from zero may denote a significant input of carbon sources external to the holobiont, despite whether Δ13C is, for example, +2 ‰ vs. -2 ‰.
The Δ15N metric relies on the stepwise process of δ15N enrichment in the coral host consumer (Minagawa and Wada, 1984), much like the latter approach (SIBER), where differentiation in coral host and symbiont isotopic niches can be driven individually by δ15N (with little δ13C differentiation) and be labelled as heterotrophic. The fact that corals assimilate DIN (which can vary in δ15N), and also recycle both heterotrophic and autotrophic nitrogen within the holobiont, makes this metric particularly challenging to assess. In addition, a recent re-analysis of pooled data from previously published studies (Lesser et al., 2022) found no overall trends between δ15N and heterotrophy in mesophotic corals, and also symbiont type can influence DIN assimilation in relation to light availability (Ezzat et al., 2017). Thus, we argue that using δ15N as an indication of heterotrophy is not consistent across coral species and depths, particularly in mesophotic environments, and δ13C appears to be the primary determinant of mixotrophy in these ecosystems.
Protein concentration often decreases over depth in autotrophic corals (Cooper et al., 2011a; Ziegler et al., 2015), yet the capacity for heterotrophic carbon to promote tissue generation (Krueger et al., 2018) and higher protein levels (Grover et al., 2002; Houlbrèque et al., 2004; Rodrigues and Grottoli, 2006; Reynaud et al., 2009; Hoogenboom et al., 2015) suggests heterotrophic feeding at depth could benefit overall tissue protein and biomass. However, our coral samples were from much lower irradiances than other similar studies and protein concentrations showed no obvious patterns in relation to trophic strategy.
The trends we observed were consistent with species-specific protein concentrations observed by Brunner, 2015 where Leptoseris scabra had significantly higher host tissue protein concentrations than P. speciosa, despite both having similar nutrient acquisition strategies. Over depth, P. speciosa maintains a single symbiont genus (Cladocopium) with reduced photosynthetic energy (Cooper et al., 2011a), typically accompanied by reductions in tissue biomass (Cooper et al., 2011a; Brunner, 2015) and symbiont density (Brunner, 2015). By contrast, L. scabra shows the ability to maintain relatively high protein concentrations, likely as a result of specialized physiological adaptations (Brunner, 2015; Pochon et al., 2015; Padilla-Gamiño et al., 2019) and highlights the acclimatory mechanisms depth-specialists use to maximize the use of nutrients available at depth. Interestingly, all Leptoseris species examined herein do not significantly decrease protein concentrations with depth (Brunner, 2015). For example, at 80 m in the Coral Sea, P. speciosa had protein concentrations of approximately 0.9 mg cm2 (following continual decline), compared to L. scabra (1.25 mg cm2), even though L. hawaiiensis had less protein at this depth (0.75 mg cm2) than P. speciosa (Brunner, 2015) (i.e., a much brighter light environment than this study). Given this, it is likely that the low protein values reported in this study reflect reducing protein synthesis capabilities for P. speciosa at end-photic irradiances (Cooper et al., 2011a; Brunner, 2015).
The light environment at Ashmore Reef attenuated strongly (1% at 54 m, PARkd = 0.069 m-1) compared to other regions such as Bermuda and the Bahamas, where the same light availability occurs at 100 m and 81 m, respectively (Fricke et al., 1987; Lesser et al., 2010). This likely reflects the rich phytoplankton abundances present in the highly productive mesophotic ecosystems at Ashmore Reef (Kiørboe, 2011; Kahng et al., 2019). Similar end-photic depths have been observed in other highly productive systems such as the Maldives, where only 4% of surface irradiance was measured at 30 m (Radice et al., 2019a).
Our results build on information that implies P. speciosa is an obligate autotroph with restricted trophic plasticity over depth (Radice et al., 2019a; Radice et al., 2019b; Radice et al., 2022). This likely limits the depth distribution in this species and the fact that colonies were only found shallower than 60 m supports the notion of P. speciosa as a depth-generalist coral (Chow et al., 2019). Other studies also found P. speciosa typically at 60 m and shallower, with deeper observations only occurring in MCEs with exceptionally low light attenuation (e.g., the Coral Sea and French Polynesia) (Englebert et al., 2014; Pichon, 2019; Bongaerts et al., 2021). In contrast, the irradiance level at 60 m at Ashmore Reef is equivalent to light conditions beyond the photic point (1% SI) (i.e., deeper than 60 m at other locations), which suggests that P. speciosa may be capable of inhabiting lower mesophotic depths at other locations. The ability for this species to remain primarily autotrophic in such extreme light conditions is likely permitted through significantly reduced tissue mass and symbiont density (Brunner, 2015) and possibly specialized intracellular arrangements (i.e., monolayer to reduce shading) (Schlichter et al., 1986; Kahng et al., 2019) that allow it to acclimatize to lower photosynthate assimilation.
The variation in carbon and nitrogen sources was most distinct between L. hawaiiensis and L. glabra (significantly different Δ13C and symbiont Δ15N), highlighting the species-specific occupation of different ecological niches despite living in the same environments. Leptoseris species are specialized to inhabit light environments approaching photic limits and can maintain metabolic demands via heterotrophy at these low irradiances (Fricke et al., 1987; Padilla-Gamiño et al., 2019), as demonstrated by L. glabra in this study. Research by Padilla-Gamiño et al. (2019) highlighted differences in physiological adaptations between Leptoseris species also examined in this study, whereby L. scabra increased photosynthetic pigments with depth while L. hawaiiensis employed high pigment content across low symbiont densities. Symbiont type can influence photo-adaptation (Ziegler et al., 2015; Padilla-Gamiño et al., 2019) and symbiont plasticity demonstrated by Leptoseris spp. in other studies and could indicate photoautotrophic benefits over depth (Cooper et al., 2011a; Padilla-Gamiño et al., 2019; Martinez et al., 2020), compared to P. speciosa which maintains one symbiont genus over depth with reduced photosynthetic productivity (Cooper et al., 2011b; Ziegler et al., 2015). These adaptations likely enable L. scabra and L. hawaiiensis to maintain higher protein contents at depth without significant heterotrophic input, compared to the P. speciosa. Further studies are required to assess the specific physiological adaptations among mesophotic species at Ashmore Reef. This study provides the first assessment of trophic ecology in L. glabra and C. levis, hence no comparative data are available for assessing photosynthetic capacity in these species.
This study provides new insight into the ecophysiology of mesophotic corals in the Eastern Indian Ocean region. The finding that this system has relatively shallow photic end points possibly due to a high level of productivity provides an interesting contrast to other mesophotic systems in Australia such as the Coral Sea. Both depth-specialist and depth-generalist corals demonstrated the ability to remain photosynthetically reliant in just a fraction of surface irradiance. The role of nitrogen recycling in the coral holobiont is not fully understood, particularly between different trophic strategies in limited light environments, and this research provides interesting insight into potential links between heterotrophically-derived nitrogenous benefits and species-specific nitrogen demands at depth. Nevertheless, further studies are required to broaden the understanding of nutrient acquisition and allocation in mesophotic corals, and deeper investigations into how external sources and internal processes influence δ15N under autotrophic and heterotrophic reliance in low light is warranted.
Overall, this study highlights the remarkable capacity of mesophotic Scleractinia to optimize their physiology in light limited environments and provides insight into potential acclimation limits for depth specialist and generalist species. Additionally, this is the first investigation of nutrient acquisition or protein content in C. levis and demonstrates its requirement to obtain nutrients mixotrophically in low light environments. These findings contribute to the narrow yet growing pool of literature on mesophotic coral ecophysiology and underscore the need for further research that betters our understanding of how various nutrient inputs affect coral physiology and stable isotopes δ13C and δ15N at depth.
The raw data supporting the conclusions of this article will be made available by the authors, without undue reservation.
ZR and KMi conceived the project and secured funding. AC, VR, ZR, and KMc conducted experimental and analytical design. AC conducted the field work and KMi was the Chief Scientist on the field expedition. AC and AH carried out laboratory work. ZR performed all coral taxonomy. AC and VR performed statistical analyses and drafted the manuscript. All authors conducted manuscript edits. All authors contributed to the article and approved the submitted version.
This study was funded by the ARC Linkage Grant LP160101508 awarded to Zoe Richards and the Schmidt Oceans Institute, through support of RV Falkor Voyage FK210409, and by the Australian Institute of Marine Science and Parks Australia through the collaborative project (to KM) for the 2021 Ashmore Reef Mesophotic Reef Survey and Sampling Project.
Firstly, we would like to acknowledge the Traditional Custodians of the land on which we conduct all scientific research, and pay respect to their Elders past, present and future. Schmidt Ocean Institute (SOI), the Australian Institute of Marine Science (AIMS) and Parks Australia who funded the field surveys. A special thanks to the scientists and crew aboard the Falkor Expedition, including all tech crew responsible for operating the R/V Falkor, ROV Subastian and CTD, to Dr. Nerida Wilson and Corey Whisson of the WA Museum, and to Declan Stick and Chloe Anderson for support in the field. A great thank you to contributing parties Gaewyn Ellison and Dr. Mark Hackett for laboratory support, Dr. Paul Muir for support during project conception and Dr. Michel Pichon for verifying coral identifications. Thanks to Oliver Gomez for technical support at the WA Museum and members of the Coral Conservation and Research Group in the Trace and Environmental DNA Laboratory at Curtin University.
The authors declare that the research was conducted in the absence of any commercial or financial relationships that could be construed as a potential conflict of interest.
All claims expressed in this article are solely those of the authors and do not necessarily represent those of their affiliated organizations, or those of the publisher, the editors and the reviewers. Any product that may be evaluated in this article, or claim that may be made by its manufacturer, is not guaranteed or endorsed by the publisher.
The Supplementary Material for this article can be found online at: https://www.frontiersin.org/articles/10.3389/fmars.2023.1089746/full#supplementary-material
Alamaru A., Loya Y., Brokovich E., Yamd R., Shemesh A. (2009). Carbon and nitrogen utilization in two species of red Sea corals along a depth gradient: insights from stable isotope analysis of total organic material and lipids. Geochimica Cosmochimica Acta 73, 5333–5342. doi: 10.1016/j.gca.2009.06.018
Anthony K. R. N., Fabricius K. E. (2000). Shifting roles of heterotrophy and autotrophy in coral energetics under varying turbidity. J. Exp. Mar. Biol. Ecol. 252, 221–253. doi: 10.1016/S0022-0981(00)00237-9
Armstrong R. A., Pizarro O., Roman C. (2019). “Underwater robotic technology for imaging mesophotic coral ecosystems,” in Mesophotic coral ecosystems. coral reefs of the world. Eds. Loya Y., Puglise K., Bridge T. (Cham: Springer).
Arrigoni R., Berumen M. L., Stolarski J., Terraneo T. I., Benzoni F. (2019). Uncovering hidden coral diversity: a new cryptic lobophylliid scleractinian from the Indian ocean. Cladistics 35, 301–328. doi: 10.1111/cla.12346
Baker A. C. (2003). Flexibility and specificity coral-algal symbiosis: diversity, ecology, and biogeography of symbiodinium. Annu. Rev. Ecology Evolution Systematics 34, 661–689. doi: 10.1146/annurev.ecolsys.34.011802.132417
Ben-David M., Flaherty E. A. (2012). Stable isotopes in mammalian research: a beginner's guide. J. Mammalogy 92, 312–328. doi: 10.1644/11-MAMM-S-166.1
Benzoni F., Arrigoni R., Stefani F., Stolarski J. (2012). Systematics of the coral genus Craterastrea (Cnidaria, anthozoa, scleractinia) and description of a new family through combined morphological and molecular analyses. Systematics Biodiver. 10, 417–433. doi: 10.1080/14772000.2012.744369
Bhagooli R., Mattan-Moorgawa S., Kaullysing D., Louis Y. D., Gopeechund A., Ramah S., et al. (2021). Chlorophyll fluorescence – a tool to assess photosynthetic performance and stress photophysiology in symbiotic marine invertebrates and seaplants. Mar. pollut. Bull. 165, 112059–112059. doi: 10.1016/j.marpolbul.2021.112059
Bongaerts P., Cooke I. R., Ying H., Wels D., Haan Den S., Hernandez-Agreda A., et al. (2021). Morphological stasis masks ecologically divergent coral species on tropical reefs. Curr. Biol. 31, 2286–2298.e8. doi: 10.1016/j.cub.2021.03.028
Bongaerts P., Frade P. R., Hay K. B., Englebert N., Latijnhouwers K. R. W., Bak R. P. M., et al. (2015). Deep down on a Caribbean reef: lower mesophotic depths harbor a specialized coral-endosymbiont community. Sci. Rep. 5 (1), 7652. doi: 10.1038/srep07652
Bongaerts P., Smith T. B. (2019). “Beyond the “Deep reef refuge” hypothesis: a conceptual framework to characterize persistence at depth,” in Mesophotic coral ecosystems. coral reefs of the world. Eds. Loya Y., Puglise K., Bridge T. (Cham: Springer Nature).
Brakel W. H. (1979). Small-scale spatial variation in light available to coral reef benthis: quantum irradiance measurements from a Jamaican reef. Bull. Mar. Sci. 29, 406–413.
Bridge T. C. L., Done T. J., Beaman R. J., Friedman A., Williams S. B., Pizarro O., et al. (2011). Topography, substratum and benthic macrofaunal relationships on a tropical mesophotic shelf margin, central great barrier reef, Australia. Coral Reefs 30, 143–153. doi: 10.1007/s00338-010-0677-3
Brunner C. A. (2015). Physiological adaptations of mesophotic corals across a depth gradient – leptoseris spp. & pachyseris speciosa (Scleractinia: agariciidae) (Bremen, Germany: Universität Bremen).
Bythell J. C. (1990). Nutrient uptake in the reef-building coral Acropora palmata at natural environmental concentrations. Mar. Ecol. Prog. Ser. 68, 65–69. doi: 10.3354/meps068065
Chow G. S. E., Chan Y. K. S., Jain S. S., Huang D. (2019). Light limitation selects for depth generalists in urbanised reef coral communities. Mar. Environ. Res. 147, 101–112. doi: 10.1016/j.marenvres.2019.04.010
Conti-Jerpe I. E., Thompson P. D., Wong C. W. M., Oliveira N. L., Duprey N. N., Moynihan M. A., et al. (2020). Trophic strategy and bleaching resistance in reef-building corals. Sci. Adv. 6, eaaz5443–eaaz5443. doi: 10.1126/sciadv.aaz5443
Cooper T. F., Karin E. U., Sana S. D., Andrew J. H., Michael K., Andrew M., et al. (2011a). Niche specialization of reef-building corals in the mesophotic zone: metabolic trade-offs between divergent symbiodinium types. Proc. Biol. Sci. 278, 1840–1850. doi: 10.1098/rspb.2010.2321
Cooper T. F., Lai M., Ulstrup K. E., Saunders S. M., Flematti G. R., Radford B., et al. (2011b). Symbiodinium genotypic and environmental controls on lipids in reef building corals. PLoS One 6, e20434. doi: 10.1371/journal.pone.0020434
Coplen T. B. (2011). Guidelines and recommended terms for expression of stable-isotope-ratio and gas-ratio measurement results. Rapid Commun. Mass Spectrometry 25, 2538–2560. doi: 10.1002/rcm.5129
Coplen T. B., Brand W. A., Gehre M., Gröning M., Meijer H. A. J., Toman B., et al. (2006). New guidelines for δ13C measurements. Analytical Chem. 78, 2439–2441. doi: 10.1021/ac052027c
Crandall J. B., Teece M. A., Estes B. A., Manfrino C., Ciesla J. H. (2016). Nutrient acquisition strategies in mesophotic hard corals using compound specific stable isotope analysis of sterols. J. Exp. Mar. Biol. Ecol. 474, 133–141. doi: 10.1016/j.jembe.2015.10.010
Dinesen Z. D. (1980). A revision of the coral genus Leptoseris (Scleractinia: fungiia: agariciidae). Mem Queens Mus 20, 181–235. Available at: https://biostor.org/reference/151870.
Einbinder S., Mass T., Brokovich E., Dubinsky Z., Erez J., Tchernov D. (2009). Changes in morphology and diet of the coral Stylophora pistillata along a depth gradient. Mar. Ecol. Prog. Ser. 381, 167–174. doi: 10.3354/meps07908
Englebert N., Bongaerts P., Muir P., Hay K. B., Hoegh-Gulberg O. (2014). Deepest zooxanthellate corals of the great barrier reef and coral Sea. Mar. Biodiver. 45, 1–2. doi: 10.1007/s12526-014-0221-8
Englebert N., Bongaerts P., Muir P. R., Hay K. B., Pichon M., Hoegh-Guldberg O. (2017). Lower mesophotic coral communities (60-125m depth) of the northern great barrier reef and coral Sea. PLoS One 12, e0170336. doi: 10.1371/journal.pone.0170336
Erler D. V., Wang X. T., Sigman D. M., Scheffers S. R., Shepherd B. O. (2015). Controls on the nitrogen isotopic composition of shallow water corals across a tropical reef flat transect. Coral Reefs 24, 329–338. doi: 10.1007/s00338-014-1215-5
Eyal G., Laverick J. H., Bongaerts P., Levy O., Pandolfi J. M. (2021). Mesophotic coral ecosystems of the great barrier reef are understudied and underexplored. Front. Mar. Sci. 8, 622856. doi: 10.3389/fmars.2021.622856.
Ezzat L., Fine M., Grover R., Ferrier-Pagés C. (2017). Carbon and nitrogen acquisition in shallow and deep holobionts of the scleractinian coral S. pistillata. Front. Mar. Sci. 4. doi: 10.3389/fmars.2017.00102
Falkowski P. G., Dubinsky Z., Muscatine L., Mccloskey L. (1993). Population control in symbiotic corals. BioScience 43, 606–611. doi: 10.2307/1312147
Farquhar G. D., Ehleringer J. R., Hubick K. T. (1989). Carbon isotope discrimination and photosynthesis. Annu. Rev. Plant Physiol. Plant Mol. Biol. 40, 503–537. doi: 10.1146/annurev.pp.40.060189.002443
Ferrier-Pagès C., Bednarz V., Grover R., Benayahu Y., Maguer J. F., Rottier C., et al. (2021). Symbiotic stony and soft corals: is their host-algae relationship really mutualistic at lower mesophotic reefs? Limnology Oceanogr. 67, 261–271. doi: 10.1002/lno.11990
Ferrier-Pagès C., Peirano A., Abbate M., Cocito S., Negri A., Rottier C., et al. (2011). Summer autotrophy and winter heterotrophy in the temperate symbiotic coral Cladocora caespitosa. Limnology Oceanogr. 56, 1429–1438. doi: 10.4319/lo.2011.56.4.1429
Ferrier-Pagès C., Witting J., Tambutté E., Sebens K. P. (2003). Effect of natural zooplankton feeding on the tissue and skeletal growth of the scleractinian coral Stylophora pistillata. Coral Reefs 22, 229–240. doi: 10.1007/s00338-003-0312-7
Fontugne M., Claude Duplessy J. (1978). Carbon isotope ratio of marine plankton related to surface water masses. Earth Planetary Sci. Lett. 41, 365–371. doi: 10.1016/0012-821X(78)90191-7
Fricke H. W., Vareschi E., Schlichter D. (1987). Photoecology of the coral Leptoseris fragilis in the red Sea twilight zone (an experimental study by submersible). Oecologia 73, 371–381. doi: 10.1007/BF00385253
Genty B., Briantais J.-M., Baker N. R. (1989). The relationship between the quantum yield of photosynthetic electron transport and quenching of chlorophyll fluorescence. Biochim. Biophys. Acta (BBA) - Gen. Subj. 990, 87–92. doi: 10.1016/S0304-4165(89)80016-9
Gilmour J. P., Cook K. L., Ryan N. M., Puotinen M. L., Green R. H., Shedrawi G., et al. (2019). The state of Western australia’s coral reefs. Coral Reefs 38, 651–667. doi: 10.1007/s00338-019-01795-8
Glynn P. W. (1996). Coral reef bleaching: facts, hypotheses and implications. Global Change Biol. 2, 495–509. doi: 10.1111/j.1365-2486.1996.tb00063.x
Grover R., Maguer J.-F., Reynaud-Vaganay S., Ferrier-Pagès C. (2002). Uptake of ammonium by the scleractinian coral Stylophora pistillata: effect of feeding, light, and ammonium concentrations. Limnology Oceanogr. 47, 782–790. doi: 10.4319/lo.2002.47.3.0782
Heikoop J. M., Dunn J., Risk M. J., Sanderman I. M., Schwarcz H., Waltho N. (1998). Relationship between light and the δ15N of coral tissue: examples from Jamaica and Zanzibar. Limnology Oceanogr. 43, 909–920. doi: 10.4319/lo.1998.43.5.0909
Heyward A., Radford B. (2019). “Northwest Australia,” in Mesophotic coral ecosystems. Eds. Loya Y., Puglise K., Bridge T. (Cham: Springer).
Hoegh-Guldberg O. (1988). A method for determining the surface area of corals. Coral Reefs 7, 113–116. doi: 10.1007/BF00300970
Hoegh-Guldberg O., Williamson J. (1999). Availability of two forms of dissolved nitrogen to the coral Pocillopora damicornis and its symbiotic zooxanthellae. Mar. Biol. 133, 561–570. doi: 10.1007/s002270050496
Hoeksema B. W., Bongaerts P., Baldwin C. C. (2017). High coral cover at lower mesophotic depths: a dense Agaricia community at the leeward side of curaçao, Dutch Caribbean. Mar. Biodiver. 47, 67–70. doi: 10.1007/s12526-015-0431-8
Hoogenboom M., Rottier C., Sikorski S., Ferrier-Pagès C. (2015). Among-species variation in the energy budgets of reef-building corals: scaling from coral polyps to communities. J. Exp. Biol. 218, 3866–3877. doi: 10.1242/jeb.124396
Houlbrèque F., Ferrier-Pagès C. (2009). Heterotrophy in tropical scleractinian corals. Biolovical Rev. 84, 1–17. doi: 10.1111/j.1469-185X.2008.00058.x
Houlbrèque F., Tambutte E., Ferrier-Pagès C. (2003). Effect of zooplankton availability on the rates of photosynthesis, and tissue and skeletal growth in the scleractinian coral Stylophora pistillata. J. Exp. Mar. Biol. Ecol. 292, 145–166. doi: 10.1016/S0022-0981(03)00259-4
Houlbrèque F., Tambutté E., Allemand D., Ferrier-Pagès C. (2004). Interactions between zooplankton feeding, photosynthesis and skeletal growth in the scleractinian coral Stylophora pistillata. J. Exp. Biol. 207, 1461–1469. doi: 10.1242/jeb.00911
Isaji Y., Yoshikawa C., Ogawa N. O., Matsumoto K., Makabe A., Toyoda S., et al. (2022). Nitrogen sources for phytoplankton in the Eastern Indian ocean determined from δ15N of chlorophyll a and divinyl chlorophyll a. Geochem. Geophysics Geosystems 23, e2021GC010057. doi: 10.1029/2021GC010057
Jackson A. L., Inger R., Parnell A. C., Bearhop S. (2011). Comparing isotopic niche widths among and within communities: SIBER – stable isotope Bayesian ellipses in R. J. Anim. Ecol. 80, 595–602. doi: 10.1111/j.1365-2656.2011.01806.x
Kahng S. E., Akkaynak D., Shlesinger T., Hochberg E. J., Wiedenmann J., Tamir R., et al. (2019). “Light, temperature, photosynthesis, heterotrophy, and the lower depth limits of mesophotic coral ecosystems,” in Mesophotic coral ecosystems (Cham: Springer International Publishing).
Kahng S. E., Copus J. M., Wagner D. (2014). Recent advances in the ecology of mesophotic coral ecosystems (MCEs). Curr. Opin. Environ. Sustainability 7, 71–81. doi: 10.1016/j.cosust.2013.11.019
Kiørboe T. (2011). How zooplankton feed: mechanisms, traits and trade-offs. Biol. Rev. 86, 311–339. doi: 10.1111/j.1469-185X.2010.00148.x
Kirk J. T. O. (2011). Light and photosynthesis in aquatic ecosystems. 3rd ed (New York: Cambridge University Press).
Krueger T., Bodin J., Horwitz N., Loussert-Fonta C., Sakr A., Escrig S., et al. (2018). Temperature and feeding induce tissue level changes in autotrophic and heterotrophic nutrient allocation in the coral symbiosis – a NanoSIMS study. Sci. Rep. 8, 12710. doi: 10.1038/s41598-018-31094-1
Land L. S., Lang J. C., Smith B. C. (1975). Preliminary observations on the carbon isotopic composition of some reef coral tissues and symbiotic zooxanthellae. Limnology Oceanogr. 20, 283–287. doi: 10.4319/lo.1975.20.2.0283
Laverick J. H., Piango S., Andradi-Brown D. A., Exton D. A., Bongaerts P., Bridge T. C. L., et al. (2018). To what extent do mesophotic coral ecosystems and shallow reefs share species of conservation interest? a systematic review. Environ. Evidence 7, 15. doi: 10.1186/s13750-018-0127-1
Lesser M. P., Falcón L. I., Rodríguez-Román A., Enríquez S., Hoegh-Guldberg O., Iglesias-Prieto R. (2007). Nitrogen fixation by symbiotic cyanobacteria provides a source of nitrogen for the scleractinian coral montastraea cavernosa. Mar. Ecol. Prog. Ser. 346, 143–152. doi: 10.3354/meps07008
Lesser M. P., Slattery M., Laverick J. H., Macartney K. J., Bridge T. C. (2019). Global community breaks at 60 m on mesophotic coral reefs. Global Ecol. Biogeogr. 28, 1403–1416. doi: 10.1111/geb.12940
Lesser M. P., Slattery M., Macartney K. J. (2022). Using stable isotope analyses to assess the trophic ecology of scleractinian corals. Oceans 3, 527–546. doi: 10.3390/oceans3040035
Lesser M. P., Slattery M., Stat M., Ojimi M., Gates R. D., Grottoli A. (2010). Photo accolimatization by the coral Montastraea cavernosa in the mesophotic zone: light, food, and genetics. Ecology 91, 990–1003. doi: 10.1890/09-0313.1
Lewis J. B., Price W. S. (1975). Feeding mechanisms and feeding strategies of Atlantic reef corals. J. Zoology 176, 527–544. doi: 10.1111/j.1469-7998.1975.tb03219.x
López-Londoño T., Gómez-Campo K., Hernández-Pech X., Enríquez S., Iglesias-Prieto R. (2022). Photosynthetic usable energy explains vertical patterns of biodiversity in zooxanthellate corals. Sci. Rep. 12, 20821. doi: 10.1038/s41598-022-25094-5
Loya Y., Eyal G., Treibitz T., Lesser M. P., Appeldoorn R. (2016). Theme section on mesophotic coral ecosystems: advances in knowledge and future perspectives. Coral Reefs 35, 1–9. doi: 10.1007/s00338-016-1410-7
Loya Y., Puglise K. A., Bridge T. C. K. (2019). Mesophotic coral ecosystems (Switzerland: Springer Nature).
Marsh J. A. (1970). Primary productivity of reef-building calcareous red algae. Ecology 51, 255–263. doi: 10.2307/1933661
Martinez S., Kolodny Y., Shemesh E., Scucchia F., Nevo R., Levin-Zaidman S., et al. (2020). Energy sources of the depth-generalist mixotrophic coral Stylophora pistillata. Front. Mar. Sci. 7. doi: 10.3389/fmars.2020.566663
Mass T., Kline D. I., Roopin M., Veal C. J., Cohen S., Iluz D., et al. (2010). The spectral quality of light is a key driver of photosynthesis and photoadaptation in Stylophora pistillata colonies from different depths in the red Sea. J. Exp. Biol. 213, 4084–4091. doi: 10.1242/jeb.039891
McCree K. J. (1981). “Photosynthetically active radiation,” in Physiological plant ecology I: responses to the physical environment. Eds. Lange O. L., Nobel P. S., Osmond C. B., Ziegler H. (Berlin, Heidelberg: Springer Berlin Heidelberg).
Minagawa M., Wada E. (1984). Stepwise enrichment of δ15N along food chains: further evidence and the relation between δ15N and animal age. Geochimica Cosmochimica Acta 48, 1135–1140. doi: 10.1016/0016-7037(84)90204-7
Miyake Y., Wada E. (1967). The abundance ratio of 15N/14N in marine environments. Records Oceanogrphic Works Japan 9.
Morrow K. M., Fiore C. L., Lesser M. P. (2016). Environmental drivers of microbial community shifts in the giant barrel sponge, Xestospongia muta, over a shallow to mesophotic depth gradient. Environ. Microbiol. 18, 2025–2038. doi: 10.1111/1462-2920.13226
Muir P. R., Pichon M. (2019). “Biodiversity of reef-building scleractinian corals,” in Mesophotic coral ecosystems. Eds. Loya Y., Puglise K. A., Bridge T. (Switzerland: Springer Nature).
Muir P., Wallace C., Bridge T. C. L., Bongaerts P. (2015). Diverse staghorn coral fauna on the mesophotic reefs of north-east Australia. PLoS One 10, e0117933–e0117933. doi: 10.1371/journal.pone.0117933
Muller-Parker G., D'Elia C. F., Cook C. B. (2015). Interactions between corals and their symbiotic algae (Dordrecht: Springer).
Mullin M. M., Rau G. H., Eppley R. W. (1984). Stable nitrogen isotopes in zooplankton: some geographic and temporal variations in the north pacific. Limnology Oceanogr. 29, 1267–1273. doi: 10.4319/lo.1984.29.6.1267
Muscatine L., D’Elia C. F. (1978). The uptake, retention, and release of ammonium by reef corals. Limnology oceanogr. 23, 725–734. doi: 10.4319/lo.1978.23.4.0725
Muscatine L., Porter J. W., Kaplan I. R. (1989). Resource partitioning by reef corals as determined from stable isotope composition. Mar. Biol. 100, 185–193. doi: 10.1007/BF00391957
Padilla-Gamiño J. L., Roth M. S., Rodrigues L. J., Bradley C. J., Bidigare R. R., Gates R. D., et al. (2019). Ecophysiology of mesophotic reef-building corals in hawai’i is influenced by symbiont–host associations, photoacclimatization, trophic plasticity, and adaptation. Limnology Oceanogr. 64, 1980–1995. doi: 10.1002/lno.11164
Palardy J. E., Rodrigues L. J., Grottolic A. G. (2008). The importance of zooplankton to the daily metabolic carbon requirements of healthy and bleached corals at two depths. J. Exp. Mar. Biol. Ecol. 376, 180–188. doi: 10.1016/j.jembe.2008.09.015
Pernice M., Meibom A., Heuvel A. V. D., Kopp C., Domart-Coulon I., Hoegh-Guldberg O., et al. (2012). A single-cell view of ammonium assimilation in coral–dinoflagellate symbiosis. ISME J. 6, 1314–1324. doi: 10.1038/ismej.2011.196
Peterson B. J., Fry B. (1987). Stable isotopes in ecosystem studies. Annu. Rev. Ecology Evolution Systematics 18, 293–320. doi: 10.1146/annurev.es.18.110187.001453
Pichon M. (2019). French Polynesia. In Mesophotic coral ecosystems. Coral reefs of the world. (Springer), 12, 425–443. doi: 10.1007/978-3-319-92735
Pinheiro H. T., Eyal G., Shepherd B., Rocha L. A. (2019). Ecological insights from environmental disturbances in mesophotic coral ecosystems. Ecosphere 10, e02666–e02n/a. doi: 10.1002/ecs2.2666
Pochon X., Forsman Z. H., Spalding H. L., Padilla-Gamiño J. L., Smith C. M., Gates R. D. (2015). Depth specialization in mesophotic corals (Leptoseris spp.) and associated algal symbionts in hawai'i. R. Soc. Open Sci. 2 (2), 140351. doi: 10.1098/rsos.140351
Price J. T., McLachlan R. H., Jury C. P., Toonen R. J., Grottoli A. G. (2021). Isotopic approaches to estimating the contribution of heterotrophic sources to Hawaiian corals. Limnol. Oceanogr. 66 (6), 2393–2407.
Qi H., Coplen T. B., Geilmann H., Brand W. A., Böhlke J. K. (2003). Two new organic reference materials for δ13C and δ15N measurements and a new value for the δ13C of NBS 22 oil. Rapid Commun. Mass Spectrometry 17, 2483–2487. doi: 10.1002/rcm.1219
Radecker N., Pogoreutz C., Voolstra C. R., Wiedenmann J., Wild C. (2015). Nitrogen cycling in corals: the key to understanding holobiont functioning? Trends Microbiol. 23, 490–497. doi: 10.1016/j.tim.2015.03.008
Radice V. Z., Brett M. T., Fry B., Fox M. D., Hoegh-Guldberg O., DOVE S. G. (2019a). Evaluating coral trophic strategies using fatty acid composition and indices. PLoS One 14, e0222327. doi: 10.1371/journal.pone.0222327
Radice V. Z., Fry B., Brown K. T., Dove S., Hoegh-Guldberg O. (2022). Biogeochemical niches and trophic plasticity of shallow and mesophotic corals recovering from mass bleaching. Limnology Oceanogr. 67, 1617–1630. doi: 10.1002/lno.12157
Radice V. Z., Fry B., Dove S. G., Hoegh-Guldberg O. (2021). Biogeochemical variability and trophic status of reef water column following a coral bleaching event. Coral reefs 40, 1–7. doi: 10.1007/s00338-020-02021-6
Radice V. Z., Hoegh-Guldberg O., Fry B., Fox M. D., Dove S. G. (2019b). Upwelling as the major source of nitrogen for shallow and deep reef-building corals across an oceanic atoll system. Funct. Ecol. 33, 1120–1134. doi: 10.1111/1365-2435.13314
Ralph P. J., Hill R., Doblin M. A., Davy S. K. (2015). “Theory and application of pulse amplitude modulated chlorophyll fluorometry in coral health assessment,” in Diseases of coral. Eds. Cheryl M., Woodley C. M., Downs C. A., Bruckner A. W., Porter J. W., Galloway S. B. (Hoboken, New Jersey, United States: John Wiley & Sons), 506–523.
Ralph P. J., Schreiber U., Gademann R., Kuhl M., Larkum A. W. D. (2005). Coral photobiology studied with a new imaging pulse amplitude modulated fluorometer. J. Phycology 41, 335–342. doi: 10.1111/j.1529-8817.2005.04034.x
Rangel M. S., Erler D., Tagliafico A., Cowden K., Scheffers S., Christidis L. (2019). Quantifying the transfer of prey δ15N signatures into coral holobiont nitrogen pools. Mar. Ecol. Prog. Ser. 610, 33–49. doi: 10.3354/meps12847
Reynaud S., Ferrier-Pagès C., Sambrotto J.-L., Jaubert J., Gattuso J.-P. (2002). Effect of feeding on the carbon and oxygen isotopic composition in the tissues and skeleton of the zooxanthellate coral Stylophora pistillata. Mar. Ecol. Prog. Ser. 238, 81–89. doi: 10.3354/meps238081
Reynaud S., Martinez P., Houlbrèque F. (2009). Effect of light and feeding on the nitrogen isotopic composition of a zooxanthellate coral: role of nitrogen recycling. Mar. Ecol. Prog. Ser. 392, 103–110. doi: 10.3354/meps08195
Richards Z. T., Sampey A., Marsh L. (2014). Kimberley Marine biota. historical data: scleractinian corals. Records Western Aust. Museum Supplement 84, 111–132. doi: 10.18195/issn.0313-122x.84.2014.111-132
Riegl B., Piller W. E. (2003). Possible refugia for reefs in times of environmental stress. Int. J. Earth Sci. 92, 520–531. doi: 10.1007/s00531-003-0328-9
Rodrigues L. J., Grottoli A. G. (2006). Calcification rate and the stable carbon, oxygen, and nitrogen isotopes in the skeleton, host tissue, and zooxanthellae of bleached and recovering Hawaiian corals. Geochimica Cosmochimica Acta 70, 2781–2789. doi: 10.1016/j.gca.2006.02.014
Rouzé H., Galand P. E., Medina M., Bongaerts P., Pichon M., Pérez-Rosales G., et al. (2021). Symbiotic associations of the deepest recorded photosynthetic scleractinian coral (172 m depth). ISME J. 15 (5), 1564–1568. doi: 10.1038/s41396-020-00857-y
RStudio Team (2020). Integrated development for R (Boston, MA: R Studio: PBC). Available at: http://www.RSTUDIO.com/.
Schlichter D., Fricke H. W., Weber W. (1986). Light harvesting by wavelength transformation in a symbiotic coral of the Red Sea twilight zone. Mar. Biol. 91, 403–407. doi: 10.1007/BF00428634
Schreiber U. (2004). “Pulse-Amplitude-Modulation (PAM) fluorometry and saturation pulse method: an overview,” in Chlorophyll a fluorescence: a signature of photosynthesis. Eds. Papageorgiou G. C., Govindjee (Netherlands: Springer).
Sebens K. P., Vandersall K. S., Savina L. A., Graham K. R. (1996). Zooplankton capture by two scleractinian corals, Madracis mirabilis and Montastrea cavernosa, in a field enclosure. Mar. Biol. 127, 303–317. doi: 10.1007/BF00942116
Seemann J. (2013). The use of 13C and 15N isotope labeling techniques to assess heterotrophy of corals. J. Exp. Mar. Biol. Ecol. 442, 88–95. doi: 10.1016/j.jembe.2013.01.004
Seemann J., Carballo-Bolaños R., Berry K. L., González C. T., Richter C., Leinfelder R. R. (2012). “Importance of heterotrophic adaptations of corals to maintain energy reserves,” in Proceedings of the 12th International Coral Reef Symposium, Cairns, Australia, 19A, pp. 9–13. Available at: http://www.icrs2012.com/proceedings/manuscripts/ICRS2012_19A_4.pdf.
Serrano X., Baums I. B., O'reilley K., Smith T. B., Jones R. J., Shearer T. L., et al. (2014). Geographic differences in vertical connectivity in the Caribbean coral Montastraea cavernosa despite high levels of horizontal connectivity at shallow depths. Mol. Ecol. 23, 4226–4240. doi: 10.1111/mec.12861
Sigman D. M., Difiore P. J., Hain M. P., Deutsch C., Wang Y., Karl D. M., et al. (2009). The dual isotopes of deep nitrate as a constraint on the cycle and budget of oceanic fixed nitrogen. Deep-sea Res. Part I Oceanographic Res. papers 56, 1419–1439. doi: 10.1016/j.dsr.2009.04.007
Sigman D. M., Fripiat F. (2019). Nitrogen isotopes in the ocean. Encyclopedia Ocean Sci (Elsevier) 263–278. doi: 10.1016/B978-0-12-409548-9.11605-7
Sturaro N., Hsieh Y. E., Chen Q., Wang P.-L., Denis V. (2019). Toward a standardised protocol for the stable isotope analysis of scleractinian corals. Rapid Commun. Mass Spectrometry 34, e8663. doi: 10.1002/rcm.8663
Sturaro N., Hsieh Y. E., Chen Q., Wang P.-L., Denis V. (2021). Trophic plasticity of mixotrophic corals under contrasting environments. Funct. Ecol. 35, 2841–2855. doi: 10.1111/1365-2435.13924
Sutton D. C., Hoegh-Guldberg O. (1990). Host-zooxanthella interactions in four temperate marine invertebrate symbioses: assessment of effect of host extracts on symbionts. Biol. Bull. 178, 175–186. doi: 10.2307/1541975
Swart P. K. (1983). Carbon and oxygen isotope fractionation in scleractinian corals: a review. Earth-Science Rev. 19, 51–80. doi: 10.1016/0012-8252(83)90076-4
Tamir R., Eyal G., Kramer N., Laverick J. H., Loya Y. (2019). Light environment drives the shallow-to-mesophotic coral community transition. Ecosphere 10 (9), e02839. doi: 10.1002/ecs2.2839
Tremblay P., Maguer J. F., Grover R., Ferrier-Pagès C. (2015). Trophic dynamics of scleractinian corals: stable isotope evidence. J. Exp. Biol. 218, 1223–1234. doi: 10.1242/jeb.115303
Turner J. A., Babcock R. C., Hovey R., Kendrick G. A. (2017). Deep thinking: a systematic review of mesophotic coral ecosystems. ICES J. Mar. Sci. 74, 2309–2320. doi: 10.1093/icesjms/fsx085
Turner J. A., Babcock R. C., Hovey R., Kendrick G. A. (2018). AUV-based classification of benthic communities of the ningaloo shelf and mesophotic areas. Coral Reefs 37, 7630778. doi: 10.1007/s00338-018-1700-3
Veal C. J., Holmes G., Nunez M., Hoegh-Guldberg O., Osborn J. (2010). A comparative study of methods for surface area and threedimensional shape measurement of coral skeletons. Limnology Oceanogr.: Methods 8, 241–253. doi: 10.4319/lom.2010.8.241
Vize P. D. (2006). Deepwater broadcast spawning by Montastraea cavernosa, Montastraea franksi, and Diploria strigosa at the flower garden banks, gulf of Mexico. Coral reefs 25, 169–171. doi: 10.1007/s00338-005-0082-5
Whitaker J. R., Granum P. E. (1980). An absolute method for protein determination based on difference in absorbance at 235 and 280 nm. Analytical Biochem. 109, 156–159. doi: 10.1016/0003-2697(80)90024-X
Zanden M. J. V., Rasmussen J. B. (2001). Variation in δ15N and δ13C trophic fractionation: implications for aquatic food web studies. Limnology Oceanogr. 46, 2061–2066. doi: 10.4319/lo.2001.46.8.2061
Keywords: stable isotope analysis, trophic ecology, mesophotic coral ecosystems, coral physiology, autotrophy, heterotrophy, Leptoseris, Pachyseris
Citation: Carmignani A, Radice VZ, McMahon KM, Holman AI, Miller K, Grice K and Richards Z (2023) Levels of autotrophy and heterotrophy in mesophotic corals near the end photic zone. Front. Mar. Sci. 10:1089746. doi: 10.3389/fmars.2023.1089746
Received: 04 November 2022; Accepted: 17 April 2023;
Published: 10 May 2023.
Edited by:
Raúl A. González-Pech, The Pennsylvania State University (PSU), United StatesReviewed by:
Tomás López-Londoño, The Pennsylvania State University (PSU), United StatesCopyright © 2023 Carmignani, Radice, McMahon, Holman, Miller, Grice and Richards. This is an open-access article distributed under the terms of the Creative Commons Attribution License (CC BY). The use, distribution or reproduction in other forums is permitted, provided the original author(s) and the copyright owner(s) are credited and that the original publication in this journal is cited, in accordance with accepted academic practice. No use, distribution or reproduction is permitted which does not comply with these terms.
*Correspondence: Amy Carmignani, YW15LmNhcm1pZ25hbmlAcmVzZWFyY2gudXdhLmVkdS5hdQ==
†Present address: Amy Carmignani, The West Australian Biogeochemistry Centre, School of Biological Sciences, The University of Western Australia, Crawley, WA, Australia
Disclaimer: All claims expressed in this article are solely those of the authors and do not necessarily represent those of their affiliated organizations, or those of the publisher, the editors and the reviewers. Any product that may be evaluated in this article or claim that may be made by its manufacturer is not guaranteed or endorsed by the publisher.
Research integrity at Frontiers
Learn more about the work of our research integrity team to safeguard the quality of each article we publish.