- Institute of Marine Biotechnology and Bio-resource Utilization, College of Oceanography, Hohai University, Nanjing, China
Biomineralization is a universal phenomenon in the ocean that plays an important role in marine geochemical circulation. The genus Alteromonas is an indigenous taxon with a wide distribution and various ecological roles in the ocean, but biomineralization by this genus has not been reported. In this study, five Alteromonas spp. were found to induce mineral crystal formation of different shapes and sizes in agar media. Further studies on deep-sea strains A. alteriprofundi HHU 13199T and A. alterisediminis N102T showed that they could produce mineral crystals with similar morphology when grown in agar or broth media with different concentrations of sea salts (i.e., 2%, 4%, 6%, and 8%), and that their growth was dependent on Ca2+ and/or Mg2+ ion concentrations. Genomic analysis showed that the genus Alteromonas universally possessed the ammonification metabolism pathway and that, during the culture of these bacteria, the production of mineral crystals was accompanied by an increase in ammonia concentration and pH value and a decrease in nitrate nitrogen concentration. The addition of ammonia to broth media (≈ 572.7 mg/L) simulated the ammonia content in media on days 5 and 6 of bacterial growth and also induced mineral crystals to form. Through the analysis using scanning electron microscope–energy-dispersive spectrometry (SEM-EDS), X-ray diffraction (XRD), Fourier-transform infrared microscopy (FTIR), thermogravimetric (TG) analysis, and differential thermal gravity and differential scanning calorimetry (DTG–DSC), mineral crystals induced by bacterial strains and the non-strain (ammonia-added sample) were all identified as struvite mineral. In addition, the characteristics of the struvite mineral induced by bacterial strains were different from the characteristics of the struvite synthesized by non-strain and of a struvite mineral standard. Thus, this study deduces that Alteromonas spp. possess the ability to induce struvite formation. The mechanism mainly lies in the presence of an ammonification metabolism pathway to produce ammonia, which should be recognized as biologically induced mineralization (BIM). This study provides insight into a new ecological role of indigenous marine taxa of the genus Alteromonas.
1 Introduction
Oceans and seas are composed of 96.7% water and 3.3% dissolved salts; almost all elements in the periodic table can be found in seawater, although many occur at very low concentrations (Loganathan et al., 2017). Many ions, which make up salts, are essential micronutrients for microbial growth, and are able to participate in biological processes inside the cells, such as coenzyme reactions, redox reactions, and osmotic regulation (Nies, 1999; Bruins et al., 2000). From a different perspective, marine microorganisms possess unique properties owing to the need to adapt to disadvantageous environmental conditions caused by ions, such as alkaline and acidic water, and high osmotic stress. For example, microorganisms can change the valence, existence form, and solubility of the ion (Beam et al., 2018; Vigliaturo et al., 2020; Sanz-Saez et al., 2022; van de Velde et al., 2022).
Biomineralization is the biological process in which organisms deposit associated ions by raising the pH around cells to supersaturate mineral ion concentrations (Han et al., 2015; Falagán et al., 2017). The formation of minerals and rocks is also closely related to biomineralization; for example, some aerobic bacteria can induce the formation of dolomite, calcium magnesium carbonate, and high-magnesium calcite (Deng et al., 2010; Li et al., 2017). In addition, some microorganisms in hypersaline brackish lakes can induce the deposition of Ca2+ and Mg2+ ions to form modern carbonate stromatolites (Spadafora et al., 2010). Based on biological control, biomineralization is currently categorized into three distinct groups (Park and Faivre, 2022): (i) biologically influenced biomineralization (BFM), a passive mineralization process depending on negatively charged molecules on the cell surface, such as proteins, lipids, and glycoproteins on the bacterial cell wall and extracellular polymeric substances (EPS); (ii) biologically induced biomineralization (BIM) (Frankel and Bazylinski, 2003), which depends on inorganic materials in the environment driven by cellular activities that change the chemical composition of the cell’s interstitial space, resulting in a high-pH microenvironment, such as photosynthesis, urea hydrolysis, nitrate reduction, deamination, and sulfate reduction; and (iii) biologically controlled biomineralization (BCM), associated with an organic matrix that forms an isolated intracellular environment and refers to the precipitation of minerals by elaborate cellular control. Many microbial taxa have been reported to possess biomineralization capabilities, some of which have been used in wastewater treatment (Han et al., 2014; Ronholm et al., 2014; Han et al., 2015; Sun et al., 2020; Leng and Soares, 2021; Zhang et al., 2022). There is a large variety and wide distribution of bacteria in oceans and seas. Therefore, it is of great research significance to study the biomineralization by microorganisms in the ocean for the regulation of metal ions in seawater.
Alteromonas spp. are widespread, abundant, and phytoplankton-associated indigenous marine copiotrophs, and live in a variety of habitats, especially the seawaters from the tropics to the poles, and even hadal zones (Ivars-Martinez et al., 2008; Gonzaga et al., 2012; Gago et al., 2021). The Tara Oceans expedition found that Alteromonas had an occurrence rate reaching 80%, with consistently high relative abundances (Nayfach et al., 2016). In phytoplankton blooms of the western North Pacific Ocean, members of the genus Alteromonas contributed to the total bacterial biomass as much as the Cytophaga–Flavobacterium–Bacteroides (CFB) group and Roseobacter (Tada et al., 2011; Sarmento and Gasol, 2012). In the Challenger Deep of the Mariana Trench, Alteromonas was found to still be abundant, with a 16S rRNA gene proportion > 5% of the microbial community across the near-bottom water (> 10,000 m below the surface and ≈ 300–400 m from the seafloor) (Liu et al., 2019). Metatranscriptomic studies have shown Alteromonas to be a key member of global ocean carbon and nitrogen cycling, because of their strong capability to recycle dissolved organic carbon (McCarren et al., 2010; Pedler et al., 2014). In addition, Alteromonas strains have also been found to make significant contributions to marine iron cycling and transport, and many gene clusters have been identified to be involved with resistance to heavy metals (Debeljak et al., 2019; Manck et al., 2020; Cusick et al., 2021; Zhang et al., 2021). It has also been reported that Alteromonas spp. produce extracellular polysaccharides (EPSs), enzymes, and secondary metabolites that have been used for biotechnological purposes with different applications (Lelchat et al., 2015; Zykwinska et al., 2018; Lee and Jun, 2019). Overall, the genus Alteromonas possesses great potential in applications of the biotechnological industry and plays important roles in the geochemical cycling of oceans.
The genus Alteromonas currently includes 35 validly published species (https://lpsn.dsmz.de/genus/alteromonas) (Parte et al., 2020), including five transferred from the genus Salinimonas for the consistent monophyletic nature of members of the two genera (Gago et al., 2021). During the taxonomic identification of A. alteriprofundi (= S. profundi) (Zhang et al., 2021), we found that this strain and its four close relatives, A. alterisediminis (= S. sediminis), A. chungwhensis (= S. chungwhensis), A. iocasae (= S. iocasae), and A. lutimaris (= S. lutimaris), could consistently produce macroscopic crystals in agar media. Although Alteromonas spp. are versatile in marine environments and have applications in the biotechnology industry, as suggested above, their biomineralization function has not been reported. Here, we further characterize crystals induced by these five Alteromonas spp., and provide insight into the related mechanism. This study suggested the potential involvement of species of the genus Alteromonas in marine struvite deposition and sheds new light on their ecological roles in marine environments.
2 Materials and methods
2.1 Bacterial strains and culture conditions
The experiments were performed with five strains of the genus Alteromonas: A. alterisediminis N102T, isolated from deep-sea (4,700 m) sediment of the New Britain Trench (Cao et al., 2018); A. alteriprofundi HHU 13199T, isolated from a marine sediment sample from the South China Sea at a depth of 2,918 m (Zhang et al., 2021); A. chungwhensis KCTC 12239T, isolated from a solar saltern in South Korea (Jeon et al., 2005); A. iocasae MCCC 1K03884T, isolated from a polychaete tube in a hydrothermal field of the Okinawa Trough (Zhang et al., 2020); and A. lutimaris KCTC 23464T, isolated from a tidal flat on the southern coast of South Korea (Yoon et al., 2012). These strains were maintained on 2216E medium (Solarbio, Beijing, China) and stored in aqueous glycerol suspensions (20%, v/v; volume to volume) at –80°C.
2.2 Sea salt dependence during bacterial growth
As previously reported, A. alteriprofundi HHU 13199T could not be grown in tryptic soy agar (TSA, Bacto™) media supplemented with 2.5% NaCl (w/v), whereas the other four strains could (Jeon et al., 2005; Yoon et al., 2012; Cao et al., 2018; Zhang et al., 2020; Zhang et al., 2021). In this study, four different types of TSA media with Backhaus artificial seawater (ASW) of incomplete formula (I–IV, Table S1) were prepared to clarify which mineral salts were necessary and/or advantageous for bacteria growth. The TSA I–IV media contained (per liter): 10.0 g of tryptone, 5.0 g of soya peptone, 481 mM NaCl, and incomplete ASW I–IV. To clarify the optimal quantity, different concentrations (i.e., 0–1× of the original formula, at 0.1× intervals) of CaCl2 (0–1.379 g/L) and MgCl2·6H2O/MgSO4·7H2O (0–12.291 g/L), respectively, were supplemented with tryptic soy broth (TSB) media to determine the optimal additive amount. In addition, salinity differences of the original ASW formula for each treatment were eliminated by adding the corresponding weight of NaCl. A control group with no bacterial incubation was set up for each concentration. Bacterial growth was monitored by an automatic growth curve analyzer (Bioscreen C Pro; OY Growth Curves Ab Ltd, Helsinki, Finland), and measurements were taken every 2 hours.
2.3 Changes in pH, ammonia nitrogen and nitrate nitrogen during bacterial growth
The 2216E broth was used to monitor changes in pH, ammonia nitrogen (NH3-N), and nitrate nitrogen (NO3-N) during bacterial growth because it is oligotrophic and contains levels of nutrients much closer to the real marine environment than TSB. The initial pH of the 2216E broth was adjusted to 7.5 with 0.5 M NaOH and 0.5 M HCl solutions. The bacteria were inoculated into a volume of 500 mL of 2216E broth using a conical flask of 1,000 mL (2216E broth with no bacterial incubation was used as a control), cultured at 28°C for 120 hours with vibration (180 rpm), and sampled (30 mL) at 24-hour intervals. The samples were centrifuged at 6,000 × g for 10 minutes to obtain the supernatant. The pH value of the supernatant was measured using a water quality tester (PHSJ-4F; Oustor, Shanghai, China). NH3-N content was determined by Nessler’s reagent spectrophotometry (Gao et al., 2018). NO3-N content was determined by alkaline potassium persulfate digestion followed by UV spectrophotometry (Purcell and King, 1996).
2.4 Mineral crystallization and isolation
Mineral crystallization was investigated by incubating bacteria in TSA/TSB (Difco) supplemented with 3.0% (w/v) sea salt media and 2216E broth for 10 days, at 28°C, and with an initial pH of 7.5. To investigate the effect of salinity on crystallization, bacterial strains were inoculated in TSB media with 2.0%, 4.0%, 6.0%, and 8.0% sea salt. For structural and element content analysis, mineral crystals were isolated from TSA agar medium supplemented with 3.0% sea salt, because of a higher yield in such a condition. The mineral crystals were formed in the agar medium or on the surface. Potassium iodide (KI) was used as the chaotropic salt that conforms to the Hofmeister series (Saito, 1969; Zhang and Cremer, 2006). The TSA medium was cut into cubes and placed into 100 mL of pure water before KI was added. When heated at 70°C for half an hour, the mineral crystals were seen to precipitate from the agar. We also added 200 µL of ammonia solution of analytical grade (Jiuyi, Shanghai, China) to 39.8 mL of TSB medium supplemented with 3.0% sea salt, and no bacterial incubation to induce mineral crystal as a control, in which the final ammonia concentration measured was 572.7 mg/L. This concentration was of a similar level to that of the 2216E medium after 6 days’ incubation. A commercial struvite (NH4MgPO4·6H2O) (Macklin, Shanghai, China) was used as standard for the identification of mineral crystals induced by bacterial strains.
2.5 Mineral crystal characterization
Scanning electron microscopy–energy-dispersive spectrometry (SEM-EDS; FEI, USA) using double-beam scanning was used to detect the difference in elemental composition between mineral crystals induced by bacterial strains, mineral crystals produced by adding ammonia to TSB medium, and commercial struvite. Fourier-transform infrared microscopy (FTIR; IS50, Thermo Fisher Scientific Inc., USA) was performed in a scanning range of 400–4,000 cm–1, with the potassium bromide technique used to detect the surface functional groups of the mineral crystal. The scanning angle (2θ) of the X-ray diffractometer (XRD; D8 Advance, Brucker, Germany) ranged from 10° to 90°, with a step size of 0.02° and a count time of 8° min–1. Data from the XRD were analyzed by Jade (v6.5) software (Mechanical Dynamics, Inc., USA) to determine the composition of mineral crystal induced by bacterial strains. A comparison was made with the experimental diffraction data of struvite standard in the powder diffraction file (PDF) of the International Center for Diffraction Data (ICDD) to confirm whether or not the mineral crystal was struvite mineral. Thermogravimetric (TG) analysis, differential thermal gravity (DTG), and differential scanning calorimetry (DSC) analysis were conducted with a simultaneous thermal analyzer (STA 449F3, Netzsch, Germany) in a temperature range of 29–1,000°C at a heating rate of 10°C/min to assess the thermal stability and composition of the mineral crystal.
2.6 Genetic analysis of ammonia-producing metabolic pathways
To determine pathways involved in producing ammonia among the genus Alteromonas, gene families of ammonification, which includes glutamate dehydrogenation (gdh_K00260, gdh_K00261, gdh_K00262, and gdh_K15371), assimilatory nitrate reduction (nasA, nasB, nirA, NR, narB, and narC), and dissimilatory nitrate reduction (napA, NapA, napB, napC, narG, narH, narJ, narI, narZ, nary, narV, narW, nirB, nirD, nrfA, nrfB, nrfC, and nrfD), were extracted from the NCycDB database (Tu et al., 2019). Sequences of each gene family were sorted by length, the longest quarter and shortest quarter were removed, and the remaining half was used to build profile hidden Markov models (HMMs) using the program hmmbuild in the HMMER package, version 3.0 (http://hmmer.org). The program hmmsearch in HMMER was used for homolog identification of ammonification genes against genomes of the genus Alteromonas with an E-value cut-off point of 1e-10. All the candidate genes were further submitted to the online annotation tool BlastKOALA (https://www.kegg.jp/blastkoala/) (Kanehisa et al., 2016) to verify whether or not they were a member of related gene family. All the genomes of the genus Alteromonas available in the RefSeq database on NCBI (National Center for Biotechnology Information) were downloaded and subjected to ammonification gene analysis. The bac120 gene set was used to build a genome-based phylogenetic tree with EasyCGTree, version 4.0 (https://github.com/zdf1987/EasyCGTree4) (Xue et al., 2021).
3 Results
3.1 Alteromonas spp. can induce mineral crystals in either agar or broth media with different sea salt concentrations
After incubation for 7 days at 28°C on TSA plates, mineral crystals induced by the five Alteromonas strains were visible to the naked eye. The crystals had radial or acicular forms and were of different sizes (Figure 1). The crystals produced by A. alteriprofundi HHU 13199T and A. lutimaris KCTC 23464T had smaller sizes, of < 3 mm, whereas those of A. chungwhensis KCTC 12239T had larger sizes, of about 1 cm. Subsequently, A. alteriprofundi HHU 13199T and A. alterisediminis N102T were selected as representatives to analyze the effect of different sea salt concentrations on mineral crystallization for their apparent divergence among the five strains (Zhang et al., 2021). Mineral crystals were induced in the TSB medium with different sea salt concentrations (2%, 4%, 6%, and 8%) after about 10 days of incubation (Figure 2). The results of scanning electron microscopy (SEM) suggested that the size and morphology of the mineral crystal particles did not change significantly as the sea salt concentrations increased. The crystals were approximately 200–300 μm in size and relatively regular in shape, with several crystals coalescing at low concentrations (Figure 2). With higher sea salt concentrations, there was a greater tendency to form individual, elongated crystals in strips, and the surface of all crystals was relatively smooth. The crystals induced in the TSB media were found to be not as large in size as those observed in TSA media; the reason for this may be that the large crystals were broken down during culturing vibration and sample collection.
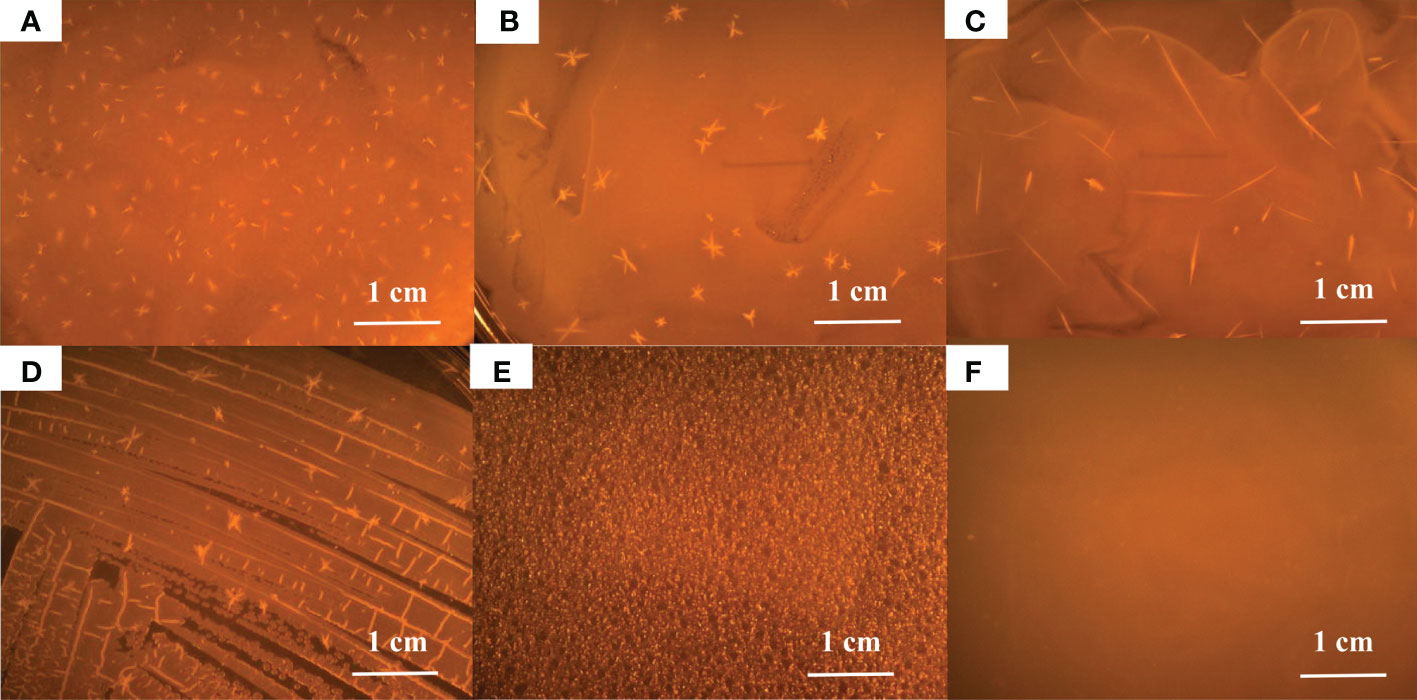
Figure 1 Mineral crystals induced by Alteromonas on TSA+2.5% sea salt. (A), A. alteriprofundi HHU 13199T; (B), A. alterisediminis N102T; (C), A. chungwhensis KCTC 12239T; (D), A. iocasae MCCC 1K03884T; (E), A. lutimaris KCTC 23464T; (F), control.
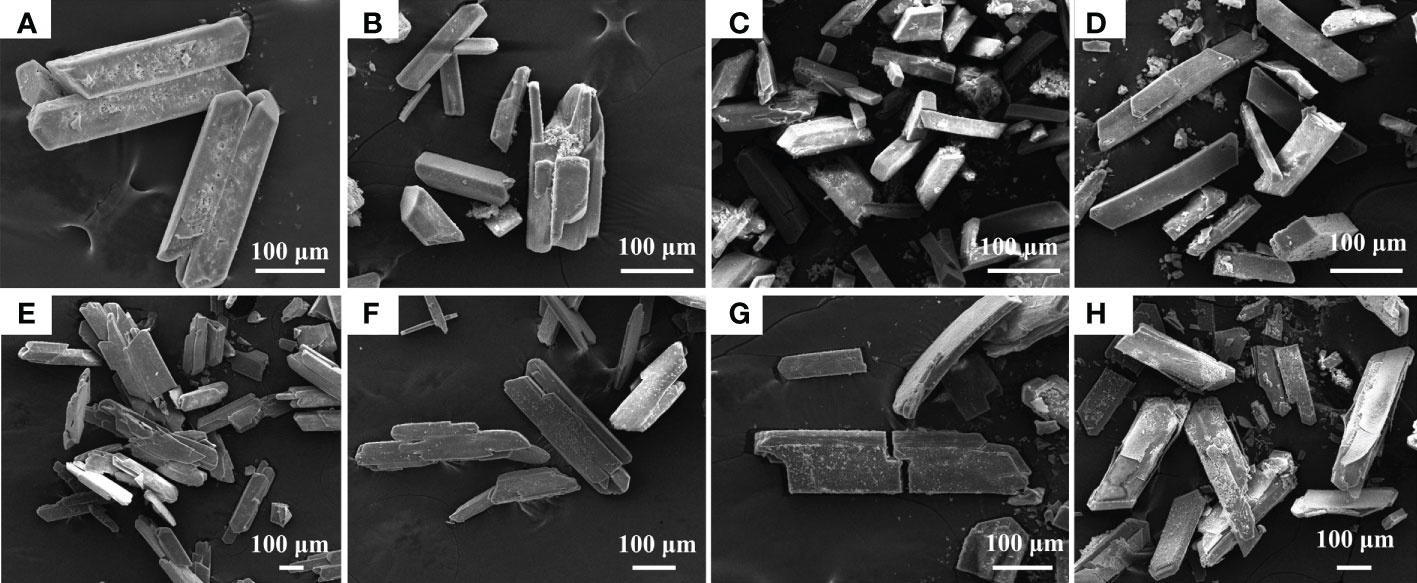
Figure 2 Scanning electron microscope (SEM) analysis of mineral crystals induced by Alteromonas at different concentrations of sea salt. Alteromonas spp. were cultured in TSB supplemented with different sea salt concentrations (2%, 4%, 6%, 8%, from left to right) for 5 days. (A–D), A. alteriprofundi HHU 13199T; (E–H), A. alterisediminis N102T.
3.2 Alteromonas spp. induce mineral crystals by ammonification
During induction experiments of mineral crystal formation in 2216E medium, we noticed that the pH increased to > 8.0 after incubation. Therefore, it is hypothesized that the production of ammonia contributes to an increase in pH. Strains A. alteriprofundi HHU 13199T and A. alterisediminis N102T were incubated in 2216E broth to monitor ammonia content during culture. The results (Figure 3A) show that the pH of the 2216E medium increased from 7.5 to 8.6 after 5 days of incubation for both strains, and that the increase in pH may have provided an alkaline environment that favored the formation of struvite. The initial NH3-N concentration was approximately 20.3 mg/L, increasing to approximately 606.7 mg/L after 5 days (Figure 3B). The increased NH3-N content in the medium also contributed toward the alkaline environment. The NO3-N content decreased from an initial level of approximately 130–150 mg/L to approximately 10–30 mg/L (Figure 3C). This indicates that A. alteriprofundi HHU 13199T and A. alterisediminis N102T both have the ability to produce ammonia by nitrate reduction and to cause a pH increase in the environment.
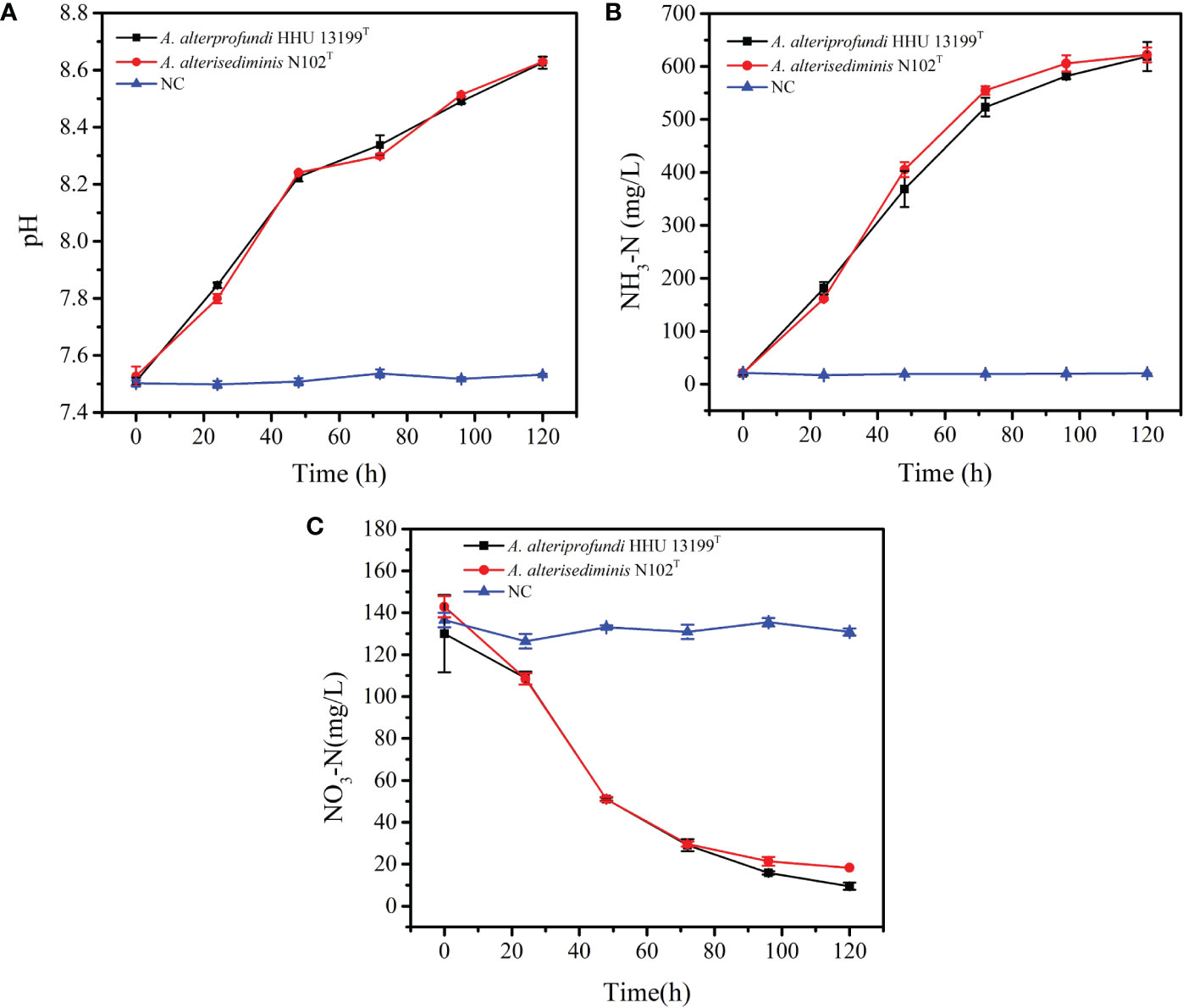
Figure 3 Changes in pH value, NH3-N, and NO3-N concentration in 2216E medium during the incubation of strains A. alteriprofundi HHU 13199T and A. alterisediminis N102T. (A), pH value; (B), NH3-N concentration; (C), NO3-N concentration.
3.3 Characterization of mineral crystals
Strains A. alteriprofundi HHU 13199T and A. alterisediminis N102T were grown on TSA medium to collect mineral crystals for further characterization because of the higher yields of crystals obtained when the strains were grown in this medium. SEM-EDS was used to determine the morphology and elemental composition of the mineral crystals. SEM results showed that mineral crystals induced by A. alteriprofundi HHU 13199T (Figures 4A, B) mainly had a thin columnar form. Mineral crystals induced by A. alterisediminis N102T were also mainly of thin columnar form, with some irregular forms (Figures 4D, E). Mineral crystals produced by adding ammonia to TSB medium were regular and blocky in shape (Figures 4G, H). Commercial struvite crystals were blocky and had a thin columnar form (Figures 4J, K). The mineral particles mentioned above varied in size on the micro-scale. EDS results showed that the elemental composition of mineral crystals induced by A. alteriprofundi HHU 13199T and A. alterisediminis N102T was C, O, Na, Mg, P, K, and Ca (Figures 4C, F). The mineral crystals produced by adding ammonia to the TSB medium had an elemental composition of O, Na, Mg, P, K, and Ca (Figure 4I).
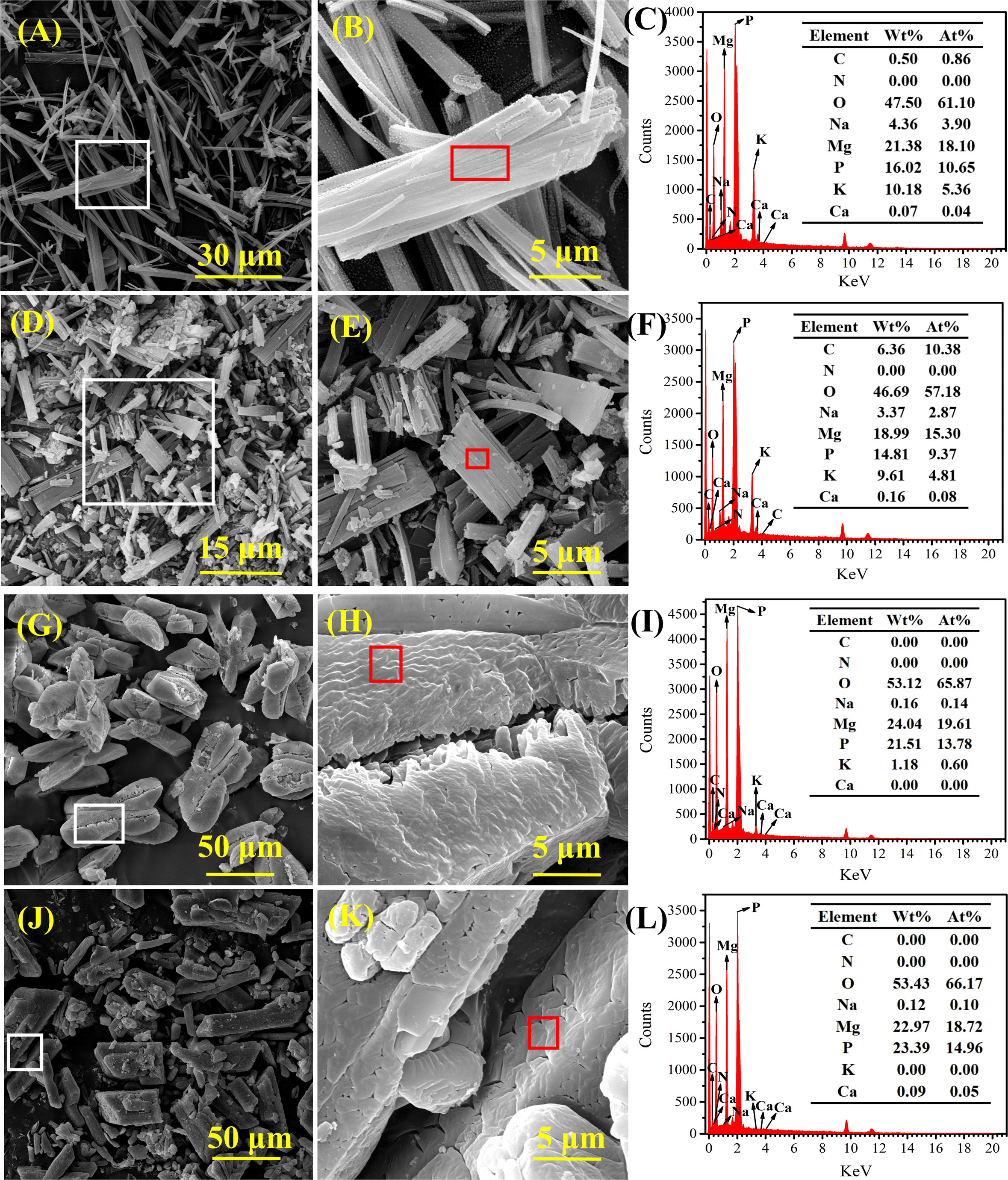
Figure 4 Scanning electron microscope (SEM) and energy dispersive spectrum (EDS) images of mineral crystals induced by strains A. alteriprofundi HHU 13199T (A–C), A. alterisediminis N102T (D–F), ammonia solution (G–I), and commercial struvite standard (NH4MgPO4·6H2O) (J–L), respectively.
XRD was used to confirm the crystal phase and the structure of the mineral crystals induced by A. alteriprofundi HHU 13199T and A. alterisediminis N102T. The results (Figure 5Aa) implied that the diffraction peaks (2θ) of mineral crystals induced by A. alteriprofundi HHU 13199T at 15.226, 15.960, 16.695, 21.063, 21.676, 25.207, 27.289, 30.270, 30.759, and 33.413 were relevant to the Miller (hkl) indices (110), (020), (011), (111), (021), (121), (130), (012), (211), and (022), respectively. The interplanar crystal spacing values (d) corresponding to the diffraction peaks (2θ) were 5.8141, 5.5484, 5.3058, 4.2144, 4.0967, 3.5301, 3.2653, 2.9502, 2.9044, and 2.6795, respectively. The diffraction peaks (2θ) of mineral crystals induced by A. alterisediminis N102T (Figure 5Ab) at 15.266, 16.062, 16.756, 21.104, 21.716, 30.453, 30.820, 33.943, 38.577, and 46.599 were relevant to the (hkl) indices (110), (020), (011), (111), (021), (012), (211), (221), (231), and (103), respectively. The interplanar crystal spacing values (d) corresponding to the diffraction peaks (2θ) were 5.7991, 5.5133, 5.2866, 4.2062, 4.0890, 2.9329, 2.8988, 2.6389, 2.3319, and 1.9474, respectively. The diffraction peaks (2θ) of mineral crystals produced by adding ammonia to the TSB medium (Figure 5Ac) at 15.205, 16.000, 16.613, 21.043, 21.655, 25.840, 27.248, 29.718, 30.292, 30.799, 32.107, 33.331, 33.862, 38.455, 45.130, and 46.518 were relevant to the (hkl) indices (110), (020), (011), (111), (021), (200), (130), (201), (012), (211), (040), (022), (221), (231), (151), and (103), respectively. The interplanar crystal spacing values (d) corresponding to the diffraction peaks (2θ) were 5.8221, 5.5348, 5.3317, 4.2183, 4.1005, 3.4450, 3.2701, 3.0037, 2.9386, 2.9026, 2.7856, 2.6858, 2.6450, 2.3390, 2.0074, and 1.9507, respectively. The diffraction peaks (2θ) of the commercial struvite (NH4MgPO4·6H2O) (Figure 5Ad) at 15.144, 15.960, 16.614, 21.002, 21.614, 25.779, 27.228, 29.678, 30.351, 30.759, 32.066, 33.413, 33.801, 38.414, 45.068, and 46.457 were relevant to the (hkl) indices (110), (020), (011), (111), (021), (200), (130), (201), (012), (211), (040), (022), (221), (231), (151), and (103), respectively. The interplanar crystal spacing values (d) corresponding to the diffraction peaks (2θ) were 5.8462, 5.5491, 5.3316, 4.2267, 4.1081, 3.4532, 3.0079, 2.9423, 2.9044, 2.7890, 2.6795, 2.6496, 2.3414, 2.0099, and 1.9530, respectively. The mineral crystals induced by the bacterial strains and the ammonia-added sample were compared with the PDF of the struvite mineral (no. 15-0762).
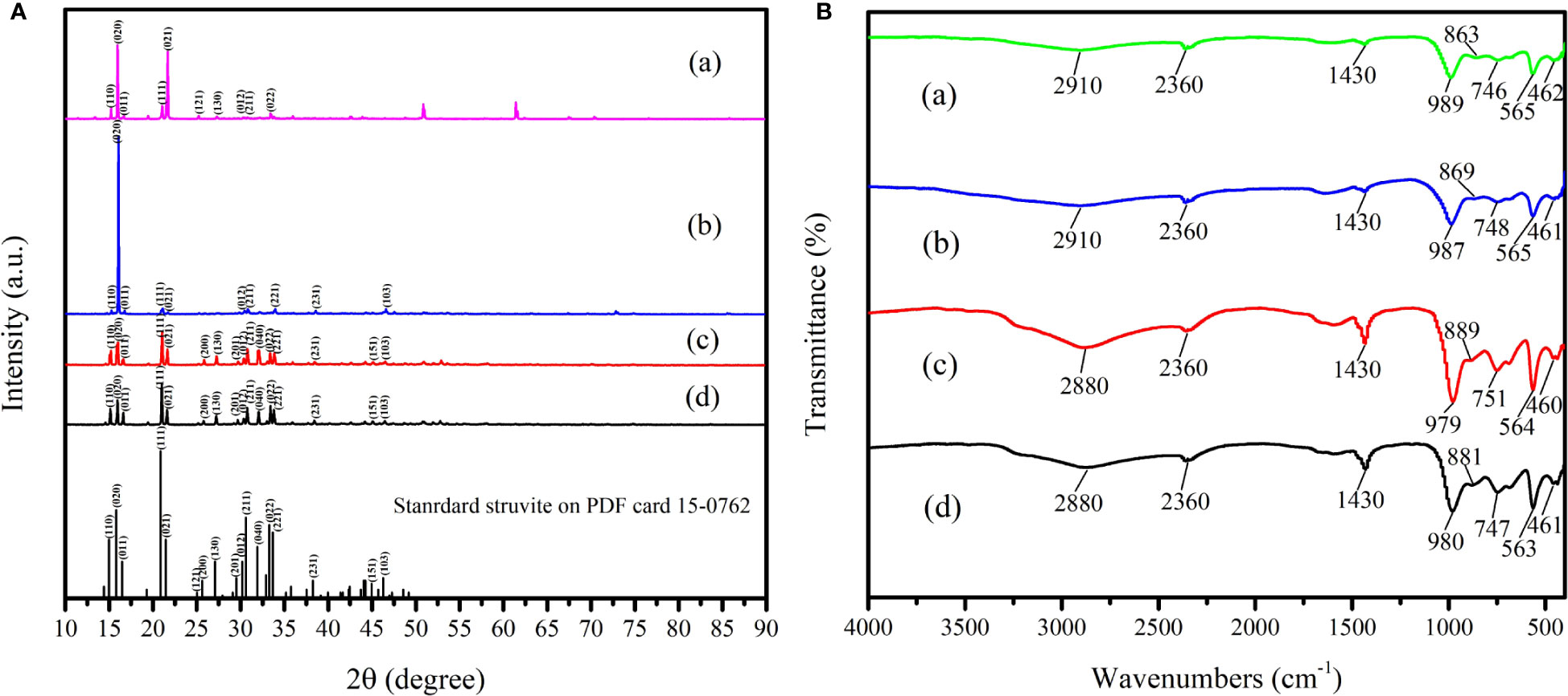
Figure 5 X-ray diffraction (XRD, A) and Fourier-transform infrared microscopy (FTIR, B) analysis of mineral crystals induced by bacterial strains in TSA medium, and ammonia solution in TSB medium. (a), the mineral crystals obtained in TSA medium with A. alteriprofundi HHU 13199T; (b), the mineral crystals obtained in TSA medium with A. alterisediminis N102T; (c), the mineral crystals obtained in TSB medium with ammonia; (d), the commercial struvite standard. Black bars show the struvite standard in PDF no. 15-0762.
To determine the group composition of mineral crystals induced by bacterial strains and in the ammonia-added sample, FTIR analysis was carried out. The results (Figure 5B) indicate that absorption peaks at 2,910, 2,880, and 2,360 cm–1 are in the vibration peaks of the symmetric stretching vibration and unsymmetrical stretching vibration of the hydrogen bond (O-H) of water (Sinha et al., 2014) and the N-H in the group. The peaks at 1,430 cm–1 arise from the unsymmetrical bending vibration of the N-H () (Korchef et al., 2011; Moulessehoul et al., 2017; Manzoor et al., 2019; Guan et al., 2021). The peaks at 979, 980, 987, 989, 563, 564, 565, 460, 461, and 462 cm–1 are the vibration peaks of the phosphoric acid group. The peaks at 746, 747, 748, and 751 cm–1 are assigned to the vibration of the hydrogen bonds among the water molecules.
The TG–DTG–DSC results (Figure 6A) imply that thermal weight loss of mineral crystal induced by A. alteriprofundi HHU 13199T was mainly divided into two stages: the first stage was from 29°C to 146.93°C, accounting for 33.83% of the total weight; and the second stage was from 146.93°C to 1,000°C, accounting for 17.97% of the total weight. The results (Figure 6B) show that the thermal weight loss of the mineral crystal induced by A. alterisediminis N102T was also divided into two stages: the first stage was from 29°C to 148.98°C, accounting for 36.03% of the total weight; and the second stage was from 148.98°C to 1,000°C, accounting for 16.39% of the total weight. The first stages of struvite formation induced by strains were mainly caused by the loss of crystalline water, and the second stages were attributed to the instability of ammonium and decomposition of ammonium to ammonia. Commercial struvite mineral (NH4MgPO4·6H2O) (Figure 6D), struvite mineral obtained in TSB medium with ammonia (Figure 6C), and struvite mineral induced by bacterial strains in the TSA medium (Figures 6A, B) all had two weight loss stages, which were caused by the loss of crystal water and the instability of ammonium and decomposition of ammonium to ammonia, respectively. The thermal stability was similar between mineral crystals induced by the bacterial strains, mineral crystals produced by the ammonia-added sample and commercial struvite mineral.
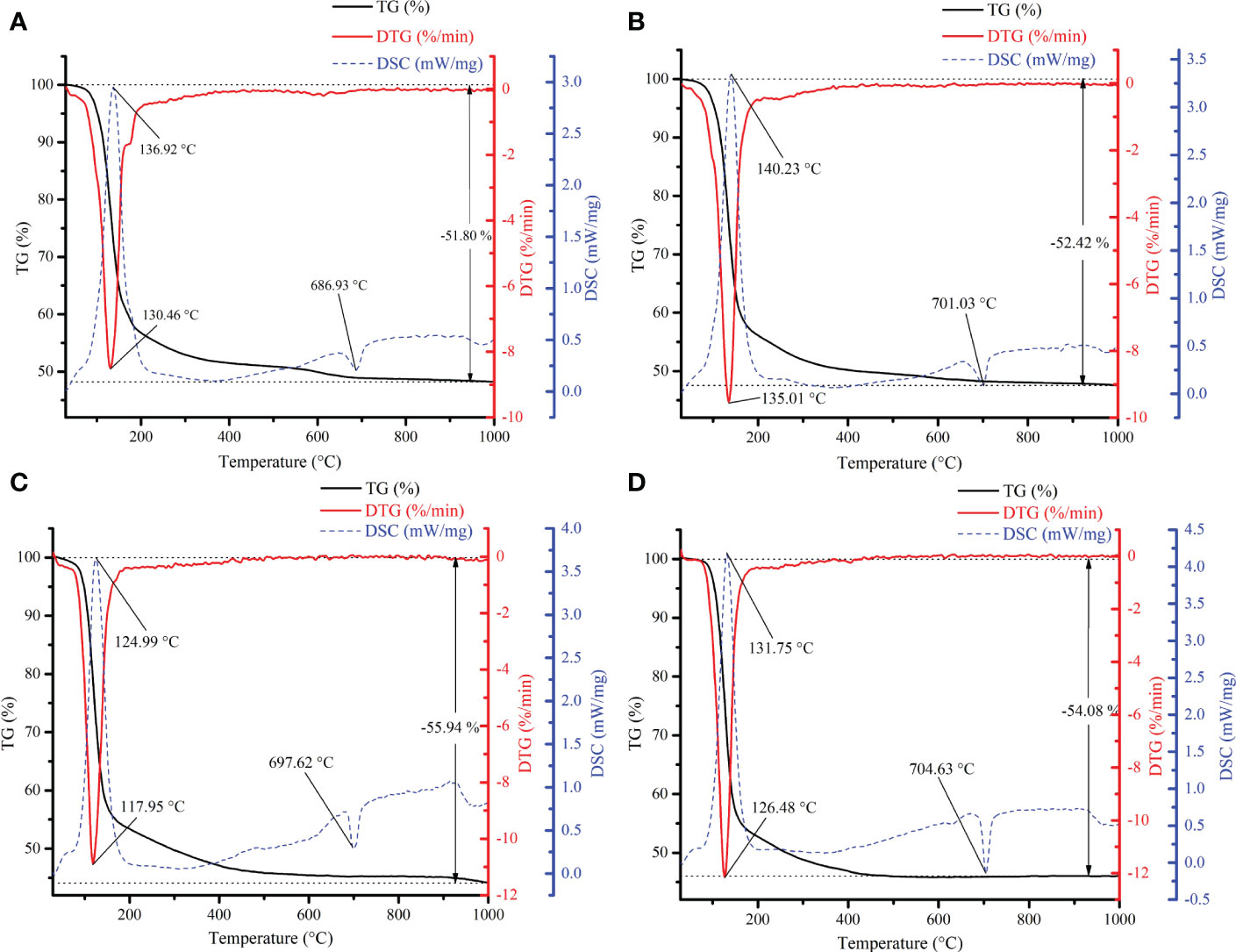
Figure 6 Thermogravimetric, differential thermal gravity and differential scanning calorimetry (TG–DTG) analysis of mineral crystals. (A), the mineral crystals induced by A. alteriprofundi HHU 13199T in TSA medium; (B), the mineral crystals induced by A. alterisediminis N102T in TSA medium; (C), the struvite obtained in TSB medium with ammonia; (D), the commercial struvite standard.
3.4 Ca2+ and/or Mg2+ can promote growth of Alteromonas spp.
By gradually reducing the components in seawater (Table S2), it was found that A. alteriprofundi HHU 13199T could grow on media TSA I and TSA II, which contained Mg2+ and Ca2+, respectively, but no growth occurred on media TSA III and TSA IV. Subsequently, A. alteriprofundi HHU 13199T and A. alterisediminis N102T were cultured in TSB media supplemented with NaCl and different concentrations of eight Mg2+ or Ca2+, respectively.
Both Ca2+ and Mg2+ had a growth-stimulating effect on both strains of the genus Alteromonas (Figure 7, Table S3). However, different concentrations of Ca2+ and Mg2+ had different stimulating effects on the growth of the strains. When CaCl2 was added at a concentration less than 0.414 g/L (0.3×, ≈ 3.6 mM Ca2+), growth of A. alteriprofundi HHU 13199T (Figure 7A) and A. alterisediminis N102T (Figure 7B) was suppressed (generation time > 50 h; Table S3). When MgCl2·6H2O/MgSO4·7H2O was added at a concentration less than 0.2× (9.50 mM Mg2+) and 0.1× (4.75 mM Mg2+), growth of A. alteriprofundi HHU 13199T (Figure 7D) and A. alterisediminis N102T (Figure 7D) was suppressed, respectively (generation time > 50 h; Table S3). When higher concentrations (especially >0.7×) of Ca2+ or Mg2+ were added, the growth rate and the final biomass tended to reach a steady state. These results suggest that the Ca2+ and Mg2+ contents in normal Backhaus ASW were very close to the optimal concentration required for the growth of both strains. In addition, A. alteriprofundi HHU 13199T needed higher Ca2+ and/or Mg2+ content to start reproducing, which implied a dependence on Ca2+ and/or Mg2+ for its growth.
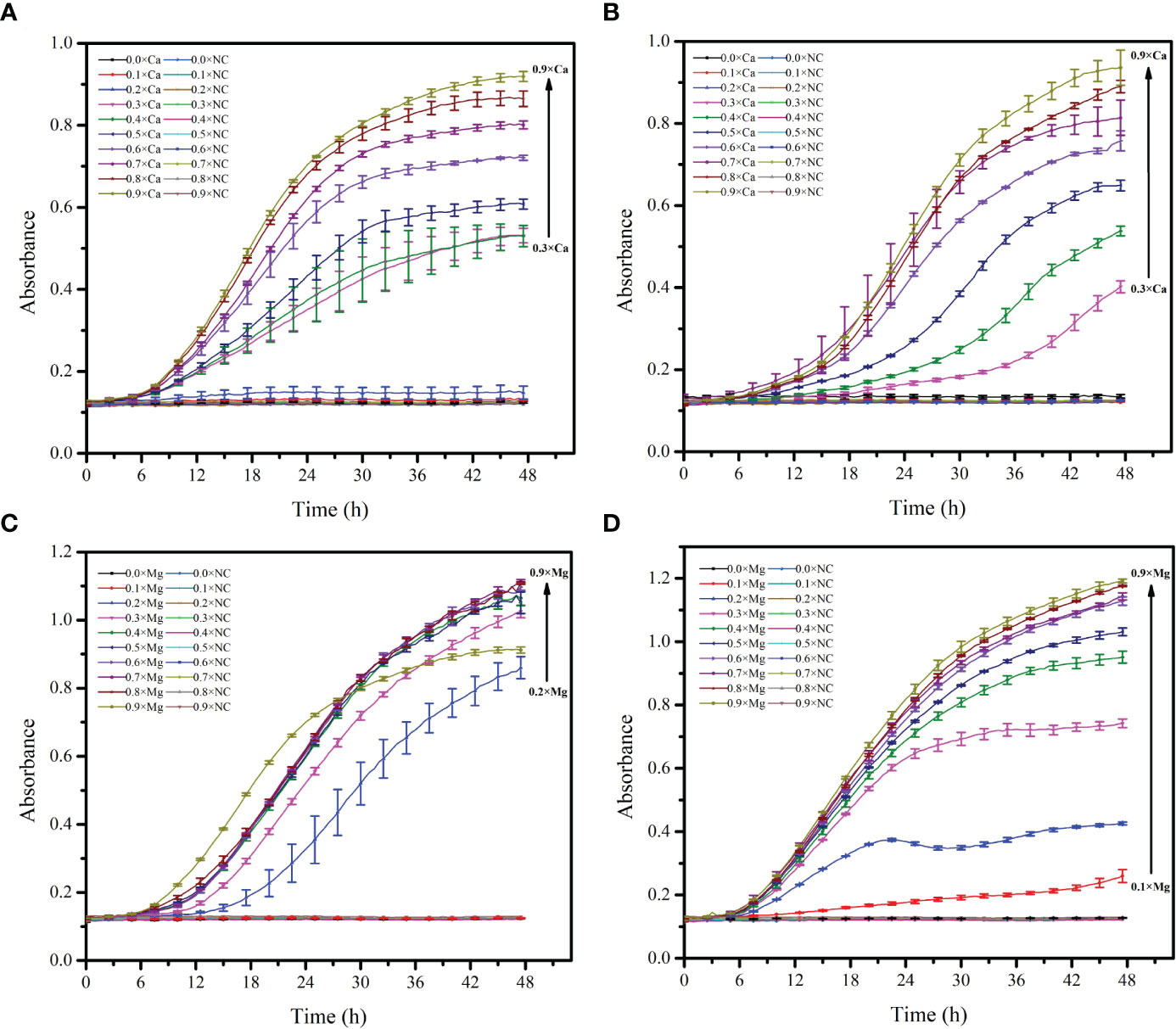
Figure 7 Growth curve of Alteromonas with different concentrations of Ca2+ and Mg2+. (A, C), A. alteriprofundi HHU 13199T; (B, D), A. alterisediminis N102T.
3.5 Pathways of ammonification metabolism are commonly present in the genus Alteromonas
In total, 126 genomes of the genus Alteromonas were retrieved from the RefSeq database, comprising 82 strains from 32 known species and 44 unclassified isolates (Figure 8). All the strains were found to be harboring at least three genes related to ammonification metabolism, and 10 genes were detected in the genus Alteromonas. For gene-encoding glutamate dehydrogenase, homologs of gdh_K00262 and gdh_K15371 were found in 99 (78.6%) and 125 (99.2%) genomes, respectively. For genes conferring dissimilatory nitrate reduction, narG, narH, and narI were detected in five (4.0%), four (3.2%), and five (4.0%) genomes, respectively. For genes conferring dissimilatory nitrite reduction, nirB and nirD were detected in 113 (89.7%) and 114 (90.5%) genomes, respectively. For genes conferring assimilatory nitrate reduction, nasA and narW/narJ were detected in 111 (88.1%) and five (4.0%) genomes, respectively. For genes conferring assimilatory nitrite reduction, nirA was detected in 124 (98.4%) genomes. A. chungwhensis KCTC 12239T, A. iocasae MCCC 1K03884T, A. lutimaris KCTC 23464T, and A. alteriprofundi HHU 13199T harbored gdh_K00262 and gdh_K15371, and nirA, whereas A. alterisediminis N102T harbored nirB and nirD. These five strains had fewer genes detected to be associated with ammonification metabolism among the genus Alteromonas. In contrast, three species, A. alba (GCF 002993365.1), A. mediterranea (GCF 927797765.1), and unclassified Alteromonas sp. (GCF 020515205.1), were found to be harboring 10 genes related to ammonification metabolism. Gene content identical to A. alterisediminis N102T was also identified in A. macleodii (GCF 000299995.1), whereas gene content identical to the other four strains was also found in A. sediminis (GCF 003820355.1), A. ponticola (GCF 012911815.1), A. halophila (GCF 014651815.1), Salinimonas marina (GCF 015644725.1), A. hispanica (GCF 010500915.1), and Alteromonas sp. (GCF 000753915.1 and GCF 000753865.1).
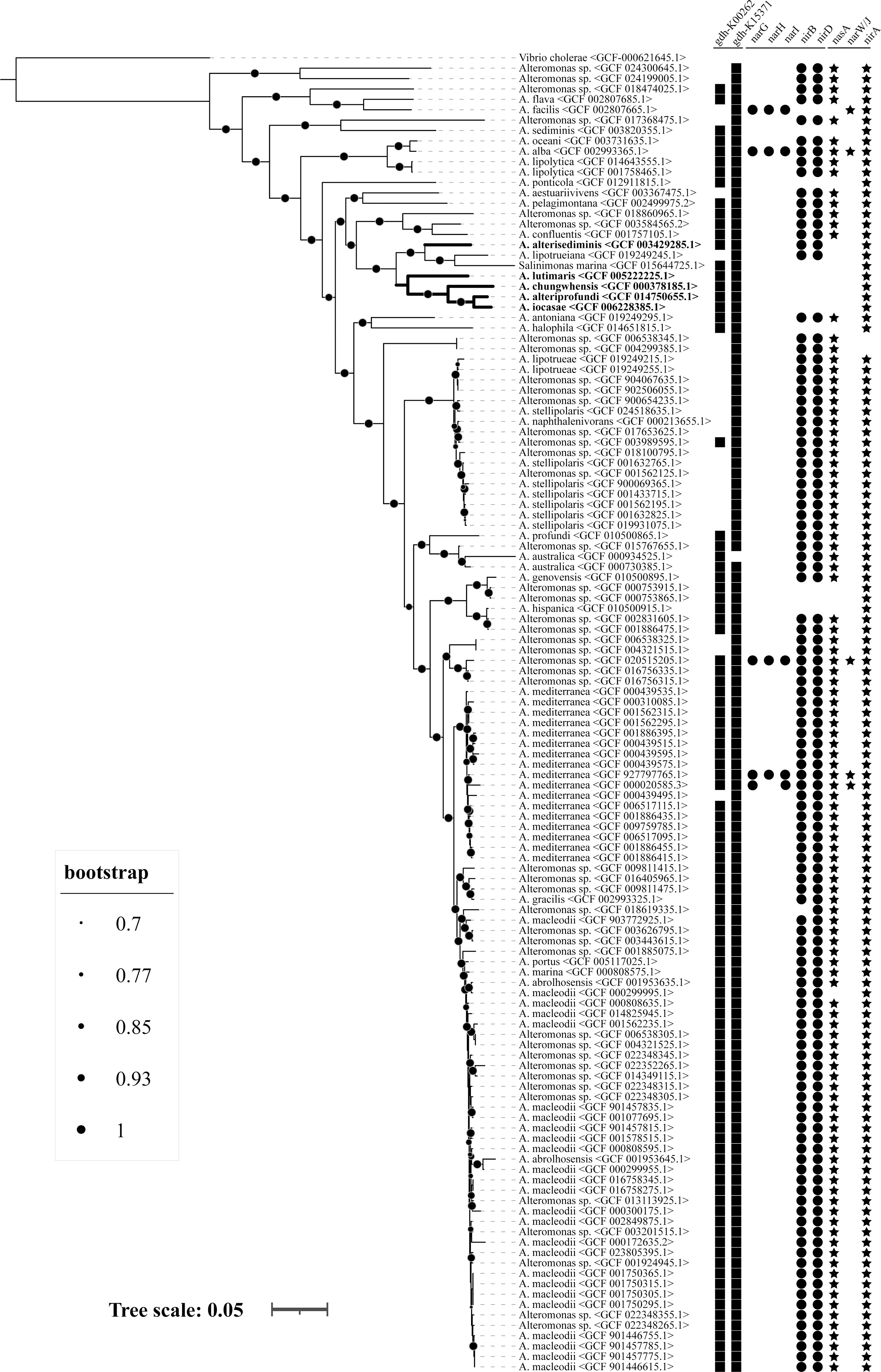
Figure 8 Genome-based phylogenetic tree and prevalence of ammonia-producing metabolic pathway in the genus Alteromonas. A total of 126 genomes, comprising 82 strains from 32 known species and 44 unclassified isolates, were used for analysis. Different sizes of dots on the branch represent different bootstrap values. Squares represent genes gdh_K00262 and gdh_K15371; dots represent genes narD, narH, narI, nirB, and nirD; stars represent genes nasA, narW/J, and nirA, respectively.
4 Discussion
The phenomenon of bacterial biomineralization has long been recognized, and a variety of minerals have been reported to be induced by species of bacteria (Beveridge, 1989; Gonzalez-Munoz et al., 2012; Diaz et al., 2017; Vigliaturo et al., 2020). For example, Bacillus licheniformis can induce calcite, vaterite, monohydrocalcite, and nesquehonite to remove calcium and magnesium from the environment by desalination (Zhao et al., 2019). Leclercia adecarboxylata JLS1 and Enterobacter sp. EMB19 can enrich phosphorus in the environment and mineralize to form struvite (Sinha et al., 2014; Han et al., 2017). Citrobacter freundii isolated from marine sediments can be used to induce the precipitation of carbonate and phosphate minerals under anaerobic conditions (Sun et al., 2020). However, reports focusing on biomineralization of marine bacteria are rare, in spite of the fact that precipitation of barite and calcite by marine bacteria have been documented (Gonzalez-Munoz et al., 2012; Sullivan and Murdaugh, 2014; Torres-Crespo et al., 2015; Wei et al., 2015; Sarayu et al., 2016; Yasumoto-Hirose et al., 2022). In this study, we found that five Alteromonas spp. from marine environments possess the ability to induce mineral crystals with special morphology in both agar and broth media. These results broadened the range of bacterial taxa that are responsible for mineral production.
In this study, it was observed that bacterial strains induced more struvite crystals in TSA medium than in 2216E medium, but the exact number and size differences were not recorded. It is thought that one of the reasons for this is that TSA medium (Bacto™) is eutrophic, with more nutrients, such as peptide/protein and phosphate, than 2216E medium, which facilitates bacterial induction of struvite crystal production. All five Alteromonas spp. induced mineral crystal formation in TSA agar medium that was supplemented with 2.5% sea salt. The strain A. lutimaris KCTC 23464T formed hard biofilms on the medium surface, and mineral crystals were formed in both the biofilm and the medium (Figure 1E). This is different from the other four strains. The mineral crystals formed by the different Alteromonas spp. showed differences in shape, and it can be deduced that the morphology of the mineral crystals is influenced by the strains themselves. Because strains A. alteriprofundi HHU 13199T and A. alterisediminis N102T were the furthest apart in the evolutionary tree, with the other three strains more closely related (Figure 8), the results from these two strains were taken to be representative of the mineral crystals induced by Alteromonas spp. in this study. SEM results showed that there was no significant change in the size and morphology of the mineral crystals induced by either strain when increasing the sea salt concentrations, indicating that the sea salt concentration had limited influence on the morphology of the mineral crystals. FTIR results showed that mineral crystals induced by bacterial strains and the ammonia-added sample all included the characteristic absorption bands of crystalline water, , and , may be struvite mineral (Han et al., 2015). SEM–EDS results indicated that mineral crystals induced by bacterial strains and the ammonia-added sample both had the elements O, Mg, P, K, Ca, and Na. Among them, O, Mg, and P are the base components of struvite mineral (Figure 4), and C, Na, K, and Ca may come from organic matter metabolized by strains (Wang et al., 2022). The XRD results showed that mineral crystals induced by bacterial strains had the strongest diffraction peak intensity in the crystal plane (020), indicating that mineral crystals mainly grow along the crystal plane (020), whereas mineral crystals of the ammonia-added sample and the standard struvite mineral of the PDF (no. 15-0762) mainly grow along the crystal plane (011). According to the results (Table 1) of the cell parameters analyzed with Jade (v6.5), both mineral crystals induced by bacterial strains and the ammonia-added sample were more compact than the commercial struvite, but mineral crystals induced by bacterial strains were more compact than those induced by the ammonia-added sample. All mineral crystal samples had similar interplanar spacing. This shows that the growth direction of mineral crystals produced by A. alteriprofundi HHU 13199T and A. alterisediminis N102T was the same, but different from that of the ammonia-added sample and the standard struvite crystals. This can be used to support the hypothesis that bacteria and their metabolites can influence nucleation and crystal growth (Han et al., 2017). The results of SEM–EDS and XRD proved that mineral crystals induced by the bacterial strains and the ammonia-added sample were struvite mineral when compared with the standard struvite mineral in the PDF (no. 15-0762).
The formation of struvite induced by bacteria was first reported in 1889 (Han et al., 2015), and, to date, many microorganisms have been reported to possess the ability to induce the formation of struvite, including Acinetobacter calcoaceticus, Azotobacter, Bacillus pumilus, Brevibacterium antiquum, Brucella, Corynebacterium, Enterobacter, Flavobacterium, Halobacterium salinarumand, Idiomarina loihiensis, Myxococcus xanthus, Proteus mirabilis, Pseudomonas, Shewanella oneidensis, and Ureaplasma urealyticum (Da Silva et al., 2000; Sun et al., 2012; Sinha et al., 2014; Han et al., 2015; Han et al., 2017; Manzoor et al., 2018; Leng and Soares, 2021; Zhang et al., 2022). However, to our knowledge, there are few marine bacteria that have been reported to be responsible for the formation of struvite. As mentioned above, the genus Alteromonas comprises versatile microorganisms playing essential roles in marine biogeochemical cycles (Lelchat et al., 2015; Chen et al., 2020; Cusick et al., 2021; Gago et al., 2021). In this study, we suggest that Alteromonas spp. can induce struvite formation under various laboratory conditions (Figure 1), which sheds new light on the ecological roles of these bacteria in marine environments. Furthermore, A. alteriprofundi HHU 13199T and A. alterisediminis N102T were taken as representative and their growth was determined with the dependence on Ca2+ and/or Mg2+ content. Surprisingly, the optimal concentration of Ca2+ and Mg2+ was very close to that in normal sea water (Figure 7), which further proved that Alteromonas spp. are indigenous to marine environments.
The formation of minerals in environments is very closely associated with the physiological and biochemical activities of microorganisms, and it was believed that struvite could be induced by microbes when the correct quantities of magnesium, ammonium, and phosphate were present (Rivadeneiea et al., 1985; Ronholm et al., 2014; Han et al., 2015; Han et al., 2017). In this study, struvite induced by Alteromonas spp. and ammonium solution were determined to have similar traits (Figure 4), which implied that Alteromonas spp. induced struvite by releasing ammonium to raise the environmental pH and provided for the formation of struvite. Together with the observation that the struvite was mainly formed in the agar media and rarely linked to bacterial mass, the related mechanism of Alteromonas spp. could be categorized as BIM, according to previous descriptions (Frankel and Bazylinski, 2003; Park and Faivre, 2022). The monitoring of NH-N and NO3-N content changes in the liquid culture of Alteromonas spp. also provided evidence for this conjecture (Figure 3). Further genetic analysis showed that pathways of ammonification metabolism were universally present in the genus Alteromonas (Figure 8). The five Alteromonas spp. that could produce ammonium and induce struvite possessed fewer genes involved in ammonification metabolism. In particular, genes conferring assimilatory or dissimilatory nitrate reduction were not detected except in A. alterisediminis. These results suggest that these bacteria probably harbor novel gene(s) to accomplish the function of nitrate reduction. In addition, these results imply that other bacteria of the genus Alteromonas with gene content identical to A. alterisediminis N102T or the other four strains used in this study could perform a biomineralization function.
5 Conclusion
In summary, we suggested that five Alteromonas spp. can induce mineral crystallization in laboratory conditions, and further characterized the mineral crystals induced by A. alteriprofundi HHU 13199T and A. alterisediminis N102T as struvite. The related mechanism was categorized as BIM, with ammonium release causing pH increase by the strains during growth through nitrate reduction. Nevertheless, some genes essential to conferring the function of nitrate reduction to ammonium were identified to be absent in four of the five strains used to induce mineral crystals. These results implied that there may be a novel gene family present in these strains, which should be clarified in the future. Prevalence analysis of ammonification metabolism pathways suggested that ammonium production was ubiquitous among the genus Alteromonas. Considering Alteromonas is indigenous in marine environments and has adapted to high salinity (Ivars-Martinez et al., 2008; Gonzaga et al., 2012; Chen et al., 2020; Gago et al., 2021), it is reasonable to question the role of Alteromonas spp. in struvite deposition in the ocean and sea. This will lead to a clearer picture of the role that the genus Alteromonas plays in marine geochemical circulation.
Data availability statement
The datasets presented in this study can be found in online repositories. The names of the repository/repositories and accession number(s) can be found in the article/Supplementary Material.
Author contributions
WH: data curation, formal analysis, investigation, visualization, and writing—original draft. H-PX: methodology, data curation, formal analysis, and investigation. CL: methodology, data curation, formal analysis, writing—review, and editing. AZ: methodology, resources, and formal analysis. J-KH: writing—review and editing. D-FZ: conceptualization, methodology, project administration, writing—review and editing, and funding acquisition. All authors contributed to the article and approved the submitted version.
Funding
The study was supported by the National Natural Science Foundation of China (No. 31900001), and the Fundamental Research Funds for the Central Universities (B210202140).
Conflict of interest
The authors declare that the research was conducted in the absence of any commercial or financial relationships that could be construed as a potential conflict of interest.
Publisher’s note
All claims expressed in this article are solely those of the authors and do not necessarily represent those of their affiliated organizations, or those of the publisher, the editors and the reviewers. Any product that may be evaluated in this article, or claim that may be made by its manufacturer, is not guaranteed or endorsed by the publisher.
Supplementary material
The Supplementary Material for this article can be found online at: https://www.frontiersin.org/articles/10.3389/fmars.2023.1085345/full#supplementary-material
References
Beam J. P., Scott J. J., McAllister S. M., Chan C. S., McManus J., Meysman F. J. R., et al. (2018). Biological rejuvenation of iron oxides in bioturbated marine sediments. ISME J. 12 (5), 1389–1394. doi: 10.1038/s41396-017-0032-6
Beveridge T. J. (1989). Role of cellular design in bacterial metal accumulation and mineralization. Annu. Rev. Microbiol. 43, 147–171. doi: 10.1146/annurev.mi.43.100189.001051
Bruins M. R., Kapil S., Oehme F. W. (2000). Microbial resistance to metals in the environment. Ecotox. Environ. Safe. 45 (3), 198–207. doi: 10.1006/eesa.1999.1860
Cao J., Lai Q., Liu P., Wei Y., Wang L., Liu R., et al. (2018). Salinimonas sediminis sp. nov., a piezophilic bacterium isolated from a deep-sea sediment sample from the new Britain trench. Int. J. Syst. Evol. Microbiol. 68 (12), 3766–3771. doi: 10.1099/ijsem.0.003055
Chen M., Song Y., Feng X., Tang K., Jiao N., Tian J., et al. (2020). Genomic characteristics and potential metabolic adaptations of hadal trench Roseobacter and Alteromonas bacteria based on single-cell genomics analyses. Front. Microbiol. 11. doi: 10.3389/fmicb.2020.01739
Cusick K., Iturbide A., Gautam P., Price A., Polson S., MacDonald M., et al. (2021). Enhanced copper-resistance gene repertoire in Alteromonas macleodii strains isolated from copper-treated marine coatings. PloS One. 16 (9), e0257800. doi: 10.1371/journal.pone.0257800
Da Silva S., Bernet N., Delgenes J. P., Moletta R. (2000). Effect of culture conditions on the formation of struvite by Myxococcus xanthus. Chemosphere. 40 (12), 1289–1296. doi: 10.1016/S0045-6535(99)00224-6
Debeljak P., Toulza E., Beier S., Blain S., Obernosterer I. (2019). Microbial iron metabolism as revealed by gene expression profiles in contrasted southern ocean regimes. Environ. Microbiol. 21 (7), 2360–2374. doi: 10.1111/1462-2920.14621
Deng S., Dong H., Lv G., Jiang H., Yu B., Bishop M. E. (2010). Microbial dolomite precipitation using sulfate reducing and halophilic bacteria: Results from qinghai lake, Tibetan plateau, NW China. Chem. Geology. 278 (3), 151–159. doi: 10.1016/j.chemgeo.2010.09.008
Diaz M. R., Eberli G. P., Blackwelder P., Phillips B., Swart P. K. (2017). Microbially mediated organomineralization in the formation of ooids. Geology. 45 (9), 771–774. doi: 10.1130/G39159.1
Falagán C., Yusta I., Sánchez-Espa A. ,. J., Johnson D.B.J.M.E. (2017). Biologically-induced precipitation of aluminium in synthetic acid mine water. Miner. Eng. 106, 79–85. doi: 10.1016/j.mineng.2016.09.028
Frankel R. B., Bazylinski D. A. (2003). Biologically induced mineralization by bacteria. Biomineralization. 54, 95–114. doi: 10.2113/0540095
Gago J. F., Viver T., Urdiain M., Pastor S., Kämpfer P., Ferreira E., et al. (2021). Description of three new Alteromonas species alteromonas antoniana sp. nov., Alteromonas lipotrueae sp. nov. and Alteromonas lipotrueiana sp. nov. isolated from marine environments, and proposal for reclassification of the genus Salinimonas as Alteromonas. Syst. Appl. Microbiol. 44 (4), 126226. doi: 10.1016/j.syapm.2021.126226
Gao X., Wen Y., Qu D., An L., Luan S., Jiang W., et al. (2018). Interference effect of alcohol on nessler's reagent in photocatalytic nitrogen fixation. ACS Sustain Chem. Eng. 6, 5342–5348. doi: 10.1021/acssuschemeng.8b00110
Gonzaga A., Martin-Cuadrado A. B., Lopez-Perez M., Megumi Mizuno C., Garcia-Heredia I., Kimes N. E., et al. (2012). Polyclonality of concurrent natural populations of Alteromonas macleodii. Genome Biol. Evol. 4 (12), 1360–1374. doi: 10.1093/gbe/evs112
Gonzalez-Munoz M. T., Martinez-Ruiz F., Morcillo F., Martin-Ramos J. D., Paytan A. (2012). Precipitation of barite by marine bacteria: A possible mechanism for marine barite formation. Geology. 40 (8), 675–678. doi: 10.1130/G33006.1
Guan Q., Zeng G., Song J., Liu C., Wang Z., Wu S. (2021). Ultrasonic power combined with seed materials for recovery of phosphorus from swine wastewater via struvite crystallization process. J. Environ. Manage. 293, 112961. doi: 10.1016/j.jenvman.2021.112961
Han Z., Sun B., Zhao H., Yan H., Han M., Zhao Y., et al. (2017). Isolation of Leclercia adcarboxglata strain JLS1 from dolostone sample and characterization of its induced struvite minerals. Geomicrobiol J. 34 (6), 500–510. doi: 10.1080/01490451.2016.1222469
Han Z., Yan H., Zhao H., Zhou S., Han M., Meng X., et al. (2014). Bio-precipitation of calcite with preferential orientation induced by Synechocystis sp. PCC6803. Geomicrobiol. J. 31 (10), 884–899. doi: 10.1080/01490451.2014.907379
Han Z., Zhao Y., Yan H., Zhao H., Han M., Sun B., et al. (2015). Struvite precipitation induced by a novel sulfate-reducing bacterium Acinetobacter calcoaceticus SRB4 isolated from river sediment. Geomicrobiol. J. 32 (10), 868–877. doi: 10.1080/01490451.2015.1016247
Ivars-Martinez E., Martin-Cuadrado A. B., D'Auria G., Mira A., Ferriera S., Johnson J., et al. (2008). Comparative genomics of two ecotypes of the marine planktonic copiotroph Alteromonas macleodii suggests alternative lifestyles associated with different kinds of particulate organic matter. ISME J. 2 (12), 1194–1212. doi: 10.1038/ismej.2008.74
Jeon C. O., Lim J. M., Park D. J., Kim C. J. (2005). Salinimonas chungwhensis gen. nov., sp,, nov., a moderately halophilic bacterium from a solar saltern in Korea. Int. J. Syst. Evol. Microbiol. 55, 239–243. doi: 10.1099/ijs.0.63279-0
Kanehisa M., Sato Y., Kawashima M., Furumichi M., Tanabe M. (2016). KEGG as a reference resource for gene and protein annotation. Nucl. Acids Res. 44 (D1), D457–D462. doi: 10.1093/nar/gkv1070
Korchef A., Saidou H., Amor M. B. (2011). Phosphate recovery through struvite precipitation by CO2 removal: Effect of magnesium, phosphate and ammonium concentrations. J. Hazard. Mater. 186 (1), 602–613. doi: 10.1016/j.jhazmat.2010.11.045
Lee S. H., Jun B. H. (2019). Silver nanoparticles: Synthesis and application for nanomedicine. Int. J. Mol. Sci. 20 (4), 865. doi: 10.3390/ijms20040865
Lelchat F., Cozien J., Le Costaouec T., Brandilly C., Schmitt S., Baudoux A. C., et al. (2015). Exopolysaccharide biosynthesis and biodegradation by a marine hydrothermal alteromonas sp. strain. Appl. Microbiol. Biotechnol. 99 (6), 2637–2647. doi: 10.1007/s00253-014-6075-y
Leng Y. R., Soares A. (2021). The mechanisms of struvite biomineralization in municipal wastewater. Sci. Total Environ. 799, 149261. doi: 10.1016/j.scitotenv.2021.149261
Li F., Jing X., Zou C., Zhang H., Xiang F. (2017). Facies analysis of the callovian–oxfordian carbonates in the northeastern amu darya basin, southeastern Turkmenistan. Mar. Petrol. Geol. 88, 359–380. doi: 10.1016/j.marpetgeo.2017.08.038
Liu J. W., Zheng Y. F., Lin H. Y., Wang X. C., Li M., Liu Y., et al. (2019). Proliferation of hydrocarbon-degrading microbes at the bottom of the Mariana trench. Microbiome. 7, 47. doi: 10.1186/s40168-019-0652-3
Loganathan P., Naidu G., Vigneswaran S. (2017). Mining valuable minerals from seawater: a critical review. Environ. Sci.: Water Res. Technol. 3 (1), 37–53. doi: 10.1039/C6EW00268D
Manck L. E., Espinoza J. L., Dupont C. L., Barbeau K. A. (2020). Transcriptomic study of substrate-specific transport mechanisms for iron and carbon in the marine copiotroph Alteromonas macleodii. mSystems. 5 (2), e00070–20. doi: 10.1128/mSystems.00070-20
Manzoor M. A. P., Duwal S. R., Mujeeburahiman M., Rekha P. D. (2018). Vitamin c inhibits crystallization of struvite from artificial urine in the presence of Pseudomonas aeruginosa. Int. Braz. J. Urol. 44 (6), 1234–1242. doi: 10.1590/S1677-5538.Ibju.2017.0656
Manzoor M. A. P., Mujeeburahiman M., Duwal S. R., Rekha P. D. (2019). Investigation on growth and morphology of in vitro generated struvite crystals. Biocatal. Agric. Biotechnol. 17, 566–570. doi: 10.1016/j.bcab.2019.01.023
McCarren J., Becker J. W., Repeta D. J., Shi Y., Young C. R., Malmstrom R. R., et al. (2010). Microbial community transcriptomes reveal microbes and metabolic pathways associated with dissolved organic matter turnover in the sea. Proc. Natl. Acad. Sci. U.S.A. 107 (38), 16420–16427. doi: 10.1073/pnas.1010732107
Moulessehoul A., Gallart-Mateu D., Harrache D., Djaroud S., de la Guardia M., Kameche M. (2017). Conductimetric study of struvite crystallization in water as a function of pH. J. Cryst. Growth. 471, 42–52. doi: 10.1016/j.jcrysgro.2017.05.011
Nayfach S., Rodriguez-Mueller B., Garud N., Pollard K. S. (2016). An integrated metagenomics pipeline for strain profiling reveals novel patterns of bacterial transmission and biogeography. Genome Res. 26 (11), 1612–1625. doi: 10.1101/gr.201863.115
Nies D. H. (1999). Microbial heavy-metal resistance. Appl. Microbiol. Biotechnol. 51 (6), 730–750. doi: 10.1007/s002530051457
Park Y., Faivre D. (2022). Diversity of microbial metal sulfide biomineralization. Chempluschem. 87 (1), e202100457. doi: 10.1002/cplu.202100457
Parte A. C., Sardà Carbasse J., Meier-Kolthoff J. P., Reimer L. C., Göker M. (2020). List of prokaryotic names with standing in nomenclature (LPSN) moves to the DSMZ. Int. J. Syst. Evol. Microbiol. 70, 11, 5607–5612. doi: 10.1099/ijsem.0.004332
Pedler B. E., Aluwihare L. I., Azam F. (2014). Single bacterial strain capable of significant contribution to carbon cycling in the surface ocean. Proc. Natl. Acad. Sci. U.S.A. 111 (20), 7202–7207. doi: 10.1073/pnas.1401887111
Purcell L., King A. (1996). Total nitrogen determination in plant material by persulfate digestion. Agron. J. 88, 111–113. doi: 10.2134/agronj1996.00021962008800010023x
Rivadeneiea M. A., Gonzalez-Lopez J., Ramos-Cormenzana A. (1985). Influence of ammonium ions on calcite and struvite formation byazotobacter in chemically defined media. Folia Microbiologica. 30 (1), 55–57. doi: 10.1007/BF02922498
Ronholm J., Schumann D., Sapers H. M., Izawa M., Applin D., Berg B., et al. (2014). A mineralogical characterization of biogenic calcium carbonates precipitated by heterotrophic bacteria isolated from cryophilic polar regions. Geobiology 12, 6, 542–556. doi: 10.1111/gbi.12102
Saito S. (1969). Salt effect on polymer solutions. J. Polym. Sci. Pol. Chem. 7 (7), 1789–1802. doi: 10.1002/pol.1969.150070719
Sanz-Saez I., Pereira-Garcia C., Bravo A. G., Trujillo L., Pla I. F. M., Capilla M., et al. (2022). Prevalence of heterotrophic methylmercury detoxifying bacteria across oceanic regions. Environ. Sci. Technol. 56 (6), 3452–3461. doi: 10.1021/acs.est.1c05635
Sarayu K., Iyer N. R., Annaselvi M., Murthy A. R. (2016). The micro-mechanism involved and wollastonite signature in the calcareous precipitates of marine isolates. Appl. Biochem. Biotechnol. 178 (6), 1069–1080. doi: 10.1007/s12010-015-1929-z
Sarmento H., Gasol J. M. (2012). Use of phytoplankton-derived dissolved organic carbon by different types of bacterioplankton. Environ. Microbiol. 14 (9), 2348–2360. doi: 10.1111/j.1462-2920.2012.02787.x
Sinha A., Singh A., Kumar S., Khare S. K., Ramanan A. (2014). Microbial mineralization of struvite: A promising process to overcome phosphate sequestering crisis. Water Res. 54, 33–43. doi: 10.1016/j.watres.2014.01.039
Spadafora A., Perri E., McKenzie J., Vasconcelos C. (2010). Microbial biomineralization processes forming modern Ca:Mg carbonate stromatolites. Sedimentology. 57, 27–40. doi: 10.1111/j.1365-3091.2009.01083.x
Sullivan A. L., Murdaugh A. E. (2014). The effects of marine bacteria on barite growth and morphology. Biophysic. J. 106 (2), 799a–799a. doi: 10.1016/j.bpj.2013.11.4381
Sun J. Y., Chen L., Wang X. X., Cao S., Fu W. J., Zheng W. C. (2012). Synthesis of struvite crystals by using bacteria Proteus mirabilis. Synth. Reac. Inorg. M. 42 (4), 445–448. doi: 10.1080/15533174.2011.611850
Sun B., Zhao H., Zhao Y., Tucker M. E., Han Z., Yan H. (2020). Bio-precipitation of carbonate and phosphate minerals induced by the bacterium Citrobacter freundii ZW123 in an anaerobic environment. Minerals. 10 (1), 65. doi: 10.3390/min10010065
Tada Y., Taniguchi A., Nagao I., Miki T., Uematsu M., Tsuda A., et al. (2011). Differing growth responses of major phylogenetic groups of marine bacteria to natural phytoplankton blooms in the western north pacific ocean. Appl. Environ. Microbiol. 77 (12), 4055–4065. doi: 10.1128/AEM.02952-10
Torres-Crespo N., Martinez-Ruiz F., Gonzalez-Munoz M. T., Bedmar E. J., De Lange G. J., Jroundi F. (2015). Role of bacteria in marine barite precipitation: A case study using Mediterranean seawater. Sci. Total Environ. 512, 562–571. doi: 10.1016/j.scitotenv.2015.01.044
Tu Q., Lin L., Cheng L., Deng Y., He Z. (2019). NCycDB: a curated integrative database for fast and accurate metagenomic profiling of nitrogen cycling genes. Bioinformatics. 35 (6), 1040–1048. doi: 10.1093/bioinformatics/bty741
van de Velde S. J., Burdorf L. D. W., Hidalgo-Martinez S., Leermakers M., Meysman F. J. R. (2022). Cable bacteria activity modulates arsenic release from sediments in a seasonally hypoxic marine basin. Front. Microbiol. 13. doi: 10.3389/fmicb.2022.907976
Vigliaturo R., Marengo A., Bittarello E., Perez-Rodriguez I., Drazic G., Giere R. (2020). Micro- and nano-scale mineralogical characterization of Fe(II)-oxidizing bacterial stalks. Geobiology. 18 (5), 606–618. doi: 10.1111/gbi.12398
Wang X., He X., Xia D., Sun M., An X., Lian B. (2022). The phase transformation of microbial induced struvite and its Cd(II) immobilization mechanism. J. Environ. Chem. Eng. 10 (3), 107695. doi: 10.1016/j.jece.2022.107695
Wei S. P., Cui H. P., Jiang Z. L., Liu H., He H., Fang N. Q. (2015). Biomineralization processes of calcite induced by bacteria isolated from marine sediments. Brazil J. Microbiol. 46 (2), 455–464. doi: 10.1590/S1517-838246220140533
Xue H. P., Zhang D. F., Xu L., Wang X. N., Zhang A. H., Huang J. K., et al. (2021). Actirhodobacter atriluteus gen. nov., sp. nov., isolated from the surface water of the yellow Sea. Antonie Van Leeuwenhoek. 114 (7), 1059–1068. doi: 10.1007/s10482-021-01576-w
Yasumoto-Hirose M., Yasumoto K., Iijima M., Nishino T., Ikemoto E., Nishijima M., et al. (2022). Mg-rich calcite-producing marine bacterium Pseudovibrio sp. isolated from an ascidian in coral reefs at Okinawa, Japan. Fisheries Sci. 88 (5), 625–634. doi: 10.1007/s12562-022-01627-9
Yoon J. H., Kang S. J., Lee S. Y. (2012). Salinimonas lutimaris sp nov., a polysaccharide-degrading bacterium isolated from a tidal flat. Antonie Van Leeuwenhoek 101 (4), 803–810. doi: 10.1007/s10482-011-9695-6
Zhang Y., Cremer P. S. (2006). Interactions between macromolecules and ions: the hofmeister series. Curr. Opin. Chem. Biol. 10 (6), 658–663. doi: 10.1016/j.cbpa.2006.09.020
Zhang D. F., Cui X. W., Li W. J., Zhang X. M., Xue H. P., Huang J. K., et al. (2021). Description of Salinimonas profundi sp. nov., a deep-sea bacterium harboring a transposon Tn6333. Antonie Van Leeuwenhoek. 114 (1), 69–81. doi: 10.1007/s10482-020-01501-7
Zhang P., Liu W. P., Zhao T. L., Yao Q. Z., Li H., Fu S. Q., et al. (2022). Biomineralization of struvite by Shewanella oneidensis MR-1 for phosphorus recovery: Cr(VI) effect and behavior. J. Environ. Chem. Eng. 10 (1), 106923. doi: 10.1016/j.jece.2021.106923
Zhang H., Wang H., Cao L., Chen H., Wang M. X., Lian C., et al. (2020). Salinimonas iocasae sp. nov., a halophilic bacterium isolated from a polychaete tube in a hydrothermal field. Int. J. Syst. Evol. Microbiol. 70 (6), 3899–3904. doi: 10.1099/ijsem.0.004258
Zhao Y., Yan H., Zhou J., Tucker M. E., Han M., Zhao H., et al. (2019). Bio-precipitation of calcium and magnesium ions through extracellular and intracellular process induced by Bacillus licheniformis SRB2. Minerals. 9 (9), 526. doi: 10.3390/min9090526
Zykwinska A., Berre L. T., Sinquin C., Ropartz D., Rogniaux H., Colliec-Jouault S., et al. (2018). Enzymatic depolymerization of the GY785 exopolysaccharide produced by the deep-sea hydrothermal bacterium Alteromonas infernus: Structural study and enzyme activity assessment. Carbohydr. Polym. 188, 101–107. doi: 10.1016/j.carbpol.2018.01.086
Keywords: Alteromonas, biomineralization, struvite, nitrate reduction, ammonia
Citation: He W, Xue H-P, Liu C, Zhang AH, Huang J-K and Zhang D-F (2023) Biomineralization of struvite induced by indigenous marine bacteria of the genus Alteromonas. Front. Mar. Sci. 10:1085345. doi: 10.3389/fmars.2023.1085345
Received: 03 November 2022; Accepted: 23 February 2023;
Published: 17 March 2023.
Edited by:
John Lee Ferry, University of South Carolina, United StatesReviewed by:
Ana Soares, Cranfield University, United KingdomRahul Nitnavare, Rothamsted Research, United Kingdom
Copyright © 2023 He, Xue, Liu, Zhang, Huang and Zhang. This is an open-access article distributed under the terms of the Creative Commons Attribution License (CC BY). The use, distribution or reproduction in other forums is permitted, provided the original author(s) and the copyright owner(s) are credited and that the original publication in this journal is cited, in accordance with accepted academic practice. No use, distribution or reproduction is permitted which does not comply with these terms.
*Correspondence: Dao-Feng Zhang, emRmQGhodS5lZHUuY24=; Chuang Liu, MjAxOTAwOTlAaGh1LmVkdS5jbg==