- 1Biological and Environmental Sciences, School of Natural Sciences, University of Stirling, Stirling, United Kingdom
- 2National Institute of Marine Geology and GeoEcology - GeoEcoMar, Bucharest, Romania
Many deltas around the world have recorded a decreasing sediment input, mostly due to retention in dams constructed on the river or in the river basin. The Danube River has recorded a significant decrease of its sediment supply to the Danube Delta and the NW Black Sea. This study uses 210Pb and 137Cs dating, to investigate the effects of the decreasing sediment flux in lakes, lagoons, delta front and prodelta area. Both the effects of the Iron Gate I and II dams and other local factors are discussed. These results define the period of 1960-1990 as the ‘major anthropic interventions period’ in the Danube Delta. Results show a decrease in siliciclastic flux, especially in lakes, the Sahalin lagoon and the prodelta area and a general increase in the Musura lagoon and the delta front area. Sand content is also shown to decrease in most areas and is replaced by silt. The changes in sediment accumulation rates depend mostly on the hydrological connectivity of the area and the local hydrotechnical works. Overall, the local anthropic interventions in the delta affect sediment flux in the subaerial delta and the delta front, while the prodelta is affected by the overall decrease caused by interventions in the river basin. This study can contribute to improving management strategies in the area and to a better understanding of future research needs in the Danube Delta-Black Sea system.
1 Introduction
The change in sediment budget is one of the most important human impacts on river-delta-sea systems (Syvitski and Kettner, 2011; Maselli and Trincardi, 2013; Day et al., 2016; Tessler et al., 2018). The sediment load of rivers is a key component in river basin and coastal management, for: stability and function of deltas and estuaries, navigation and harbour maintenance, coastal fisheries, benthic ecology, water quality, and geomorphic and geochemical flux studies (Syvitski, 2011).The sediment load of rivers varies at different time scales: short term (days to months) variations are related to catchment influences (e.g. floods variability), whilst long term (years to centuries) variations are related to river human activities (decennial to secular time scale) and climate variability (Pont et al., 2002). The suspended sediment budget of a river, especially on long term, depends on the geomorphic/geological influences of basin area (i.e. vertical lithospheric movements, sea level rise), lithology and relief, geographical influences of basin temperature, runoff and ice extent, and human activities that may accelerate or mitigate soil erosion and/or trap sediment (Walling and Fang, 2003; Syvitski and Milliman, 2007).
In the last two thousand years, the major world rivers showed an increase in their sediment loads due mainly to deforestation and increased soils erosion (Maselli and Trincardi, 2013), but have strongly decreased since the 1950s, due to dams and diversions within their drainage basins. Extensive scientific literature shows the effect of dams, both in use and whilst under construction, on diminishing sediment loads of rivers in several systems across the world (e.g. Panin and Jipa, 2002; Syvitski and Kettner, 2007; Wang et al., 2007; Xeidakis et al., 2010; Day et al., 2016; Latrubesse et al., 2017). The main effects are seen notably in the terminal areas of rivers, such as deltas or estuaries – coastal erosion, habitat loss and groundwater salinisation, just to name the most obvious.
Deltas, estuaries and marshes are river-sea interaction zones, and they concentrate an important part of the world resources, being hot spots for ecosystem services, for example food and energy production, touristic activities, shipping and navigation, and water supply. The main pressures on these areas currently result from: local hydrotechnical interventions, followed by local activities (fishing and aquaculture, agriculture, etc.), climate change (especially extreme events such as flood and storms) and changes in water and sediment fluxes that feed these transitional environments and support their ecosystems (Giosan et al., 2014; Tessler et al., 2018).
Fewer channels decrease the rate of delta-plain aggradation, whilst locally increasing the rate of sedimentation in the coastal zone near the channel mouths. Fewer channels also increase the rate of coastal erosion in areas that once received distributary sediment flux (Giosan et al., 2006). This is the case for the Indus and the Nile deltas (Overeem et Syvitski, 2009) as well as for the Danube (Stǎnicǎ and Panin, 2009; Giosan et al., 2013). Climate change, evidenced through changes in hydrological conditions (especially droughts) increased storminess and sea level rise, is resulting in more frequent storm surges that add to the loss of sediment in the coastal zone (Syvitski et al., 2009; Giosan et al., 2014; Tessler et al., 2015a; Wu et al., 2020).
The Danube River - Danube Delta - Black Sea is one of the largest river-delta-sea systems of Europe. Although the complex ecosystems in the Danube watershed provide resources and services to over 83 million people, they are under increasing pressure from multiple interacting human activities. The last century has been marked by extensive anthropic interventions in the Danube basin with the extent of agricultural lands, embankment for flood protection (Constantinescu et al., 2015a; Hein et al., 2016), construction of dams and reservoirs (Habersack et al., 2016) as well as direct interventions in the delta complex (Stănică et al., 2011; Panin and Overmars, 2012). Coastal erosion in the Danube Delta is a direct impact of these interventions (Panin and Jipa, 2002; Stǎnicǎ et al., 2007; Stǎnicǎ and Panin, 2009) however, the effect on the delta, delta front and prodelta needs to be studied further.
This research aims to address this gap in knowledge between the river, the delta and the delta front sedimentation. It builds, for the first time, a century scale chronostratigraphic framework (1900-2016) for sediment flux changes in the Danube-Black Sea transition zone, assessing the effects of a reduction in sediment supply from the sub-aerial delta to the Black Sea shelf.
2 Regional setting
2.1 The Danube-Black Sea System
The Danube has the largest drainage basin of the European Union (801,463 km²), and second largest in Europe, after Volga, comprising 19 countries, flowing 2857 km from Germany to the Black Sea. The Danube River is the most important source of water and sediment in the NW Black Sea, with a water discharge of about 205 km3/y (60% of the total water runoff in the Black Sea basin) and a sediment discharge of 36.3 to 52.4 Mt/y respectively (48% of the total sediment reaching the Black Sea) (Mikhailov et al., 2008; Ludwig et al., 2009) (Figure 1A). Together with the Dnieper, Dniester and Southern Bug, their basins comprise one third of continental Europe. The influence of the Danube waters extends far southwards, to the Bosphorous region, as well as to the deep-sea zone (Panin and Jipa, 2002).
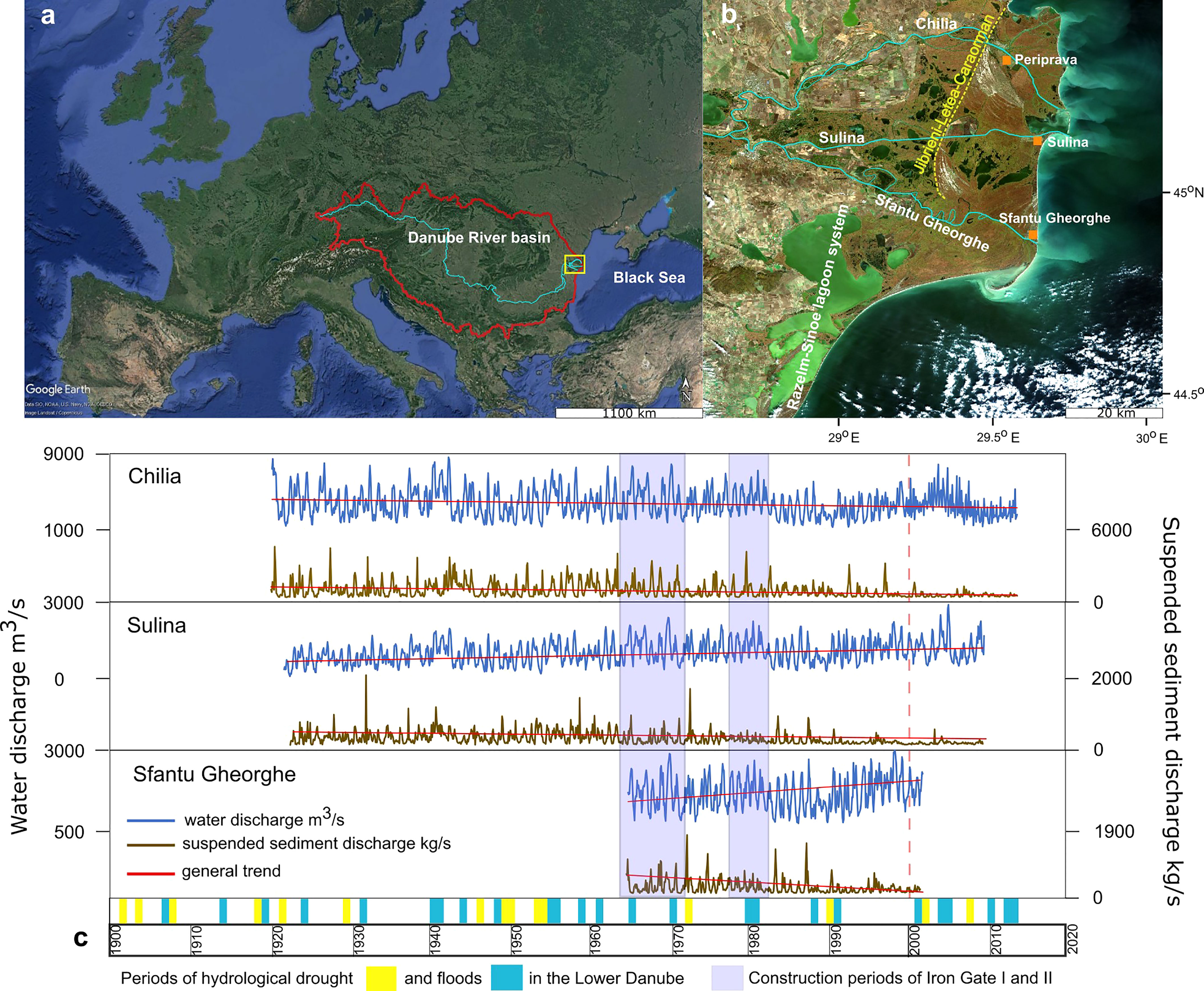
Figure 1 (A) Location of Danube Basin in Europe and of Danube Delta (Source image: Google Earth). (B) Danube Delta, with its three discharging arms, and locations of Periprava village, port of Sulina and Sfantu Gheorghe village (RGB image - mosaic of 4 Sentinel 2 images). (C) Monthly averages of water (blue line) and suspended sediment (brown line) discharge and general trend (red line) on Chilia Branch at Periprava (1965-2002) (GeoEcoMar; unpublished data), in port of Sulina (1921-2010) (Constantinescu; 2020); and at Sfantu Gheorghe village (1965-2002) (Tiron, 2010). The blue and yellow squares mark periods of major floods (1907, 1914, 1919, 1924, 1932, 1940-1941, 1944, 1947, 1954-1956, 1958, 1962, 1965, 1970, 1975, 1980-1981, 1988, 1991, 2002, 2005-2006, 2010, 2013-2014) and hydrological drought (1902, 1904, 1908, 1918, 1921, 1929, 1946, 1949-1950, 1953-1954, 1972, 1990, 2003, 2007) in the Lower Danube.
The Danube River basin landscape is very diverse, from mountain ranges (the Alps, the Carpathians) to hills and lowlands. The slope of the river valley decreases from 0.4‰ in the upper valley to 0.004‰ in the final 250 km before it enters the Black (Sommerwerk et al., 2009).
The Danube River basin covers several climatic zones, Atlantic influence in the west to temperate continental climate in the east. The maximum average precipitation is 3,200 mm/y in the high mountain range of the Alps and the most arid area is the lowland at the Black Sea with an average of only 350 mm/y. Extreme events like floods and droughts are common in the basin, with around 26 major floods and 15 extreme drought periods, recorded since the beginning of the 20th century (Bondar and Panin, 2001; Bondar, 2003; Pekarova and Pekar, 2006; Mikhailov et al., 2008; Cioaca et al., 2010; Bondar and Iordache, 2012; Mikhailova et al., 2012; Pekárová et al., 2013; GeoEcoMar unpublished data).
Nowadays, the Danube Basin is home to 83 million people (average density of 103 persons/km2), from which 20 million depend on the river for their drinking water (ICPDR 2009). Land use changes (expansion of agricultural land and deforestation) occurred in Europe and thus all around the Danube basin as early as 7500 y BP (Giosan et al., 2012). The last century, particularly its second half, land use changed, especially the extent of wetlands. In Romania (Lower Danube), in the last 60 years for example, the conversion of the Lower Danube wetlands and part of the Danube Delta, decreased their area to 22% and to 80%, respectively, from the initial surface (Vadineanu et al., 2003), leading to a decrease in flood attenuation capacity of 4.5 km3 (Cristofor et al., 2003). Nowadays, land in the basin is mainly used for agriculture (45%) and forest areas (35%), grasslands and heathlands (15%), while urban areas only occupy 5% and waterbodies less than 2% (Karabulut et al., 2016).
2.2 Danube-Black Sea transitional area
The Danube discharges into the NW Black Sea over a wide and flat area, the Danube Delta (Figure 1B). It is the largest delta in the European Union (second largest in Europe after the Volga). Its present-day morphology is the results of the interaction between the river and sea level changes in the last 10,000 years (Panin and Jipa, 2002). The total area of the sub-aerial Danube Delta is 5800 km2, of which one fifth is located in Ukraine. The average altitude is of about 0.52 m above the Black Sea mean level, with 93% of delta territory under 2 m (Bondar and Panin, 2001). It has two distinct regions, separated by the Jibrieni-Letea-Raducu-Ceamurlia-Caraorman line: the fluvial delta, with a network of channel and lakes, constructed in the former Danube Bay, and the marine delta, developed in the Black Sea, characterised by alongshore oriented beach ridges (Panin, 1996).
The present-day hydrological network consists of three main branches i) Chilia, 111 km long, <50% of the total discharge, ii) Sulina, the branch opened for maritime navigation, 83 km, 19-23% of discharge and iii) Sfantu Gheorghe, 108 km long initially, and 70 km after meander cutting, 24-30% of total discharge (Popa, 1997; Bondar and Panin, 2001) and 500 km of channels and canals, connecting around 500 lakes (Cioaca et al., 2009) (Figure 1B).
The cutting of Sulina and Sfantu Gheorghe (middle and southernmost) branches meander loops altered the hydrologic conditions locally, shifting the distribution of water and sediment discharge in all three distributaries (Jugaru Tiron et al., 2009; Panin and Overmars, 2012), decreasing on Chilia and increasing on Sulina and Sfantu Gheorghe (Figure 1C). This resulted in a change of grain size and distribution of bottom sediments. Nowadays, along these two branches the bottom sediments are dominated by fine sand and silt, while on Chilia the bottom sediments are fine sand, silt and clay (Oaie et al., 2005; Opreanu et al., 2007). Most of the lake sediments of the delta plain consist of clay (20-30%), silts (>65%), fine sand (6-15%), and shell fragments (2-4%) (Mihailescu et al., 1996) and are significantly bioturbated (Mihailescu et al., 1982).
The entrance and circulation of the Danube waters in the delta depends on the hydrologic regime of the river (Bondar, 1994), brought by the main distributaries and conveyed laterally through channels and canals, but also by overbank spilling when the water discharge at the delta apex exceeds 9100 m3/s (Bondar, 1996). Bondar (1994) calculated that the water discharge of the Danube releases in the delta plain increased from 1858 to 1980s almost four times.
The sediment transfer of the Danube onto the Black Sea shelf and the formation and development of the delta front and prodelta, as well as into the deep basin was mainly controlled by climate, the hydrological regime of the Danube and sea level for the last 30,000 years (Popescu et al., 2004; Lericolais et al., 2009; Constantinescu et al., 2015b). The recent sediment patterns are described by Panin and Jipa (2002), as grouped into two main regions with different depositional processes: the internal shelf, largely corresponding to the delta front (1300 km2) and prodelta (6000 km2) (A and B in Figure 2), fed by the Danube, and the sediment starved external area. The external sediment starved shelf sedimentation is dominated by relict sediments or shells and it rarely receives direct inputs of the Danube siliciclastic sediments (Panin and Jipa, 2002). The Danube-Black Sea interaction zone is thus largely restricted mainly to the internal shelf.
The sediment input from the Danube branches is reworked by waves and currents. The astronomic tide does not have an important role, as the Black Sea has a microtidal regime (7- 11 cm; Bondar et al., 1973). The longshore sediment drift is generated by the wind and wave longshore current system, which transports up to 1.23 million m3/y of sediment (Giosan et al., 1997). The wave climate in the NW part of the Black Sea is dominated by three main directions (Dan et al., 2007; Dan et al., 2009). The northern directions, from N to ENE, have an occurrence of 102 days/y, mostly in winter season. These waves have the largest average heights and are produced by winds with the highest speeds (34 and 40 m/s north). The eastern direction, E to SE, have the lowest average wave heights and they only occur less than 30 days/y. The Southern directions, from SSE to WSW produce medium average wave heights and occur around 90 days/year. The maximum wind speed from these directions is 30 m/s (from south-west direction) and they occur mostly in summer season (Dan et al., 2009). Storms play an important role in the short-term dynamics of the shoreline and increase the longshore sediment transport (Vespremeanu-Stroe et al., 2007; Stănică et al., 2011). Recent results of wind data analysis (Dan et al., 2009; Stănică et al, 2011) for the time interval of 1991 to 2000, for the NW Black Sea, consider extreme events to occur 1 or 2 times in ten years (wind speeds 28-40 m/s) and milder storms which occur almost yearly (wind speeds 15-19 m/s).
The Danube plume forms from Chilia and Sulina in the N and Sfantu Gheorghe in the S. Chilia’s waters and sediment prevail in the northern plume, while the waters of Sfantu Gheorghe usually follow the Sahalin sediment spit and remain confined to the shores (Bajo et al., 2014). The plume extension is mainly restricted to the coast and shelf (maximum offshore extension of 70 km; Güttler et al., 2013), with a penetration depth of 15 m near the coast (Karageorgis et al., 2009), while the influence of the Danube waters, traced through simulated inorganic N/P molar ratios at the sea surface, can extend to the Turkish coast (Tsiaras et al., 2008). The main controlling factors for the formation and extension of the plume are hydrological (solid and liquid river discharge that varies seasonally), meteorological (wind speed and direction), topography of the shoreline and the Coriolis force (Panin, 1999; Karageorgis et al., 2009; Güttler et al., 2013). Wind seems to be the primary factor for surface water direction of flow but the haline stratification can limit its influence in the deeper layers, especially near the delta, where the water is more stratified (Bajo et al., 2014).
2.3 Sediment fluxes in the Danube-Black Sea System. From basin to local factors
The history of human occupation in the Danube Basin starts in the Palaeolithic but it is the last 150 years which brought important changes in water and sediment flux due to anthropic pressure. Both anthropic and climatic factors have acted over time to the change in sediment and water flux, but it is very difficult to separate their effects.
The sediment flux was altered by land use changes (deforestation and intensification of agriculture), embankment and loss of wetlands (Constantinescu et al., 2015a; Habersack et al., 2016), sediment extraction for construction material and dredging for navigation (Schwarz, 2008), and construction of dams and reservoirs. Nowadays, there are hundreds of dams in the Danube Basin, 187 dams in Romania alone (Rãdoane and Rãdoane, 2005) and 78 along the Danube (Habersack et al., 2016). Among these, Iron Gate I and II, built between 1964 and 1984, are the largest. Since their construction, they trap more than 20 Mt of sediment per year, decreasing the sediment budget downstream the dam by 50-70% (Panin and Jipa, 2002; McCarney-Castle et al., 2011) and hence, the suspended sediment discharge on the main Danube branches (Figure 1C) (GeoEcoMar, unpublished data; Constantinescu, 2020; Tiron, 2010). Presently, 341 dams are planned for or are under construction in the Danube basin, mainly in the Upper Danube and the Balkans (Zarfl et al., 2014).
The sediment transported by the upper and middle Danube is mainly represented by fine fractions (silt), most of the sand stopping in the Iron Gates dams (Iron Gates I - about 950 kilometres upstream of the river mouths, Oaie et al., 2005) while the sand material reaching the Danube Delta originates from erosion and scouring of the riverbed downstream the Iron Gates II Dam (about 800 kilometres upstream of the river mouths, Bondar, 2008). The sediment supply reaching the delta is 25–35 million t/y, out of which 4–6 million t/y of sand, which are the only sediments supplying the inner shelf and littoral zone (Panin and Jipa, 2002).
The period of major anthropic interventions in the Danube Delta (canal cutting, creation of fish-ponds and agricultural polders) was between 1960 and 1990 (Stănică et al., 2011). The cutting and dredging of shallow canals were a constant and extensive action in the Danube Delta, since the beginning of the 20th century (Antipa, 1941), and it accelerated after the WWII (the total length of the canals doubled; Gâsteşcu et al., 1983). They were dug to improve access for fishing and reed harvesting (Oosterberg et al., 2000). This network of channels is considered to play an important role in the present-day sediment transport and accumulation in the sub-aerial delta, by increasing water and sediment delivery and overbank sediment transfer and keeping the sedimentation rates above the contemporary relative sea level rise rate in the Black Sea, i.e., 3-4 mm/y (Panin, 1999; Giosan et al., 2013).
The formation and transformation of the Danube Delta coastal zone, including the three mouth areas of the main branches, has been under the influence of both natural and anthropic factors over the last 150 years (Giosan et al., 1999; Ungureanu and Stanica, 2000; Stǎnicǎ et al., 2007; Stǎnicǎ and Panin, 2009; Stănică et al., 2011). This period is marked by the cutting of the Sulina distributary and changes in water and sediment load (from 9% in 1860 to 20% presently; Figure 1C) on the distributary as well as the gradual construction of the Sulina jetties, which reached a length of 8 km (Panin, 1999). This pair of jetties acts like a barrage, blocking most of the alongshore drift from the secondary delta of Chilia branch towards S. This resulted in the clogging of Musura Bay (updrift of the jetties), sediment starvation for the littoral areas downdrift, increased erosion in the Sulina - Sfantu Gheorghe sector (downdrift of the jetties), and a dramatic decrease or even cessation of sediment supply to the southern shores of the Danube Delta (the sand bar closing the Razelm-Sinoe lagoon) (Stănică et al, 2007; Stǎnicǎ and Panin, 2009; Stănică et al., 2011). A sand bar forms periodically in front of Sulina mouth, originating from bed-load accumulation, which is dredged to maintain the water depth for navigation (8 m). The sediments are discharged offshore, so they are not part of the near-shore transport system (Giosan et al., 1999; Stǎnicǎ et al., 2007).
The Chilia secondary delta (Chilia III) developed and grew strongly beginning with the 19th century, prograding N, E and S, with a shallow platform towards E and S (Panin and Overmars, 2012). The decrease in sediment delivery in the 20th century, due to the extent of the lobe in deeper water, has slowed accretion, leading even to a general erosion trend that can still be observed today (Filip and Giosan, 2014).
Sfantu Gheorghe branch developed a secondary delta, with a shallow delta front platform (Sfantu Gheorghe II). This is a wave-controlled delta, formed with the sediment brought by the river and then distributed in beach ridges by the coastal current (Panin, 1996; Panin and Overmars, 2012). This delta is still prograding today and is protected by the Sahalin sediment spit in the south. Sahalin grew from 1897 (Antipa, 1941), lengthening by 200 m/y on average, and migrating by overwash towards the shore (30-70 m/y), with a tendency of closing the Sahalin lagoon in the next two centuries (Dan et al., 2011; Panin and Overmars, 2012). Between 1984 and 1989, the naturally meandered Sfantu Gheorghe branch was cut to improve navigation conditions. This shortened the branch and increased the hydraulic slope, which resulted in an increase in the water and sediment flow, changing the dynamics of the cut meanders (Jugaru Tiron et al., 2009; Figure 1C).
The fate of the Danube sediments, fine sand, silt and clay (Oaie et al., 1999), discharged through its three mouths, depends on the wave regime, wind speed and direction and fluvial discharge (Bondar, 1998; Karageorgis et al., 2009; Güttler et al., 2013; Constantin et al., 2017). The coarser fraction settles near the mouths, and forms sediment spits – Musura, Sahalin, and part of it feeds beach sectors, while the finer fraction is transported in suspension and disperse on the shelf (Bondar, 1998; Oaie et al., 1999; Panin, 1999; Panin and Jipa, 2002). These studies (Panin and Jipa, 2002; Oaie et al., 2004), however, don’t provide a quantification of sedimentary rates on the delta front. Oaie et al. (2004) gives an average sedimentation rate of 4 cm/y for the prodelta, characterising sedimentation as dominated by the terrigenous input of the Danube, represented by well sorted silt and silty clay. The difference in sedimentation between the internal shelf and the external shelf is considerable. In a core retrieved from the shelf edge, Ayçik et al. (2004) found a sedimentary rate of 0.20 ± 0.01 cm/y (210Pb) to 0.15 ± 0.03 cm/y (137Cs). Numerical simulations showed that fine sediments could cross the continental slope propagating into the deep ocean, while coarser fraction has a very limited penetration into the deeper areas (Stanev and Kandilarov, 2012).
Understanding the effect of both basin and local factors and quantifications of sediment flux are essential for an effective management of river-sea transitional zones, such as deltas and wetlands. However, very often, studies are restricted to sub-aerial deltas which are of main economic interest. This study will evaluate the effects of a reduced sediment flux on the entire delta complex, for ca. 100 years (1900-2015), discussing both basin and local influencing factors.
3 Materials and methods
3.1 Sedimentary cores
This study is based on twelve cores retrieved from the Danube subaerial delta, delta front and prodelta area. Seven sediment cores were retrieved from various environments in the Danube Delta in August 2015, on Board R/V Istros (Figure 2, Table 1), from areas with different sedimentary patterns and connectivity to the hydrological network: one core was collected from the fluvial delta, one from the marine delta, both in lakes connected to the main branches through channels; two cores from active channels in the active delta of Sfantu Gheorghe; three in the terminal lagoons Musura and Sahalin, representing the active deltas of Chilia and Sfantu Gheorghe.
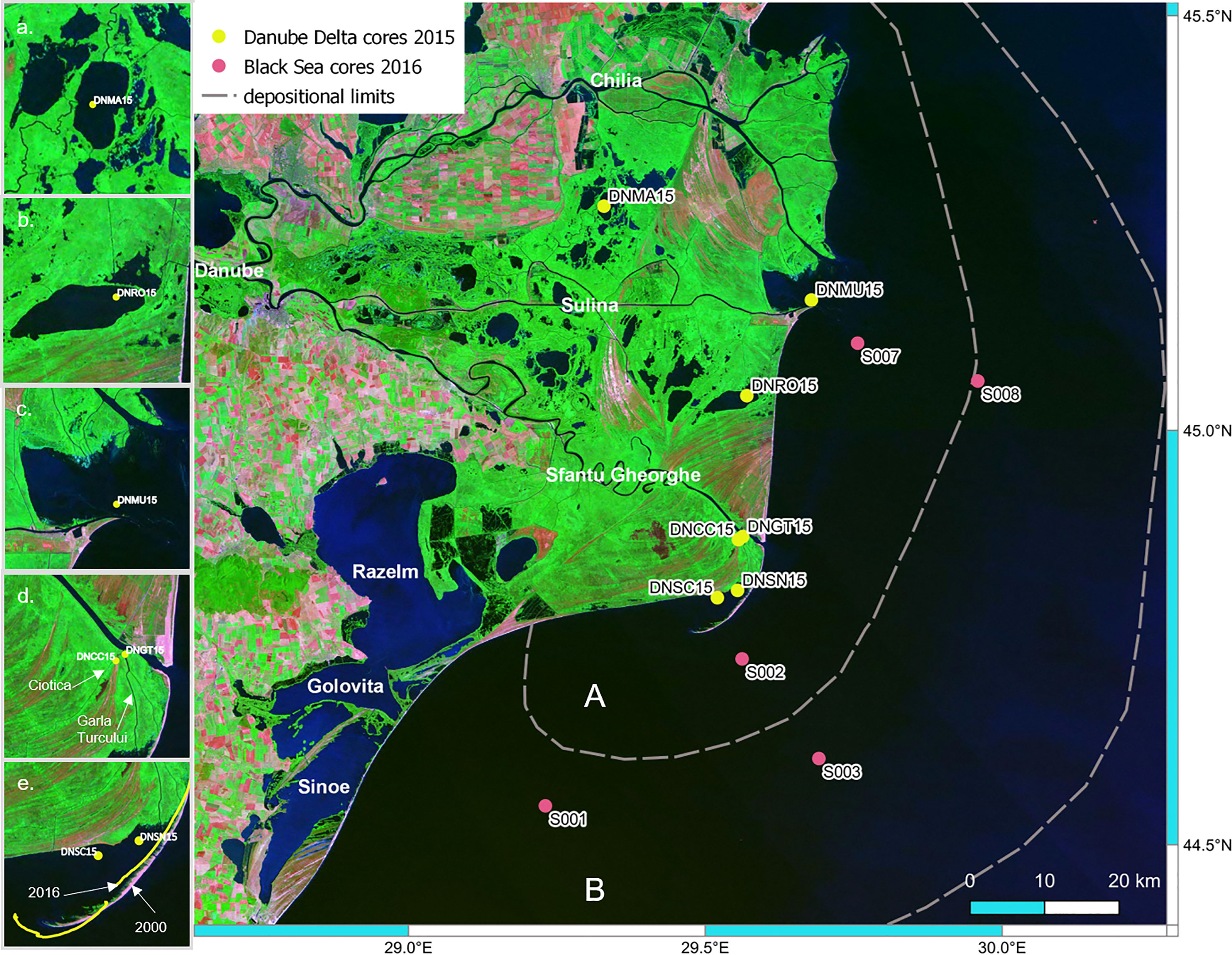
Figure 2 The Danube Delta and the NW Black Sea shelf, with the locations all cores used for this study (false colour Landsat images). The dotted lines represent the depositional limits of the delta front (A) and prodelta (B) (modified, after Panin and Jipa, 2002). The exact location of all cores is presented in Table 1. Detailed locations of the cores retrieved in the Danube Delta are: (a) Lake Matita, (b) Lake Rosu, (c) Musura Lagoon, (d) Garla Turcului and Canal Ciotica, (e) Sahalin Lagoon (note Sahalin sand bar position in 2000 and 2016; GeoEcoMar, unpublished data).
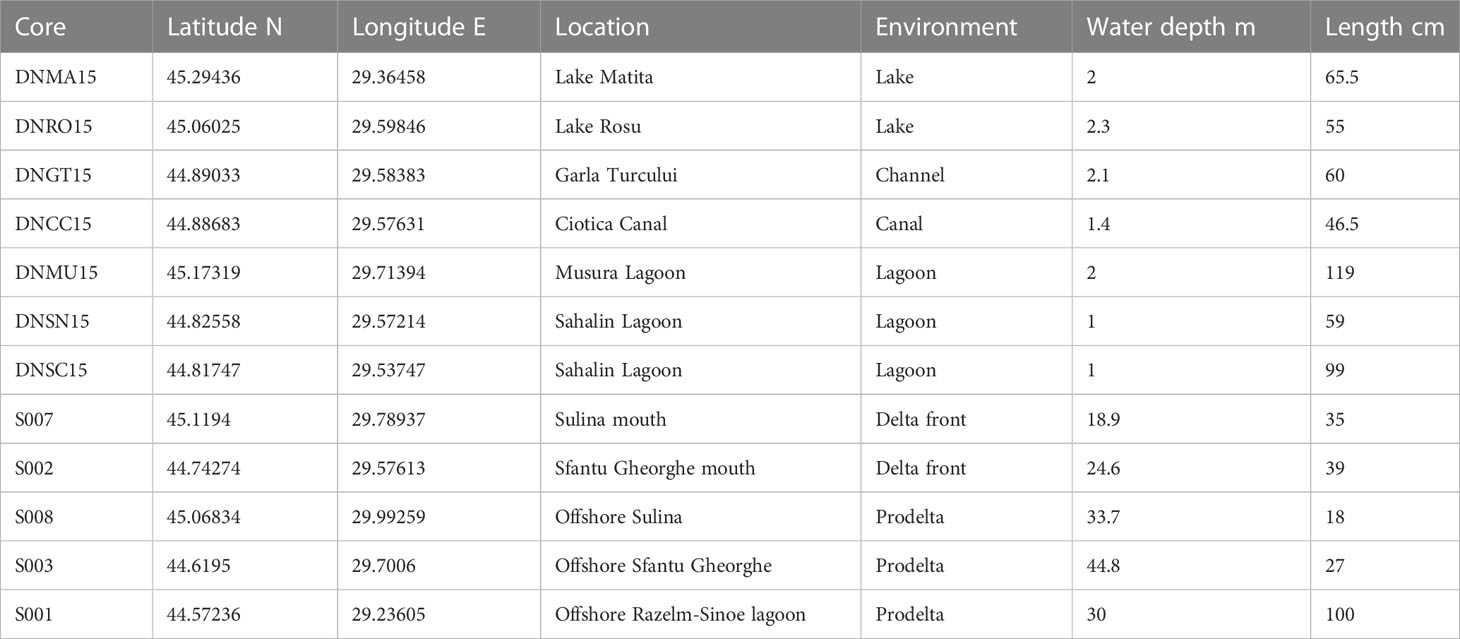
Table 1 Key parameters of the sedimentary cores analysed in this study, including name, geographical position, geographic location, physiography, water depth and length.
Five cores were retrieved from the NW Black Sea shelf, in the delta front and prodelta of the Danube in May 2016, during the EuroFleets 2 ReCoReD research cruise on board R/V Mare Nigrum (Figure 2, Table 1). Sampling locations were chosen to capture the depositional areas of the Danube plume, and the direction of the southern drift. The cores were collected with a Mark II Octopus Multicorer, equipped with 8 perspex tubes of 60 cm in length and 10 cm in diameter, and a gravity corer (S001), available on board the RV.
3.2 Sediment analysis
All cores were photographed and described visually. Sub-samples of 1 to 3 cm were split for both grain size analysis and 137Cs and 210Pb radiometric dating. For each sample, wet and dry weight and water content were determined (samples were dried in ovens at 55°C for 48h). In addition, the shell content was measured for each sample.
The grain size analysis was done using a Coulter Counter LS 230. The instrument measures the size distributions of particles with a size range of 0.04 to 2000 µm. Control samples were used at the beginning of each working day, to check distribution of particle sizes. Measurements were repeated for those samples where the distribution curves were noisy.
3.3 Chronostratigraphic framework
All sediment samples were ground after drying and made into pellets of known volume and mass. Samples were analysed in the ISO 17025 accredited ERL (Environmental Radioactivity Laboratory) of the University of Stirling, with two high purity-Germanium, low 210Pb background GEM HPGe detectors. All efficiency calibrations were performed including 210Pb and 137Cs within a mix of gamma emitting radionuclides and are traceable to international standard reference materials for a range of environmental media and account for variations in self-absorption as result of changes in sediment composition and sample density. All cores were analysed for 210Pb and 137Cs, and excess 210Pb calculated following the subtraction of intrinsic 210Pb estimated from 214Bi and 214Bi daughters of 226Ra following a minimum of 21 days of 222Rn equilibration. This provided a 137Cs and an excess 210Pb depth profile (Figures 4 and 5) from which a ca. 100 years chronology of the sedimentary processes and their magnitude across the delta and the shelf could be estimated.
The CRS (Constant Rate of Supply) model (Appleby and Oldfield, 1978; Swarzenski, 2014) was considered to be appropriate for obtaining an estimate of the age profiles for all cores, because it was proven to be more reliable, coupled with 137Cs, in environments with very variable sedimentation rates (Appleby, 2008) that also result in complex grain size profiles. This model has been previously used by Begy et al. (2018) to date sediments from the Danube Delta lakes. The estimated excess 210Pb obtained ages were compared with the 137Cs activity profiles, including the initial and second rise (1954 and 1961-1963) and the Chernobyl event (1986). The comparison between these ages were typically found to coincide to within 1 to 5 years.
Mass accumulation rates (MAR) were calculated for all cores (Figures 6, 7, Table 2), based on the 210Pb CRS age model, using mass depth, that expresses the mass of sediment (grams) accumulated per unit (cm2) area as a function of dry sample density for each sampled layer. This takes into account sample compaction and is a more meaningful measure of sedimentation per length when reconstructing sediment accumulation and budgets. Simple linear depth fails to account for compaction and is therefore misleading in any interpretation of change with time. MAR are described for the periods pre- and post-dams (before and after 1984). For purposes of comparison with published data, sedimentation rates (SR) (cm/y) were also computed pre- and post-dams (Table 2). The 137Cs was used to validate and check the 210Pb CRS age model. MAR and SR are also calculated using the 1963- and 1986-time horizons. The year 1986 can be considered the limit between the pre- and post-dam periods, because Iron Gate II was finished and functional by that time. The MAR at 1963 is thus comparable with the pre-dams value, while the one calculated at 1986 can be compared with the post-dam value. They are presented for comparison in Table 2.
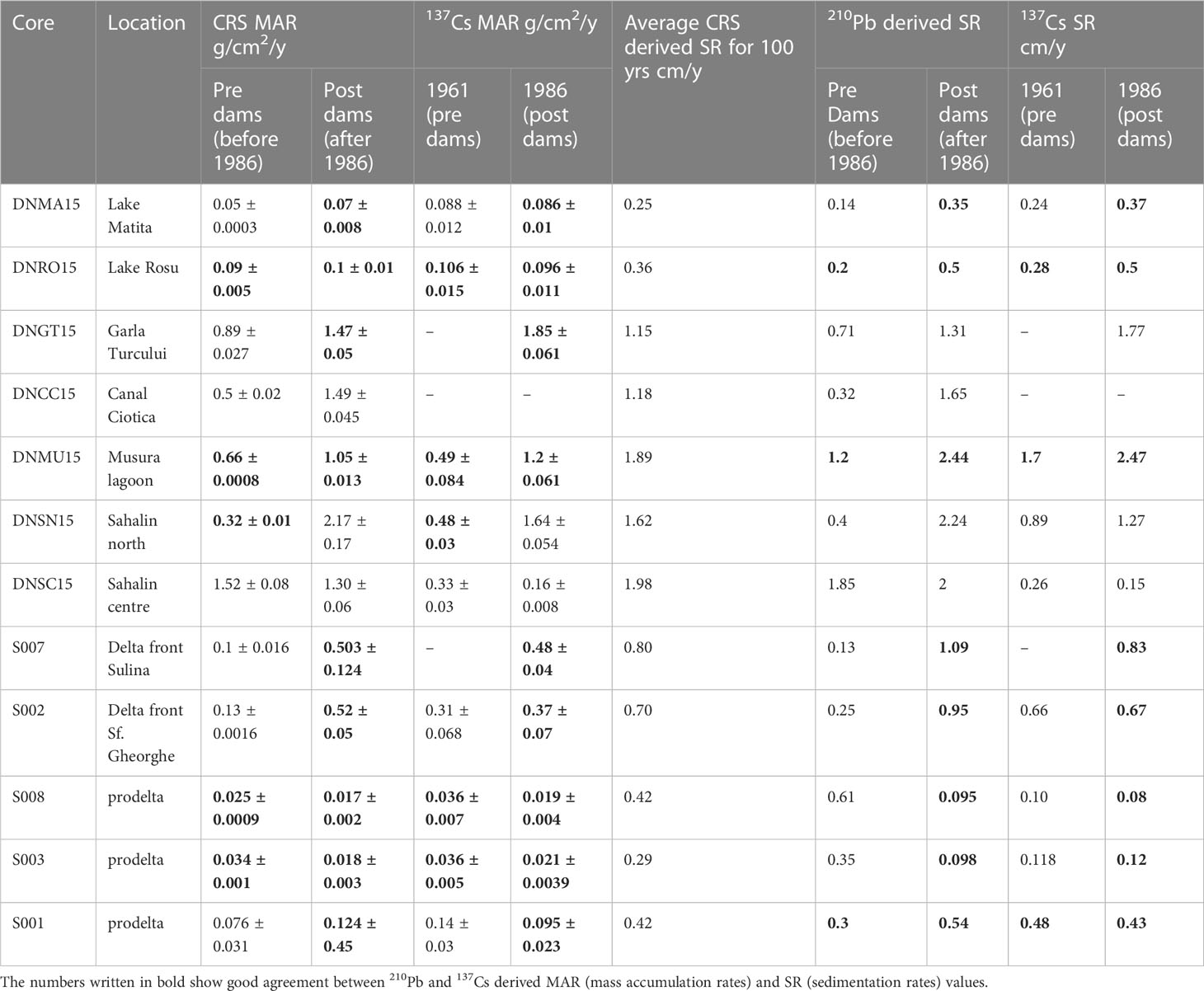
Table 2 Average mass accumulation rates (MAR) and sedimentation rates (SR), calculated based on 210Pb and 137Cs dating.
4 Results
4.1 Sedimentary facies
4.1.1 Lakes
The sediment accumulated during the studied period in the studied lakes (cores DNMA15 and DNRO15) (Figure 3) is characterised by a distinct brown layer of sandy silt (Catianis et al., 2014), very rich in fragments of shell and vegetation (25 cm in Lake Matita and 26 cm in Lake Rosu). In Lake Rosu, a light-grey, laminated sandy silt layer (26-35 cm) precedes the brown layer.
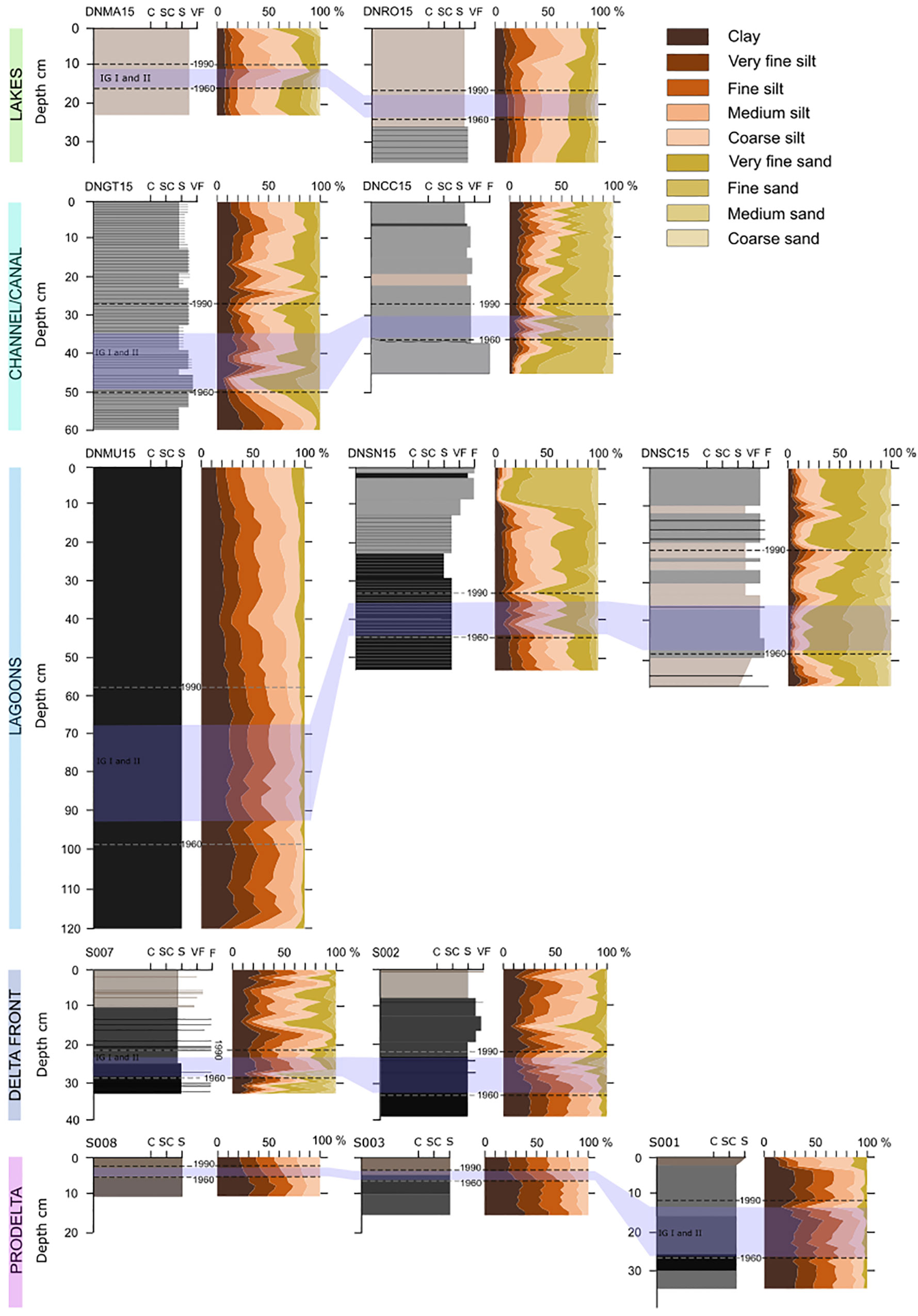
Figure 3 Sedimentary logs (real colour from visual descriptions) and grain size (in percentage of fractions) for the analysed cores from the Danube Delta, delta front and prodelta areas, showing the period of major anthropic interventions 1960-1990 (dotted lines) and the period of construction of Iron Gate I and II (1964-1984) (light purple markers).
4.1.2 Channels
In the studied channels, the sediment succession is an alternance of grey sandy silt and silty sand (core DNGT5 - 60 cm in Garla Turcului and DNCC15 - 46 cm in Canal Ciotica), very finely laminated, especially in core DNGT15 (Figure 3).
4.1.3 Lagoons
The core in Musura lagoon, DNMU15, consists only of very well sorted black silt (119 cm), very homogenous in both texture and colour, with no internal structure. The sediment shows signs of anoxia, changing colour immediately after opening of the core. The sedimentary sequence in the Sahalin lagoon is very different, with 58 cm in the centre, DNSC15, and 54 cm in the N, DNSN15, of alternating grey silty sand and sandy silt, with some differences between the two areas (coarser sediment in the centre, signs of anoxia in the northern core, which also becomes coarser at the top) (Figure 3).
4.1.4 Delta front
The sedimentary sequence of the studied period is very similar for both sampled areas, with a brown silt layer at the top (11 cm in S007 and 8 cm in S002), a grey sandy silt layer (11-26 cm in S007 and 8-23 cm in S002), and a black clayey silt in the bottom (Briceag and Ion, 2014). Both cores show distinct sand laminae (Figure 3).
4.1.5 Prodelta
Sedimentary sequences are very different in the E (cores S008 and S002) and the S (core S001). In the E they consist of silt of different colours, with a very high percentage of shells (up to 80%). Core S001 consists mostly of clayey silt of different colours, with a very low shell content (<5%) (Figure 3).
4.2 Evolution of sediment accumulation and grain size variations
The values for the mass accumulation rates (MAR) are based on the 210Pb and 137Cs profiles (Figures 4, 5), and they are presented, in comparison, in Figures 6, 7 and Table 2.
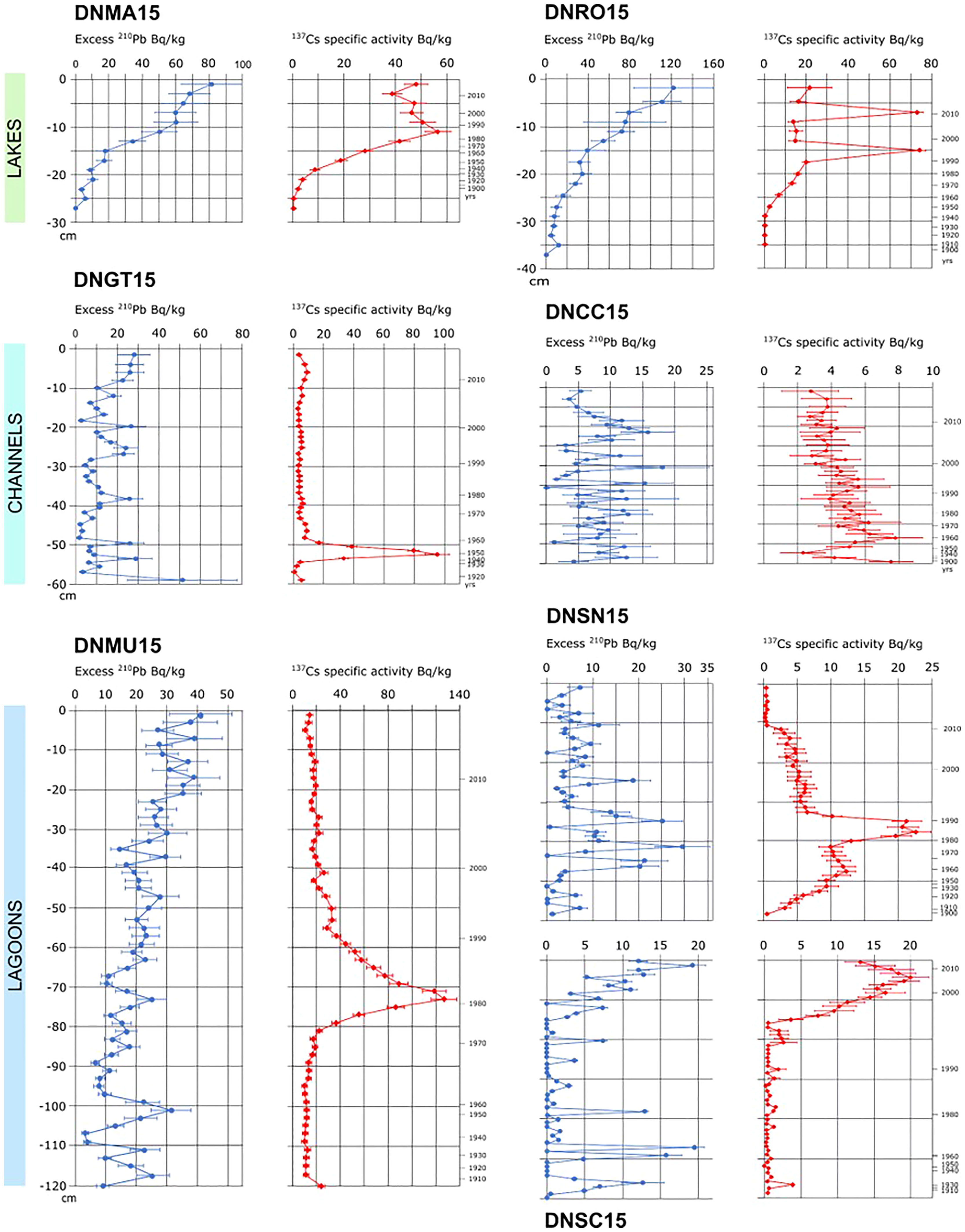
Figure 4 Excess 210Pb (Bq/kg) with depth in the core (blue graph) and 137Cs activity with depth (red graph) for the Danube Delta cores.
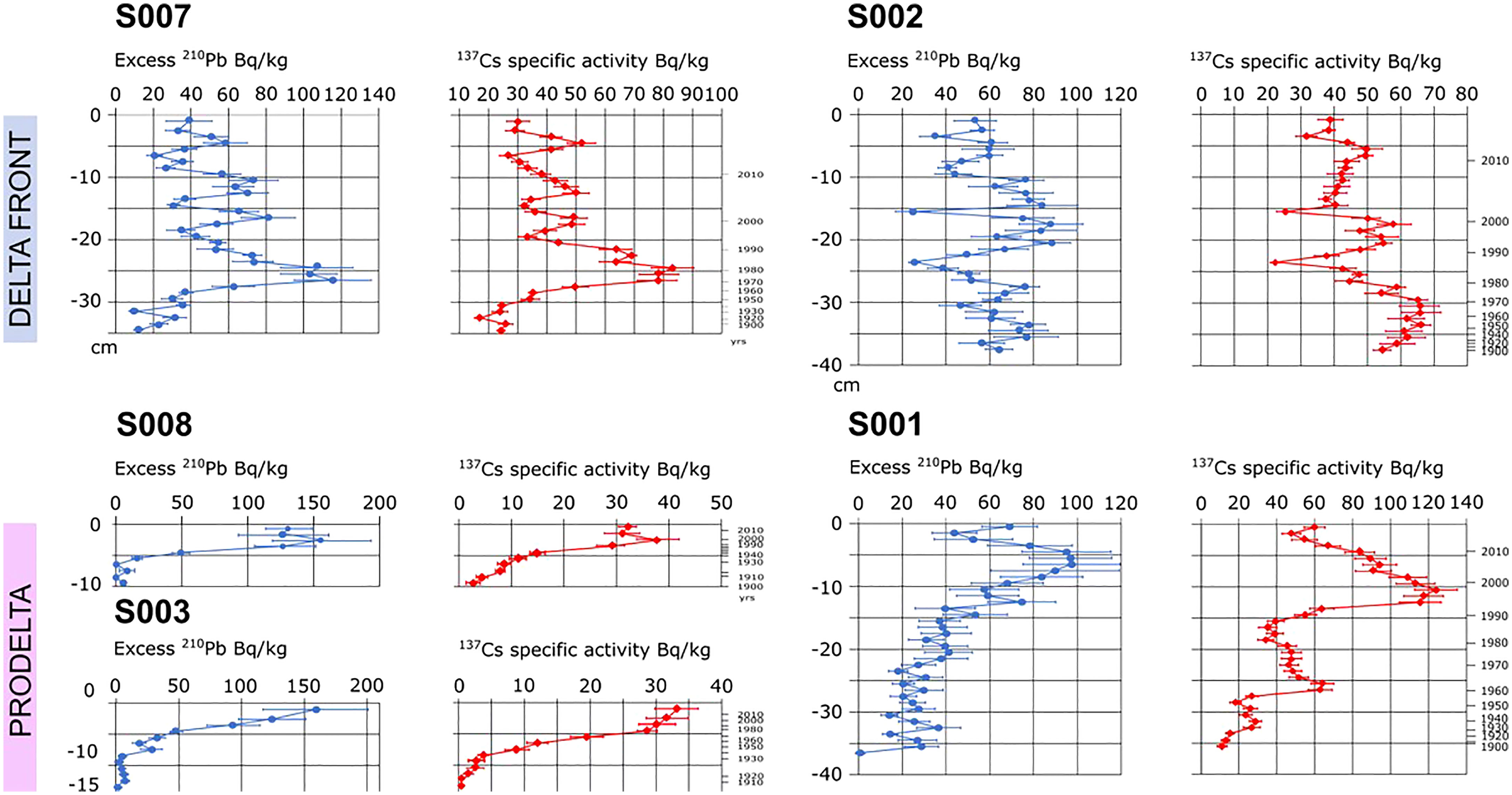
Figure 5 Excess 210Pb (Bq/kg) with depth in the core (blue graph) and 137Cs activity with depth (red graph) for Danube delta front and prodelta areas.
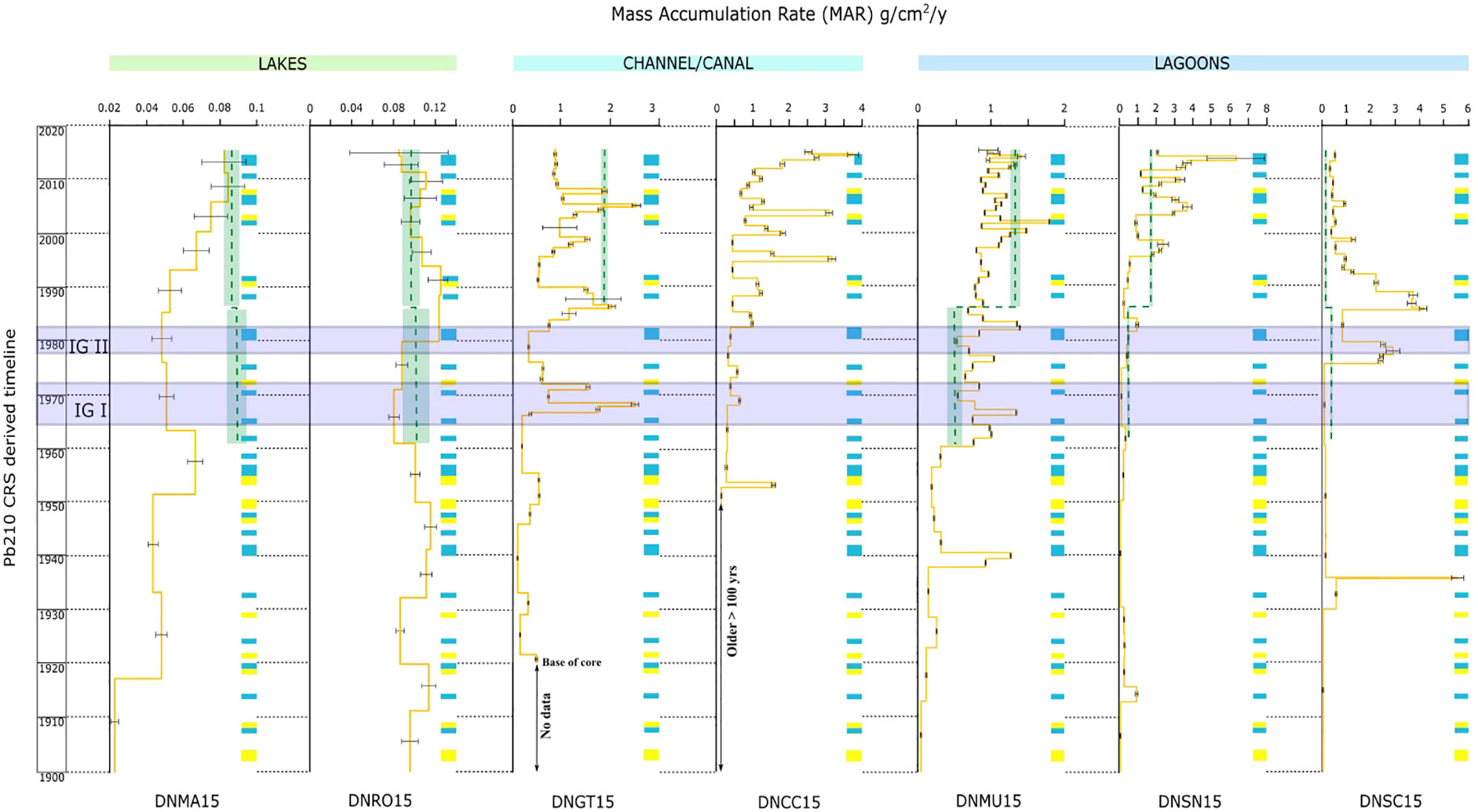
Figure 6 Mass accumulation rates in g/cm2/y for lakes, channels and lagoons of the Danube Delta, based on the time line obtained from 210Pb dating (orange line), using the CRS (Constant Rate of Supply) model and 137Cs (green line). The input of dams on the time line (Iron Gate I – 1964-1972 and Iron Gate II – 1978-1982) is marked by the purple squares. The blue and yellow squares mark major floods (1907, 1914, 1919, 1924, 1932, 1940-1941, 1944, 1947, 1954-1956, 1958, 1962, 1965, 1970, 1975, 1980-1981, 1988, 1991, 2002, 2005-2006, 2010, 2013-2014) and hydrological drought (1902, 1904, 1908, 1918, 1921, 1929, 1946, 1949-1950, 1953-1954, 1972, 1990, 2003, 2007) in the Lower Danube.
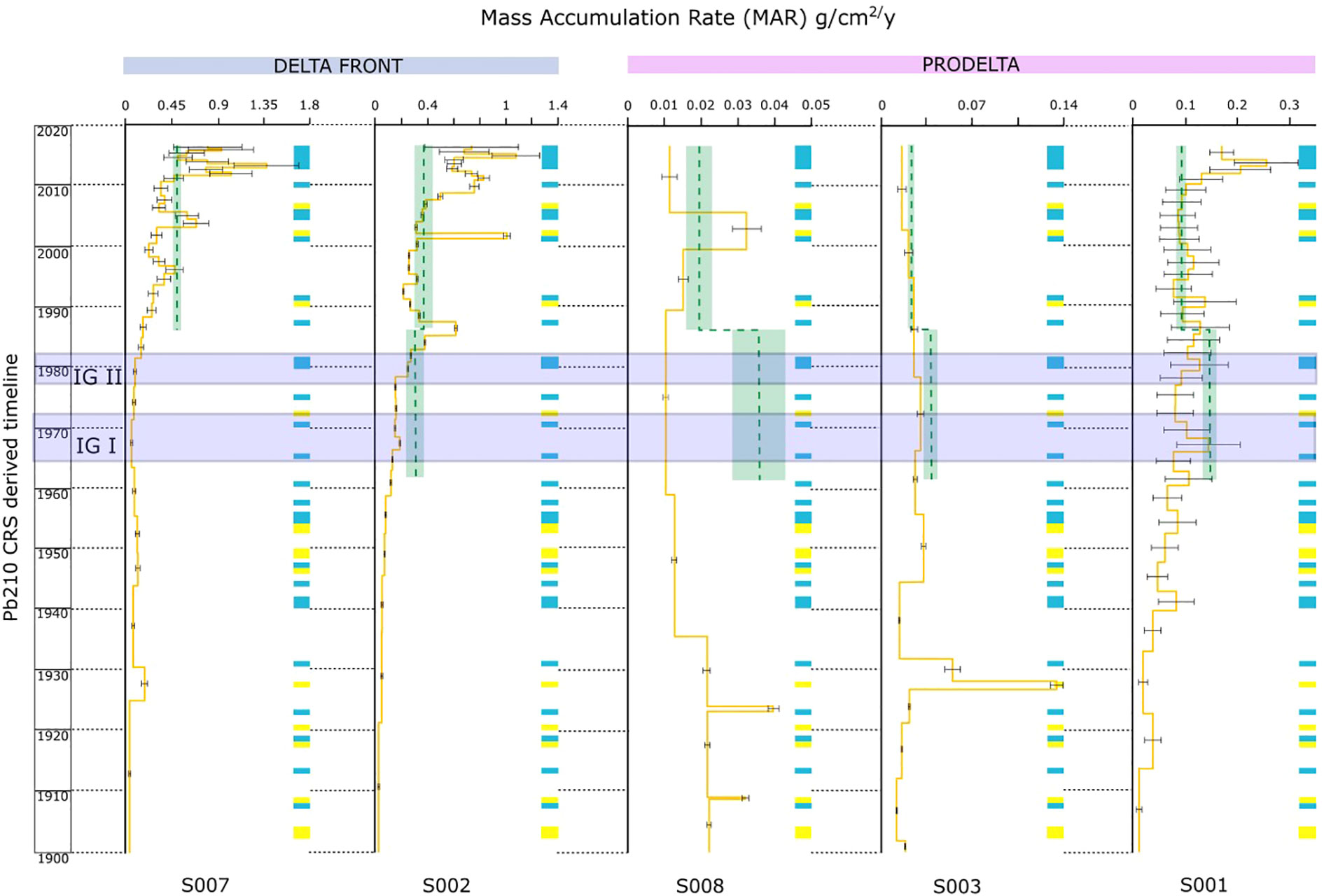
Figure 7 Mass accumulation rates in g/cm2/y for the Danube delta front and the prodelta, based on the time line obtained from 210Pb dating (orange line), using the CRS (Constant Rate of Supply) model and 137Cs (green line). The input of dams on the time line (Iron Gate I – 1964-1972 and Iron Gate II – 1978-1982) is marked by the purple square. The blue and yellow squares mark major floods (1907, 1914, 1919, 1924, 1932, 1940-1941, 1944, 1947, 1954-1956, 1958, 1962, 1965, 1970, 1975, 1980-1981, 1988, 1991, 2002, 2005-2006, 2010, 2013-2014) and hydrological droughts (1902, 1904, 1908, 1918, 1921, 1929, 1946, 1949-1950, 1953-1954, 1972, 1990, 2003, 2007) in the Lower Danube.
The two lakes analysed (DNMA15 and DNRO15) have a relatively similar low sedimentation during the last century with little change post dam construction. For Lake Matita (DNMA15), the 210Pb MAR data suggests an increase from the beginning of the century until 1960’s, then a decrease until early 1990’s followed by an increase (Figure 6). The pre-dams average is 0.05 ± 0.0003 g/cm2/y and post dam construction is 0.07 ± 0.008 g/cm2/y, marking an increase of 32%. The overall 137Cs MAR are slightly higher, but they show a slight decrease of 3% post-dam (Table 2). In Lake Rosu (DNRO15) the 210Pb indicates MAR ranging from 0.08 ± 0.004 g/cm2/y to 0.12 ± 0.005 g/cm2/y, with a pre-dams average of 0.09 ± 0.005 g/cm2/y and a post-dams average of 0.1 ± 0.01 g/cm2/y, marking an increase of 16%. The 137CS MAR are similar, 0.106 ± 0.015 g/cm2/y, and 0.096 ± 0.011 g/cm2/y, and they mark a decrease of 9% post dams (Table 2). The 137Cs MAR are remarkably consistent between the two lakes systems, although the magnitude of the 137Cs peak is replicated (Figure 4) indicating a secondary event of contaminated sediment movement perhaps related to the flood event (Figure 6). The grain size in the two lakes is predominantly represented by silt, for the last century. There is a change in grain size in both lakes, post-dams, from almost 40-50% sand fraction to less than 30%, and an increase in silt content of 10-15% (Figure 6). Lake Rosu records more of the flood pulses than Lake Matita, marked in Figure 6, but only before 1960.
The two channels, Garla Turcului and Canal Ciotica, are pathways for sediment transport and they show rapid sediment accumulation. The radiometric profiles suggest that the profiles are incomplete and possibly well mixed, and consequently the 210Pb profiles may underestimate the sedimentation rate, with the exception of a clear 137Cs peak below 50 cm depth in DNGT15 (Figure 4). A conservative interpretation of DNGT15 shows a low 210Pb MAR until the late 1960’s, with high variations afterwards. The 210Pb MAR values range from 0.08 ± 0.003 g/cm2/y to 2.53 ± 0.09 g/cm2/y, with a pre-dams average of 0.71 ± 0.025 g/cm2/y and post-dams average of 1.22 ± 0.05 g/cm2/y, marking an increase of 40%. The 137Cs MAR post-dams estimate is much higher, 1.85 ± 0.05 g/cm2/y, and shows an overall high sediment accumulation in the area – strongly confirming that the 210Pb are incomplete. In Canal Ciotica (DNCC15), the 210Pb MAR also shows a significant increase after 1990 and only in pulses, around 1996, 2004 and 2015. MAR values ranging from 0.13 ± 0.04 g/cm2/y to 3.7 ± 0.14 g/cm2/y, with a pre-dam average of 0.5 ± 0.02 g/cm2/y and a post-dam average of 1.49 ± 0.045 g/cm2/y, marking an increase of 66%. However, the 137Cs is likely also to be incomplete and is difficult to interpret. In Garla Turcului and Ciotica, the grain size varies, with sand representing up to 70% in DNGT15 and 90% in DNCC15, but decreasing over time. Sand decreases to 30-40% in DNGT15 being replaced by silt, especially after the Iron Gates I and II dams input, and not exceeding 50% in laminae. DNCC15 shows a systematic decrease of sand in time, from 90 to 40% (Figure 3).
The MAR varies spatially and depicts different processes in the lagoons, because of their formation and evolution (Figure 6). In Musura (DNMU15), MAR increases from 1900 to 2015, showing 210Pb MAR values ranging from 0.036 g/cm2/y to 1.8 ± 0.06 g/cm2/y. The pre-dams average is of 0.66 ± 0.0008 g/cm2/y and the post-dams average is 1.05 ± 0.013 g/cm2/y, marking an increase of 37%. The 137Cs MAR values of 0.49 ± 0.084 g/cm2/y pre-dams, and 1.2 ± 0.061 g/cm2/y post-dams, are very consistent with the above averages, but reflect an increase of 58% in sedimentation rate (Table 2). This core was taken in the distal part of the Musura lagoon and considering the evolution of the lagoon in the XXth century (Stǎnicǎ et al., 2007; Giosan et al., 2013), this is probably an area with lower overall sedimentation rates. The grain size of the core consists of 90% silt and with a very good sorting. There is an increase in sand content to almost 10%, around 2010 (Figure 3). This area, which is clogging rapidly, is sensitive to both floods and drought. Floods are marked by an increase in MAR over a longer period of time, while low water levels are marked by a low MAR (1949-1950, 1953-1954, 1972, 1990) (Figure 6).
The two areas in the Sahalin lagoon show different evolutions in the studied period. Core DNSN15, taken in the N of the lagoon, closer to Garla Turcului discharge, shows 210Pb MAR values ranging from 0.07 ± 0.002 g/cm2/y to 6.33 ± 0.48 g/cm2/y, with a pre-dams average of 0.32 ± 0.01 g/cm2/y and a post-dams average of 2.17 ± 0.12 g/cm2/y, indicating an increase of 85%. The 137Cs MAR values are similar, 0.48 ± 0.0.03 g/cm2/y and 1.64 ± 0.0.05 g/cm2/y, marking an increase of 70%, indicating rapid sedimentation in the area (Figure 6; Table 2). Core DNSC15, sampled towards the centre of the lagoon, shows a variable but overall lower MAR over the studied period. Values remain low until 1975, with an anomaly around 1936, of 5.57 ± 0.25 g/cm2/y, and otherwise varying from 0.02 ± 0.002 g/cm2/y to 0.56 ± 0.003 g/cm2/y. The 210Pb MAR increases after 1975 to a maximum of 4.16 ± 0.14 g/cm2/y in 1986. The 210Pb MAR decreases afterwards, to values below 1 g/cm2/y. The pre-dams average is of 1.52 ± 0.08 g/cm2/y and the post-dams average is of 1.30 ± 0.06 g/cm2/y, with a decrease of 15% (Table 2). The 137Cs MAR values are lower, reflecting the near surface 137Cs profile (Figure 6), 0.33 ± 0.03 g/cm2/y and 0.16 ± 0.008 g/cm2/y, marking a decrease of 50%. The variation of grain size in time in the north of the Sahalin lagoon shows an increase in sand content and a decrease in silt and clay contents over time (Figure 3). From 2009-2010 there is a marked increase in sand content from 50 to 80-90%. The centre of the Sahalin lagoon, as shown in DNSC15 is dominated by sand, 50-90% of the sediment. There is a slight decrease in sand content after the building of dams. This lagoon may seem less sensitive to floods and droughts but the northern area records some of the floods after 1990.
At the delta front, the two depocenters, Sulina and Sfantu Gheorghe have different radiometric profiles, with the influence of sand on S002 (Figure 3) having an impact on the 137Cs profile (Figure 5). These profiles suggest that the radiometric profile is not complete and therefore may underestimate the 210Pb derived MAR estimates for S002. Taking the values provided, collectively, the radiometric profiles reveal a similar evolution of the MAR in the studied period, which increases gradually since the beginning of the century and peaks after 2010 (Figure 5). Core S007, which records the sediment discharge of Sulina and to a certain extent of Chilia, shows 210Pb MAR values range from 0.041 ± 0.007 g/cm2/y to 1.39 ± 0.31 g/cm2/y. The pre-dams average of 0.1 ± 0.001 g/cm2/y and post-dams average of 0.53 ± 0.12 g/cm2/y suggest an increase of MAR of 83%. The 137Cs derived MAR shows similar values to the post-dam average, of 0.47 ± 0.04 (Table 2). Core S002, which records mainly the sediment discharge of Sfantu Gheorghe branch, shows 210Pb MAR range from 0.02 ± 0.0002 g/cm2/y to 1.07 ± 0.18 g/cm2/y, with a pre-dams average of 0.12 ± 0.001 g/cm2/y and post-dams average of 0.52 ± 0.04 g/cm2/y, showing an increase of 76%. The 137Cs MAR values are 0.31 ± 0.06 g/cm2/y before 1986 and 0.37 ± 0.07 g/cm2/y afterwards, showing an increase of 15% (Table 2). However, the incomplete nature of these core S002 may well result in an underestimate of the 210Pb MAR. In the Sulina depositional area, the grain size is mainly represented by silt, ~60%, and sand only represents >20%. There is a marked decrease of grain size after year 1965 (CRS date), with sand decreasing from <60% to ~20%, being replaced mainly by silt. The sand content increases again after 2000, up to 30-50% but it is mainly present in fine (1-2 mm) laminae (Figure 3). In the Sfantu Gheorghe depositional area the sand content increases after 1980’s but is only presented on certain intervals and fine (1-2 mm) laminae. It increases up to 50% between 2000 and 2006, marking a 4 cm interval (13-17 cm depth in the core) (Figure 3). The delta front area also seems to records some of the floods, especially in the N (core S007).
In the prodelta, the MAR profiles vary from the northern area to the southern area, over the studied period. Complete radiometric profiles have across very different depth profiles. In the northern area (S008), MAR is very low, <0.05 g/cm2/y. The shallow 10 cm profile of S008 suggests that it decreases from the beginning of the XXth century until 1940 and it increases again from 2000 to 2005, with a decrease thereafter. S008 records the contribution of Sulina and Chilia branches. The 210Pb MAR values range from 0.01 ± 0.0007 g/cm2/y to 0.04 ± 0.001 g/cm2/y, with a pre-dams average of 0.025 ± 0.001 g/cm2/y and a post-dams average of 0.017 ± 0.002 g/cm2/y, marking a decrease in MAR of 30%. The 137Cs MAR values although difficult to interpret on short core are comparable, 0.036 ± 0.007 g/cm2/y and 0.02 ± 0.004 g/cm2/y, but perhaps marking a reduction by as much as 48% (Table 2). In front of Sfantu Gheorghe (S003), the radiometric profile is slightly longer (15 cm) reflecting the low MAR, although slightly higher than S008. The years 1923-1932 are marked by a peak of MAR and a general decrease afterwards. Core S003 records mainly the Sfantu Gheorghe sediment discharge but, to some extent, also the contribution of the northern plume of Sulina and Chilia. The 210Pb MAR values range from 0.005 ± 0.0002 g/cm2/y to 0.14 ± 0.005 g/cm2/y, with a pre-dams average of 0.03 ± 0.001 g/cm2/y and a post-dams average of 0.017 ± 0.003 g/cm2/y, showing a decrease of 50%. The 137Cs MAR value are in good agreement, 0.065 ± 0.001 g/cm2/y pre-dam and 0.02 ± 0.001 g/cm2/y, marking a reduction of 53% (Table 2). In the southern area (S001) the MAR suggest a general increase in MAR from the beginning of the century until 1970’s, and almost constant afterwards, with one peak in 2014 (Figure 7). Core S001 records the overall contribution of all branches, being located in the distal part of the prodelta, south from the Danube mouths. The CRS derived MAR values range from 0.014 ± 0.005 g/cm2/y to 0.25 ± 0.06 g/cm2/y, with a pre-dams average of 0.076 ± 0.031 g/cm2/y and a post-dams average of 0.124 ± 0.044 g/cm2/y, marking an increase of 39%. The MAR values calculated using 137Cs dating are comparable, but lower, with a pre-dam value of 0.14 ± 0.03 g/cm2/y and a post-dams value of 0.09 ± 0.02 g/cm2/y, marking a decrease of 33% (Table 2). Grain size is very similar in cores S008 and S003, with 20% clay and 80% silt, very well sorted. Silt content increases in time. In the southern prodelta (S001), grain size is dominated by silt ~80%. There is a slight increase of sand content, on certain intervals, just after 1990 and after 2010, up to 10% (Figure 3). Flood peaks mark an increase in sediment flux in certain areas of the prodelta (S008 and S001), but they are more marked in all cores before the construction of dams.
5 Discussion
5.1 Sediment flux variation in the Danube Delta complex from 1900 to 2016
5.1.1 Sedimentary evolution of the delta complex
5.1.1.1 Lakes
The 210Pb MAR post-damming values obtained in the present study in Lake Matita, of 0.07 ± 0.008 g/cm2/y and 137Cs MAR of 0.086 ± 0.01 g/cm2/y are a factor of two lower than the one found by Duliu et al. (1996), using 137Cs dating, of 0.15 ± 0.05 g/cm2/y. Both values are lower than the ones found by Simon et al. (2017) of 0.59 ± 0.06 g/cm2/y and 0.52 ± 0.06 g/cm2/y and Giosan et al. (2013), of 0.53 g/cm2/y and 0.31 g/cm2/y, respectively. The difference may result from the natural heterogeneity of sediment accumulation and the chosen coring sites, reflecting for examples the position of the cores within the lake area (closer or farther to the centre, along the lake shores, closer to the connecting channels, positions of the coring sites in relation to the usual routes of boats and speedboats, which locally redistribute sediments in shallow water). Overall, the degree of connectivity to the main hydrological network of the delta determines the evolution of sedimentation patterns in most lakes of the delta (Begy et al., 2018; Catianis et al., 2020). From the more natural state (before 1960) to the period when the delta hydrological regime was altered by the cutting of numerous canals, which also coincides with the input of the major dams (1960-1990), in the two sampled lakes, sedimentation is increasing relatively (Figure 6), of 30% in Matita and 10% in Lake Rosu. When discussing the variation of sedimentary rates around the delta, organic matter content of sediments, especially in lakes and lagoons, must be considered (Giosan et al., 2013; Begy et al., 2018), however, we did not perform this analysis. Previous results show very high organic matter and carbonate content in lake sediments, of in-situ origin, reaching 93% in Lake Matita and 96% in Lake Rosu, and slightly decreasing in depth, but not below 50% (Catianis et al., 2013; Radan et al., 2014; Catianis et al., 2021). Assuming the average sedimentation rate of this study in similar coring sites and the MAR, this leads to an actual decrease in siliciclastic flux of 70% in Lake Rosu and to a very low increase of 5% in Lake Matita. The decrease of sand in bottom sediments of Lake Matita, may thus, result from the overall decrease in siliciclastic load in the delta complex, after the input of dams. Apart from the decrease of sediment flux, another important factor was the transformation, from 1960 to 1990 of the area east to Lake Rosu. This lake had been connected to the sea through Garla Imputita and Canalul cu Sonda (2 natural secondary arms of the delta), which were blocked when the embankment along which the new Sulina – Sf. Gheorghe Bypass canal was cut (with the embankment having a road on top, to connect the village and branch Sfantu Gheorghe with the Sulina branch, town and port). This blocked the water circulation from Lake Rosu to the sea and, most probably, is the main cause of the abrupt decrease of siliciclastic load. This is reflected also by the decrease in sand content of lake bottom sediments by 10-15%.
5.1.1.2 Channels
Although absolute values for sedimentation rates may be hindered by the incomplete 210Pb profiles, the DNGT 15 and DNCC15 indicate rapid sedimentation in the course of just 40 years (1970-2010). DNCC15 does not show any 137Cs activity peaks and may be well mixed. Canal Ciotica was dug in the early 1950’s and the 210Pb profile, although incomplete, suggests a confirmation of rapid sedimentation. When considering the erosional surface of the bottom sand layer and the change in grain size, we interpret this horizon as the bottom of the dug canal, to be early 1950’s. The two channels in the Sfantu Gheorghe secondary delta and the northern area of the Sahalin lagoon (site DNSN15) shows an increase of sedimentation accumulation rates, after the construction of the Iron Gates dams, but with different evolutions (Figure 6). Garla Turcului is the main channel for water and sediment flux to the north of the Sahalin lagoon, and it shows sharp variations in MAR, with the lowest values during periods of floods and peaks in between. This is probably due to water dynamics, increasing water discharge results in erosion of the riverbed, while a lower discharge allows for sediment accumulation. Sand content of bottom sediments decreases by 30% in Garla Turcului, as a result of the overall decrease in the delta complex. In Canal Ciotica, the evolution of sedimentation, with a high increase after 1990, marks most probably a natural silting process in this particular area, rather than a general trend. This canal is gradually clogging and narrowing because of extensive reed growth (Phragmites autralis, the most common species of macrophyte in the Danube Delta – Rudescu et al., 1964), which is the case for other similar channels and canals inside the Danube Delta, which are not dredged periodically. The decrease of sand content of its sediments by 50% is also an indication of the rapid clogging and silting process.
5.1.1.3 Lagoons
In the two analysed lagoons, Musura and Sahalin, the radiometric profiles are reasonably complete with the possible exception of the longest 120 cm core at DNMU15. Overall, the results suggest an increase in sedimentation (Figure 6). This reflects the sedimentation patterns of the two active depocentres, the secondary deltas of Chilia in the north and Sfantu Gheorghe in the south.
Musura lagoon (DNMU15), part of the Chilia modern delta, indicates an increase of 50% in sedimentation post dam construction. This area has evolved rapidly in the past 150 years, from a bay to a lagoon, with the building and lengthening of the Sulina jetties, with a maximum of sedimentation rates in the northern area (Stǎnicǎ et al., 2007). The results of this study show a gradual increase in MAR in the southern area, where the core was taken, reflecting the rapid sedimentation and clogging tendency of this lagoon. However, considering the sampling site, this is probably one of the lowest MAR values in the lagoon. The sorting and grain size of the core of homogenous silt also supports this result. Teodoru et al. (2006) finds a sedimentation rate in the Musura Bay of 1.7 cm/y, using the 137Cs peak of 1986, which is lower but comparable with the 2.47 cm/y value of this study (Table 2).
In the Sahalin lagoon, the northern area records an increase in sedimentation of 70-80% from 2000. While this area was prograding rapidly, due to sediment input trough the Turcului Channel (Garla Turcului) and subsequent aggradation processes, with rates of 5-8 m/y (Vespremeanu-Stroe, 2007), this large increase in sedimentation, coupled with the change in grain size from silt to sand reflects the evolution of the sand bar closing the lagoon, which is moving towards the shore and rotating clockwise (Figure 2E, GeoEcoMar – unpublished data). DNSC15 shows very low sedimentation until 1975, with the exception of 1930-1936 period (Figure 3). This is most probably the result of the depositional conditions, because the sediment of the Sahalin lagoon is reworked constantly, trough over-wash (Giosan et al., 1999; Dan et al., 2009; Stǎnicǎ and Panin, 2009) and resuspension during storms or flood events (Catianis et al., 2019), so the 210Pb and 137Cs content is partially diluted or even removed. This makes the interpretation of the results more difficult, as it is the case for areas with complex hydrological and oceanographic conditions (Ruiz-Fernández et al., 2020). This area records some flood pulses, especially between 2005 to 2015. The centre records a decrease in sediment flux, after 1990 and records less the natural variability of water and sediment fluxes. This lagoon is as sensitive to sediment flux changes as other areas, but only part of the sediment originates directly from the discharge of Sf. Gheorghe, whilst the rest comes indirectly from the reworking of the sand bar and nearshore sandy sediments by waves and currents. However, the general decrease in siliciclastic load is also reflected here, with a decrease in sand content of the analysed sediments by 20%.The evolution of sedimentation in this area is a result of the growth and development of the sand bar closing the lagoon, the commonly occurring influence of high waves and the anti-cyclonal currents (Dan et al., 2009) and resuspension in the area (Catianis et al., 2019).
Organic matter in these two lagoons does not exceed 45% in Musura and 48% in Sahalin (GeoEcoMar, unpublished data), increasing in recent sediments in Musura and decreasing in Sahalin. However, only considering the siliciclastic content of sediments, percentages of increase and decrease of the siliciclastic flux are similar to the entire sediment flux. Musura lagoon is an area with a high sedimentation rate, and the construction of dams does not appear to have modified the amount of siliciclasts arriving in the its southern area, nor the total flux of sediment. This area still records most of the flood pulses and droughts.
5.1.1.4 Delta front
Published data on sedimentation rates for the NW Black Sea is scarce, with values published for the area close to the Danube mouths. These range from 4 cm/y, obtained by Oaie et al. (2004) using the 137Cs isotope, to 1 cm/y estimation from both 210Pb and 137Cs dating from Teodoru et al. (2007). The value obtained by Oaie et al. (2004) is five times greater than the one obtained for the delta front in this study, of 0.7-0.8 cm/y, but closer to the value of Teodoru et al. (2007).
Gulin et al. (2002), obtains an MAR in front of the Danube Delta, using 137Cs dating of 0.4 g/cm2/y, which is slightly lower than the post-dam MAR values from the delta front area, 0.37 g/cm2/y and 0.48 g/cm2/y, of this study. The value obtained by Ayçik et al. (2004), for the far shelf, of 0.20 ± 0.01 cm/y (210Pb dating) and 0.15 ± 0.03 cm/y (137Cs dating) is higher than the ones obtained for the prodelta area in this study. On the Danube Delta front, the sedimentation is increasing over the studied period century, by 70 and 80% in the post-dam period, compared to the pre-dam period, and the increase is more marked in the northern area (Figure 7). In the same time, sand content decreases by 40% in the north (S007). The overall sedimentary rates are higher in the south but they are similar between the north and the south after 2010. The Sulina depocenter records sediments coming from both Chilia and Sulina, while Sfantu Gheorghe records mostly the sediments coming from Sfantu Gheorghe. On the coast, the Chlia and Sfantu Gheorghe secondary deltas remain the active depocentres, while the littoral sedimentary cell between Sulina and Sfantu Gheorghe is eroding, due to the negative sedimentary budget (Stănică et al., 2011), most sediment, and especially sand, being trapped north of the jetties, with only the finer fractions, silt and clay being transported south, by the Danube plume. The increase in sedimentation rate in front of the Sulina jetties reflects the local anthropic changes on the Sulina channel, and the cutting and channelling of this branch that led to a gradual increase of water and sediment from the beginning of the century (Panin and Overmars, 2012), as well as to the channelling of most water and sediment through the jetties. There is no natural overbank spilling at high water, only lateral distribution in the smaller channels and canals to some lakes. The sandbar that forms naturally in front of the jetties is dredged periodically and the sand is displaced offshore (Stǎnicǎ et al., 2007). This may increase sediment accumulation rates locally, at the site of core S007.
The main factor, which can explain the overall increase in MAR at site S002, after 1990, is the cutting off all the meanders of Sfantu Gheorghe, between 1984 and 1988, which led to an accelerated flow in the main channel and scouring of its river bed (Jugaru Tiron et al., 2009). The suspended sediment concentration increased in the main channel by 73%, in the 1990s and 2000, (Tiron, 2010). This percentage is very similar to the value of 76% increase in MAR at this site and is also reflected in the sharp increase of sand content, at the same time, in the analysed sediment core. Also, for the next period, after 2000, from 2005 to present day, the Danube Delta coastal area records the lowest storminess (Zăinescu et al., 2017), which means less removal of sediment, and several major floods (2005-2006, 2010, 2013-2014), which probably adds to the increase in MAR at this site.
In both of the active depocentres, flood pulses are well recorded. The main difference between these two areas of the delta front is that, in the near shore domain, the Sfantu Gheorghe branch flows in fairly natural conditions and feeds a secondary delta, on a much wider area than the Sulina depocentre. Giosan et al. (2013) describes the delta front area of Sfantu Gheorghe as an effective temporary sediment trap. Our results confirm this statement, with most of the sediment deposited being displaced by storms and transported southward (Zăinescu et al., 2019).
5.1.1.5 Prodelta
The prodelta radiometric profiles are complete although shallow for S008 and S003 reflecting very low sedimentations rates. MARs are similar between 137Cs and 210Pb, although contradictory for S008 reflecting the lack of resolution with depth. However, an overall interpretation is a decline in sediment accumulation, of 40 to 55% in the eastern area and a relative increase in the south (Figure 7). The decrease is greater offshore Sfantu Gheorghe than offshore Sulina. This shows that most of the sediment carried by the Danube plume outside the delta front, which is acting as a temporary depocenter, is transported southwards, along the continental shelf, by the coastal current, from north to south. Most of the sediment is deposited down to a depth of 15- 30 m (Karageorgis et al., 2009; Giosan et al., 2013), while the eastern prodelta area is only reached by very fine sediment (<63µm) occasionally, when the plume extension in this area is large enough. This is also the case for several other delta complexes in the world, several studies of suspended-sediment transport indicate a stronger transport and deposition along the continental shelf, with only a minor component across-shelf e.g. Mekong, Yangtze (Liu et al., 2009; Driscoll and Karner, 1999), Amazon, Eel, Columbia (Wright and Nittrouer, 1995), Po, Ebro (Cattaneo et al., 2004).
The overall reduction in sediment flux reaching the Danube Delta complex does not produce major changes in the distribution of depocentres. The changes are mainly represented by an increase or decrease of sedimentation rate, and a general decrease in sand content (of 10 to 50%), while the general evolution of the delta stays the same. Both Sfantu Gheorghe and Chilia deltas remain the most active. The results of this research show only one value for the Chilia delta and considering the location of the sampling site, the increase in sedimentation rate in the Musura Lagoon might be underestimated. The Sfantu Gheorghe delta shows an increase in sedimentation rate on the secondary channels reaching the Sahalin lagoon and its northern area, but a decrease in the other regions.
Even if the Sulina discharge area records an increase in sedimentation rates, the overall value remains lower than the value measured for Sf. Gheorghe. This relative increase is, most probably, a combined effect of the changeling of water and sediment by the Sulina jetties and a contribution from the discharging arm of Chilia.
The Sulina jetties are not the only local disturbance to change distribution of sediment flux. The canals, such as Ciotica, which were initially cut from the beginning of the 20th century to around 1980’s, for access, fishing and reed harvesting (Oosterberg et al., 2000), are now clogging. The clogging of smaller channels decreases sedimentation rates and the aggradation process, while sediment concentration remains high in the main channels. Smaller channels become clogged, they either behave like lakes if there’s still enough water (e.g., Mahmudia meander on Sfantu Gheorghe) or they gradually fill with macrophytes – especially Phragmitres australis, the common reed, the most common emergent vegetation in the Danube Delta (Rudescu, 1965) (e.g. Canal Ciotica). Meander cutting on both Sulina and Sfantu Gheorghe has had, as main effect, an increase of water and sediment flux in the main channel, while the old meanders remained elevated and partially disconnected from the main flow, dramatically enhancing deposition in these area (Jugaru Tiron et al., 2009; Tiron, 2010). The poor circulation of water and sediment in these areas is adding to the poor general circulation of channels and canals, as these meanders are connected to the delta plain, being part of the main conveyor of water and sediment before the cutting. While this is a general observation for the Danube Delta, it is clear, from the results of this research, that even major hydrological events (e.g. the major, 100 years flood of 2006) are not clearly identifiable in the sediments of the delta lakes. The decrease in sand content measured in the lakes, channels and the Sahalin lagoon may also be an effect of the deepening of the Sulina and Sfantu Gheorghe branches, with associated scouring and erosion of river bed (Duţu et al., 2022), resulting in more of the coarser sediment being conveyed directly in the delta front area.
The radiometric profiles vary significantly between cores. The comparison between 137Cs and 210Pb provide a corroboration between estimates. Caution is required where discrepancies occur between the two estimates (Figures 4, 5). A more accurate estimation of sediment flux is not possible, based on these results alone and the MAR values may be under or overestimated, due to the methods used. Some of the cores did not sample a complete 210Pb profile, which leads to an underestimation of MAR. Both 210Pb and 137Cs content can be influenced by a series of factors in the sediment column, which is a source of errors for dating and calculating MAR. Localised sedimentary process, like early diagenesis, linked to clay and organic matter content, sediment mixing associated with bottom currents and bioturbation and anoxia can modify the values of 210Pb and 137Cs activity in the sediment column (Baskaran et al., 2014; Corcoran and Kelley, 2006).
5.1.2 Influences of catchment changes
The sedimentological analysis, together with the chronostratigraphic framework of the twelve analysed cores provide a complex picture of sediment flux changes in the Danube-Black Sea transition zone, from 1900 to 2016. All the results show a continuity in sediment flux, with several periods of variations. A few studies have been carried out in this area to evaluate the evolution of the Danube Delta complex in modern times (~100 years) and to assess variation in sediment flux. Their results, based mainly on 137Cs (Duliu et al., 1996; Giosan et al., 2013) or both 137Cs and 210Pb dating (Simon et al., 2017; Begy et al., 2018), report mass accumulation rates from cores sampled mainly in lakes. Going beyond the lakes, our approach was to sample all active areas of the Danube Delta, including channels and lagoons, as well as the delta front and prodelta area. This approach adds greater spatial interpretation and whilst individual cores need to be interpreted carefully, depending on the completeness of the radiometric profile, collectively, they add to the holistic systems understanding of the recent sediment evolution of the Danube Delta and the NW Black Sea.
Broadly, our results show that sediment flux increases apparently in Lake Matita, Musura lagoon and the sampled areas of the delta front, as well as the south of the prodelta area and decreases in the Sahalin lagoon just after 1990 and in the prodelta area, after the input of dams. This is also reflected in the two channels, but need to be treated cautiously given the incomplete nature of the cores, especially DNCC15. The sand content decreases in most sites, reflecting the overall decrease already documented in the Danube River. The effect of major floods can be traced in most of the studied sites, but especially the major floods of the 2000-2015 period. The low water levels of the Danube (Figures 6, 7) do not seem to mark any important changes in sediment flux. There is a general increase in most sites until the 1960’s, except in Lake Rosu and the prodelta area, where the sediment flux is decreasing. This period is marked in the Danube Basin by the construction of power plants in the upper reaches of the Danube and tributaries and dredging for navigation (Schwarz, 2008) which may have led to decreased sediment bedloads. These practices do not seem to lead to a decrease in overall sediment flux reaching the delta. In Romania, while dam building is as old as the XII century, only 128 reservoirs were built before 1940, with very low capacity, and the process accelerated betwen1960 and 1991 (Rãdoane and Rãdoane, 2005). At the same time, several channels were dug in the delta, to facilitate access for fishing and reed harvesting, including one reaching lake Matita, locally increasing sediment accumulation rates (Giosan et al., 2013).
The following 50 years (1950-2000) in the Danube Basin are marked by the construction of the largest dams Iron Gate I and II, Gabcikovo and Wien-Freudnau (Schwarz, 2008). Many other dams were also constructed along the Danube, totalling 78 to date and on the main tributaries (Habersack et al., 2016), with further 187 dams in Romania alone until 1991 (Rãdoane and Rãdoane, 2005). The same period is marked by intensification of agricultural practices and land use changes which led to an increase in sediment flux delivered to rivers, however, this increase is masked by the trapping of more and more sediment in reservoirs. (McCarney-Castle et al., 2011). In the Danube Delta, the first part of this period, until mid-1980s is marked by a decrease of MAR in the sampled lakes and the prodelta area, and an increase in the lagoons and also suggested by the sampled channels, compared to the previous period. The pro-delta site S001 shows an almost constant sedimentation rate in this period, until 2010. After 1990’s, sedimentation increases in Lake Matita, the sampled channels, Musura, northern Sahalin and the delta front, but it is mostly marked by pulses – short periods of increase, followed by longer periods of lower deposition rates. For the same time period, sedimentation decreases in Lake Rosu, Sahalin lagoon and in the prodelta. Site S008 shows a sudden increase in sedimentation, in the 1990’s until 2007, particularly between 2000 and 2007. This period of time is marked by severe storms (Zăinescu et al., 2017) and a few major floods (2000-2007), which may have led to the transport of more sediment offshore.
5.2 Considerations for the future
The Danube River is highly fragmented and regulated and this phenomenon will only grow in the future (Grill et al., 2015; Habersack et al., 2016). The sediment fluxes of the Danube have been decreasing since the beginning of the century, even before the input of dams (McCarney-Castle et al., 2011). The additional 341 dams which are planned or under construction in the Danube basin (Zarfl et al., 2014) could lead to an additional decrease of 60% of the Danube sediment fluxes when they are completed (Tessler et al., 2018).
Extreme floods would attenuate the overall deficit of sediment supply, caused by dams, at least to a certain extent, but this trend is not certain for the future, as modelling suggests an increase in droughts rather than flooding events in the Danube catchment (Giosan et al., 2013). Recent analysis on hydrological data shows that the flood phenomena increased in the Danube Basin in the last decades (Mikhailova et al., 2012). One would expect to see their effects in the sediment deposition all around the delta plain and in the new delta deposits of Chilia and Sfantu Gheorghe, i.e. Musura and Sahalin lagoons. However, the effect is not marked in all of the analysed areas, perhaps with the exception of the delta front area. Moreover, the siliciclastic flux is decreasing in certain lakes, and in the Musura lagoon.
Natural variability of water and sediment pulses of rivers are attenuated by dams, as shown in other systems (Syvitski and Saito, 2007; Sylaios et al., 2010). Recent results (Constantinescu et al., in preparation) show that the natural fluctuations of water and sediment fluxes that arrive in the Danube Delta are attenuated, beginning with the mid 1970’s, which coincides with the construction of Iron Gate I and II. The sedimentation rates of this study show that only areas that are directly connected to the main branches (larger channels, some areas of the terminal lagoons and mouths of the main branches) record some of these pulses, depending on the degree of connection. The accumulation of sediment on the delta front seems to depend not only on the overall sediment flux variations but also on the flow regime of the main branches, the marine conditions and storminess and the anthropic interventions in the delta itself and on the coast. Under current climate change scenarios, river runoff in Europe will change, increasing in northern areas and decreasing in Central Europe and the Mediterranean region (Arnell and Gosling, 2013). In the last 57 years, winter precipitation has decreased in Eastern Europe (Croitoru and Minea, 2015; Croitoru et al., 2016), while mean winter temperature has increased (Ionita et al., 2018) which mean a decrease in both water discharge of rivers and surface erosion in this region.
The question remains, if the present sediment flux is enough to maintain the aggradation process in the future, in the Danube Delta, above mean local sea level rise of 3-4 mm/y (Panin, 1999; Giosan et al., 2013), and especially, if there will be enough sand in the system to feed the coastal zone. (Tessler et al., 2015b) calculate a subsidence of 5.5 mm/y for delta maintenance, given constant sediment flux, sediment deposition, and sea level rise rate under pristine, nonanthropogenic conditions and a relative sea level rise rate of 10 mm/y for contemporary, potential reservoir growth, and low sediment retention scenarios. The natural subsidence rate for the Danube Delta was previously estimated to 1.8 mm/y (Panin, 1999) and, for the moment, our results show sedimentation rates above these values, especially in the sub-aerial delta – 3 mm for lakes, 11.5-11.8 mm in channels, 18.9 mm in Musura lagoon and 16.2-19.8 mm in Sahalin lagoon. To answer the question, we need to calculate the sediment flux passing through the sub-aerial delta complex and its retention capacity. Also, the in-situ organic component of sedimentation, in all areas of the delta, needs to be quantified. The results of this study and of previous work (Giosan et al., 2013) suggest that the trapping efficiency of the delta depends mostly on its hydrological connectivity and local hydrotechnical constructions. The sampling site in Canal Ciotica and results from Anthony et al. (2021) and Möller et al. (2022) suggest that the growth of reed beds reduces water energy and subsequently, sediment trapping locally, however, a large scale study has not been conducted yet in the Danube Delta. Trapping efficiencies of other deltas were calculated at 30-50% and for the delta fronts at about 25-30% (Asian river deltas; Liu et al., 2009) but this is not yet known for our study area.
6 Conclusions and future work
This study provides, for the first time, results on sediment accumulation rates in the Danube-Black Sea interaction zone, in lakes, channels, lagoons, delta front and prodelta area, adding to the holistic systems understanding of the recent sediment evolution of the area.
The sedimentary evolution over the period of 1900 to 2016 is characterised by:
* A general increase in sediment flux, in most of the sampled areas, until the construction of the Iron Gate I and II dams (pre-dams) on the Lower Danube and the massive human interventions of 1960-1990, which affected the hydrological equilibrium of the delta complex.
* A decrease of siliciclastic flux post-dams in lakes, centre of Sahalin lagoon, while overall sediment flux decreases markedly in the prodelta area.
The impact of decreasing sediment flux over the various environments of the delta is different. The overall reduction in sediment flux does not produce major changes in depocentres. The changes are mainly represented by a decrease of sedimentation rate, and a decrease of sand by up to 50%, while the general evolution stays the same. In the sub-aerial delta, this is mainly controlled by the degree of hydrological connectivity to the main branches and by local anthropic interventions in the area, while in the sub-marine delta, marine conditions, storminess and the coastal circulation add to sediment dynamics. The anthropic changes - meanders cutting and river bed scouring on Sfantu Gheorghe and cutting and channelling for maritime navigation, with an increase of water and sediment and the construction of jetties on Sulina, increase sediment supply locally, so most of the sediment is deposited in the marginal lagoons or near shore delta front area. This sedimentation pattern, coupled with the overall decrease in grain size of deposited sediments, raises the question of trapping efficiency, especially that these areas are the already vulnerable to erosion and to storms and additional sediment removal.
The extreme hydrological events, such as the major floods of the last century can only be traced in certain areas – larger channels, lagoons and delta front, which are directly connected to the main hydrologic network, represented by the three discharging branches. The hydrological droughts also decrease accumulation rates in these areas.
The results of this study show, that overall, the local effects on sediment flux affect more the subaerial delta and the delta front, while the prodelta is affected mainly by the construction of the dams and overall decrease of sediment flux. This may be a positive outcome, at least on medium term, and could be used to improve management strategies for delta maintenance.
Some questions still remain. Is this sediment deficit low enough to allow for the aggradation and progradation process to continue and maintain the delta above sea level rise in the 21st century? What will be the tipping point for the coastal area, which is the most affected by the decrease of sediment supply? Calculating more precisely the sediment flux and the trapping efficiency of the delta, considering both siliciclastic flux and in-situ organic sedimentation, as well as integrating the role of vegetation in sediment trapping efficiency, could answer these questions.
Data availability statement
The original contributions presented in the study are included in the article/Supplementary Material. Further inquiries can be directed to the corresponding author.
Author contributions
AC, AT, AS, ES, and PH designed the study. AC carried out the sediment analysis and drafted the manuscript. AT, AS, ES, and PH supervised the PhD project of AC. IC provided data and guidance for the study. NP provided guidance and advice on historical data and delta evolution. AC, AT, AS, ES, IC, NP, and PH contributed to the manuscript. All authors contributed to the article and approved the submitted version.
Funding
This study is the result of a joint PhD project, jointly funded by the University of Stirling (United Kingdom) and the National Institute of Marine Geology and GeoEcology – GeoEcoMar (Romania). We acknowledge financial support from several projects: ReCoReD (Reconstructing the Changing Impact of the Danube on the Black Sea and Coastal Region), funded by TNA FP7 EuroFleets 2, FP7 RISES-AM (GA no 603396) and H2020 DANUBIUS-PP (GA no 739562), H2020 DOORS (GA no 101000518), funded by the European Commission; PN 09 41 03 02, PN 09 41 03 04, PN 16 45 01 04, PN 18 16 01 02, PN 19 20 02 01 – Romanian Core Program for Research, funded by the Romanian Government.
Acknowledgments
We are very grateful to captains and crews of RV Mare Nigrum and RV Istros, GeoEcoMar, Romania, for their assistance during cruises. We thank the technical staff of Biological and Environmental Sciences, School of Natural Sciences, University of Stirling, for help with sedimentary analysis and radiometric dating of cores. We also thank colleagues from GeoEcoMar: Dumitru Grosu, Sorin Balan, Mihaela Muresan and Adrian Teaca for helping with sedimentary core retrieval and sampling and Laura Dutu, Pop Ioan-Cornel and Gabriel Iordache for help with additional data and useful discussions.
Conflict of interest
The authors declare that the research was conducted in the absence of any commercial or financial relationships that could be construed as a potential conflict of interest.
Publisher’s note
All claims expressed in this article are solely those of the authors and do not necessarily represent those of their affiliated organizations, or those of the publisher, the editors and the reviewers. Any product that may be evaluated in this article, or claim that may be made by its manufacturer, is not guaranteed or endorsed by the publisher.
Supplementary material
The Supplementary Material for this article can be found online at: https://www.frontiersin.org/articles/10.3389/fmars.2023.1068065/full#supplementary-material
References
Anthony E. J., Besset M., Zainescu F., Sabatier F. (2021). Multi-decadal deltaic land-surface changes: Gauging the vulnerability of a selection of mediterranean and black sea river deltas. J. Mar. Sci. Eng. 9. doi: 10.3390/jmse9050512
Antipa G. (1941). “Black sea. volume 1: Oceanography, bionomy and general biology of the black Sea,” in National printing house of Romania 1941 (Iasi, Romania: TipoMoldova Printing House).
Appleby P. G. (2008). Three decades of dating recent sediments by fallout radionuclides: A review. Holocene 18, 83–93. doi: 10.1177/0959683607085598
Appleby P. G., Oldfield F. (1978). The calculation of lead-210 dates assuming a constant rate of supply of unsupported210Pb to the sediment. Catena 5, 1–8. doi: 10.1016/S0341-8162(78)80002-2
Arnell N. W., Gosling S. N. (2013). The impacts of climate change on river flow regimes at the global scale. J. Hydrology 486, 351–364. doi: 10.1016/j.jhydrol.2013.02.010
Ayçik G., Çetaku D., Erten H., Salihoglu I. (2004). Dating of black Sea sediments from Romanian coast using natural 210Pb and fallout 137Cs. J. Radioanalytical Nucl. Chem. 259, 177–180. doi: 10.1023/B:JRNC.0000015825.67418.18
Bajo M., Ferrarin C., Dinu I., Umgiesser G., Stanica A. (2014). The water circulation near the Danube delta and the Romanian coast modelled with finite elements. Continental Shelf Res. 78, 62–74. doi: 10.1016/j.csr.2014.02.006
Baskaran M., Nix J., Kuyper C., Karunakara N. (2014). Problems with the dating of sediment core using excess 210Pb in a freshwater system impacted by large scale watershed changes. Journal of Environmental Radioactivity 138, 355–363. doi: 10.1016/j.jenvrad.2014.07.006
Begy R. C., Simon H., Kelemen S., Preoteasa L. (2018). Investigation of sedimentation rates and sediment dynamics in Danube delta lake system (Romania) by 210Pb dating method. J. Environ. Radioactivity 192, 95–104. doi: 10.1016/j.jenvrad.2018.06.010
Bondar C. (1998). Hydromorphological relation river mouths the Danube and the coastal zone in front of the Danube delta. Geo-Eco-Marina 3, 99–102.
Bondar C., Panin N. (2001). The Danube delta hydrologic database and modelling. Geo-Eco-Marina 5–6, 5–52.
Bondar C., Roventa V., State I. (1973). “La mer noire dans la zone du littoral roumain,” in Monographie hydrologique (Bucharest, Romania: Editura Tehnica), 516.
Bondar C. (2003). The high floods along the Danube river. Sci. Ann. Danube Delta Institute Res. Dev. (Tulcea-Romania) IX, 1–9.
Bondar C. (2008). Hydromorphological balance of the Danube river channel on the sector between bazias (km 1072.2) and Danube delta inlet (km 80.5). Int. Expert Conf. ‘The Saf. Navigation Environ. Secur. Transboundary Context Black Sea Basin (Ukraine) 24–26.
Bondar C., Iordache G. (2012). Extinderea pe trecut (pana in anul 1840) ale nivelelor si debitelor de apa zilnice ale dunarii la posturile si sectiunile hidrometrice din sectorul romanesc de granita. Conferinta stiintifica internationala INHGA din 8-10 octombrie 2012. (Bucuresti – in Romanian), 43–44.
Briceag A., Ion G. (2014). Holocene Ostracod and foraminiferal assemblages of the Romanian black Sea shelf. Quaternary Int. 345, 119–129. doi: 10.1016/j.quaint.2013.09.028
Catianis I., Constantinescu A. M., Duțu, L. T. (2021). “Assessing the lithological composition of core sediment samples to investigate the influence of natural and anthropogenic stressors. 5th International Conference on “Water resources and wetlands, Tulcea, Romania, Conference Proceedings Book, 44–52 Transversal – Târgoviște.
Catianis I., Constantinescu A. M., Ispas B.-A., Pojar I., Grosu D., Dobre O. (2020). Assessing and mapping spatial distribution of the main lithological components of recent sediments in fortuna lake, Danube delta biosphere reserve, Romania title. Sci. Ann. Danube Delta Institute 25, 73–82. doi: 10.7427/DDI.25.08
Catianis I., Rǎdan S., Grosu D. (2013). Distribution of lithological components of recent sediments from some lakes in the danube delta; environmental significance. Carpathian J. Earth Environ. Sci. 8, 55–68.
Catianis I., Rădan S., Grosu D. (2014). Loss of ignition as a proxy indicator for assessing the lithological composition of the recent sediments accumulated in some freshwater lakes from the Danube delta , Romania. Int. J. Innovation Appl. Stud. 9, 260–278.
Catianis I., Vasiliu D., Constantinescu A. M., Pojar I., Grosu D. (2019). Water quality assessment in a river-Sea transition zone . recent results from distinct aquatic environments of the Danube delta biosphere reserve area , Romania. Geo-Eco-Marina 25, 99–118.
Cattaneo A., Correggiari A., Marsset T., Thomas Y., Marsset B., Trincardi F. (2004). Seafloor undulation pattern on the Adriatic shelf and comparison to deep-water sediment waves. Mar. Geology 213, 121–148. doi: 10.1016/j.margeo.2004.10.004
Cioaca E., Bondar C., Borcia C. (2009). Danube Delta biosphere reserve hydrographic network morphological dynamics. Sci. Ann. Danube Delta Institute 15, 137–148.
Cioaca E., Bondar C., Borcia C., Mierla M. (2010). Mathematical model of the Danube delta hydrographical network morphological dynamics. Sc. Ann. DDI Tulcea Romania 16, 57–64.
Constantin S., Constantinescu Ștefan, Doxaran D. (2017). Long-term analysis of turbidity patterns in Danube delta coastal area based on MODIS satellite data. J. Mar. Syst. 170, 10–21. doi: 10.1016/j.jmarsys.2017.01.016
Constantinescu A. M., Toucanne S., Dennielou B., Jorry S. J., Mulder T., Lericolais G. (2015b). Evolution of the Danube deep-Sea fan since the last glacial maximum: new insights into black Sea water-level fluctuations. Mar. Geology 367, 50–68. doi: 10.1016/j.margeo.2015.05.007
Constantinescu A. M. (2020). “Reconstructing changes in sediment input from the Danube into the black Sea,” (United Kingdom: University of Stirling). Available at: http://hdl.handle.net/1893/31886
Constantinescu S., Achim D., Rusu I., Giosan L. (2015a). “Embanking the lower danube: from natural to engineered floodplains and back,” in Geomorphic approaches to integrated floodplain, management of lowland fluvial systems in North America and Europe. Eds. Hudson P., Middelkoop H. (New York: Springer)
Corcoran M.K., Kelley J.R. (2006). Sediment-tracing technology: An overview.ERDC TN-SWWRP-06-10, (Vicksburg, MS: U.S. Army Engineer Research andDevelopment Center). Available at: https://swwrp.usace.army.mil/
Cristofor S., Vadineanu A., Sarbu A., Postolache C., Dobre R., Adamescu M. (2003). Long-term changes of submerged macrophytes in the lower Danube wetland system. Hydrobiologia 506–509, 625–634. doi: 10.1023/B:HYDR.0000008601.16757.35
Croitoru A. E., Minea I. (2015). The impact of climate changes on rivers discharge in Eastern Romania. Theor. Appl. Climatology 120, 563–573. doi: 10.1007/s00704-014-1194-z
Croitoru A. E., Piticar A., Burada D. C. (2016). Changes in precipitation extremes in Romania. Quaternary Int. 415, 325–335. doi: 10.1016/j.quaint.2015.07.028
Dan S., Stive M. J. F., Walstra D.-J. R., Panin N. (2009). Wave climate, coastal sediment budget and shoreline changes for the Danube delta. Mar. Geology 262, 39–49. doi: 10.1016/j.margeo.2009.03.003
Dan S., Stive M., Walstra D. J., Sabatier F. (2007). Sediment budget of the Danube delta coastal zone. Coast. sediments, S.U.A., New Orleans, pp. 207–220.
Dan S., Walstra D.-J. R., Stive M. J. F., Panin N. (2011). Processes controlling the development of a river mouth spit. Mar. Geology 280, 116–129. doi: 10.1016/j.margeo.2010.12.005
Day J. W., Agboola J., Chen Z., Elia C. D., Forbes D. L., Giosan L., et al. (2016). Approaches to defining deltaic sustainability in the 21st century. Estuarine Coast. Shelf Sci. 183, 275–291. doi: 10.1016/j.ecss.2016.06.018
Duliu O., Dinescu L., Dinescu M., Dorcioman R., Mihailescu N., Vanghelie I. (1996). Some considerations concerning 137Cs vertical profile in the Danube delta: Matita lake core. Sci. Total Environ. 188, 9–14. doi: 10.1016/0048-9697(96)05130-3
Duţu F., Duţu L. T., Catianis I., Ispas B.-A. (2022). Sediment dynamics and hydrodynamical processes in the Danube delta (Romania): A response to hydrotechnical works. Z. für Geomorphologie 64, 365–378. doi: 10.1127/zfg/2021/0707
Driscoll N. W., Karner G. D. (1999). Three-dimensional quantitative modelling of clinoform development. Mar. Geology 154 (1-4), 383–398.
Filip F., Giosan L. (2014). Evolution of chilia lobes of the Danube delta: Reorganization of deltaic processes under cultural pressures. Anthropocene 5, 65–70. doi: 10.1016/j.ancene.2014.07.003
Gâştescu P., Driga B., Anghel C. (1983). Caracteristici morfohidrografice ale deltei dunarii. Hidrobiologia (Academia Romana, Bucuresti – in Romanian) 17.
Giosan L., Bokuniewicz H., Panin N., Postolache I. (1997). Longshore sediment transport pattern along Romanian Danube delta coast. Geo-Eco-Marina 2, 11–24.
Giosan L., Bokuniewicz H., Panin N., Postolache I. (1999). Longshore sediment transport pattern along the Romanian Danube delta coast. J. Coast. Res. 15, 859–871.
Giosan L., Constantinescu S., Filip F., Deng B. (2013). Maintenance of large deltas through channelization: Nature vs. humans in the Danube delta. Anthropocene 1, 35–45. doi: 10.1016/j.ancene.2013.09.001
Giosan L., Coolen M. J. L., Kaplan J. O., Constantinescu S., Filip F., Filipova-Marinova M., et al. (2012). Early anthropogenic transformation of the Danube-black Sea system. Sci. Rep. 2, 1–6. doi: 10.1038/srep00582
Giosan L., Donnelly J. P., Constantinescu S., Filip F., Ovejanu I., Vespremeanu-Stroe A., et al. (2006). Young Danube delta documents stable black Sea level since the middle Holocene: Morphodynamic, paleogeographic, and archaeological implications. Geology 34, 757–760. doi: 10.1130/G22587.1
Giosan L., Syvitski J., Constantinescu S., Day J. (2014). Protect the world’s deltas. Nature 516, 31–33. doi: 10.1038/516031a
Grill G., Lehner B., Lumsdon A. E., Macdonald G. K., Zarfl C., Reidy Liermann C. (2015). An index-based framework for assessing patterns and trends in river fragmentation and flow regulation by global dams at multiple scales. Environ. Res. Lett. 10. doi: 10.1088/1748-9326/10/1/015001
Gulin S. B., Polikarpov G. G., Egorov V. N., Martin J. M., Korotkov A., Stokozov N. A. (2002). Radioactive contamination of the north-western black Sea sediments. Estuarine Coast. Shelf Sci. 54, 541–549. doi: 10.1006/ecss.2000.0663
Güttler F. N., Niculescu S., Gohin F. (2013). Turbidity retrieval and monitoring of Danube delta waters using multi-sensor optical remote sensing data: An integrated view from the delta plain lakes to the western–northwestern black Sea coastal zone. Remote Sens. Environ. 132, 86–101. doi: 10.1016/j.rse.2013.01.009
Habersack H., Hein T., Stanica A., Liska I., Mair R., Jäger E., et al. (2016). Challenges of river basin management: Current status of, and prospects for, the river Danube from a river engineering perspective. Sci. Total Environ. 543, 828–845. doi: 10.1016/j.scitotenv.2015.10.123
Hein T., Schwarz U., Habersack H., Nichersu I., Preiner S., Willby N., et al. (2016). Current status and restoration options for floodplains along the Danube river. Sci. Total Environ. 543, 778–790. doi: 10.1016/j.scitotenv.2015.09.073
Ionita M., Badaluta C. A., Scholz P., Chelcea S. (2018). Vanishing river ice cover in the lower part of the Danube basin-signs of a changing climate. Sci. Rep. 8, 1–12. doi: 10.1038/s41598-018-26357-w
Jugaru Tiron L., Le Coz J., Provansal M., Panin N., Raccasi G., Dramais G., et al. (2009). Flow and sediment processes in a cutoff meander of the Danube delta during episodic flooding. Geomorphology 106, 186–197. doi: 10.1016/j.geomorph.2008.10.016
Karabulut A., Egoh B. N., Lanzanova D., Grizzetti B., Bidoglio G., Pagliero L., et al. (2016). Mapping water provisioning services to support the ecosystem-water-food-energy nexus in the Danube river basin. Ecosystem Serv. 17, 278–292. doi: 10.1016/j.ecoser.2015.08.002
Karageorgis a. P., Kourafalou V. H., Anagnostou C., Tsiaras K. P., Raitsos D. E., Papadopoulos V., et al. (2009). River-induced particle distribution in the northwestern black Sea (September 2002 and 2004). J. Geophysical Res. 114. doi: 10.1029/2009JC005460
Latrubesse E. M., Arima E. Y., Dunne T., Park E., Baker V. R., D’Horta F. M., et al. (2017). Damming the rivers of the Amazon basin. Nature 546, 363–369. doi: 10.1038/nature22333
Lericolais G., Bulois C., Gillet H., Guichard F. (2009). High frequency sea level fluctuations recorded in the black Sea since the LGM. Global Planetary Change 66, 65–75. doi: 10.1016/j.gloplacha.2008.03.010
Liu J. P., Xue Z., Ross K., Wang H. J., Yang Z. S., Li A. C., et al. (2009). Fate of sediments delivered to the sea by Asian large rivers: Long-distance transport and formation of remote alongshore clinothems. Sedimentary Rec. 7, 4–9. doi: 10.2110/sedred.2009.4.4
Ludwig W., Dumont E., Meybeck M., Heussner S. (2009). River discharges of water and nutrients to the Mediterranean and black Sea: Major drivers for ecosystem changes during past and future decades? Prog. Oceanography 80, 199–217. doi: 10.1016/j.pocean.2009.02.001
McCarney-Castle K., Voulgaris G., Kettner A. J., Giosan L. (2011). Simulating fluvial fluxes in the Danube watershed: The “Little ice age” versus modern day. Holocene 22, 91–105. doi: 10.1177/0959683611409778
Mihailescu N. (1982). Lower Danube recent alluvia: sediment and sedimentary factors. 12th Congress Carpatho-Balkan Geological Assoc. (Bucharest, Romania) 8–13.
Mikhailov V. N., Morozov V. N., Cheroy N. I., Mikhailova M. V., Zav’yalova Y. F. (2008). Extreme flood on the Danube river in 2006. Russian Meteorology Hydrology 33, 48–54. doi: 10.3103/S1068373908010081
Mikhailova M. V., Mikhailov V. N., Morozov V. N. (2012). Extreme hydrological events in the Danube river basin over the last decades. Water Resour. 39, 161–179. doi: 10.1134/S0097807812010095
Möller I., Ionescu M. S., Constantinescu A. M., Evans B. R., Scrieciu A., Stanica A., et al. (2022). Bio-physical controls on wave transformation in coastal reed beds: Insights from the razelm-sinoe lagoon system, Romania. Front. Mar. Sci. 9. doi: 10.3389/fmars.2022.813474
Oaie G., Secrieru D., Shimkus K. (2004). Black Sea Basin : Sediment types and distribution , sedimentation processes. Geo-Eco-Marina, 9–10.
Oaie G., Secrieru D., Szobotka Ş., Fulga C., Stănică A. (2005). Danube River : sedimentological , mineralogical and geochemical characteristics of the bottom sediments. Geo-Eco-Marina 11, 77–85.
Oaie G., Secrieru D., Szobotka S., Stanica A., Soare R. (1999). Pollution state of sediments dredged from the sulina distributary and their influence on the Danube delta front area. Geo-Eco-Marina 4, 37–41. doi: 10.1073/pnas.0703993104
Oosterberg W., Buijse A. D., Coops H., Ibelings B. W., Menting G. A. M., Staras M., et al. (2000). Ecological gradients in the Danube delta lakes. RIZA rapport 2000
Overeem I., Syvitski J. P. M. (2009). Dynamics and vulnerability of delta systems. LOICZ Rep. Stud. (Geesthacht, The Netherlands) 35.
Opreanu G., Oaie G., Paun F. (2007). The dynamic significance of the grain size of sediments transported and deposited by the Danube. Geo-Eco-Marina 13, 111–119.
Panin N. (1996). Danube Delta. genesis, evolution, geological setting and sedimentology. Geo-Eco-Marina 1, 7–23.
Panin N. (1999). Global changes, sea level rising and the Danube delta: risk and responses. Geo-Eco-Marina 4, 19–29.
Panin N., Jipa D. (2002). Danube River sediment input and its interaction with the north-western black Sea. Estuarine Coast. Shelf Sci. 54, 551–562. doi: 10.1006/ecss.2000.0664
Panin N., Overmars W. (2012). The Danube delta evolution during the Holocene : reconstruction attempt using geomorphological and geological data, and some of the existing cartographic documents. Geo-Eco-Marina 18, 75–104.
Pekárová P., Halmová D., Mitková V. B., Miklánek P., Pekár J., Škoda P. (2013). Historic flood marks and flood frequency analysis of the Danube river at Bratislava, Slovakia. J. Hydrology Hydromechanics 61, 326–333. doi: 10.2478/johh-2013-0041
Pekarova P., Pekar J. (2006). Long-term discharge prediction for the turnu severin station (the Danube) using a linear autoregressive model. Hydrological Processes 20, 1217–1228. doi: 10.1002/hyp.5939
Pont D., Simonnet J. P., Walter A. V. (2002). Medium-term changes in suspended sediment delivery to the ocean: Consequences of catchment heterogeneity and river management (Rhône river, France). Estuarine Coast. Shelf Sci. 54, 1–18. doi: 10.1006/ecss.2001.0829
Popa A. (1997). Environmental changes in the Danube delta caused by the hydrotechnical works on the sf. gheorghe branch. Geoecomarina 2.
Popescu I., Lericolais G., Panin N., Normand A., Dinu C., Le Drezen E. (2004). The Danube submarine canyon (Black sea): Morphology and sedimentary processes. Mar. Geology 206, 249–265. doi: 10.1016/j.margeo.2004.03.003
Radan S. C., Radan S., Catianis I., Scrieciu A. (2014). Relationship between the magnetic susceptibility and lithological composition in sediment cores from lakes of matita -merhei depression (Danube delta, romania): Towards a proxy method of sedimentological and environmental fingerprinting. Geo-Eco-Marina 20, 45–86. doi: 10.5281/zenodo.45061
Rãdoane M., Rãdoane N. (2005). Dams, sediment sources and reservoir silting in Romania. Geomorphology 71, 112–125. doi: 10.1016/j.geomorph.2004.04.010
Rudescu L., Niculescu C., Chivu L. P. (1965). Monografia stufului din delta dunarii. Editura Academiei Republicii Socialiste Romania (Romanian), 542.
Ruiz-Fernández A. C., Alonso-Hernández C., Espinosa L. F., Delanoy R., Solares Cortez N., Lucienna E., et al. (2020). 210Pb-derived sediment accumulation rates across the wider Caribbean region. J. Environ. Radioactivity, 223–224, 106366. doi: 10.1016/j.jenvrad.2020.106366
Schwarz U. (2008). “Assessment of the balance and management of sediments of the Danube,” in XXIVth Conference of the Danubian Countries on the Hydrological Forecasting and Hydrological Bases of Water Management, Bled, Slovenia, 2-4 June, 2008. Available at: http://www.ksh.fgg.uni-lj.si/bled2008/cd_2008/index.htm.
Simon H., Kelemen S., Begy R. C. (2017). Anthropic influences on the sedimentation rates of lakes situated in different geographic areas. J. Environ. Radioactivity 173, 11–17. doi: 10.1016/j.jenvrad.2016.09.001
Sommerwerk N., Baumgartner C., Bloesch J., Hein T., Ostojić A., Paunović M., et al. (2009). The Danube river basin. Rivers Europe, 59–112. doi: 10.1016/B978-0-12-369449-2.00003-5
Stǎnicǎ A., Dan S., Ungureanu V. G. (2007). Coastal changes at the sulina mouth of the Danube river as a result of human activities. Mar. pollut. Bull. 55, 555–563. doi: 10.1016/j.marpolbul.2007.09.015
Stǎnicǎ A., Panin N. (2009). Present evolution and future predictions for the deltaic coastal zone between the sulina and sf. gheorghe Danube river mouths (Romania). Geomorphology 107, 41–46. doi: 10.1016/j.geomorph.2007.04.041
Stanev E. V., Kandilarov R. (2012). Sediment dynamics in the black Sea: Numerical modelling and remote sensing observations. Ocean Dynamics 62, 533–553. doi: 10.1007/s10236-012-0520-1
Stănică A., Dan S., Jiménez J. a., Ungureanu G. V. (2011). Dealing with erosion along the Danube delta coast. the CONSCIENCE experience towards a sustainable coastline management. Ocean Coast. Manage. 54, 898–906. doi: 10.1016/j.ocecoaman.2011.06.006
Swarzenski P. W. (2014). 210Pb dating. Encyclopedia Sci. Dating Methods, 1–11. doi: 10.1007/978-94-007-6326-5_236-1
Sylaios G. K., Kamidis N., Tsihrintzis V. A. (2010). Impact of river damming on coastal stratification-mixing processes: The cases of strymon and nestos rivers, n. greec. Desalination 250, 302–312. doi: 10.1016/j.desal.2009.09.047
Syvitski J. P. M. (2011). Global sediment fluxes to the earth’s coastal ocean. Appl. Geochemistry 26, S373–S374. doi: 10.1016/j.apgeochem.2011.03.064
Syvitski J. P. M., Kettner A. J. (2007). On the flux of water and sediment into the northern Adriatic Sea. Continental Shelf Res. 27, 296–308. doi: 10.1016/j.csr.2005.08.029
Syvitski J. P. M., Kettner A. (2011). Sediment flux and the anthropocene. Philos. Trans. R. Soc. A: Mathematical Phys. Eng. Sci. 369, 957–975. doi: 10.1098/rsta.2010.0329
Syvitski J. P. M., Kettner A. J., Overeem I., Hutton E. W. H., Hannon M. T., Brakenridge G. R., et al. (2009). Sinking deltas due to human activities. Nat. Geosci. 2, 681–686. doi: 10.1038/ngeo629
Syvitski J. P. M., Milliman J. D. (2007). Geology, geography, and humans battle for dominance over the delivery of fluvial sediment to the coastal ocean. J. Geology 115, 1–19. doi: 10.1086/509246
Syvitski J. P. M., Saito Y. (2007). Morphodynamics of deltas under the influence of humans. Global Planetary Change 57, 261–282. doi: 10.1016/j.gloplacha.2006.12.001
Teodoru C., Dimopoulos A., Wehrli B. (2006). Biogenic silica accumulation in the sediments of iron gate I reservoir on the Danube river. Aquat. Sci. 68, 469–481. doi: 10.1007/s00027-006-0822-9
Teodoru C. R., Friedl G., Friedrich J., Roehl U., Sturm M., Wehrli B. (2007). Spatial distribution and recent changes in carbon, nitrogen and phosphorus accumulation in sediments of the black Sea. Mar. Chem. 105, 52–69. doi: 10.1016/j.marchem.2006.12.013
Tessler Z. D., Vorosmarty C. J., Grossberg M., Gladkova I., Aizenman H., Syvitski J. P., et al. (2015a). Profiling risk and sustainability in coastal deltas of the world. Science. doi: 10.1126/science.aab3574
Tessler Z. D., Vörösmarty C. J., Grossberg M., Gladkova I., Aizenman H., Syvitski J. P. M., et al. (2015b). Research | reports. Science 349(6248), 638–643. doi: 10.1126/science.aab3574
Tessler Z. D., Vörösmarty C. J., Overeem I., Syvitski J. P. M. (2018). A model of water and sediment balance as determinants of relative sea level rise in contemporary and future deltas. Geomorphology 305, 209–220. doi: 10.1016/j.geomorph.2017.09.040
Tiron L. (2010). Delta du Danube - Bras de St. George. Mobilité morphologique et dynamique hydro-sédimentaire depuis 150 ans. PhD thesis. Geo-Eco-Marina. Special Publication no. 4 GeoEcoMar, 2010.
Tsiaras K. P., Kourafalou V. H., Davidov A., Staneva J. (2008). A three-dimensional coupled model of the Western black Sea plankton dynamics: Seasonal variability and comparison to Sea WiFS data. J. Geophysical Research: Oceans 113. doi: 10.1029/2006JC003959
Ungureanu V. G., Stanica A. (2000). Impact of human activities on the evolution of the Romanian black Sea beaches. Lakes Reservoirs: Res. Manage. 5, 111–115.
Vadineanu A., Adamescu M., Vadineanu R., Cristofor S., Negrei C. (2003). Past and future management of lower Danube wetlands System : A bioeconomic appraisal. J. Interdiscip. Economics 14, 415–447.
Vespremeanu-Stroe A. (2007). Tarmul Deltei Dunarii. Studiu de geomorfologie Editura Universitara, 2007. Bucuresti.
Walling D. E., Fang D. (2003). The changing sediment loads of the world’s rivers. Global Planetary Change 39, 111–126. doi: 10.2478/v10060-008-0001-x
Wang H., Yang Z., Saito Y., Liu J. P., Sun X., Wang Y. (2007). Stepwise decreases of the huanghe (Yellow river) sediment load, (1950–2005): Impacts of climate change and human activities. Global Planetary Change 57, 331–354. doi: 10.1016/j.gloplacha.2007.01.003
Wright L.D., Nittrouer C.A. (1995). Dispersal of river sediments in coastal seas: six contrasting cases. Estuaries 18(3), 494–508.
Wu Z., Zhao D., Syvitski J. P. M., Saito Y., Zhou J., Wang M. (2020). Science of the total environment anthropogenic impacts on the decreasing sediment loads of nine major rivers in China , 1954 – 2015. Sci. Total Environ. 739, 139653. doi: 10.1016/j.scitotenv.2020.139653
Xeidakis G. S., Georgoulas A., Kotsovinos N., Varagouli E. (2010). Human interventions and degradation of the coastal zone of nestos river delta , north Aegean Sea , n . Greece. Bulletin of the Geological Society of Greece, 2010 Proceedings of the 12th International Congress. 2010 Patras 2002, 797–804.
Zăinescu F. I., Tătui F., Valchev N. N., Vespremeanu-Stroe A. (2017). Storm climate on the Danube delta coast: evidence of recent storminess change and links with large-scale teleconnection patterns. Natural Hazards 87, 599–621. doi: 10.1007/s11069-017-2783-9
Zăinescu F., Vespremeanu-Stroe A., Anthony E., Tătui F., Preoteasa L., Mateescu R. (2019). Flood deposition and storm removal of sediments in front of a deltaic wave-influenced river mouth. Mar. Geology 417. doi: 10.1016/j.margeo.2019.106015
Keywords: Danube Delta, Black Sea, sediment accumulation, dams, anthropic impact, local factors
Citation: Constantinescu AM, Tyler AN, Stanica A, Spyrakos E, Hunter PD, Catianis I and Panin N (2023) A century of human interventions on sediment flux variations in the Danube-Black Sea transition zone. Front. Mar. Sci. 10:1068065. doi: 10.3389/fmars.2023.1068065
Received: 12 October 2022; Accepted: 20 March 2023;
Published: 28 April 2023.
Edited by:
Pengfei Lin, Institute of Atmospheric Physics (CAS), ChinaReviewed by:
Yang Zhou, Beijing Normal University, Zhuhai, ChinaJianjun Jia, East China Normal University, China
Copyright © 2023 Constantinescu, Tyler, Stanica, Spyrakos, Hunter, Catianis and Panin. This is an open-access article distributed under the terms of the Creative Commons Attribution License (CC BY). The use, distribution or reproduction in other forums is permitted, provided the original author(s) and the copyright owner(s) are credited and that the original publication in this journal is cited, in accordance with accepted academic practice. No use, distribution or reproduction is permitted which does not comply with these terms.
*Correspondence: Adriana Maria Constantinescu, YWRyaWFuYS5jQGdlb2Vjb21hci5ybw==