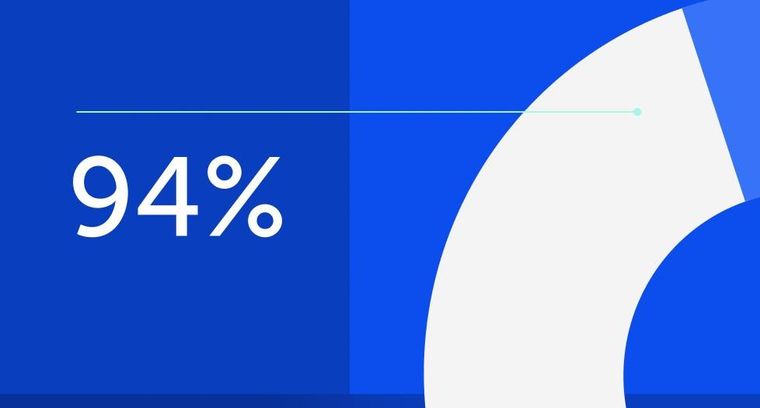
94% of researchers rate our articles as excellent or good
Learn more about the work of our research integrity team to safeguard the quality of each article we publish.
Find out more
PERSPECTIVE article
Front. Mar. Sci., 01 February 2023
Sec. Marine Molecular Biology and Ecology
Volume 10 - 2023 | https://doi.org/10.3389/fmars.2023.1017378
This article is part of the Research TopicEmerging Challenges and Solutions for Plastic PollutionView all 11 articles
Plastic is a ubiquitous material that has become an essential part of our lives. More than one hundred million tons of plastic has accumulated in the world’s oceans as a result of poor waste management. This plastic waste gradually fragments into smaller pieces known as microplastics and nanoplastics. These small plastic particles can cause significant damage to marine ecosystems, and negatively impact human health. According to a recent review of international patents, the majority of ocean-cleaning inventions are limited to microplastics larger than 20 μm. Furthermore, such technologies are ineffective for nanoplastics, which measure less than 1000 nm, or even fibrous plastics. Alternative solutions need to be considered for the large-scale in situ removal of microplastics and nanoplastics from the ocean. In this perspective, we present the concept of engineering a microbial ecosystem, which we term the microbiosphere. The concept is based on key observations that have been made for natural plastic-based ecosystems known as plastispheres. These observations relate to the solid support material, self-sustainability, attachment to plastic, degradation of plastic, and risk of pathogenicity. Inspiration can be taken from the plastisphere whereby a novel microbial ecosystem could be designed and engineered as a bioremediation tool to rid the ocean of micro- and nanoplastics. Such an engineered system could outcompete pathogens for marine plastic waste and potentially reduce the risk of infectious diseases.
Poor waste management of plastic has led to the accumulation of almost 150 million tons of plastics in the ocean, much of which emanates from landfills (Eunomia, 2016; World Economic Forum, 2016). It has been estimated that the content of one garbage truck, which can hold 12 to 14 tonnes of plastic, is released into the ocean every minute (World Economic Forum, 2016). At the present rate of plastic consumption and disposal, plastic mass will outnumber the fish biomass in the ocean by 2050 (World Economic Forum, 2016). Marine plastic waste, through exposure to heat, seawater and sunlight, becomes brittle over time and is fragmented into smaller pieces known as microplastics, which have particle sizes of 1 to 1000 μm (Hartmann et al., 2019). Microplastics can also enter the oceans directly from waste-containing paint-based materials, textiles and cosmetic products (Eunomia, 2016). These plastic pieces are ingested by marine organisms, which can negatively impact ocean ecosystems. Further fragmentation of microplastic leads to the formation of nanoplastics which range in size from 1 to 1000 nm. On account of their greater surface area-to-volume ratio, microplastics and nanoplastics can permeate cell membranes, disrupt cellular functions, and cause health issues (Ter Halle et al., 2017; Tetu et al., 2020).
After several decades of exposure to synthetic plastic, a relatively new type of ecosystem has emerged in nature known as the ‘Plastisphere.’ This terminology, coined by Zettler et al. (2013), describes a community of microbial species distinct from its surrounding environment in which the plastic debris forms the heart of the community. By studying the plastispheres at molecular, cellular and community levels, one could potentially design and engineer marine-based microbial ecosystems to clean up the ocean. In this article, we will put forward the notion of engineering a microbial ecosystem for the purpose of removing plastic waste from the ocean, which we will refer to as a microbiosphere. We will describe five key design features that would need to be incorporated into a microbiosphere to make such a concept environmentally feasible (Figures 1, 2).
Figure 2 The concept of the microbiosphere. (A) A biodegradable solid support (central brown circle) would be used to accommodate a rationally engineered community of microbial species known as the microbiosphere. This community would include phototrophic and heterotrophic species to ensure self-sustainability (green, orange and pink areas). A solid support would be critical for transporting the microbiosphere across the ocean as well as providing nutrients to the microbiosphere. (B) Micro- and nanoplastic waste that is too small to be filtered off and collected by conventional technologies could potentially cause harm to marine life, as well as humans. To remove these small plastic waste particles, microbiospheres would be released into the ocean. These biological entities would attach themselves to micro- and nanoplastic waste debris with the aid of cellular appendages and the adhesive property of the community biofilm. (C) Hydrolytic enzymes would be secreted into the extracellular environment to break down the plastic polymer into its monomers. The monomers would be taken up by microbial cells via protein transporters and converted to metabolites that support the growth and survival of the microbiosphere. (D) By outcompeting pathogenic microbial species (small circle outlined in brown) for the plastic waste, the microbiosphere could potentially reduce the risk of infectious diseases.
The core part of any plastisphere community is the plastic waste itself (Zettler et al., 2013). Thus, an obvious starting point for engineering a microbiosphere would be the use of a solid support to accommodate the microbial species that constitute the microbiosphere community. Like synthetic plastic, the supporting material would need to be resilient, durable, colonizable, and light enough to access different parts of the oceans. Unlike synthetic plastic, however, the solid support would need to be prone to degradation so that it does not persist in the natural environment for too long and lead to pernicious interactions with marine life. The most logical choice of material for supporting a microbiosphere would be an environment-friendly material with physical properties similar to conventional synthetic plastics. One ideal candidate in this regard would be polyhydroxybutyrate (Leong et al., 2014). This well-studied bioplastic can serve as energy and carbon sources for microorganisms. Furthermore, it can be synthesised and degraded through natural means (Leong et al., 2014). Lott et al. (2021) observed that, under laboratory conditions, as much as 81% of a polyhydroxybutyrate film could be degraded over a 1-year period in the presence of seawater. Other polyester-based plastics such as polylactic acid and polycaprolactone could also make excellent candidates as support materials for microbiospheres due to their biodegradable properties (Suzuki et al., 2020; Wang et al., 2020). The support material for the microbiosphere would serve two primary functions. Firstly, it would enable the microbiosphere to access different parts of the ocean. Denser solids such as sand could be mixed into the support material to create variations in buoyancy and allow the microbiosphere to operate at different ocean depths (Michels et al., 2018). Secondly, it would sustain the growth and viability of the microbiosphere by providing nutrients to the microbiosphere community.
Plastisphere communities are able to endure the harsh conditions of the ocean environment over long periods from several months to years (De Tender et al., 2017). The robustness of these communities can be attributed to the multi-species arrangement which can impart a number of beneficial traits to the community. Firstly, it enables a division of labor which reduces the metabolic burden imposed on a single member of the community (Zhang and Wang, 2016). Secondly, it increases the diversity of nutrients that can be acquired from the environment and utilized within the community. Thirdly, it reduces the stress that would inevitably arise from the dynamic conditions of the marine environment. Lastly, it prevents colonization by microbial species that may threaten the survival of the community (Pamer, 2016). Many of the members of the plastisphere community are bacterial species but also include other types of microorganisms such as archaea, fungi and microbial eukaryotes (Oberbeckmann et al., 2014; Oberbeckmann et al., 2016; Oberbeckmann et al., 2018). For a comprehensive list of species associated with plastispheres, refer to Wallbank et al., (2022). Two main groups of microorganisms, known as the photoautotrophs e.g. diatoms (Mastogloia, Navicula, Nitzschia), cyanobacteria (Phormidium, Rivularia, and Leptolyngbya) and heterotrophs, e.g. bacteria (Pseudomonas, Azotobacter, Bacillus), are typically encountered in plastisphere communities (Dey et al., 2022; Wallbank et al., 2022). The co-cultivation and coexistence of these organisms within a microbiosphere could be achieved with a symbiotic arrangement of these species (Zuñiga et al., 2020). Photoautotrophic species, in the presence of light, convert inorganic carbon CO2 into organic molecules; this would be required by heterotrophs under conditions where a source of organic carbon is not readily available. In exchange, the heterotrophs would provide the photoautotrophs with additional CO2 resulting from the heterotrophic metabolism of organic nutrients. This resulting symbiosis would confer a survival advantage to the entire community (Zuñiga et al., 2020). Another intriguing, yet poorly studied, group observed in plastisphere communities is the saprotroph (Zeghal et al., 2021). These species recycle dead organic matter within the community and typically include the Ascomycota, Basidiomycota and Chytridiomycota phyla of the fungal community (Oberbeckmann et al., 2016; Zeghal et al., 2021). An important and key point here is that mutualistic arrangement and interaction of multiple microbial species would be critical for the development of stable and robust communities that can endure the marine environment.
In a 21-month experiment using artificial seawater conditions, Kirstein et al. (2019) screened the plastisphere community for microbial species that were able to attach themselves to different types of plastics. The authors, in accordance with previous studies, observed that bacterial species from the Roseovarius, Erythrobacter, Ulvibacter and Parvularcula genera were closely associated with plastic materials (Zettler et al., 2013; Oberbeckmann et al., 2016; Viršek et al., 2017; Oberbeckmann et al., 2018). Though it could be speculated that these bacteria species may possess affinity for the chemical additives or contaminants within the plastic waste, rather than the plastic chemical itself, these initial studies nonetheless provide a preliminary indication that biological features do exist within microorganisms that promote attachment to plastic material. The underlying mechanism by which microorganisms attach specifically to plastic materials has not been established, but is likely to be facilitated in two ways. The first is with the aid of cellular appendages such as flagella, pili, fimbriae, and curli fibers (Kreve and Reis, 2021). This mechanism of attachment is known to occur within minutes and is thought to involve strong non-covalent interactions (Shteindel et al., 2019; Parreira and Martins, 2021). The second is via the extracellular matrix known as the biofilm, the formation of which can be initiated by appendage attachment (Koczan et al., 2011). The underlying core structure of the biofilm is the extracellular polymeric substance, also known as the EPS. The mixture of polymeric compounds present within the EPS, e.g. polysaccharides, proteins, lipids and DNA, generate the adhesive forces such as hydrogen bonds and London Dispersion forces which would promote surface attachment (Flemming et al., 2016). The process of attachment is known to be influenced to a great extent by the pre-conditioning of the attachment surface, as well as the type of plastic material (Eich et al., 2015). Based on these possible modes of attachment, it could be speculated that microbial species with cellular appendages or biofilms with a greater degree of hydrophobicity would be more effective at penetrating plastic surfaces. Microbial species that possess a high affinity for plastics would most certainly be an important design feature to ensure that plastic materials are specifically targeted (Gabriel et al., 2019).
Surprisingly, very few studies have identified the marine species responsible for plastic degradation within plastisphere communities. Gao and Sun, (2021) recently isolated three bacterial species, Exiguobacterium sp., Halomonas sp. and Ochrobactrum sp., capable of degrading polyethylene terephthalate and polyethylene, while Khandare et al. (2021) isolated polyvinyl chloride (PVC)-degrading bacterial species belonging to the Vibrio, Alteromonas and Cobetia genera. How have these microorganisms achieved this remarkable capacity to utilize plastic waste as a source of nutrient? There is a general consensus now that since the establishment of the plastic industry during the fifties, microorganisms have slowly evolved over the last few decades to metabolise plastic waste (Zrimec et al., 2021). This status of evolution has been made possible with the diverse range of hydrolytic enzymes that are secreted into the extracellular environment and able to degrade various plastic substrates. In a landmark study, Kohei Oda’s research team (Yoshida et al., 2016) had previously shown that the PETase and MHETase enzymes were responsible for polyethylene degradation within the soil bacterium Ideonella sakiensis. Enzyme-mediated degradation of other types of plastics such as polypropylene and polystyrene, which are the most commonly encountered plastics on the ocean surface, have also been demonstrated (Auta et al., 2017; Kaushal et al., 2021). Research is currently underway to engineer plastic-hydrolysing enzyme to improve their catalytic rates and substrate specificities for the treatment and recycling of plastics (Zhu et al., 2022).
Aside from the bulk plastic material, chemicals added to plastics to enhance their properties, e.g. antioxidants, fillers, flame retardants, UV-light stabilisers, impact modifiers, heat stabilisers, would also need to be remediated to reduce their cellular toxic effects (Hahladakis et al., 2018). This also applies to organic pollutants adsorbed to the plastic waste (Karkanorachaki et al., 2022). Several microbial species have been reported in the literature that are capable of degrading plastic additives, e.g. bisphenol, diethylhexyl-phthalate, or organic pollutants, e.g. polycyclic aromatic hydrocarbons, textile dyes (Suyamud et al., 2018; Wang et al., 2018; John et al., 2020; Wright et al., 2020). Within the context of designing and engineering a microbiosphere, both plastic- and toxin-degrading microbial species would be necessary for the complete bioremediation of marine plastic waste.
Marine plastispheres can harbor pathogenic bacterial species such as Vibrio cholera. This raises the concern that waste plastic in the ocean could serve as a vehicle for the spread of infection diseases though recent evidence suggests that Vibrio species may simply be opportunistic colonizers of the plastic rather than core, stable members of the community (Kirstein et al., 2016; Kesy et al., 2021). Another worrying concern is that plastispheres may enrich antimicrobial resistance genes via gene transfer and increase the likelihood of certain members acquiring resistance to a wide spectrum of drugs (Moore et al., 2020). To ensure that an engineered microbiosphere itself does not pose a threat to marine or human environments, non-pathogenic microbial species would need to be incorporated into the design of a microbiosphere in order to prevent the colonization of microbes that have the potential to become pathogenic. On this particular point of pathogenicity, artificially engineered communities therefore present a distinct advantage over natural communities for the degradation of plastic waste. One interesting group of microorganisms that could reduce the potential of pathogenicity is the ‘predator’ which can consume bacteria. Members of this group have been observed in plastispheres and include choanoflagellates, radiolaria, and Micromonas (Dey et al., 2022). Incorporation of predators into the microbiosphere could potentially be an effective strategy for reducing the infiltration and unwanted colonization of pathogenic species within the microbiosphere. Bdellovibrio bacteriovorus, for example, is a well-studied bacterial predator that could be used to lower the risk of colonization by pathogens such as Vibrio cholerae (Richards et al., 2012). Integration of anti-pathogenic features into the design of a microbiosphere could therefore potentially reduce the spread of infectious diseases.
Currently, there are no commercially viable technologies for the effective removal of microplastics or nanoplastics. Given the ongoing work relating to the engineering of microbial communities, one possible solution for the removal of marine waste plastic is to design and engineer microbial ecosystems capable of ocean bioremediation (Mee and Wang, 2012; Tsoi et al., 2019). As highlighted in this perspective, biological features, inspired from observations of the plastisphere community, could be used to design and engineer such systems.
It could be reasoned that plastisphere communities, which have evolved within the natural marine environment for the colonization and degradation of plastic, could be applied for the in situ removal of marine plastic waste. Clearly, they would hold an advantage with regard to immediate implementation and practical application. The main concern, however, is the risk of colonization by pathogenic microbial species in plastisphere communities. A microbiosphere, on the other hand, could be designed and engineered to significantly reduce this risk. Moreover, the efficiency and functionality of such ecosystems could be greatly improved using rational and customised approaches.
Still, a host of questions, from both application and fundamental standpoints, would need to be addressed through further experimental work in order to assess not only the technical feasibility of this concept but also its ethical implications. How rapidly could an engineered microbiosphere degrade plastic under natural conditions? Could they be engineered for plastic degradation in different ocean environments? How would these engineered ecosystems compare against plastisphere communities in terms of their plastic-degrading trait? How stable would microbiospheres be over time within the natural environment and how long would they retain their plastic-degrading property within the natural environment? Could the release of an engineered microbiosphere pose even more of a threat to the marine environment than the plastisphere itself?
To understand the enormous remedial potential of microbial ecosystems, one needs only to look at the famous explosion of the Deepwater Horizon oil rig. Nature’s response to this environmental disaster at the microbial level has been phenomenal and inspiring to the point that it has set off intensive activities in engineering more efficient microbial systems for the clean-up of oil spillages (Ganesan et al., 2022). Likewise, nature has presented its own solution to dealing with plastic in the form of plastispheres. Scientists can take inspirations from these natural microbial ecosystems in order to develop novel technologies for the large-scale removal of micro- and nanoplastics from the ocean.
The original contributions presented in the study are included in the article/supplementary materials. Further inquiries can be directed to the corresponding author.
MKA conceived the idea. MKA drafted and edited the final manuscript. KAA, LWA and MKS composed the figure. All authors contributed to the article and approved the submitted version.
This work was supported by the ‘SDG research program’ fund sourced from the United Arab Emirates University and awarded to MKA.
The authors declare that the research was conducted in the absence of any commercial or financial relationships that could be construed as a potential conflict of interest.
All claims expressed in this article are solely those of the authors and do not necessarily represent those of their affiliated organizations, or those of the publisher, the editors and the reviewers. Any product that may be evaluated in this article, or claim that may be made by its manufacturer, is not guaranteed or endorsed by the publisher.
Auta H. S., Emenike C. U., Fauziah S. H. (2017). Distribution and importance of microplastics in the marine environment: A review of the sources, fate, effects, and potential solutions. Environ. Int. 102, 165–176. doi: 10.1016/J.ENVINT.2017.02.013
De Tender C., Devriese L. I., Haegeman A., Maes S., Vangeyte J., Cattrijsse A., et al. (2017). Temporal dynamics of bacterial and fungal colonization on plastic debris in the north Sea. Environ. Sci. Technol. 51, 7350–7360. doi: 10.1021/ACS.EST.7B00697/SUPPL_FILE/ES7B00697_SI_001.PDF
Dey S., Rout A. K., Behera B. K., Ghosh K. (2022). Plastisphere community assemblage of aquatic environment: plastic-microbe interaction, role in degradation and characterization technologies. Environ. Microbiome 2022 17, 1–21. doi: 10.1186/S40793-022-00430-4
Eich A., Mildenberger T., Laforsch C., Weber M. (2015). Biofilm and diatom succession on polyethylene (PE) and biodegradable plastic bags in two marine habitats: Early signs of degradation in the pelagic and benthic zone? PloS One 10(9):e0137201. doi: 10.1371/journal.pone.0137201
Erni-Cassola G, Wright RJ, Gibson MI, Christie-Oleza JA (2019). Early colonization of weathered polyethylene by distinct bacteria in marine coastal seawater. Micro Ecol. 79 (3), 517–526. doi: 10.1007/s00248-019-01424-5
Eunomia (2016). Plastics in the marine environment: Where do they come from? where do they go? Available at: https://www.eunomia.co.uk/reports-tools/plastics-in-the-marine-environment/.
Flemming H. C., Wingender J., Szewzyk U., Steinberg P., Rice S. A., Kjelleberg S. (2016). Biofilms: An emergent form of bacterial life. Nat. Rev. Microbiol. 2016 149 14, 563–575. doi: 10.1038/nrmicro.2016.94
Ganesan M., Mani R., Sai S., Kasivelu G., Awasthi M. K., Rajagopal R., et al. (2022). Bioremediation by oil degrading marine bacteria: An overview of supplements and pathways in key processes. Chemosphere 303, 134956. doi: 10.1016/J.CHEMOSPHERE.2022.134956
Gao R., Sun C. (2021). A marine bacterial community capable of degrading poly(ethylene terephthalate) and polyethylene. J. Hazard. Mater. 416, 125928. doi: 10.1016/J.JHAZMAT.2021.125928
Hahladakis J. N., Velis C. A., Weber R., Iacovidou E., Purnell P. (2018). An overview of chemical additives present in plastics: Migration, release, fate and environmental impact during their use, disposal and recycling. J. Hazard. Mater. 344, 179–199. doi: 10.1016/J.JHAZMAT.2017.10.014
Hartmann N. B., Hüffer T., Thompson R. C., Hassellöv M., Verschoor A., Daugaard A. E., et al. (2019). Are we speaking the same language? Recommendations for a definition and categorization framework for plastic debris. Environ. Sci. Technol. 53, 1039–1047. doi: 10.1021/ACS.EST.8B05297/ASSET/IMAGES/MEDIUM/ES-2018-05297K_0006.GIF
John J., Dineshram R., Hemalatha K. R., Dhassiah M. P., Gopal D., Kumar A. (2020). Bio-decolorization of synthetic dyes by a halophilic bacterium Salinivibrio sp. Front. Microbiol. 11. doi: 10.3389/FMICB.2020.594011/BIBTEX
Karkanorachaki K., Tsiota P., Dasenakis G., Syranidou E., Kalogerakis N. (2022). Nanoplastic generation from secondary PE microplastics: Microorganism-induced fragmentation. Microplastics 2022 Vol. 1 Pages 85-101 1, 85–101. doi: 10.3390/MICROPLASTICS1010006
Kaushal J., Khatri M., Arya S. K. (2021). Recent insight into enzymatic degradation of plastics prevalent in the environment: A mini - review. Clean. Eng. Technol. 2, 100083. doi: 10.1016/J.CLET.2021.100083
Kesy K., Labrenz M., Scales B. S., Kreikemeyer B., Oberbeckmann S. (2021). Vibrio colonization is highly dynamic in early microplastic-associated biofilms as well as on field-collected microplastics. Microorganisms 9, 1–13. doi: 10.3390/MICROORGANISMS9010076
Khandare S. D., Chaudhary D. R., Jha B. (2021). Bioremediation of polyvinyl chloride (PVC) films by marine bacteria. Mar. pollut. Bull. 169, 112566. doi: 10.1016/J.MARPOLBUL.2021.112566
Kirstein I. V., Kirmizi S., Wichels A., Garin-Fernandez A., Erler R., Löder M., et al. (2016). Dangerous hitchhikers? Evidence for potentially pathogenic vibrio spp. on microplastic particles. Mar. Environ. Res. 120, 1–8. doi: 10.1016/J.MARENVRES.2016.07.004
Kirstein I. V., Wichels A., Gullans E., Krohne G., Gerdts G. (2019). The plastisphere – uncovering tightly attached plastic “specific” microorganisms. PloS One 14, e0215859. doi: 10.1371/JOURNAL.PONE.0215859
Koczan J. M., Lenneman B. R., McGrath M. J., Sundin G. W. (2011). Cell surface attachment structures contribute to biofilm formation and xylem colonization by Erwinia amylovora. Appl. Environ. Microbiol. 77, 7031. doi: 10.1128/AEM.05138-11
Kreve S., Reis A. C. D. (2021). Bacterial adhesion to biomaterials: What regulates this attachment? a review. Jpn. Dent. Sci. Rev. 57, 85. doi: 10.1016/J.JDSR.2021.05.003
Leong Y. K., Show P. L., Ooi C. W., Ling T. C., Lan J. C. W. (2014). Current trends in polyhydroxyalkanoates (PHAs). J. Biotechnol. 180, 52–65. doi: 10.1016/j.jbiotec.2014.03.020
Lott C., Eich A., Makarow D., Unger B., van Eekert M., Schuman E., et al. (2021). Half-life of biodegradable plastics in the marine environment depends on material, habitat, and climate zone. Front. Mar. Sci. 8. doi: 10.3389/FMARS.2021.662074/BIBTEX
Mee M. T., Wang H. H. (2012). Engineering ecosystems and synthetic ecologies. Mol. Biosyst. 8, 2470–2483. doi: 10.1039/C2MB25133G
Michels J., Stippkugel A., Lenz M., Wirtz K., Engel A. (2018). Rapid aggregation of biofilm-covered microplastics with marine biogenic particles. Proc. R. Soc B 285. doi: 10.1098/RSPB.2018.1203
Moore R. E., Millar B. C., Moore J. E. (2020). Antimicrobial resistance (AMR) and marine plastics: Can food packaging litter act as a dispersal mechanism for AMR in oceanic environments? Mar. pollut. Bull. 150. doi: 10.1016/J.MARPOLBUL.2019.110702
Oberbeckmann S., Kreikemeyer B., Labrenz M. (2018). Environmental factors support the formation of specific bacterial assemblages on microplastics. Front. Microbiol. 8. doi: 10.3389/FMICB.2017.02709/BIBTEX
Oberbeckmann S., Loeder M. G. J., Gerdts G., Osborn M. A. (2014). Spatial and seasonal variation in diversity and structure of microbial biofilms on marine plastics in northern European waters. FEMS Microbiol. Ecol. 90, 478–492. doi: 10.1111/1574-6941.12409
Oberbeckmann S., Osborn A. M., Duhaime M. B. (2016). Microbes on a bottle: Substrate, season and geography influence community composition of microbes colonizing marine plastic debris. PloS One 11. doi: 10.1371/JOURNAL.PONE.0159289
Pamer E. G. (2016). Resurrecting the intestinal microbiota to combat antibiotic-resistant pathogens. Science 352, 535–538. doi: 10.1126/SCIENCE.AAD9382
Parreira P., Martins M. C. L. (2021). The biophysics of bacterial infections: Adhesion events in the light of force spectroscopy. Cell Surf. 7, 100048. doi: 10.1016/J.TCSW.2021.100048
Richards G. P., Fay J. P., Dickens K. A., Parent M. A., Soroka D. S., Boyd E. F. (2012). Predatory bacteria as natural modulators of Vibrio parahaemolyticus and Vibrio vulnificus in seawater and oysters. Appl. environ. Microbiol. 78, 7455. doi: 10.1128/AEM.01594-12
Shteindel N., Yankelev D., Gerchman Y. (2019). High-throughput quantitative measurement of bacterial attachment kinetics on seconds time scale. Microb. Ecol. 77, 726–735. doi: 10.1007/S00248-018-1254-5
Suyamud B., Inthorn D., Panyapinyopol B., Thiravetyan P. (2018). Biodegradation of bisphenol a by a newly isolated Bacillus megaterium strain ISO-2 from a polycarbonate industrial wastewater. Water Air Soil pollut. 2018 22911 229, 1–12. doi: 10.1007/S11270-018-3983-Y
Suzuki M., Tachibana Y., Kasuya K.i. (2020). Biodegradability of poly(3-hydroxyalkanoate) and poly(ϵ-caprolactone) via biological carbon cycles in marine environments. Polym. J. 2020 53, 47–66. doi: 10.1038/s41428-020-00396-5
Ter Halle A., Jeanneau L., Martignac M., Jardé E., Pedrono B., Brach L., et al. (2017). Nanoplastic in the north Atlantic subtropical gyre. Environ. Sci. Technol. 51, 13689–13697. doi: 10.1021/ACS.EST.7B03667/SUPPL_FILE/ES7B03667_SI_001.PDF
Tetu S. G., Sarker I., Moore L. R. (2020). How will marine plastic pollution affect bacterial primary producers? Commun. Biol. 2020 31 3, 1–4. doi: 10.1038/s42003-020-0789-4
Tsoi R., Dai Z., You L. (2019). Emerging strategies for engineering microbial communities. Biotechnol. Adv. 37, 107372. doi: 10.1016/J.BIOTECHADV.2019.03.011
Viršek M. K., Lovšin M. N., Koren Š., Kržan A., Peterlin M. (2017). Microplastics as a vector for the transport of the bacterial fish pathogen species Aeromonas salmonicida. Mar. pollut. Bull. 125, 301–309. doi: 10.1016/J.MARPOLBUL.2017.08.024
Wallbank J. A., Lear G., Kingsbury J. M., Weaver L., Doake F., Smith D. A., et al. (2022). Into the plastisphere, where only the generalists thrive: Early insights in plastisphere microbial community succession. Front. Mar. Sci. 9. doi: 10.3389/FMARS.2022.841142/BIBTEX
Wang G. X., Huang D., Ji J. H., Völker C., Wurm F. R. (2020). Seawater-degradable polymers - fighting the marine plastic pollution. Adv. Sci. 8, 2001121. doi: 10.1002/ADVS.202001121
Wang W., Wang L., Shao Z. (2018). Polycyclic aromatic hydrocarbon (PAH) degradation pathways of the obligate marine PAH degrader Cycloclasticus sp. strain P1. Appl. Environ. Microbiol. 84, e01261–18. doi: 10.1128/AEM.01261-18
World Economic Forum (2016). The new plastics economy: Rethinking the future of plastics. Available at: https://ellenmacarthurfoundation.org/the-new-plastics-economy-rethinking-the-future-of-plastics.
Wright R. J., Bosch R., Gibson M. I., Christie-Oleza J. A. (2020). Plasticizer degradation by marine bacterial isolates: A proteogenomic and metabolomic characterization. Environ. Sci. Technol. 54, 2244–2256. doi: 10.1021/acs.est.9b05228
Yoshida S., Hiraga K., Takehana T., Taniguchi I., Yamaji H., Maeda Y., et al. (2016). A bacterium that degrades and assimilates poly(ethylene terephthalate). Science 351, 1196–1199. doi: 10.1126/SCIENCE.AAD6359
Zeghal E., Vaksmaa A., Vielfaure H., Boekhout T., Niemann H. (2021). The potential role of marine fungi in plastic degradation – a review. Front. Mar. Sci. 8. doi: 10.3389/FMARS.2021.738877/BIBTEX
Zettler E. R., Mincer T. J., Amaral-Zettler L. A. (2013). Life in the “Plastisphere”: Microbial communities on plastic marine debris. Environ Sci Technol. 47 (13), 7137–7146. doi: 10.1021/es401288x
Zhang H., Wang X. (2016). Modular co-culture engineering, a new approach for metabolic engineering. Metab. Eng. 37, 114–121. doi: 10.1016/J.YMBEN.2016.05.007
Zhu B., Wang D., Wei N. (2022). Enzyme discovery and engineering for sustainable plastic recycling. Trends Biotechnol. 40, 22–37. doi: 10.1016/J.TIBTECH.2021.02.008
Zrimec J., Kokina M., Jonasson S., Zorrilla F., Zelezniak A. (2021). Plastic-degrading potential across the global microbiome correlates with recent pollution trends. MBio 12, e021552. doi: 10.1128/MBIO.02155-21/SUPPL_FILE/MBIO.02155-21-ST003.TXT
Keywords: plastic, bioremediation, marine, microorganism, ecosystem, community, microbial
Citation: Alnahdi KA, Alali LW, Suwaidan MK and Akhtar MK (2023) Engineering a microbiosphere to clean up the ocean – inspiration from the plastisphere. Front. Mar. Sci. 10:1017378. doi: 10.3389/fmars.2023.1017378
Received: 11 August 2022; Accepted: 06 January 2023;
Published: 01 February 2023.
Edited by:
Daniel Rittschof, Duke University, United StatesReviewed by:
Jason Somarelli, Duke University Medical Center, United StatesCopyright © 2023 Alnahdi, Alali, Suwaidan and Akhtar. This is an open-access article distributed under the terms of the Creative Commons Attribution License (CC BY). The use, distribution or reproduction in other forums is permitted, provided the original author(s) and the copyright owner(s) are credited and that the original publication in this journal is cited, in accordance with accepted academic practice. No use, distribution or reproduction is permitted which does not comply with these terms.
*Correspondence: M. Kalim Akhtar, bWsuYWtodGFyQHVhZXUuYWMuYWU=
Disclaimer: All claims expressed in this article are solely those of the authors and do not necessarily represent those of their affiliated organizations, or those of the publisher, the editors and the reviewers. Any product that may be evaluated in this article or claim that may be made by its manufacturer is not guaranteed or endorsed by the publisher.
Research integrity at Frontiers
Learn more about the work of our research integrity team to safeguard the quality of each article we publish.