- 1State Key Laboratory of Estuarine and Coastal Research, East China Normal University, Shanghai, China
- 2School of Business, Ningbo University, Ningbo, China
- 3Marine Economic Research Center, Dong Hai Strategic Research Institute, Ningbo University, Ningbo, China
- 4Instituto de Investigacións Mariñas, Consejo Superior de Investigaciones Científicas (IIM-CSIC), Vigo, Spain
- 5Biogeochemistry Research Group, School of Natural Sciences, Trinity College Dublin, Dublin, Ireland
- 6School of Oceanography, Shanghai Jiao Tong University, Shanghai, China
The anthropogenic introduction of significant amounts of reactive nitrogen in the coastal zone particularly since the discovery and application of the Haber-Bosch process has profound consequences over organic carbon storage and transformations at both regional and global scales. Here, we review our current knowledge on cause-effect chains for nitrogen, especially dissolved inorganic nitrogen, on organic carbon cycling in coastal tropical systems. We focus on the feedback mechanisms for turnover of different organic carbon species to nitrogen excess and links to current environmental and climate changes. We pay special attention to organic carbon dynamics in tropical coasts due to their high primary productivity, rapid sedimentation, and significant needs of nitrogen for agriculture and industry usages. Together with land-use changes and economy development, we highlight the vulnerability of carbon storage in tropical coasts triggered by nitrogen overloading and outline possible industrial strategies with low carbon cycling disturbance to benefit the development of tropical countries.
Introduction
Coastal belts are zones of intense organic carbon storage and cycling due to the close interaction between the land, the sea, the benthic compartment and the atmosphere. Enhanced local productivity adds to the transport of terrestrially-derived organic material through surface loading or submarine groundwater discharge (Bianchi et al., 2018; Jiang et al., 2020). As the electron donor (energy provider) in heterotrophic biogeochemical reactions, the importance of organic carbon on element cycling is fundamental. Similarly, nitrogen is an essential element for life and frequently enriched in coastal zones due to its vast and intensive application in agriculture and industry (Hutchins and Capone, 2022). Nitrogen is particularly mobile in the biosphere due to its large range of chemical forms and redox states, participating in a wide range of biogeochemical reactions. Both the quantity and quality of organic carbon are important drivers of benthic heterotrophic nitrogen reaction pathways (Ibánhez and Rocha, 2017), which in turn control the abundance and composition of benthic microbiota or pelagic plankton species (Rocha et al., 2022). Moreover, the autotrophic reactions in nitrogen cycling, e.g., nitrification, are also tightly linked to carbon sources since the labile fraction of organic carbon (e.g., amino acids and polypeptide) is essential in benthic metabolism (Castaldelli et al., 2019). The high primary production characteristic of coastal zones compared to the open ocean together with carbon transport from the continent lead to high standing stocks of organic carbon. In tropical coastal systems, a significant fraction of this carbon is preserved in different compartments such as mangroves, seagrass meadows, macroalgae forests and phytoplankton, with different retention time scales (Spivak et al., 2019). The high sequestration rates in the tropical zone accumulate substantial quantities of organic carbon, in layers even reaching several meters in thickness (e.g., peat) along coastal lines (Wu et al., 2019). Despite the importance of the tropical coastal organic carbon pool in the global carbon cycle, the feedback mechanisms between organic carbon and dissolved inorganic nitrogen (DIN) are barely explored though a tight reaction chain between carbon and nitrogen exists. Here, we review organic carbon storage and cycling patterns and reactive linkages between DIN and organic carbon in tropical coastal zones. In addition, the economic dimension of these processes is discussed, with a focus on industry development targeting reduced carbon emissions and national economy development of tropical countries.
Organic carbon cycling in tropical coasts
The high temperature and intense precipitation characteristic of tropical shores (frequently > 2000 mm yr-1) accelerate the hydraulic cycle and hence favor the transport of terrestrial organic carbon (both dissolved: DOC and particulate: POC) across the land-ocean interface (Moore et al., 2013; Figure 1A). Additionally, large forest coverage in river catchments enhances terrestrial carbon content in river water. Rivers flowing through tropical peatland contain substantial humic materials and the DOC concentration in tea-color river waters can reach >2000 μM even in small river catchments (e.g., Maludam River, Malaysia; river catchment of 432 km2; Martin et al., 2018). Even in tropical rivers without peat coverage (e.g., Wenchang River, China), the riverine DOC level still ranges from 150 to 560 μM (Fu et al., 2013), frequently higher than similar rivers located in sub-tropical and temperate zones, e.g., Changjiang (108 to 133 μM, Bao et al., 2015), Rhine River (240 μM) and Thames River (298 μM; Painter et al., 2018). Highly turbid rivers (e.g., Rajang, Malaysia) are also major contributors of land-derived POC to the tropical coast (Zhu et al., 2020). This tropical riverine carbon transport gains disproportionate importance during tropical storms and erosive transport of POC to the coast (Hilton et al., 2008). Tropical rivers are estimated to account for about ~60% of the global continental carbon transport to the coast (Ludwig et al., 1996). The acceleration of the hydrologic cycle also increases the transport of organic carbon through submarine groundwater discharge (SGD), of particular importance in tropical islands (Moosdorf et al., 2015). The continental endmember of SGD is frequently enriched in high-molecular DOC species derived from plant tissue decomposition and hence SGD is a non-point source of complex DOC to tropical coasts (Wu et al., 2021).
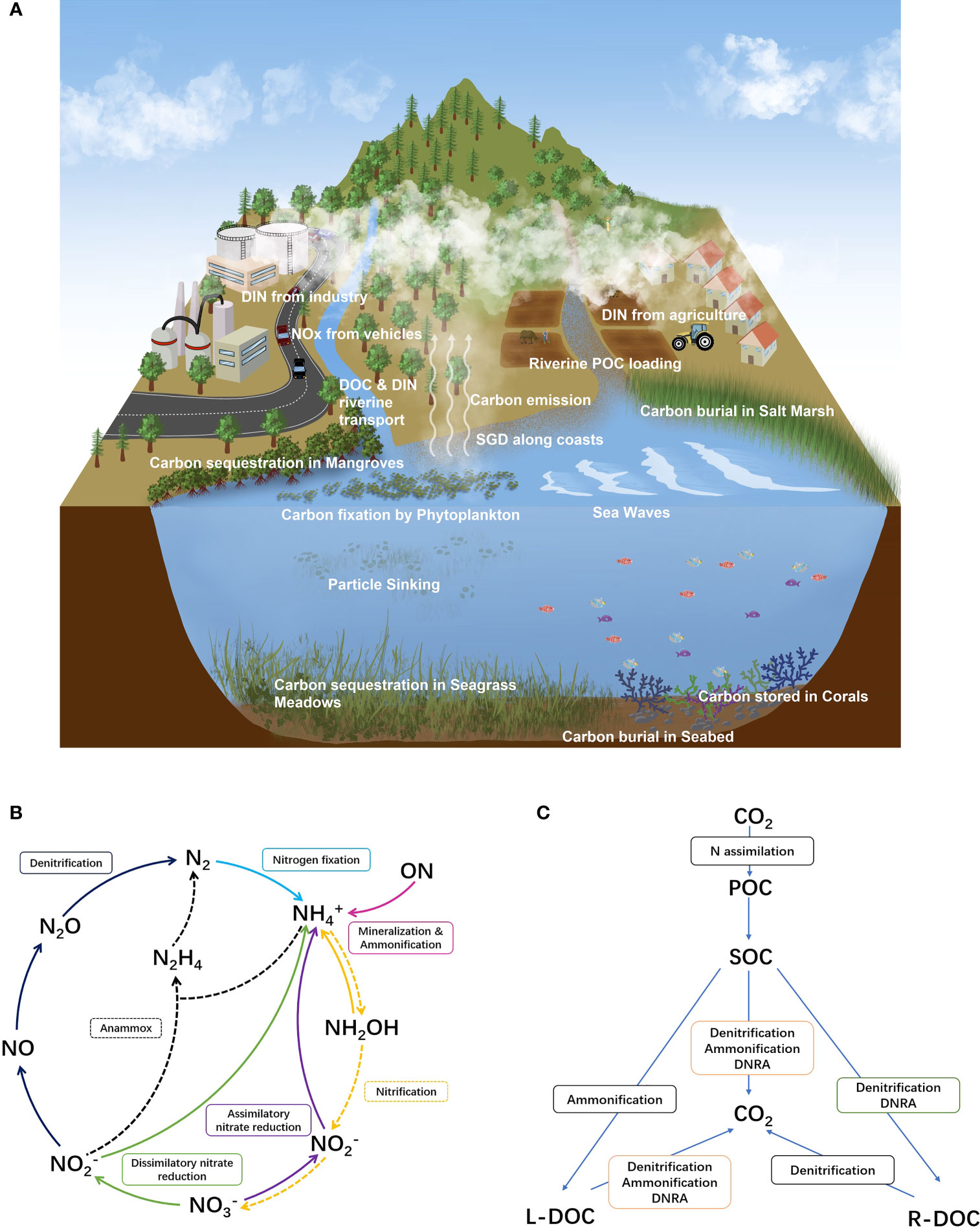
Figure 1 Sketch of carbon storage and transport routes in coastal zones (A). This figure also shows the main transport routes of terrestrial DIN to the coast; (B) Biogeochemical cycling of N in marine environments with different pathways. ON indicates organic nitrogen. In the figure, reactions with dash lines indicate autotrophic reactions while the solid indicates heterotrophic reactions; (C) transformation among SOC, labile DOC (L-DOC), recalcitrant DOC (R-DOC), POC and CO2 in sediment environments coupled with different N reaction pathways (including priming effects).
The volatile fractions of DOC would evaporate from land into the atmosphere and reach coastal zones via dry and wet deposition (Chow et al., 2010). Atmospheric transport can be also a relevant source of POC to the tropical coast, particularly during fire events as black carbon (Liu et al., 2022) and aeolian dust, particularly in the tropical Atlantic originated from the Sahara and the Sahel (Eglinton et al., 2002; Ibánhez et al., 2022). The high temperature of tropical zones further enhances coastal ventilation and terrestrial volatile compounds are rapidly spread through Walker Circulation to long distances from the source (Jiang et al., 2021a). The in-situ production of DOC and POC in tropical coastal ecosystems mainly due to the activity of different primary producers is also a large source of organic carbon due to the general high productivity of tropical coasts (Figure 1A). Decay and decomposition of this primary production and resulting debris feeds the coastal and benthic organic carbon inventory.
Before setting on the seabed, a fraction of DOC and POC, typically labile fractions (L-DOC), e.g., oligosaccharides and amino acids, is rapidly consumed in coastal waters by a wide range of microbiota-mediated reactions (Nelson and Wear, 2014). Generally, the residence time of highly labile DOC in ocean waters is up to approximately one week (Hopkinson et al., 2002). The fate of this reactive DOC pool includes adsorption onto mineral substances, biological assimilation and fully consumption to carbon dioxide (CO2). Organic matter mineralization generally increases with increasing temperature and thus, it is particularly intense in tropical coastal ecosystems (Cardoso et al., 2014). The recalcitrant DOC fraction dispersed in coastal waters, such as humic materials, presents residence times that can reach years to decades (Opsahl and Benner, 1997). Apart from exportation to the adjacent open ocean, persistent organic matter fractions are buried in coastal sediments via diagenesis, undergoing benthic aerobic (surface 2 to 10 cm depth) and anaerobic (frequently below 10 cm depth) transformations (Bianchi et al., 2018). Currently, the global flux of carbon from land to coastal oceans is assumed to be 616 Tg yr-1, of which approximately 210 Tg yr-1 is retained in coastal sediments (Barrón and Duarte, 2015). Tropical mangroves alone are responsible for ca. 26 Tg yr-1 of organic carbon burial, i.e., about 12% of the global burial rate of global coastal sediments (Breithaupt et al., 2012).
Biogeochemical feedbacks of nitrogen to carbon cycling
Coastal zones also receive substantial reactive nitrogen, mainly derived from a series of anthropogenic activities, such as agriculture, sewage disposal, petrochemical industry, fermentation engineering, etc. (Jiang et al., 2019). The geographical overlap between DIN and organic carbon enrichment in coastal zones defines the tightly biogeochemical interaction of these elements. According to the compartment, the influence of DIN on carbon cycling and storage in tropical coastal zones is summarized as:
Benthic environments: In cohesive sediments, the effects of DIN additions on carbon storage vary significantly under aerobic and anaerobic conditions. In aerobic layers, mainly the top layer (from a few mm to 1-2 cm), nitrate (NO3-) and nitrite (NO2-) compete with a series of strong oxidants, especially dissolved oxygen (DO) and ferric iron (Song et al., 2021). In this environment, NH4+ production via mineralization and ammonification requires the supply of organic matter, indicating carbon consumption (Figure 1B). Due to the relatively weak oxidation potential compared to dissolved oxygen, NO3- and NO2- may participate in benthic carbon cycling via stimulating microbial growth and adding POC in both oxic and anoxic sediments (Figure 1C). The importance of NO3- and NO2- as oxidants gains relevance in anoxic sediments due to the oxidation of organic carbon (production of CO2) via the denitrification process (Figure 1C). Heterotrophic denitrification may include the oxidation of both DOC and sediment organic carbon (SOC) in sediment columns (Plummer et al., 2015, Figure 1C), Even the degradation of recalcitrant organic pollutants in mangrove sediments could be accelerated by the presence of NO3- likely via carbon-dependent denitrification (Jiang et al., 2016). Similarly, organic carbon, especially labile fractions, such as phytoplankton debris, is also needed for the dissimilatory nitrate reduction to ammonium (DNRA) process in coastal cohesive sediments (Hardison et al., 2015). The presence of other low-molecular organic compounds, such as oxalate, citrate and glucose, could also markedly enhance DNRA rates (Liu et al., 2016). In fact, DNRA rates showed higher sensitivity to labile carbon additions than denitrification (Robinson et al., 2018). Heterotrophic denitrification and DNRA compete for electron donors from variable carbon sources in benthic systems (Figure 1C). Although the fermentative DNRA process is still poorly understood, highly reducing and organic rich conditions, high NO3- and high C to N ratios in the organic matter all seem to favor DRNA over canonical denitrification (Fazzolari et al., 1998; Tomaszek and Rokosz, 2007). As such, DNRA seems to be a dominant nitrate reduction process in tropical cohesive sediments (Dong et al., 2011). Although both heterotrophic processes produce the potent greenhouse gas N2O as byproduct, the potential prevalence of DNRA over denitrification has strong implications for C and N cycling in coastal tropical ecosystems: the end-product of DNRA is ammonium, thus recirculating N into bioavailable forms, eventually reaching surface waters where it can feed the N2O-producing nitrification process and enhance primary production. Another anoxic, microbially-mediated DIN process is the autotrophic Anammox (anaerobic ammonium oxidation), that reduces nitrite with ammonium to produce N2. Notably, anammox doesn’t produce N2O as byproduct (Van De Graaf et al., 1996). In cohesive marine sediments, anammox shows a negative correlation with the labile organic carbon content (Rich et al., 2020). In permeable sediments, DO penetration depths that can be orders of magnitude higher than in cohesive sediments, favoring the creation of anoxic microniches thus placing oxic and anoxic DIN processing in close proximity (e.g., Jiang et al., 2021b). The injection of NO3- into permeable sediment porewaters has been identified to enhance denitrification rates, although porewater DOC content, even the labile fractions, seems not to be the preferred carbon source when SOC is present (e.g., Jiang et al., 2018a; Jiang et al., 2021b). DNRA in permeable sediments also significantly relays on carbon sources. More importantly, DNRA could have stronger carbon mobilization potential than denitrification due to a higher relative proportion in NO3- removal in permeable sediments compared to cohesive sediments as found in the northern Baltic Sea (Hellemann et al., 2020). The high organic matter breakdown capacity of permeable sediments prevents the accumulation of large quantities of organic matter. These organic carbon-poor conditions could favor the occurrence of the autotrophic anammox process by limiting heterotrophic nitrate reduction pathways as observed in temperate latitudes (e.g., Kroeger and Charette, 2008). Adding to the commonly found organic C limitation of the local microbial community, N seems to be also in deficit in these environments. In temperate permeable sediments, the sudden availability of DIN within the porewater seems to act as a “primer” of SOC decomposition by precluding N limitation of the local community and increasing their ability to process refractory SOC (Ibánhez and Rocha, 2014; Ibánhez et al., 2021). Consequently, DIN addition accelerates SOC processing, limiting the ability of permeable sediments to store organic C. Despite the low sedimentary organic carbon content characteristic of coastal permeable sediments, their large volume suggests this may be an important mechanism of organic C mobilization. Whether this effect occurs in tropical permeable sediments is yet to be confirmed.
Water column: DIN amendment enhances OC storage in primary producers, while the assimilation of NO3- likely consumes DOC in cells (Figure 1B). N fixation function also requires OC from phytoplankton cells while such losses could rapidly be compensated by photosynthesis. DIN additions to coastal water can trigger desorption of DOC from suspended particle surfaces due to competition with sorption sites (Engel and Chefetz, 2016). In surface waters, DOC and POC are significantly influenced by photo-reactions, such as photobleaching, particularly intense in the tropical zone (Zhou et al., 2019). The breakdown of high-molecular carbon compounds produces labile organic matter fractions for the transformation of DIN via aerobic denitrification, typically occurring on particle surfaces (Jiang et al., 2019) and hence accelerates the consumption of organic carbon by heterotrophs. Moreover, apart from toxicity effects derived mainly from the addition of NH4+ (toxicity varied among species; Collos and Harrison, 2014), DIN additions could significantly enhance the biomass of primary producers in coastal waters and hence increase biological POC and DOC pools promoting large ecological changes (e.g., proliferation of harmful algae blooms), eutrophication and oxygen decline (e.g., Peyman et al., 2017). Eutrophication may provide water column low-oxygen (micro)environments favoring the occurrence of denitrification, anammox and DNRA in surface waters (Broman et al., 2021). As an heterotrophic process, denitrification decreases the organic carbon pool while denitrifiers in anoxic water may preferentially utilize fresher and labile autochthonous POC instead of DOC (Zeng et al., 2019). DNRA in hypoxic waters also prefers autochthonous carbon (Broman et al., 2021), while the preference between POC and DOC is still undocumented. In the tropical zone, together with high water temperatures, the loading of exogenous nutrients may easily boost the carbon pool in waters, while the organic carbon enrichment then accelerates decomposition via heterotrophic pathways. Compared with denitrification, DNRA still stores reactive nitrogen in the DIN inventory. Linked with nitrification, DNRA continuously consumes pelagic organic carbon and produces N2O. Anoxic C processing enhancement together with accelerated N cycling drove by eutrophication seems to enhance greenhouse gas production (CO2, CH4 and N2O) in tropical estuaries (Nguyen et al., 2022).
Atmosphere: Atmospheric transport is a key fertilization process to support surface ocean carbon fixation (Ibánhez et al., 2022). In addition, aerosols are also a pool for organic carbon, containing substance from terrestrial volatilization (Jiang et al., 2021b) and emission of sea spray driven by waves (Rinaldi et al., 2020). Organic fractions could be attached on the surface of colloidal particles, while the residence time is still limited due to the exposure to ultraviolet rays, varying from several hours to weeks. The main interaction between DIN species and organic carbon in the atmosphere is mainly via different photochemical reactions in aerosols. In particular, the decomposition of organic matter in aerosols near the ocean surface likely produces substantial small molecular acids, such as oxalic acid and formic acid (Carlton et al., 2007). NH4+ in aerosols tends to neutralize these acid compounds. The effect of nitrogen oxides would be more complex. NO3- in the humid atmosphere could produce OH- and O(3P) after receiving ultraviolet rays. A similar production of OH- is also obtained when NO2 and NO are exposed to strong sunlight. Both OH- and O(3P) have a great oxidation capability, accelerating the decomposition of organic carbon in aerosols (Dominé and Shepson, 2002). Currently, though research on the chemical dynamics related with organic carbon and DIN in the tropical atmospheric environment is limited, the strong sunlight exposure and high temperature likely accelerates the organic carbon decomposition and leads to lower DOC content of wet deposition.
Biota: Anthropogenic DIN enhances the growth of primary producers and increases carbon sequestration but also eutrophication and other deleterious effects over the coastal biota (Howarth, 1988). In tropical ecosystems, apart from phytoplankton and algae living in the water column, mangrove forests and seagrass meadows contribute substantially to carbon fixation. Nevertheless, seagrass meadows are very sensitive to increasing DIN loads. For instance, DIN enrichment decreases eelgrass shoot density and biomass (Deegan et al., 2002). Anthropogenic DIN additions to tropical seagrass ecosystems can promote toxicity to the individuals and large ecological changes such as the growth of epiphytes and algae with direct impacts over seagrass populations (Burkholder et al., 2007). In contrast, the amendment of NO3- and NH4+ to the surrounding water column of mangrove forests could stimulate their growth even when the loading level could be toxic to phytoplankton species. Mangrove ecosystems generally buffer the transfer of redundant N originated from land to the coast while stimulating their growth (Wu et al., 2008). This growth stimulation promoted by DIN addition varies among leaves, roots and stems. Incubation experiments showed that the addition of NH4NO3 to mangrove sediments follows the roots>leaves>stems route (Jiang et al., 2016). Compared with stems and leaves, carbon retention rates associated to roots in sediments is higher due to lower impacts of hydraulic transport. This reinforces the significance of mangrove systems in carbon sequestration along tropical coasts. Apart from direct influence on root biomass, DIN amendment also conditions the interaction between plant roots and sediments in the rhizosphere (Jiang et al., 2016). The addition of DIN can significantly stimulate root activity (Jiang et al., 2018b), thus enhancing the release of low-molecular sugar and amino acids (Weng et al., 2013). More importantly, mineralization of SOC in the rhizosphere seems not accelerated by DIN additions (Keuskamp et al., 2013). Overall, the organic carbon pool in the rhizosphere might be enhanced by increasing DIN loadings originated from land, reinforcing the large contribution of mangrove forests to the global blue carbon pool. Nevertheless, the long-term impact of N enrichment over the stored C pool of mangrove ecosystems remains uncertain. Along coasts, coral reefs (warm water species) are exclusive of tropical ecosystems and N additions promote large impacts over them and related carbon processing (Lønborg et al., 2018). Generally, coral reefs are flourished in oligotrophic environments with a rapid internal nutrient circling via decomposition and nitrogen fixation to sustain their growth (O’Neil and Capone, 2008). Low-level NH4+ likely enhance coral growth rate, while NO3- addition increases oxidative stress in corals (Zhao et al., 2021). The amendment of excess DIN to coral reefs enhances the incidence of coral diseases caused by predation on corals (Zhao et al., 2021) and hence decreases the organic carbon pool in coral systems. More importantly, eutrophication driven by DIN additions to coral reefs likely increases algal bloom frequencies and invasion of macro algae (Lapointe et al., 2019), leading to a permanent change in organic carbon storage and carbon cycling in these regions.
Economic evaluation and development
The enhanced production of greenhouse gases due to anthropogenic disturbance of tropical ecosystems promotes profound negative feedbacks in the climate system and the biosphere. To prevent further degradation, the emission of greenhouse gases to the atmosphere has been regulated in a series of global conventions. Consequently, carbon sequestration represents now economic income from a market trading while greenhouse gas emissions likely result in economic losses (Ellerman et al., 2008). Organic carbon storage and mobilization in tropical coasts is highly dynamic and strongly linked to DIN cycling. Accordingly, increased N inputs likely influence the local economy. For instance, high levels of NO3- in tropical waters trigger CO2 emissions via heterotrophic pathways (Figure 1C). To completely reduce 10 μM of riverine NO3-, approximately equal levels of SOC/DOC need to be transformed to CO2 in coastal environments. In a low discharge tropical river (e.g., the Indus River, Pakistan, 5 km3 yr-1), such CO2 emission can lead to an economic loss of around 51, 000 Euro each year based on recent costs in the European Climate Exchange (around 85 Euro ton-1 CO2 emission during 2022). In average discharge rivers (e.g., Rajang, Malaysia, 110 km3 yr-1), the annual economic losses to reduce 10 μM of riverine NO3- increases to ~1.2 million Euro. Logically, in tropical rivers with large discharges (e.g., Amazon River) or NO3- heavily enriched conditions, such economic losses would be much more significant.
Accordingly, proper management on N loading is urgent to tropical countries, both from an environmental and economic point of view. Currently, one of the most significant sources of anthropogenic DIN loads to tropical coasts is the indiscriminate use of fertilizers, which drives the leakage of both NH4+ and NO3- into the coastal region coupled with hydraulic transport (Table 1). The amount of chemical fertilizer applied in tropical countries is one of the largest globally, such as 415 kg hectare-1 yr-1 in Vietnam, 569 kg hectare-1 yr-1 in Egypt and 2106 kg hectare-1 yr-1 in Malaysia (https://data.worldbank.org/indicator/AG.CON.FERT.ZS). Together with high precipitation rates, the unconsumed fertilizer may directly drain from estuaries and subterranean estuaries into tropical coasts (Lønborg et al., 2021), which highlights the urgency and necessity of a better dimensioning of fertilizer application and replacement from chemical fertilizer to organic fertilizer and slow-release fertilizer thus reducing application quantities (Liang et al., 2022). Moreover, the potentiation of nitrogen-fixing legume species, e.g., Soybean, Groundnut and Cowpea (Peoples and Herridge, 1990), in the tropical agriculture, could favor a balanced N application on land. DIN originated from untreated sewage, i.e., a large pathway for NH4+ discharge to the coast, including both municipal and industry, is a global threat to coastal ecosystems particularly near tropical megacities (e.g., Eisenberg et al., 2016). The improvement of the water sanitation network, the installation of highly-efficient treatments in industries combined with small-scale treatments in rural areas would significantly reduce anthropogenic DIN loads to the tropical coast. As another significant contributor of NO3- to coastal systems, vehicles based on fuel combustion would necessarily transit towards a different model based on cleaner energy to reduce both greenhouse gas and anthropogenic N emissions. Notably, these schemes and management actions require financial support. In that regard, the economic compensations from improving carbon sequestration in coasts could act as an economic positive feedback mechanism in tropical countries.
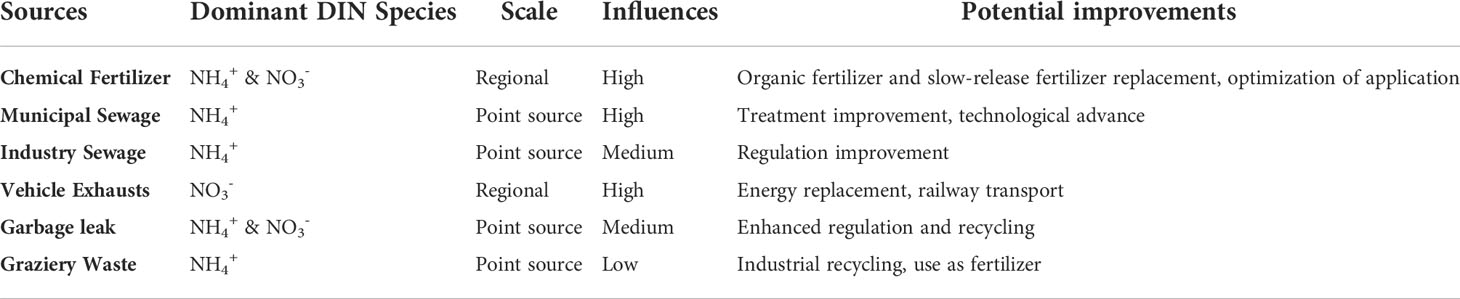
Table 1 Anthropogenic Nitrogen sources and potential approaches to reduce DIN emissions to tropical coastal environments.
From a view of long-term development, a proper industry improvement in the tropical countries would be needed to guarantee the resilience of the stored coastal carbon. Target on the traditional industries, such as agriculture, the replacement of heavily fertilizer-dependent species would be the selection, which requires a sharp understanding on the global market requirement. In addition, the development of eco-tourism in tropical coasts could be further encouraged by local governments taking advantage of investments from the World Bank, IMF and international travel corporations. The combination of environmentally friendly marine culture and recovery of tropical primary production systems, such as mangrove forests and seagrass meadows, could also enhance carbon income from the global market from long-term trading.
Conclusion
Tropical coastal ecosystems are a key node for global carbon storage, while the organic carbon inventories in many subsystems are significantly influenced by a wide range of biogeochemical factors. This study reviewed the impact of different DIN species (NH4+, NO3- and NO2-) on organic carbon storage in sediments, water column, atmosphere and biological organisms of the tropical band. Generally, apart from stimulation on primary producers, the amendment of NO3- and NO2- significantly enhances consumption of SOC and DOC, especially labile fractions in sediments and waters via heterotrophic reactions. Currently, chemical fertilizer, sewage and vehicle exhaust are key contributors to the DIN pool of tropical coasts, while the management and regulation to constrain DIN outputs require significant economic support. Based on global trading on carbon storage, the financial benefits of carbon sequestration along tropical coasts could act in support of a changing paradigm of tropical coastal uses and resilience.
Author contributions
SJ and JI conceived the study. SJ, JI, and LJ wrote the manuscript. All authors revised the manuscript. All authors read and approved the final manuscript.
Funding
This work is supported by the National Natural Science Foundation of China (Nos. 42090043 and 41876074).
Conflict of interest
The authors declare that the research was conducted in the absence of any commercial or financial relationships that could be construed as a potential conflict of interest.
Publisher’s note
All claims expressed in this article are solely those of the authors and do not necessarily represent those of their affiliated organizations, or those of the publisher, the editors and the reviewers. Any product that may be evaluated in this article, or claim that may be made by its manufacturer, is not guaranteed or endorsed by the publisher.
References
Bao H., Wu Y., Zhang J. (2015). Spatial and temporal variation of dissolved organic matter in the changjiang: Fluvial transport and flux estimation. JGR. Biogeosci. 120, 1870–1886. doi: 10.1002/2015JG002948
Barrón C., Duarte C. M. (2015). Dissolved organic carbon pools and export from the coastal ocean. Global Biogeochem. Cy. 29, 1725–1738. doi: 10.1002/2014GB005056
Bianchi T. S., Cui X., Blair N. E., Burdige D. J., Eglinton T. I., Galy V. (2018). Centers of organic carbon burial and oxidation at the land-ocean interface. Org. Geochem. 115, 138–155. doi: 10.1016/j.orggeochem.2017.09.008
Breithaupt J. L., Smoak J. M., Smith T. J., Sanders C. J., Hoare A. (2012). Organic carbon burial rates in mangrove sediments: Strengthening the global budget. Global Biogeochem. Cycles. 26, GB3011. doi: 10.1029/2012GB004375
Broman E., Zilius M., Samuiloviene A., Vybernaite-Lubiene I., Politi T., Klawonn I., et al. (2021). Active DNRA and denitrification in oxic hypereutrophic waters. Water Res. 194, 116954. doi: 10.1016/j.watres.2021.116954
Burkholder J. M., Tomasko D. A., Touchette B. W. (2007). Seagrasses and eutrophication. J. Exp. Mar. Biol. Ecol. 350, 46–72. doi: 10.1016/j.jembe.2007.06.024
Cardoso S. J., Enrich-Prast A., Pace M. L., Roland F. (2014). Do models of organic carbon mineralization extrapolate to warmer tropical sediments? Limnol. Oceanogr. 59, 48–54. doi: 10.4319/lo.2014.59.1.0048
Carlton A. G., Turpin B. J., Altieri K. E., Seitzinger S., Reff A., Lim H.-J., et al. (2007). Atmospheric oxalic acid and SOA production from glyoxal: Results of aqueous photooxidation experiments. Atmos. Environ. 41, 7588–7602. doi: 10.1016/j.atmosenv.2007.05.035
Castaldelli G., Colombani N., Soana E., Vincenzi F., Fano E. A., Mastrocicco M. (2019). Reactive nitrogen losses via denitrification assessed in saturated agricultural soils. Geoderma 337, 91–98. doi: 10.1016/j.geoderma.2018.09.018
Chow J. C., Watson J. G., Lowenthal D. H., Chen L. W. A., Motallebi N. (2010). Black and organic carbon emission inventories: review and application to California. J. Air Waste Manage. 60, 497–507. doi: 10.3155/1047-3289.60.4.497
Collos Y., Harrison P. J. (2014). Acclimation and toxicity of high ammonium concentrations to unicellular algae. Mar. pollut. Bull. 80, 8–23. doi: 10.1016/j.marpolbul.2014.01.006
Deegan L. A., Wright A., Ayvazian S. G., Finn J. T., Golden H., Merson R. R., et al. (2002). Nitrogen loading alters seagrass ecosystem structure and support of higher trophic levels. Aquat. Conserv. 12, 193–212. doi: 10.1002/aqc.490
Dominé F., Shepson P. B. (2002). Air-snow interactions and atmospheric chemistry. Science 297, 1506–1510. doi: 10.1126/science.1074610
Dong L. F., Sobey M. N., Smith C. J., Rusmana I., Phillips W., Stott A. M., et al. (2011). Dissimilatory reduction of nitrate to ammonium, not denitrification or anammox, dominates benthic nitrate reduction in tropical estuaries. Limnol. Oceanogr. 56, 279–291. doi: 10.4319/lo.2011.56.1.0279
Eglinton T. I., Eglinton G., Dupont L., Sholkovitz E. R., Montluçon D., Reddy C. M. (2002). Composition, age, and provenance of organic matter in NW African dust over the Atlantic ocean. Geochem. Geophy. Geosy. 3, 1–27. doi: 10.1029/2001GC000269
Eisenberg J. N. S., Bartram J., Wade T. J. (2016). The water quality in Rio highlights the global public health concern over untreated sewage. Environ. Health Persp. 124, 180–181. doi: 10.1289/EHP662
Ellerman D., Convery F., De Perthuis C. (2008). The European carbon market in action: Lessons from the first trading period. J. Eur. Environ. Plan L. 5, 215–233. doi: 10.1163/161372708X324213
Engel M., Chefetz B. (2016). Adsorption and desorption of dissolved organic matter by carbon nanotubes: effects of solution chemistry. Environ. pollut. 213, 90–98. doi: 10.1016/j.envpol.2016.02.009
Fazzolari É., Nicolardot B., Germon J. C. (1998). Simultaneous effects of increasing levels of glucose and oxygen partial pressures on denitrification and dissimilatory nitrate reduction to ammonium in repacked soil cores. Eur. J. Soil Biol. 34, 47–52. doi: 10.1016/S1164-5563(99)80006-5
Fu J., Tang X. L., Zhang J., Balzer W. (2013). Estuarine modification of dissolved and particulate trace metals in major rivers of East-hainan, China. Cont. Shelf Res. 57, 59–72. doi: 10.1016/j.csr.2012.06.015
Hardison A. K., Algar C. K., Giblin A. E., Rich J. J. (2015). Influence of organic carbon and nitrate loading on partitioning between dissimilatory nitrate reduction to ammonium (DNRA) and N2 production. Geochim. Cosmochim. Ac. 164, 146–160. doi: 10.1016/j.gca.2015.04.049
Hellemann D., Tallberg P., Aalto S. L., Bartoli M., Hietanen S. (2020). Seasonal cycle of benthic denitrification and DNRA in the aphotic coastal zone, northern Baltic Sea. Mar. Ecol. Prog. Ser. 637, 15–28. doi: 10.3354/meps13259
Hilton R. G., Galy A., Hovius N., Chen M.-C., Horng M.-J., Chen H. (2008). Tropical-cyclone-driven erosion of the terrestrial biosphere from mountains. Nat. Geosci. 1, 759–762. doi: 10.1038/ngeo333
Hopkinson C. S. Jr., Vallino J. J., Nolin A. (2002). Decomposition of dissolved organic matter from the continental margin. Deep-Sea. Res. Pt. II. 49, 4461–4478. doi: 10.1016/S0967-0645(02)00125-X
Howarth R. W. (1988). Nutrient limitation of net primary production in marine ecosystems. Ann. Rev. Ecol. 19, 89–110. doi: 10.1146/annurev.es.19.110188.000513
Hutchins D. A., Capone D. G. (2022). The marine nitrogen cycle: new developments and global change. Nat. Rev. Microbiol. 20, 401–414 doi: 10.1038/s41579-022-00687-z
Ibánhez J. S. P., Álvarez-Salgado X. A., Rocha C. (2021). Does nitrate enrichment accelerate organic matter turnover in subterranean estuaries? Front. Mar. Sci. 8, 661201. doi: 10.3389/fmars.2021.661201
Ibánhez J. S. P., Flores Montes M., Lefèvre N. (2022). Evidence for enhanced primary production driving significant CO2 drawdown associated with the Atlantic ITCZ. Sci. Total. Environ. 838, 156592. doi: 10.1016/j.scitotenv.2022.156592
Ibánhez J. S. P., Rocha C. (2014). Effects of recirculation of seawater enriched in inorganic nitrogen on dissolved organic carbon processing in sandy seepage face sediments. Mar. Chem. 166, 48–58. doi: 10.1016/j.marchem.2014.09.012
Ibánhez J. S. P., Rocha C. (2017). Kinetics of inorganic nitrogen turnover in a sandy seepage face on a subterranean estuary. Appl. Geochem. 87, 108–121. doi: 10.1016/j.apgeochem.2017.10.015
Jiang S., Ibánhez J. S. P., Rocha C. (2018a). Influence of labile dissolved organic matter on nitrate reduction in a seepage face. Environ. Sci. pollut. R. 25, 10654–10667. doi: 10.1007/s11356-018-1302-1
Jiang S., Jin J., Jiang S., Wu Y., Wang J., Chen J., et al. (2021a). Nitrogen in atmospheric wet depositions over the East Indian ocean and West pacific ocean: Spatial variability, source identification, and potential influences. Front. Mar. Sci. 7, 600843. doi: 10.3389/fmars.2020.600843
Jiang S., Jin J., Wu Y., Zhang Y., Wei Y., Rocha C., et al. (2021b). Response of nitrate processing to bio-labile dissolved organic matter supply under variable oxygen conditions in a sandy beach seepage face. Front. Mar. Sci. 8, 642143. doi: 10.3389/fmars.2021.64
Jiang S., Liu J., Lu H., Yan C. (2018b). Short-term influence of nutrient availability on the uptake and translocation of phenanthrene in mangrove seedlings. Toxicol. Environ. Chem. 100, 1–14. doi: 10.1080/02772248.2018.1517878
Jiang S., Lu H., Zhang Q., Liu J., Yan C. (2016). Effect of enhanced reactive nitrogen availability on plant-sediment mediated degradation of polycyclic aromatic hydrocarbons in contaminated mangrove sediment. Mar. pollut. Bull. 103, 151–158. doi: 10.1016/j.marpolbul.2015.12.027
Jiang S., Müller M., Jin J., Wu Y., Zhu K., Zhang G., et al. (2019). Dissolved inorganic nitrogen in a tropical estuary in Malaysia: transport and transformation. Biogeosciences 16, 2821–2836. doi: 10.5194/bg-16-2821-2019
Jiang S., Zhang Y., Jin J., Wu Y., Wei Y., Wang X., et al. (2020). Organic carbon in a seepage face of a subterranean estuary: turnover and microbial interrelations. Sci. Total. Environ. 725, 138220. doi: 10.1016/j.scitotenv.2020.138220
Keuskamp J. A., Schmitt H., Laanbroek H. J., Verhoeven J. T., Hefting M. M. (2013). Nutrient amendment does not increase mineralisation of sequestered carbon during incubation of a nitrogen limited mangrove soil. Soil Biol. Biochem. 57, 822–829. doi: 10.1016/j.soilbio.2012.08.007
Kroeger K. D., Charette M. A. (2008). Nitrogen biogeochemistry of submarine groundwater discharge. Limnol. Oceanogr. 53, 1025–1039. doi: 10.4319/lo.2008.53.3.1025
Lønborg C., Álvarez–Salgado X. A., Duggan S., Carreira C. (2018). Organic matter bioavailability in tropical coastal waters: The great barrier reef. Limnol. Oceanogr. 63, 1015–1035. doi: 10.1002/lno.10717
Lønborg C., Müller M., Butler E. C., Jiang S., Ooi S. K., Trinh D. H., et al. (2021). Nutrient cycling in tropical and temperate coastal waters: Is latitude making a difference? Estuar. Coast. Shelf S. 262, 107571. doi: 10.1016/j.ecss.2021.107571
Lapointe B. E., Brewton R. A., Herren L. W., Porter J. W., Hu C. (2019). Nitrogen enrichment, altered stoichiometry, and coral reef decline at looe key, Florida keys, USA: a 3-decade study. Mar. Biol. 166, 108. doi: 10.1007/s00227-019-3538-9
Liang Z., Jin X., Zhai P., Zhao Y., Cai J., Li S., et al. (2022). Combination of organic fertilizer and slow-release fertilizer increases pineapple yields, agronomic efficiency and reduces greenhouse gas emissions under reduced fertilization conditions in tropical areas. J. Clean. Prod. 343, 131054. doi: 10.1016/j.jclepro.2022.131054
Liu X., Han J. G., Ma Z. W., Wang Q., Li L. H. (2016). Effect of carbon source on dissimilatory nitrate reduction to ammonium in costal wetland sediments. J. Soil Sci. Plant Nutt. 16, 337–349. doi: 10.4067/S0718-95162016005000029
Liu D., Zhou C., Keesing J. K., Serrano O., Werner A., Fang Y., et al. (2022). Wildfires enhance phytoplankton production in tropical oceans. Nat. Commun. 13, 1–9. doi: 10.1038/s41467-022-29013-0
Ludwig W., Probst J. L., Kempe S. (1996). Predicting the oceanic input of organic carbon by continental erosion. Glob. Biogeochem. Cycles. 10, 23–41. doi: 10.1029/95GB02925
Martin P., Cherukuru N., Tan A., Sanwlani N., Mujahid A., Müller M. (2018). Distribution and cycling of terrigenous dissolved organic carbon in peatland-draining rivers and coastal waters of Sarawak, Borneo. Biogeosciences 15, 6847–6865. doi: 10.5194/bg-15-6847-2018
Moore S., Evans C. D., Page S. E., Garnett M. H., Jones T. G., Freeman C., et al. (2013). Deep instability of deforested tropical peatlands revealed by fluvial organic carbon fluxes. Nature 493, 660–663. doi: 10.1038/nature11818
Moosdorf N., Stieglitz T., Waska H., Dürr H. H., Hartmann J. (2015). Submarine groundwater discharge from tropical islands: a review. Grundwasser 20, 53–67. doi: 10.1007/s00767-014-0275-3
Nelson C. E., Wear E. K. (2014). Microbial diversity and the lability of dissolved organic carbon. P. Natl. Acad. Sci. U.S.A. 111, 7166–7167. doi: 10.1073/pnas.1405751111
Nguyen A. T., Némery J., Gratiot N., Dao T.-S., Le T. T. M., Baduel C., et al. (2022). Does eutrophication enhance greenhouse gas emissions in urbanized tropical estuaries? Environ. pollut. 303, 119105. doi: 10.1016/j.envpol.2022.119105
O’Neil J. M., Capone D. G. (2008). Nitrogen in the Marine Environment (2nd Edition). Eds. Capone, D.G., Bronk, D.A., Mulholland, M.R., Carpenter, E.J. Academic Press. Nitrogen. Mar. Environ. 949–989. doi: 10.1016/B978-0-12-372522-6.00021-9
Opsahl S., Benner R. (1997). Distribution and cycling of terrigenous dissolved organic matter in the ocean. Nature 386, 480–482. doi: 10.1038/386480a0
Painter S. C., Lapworth D. J., Woodward M. S., Kroger S., Evans C. D., Mayor D. J., et al. (2018). Terrestrial dissolved organic matter distribution in the north Sea. Sci. Total. Environ. 630, 630–647. doi: 10.1016/j.scitotenv.2018.02.237
Peoples M. B., Herridge D. F. (1990). Nitrogen fixation by legumes in tropical and subtropical agriculture. Adv. Agron. 44, 155–223. doi: 10.1016/S0065-2113(08)60822-6
Peyman N., Sany S. B. T., Tajfard M., Hashim R., Rezayi M., Karlen D. J. (2017). The status and characteristics of eutrophication in tropical coastal water. Environ. Sci. Proc. Impacts. 19, 1086–1103. doi: 10.1039/C7EM00200A
Plummer P., Tobias C., Cady D. (2015). Nitrogen reduction pathways in estuarine sediments: influences of organic carbon and sulfide. J. Geophys. Res.: Biogeosci. 120, 1958–1972. doi: 10.1002/2015JG003057
Rich J. J., Arevalo P., Chang B. X., Devol A. H., Ward B. B. (2020). Anaerobic ammonium oxidation (anammox) and denitrification in Peru margin sediments. J. Mar. Syst. 207, 103122. doi: 10.1016/j.jmarsys.2018.09.007
Rinaldi M., Paglione M., Decesari S., Harrison R. M., Beddows D. C., Ovadnevaite J., et al. (2020). Contribution of water-soluble organic matter from multiple marine geographic eco-regions to aerosols around Antarctica. Environ. Sci. Technol. 54, 7807–7817. doi: 10.1021/acs.est.0c00695
Robinson G., MacTavish T., Savage C., Caldwell G. S., Jones C. L., Probyn T., et al. (2018). Carbon amendment stimulates benthic nitrogen cycling during the bioremediation of particulate aquaculture waste. Biogeosciences 15, 1863–1878. doi: 10.5194/bg-15-1863-2018
Rocha C., Jiang S., Ibánhez J. S. P., Yang Q., Mazi K., Koussis A. D. (2022). The effects of subterranean estuary dynamics on nutrient resource ratio availability to microphytobenthos in a coastal lagoon. Sci. Total. Environ 851, 157522. doi: 10.1016/j.scitotenv.2022.157522
Song G., Liu S., Zhang J., Zhu Z., Zhang G., Marchant H. K., et al. (2021). Response of benthic nitrogen cycling to estuarine hypoxia. Limnol. Oceanogr. 66, 652–666. doi: 10.1002/lno.11630
Spivak A. C., Sanderman J., Bowen J. L., Canuel E. A., Hopkinson C. S. (2019). Global-change controls on soil-carbon accumulation and loss in coastal vegetated ecosystems. Nat. Geosci. 12, 685–692. doi: 10.1038/s41561-019-0435-2
Tomaszek J. A., Rokosz R. G. (2007). Rates of dissimilatory nitrate redcution do ammonium in two polish reservoirs: Impacts of temperature, organic matter content and nitrate concentration. Environ. Technol. 28, 771–778. doi: 10.1080/09593332808618834
Van De Graaf A. A., De Bruijn P., Robertson L. A., Jetten M. S. M., Kuenen. J. G. (1996). Autotrophic growth of anaerobic ammonium-oxidizing micro-organisms in a fluidized bed reactor. Microbiology 142, 2187–2196. doi: 10.1099/13500872-142-8-2187
Weng B., Xie X., Yang J., Liu J., Lu H., Yan C. (2013). Research on the nitrogen cycle in rhizosphere of Kandelia obovata under ammonium and nitrate addition. Mar. Pollut. Bull. 76, 227–240. doi: 10.1016/j.marpolbul.2013.08.034
Wu Y., Chung A., Tam N. F. Y., Pi N., Wong M. H. (2008). Constructed mangrove wetland as secondary treatment system for municipal wastewater. Ecol. Eng. 34, 137–146. doi: 10.1016/j.ecoleng.2008.07.010
Wu Z., Zhu H., Tang D., Wang Y., Zidan A., Cui Z. (2021). Submarine groundwater discharge as a significant export of dissolved inorganic carbon from a mangrove tidal creek to qinglan bay (Hainan island, China). Cont. Shelf Res. 2021, 223:104451. doi: 10.1016/j.csr.2021.104451
Wu Y., Zhu K., Zhang J., Müller M., Jiang S., Mujahid A., et al. (2019). Distribution and degradation of terrestrial organic matter in the sediments of peat-draining rivers, Sarawak, Malaysian Borneo. Biogeosciences 16, 4517–4533. doi: 10.5194/bg-16-4517-2019
Zeng J., Chen M., Guo L., Lin H., Mu X., Fan L., et al. (2019). Role of organic components in regulating denitrification in the coastal water of daya bay, southern China. Environ. Sci-Proc. Imp. 21, 831–844. doi: 10.1039/C8EM00558C
Zhu Z. Y., Oakes J., Eyre B., Hao Y., Sia E. S. A., Jiang S., et al. (2020). The nonconservative distribution pattern of organic matter in the rajang, a tropical river with peatland in its estuary. Biogeosciences 17, 2473–2485. doi: 10.5194/bg-17-2473-2020
Zhou Y., Martin P., Müller M.. (2019). Composition and cycling of dissolved organic matter from tropical peatlands of coastal Sarawak, Borneo, revealed by fluorescence spectroscopy and parallel factor analysis. Biogeosciences 16, 2733–2749. doi: 10.5194/bg-16-2733-2019
Keywords: biogeochemical cycling, carbon, coastal zones, development, nitrogen, tropical zones
Citation: Jiang S, Jin L, Jin J, Ibánhez JSP, Wu Y and Zhang J (2022) Exploring feedback mechanisms for nitrogen and organic carbon cycling in tropical coastal zones. Front. Mar. Sci. 9:996655. doi: 10.3389/fmars.2022.996655
Received: 18 July 2022; Accepted: 20 September 2022;
Published: 06 October 2022.
Edited by:
Michelle Jillian Devlin, Centre for Environment, Fisheries and Aquaculture Science (CEFAS), United KingdomReviewed by:
Gal Hochman, Rutgers, The State University of New Jersey, United StatesCopyright © 2022 Jiang, Jin, Jin, Ibánhez, Wu and Zhang. This is an open-access article distributed under the terms of the Creative Commons Attribution License (CC BY). The use, distribution or reproduction in other forums is permitted, provided the original author(s) and the copyright owner(s) are credited and that the original publication in this journal is cited, in accordance with accepted academic practice. No use, distribution or reproduction is permitted which does not comply with these terms.
*Correspondence: Juan Severino Pino Ibánhez, anNldmVyaW5vQGlpbS5jc2ljLmVz; Shan Jiang, c2ppYW5nQHNrbGVjLmVjbnUuZWR1LmNu