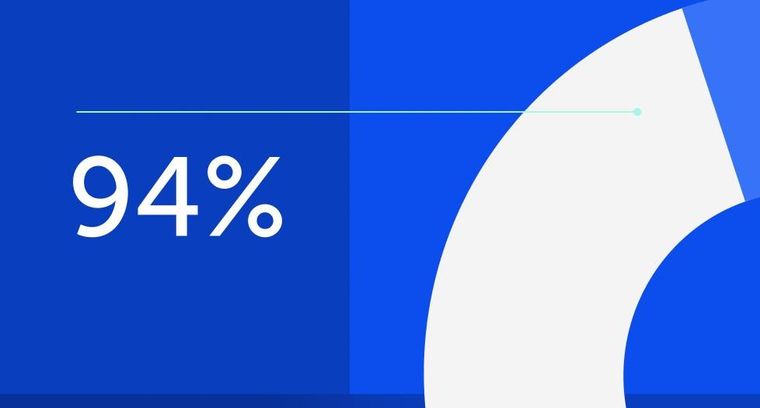
94% of researchers rate our articles as excellent or good
Learn more about the work of our research integrity team to safeguard the quality of each article we publish.
Find out more
ORIGINAL RESEARCH article
Front. Mar. Sci., 23 September 2022
Sec. Marine Ecosystem Ecology
Volume 9 - 2022 | https://doi.org/10.3389/fmars.2022.990853
A correction has been applied to this article in:
Frontiers Corrigendum for "Winter condition, physiology, and growth potential of juvenile Antarctic krill"
In recent years, substantial efforts have been made to understand the implications of climate change on Antarctic krill, Euphausia superba, because of their pivotal role in the Southern Ocean food web and in biogeochemical cycling. Winter is one of the least studied seasons in Antarctica and we have limited understanding about the strategies Antarctic krill use to survive the winter. In particular, data on the winter physiology and condition of juvenile Antarctic krill are severely lacking. From May to September (the austral autumn-winter) of 2019, we maintained juvenile Antarctic krill in large (1,330 L) aquarium tanks at Palmer Station, Antarctica and, at monthly time intervals, measured their physiology and condition. Each tank served as a “food environment scenario”, representing possible food environments the krill may encounter during winter along the Western Antarctic Peninsula. We found that, unlike adults, juvenile krill maintain relatively high respiration rates through the winter and respond positively to increased food concentrations by increasing their ingestion rates. Unlike larval krill, juveniles use lipid stores accumulated during the summer and autumn to sustain themselves through periods of starvation in the winter. We used our empirically derived measurements of physiology and condition to estimate the energy budget and growth potential of juvenile krill during the winter. We found that, given their comparatively high respiration rates, small juvenile krill (20 mg dry weight) would need to encounter food at concentrations of ~ 0.15 mg C L-1 daily to avoid loss of body carbon. Without sufficient lipid reserves, this value increases to ~ 0.54 mg C L-1, daily. The health of juvenile krill in the wintertime is dependent on their ability to accumulate lipid stores in the summer and autumn and to find sufficient food during the winter. Changes in food availability to Antarctic krill throughout the year may become problematic to juvenile krill in the future. Understanding the variability in the winter energy budget of juvenile Antarctic krill will allow us to improve population models that make assumptions on seasonal growth patterns.
Antarctic krill, Euphausia superba (hereafter “krill”), is an essential species in the Southern Ocean. As one of the most abundant species on Earth (Atkinson et al., 2009), krill supports vast numbers of top predators (Laws, 1977; Reid, 1995; Nicol et al., 2008), contributes to biogeochemical cycling (Atkinson et al., 2012; Gleiber et al., 2012), and is the subject of a rapidly growing fishery (Kawaguchi and Nicol, 2007; Nicol et al., 2011). Effective management of the krill fishery requires accurate growth models that take into account the variability in growth with season, ontogeny, food availability, and temperature (Constable et al., 2018). Winter, in particular, is also one of the least understood aspects of the krill life cycle, yet it is during winter that krill are most susceptible to the impacts of climate change (Flores et al., 2012). While important advances have been made in our understanding of the variability in growth rates of larval (Quetin et al., 2003; Ross et al., 2004; Meyer et al., 2009) and adult krill (Tarling et al., 2016) in the winter, we know little about variability in growth of juvenile krill during the dark season.
Much of our current conceptual framework for the autumn-winter physiology and condition of krill is based on relatively recent studies that have taken place in the Weddell and Lazarev Seas (see for example Hagen et al., 2001; Atkinson et al., 2002; Tarling et al., 2006; Meyer, 2012; Schmidt et al., 2014), earlier studies from the northern part of the Western Antarctic Peninsula (WAP) (see for example Quetin and Ross, 1991; Ross and Quetin, 1991; Ross et al., 2000; Quetin et al., 2003; Daly, 2004; Ross et al., 2004), and an older body of literature that set the foundations of our knowledge on krill physiology and condition (see for example Clarke and Morris, 1983; Ikeda, 1985; Ikeda and Bruce, 1986; Kawaguchi et al., 1986). Although this is a substantial body of work, there is still much that we do not know since (i) krill exhibit a high degree of regional variability (Schmidt et al., 2014), and (ii) there has been a severe lack of intensive, long-term, studies focused on krill physiology, due primarily to logistical constraints and challenges associated with conducting long-term experiments (> 1 month) on krill (Meyer and Teschke, 2016).
There are ontogenetic (Meyer and Teschke, 2016) differences in krill physiology that determine their ability to survive the winter, and it is important that we know the physiological response of all stages in the krill life cycle (Meyer and Teschke, 2016). Most winter research on Antarctic krill has been conducted on the larval stage and on adults. Larval krill have high respiration rates and must continue to feed, or they will not survive the winter (Meyer and Teschke, 2016; Ryabov et al., 2017). In contrast, adult krill suppress their metabolism in winter and survive on lipid reserves built up during the summer and autumn (Meyer and Teschke, 2016). Further, while larval krill respond to increased food availability with increased ingestion rates (Meyer et al., 2002), winter ingestion rates of adult krill do not increase even when adult krill are provided with enhanced food supplies (Atkinson et al., 2002). It is less certain precisely where juvenile krill (specifically 1-year old krill) fit on this spectrum, but it is likely they adopt a mix of both larval and adult strategies (Atkinson et al., 2002).
Here, we used long-term experiments to monitor seasonal variability in the physiology and condition of juvenile Antarctic krill from autumn through winter at the Antarctic Peninsula. We used the empirical data derived from this experiment to model food concentration dependent winter growth potential in juvenile krill. We discuss these findings within the context of ecosystem level changes currently observed and predicted for the Western Antarctic Peninsula.
Between April and September 2019, we maintained juvenile krill in four large circular aquarium tanks (1,330-L seawater capacity) over 20-weeks to assess variability in juvenile krill overwintering strategies. Each of the four circular tanks were treated as “food environment” scenarios covering a range of food environment possibilities.
Krill were collected at night in the Gerlache Strait and Wilhelmina Bay (Figure 1) aboard the ARSV Laurence M. Gould using an Isaacs-Kidd Midwater Trawl (IKMT) towed obliquely between the surface and an average of 50 m, targeting krill aggregations observed acoustically. Net contents were immediately emptied into tubs filled with seawater and individual krill were scooped out using soup ladles and transferred to one of two large, insulated holding tanks (~1,000 L). The holding tanks were fitted with a flow-through seawater system to ensure a continuous supply of oxygenated seawater and food, and to flush out waste products. Roughly 5000 individual juvenile krill (~30 mm) were collected. Once at Palmer Station, the insulated holding tanks were transferred from the ship to shore-side aquarium facilities.
Figure 1. Map showing region where juvenile Antarctic krill were collected using the Isaacs-Kidd Midwater Trawl (IKMT).
Once at Palmer Station, healthy, actively swimming krill (~ 30 mm in length, representing juveniles) were gently transferred from the insulated holding tanks and divided randomly between the four circular indoor aquarium tanks. Each tank was filled with ~1,330 L of seawater and was stocked with a packing density of no more than 0.5 krill L-1 (~660 krill). The tanks were supplied with natural unfiltered seawater in a flow-through system. The Palmer Station seawater intake pipe is located on the rocky seafloor at approximately 6 m depth. During the winter, sea ice formed in that area in August and remained until our experiment ended in September. Each tank represented one of four food environment scenarios (FES). For the first 70 days of the 140-day experiment, krill in FES1 and FES2 were supplemented with a diet enriched in a freeze-dried, ground mixed zooplankton assemblage (MZA). Prior to freeze-drying and grinding the zooplankton catch, we assessed its broad taxonomic composition. The zooplankton catch used for MZA was dominated by calanoid copepods, such Calanus propinquus and Metridia spp, and cyclopoid copepods, including Oithona spp. Krill provided with the MZA diet were seen to actively feed on the substance and other researchers have used similarly inert food items to feed krill in experiments (e.g., Hagen et al., 2007). Krill in FES3 and FES4 were supplemented with a locally grown diatom culture (DIA) consisting of Synedropsis sp. At Day 71, the supplemental food in FES1 & FES3 was discontinued, restricting those krill to the limited amount of food in the natural seawater assemblage (NAT). In contrast, FES2 & FES4 continued to receive supplemental diets of MZA and DIA, respectively. For clarity, for the remainder of the paper we will refer to FES1 as MZA-NAT, FES2 as MZA-MZA, FES3 as DIA-NAT, and FES4 as DIA-DIA.
Krill were fed every 3-5 days with either the freeze-dried mixed zooplankton feed (MZA) or the diatom culture (DIA). The concentrations of dried zooplankton and diatoms, once dispersed within the tanks, were ~0.12 mg C L-1 and ~0.05 mg C L-1, respectively. Concentrations of the dried zooplankton diet were relatively high, but within the range of those reported for under-ice zooplankton biomass in the Antarctic (Table 1). The diatom concentrations were also high but within the range of those reported for phytoplankton in the fall and sea ice algae in the winter (Table 1). Particulate organic carbon (POC) was measured from seawater samples taken from each tank every 3 days to assess background food concentrations. Throughout the entire period, background POC ranged from 0.06 to 0.14 mg C L-1 in the MZA-NAT tank, 0.05 to 0.13 mg C L-1 in the MZA-MZA tank, 0.06 to 0.15 mg C L-1 in the DIA-NAT tank, and 0.05 to 0.14 mg C L-1 in the DIA-DIA tank.
Each morning, temperature, salinity, and dissolved oxygen (DO) measurements were made in each tank. During our study, seawater temperatures in the tanks ranged from a maximum of 1.9°C at the start of the experiment to a minimum of -0.5˚C from July onwards (Supplemental Figure 1A). Lowest weekly average temperatures were recorded in the final week of our experiment in September (-0.30˚C). Weekly average temperatures did not vary significantly between tanks (One-Way ANOVA, F(3,96) = 0.204, p = 0.893). Salinity increased gradually through the study period, from a low of 33.5 to a high of 35.2 PSU (Supplemental Figure 1B). Weekly average salinities did not vary significantly between tanks (One-Way ANOVA, F(3,96) = 0.209, p = 0.89). There was no trend in DO through the study and DO ranged from 9.16 to 12.11 mg L-1 (Supplemental Figure 1C). Weekly average DO did vary significantly between tanks (One-Way ANOVA, F(3,96) = 14.97, p< 0.001), however the differences were not sufficient to indicate oxygen depletion and were thus not biologically meaningful. Further, because the DO measurements were not consistent between tanks, much of the variability was likely from the sensor itself. The DO sensor membrane was replaced and recalibrated every 4 weeks.
Due to space limitations and logistic constraints, we were not able to replicate each FES. The option to keep krill in smaller 200-L tanks that could have been replicated was considered, however because we needed large numbers of krill for the suite of experiments and measurements made throughout the study, packing densities in the 200-L tanks would have exceeded 0.5 krill L-1. Krill are an aggregating, pelagic species and do not behave normally in confined spaces (Price et al., 1988). Also, because Antarctic krill often exist in swarms in their natural environment, we decided that maintaining high numbers of individuals in each tank throughout the study was important. We have chosen statistical analyses that are appropriate for unreplicated designs (Quinn and Keough, 2004) and have analyzed our data within these constraints (see section 2.8 Statistical analyses).
Throughout the course of our experiment, we removed a random selection of krill (60-120) from each tank at approximately 28-day intervals for a suite of experiments and measurements (numbers of krill removed are provided below for each experiment and measurement). All krill removed from each tank at each time point were measured for length (Standard Length 1, Mauchline, 1981) and either wet weight (WW, mg), dry weight (DW, mg), or both. For DW, individuals were oven-dried at 60˚C for at least 24 hours prior to weighing. For all weight measurements, each krill was measured individually. We used these data to develop regression equations to convert total length to DW (Supplemental Figure 2A) and WW (Supplemental Figure 2B), and to convert between WW and DW (Supplemental Figures 2C-D). In addition, we determined carbon biomass (B, mg) of a smaller subset of krill from each tank and time point and developed regression equations to convert DW to B (Supplemental Figure 2E). The particulate organic carbon (POC) content of krill was determined following the method of Goñi et al. (2005). Briefly, dried samples of whole krill were homogenized individually with a mortar and pestle and weighed into silver boats, which were exposed to hydrochloric acid fumes to remove any inorganic carbon. After drying the samples in an oven at 50°C for 24 hours to remove excess acid, the samples were combusted at 1000 ˚C in a Thermo Quest EA2500 Elemental Analyzer and the carbon dioxide produced by the combustion process was measured. Multiple standards of known carbon content and mass were used to develop multi-level daily calibrations that were then applied to calculate the amount of organic carbon in each of the krill samples and reported as weight percent carbon content.
Respiration rate experiments were run at approximately 28-day intervals. For each respiration experiment, 4 krill were randomly selected from each FES tank and placed into one of four 20-L buckets (corresponding to their FES) containing 0.2 µm-filtered (Whatman) particle-free (PF) seawater for 2-4 hours to empty their guts in the dark in a temperature-controlled room set at -0.5°C. Following this, each krill was placed into one of sixteen 500-mL clear, wide-mouth, Nalgene bottles containing ~200 mL of PF seawater. The Nalgene bottles were then filled completely by submerging fully in a bucket of PF seawater and tapping to remove any remaining air bubbles and were capped while submerged underwater. An additional 4 Nalgene bottles were filled with PF seawater to serve as controls. Each Nalgene bottle was outfitted inside with a Contactless Sensor Spot (PyroScience) for continuous measurement of oxygen concentrations using a FireStingO2 oxygen meter (PyroScience) as described in Bernard et al. (2018). Each FireStingO2 unit also had a temperature sensor that allowed for precise temperature compensation of oxygen measurements. Although the temperature-controlled room was set at -0.5°C, mean temperatures in the experimental units ranged from -1.09 to -1.23°C. Each set of respiration experiments were run 1-3 times at each time point, yielding a total of 4-12 individual measurements per month per FES.
Respiration experiments were run for 25-50 hours with measurements being recorded every second. The first ~3 hours were considered an acclimation period for the krill, and data collected within that timeframe were not included in determining respiration rates. Only the first 24 hours after the initial acclimation period were used to calculate respiration rates. Individual respiration rates (µl O2 ind-1 hr-1) were calculated from the slope of the linear regression of oxygen concentration over time (i.e., hour 3 to hour 27 of each experiment). At the end of each experiment, krill were removed from experimental chambers, their lengths were measured (Standard Length 1, Mauchline, 1981), and their wet weights (WW, mg) were obtained. A subset of individuals was subsequently dried at 60˚C for at least 24 hours and re-weighed to obtain their dry weight (DW, mg). The remaining individuals were used for other measurements requiring wet samples. For krill that were not dried we used the length to dry weight regression described above (Supplemental Figure 2A). Individual respiration rates were converted to DW-specific respiration rates (µl O2 (mg DW)-1 hr-1) for each krill.
Oxygen consumption rates were converted to mass of carbon respired (CR, mg C ind.-1 day-1) following the methods described by Ikeda et al. (2000):
Where RR is respiration rate (ml O2 ind.-1 hour-1), RQ is the molar ratio of carbon dioxide produced to oxygen consumed (a value of 0.97 is recommended by Ikeda et al., 2000), and 12/22.4 is the weight (12 grams) of carbon in 1 mol of carbon dioxide (22.4 liters is the molar volume constant). The product is multiplied by 24 to convert from hourly values to daily values. Note that CR can also be considered as the minimum carbon uptake (MCU) required to meet basal metabolic demands.
Percent loss of carbon biomass per day (CL, % carbon biomass, B in mg, day-1) was calculated as:
We ran a series of food removal experiments in July, August, and September to determine the effect of increasing food concentration on juvenile krill clearance, ingestion, egestion, and assimilation rates and daily rations. For each experiment, twenty krill were randomly collected from each FES tank and divided equally between two 20-L buckets simulating the correspondent feeding regime (Table 2). For each feeding regime, an extra 20-L bucket without krill was used as experimental control. Lids were placed on the buckets and buckets were placed in the dark in an environmental room set at -0.5°C to maintain ambient seawater temperature. The experiments ran for ~24 hours to encompass diel variability in both feeding rates and fecal pellet production. Clarke et al. (1988) recommend that egestion rates are measured over a 40-minute period because after 40 minutes rates reach an asymptote. However, their study was conducted on krill during the summer, when food availability and feeding rates are significantly higher. During our study, fecal pellet production was low and there was not sufficient fecal mass for carbon analysis after 12 hours, thus we found it necessary to increase the duration of our experiments to 24 hours. Each experiment was repeated 2-3 times in each of July, August, and September (Table 2).
At the end of each experiment, all krill were removed, measured for length (SL1), and weighed to obtain WW (mg). A subset of krill was then dried at 60°C for ~24 hours prior to being re-weighed to obtain DW (mg). The remaining individuals were used for other measurements requiring wet samples, such as lipid analysis. For krill that were not dried we used the length to dry weight regression determined for krill during our study (Supplemental Figure 2A). In addition, dry weight was converted to carbon mass (mg C) for each krill using the dry weight to carbon mass regression determined for krill during our study (Supplemental Figure 2E).
After krill were removed, a 2-L aliquot of seawater from each bucket was carefully siphoned from just beneath the surface to avoid stirring up the fecal pellets. These aliquots were then filtered through 25-mm GF/F filters, placed into 20-mL glass scintillation vials, and stored at -80°C for later analysis of particulate organic carbon (POC) at Oregon State University. In addition, a 500-mL aliquot of seawater was removed from the buckets that were supplemented with the diatom culture for chl-a analysis. We then siphoned off most of the remaining seawater and carefully pouring the rest into a watch glass. There, the pellets were rinsed several times with GF/F filtered seawater to remove any attached debris. The pellets were subsequently placed onto pre-ashed 25-mm GF/F filters and oven dried at 60°C for 24 hours prior to being re-weighed for DW. Filters and pellets were then placed into 20-mL glass scintillation vials and stored at -80°C for later POC analysis. Organic carbon contents of fecal pellets and food were determined following the methods of Goñi et al. (2005) described in section 2.3 above.
Clearance rates (F, L ind.-1 hour-1) were calculated as following Conover (1978) and Båmstedt et al. (2000):
Where V is volume (L), C0 is the initial food concentration (mg C L-1), Ct is the final food concentration (mg C L-1), n is the number of krill, t is the amount of time (hours) that the experiment ran for, and [C] is the average food concentration according to Conover (1978), calculated as:
Where g` is the coefficient defining the instantaneous change in food concentration, calculated as:
Daily ingestion rates (I, mg C ind.-1 day-1) were calculated according to Båmstedt et al. (2000) as:
Daily egestion rates (E) were measured in terms of carbon mass produced per day by an individual krill (mg C ind.-1 day-1). Assimilated carbon (A, mg C ind.-1 day-1) was calculated:
Daily ration (DR, % B day-1) of the food consumed by each krill was calculated as:
A subset of krill (6-9 individuals) from each tank and time point were used for total lipid content analyses. Lipid extraction and analysis was carried out in the laboratory of Dr. Louise Copeman (National Oceanic and Atmospheric Administration). Samples were homogenized and vortexed in chloroform and methanol and total lipids were extracted in a solution of chloroform:methanol:water (8:4:3) using a modified Folch procedure (Folch et al., 1956) developed by Parrish (1987). Lipid classes were determined using thin layer chromatography with flame ionization detection (TLC/FID) with a MARK VI Iatroscan (Iatron Laboratories, Tokyo, Japan) (Lu et al., 2008; Copeman et al., 2017). Total lipids were estimated by using the summation of the individual calibrated lipid classes (Parrish, 1987). Due to logistical constraints, total lipids were only measured in July, August, and September of our study period, thus our calculations of the utilization of stored lipids by juvenile krill is limited to the winter months only. The rates of stored lipid usage were calculated as the slope of the linear regression of total lipid content over time (in months). These values were then converted to the daily utilization of lipids in the form of carbon using the multiple linear regression presented in Fäber-Lorda et al. (2009):
Where C is carbon (mg ind.-1), L is total lipid content (mg ind.-1) and DW is dry weight (mg ind.-1).
Growth (G, mg C ind.-1 day-1) can be calculated according to Runge and Roff (2000) as:
Where A is assimilated carbon (mg C ind.-1 day-1), LC is lipid utilization (mg C ind.-1 day-1), and CR is respired carbon (mg C ind.-1 day-1). However, because it was not possible to quantify actual I (ingestion rates) and therefore A of krill in our experimental tanks, we have used I and A determined from the functional response feeding experiments (section 2.5 above), and thus we have estimated growth potential (Gp) across a range of possible food concentrations (0 to 600 mg C m-3). Because we found that RR, and therefore CR, varied significantly from month to month in our study (see Results), we have estimated Gp for each month. As RR, and therefore CR, vary with dry weight of an individual krill, we have estimated Gp for a juvenile krill with a dry weight of 33 mg, which is the mean dry weight of the krill used in our study. Juvenile krill smaller than our average individual size may have a different growth potential so we also calculated growth potential for juveniles of 20 mg dry weight.
After testing for normality and homogeneity of variance (sphericity) in our data and checking for and removing any extreme outliers, we used an Analysis of Variance (ANOVA) to test for significant differences in dry weight, body length, body carbon biomass, mean respiration rates between FES using time as a blocking factor, an approach recommended by Quinn and Keough (2004) for unreplicated two factor designs. After finding no significant differences in respiration rate with FES, we subsequently ran an ANOVA followed by Tukey HSD to test for significant differences in mean respiration rates over time. We compared the rate of decline in total body lipid content over time in each FES using Analysis of Covariance (ANCOVA) followed with a post-hoc simple main effects analysis. ANCOVA is an appropriate method to compare slopes of two or more regression lines (Quinn and Keough, 2004). The assumptions for an ANCOVA were tested prior to this; these included linearity between time and total lipid content at each FES level, homogeneity of regression slopes, normality, homoscedasticity, and no significant outliers. Functional responses in ingestion rates and daily rations to increasing food concentrations were determined using linear regression models. A second order polynomial model was used to describe the functional response of clearance rates to increasing food concentrations. Linear regression models were used to describe the functional response in egestion and assimilation rates to increasing ingestion rates. All tests were carried out in RStudio, 2022.07.1 (RStudio, 2022).
There were no significant differences in dry weight of juvenile krill used in our study either between FES (F(3,12) = 3.73, p = 0.42) or over time (F(4,12) = 1.136, p = 0.39). Body length did not vary significantly over time (F(4,12) = 1.168, p = 0.37). However, an ANOVA and post-hoc Tukey HSD test revealed significant differences in body length between COP-COP (mean = 29.50 mm, SD = 1.25 mm) and DIA-NAT (mean = 28.00 mm, SD = 0.51 mm; F(3,12) = 4.10, p = 0.04). While an intitial ANOVA revealed significant differences in body carbon between FES (F(3,12) = 3.691, p = 0.043), the post-hoc Tukey HSD test found no significant differences (p > 0.05 for all comparisons). Body carbon did not vary significantly over time (F(4,12) = 1.131, p = 0.39). Overall, mean body length was 28.41 mm (SD = 0.43 mm), mean dry weight was 33 mg (SD = 1.75 mg), and mean body carbon was 14.10 mg C (SD = 0.81 mg C).
There was no significant differences in respiration rates between FES with time as a blocking factor (F(3,12) = 1.459, p = 0.275). Respiration rates varied significantly across time points (F(4,15) = 12.76, p< 0.001) and a post-hoc Tukey HSD test revealed that respiration rates were initially low, averaging 0.318 [µL O2 (mg DW)-1 hour-1] and 0.274 [µL O2 (mg DW)-1 hour-1] in May and June, respectively. Following this, respiration rates increased significantly to 0.474 [µL O2 (mg DW)-1 hour-1] in July (p< 0.05), reaching a maximum of 0.486 [µL O2 (mg DW)-1 hour-1] in August, and then decreased in September to an average of 0.395 [µL O2 (mg DW)-1 hour-1] (Figure 2).
Figure 2. Monthly means for hourly dry weight standardized respiration rates [µL O2 (mg DW)-1 hour-1] for juvenile krill in our study. Error bars are standard deviation. Compact letter display indicates significant differences (p < 0.05).
Because of the statistically significant variability in respiration rates over time in our study, we analyzed the relationship between carbon mass respired (mg C ind.-1 day-1) and DW (mg) for each time point (Supplemental Figure 3). These monthly relationships were applied to our calculations of growth potential (see below). We have also analyzed the average winter relationship between carbon respired (mg C ind.-1 day-1) and DW (mg) by combining values from the entire study period to obtain a regression that could be applied in other studies (Figure 3A). During our study, the average percent loss of body carbon through respiration was highest for smallest juveniles (~10 mg DW) and decreased steeply for individuals between 10 mg and 50 mg in DW with little change for individuals with > 50 mg in DW (Figure 3B). Percent loss of body carbon through respiration observed in our study ranged between 0.4 and 2.8% day-1.
Figure 3. (A) Relationship between carbon respired (mg C ind.-1 day-1) and dry weight (mg) of juvenile krill during the fall and winter. The linear regression equation is provided, as are the adjusted r-squared and p-values. (B) Relationship between percent body carbon loss per day (% day-1) and dry weight (mg) of juvenile krill during the fall and winter. The non-linear regression equation is provided.
Clearance rates exhibited a second order polynomial response to increasing food concentrations (Figure 4A). Clearance rates increased from an average low of 0.43 mL (mg DW)-1 hour-1 at food concentrations of 80 mg C m-3 to a maximum average of 1.45 mL (mg DW)-1 hour-1 at food concentrations of 200 mg C m-3, and then subsequently declined to an average of 0.77 mL (mg DW)-1 hour-1 at food concentrations of 300 mg C m-3 (Figure 4A).
Figure 4. Functional responses of juvenile krill provided natural seawater assemblage (NAT), the addition of a freeze-dried mixed zooplankton assemblage (MZA), and the addition of cultured diatoms (DIA). (A) Relationship between clearance rate [F, mL (mg DW)-1 hour-1] and food concentration (mg C m-3). The second-order polynomial regression equation is provided, as are the adjusted r-squared and p-values. (B) Relationship between carbon ingested (I, mg C ind.-1 day-1) and food concentration (mg C L-1). The linear regression equation is provided, as are the adjusted r-squared and p-values. (C). Relationship between carbon egested (E, mg C ind.-1 day-1) and carbon ingested (I, mg C ind.-1 day-1). The linear regression equation is provided, as are the adjusted r-squared and p-values. (D) Relationship between carbon assimilated (A, mg C ind.-1 day-1) and carbon ingested (I, mg C ind.-1 day-1). The linear regression equation is provided, as are the adjusted r-squared and p-values. (E) Relationship between percent body carbon ingested, or daily ration (DR, % day-1) and food concentrations (mg C m-3). The linear regression equation is provided, as are the adjusted r-squared and p-values.
Ingestion rates increased significantly with food concentration from an average low of 0.03 mg C ind.-1 day-1 at 0.08 mg C L-1 (or 80 mg C m-3) to an average high of 0.3 mg C ind.-1 day-1 at 0.3 mg C L-1 (Figure 4B). There was no significant trend of carbon egested with carbon ingested (Figure 4C) and mean carbon egestion rates during our study were 0.039 mg C ind.-1 day-1. Assimilated carbon increased proportionately to ingested carbon (Figure 4D) reaching maximum values of 0.252 mg C ind.-1 day-1 at ingestion rates of 0.298 mg C ind.-1 day-1. Percent body carbon consumed increased from an average low of 0.34% at food concentrations of 80 mg C m-3 to an average high of 1.66% at food concentrations of 300 mg C m-3 (Figure 4E).
Utilization of lipid reserves by juvenile krill through the winter was calculated for each FES as the slope of the linear regression of total lipid content over time (Figure 5). In all but the MZA-MZA tank, stored lipid utilization from July to September was significant (Figure 5). We found that the rate of lipid utilization over time varied significantly by FES (ANCOVA; F(3,70) = 16.16; p< 0.001). A post-hoc Tukey HSD test revealed that lipid utilization by krill in the DIA-NAT tank (3.9% per month) was significantly greater than in any of the other three FES tanks (p< 0.05 for all three comparisons). Lipid utilization in krill in the MZA-MZA tank (0.95% per month) was not significantly different to that in the MZA-NAT tank (2.1% per month; p = 0.08). Krill in the DIA-DIA tank (3.4% per month) showed no significant differences in lipid utilization to krill in the MZA-NAT tank (p = 0.51), but lipid utilization by krill in the DIA-DIA tank was significantly higher than in the MZA-MZA tank (p< 0.05).
Figure 5. Total lipid content as a percentage of dry weight (mg) of juvenile krill in the four different feeding environment scenarios. (A) MZA-NAT = fed a mixed zooplankton assemblage for 2 months and then switched to natural unfiltered seawater for the remaining 2 months. (B) MZA-MZA = fed a mixed zooplankton assemblage for the full 4-month period. (C) DIA-NAT = fed cultured diatoms for 2 months and then switched to natural unfiltered seawater for the remaining 2 months. (D) DIA-DIA = fed cultured diatoms for the full 4-month period. Total lipid utilization is calculated as the slope of the linear regression of total lipid content over time (months).
Despite the significant difference in rate of lipid utilization over time between the four FES tanks, we used the average value of 2.6% DW to calculate the carbon equivalent of lipid utilization in the growth potential calculations (see below).
For a juvenile krill with a DW of 33 mg, our empirically derived algorithms suggest respiration rates ranging from a low of 8.7 µL O2 ind.-1 hour-1 in June to a high of 14.95 µL O2 ind.-1 hour-1 in July. This equates to respired carbon values ranging from 0.11 to 0.19 mg C ind.-1 day-1. Growth potential (Gp) was then calculated as the difference between the sum of assimilated carbon based on food concentrations ranging between 0 and 1 mg C L-1 (0 and 1000 mg C m-3) and the carbon equivalent of stored lipid utilization and carbon respired. Given the observed respiration rates during our study, a 33 mg DW juvenile krill would experience positive growth with minimal food availability (Figure 6A). In contrast, a smaller juvenile krill with a DW of 20 mg would need to encounter food concentrations of at least ~ 0.15 mg C L-1 to avoid loss of carbon biomass during the winter (Figure 6B).
Figure 6. Growth potential (Gp, mg C ind.-1 day-1) of (A) a 33 mg DW juvenile krill and (B) a 20 mg DW juvenile krill at a range of food concentrations (mg C L-1) from May to September. The horizontal dashed lines indicate zero growth, values above this represent growth, while values below represent shrinkage. The vertical dashed lines denote mark the point of zero growth and the corresponding food concentration in each month, colored accordingly.
Over a four-month period from mid-autumn through to late winter/early spring, we maintained juvenile krill under four different feeding environment scenarios (FES) and, at monthly intervals, assessed their physiology and condition. Our study has revealed new information about how juvenile Antarctic krill survive the winter months. We have found that juvenile krill adopt a mix of strategies used by larval stages and adults. Specifically, juvenile krill maintain relatively high respiration rates (in contrast to adults), respond positively to available food concentrations (in contrast to adults), and make use of lipid stores (in contrast to larvae). We discuss these findings and their implications for growth potential below.
Respiration rates of juvenile krill determined during our study were higher than what has been reported for adults (Atkinson et al., 2002) and within the range of those reported for juvenile krill in the autumn and winter at the Antarctic Peninsula and in the Lazarev Sea (Atkinson et al., 2002; Bernard et al., 2018). Unlike what has been observed for larval krill (Meyer et al., 2002), we observed an increase in respiration rate from autumn to winter, with a drop at the onset of spring. This was not anticipated but may reflect the energetic demand of early reproductive development (Nicol et al., 1995), because in a concurrent study we also observed a coincident increase in maturity of young female krill in July and August (Steinke et al., submitted). The decrease in respiration rate from August to September may be an energy conservation strategy prior to the onset of the growing season, though we do not have sufficient data to support this hypothesis here. Mass-specific respiration rates were highest for smallest individuals in our study and corresponded to the loss of body carbon through respiration of up to 2.8% day-1. This value does not account for the energetic costs of swimming against currents or of diel vertical migration, which can be considerable (Swadling et al., 2005).
We found that the metabolic demands of overwintering juvenile krill can be met through either the consumption of food or the utilization of stored lipid reserves. Below, we discuss these mechanisms in more detail.
Although we were not able to directly measure feeding rates (including clearance, ingestion, and assimilation) by krill in each of the FES tanks in our study due to logistical constraints, we did determine functional responses of juvenile krill feeding rates to food concentrations. Clearance rates for juvenile krill during our study followed a second-order polynomial fit in response to increasing food concentration, with an average value of 0.95 mL (mg DW)-1 hour-1, which is similar to what was observed for juvenile krill in autumn in the Lazarev Sea (Atkinson et al., 2002). Carbon ingestion rates by juvenile krill increased linearly with increasing food concentrations. And, because egestion rates showed no significant change with increasing food concentration, carbon assimilation rates of juvenile krill also responded significantly to increased food concentrations.
The ingestion rates recorded during our study equate to daily rations reaching up to nearly 2% body carbon. While this is low compared to daily rations in the summer months (Atkinson and Snÿder, 1997), it is close to the upper range of percent body carbon lost through respiration that we recorded during our study, 2.8% day-1. Our functional response slope for daily ration with increasing food concentration is also considerably higher than that reported in the autumn for juvenile krill in the Lazarev Sea (Atkinson et al., 2002). This may seem surprising because our average clearance rates were similar to those recorded by Atkinson et al. (2002). However, the average dry weight of juvenile krill used in their study was substantially higher, at 54 mg, than that measured in our study, at 33 mg. Growth and development of juvenile krill in the Lazarev Sea are delayed compared to those at the Antarctic Peninsula (Schmidt et al., 2014) and it is plausible that higher daily rations observed in our study could be in response to the associated elevated energetic demand. Alternatively, it is possible that more abundant food resources at the Antarctic Peninsula could promote earlier growth, in contrast to the low food environment of the Lazarev Sea.
Clearance rates, and therefore ingestion and daily rations, can be severely underestimated if experiments are run in small containers (Quetin et al., 1991). For instance, Price et al. (1988) found that clearance rates of krill were double what they were in large containers (50 L) than in small containers (5 L). And Quetin et al. (1988) reported clearance rates based on field ingestion rates that were even higher than those measured by Price et al. (1988) in the 50 L containers. We ran each of our ingestion/egestion rate experiments in 15 L of seawater in buckets. Therefore, it is likely that our estimates of feeding in response to food concentrations may be on the low end of the spectrum.
During the winter months of our study, juvenile krill made use of lipid reserves. We observed lipid utilization rates ranging from as low as 0.95% DW per month to as high as 3.9% DW per month. This use of lipid reserves by the juvenile krill in our study equated to approximately 0.53 mg C ind.-1 day-1. Lipid reserves are accumulated by juvenile krill during their first summer as post-larvae. In Antarctic krill, triacylglycerols (TAGs) serve as the primary storage lipids and are acquired through a carnivorous diet (Hagen et al., 2007; Schmidt et al., 2014). Indeed, krill can survive short-term starvation during winter by using stored TAGs (Hagen et al., 2007) and we observed significantly greater lipid utilization in those krill that were supplemented with the DIA diet compared to those supplemented with the MZA diet. In addition, those krill that were initially provided with MZA and then switched to NAT used less stored lipids than those provided with DIA the entire time. Thus, while a diet of diatoms is important for growth and development in juvenile krill (Ross et al., 2000; Pond et al., 2005; Atkinson et al., 2006), predation on other zooplankton, such as copepods, allows juvenile krill to build their lipid reserves for the winter months. A recent study found that omnivory was critical for juvenile Antarctic krill during summer in coastal waters off Anvers Island, Western Antarctic Peninsula (Conroy, 2022). That study showed the metazoans were the primary food source for juvenile krill, even in the summer when diatom concentrations are typically high. Their findings and our own suggest that carnivory plays a more important role in juvenile Antarctic krill survival than previously thought.
We calculated growth potential of a juvenile krill with an average dry weight of 33 mg and assumed that it would have used 0.59 mg C day-1 in lipids stores based on the observed rates of lipid utilization. Given the carbon respired by that individual, based on our monthly respiration rate measurements, we estimated the minimum food concentrations required to avoid negative growth during the winter as being negligible. However, without lipid reserves, an overwintering juvenile krill of 33 mg DW would need to encounter food concentrations of ~ 0.58 mg C L-1 to avoid body carbon loss through the winter. For juvenile krill < 35 mg DW, the potential for negative growth increases, and higher food concentrations are necessary to avoid loss of body carbon through the winter months, even if lipid stores are present. For instance, a 20 mg DW juvenile krill using ~ 0.39 mg C day-1 in lipid stores would need to encounter food at concentrations of ~ 0.15 mg C L-1 to avoid negative growth. Without sufficient lipid reserves, a 20 mg DW juvenile krill would need to encounter food concentrations of ~ 0.54 mg C L-1 during the winter. The supplemental food we provided krill during our study were at concentrations of ~ 0.05 mg C L-1 (DIA) and 0.12 mg C L-1 (MZA), which is at the low end of what would sufficiently meet their metabolic demands. However, that food in addition to the use of stored lipids, was likely sufficient to prevent large losses of body carbon mass in the krill. Indeed, the fact that young female krill used in our study initiated reproductive development in July and August (Steinke et al., submitted) demonstrates that growth potential was positive in those months.
Food concentrations on the order of ~ 0.05 mg C L-1 are not uncommon during the winter months along the Antarctic Peninsula (Ashjian et al., 2004; Espinasse et al., 2012; Vernet et al., 2012). However, higher concentrations at and above 0.15 mg C L-1 have only been observed associated with sea ice during the winter (Ashjian et al., 2004; Fritsen et al., 2008; Kramer et al., 2011; Wallis et al., 2016). Schaafsma et al. (2017) found that the stomachs of larval and juvenile krill in the winter were primarily filled with sea ice-associated species, including the centric diatom Actinocyclys actinochilus, the pennate diatom Fragilariopsis spp., dinoflagellate cysts, and the copepod Stephos longipes. Stable isotope analysis studies have also demonstrated that larval and juvenile krill feed predominantly on sea ice-associated prey during the winter (Jia et al., 2016; Kohlbach et al., 2017). Thus, in some regions and during years when sea ice is present in the winter, it is possible that juvenile krill may find sufficient food to sustain the metabolic requirements and avoid losing mass. Although losing mass during the winter months may not necessarily be lethal, juvenile krill that maintain their mass in the winter, or even grow, will be in a better position come spring.
Sea ice coverage along the Western Antarctic Peninsula (WAP) has declined significantly in the last 30 years (Stammerjohn et al., 2012), with a concomitant decrease in ice algae biomass (Fritsen et al., 2008) and, presumably, ice-associated biota, such as the copepods Stephos longipes and Paralabidocera antarctica that typically are less abundant as waters warm and sea ice retreats (Granata et al., 2022). It is thus likely crucial that juvenile krill feed carnivorously in the summer to accumulate storage lipids and reduce the need to feed as much in the winter. Recent studies using genetic and stable isotope approaches have demonstrated that the diet of Antarctic krill shifts from one that is primarily herbivorous in the spring, during the spring phytoplankton blooms (Cleary et al., 2018; Conroy, 2022), to one that is predominantly carnivorous in the summer and early autumn (Pauli et al., 2021; Conroy, 2022). The summer-autumn carnivorous diet is dominated by copepods, including Oithona spp., Metridia spp., and Calanus spp. (Pauli et al., 2021), which have become more abundant along the Western Antarctic Peninsula in the summer over the last 2 decades in response to sea ice decline in that region (Gleiber, 2014). Thus, the potential negative impacts of sea ice decline on the winter diet and ultimately physiology and condition of juvenile krill may be balanced by an increase in the summer abundances of their copepod prey.
However, extrapolating beyond this should be done with caution. It remains unclear how continued warming and the consequential changes in the quality of prey available to krill might affect their winter physiology and condition in the future. Regional variability in total lipid contents of Antarctic krill has been observed in the summer along the WAP, with significantly higher total lipid contents seen in krill in the southern WAP compared to the north (Ruck et al., 2014). The latitudinal climate gradient of the WAP, where the north is a warmer, wetter, more maritime environment in contrast to the colder, drier, more polar environment of the south, has been used by researchers as a proxy to understand past ecosystem changes and to predict future ones (e.g., Ducklow et al., 2013). Using this approach, one could postulate that the ability of juvenile Antarctic krill to accumulate stored lipids in the summertime might decrease with continued warming, i.e., follow the trends of the northern WAP. In addition to causing shifts in the community structure and quality of prey for krill, warmer winters may cause an increase in the metabolic rates of krill. This would increase the energy demand of krill during the winter, forcing them either to consume more during the winter or to build up greater lipid stores in the summer and autumn.
Juvenile Antarctic krill adopt a mix of strategies to survive the winter when food is scarce. By using stored lipid reserves (similarly to adult krill) and taking advantage of sporadic elevated food concentrations (similarly to larval krill), juvenile krill can maintain relatively high respiration rates (similarly to larval krill) without losing substantial carbon body mass. This approach allows juvenile krill to respond with flexibility to their environment. With warming along the WAP and the decrease in sea-ice-associated prey for krill, it is important that juvenile krill build lipid reserves they can draw on during periods of starvation in the winter by feeding carnivorously during the summer and autumn. Our study provides new information on the physiology, condition, and growth potential of juvenile Antarctic krill in the winter. Our findings also highlight the importance of carnivory in juvenile Antarctic krill. Our results can be used to improve population models for Antarctic krill as well as food web models for the Southern Ocean.
The raw data supporting the conclusions of this article will be made available by the authors, without undue reservation.
KB conceived of the research, acquired the funding, designed the experiment, conducted the field work, analysed data, and wrote the manuscript. KS conducted the field work, analysed data, and contributed to the manuscript. JF conducted the field work, analysed data, and contributed to the manuscript. All authors contributed to the article and approved the submitted version.
Funding for this study was provided by the United States National Science Foundation (NSF) Office for Polar Programs (OPP) as an Early Career Award. Grant number 1753101.
This work could not have been accomplished without the logistical support of the US Antarctic Program, the Antarctic Support Contractors at Palmer Station and aboard the ARSV Laurence M. Gould, and the Captain and crew of the ARSV Laurence M. Gould.
The authors declare that the research was conducted in the absence of any commercial or financial relationships that could be construed as a potential conflict of interest.
All claims expressed in this article are solely those of the authors and do not necessarily represent those of their affiliated organizations, or those of the publisher, the editors and the reviewers. Any product that may be evaluated in this article, or claim that may be made by its manufacturer, is not guaranteed or endorsed by the publisher.
The Supplementary Material for this article can be found online at: https://www.frontiersin.org/articles/10.3389/fmars.2022.990853/full#supplementary-material
Ashjian C. J., Rosenwaks G. A., Wiebe P. H., Davis C. S., Gallager S. M., Copley N. J., et al. (2004). Distribution of zooplankton on the continental shelf off Marguerite Bay, Antarctic Peninsula, during austral fall and winter 2001. Deep-Sea Res. II 51, 2073–2098. doi: 10.1016/j.dsr2.2004.07.025
Atkinson A., Meyer B., Stübing D., Hagen W., Schmidt K., Bathmann U. V. (2002). Feeding and energy budgets of Antarctic krill Euphausia superba at the onset of winter – II. juveniles and adults. Limnol Oceanogr 47 (4), 953–966. doi: 10.4319/lo.2002.47.4.0953
Atkinson A., Schmidt K., Fielding S., Kawaguchi S., Geissler P. A. (2012). Variable food absorption by Antarctic krill: Relationships between diet, egestion rate and the composition and sinking rates of their fecal pellets. Deep-Sea Res. II 59-60, 147–158. doi: 10.1016/j.dsr2.2011.06.008
Atkinson A., Shreeve R. S., Hirst A. G., Rothery P., Tarling G. A., Pond D. W., et al. (2006). Natural growth rates in Antarctic krill (Euphausia superba): II. predictive models based on food, temperature, body length, sex, and maturity stage. Limnol Oceanogr 51 (2), 973–987. doi: 10.4319/lo.2006.51.2.0973
Atkinson A., Siegel V., Pakhomov E. A., Jessopp M. J., Loeb V. (2009). A re-appraisal of the total biomass and annual production of Antarctic krill. Deep-Sea Res. I 56, 727–740. doi: 10.1016/j.dsr.2008.12.007
Atkinson A., Snÿder R. (1997). Krill-copepod interacations at south Georgia, Antarctica, i. omnivory by Euphausia superba. Mar. Ecol. Prog. Ser. 160, 63–76. doi: 10.3354/meps160063
Båmstedt U., Gifford D. J., Irigoien X., Atkinson A., Roman M. (2000). “Feeding,” in ICES zooplankton methodology manual. Eds. Harris R. P., Wiebe P. H., Lenz J., Skjodal H. R., Huntley M. (London: Academic Press), 297–399.
Bernard K. S., Gunther L. A., Mahaffey S. H., Qualls K. M., Sugla M., Saenz B. T., et al. (2018). The contribution of ice algae to the winter energy budget of juvenile Antarctic krill in years with contrasting sea ice conditions. Ices J. Mar. Sci. 76 (1), 206–216. doi: 10.1093/icesjms/fsy145
Clarke A., Morris D. J. (1983). Towards an energy budget for krill: The physiology and biochemistry of Euphausia superba Dana. Polar Biol. 2, 69–86. doi: 10.1007/BF00303172
Clarke A., Quetin L. B., Ross R. M. (1988). Laboratory and field estimates of the rate of faecal pellet production by Antarctic krill, Euphausia superba. Mar. Biol. 98, 557–563. doi: 10.1007/BF00391547
Cleary A. C., Durbin E. G., Casas M. C. (2018). Feeding by Antarctic krill Euphausia superba in the West Antarctic Peninsula: Differences between fjords and open waters. Mar. Ecol. Prog. Ser. 595, 39–54. doi: 10.3354/meps12568
Cleary A. C., Durbin E. G., Casas M. C., Zhou M. (2016). Winter distribution and size structure of Antarctic krill Euphausia superba populations in-shore along the West Antarctic Peninsula. Mar. Ecol. Prog. Ser. 552, 115–29. doi: 10.3354/meps11772
Conover R. J. (1978). “Transformation of organic matter,” in Marine ecology. Ed. Kinne O. (Chichester: John Wiley), 221–499.
Conroy J. A. (2022). diel, seasonal, and interannual changes in coastal Antarctic zooplankton community composition and trophic ecology (Williamsburg, VA, USA: College of William & Mary).
Constable A. J., Kawaguchi S., Plourde S. (2018). Modelling growth and reproduction of Antarctic krill, Euphausia superba, based on temperature, food and resource allocation amongst life history functions. Ices J. Mar. Sci. 75 (2), 738–750. doi: 10.1093/icesjms/fsx190
Copeman L. A., Laurel B. J., Spencer M., Sremba A. (2017). Temperature impacts on lipid allocation among juvenile gadid species at the pacific Arctic-Boreal interface: an experimental laboratory approach. Mar. Ecol. Prog. Ser. 566, 183–198. doi: 10.3354/meps12040
Daly K. L. (2004). Overwintering growth and development of larval Euphausia superba: An interannual comparison under varying enviornmental conditions west of the Antarctic Peninsula. Deep-Sea Res. II 51, 2139–2168. doi: 10.1016/j.dsr2.2004.07.010
Ducklow H. W., Fraser W. R., Meredith M. P., Stammerjohn S. E., Doney S. C., Martinson D. G., et al. (2013). West Antarctic Peninsula: An ice-dependent coastal marine ecosystem in transition. Oceanography 26 (3), 190–203. doi: 10.5670/oceanog.2013.62
Espinasse B., Zhou M., Zhu Y., Hazen E. L., Friedlaender A. S., Nowacek D. P., et al. (2012). Austral fall-winter transition of mesozooplankton assemblages and krill aggregations in an embayment west of the Antarctic Peninsula. Mar. Ecol. Prog. Ser. 452, 63–80. doi: 10.3354/meps09626
Fäber-Lorda J., Gaudy R., Mayzaud P. (2009). Elemental composition, biochemical composition and caloric value of Antarctic krill. Implications in energetics and carbon balances. J. Mar. Syst. 78, 518–524. doi: 10.1016/j.jmarsys.2008.12.021
Flores H., van Franeker J. A., Siegel V., Haraldsson M., Strass V., Meesters E. H., et al. (2012). The association of Antarctic krill Euphausia superba with the under-ice habitat. PloS One 7 (2), e31775. doi: 10.1371/journal.pone.0031775
Folch J., Less M., Sloane Stanley G. H. (1956). A simple method for the isolation and purification of total lipids from animal tissues. J. Biol. Chem. 22, 497–509. doi: 10.1083/jcb.1.2.173
Fritsen C. H., Memmott J., Stewart F. J. (2008). Inter-annual sea-ice dynamics and micro-algal biomass in winter pack ice of Marguerite Bay, Antarctica. Deep-Sea Res. II 55, 2059–2067. doi: 10.1016/j.dsr2.2008.04.034
Gleiber M. R. (2014). Long-term changes in copepod community structure in the Western Antarctic Peninsula: Linkage to climate and implications for carbon cycling (Gloucester Point, VA: The College of William and Mary).
Gleiber M. R., Steinberg D. K., Ducklow H. W. (2012). Time series of vertical flux of zooplankton fecal pellets on the continental shelf of the western Antarctic Peninsula. Mar. Ecol. Prog. Ser. 471, 23–36. doi: 10.3354/meps10021
Goñi M. A., Cathey M. W., Kim Y. H., Voulgaris G. (2005). Fluxes and sources of suspended organic matter in an estuarine turbidity maximum region during low discharge conditions. Estuar Coast. Shelf Sci. 63, 683–700. doi: 10.1016/j.ecss.2005.01.012
Granata A., Weldrick C. K., Bergamasco A., Saggiomo M., Grillo M., Bergamasco A., et al. (2022). Diversity in zooplankton and sympagic biota during a period of rapid Sea ice change in Terra Nova Bay, Ross Sea, Antarctica. Diversity 14 (6), 425–446. doi: 10.3390/d14060425
Hagen W. (2000). “Lipids,” in ICES zooplankton methodology manual. Eds. Harris R. P., Wiebe P. H., Lenz J., Skjodal H.-R., Huntley M. (London, UK: Academic Press), 113–147.
Hagen W., Kattner G., Terbrüggen A., Van Vleet E. S. (2001). Lipid metabolism of the Antarctic krill Euphausia superba and its ecological implications. Mar. Biol. 139, 95–104. doi: 10.1007/s002270000527
Hagen W., Yoshida T., Virtue P., Kawaguchi S., Swadling K. M., Nicol S., et al. (2007). Effets of a carnivorous diet on the lipids, fatty acids and condition of antartcic krill, Euphausia superba. Antarctic Sci. 19 (2), 183–188. doi: 10.1017/S0954102007000259
Hopkins T. L.. (1985). The zooplankton community of Croker Passage, Antarctic Peninsula. Polar Biology 4 (3), 161–70. doi: 10.1007/BF00263879
Ikeda T. (1985). Life history of Antarctic krill Euphausia superba: A new look from an experimental approach. Bull. Mar. Sci. 37, 599–608.
Ikeda T., Bruce B. (1986). Metabolic activity and elemental composition of krill and other zooplankton from Prydz Bay, Antarctica, during early summer (November-December). Mar. Biol. 92, 545–555. doi: 10.1007/BF00392514
Ikeda T., Torres J. J., Hernández-León S., Geiger S. P. (2000). “Metabolism,” in ICES zooplankton methodology manual. Eds. Harris R., Wiebe P., Lenz J., Skjodal H. R., Huntley M. (San Diego: Academic Press), 455–532.
Jia Z., Swadling K. M., Meiners K., Kawaguchi S., Virtue P. (2016). The zooplankton food web under East Antarctic pack ice - a stable isotope study. Deep-Sea Res. II 131, 189–202. doi: 10.1016/j.dsr2.2015.10.010
Kawaguchi K., Ishikawa S., Matsudo O. (1986). The overwintering strategy of Antarctic krill (Euphausia superba Dana) under the coastal fast ice off the Ongul Islands in Lützow-Holm Bay, Antarctica. Memoirs Natl. Instit Polar Res. Special Issue 44, 67–85.
Kawaguchi S., Nicol S. (2007). Learning about Antarctic krill from the fishery. Antarctic Sci. 19 (2), 219–230. doi: 10.1017/S0954102007000296
Kohlbach D., Lange B. A., Schaafsma F. L., David C., Vortkamp M., Graeve M., et al. (2017). Ice algae-produced carbon is critical for overwintering of Antarctic krill Euphausia superba. Front. Mar. Sci. 4, 310. doi: 10.3389/fmars.2017.00310
Kramer M., Swadling K. M., Meiners K. M., Kiko R., Scheltz A., Nicolaus M., et al. (2011). Antarctic Sympagic meiofauna in winter: Comparing diversity, abundance and biomass between perennially and seasonally ice-covered regions. Deep Sea Res. Part II: Topical Stud. Oceanogr 58 (9-10), 1062–1074. doi: 10.1016/j.dsr2.2010.10.029
Lu Y., Ludsin S. A., Fanslow D. L., Pothoven S. A. (2008). Comparison of three microquantity techniques for measuring total lipids in fish. Can. J. Fish. Aquat. Sci. 65, 2233–2241. doi: 10.1139/F08-135
Mauchline J. (1981). “Measurement of body length of Euphausia superba Dana,” in BIOMASS handbook no. 4. SCAR/SCOR/IABO/ACMRR, 9. Oban, United Kingdom.
Meyer B. (2012). The overwintering of Antarctic krill, Euphausia superba, from an ecophysiological perspective. Polar Biol. 35, 15–37. doi: 10.1007/s00300-011-1120-0
Meyer B., Atkinson A., Stübing D., Oettl B., Hagen W., Bathmann U. V. (2002). Feeding and energy budgets of Antarctic krill Euphausia superba at the onset of winter - i. furcilia III larvae. Limnol Oceanogr 47 (4), 943–952. doi: 10.4319/lo.2002.47.4.0943
Meyer B., Fuentes V., Guerra C., Schmidt K., Atkinson A., Spahić S., et al. (2009). Physiology, growth, and development of larval krill Euphausia superba in autumn and winter in the lazarev Sea, Antarctica. Limnol Oceanogr 54 (5), 1595–1614. doi: 10.4319/lo.2009.54.5.1595
Meyer B., Teschke M. (2016). “Physiology of Euphausia superba,” in Biology and ecology and Antarctic krill. Ed. Siegel V. (Switzerland: Springer), 145–174.
Moreau S., Ferreyra G. A., Mercier B., Lemarchand K., Lionard M., Roy S., et al (2010). Variability of the microbial community in the western Antarctic Peninsula from late fall to spring during a low ice cover year. Polar Biology 33 (12), 1599–1614. doi: 10.1007/s00300-010-0806-z
Nicol S., Clarke J., Romaine S. J., Kawaguchi S., Williams G., Hosie G. W. (2008). Krill (Euphausia superba) abundance and adélie penguin (Pygoscelis adeliae) breeding performance in the waters off the Béchervaise Island colony, East Antarctica in 2 years with contrasting ecological conditions. Deep-Sea Res. II 55, 540–557. doi: 10.1016/j.dsr2.2007.11.013
Nicol S., de la Mare W. K., Stolp M. (1995). The energetic cost of egg production in Antarctic krill (Euphausia superba Dana). Antarctic Sci. 7 (1), 25–30. doi: 10.1017/S0954102095000058
Nicol S., Foster J., Kawaguchi S. (2011). The fishery for Antarctic krill – recent developments. Fish Fisheries 13, 30–40. doi: 10.1111/j.1467-2979.2011.00406.x
Parrish C. C. (1987). Separation of Aquatic Lipid Classes by Chromarod Thin-Layer Chromatography with Measurement by latroscan Flame Ionization Detection. Can. J. Fish. Aquat. Sci. 44, 722–731.
Pauli N. C., Metfies K., Pakhomov E. A., Neuhaus S., Graeve M., Wenta P., et al. (2021). Selective feeding in southern ocean key grazers-diet composition of krill and salps. Commun. Biol. 4 (1), 1061. doi: 10.1038/s42003-021-02581-5
Pond D. W., Atkinson A., Shreeve R. S., Tarling G., Ward P. (2005). Diatom fatty acid biomarkers indicate recent growth rates in Antarctic krill. Limnol Oceanogr 50 (2), 732–736. doi: 10.4319/lo.2005.50.2.0732
Price H. J., Boyd K. R., Boyd C. M. (1988). Omnivorous feeding behavior of the Antarctic krill Euphausia superba. Mar. Biol. 97, 67–77. doi: 10.1007/BF00391246
Quetin L. B., Ross R. M. (1991). Behavioral and physiological characteristics of the Antarctic krill, Euphausia superba. Am. Zoologist 31, 49–63. doi: 10.1093/icb/31.1.49
Quetin L. B., Ross R. M., Amsler M. O. (1988). Field ingestion rates of Euphausia superba. EOS 68, 1785.
Quetin L. B., Ross R. M., Clarke A. (1991). “Krill energetics: seasonal and environmental aspects of the physiology of Euphausia superba,” in Southern ocean ecology: The BIOMASS perspectiveBIOMASS program. Ed. El-Sayed S. Z.(Cambridge, United Kingdom: Cambridge University Press).
Quetin L. B., Ross R. M., Frazer T. K., Amsler M. O., Wyatt-Evans C., Oakes S. A. (2003). Growth of larval krill, Euphausia superba, in fall and winter west of the Antarctic Peninsula. Mar. Biol. 143, 833–843. doi: 10.1007/s00227-003-1130-8
Quinn G. P., Keough M. J. (2004). Experimental design and data analysis for biologists (Cambridge, England: Cambridge University Press).
Reid K. (1995). The diet of Antarctic fur seals (Arctocephalus gazella Peters 1875) during winter at south Georgia. Antarctic Sci. 7 (3), 241–249. doi: 10.1017/S0954102095000344
Ross R. M., Quetin L. B. (1991). Ecological physiology of larval euphausiids, Euphausia superba (Euphausiaceae). Memoirs Queensland Museum 31, 321–333.
Ross R. M., Quetin L. B., Baker K. S., Vernet M., Smith R. C. (2000). Growth limitation in young Euphausia superba under field conditions. Limnol Oceanogr 45 (1), 31–43. doi: 10.4319/lo.2000.45.1.0031
Ross R. M., Quetin L. B., Newberger T., Oakes S. A. (2004). Growth and behavior of larval krill (Euphausia superba) under the ice in late winter 2001 west of the Antarctic Peninsula. Deep-Sea Res. II 51, 2169–2184. doi: 10.1016/j.dsr2.2004.07.001
Ruck K. E., Steinberg D. K., Canuel E. A. (2014). Regional differences in quality of krill and fish as prey along the Western Antarctic Peninsula. Mar. Ecol. Prog. Ser. 509, 39–55. doi: 10.3354/meps10868
Runge J. A., Roff J. C. (2000). “The measurement of growth and reproductive rates,” in ICES zooplankton methodology manual. Eds. Harris R. P., Wiebe P. H., Lenz J., Skjodal H. R., Huntley M. (London: Academic Press), 401–454.
Ryabov A. B., de Roos A. M., Meyer B., Kawaguchi K., Blasius B. (2017). Competition-induced starvation drives large-scale population cycles in Antarctic krill. Nat. Ecol. Evol. 1, 0177. doi: 10.1038/s41559-017-0177
Schaafsma F. L., Kohlbach D., David C., Lange B. A., Graeve M., Flores H., et al. (2017). Spatio-temporal variability in the winter diet of larval and juvenile Antarctic krill, Euphausia superba, in ice-covered waters. Mar. Ecol. Prog. Ser. 580, 101–115. doi: 10.3354/meps12309
Schmidt K., Atkinson A., Pond D. W., Ireland L. C. (2014). Feeding and overwintering of Antarctic krill across its major habitats: The role of sea ice cover, water depth, and phytoplankton abundance. Limnol Oceanogr 59 (1), 17–36. doi: 10.4319/lo.2014.59.1.0017
Stammerjohn S., Massom R., Rind D., Martinson D. (2012). Regions of rapid sea ice change: An inter-hemispheric seasonal comparison. Geophysical Res. Lett. 39, L06501. doi: 10.1029/2012GL050874
Swadling K. M., Ritz D. A., Nicol S., Osborn J. E., Gurney L. J. (2005). Respiration rate and cost of swimming for Antarctic krill, Euphausia superba, in large groups in the laboratory. Mar. Biol. 146, 1169–1175. doi: 10.1007/s00227-004-1519-z
Tarling G. A., Hill S., Peat H., Fielding S., Reiss C., Atkinson A. (2016). Growth and shrinkage in Antarctic krill Euphausia superba is sex dependent. Mar. Ecol. Prog. Ser. 547, 61–78. doi: 10.3354/meps11634
Tarling G. A., Shreeve R. S., Hirst A. G., Atkinson A., Pond D. W., Murphy E. J., et al. (2006). Natural growth rates in Antarctic krill (Euphausia superba): I. improving methodology and predicting intermolt period. Limnol Oceanogr 51 (2), 959–972. doi: 10.4319/lo.2006.51.2.0959
Vernet M., Kozlowski W. A., Yarmey L. R., Lowe A. T., Ross R. M., Quetin L. B., et al. (2012). Primary production throughout austral fall, during a time of decreasing daylength in the western Antarctic Peninsula. Mar. Ecol. Prog. Ser. 452, 45–61. doi: 10.3354/meps09704
Wallis J. R., Swadling K. M., Everett J. D., Suthers I. M., Jones H. J., Buchanan P. J., et al. (2016). Zooplankton abundance and biomass size spectra in the East Antarctic sea-ice zone during the winter–spring transition. Deep Sea Res. Part II: Topical Stud. Oceanogr 131, 170–181. doi: 10.1016/j.dsr2.2015.10.002
Keywords: Antarctic krill, Euphausia superba, winter, energy budget, physiology, condition, growth potential
Citation: Bernard KS, Steinke KB and Fontana JM (2022) Winter condition, physiology, and growth potential of juvenile Antarctic krill. Front. Mar. Sci. 9:990853. doi: 10.3389/fmars.2022.990853
Received: 10 July 2022; Accepted: 06 September 2022;
Published: 23 September 2022.
Edited by:
Sang Heon Lee, Pusan National University, South KoreaReviewed by:
Sílvia Lourenço, Instituto Politécnico de Leiria, PortugalCopyright © 2022 Bernard, Steinke and Fontana. This is an open-access article distributed under the terms of the Creative Commons Attribution License (CC BY). The use, distribution or reproduction in other forums is permitted, provided the original author(s) and the copyright owner(s) are credited and that the original publication in this journal is cited, in accordance with accepted academic practice. No use, distribution or reproduction is permitted which does not comply with these terms.
*Correspondence: Kim S. Bernard, a2ltLmJlcm5hcmRAb3JlZ29uc3RhdGUuZWR1
Disclaimer: All claims expressed in this article are solely those of the authors and do not necessarily represent those of their affiliated organizations, or those of the publisher, the editors and the reviewers. Any product that may be evaluated in this article or claim that may be made by its manufacturer is not guaranteed or endorsed by the publisher.
Research integrity at Frontiers
Learn more about the work of our research integrity team to safeguard the quality of each article we publish.