- 1Yellow Sea Fisheries Research Institute, Chinese Academy of Fishery Sciences, Qingdao, China
- 2Laboratory for Marine Ecology and Environmental Science, Qingdao National Laboratory for Marine Science and Technology, Qingdao, China
Metallic nanoparticles (NPs) are increasingly being used and inevitably entering the marine environment. Therefore, the adverse effects of NPs on individual marine species have attracted increasing attentions. However, to date, the ecological risks of NPs on the marine ecosystem remain poorly understood. In this study, species sensitivity distributions (SSDs) were constructed for Ag, ZnO, CuO, and TiO2 NPs to understand their ecotoxicity to the marine ecosystem and the contribution of size effect and ion effect to the nanotoxicity. Furthermore, a case study on the assessment of marine ecological risk and marine environment carrying capacity of metallic NPs was performed in Jiaozhou Bay, China. The results showed the toxicity of metallic NPs to marine species following the order of Ag > ZnO > CuO > TiO2. Both size effect and ion effect contributed to the toxicity of NPs. Environmental concentrations of Ag, ZnO, CuO, and TiO2 NPs are much lower than the predicted no-effect concentrations, indicating that these metallic NPs have not yet posed risks to the marine ecosystem based on currently limited measured environmental concentrations of metallic NPs in seawater. And the marine environment carrying capacity of Ag, ZnO, CuO, and TiO2 NPs in Jiaozhou Bay seawater were determined to be 5.03, 8.72, 93.06, and 629.79 t, respectively. The results help us understand the ecological risk of NPs in marine environments and provide a scientific basis for the sustainable development of nanotechnology.
Introduction
With the rapid development of nanotechnology, the number of products containing engineered nanoparticles (NPs) has been rapidly increased in the past decades (Zhao et al., 2020). Among these nano-products, metallic NPs such as Ag, CuO, ZnO, and TiO2 NPs are the very commonly used NPs for these products. These NPs will inevitably release from products into various environments during the production, consumption, and disposal of the nano-products (Zheng and Nowack, 2021). Because the ocean is the ultimate sink for NPs (Klaine et al., 2008), concerns are increasing about the possible risks posed by NPs to marine environments and especially to marine organisms. An increasing number of studies have reported that the metallic NPs caused significant toxicity to marine organisms, including marine bacteria (Rotini et al., 2017; Schiavo et al., 2018), algae (Xia et al., 2015; Fazelian et al., 2019; Thiagarajan et al., 2019), copepods (Rotini et al., 2018; Farkas et al., 2020), crustaceans (Rotini et al., 2018; Prato et al., 2021), echinoderms (Rotini et al., 2018; Prato et al., 2021), and mollusks (Libralato et al., 2013; Zha et al., 2019; Zha et al., 2022), with a 50% lethal/effect concentration (LC50/EC50) varied widely from 0.001–116.981 mg/L.
The species sensitivity distributions (SSDs) have been developed to evaluate the potential environmental risks of NPs based on the toxic endpoints of acute x% lethal/effective/inhibiting concentration (LCx/ECx/ICx) or chronic no observed effect concentration (NOEC) from laboratory toxicity tests (Gottschalk and Nowack, 2013). SSDs rank the species based on their sensitivity to a certain toxicant and fit a statistical distribution function to the proportion of species affected as a function of exposure concentration of this substance (Garner et al., 2015). This model allows extrapolating from limited single-species toxicity testing to effects at the ecosystem level regarding the chemicals. SSDs can estimate the hazard concentration of 5% (HC5), which indicated that 95% of the species are not expected to be affected. The predicted no-effect concentration (PNEC), which is supposed to be the concentration sufficient to protect the ecosystems and is commonly used as the threshold in environmental risk assessment, can be derived from the HC5 value of each SSD by adding a safety factor that ranges between 1 and 5. Risk assessment of NPs is further performed by comparing the environmental concentrations of pollutants with the calculated PNEC (ECHA, 2008a).
Several SSDs have been built for multiple nanomaterials under different environmental compartments (Adam et al., 2015; Garner et al., 2015). For instance, SSDs were established for the TiO2, Ag, ZnO, CNT, and fullerenes nanomaterials in freshwater, soils, and sediments (Coll et al., 2016). SSDs were also generated for metallic NPs (Ag, CeO2, CuO, TiO2, and ZnO NPs) considering the characteristics of nanomaterials, experimental conditions, and different types of endpoints in aquatic environments (Chen et al., 2018). In addition, Zhao et al. (2020) reported the SSDs of TiO2, Ag, CuO and graphene nanomaterials in aquatic environments. However, these researches mainly focused on the environmental risks of NPs to a vast majority of freshwater organisms. Very few data associated with marine species were included in the SSDs for nanomaterials. Due to the differences between freshwater and saltwater species’ sensitivities to pollutants, it would be not quite appropriate for using freshwater toxicity data to extrapolate saltwater effects (Wheeler et al., 2002). Thus, there is still no consensus on the ecological risk of nanomaterials in marine environments, and SSDs derived from saltwater ecotoxicity data is needed to fill this gap.
The marine environment carrying capacity (MECC) was defined as the maximum pollutant load that the marine environment can accept without destructing water quality (GESAMP, 1986). The assessment of MECC has been applied in marine environmental protection and contamination control, and is useful for maintaining the sustainable development of the economy-marine environment (Wang et al., 2018). There has been intensive research on MECC, which mainly focused on conventional pollutants such as ammonium, phosphate, total suspended solids, and biological oxygen (Liao et al., 2013; Bui and Tran, 2022). Nonetheless, it still requires the development of evaluation methods on emerging contaminants such as NPs. Once the amount of NPs exceeds the MECC, ecological risks will occur on account of the imbalances between marine environment safety and nano-industry development. Therefore, it is essential to study the carrying capacity of marine environments for metallic NPs.
This study aims to collect currently published marine ecotoxicity data of metallic NPs (i.e. Ag, CuO, ZnO, and TiO2 NPs) and construct SSDs for NPs to better understand their marine ecological risks. Considering that not only responses to individual NPs are reflected in these SSDs, SSDs for the corresponding bulk or ionic form of metallics were also built and thus the impact of size effect and ion effect on the toxicity was estimated. A case study of the marine ecological risk assessment for these metallic NPs was performed in Jiaozhou Bay, China by employing a risk characterization ratio (RCR). Subsequently, the seawater carrying capacity (SECC) of metallic NPs was also calculated in Jiaozhou Bay. This work will improve our understanding of the ecological risk of metallic NPs to marine environments. To our knowledge, this is the first report on the risk assessment of NPs in marine ecosystems.
Materials and methods
Data collection
Toxicity data of the Ag, CuO, ZnO, and TiO2 NPs, as well as their bulk form and ion, on marine organisms, were collected from published literature. The scientific websites, Web of Science, were searched using the keywords “nanoparticles OR bulk OR ion AND marine AND toxicity”. All data published before June 2022 were taken into account. Publications were retained according to the following criteria: only effects on survival, growth and reproduction were considered, and the endpoints including NOEC, the highest observed no-effect concentration (HONEC), lowest observed effect concentration (LOEC), LCx, ICx, and ECx were collected (Coll et al., 2016; Chen et al., 2018). For SSD building, these toxicity data were transformed by two different assessment factors (AFs): 1) to convert the observed effect to NOEC with the AFno-effect (factor 10 was used for LC50/EC50, factor 2 for LOEC and factor 1 for NOEC and HONEC values); 2) to extrapolate from acute data to chronic data with the AFtime (factor 10 was assigned to short-term studies and factor 1 to long-term studies) (Coll et al., 2016).
In order to evaluate the risk of metallic NPs in the marine environment, the environmental concentrations of various NPs in seawater were also collected from published data. Published studies were selected via a search of Web of Science on June 2022, using the keywords “nanoparticles AND concentration AND marine OR seawater”. Only studies containing measured environmental concentrations of NPs in seawater were retained.
SSD modeling
For each kind of NPs and their bulk or ionic counterparts, the converted data were ranked from lowest to highest according to the equation of USEPA (1998):
SSDs were generated using an optimal log-normal distribution, which was fitted by the ‘fitdistr’ function and ‘quantile’ function in the R statistical software (version 4.2.0) (R Core Team, 2022). For each SSD curve, the log10-transformed NOEC value of different species was plotted against the cumulative probability which reflects the proportion of species affected at a certain concentration. HC5, i.e. the concentrations corresponding to the 5th percentile of the distribution of each SSD were extracted and then used as PNEC value according to the recommendation by the European Chemicals Agency (ECHA, 2008b). The 95% confidence interval of the fitted regressions was also estimated with a parametric bootstrap procedure.
Risk assessment
To assess the ecological risks that metal-based NPs might pose to the marine environment, the RCR was calculated as follows (ECHA, 2008a):
where RCR represents as the risk characterization ratio of NPs; EC represents as the environmental concentration of NPs in seawater (μg/L); and PNEC represents as predicted no-effect concentration of NPs for marine species (μg/L). If the RCR ≤ 0.1, a low risk is expected for marine species at particular environmental concentrations of NPs; if the 0.1< RCR< 1.0, a moderate risk is expected; if the RCR ≥ 1.0, a high risk is expected (Umbría-Salinas et al., 2021).
Environment carrying capacity
The SECC for metallic NPs was evaluated based on marine environment static capacity, which is the theoretically maximum load of metallic NPs that the sea can withstand before an unacceptable ecological risk occurs. The SECC was calculated by a simplified model as follows:
where SECC represents as environment carrying capacity of seawater (t); S represents as sea area (km2); D represents as mean water depth (m); PNEC represents as the predicted no-effect concentrations for NPs (μg/L); and EC represents as the environmental concentration of NPs in seawater (μg/L).
Results and discussion
Data availability for generating SSDs
After data evaluation, the final dataset for marine risk assessment included 107 records retrieved from 40 publications. The toxicity endpoints that are available for developing SSDs after transformation include LC50, EC50, IC50, NOEC, and HONEC. It seems that the data availability is very limited due to the lack of thorough toxicity tests concerning NPs to marine species (Chen et al., 2018). SSDs were built for Ag NPs (10 data points), Ag+ (8 data points), ZnO NPs (14 data points), bulk ZnO (9 data points), Zn2+ (12 data points), CuO NPs (10 data points), bulk CuO (3 data points), Cu2+ (8 data points), TiO2 NPs (24 data points), and bulk TiO2 (9 data points). The source data are provided in Supplemental Information (Table S1–S10).
In fact, Ag, CuO, ZnO, and TiO2 NPs have the highest yield compared with other metallic NPs (Bondarenko et al., 2013) and therefore have received the most research attention (Wang et al., 2016; Schiavo et al., 2018; Han et al., 2019; Shi et al., 2020; Dong et al., 2021). Considering that these metallic NPs are inevitably entering the marine environment and potentially pose impacts on marine species, the marine risk assessment of the NPs should be performed and clarified if these mass-produced metallic NPs are safe. One of the well-established methods to control the risks brought by nanoproducts is the development of SSDs (Gottschalk and Nowack, 2013). This model needs extensive toxicity evaluations to be tested on a wider range of nanomaterials, and also more species from diverse taxa and trophic levels in order to minimize the uncertainty in the predicted results (Chen et al., 2018). Additionally, the SSD methodology required a minimum number of data to construct an SSD to maintain the model reliability. USEPA recommended that no less than eight species should be used for modeling (USEPA, 1998). Moreover, Cedergreen et al. (2004) proposed that the range of the species number should be from six to eight, while Garner et al. (2015) suggested that a minimum of four species should be covered in an SSD. Excluding bulk CuO, at least six marine species were included in each SSDs in this study.
SSDs of metallic nanoparticles
The SSDs for Ag, CuO, ZnO, and TiO2 NPs in seawater are shown in Figure 1. The individual species NOECs calculated from the endpoints reported in toxicity studies were exhibited as points. Ag NPs showed toxicity towards different taxonomic groups, including cnidarian, arthropod, and algae; and ZnO NPs showed toxicity towards to amphipod, algae, bacteria, and mollusk. CuO NPs displayed toxicity to different species of mollusk, arthropod, rotifer, bacteria, and algae. While TiO2 NPs were toxic to arthropod, mollusk, algae, and rotifer. Diverse taxonomic groups showed large variation in the sensitivities to NP toxicity and there is no clear pattern of species distribution among different NPs. These SSD curves indicated that Ag and ZnO NPs were toxic to most species at the level of μg/L, while TiO2 NPs showed toxicity to the majority of species at mg/L level.
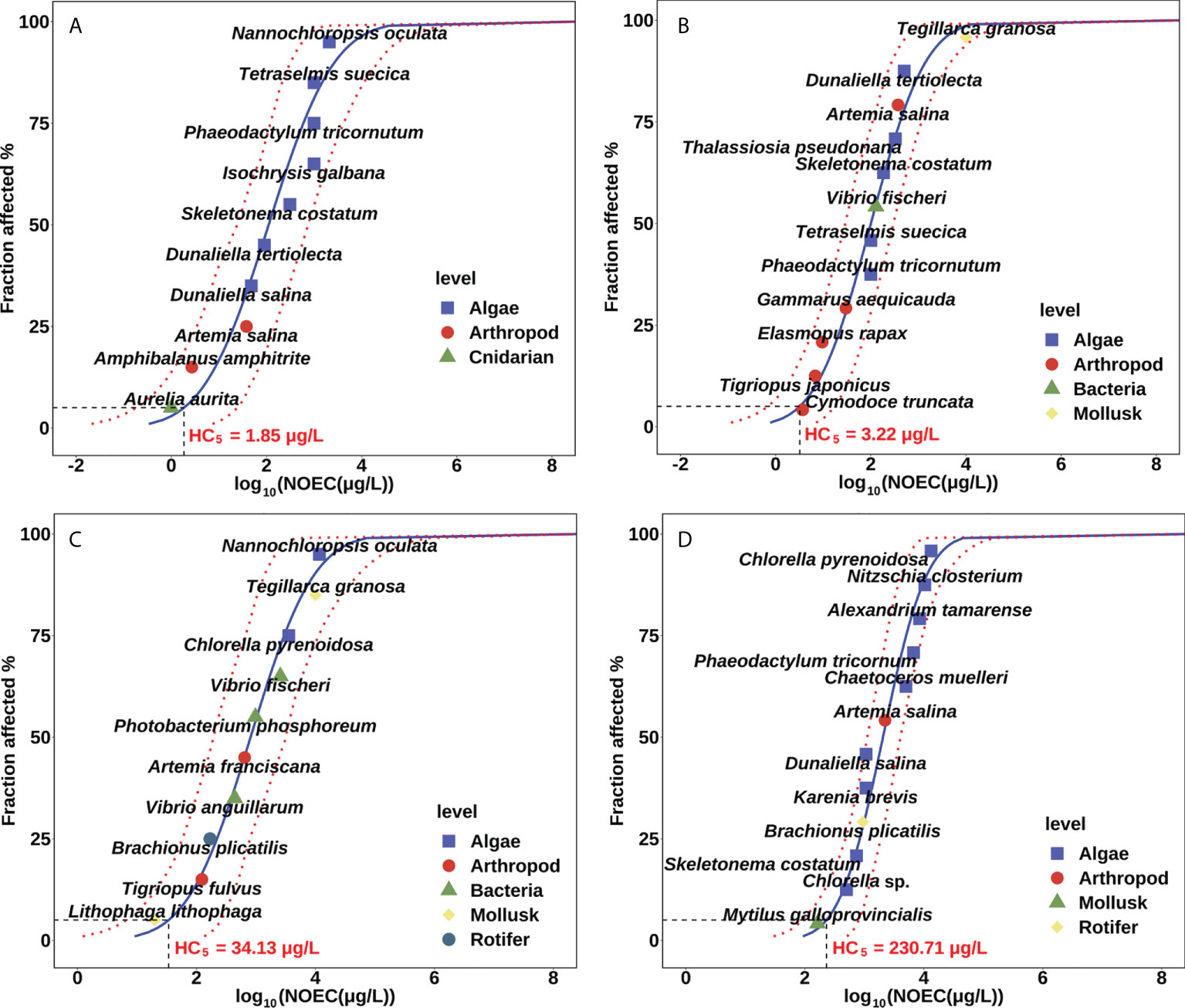
Figure 1 Marine species sensitivity distribution of (A) Ag, (B) ZnO, (C) CuO, and (D) TiO2 nanoparticles.
The PNEC distributions for marine species were derived from the 5th percentiles of the SSD curves. The derived PNEC of Ag NPs is 1.85 μg/L, with the 95% confidence interval ranging from 0.27 to 22.19 μg/L. And the PNEC of ZnO, CuO, and TiO2 NPs is 3.22 μg/L (95% confidence interval: 0.55−18.23 μg/L), 34.13 μg/L (7.47−219.46 μg/L), and 230.71 μg/L (83.89−817.21 μg/L), respectively. The PNEC of NPs follows the order of Ag< ZnO< CuO< TiO2, suggesting the highest toxicity for Ag NPs, and the lowest toxicity for TiO2 NPs in marine environments. For a freshwater dataset, Coll et al. (2016) inferred the PNECs from the most sensitive to least sensitive in the following order: Ag (0.017 μg/L)< ZnO (1.0 μg/L)< TiO2 (15.7 μg/L). Zhao et al. (2020) also reported the PNECs mainly derived from freshwater organisms in the order of Ag (0.0485 μg/L)< CuO (9.06 μg/L)< TiO2 (192 μg/L). The results of this study were consistent with previous research in the toxicity order of NPs. However, the reduced toxicity of NPs was observed in seawater environment compared with the freshwater environment.
From the toxicity comparison of nanomaterials for single species, it was concluded that Ag NPs are generally considered to have high biological toxicity, while TiO2 NPs are generally considered to be less toxic or non-toxic (Rekulapally et al., 2019; Geppert et al., 2021). Nanoparticles can be classified as highly insoluble materials such as TiO2 NPs, and slightly soluble materials such as Ag, ZnO, and CuO NPs (Brunner et al., 2006). The toxicity of insoluble NPs is mainly due to the size effect, which leads to physical damage, NP internalization, and oxidative stress to the organisms (Xia et al., 2015); whereas the toxicity of soluble NPs could be explained not only by the size-dependent effect but also by the release of noxious metal ions (Jassim et al., 2022; Wu et al., 2022). Overall, the characteristics of NPs in the aqueous system (e.g. chemical composition, solubility, or aggregation) are considered to be important factors that affect NP toxicity (Vicario-Parés et al., 2014; Duffy et al., 2018). Moreover, the unique conditions of the marine environment (e.g. pH, ionic strength, presence of organic matter) can alter the environmental behavior of NPs and thus reduce their biotoxicity (Baalousha, 2017). This might explain why above NPs appear less toxic in seawater than in freshwater. To elucidate the origins underlying the toxicity of NPs, the impact of size effect and ion effect on NP toxicity were estimated in the following study.
SSDs of bulk materials
To gain insight into the size effect of NPs, SSDs of bulk ZnO and CuO were generated (Figure 2) and the effect of size on NP toxicity was analyzed. The SSD curve indicated that bulk ZnO was toxic to most species at the concentration range of 101–104 μg/L. The derived PNEC of bulk ZnO was 42.95 μg/L (9.86–233.94 μg/L), which was a dozen times higher than that of ZnO NPs, suggesting that bulk ZnO had relatively lower toxicity than the NPs. Similarly, the derived PNEC of bulk TiO2 was 340.32 μg/L (109.10–1224.98 μg/L), suggesting a decline in TiO2 toxicity with increasing particle size. For bulk CuO, the fitting result showed that the PNEC of bulk CuO was more than 1500 μg/L. However, it limited the understanding of the toxicity of bulk CuO due to insufficient available data on bulk CuO. Too little data may lead to a reduction in the goodness of fit and an increase in the uncertainty (ECB, 2003).
Nanoscale particles can exhibit special characteristics and high reactivity because of the larger proportion of atoms or molecules and the numbers of reactive groups distributed on their surface (Nel et al., 2006). Therefore, the environmental and health risks of NPs have attracted widespread concerns and some studies have revealed the adverse effects of NPs on marine organisms (Qian et al., 2020; Du et al., 2021; Zhu et al., 2022). It is generally considered that the toxicity of NPs is size-dependent, which means that smaller NPs are more toxic than larger ones. For example, our previous study found that the toxicity of TiO2 particles to marine microalga Nitzschia closterium was negatively correlated with particle size, which was proved by the algal growth inhibition effects (96 h-EC50: 88.78 mg/L (21 nm)< 118.80 mg/L (60 nm)< 179.05 mg/L (400 nm)) (Xia et al., 2015). Similarly, Ates et al. (2013) found that ZnO NPs (10–30 nm) caused higher toxicity to brine shrimp (Artemia salina) larvae than bulk ZnO (200 nm). One of the main reasons is that the cellular uptake of NPs would be affected by particle size, as nanoscale particles are more liable to internalize into cells through penetration, diffusion, or endocytosis (Liu and Tang, 2020). Previous studies confirmed that the internalization of NPs was closely related to oxidative stress, physical injury, and other cytotoxicity (Zhao et al., 2016). Besides, the “Trojan horse” effect maybe also contribute to the toxicity of metallic NPs with the ability to release ions, which means NPs could enter the cell and then start releasing toxic ions (Wang and Wang, 2014; Moreno-Garrido et al., 2015). Although the aggregation of NPs was observed in seawater, which was driven by the high ionic strength, there are still some particles that remain at the nanoscale and exhibit the nano-size effect (Xia et al., 2018; Zhu et al., 2022).
SSDs of metal ions
In this study, SSDs of metal ion were also built in order to understand the ion effect of metallic NPs (Figure 3). The derived PNEC of Ag+, Zn2+ and Cu2+ was 0.62 μg/L (0.17−5.53 μg/L), 2.65 μg/L (0.70−17.24 μg/L) and 0.55 μg/L (0.11−3.43 μg/L), respectively, which was relatively lower than the corresponding NPs, reflecting the major contribution of metal ions to the toxicity of NPs.
Indeed, there is still an open debate on the causation of NP toxicity, and it remains unclear whether the toxicity of NPs could be completely explained by the ion release or not (Moreno-Garrido et al., 2015). For example, Miao et al. (2009) attributed the toxicity of Ag NPs to marine diatom Thalassiosira weissflogii to the leached Ag+. However, Šiller et al. (2013) observed that the toxicity of Ag NPs to sea urchin Paracentrotus lividus were more toxic than that of equivalent Ag+ dose, implying that the contribution of nanoparticle effect to the observed toxicity cannot be ignored. Sun et al. (2017) further calculated the relative contributions of particles and ions to the overall toxicity of CuO NPs were 62.07% and 37.93%, respectively, indicating that size-effect is the major toxic source of CuO NPs to the marine scallop (Chlamys farreri) hemocytes. These independent studies only covered single species, and the sensitivity inherent to different species varies greatly (Rotini et al., 2018; Thiagarajan et al., 2019). In this study, the model results demonstrated that the toxicity of NPs is relatively lower than that of corresponding metal ions, which may be caused by the incomplete dissolution of NPs in seawater. It is necessary to note that NPs are inclined to aggregate in the marine environment, and ions dissolved from NPs show an inverse relationship with the size of the aggregates (He et al., 2013). Thus, from the angles of the response from the ecosystem, the statistical results of this study supported the opinion that the toxic effects of nanomaterials derived from the combined effect of nano-size effect and ion effect.
Ecological risks of metallic nanoparticles
To further perform the risk assessment, the environmental concentrations of metallic NPs were collected from published literature that were conducted by field measurement. However, limited information is available on the environmental concentrations of metallic NPs in marine environments. Due to the limitation of detection technology, only one study reported the actual concentrations of NPs in seawater all over the world (Xu et al., 2020). And the particle concentrations of Ag, Zn, Cu and Ti bearing NPs in surface seawater of Jiaozhou Bay, China were 0.54×107, 0.46×107, 0.79×107, and 0.65×107 particles/mL, respectively, with their mean sizes in seawater were 30.5, 126.2, 117.4, and 105.2 nm, respectively (Xu et al., 2020). We then assumed the measured NPs were all Ag, CuO, ZnO and TiO2 NPs and speculated the mass concentrations of these NPs were 8.41×10-3, 2.71×10-2, 4.22×10-2, and 1.68×10-2 μg/L, respectively, based on their particle concentrations, mean size and density (Table S11).
RCRs, the classic risk assessment index, were calculated by comparing environmental concentrations with PNECs (Coll et al., 2016; Adam et al., 2021). For all the four NPs in seawater, RCR values were lower than 0.1 (Figure 4A), indicating the low risk of metallic NPs in the current marine environments. This result was similar to those RCRs reported in surface waters (Musee, 2011; Kjølholt et al., 2015; Coll et al., 2016), except for one research evaluated the RCRs of Ag NPs in the Europe surface waters were slightly higher than 1 (Gottschalk et al., 2009). In addition, some special exposure scenarios, such as sewage treatment plant effluents discharged directly into the sea, should be paid more attention to because they would carry higher concentrations of NPs and thus lead to elevated environmental risks (Gottschalk et al., 2009; Kjølholt et al., 2015). Furthermore, with the exponential increase in the production of nano-products (Robichaud et al., 2009), more and more NPs will inevitably release into the marine environment over the life-cycle of nano-products (Zheng and Nowack, 2021). Thus, the concentrations of NPs in the marine environments are expected to be further increased and more environmental concentrations of NPs in the marine environment are strongly needed to be determined.
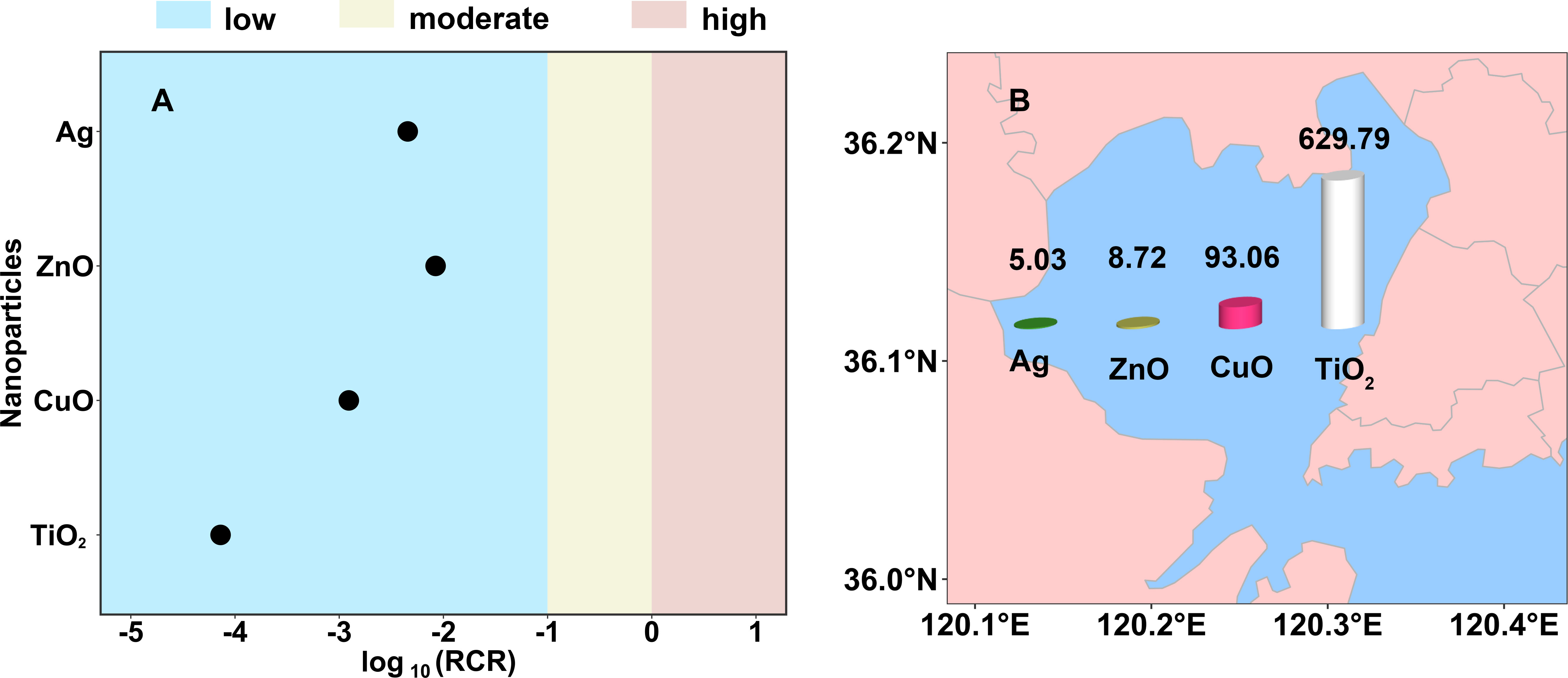
Figure 4 (A) Marine ecological risk and (B) seawater environment carrying capacity (t) of Ag, ZnO, CuO, and TiO2 nanoparticles in Jiaozhou Bay, China.
Environment carrying capacity of metallic nanoparticles
The SECC of metallic NPs was calculated in Jiaozhou Bay, where the actual concentrations of NPs in seawater were measured (Xu et al., 2020). The sea area of Jiaozhou Bay is about 390 km2 with a mean water depth of 7 m (Liu et al., 2005). Thus, the obtained SECC values of Ag, ZnO, CuO, and TiO2 NPs in Jiaozhou Bay were 5.03, 8.72, 93.06, and 629.79 t, respectively (Figure 4B). Among the four NPs, Ag NPs have the lowest SECC value, mainly because they posed the greatest threat to the marine ecosystem. This reminded us that more attention should be paid to the regulation of Ag NPs emissions into Jiaozhou Bay.
The concept of “carrying capacity” is complex and disputed in the course of development (Zhou et al., 2019). Several concepts and tools have been proposed for modelling the impacts of environmental pollution on natural systems (Liao et al., 2013; Bui and Tran, 2022; Yang et al., 2022). One approach is proposed based on the data of pollutants in water bodies, the water quality standards, combined the volume of water bodies, and the retention time of water (Bui and Tran, 2022). In this study, a mathematical model was established for calculating the SECC of metallic NPs in the Jiaozhou Bay. Due to the lack of evaluation standard of marine environmental quality for metallic NPs, the derived PNEC of metallic NPs was adopted to calculate the SECC. Although the model is simplified and needs to be further improved, the simulated values suggests that the carrying capacity of marine environments for metallic NPs is not infinite. Understanding the SECC on metallic NPs have important implications for curbing the environmental pollution and the corresponding ecological risks of metallic NPs.
Conclusion
SSDs were constructed for Ag, ZnO, CuO, and TiO2 NPs, as well as their bulk and ionic counterparts, to better understand the marine ecological risks of NPs. The PNECs of Ag, ZnO, CuO, and TiO2 NPs were 1.85, 3.22, 34.13, and 230.71 μg/L, respectively, and the toxicity order of these metallic NPs to marine species was estimated as Ag > ZnO > CuO > TiO2. Furthermore, the nano-size effect and ion effect on these distributions were also estimated on the basis of the marine toxicity datasets. The toxicity of ZnO, CuO, and TiO2 NPs was more toxic than their bulk form. Meanwhile, the toxicity of Ag, ZnO, and CuO NPs was less than the corresponding metal ion. Therefore, the toxicity of NPs to marine species was derived from the combined effect of size effect and ion effect. Based on the available information, Ag, ZnO, CuO, and TiO2 NPs have not yet posed risks to the marine environment in Jiaozhou Bay at present. The Jiaozhou Bay can carry additional quantities of Ag, ZnO, CuO and TiO2 NPs are 5.03, 8.72, 93.06, and 629.79 t, respectively, before an unacceptable ecological risk occurs. In the future, studies are strongly required to investigate the marine environmental concentrations of metallic NPs to accurately perform the environmental risk assessment.
Data availability statement
The original contributions presented in the study are included in the article/Supplementary Material. Further inquiries can be directed to the corresponding authors.
Author contributions
LZ and BX designed the study. SF, QS and XS collected the data. SF and XZ conducted the analyses. SF, LZ and BX wrote the manuscript. BC and KQ provided oversight of the project. All authors contributed to the article and approved the submitted version.
Funding
This work was supported by the National Natural Science Foundation of China (No. 41706130 and 41976143), the Marine S&T Fund of Shandong Province for Pilot National Laboratory for Marine Science and Technology (No. 2022QNLM040002-3), the Key Technology Research and Development Program of Shandong province (No. 2020CXGC010703), and the Central Public-interest Scientific Institution Basal Research Fund, CAFS (No. 2020TD12).
Conflict of interest
The authors declare that the research was conducted in the absence of any commercial or financial relationships that could be construed as a potential conflict of interest.
Publisher’s note
All claims expressed in this article are solely those of the authors and do not necessarily represent those of their affiliated organizations, or those of the publisher, the editors and the reviewers. Any product that may be evaluated in this article, or claim that may be made by its manufacturer, is not guaranteed or endorsed by the publisher.
Supplementary material
The Supplementary Material for this article can be found online at: https://www.frontiersin.org/articles/10.3389/fmars.2022.985195/full#supplementary-material
References
Adam N., Schmitt C., Bruyn L. D., Knapen D., Blust R. (2015). Aquatic acute species sensitivity distributions of ZnO and CuO nanoparticles. Sci. Tot. Environ. 526, 233–242. doi: 10.1016/j.scitotenv.2015.04.064
Adam V., Wyl A. V., Nowack B. (2021). Probabilistic environmental risk assessment of microplastics in marine habitats. Aquat. Toxicol. 230, 105689. doi: 10.1016/j.aquatox.2020.105689
Ates M., Daniels J., Arslan Z., Farah I. O., Rivera H. F. (2013). Comparative evaluation of impact of zn and ZnO nanoparticles on brine shrimp (Artemia salina) larvae: effects of particle size and solubility on toxicity. Environ. Sci. Proc. Imp. 15, 225–233. doi: 10.1039/c2em30540b
Baalousha M. (2017). Effect of nanomaterial and media physicochemical properties on nanomaterial aggregation kinetics. NanoImpact 6, 55–68. doi: 10.1016/j.impact.2016.10.005
Bondarenko O., Juganson K., Ivask A., Kasemets K., Mortimer M., Kahru A. (2013). Toxicity of Ag, CuO and ZnO nanoparticles to selected environmentally relevant test organisms and mammalian cells in vitro: a critical review. Arch. Toxicol. 87, 1181–1200. doi: 10.1007/s00204-013-1079-4
Brunner T. J., Wick P., Manser P., Spohn P., Grass R. N., Limbach L. K., et al. (2006). In vitro cytotoxicity of oxide nanoparticles: Comparison to asbestos, silica, and the effect of particle solubility. Environ. Sci. Technol. 40, 4374–4381. doi: 10.1021/es052069i
Bui L. T., Tran D. L. T. (2022). Assessing marine environmental carrying capacity in semi-enclosed coastal areas — models and related databases. Sci. Tot. Environ. 838, 156043. doi: 10.1016/j.scitotenv.2022.156043
Cedergreen N., Spliid N. H., Streibig J. C. (2004). Species-specific sensitivity of aquatic macrophytes towards two herbicide. Ecotox. Environ. Safe. 58, 314–323. doi: 10.1016/j.ecoenv.2004.04.002
Chen G., Peijnenburg W. J. G. M., Xiao Y., Vijver M. G. (2018). Developing species sensitivity distributions for metallic nanomaterials considering the characteristics of nanomaterials, experimental conditions, and different types of endpoints. Food Chem. Toxicol. 112, 563–570. doi: 10.1016/j.fct.2017.04.003
Coll C., Notter D., Gottschalk F., Sun T. Y., Som C., Nowack B. (2016). Probabilistic environmental risk assessment of five nanomaterials (nano-TiO2, nano-Ag, nano-ZnO, CNT, and fullerenes). Nanotoxicology 10, 436–444. doi: 10.3109/17435390.2015.1073812
Dong F., Wu C., Miao A.-J., Pan K. (2021). Reduction of silver ions to form silver nanoparticles by redox-active organic molecules: Coupled impact of the redox state and environmental factors. Environ. Sci. Nano. 8, 269–281. doi: 10.1039/D0EN00820F
Duffy L. L., Osmond-McLeod M. J., Judy J., King T. (2018). Investigation into the antibacterial activity of silver, zinc oxide and copper oxide nanoparticles against poultry-relevant isolates of Salmonella and Campylobacter. Food Control. 92, 293–300. doi: 10.1016/j.foodcont.2018.05.008
Du X., Zhou W., Zhang W., Sun S., Han Y., Tang Y., et al. (2021). Toxicities of three metal oxide nanoparticles to a marine microalga: Impacts on the motility and potential affecting mechanisms. Environ. pollut. 290, 118027. doi: 10.1016/j.envpol.2021.118027
ECB (2003). Technical guidance document on risk assessment-part II (Italy: Institute for Health and Consumer Protection).
ECHA (2008a). Guidance on information requirements and chemical safety assessment (Helsinki, Finland: European Chemicals Agency).
ECHA (2008b). “Characterisation of dose [concentration]-response for environment,” in Guidance on information requirements and chemical safety assessment (Helsinki, Finland: European Chemicals Agency), 8. (Chapter R.10).
Farkas J., Cappadona V., Olsen A. J., Hansen B. H., Posch W., Ciesielski T. M., et al. (2020). Combined effects of exposure to engineered silver nanoparticles and the water-soluble fraction of crude oil in the marine copepod Calanus finmarchicus. Aquat. Toxicol. 227, 105582. doi: 10.1016/j.aquatox.2020.105582
Fazelian N., Movafeghi A., Yousefzadi M., Rahimzadeh M. (2019). Cytotoxic impacts of CuO nanoparticles on the marine microalga Nannochloropsis oculata. Environ. Sci. pollut. R. 26, 17499–17511. doi: 10.1007/s11356-019-05130-0
Garner K. L., Suh S., Lenihan H. S., Keller A. A. (2015). Species sensitivity distributions for engineered nanomaterials. Environ. Sci. Technol. 49, 5753–5759. doi: 10.1021/acs.est.5b00081
Geppert M., Sigg L., Schirmer K. (2021). Toxicity and translocation of Ag, CuO, ZnO and TiO2 nanoparticles upon exposure to fish intestinal epithelial cells. Environ. Sci. Nano. 8, 2249–2260. doi: 10.1039/d1en00050k
GESAMP (1986). Environmental capacity. an approach to marine pollution prevention. Reports and studies (Rome, Italy: IMO/FAO/Unesco/WMO/WHO/IAEA/UN/UNEP Joint Group of Experts on the Scientific Aspects of Marine Pollution), Vol. 30. 49.
Gottschalk F., Nowack B. (2013). A probabilistic method for species sensitivity distributions taking into account the inherent uncertainty and variability of effects to estimate environmental risk. Integr. Environ. Asses. 9, 79–86. doi: 10.1002/ieam.1334
Gottschalk F., Sonderer T., Scholz R. W., Nowack B. (2009). Modeled environmental concentrations of engineered nanomaterials (TiO2, ZnO, Ag, CNT, fullerenes) for different regions. Environ. Sci. Technol. 43, 9216–9222. doi: 10.1021/es9015553
Han Y., Shi W., Rong J., Zha S., Guan X., Sun H., et al. (2019). Exposure to waterborne nTiO2 reduces fertilization success and increases polyspermy in a bivalve mollusc: a threat to population recruitment. Environ. Sci. Technol. 53, 12754–12763. doi: 10.1021/acs.est.9b03675
He D., Bligh M. W., Waite T. D. (2013). Effects of aggregate structure on the dissolution kinetics of citrate-stabilized silver nanoparticles. Environ. Sci. Technol. 47, 9148–9156. doi: 10.1021/es400391a
Jassim A. Y., Wang J. J., Chung K. W., Loosli F., Chanda A., Scott G. I., et al. (2022). Comparative assessment of the fate and toxicity of chemically and biologically synthesized silver nanoparticles to juvenile clams. Colloid. Surface. B. 209, 112173. doi: 10.1016/j.colsurfb.2021.112173
Kjølholt J., Gottschalk F., Brinch A., Lützhøft H. C. H., Hartmann N. B., Nowack B., et al (2015). Environmental assessment of nanomaterial use in Denmark: final report (Copenhagen:Danish Environmental Protection Agency). Available at: http://mst.dk/service/publikationer/publikationsarkiv/2015/nov/environmentalassessment-of-nanomaterial-use-in-denmark.
Klaine S. J., Alvarez P. J. J., Batley G. E., Fernandes T. F., Handy R. D., Lyon D. Y., et al. (2008). Nanomaterials in the environment: Behavior, fate, bioavailability, and effects. Environ. Toxicol. Chem. 27, 1825–1851. doi: 10.1897/08-090.1
Liao E., Jiang Y., Yan X.-H., Chen Z., Wang J., Zhang L. (2013). Allocation of marine environmental carrying capacity in the xiamen bay. Mar. pollut. Bull. 75, 21–27. doi: 10.1016/j.marpolbul.2013.08.023
Libralato G., Minetto D., Totaro S., Mičetić I., Pigozzo A., Sabbioni E., et al. (2013). Embryotoxicity of TiO2 nanoparticles to Mytilus galloprovincialis (Lmk). Mar. Environ. Res. 92, 71–78. doi: 10.1016/j.marenvres.2013.08.015
Liu N., Tang M. (2020). Toxic effects and involved molecular pathways of nanoparticles on cells and subcellular organelles. J. Appl. Toxicol. 40, 16–36. doi: 10.1002/jat.3817
Liu S., Zhang J., Chen H., Zhang G. (2005). Factors influencing nutrient dynamics in the eutrophic jiaozhou bay, north China. Prog. Oceanogr. 66, 66–85. doi: 10.1016/j.pocean.2005.03.009
Miao A.-J., Schwehr K. A., Xu C., Zhang S.-J., Luo Z., Quigg A., et al. (2009). The algal toxicity of silver engineered nanoparticles and detoxification by exopolymeric substances. Environ. pollut. 157, 3034–3041. doi: 10.1016/j.envpol.2009.05.047
Moreno-Garrido I., Pérez S., Blasco J. (2015). Toxicity of silver and gold nanoparticles on marine microalgae. Mar. Environ. Res. 111, 60–73. doi: 10.1016/j.marenvres.2015.05.008
Musee N. (2011). Simulated environmental risk estimation of engineered nanomaterials: A case of cosmetics in Johannesburg city. Hum. Exp. Toxicol. 30, 1181–1195. doi: 10.1177/0960327110391387
Nel A., Xia T., Mädler L., Li N. (2006). Toxic potential of materials at the nanolevel. Science 311, 622–627. doi: 10.1126/science.1114397
Prato E., Fabbrocini A., Libralato G., Migliore L., Parlapiano I., D'Adamo R., et al. (2021). Comparative toxicity of ionic and nanoparticulate zinc in the species Cymodoce truncata, Gammarus aequicauda and Paracentrotus lividus. Environ. Sci. pollut. R. 28, 42891–42900. doi: 10.1007/s11356-021-13712-0
Qian W., Chen C. C., Zhou S., Huang Y., Zhu X., Wang Z., et al. (2020). TiO2 nanoparticles in the marine environment: Enhancing bioconcentration, while limiting biotransformation of arsenic in the mussel Perna viridis. Environ. Sci. Technol. 54, 12254–12261. doi: 10.1021/acs.est.0c01620
R Core Team (2022) The r project for statistical computing. Available at: https://www.R-project.org/ (Accessed July 18, 2022).
Rekulapally R., Chavali L. N. M., Idris M. M., Singh S. (2019). Toxicity of TiO2, SiO2, ZnO, CuO, au and Ag engineered nanoparticles on hatching and early nauplii of artemia sp. PeerJ 6, e6138. doi: 10.7717/peerj.6138
Robichaud C. O., Uyar A. E., Darby M. R., Zucker L. G., Wiesner M. R. (2009). Estimates of upper bounds and trends in nano-TiO2 production as a basis for exposure assessment. Environ. Sci. Technol. 43, 4227–4233. doi: 10.1021/es8032549
Rotini A., Gallo A., Parlapiano I., Berducci M. T., Boni R., Tosti E., et al. (2018). Insights into the CuO nanoparticle ecotoxicity with suitable marine model species. Ecotox. Environ. Safe. 147, 852–860. doi: 10.1016/j.ecoenv.2017.09.053
Rotini A., Tornambè A., Cossi R., Iamunno F., Benvenuto G., Berducci M. T., et al. (2017). Salinity-based toxicity of CuO nanoparticles, CuO-bulk and Cu ion to Vibrio anguillarum. Front. Microbiol. 8. doi: 10.3389/fmicb.2017.02076
Schiavo S., Oliviero M., Li J., Manzo S. (2018). Testing ZnO nanoparticle ecotoxicity: Linking time variable exposure to effects on different marine model organisms. Environ. Sci. pollut. R. 25, 4871–4880. doi: 10.1007/s11356-017-0815-3
Shi W., Guan X., Sun S., Han Y., Du X., Tang Y., et al. (2020). Nanoparticles decrease the byssal attachment strength of the thick shell mussel Mytilus coruscus. Chemosphere 257, 127200. doi: 10.1016/j.chemosphere.2020.127200
Šiller L., Lemloh M.-L., Piticharoenphun S., Mendis B. G., Horrocks B. R., Brümmer F., et al. (2013). Silver nanoparticle toxicity in sea urchin Paracentrotus lividus. Environ. pollut. 178, 498–502. doi: 10.1016/j.envpol.2013.03.010
Sun X., Chen B., Xia B., Han Q., Zhu L., Qu K. (2017). Are CuO nanoparticles effects on hemocytes of the marine scallop (Chlamys farreri) caused by particles and/or corresponding released ions? Ecotox. Environ. Safe. 139, 65–72. doi: 10.1016/j.ecoenv.2017.01.014
Thiagarajan V., Ramasubbu S., Natarajan C., Mukherjee A. (2019). Differential sensitivity of marine algae dunaliella salina and chlorella sp. to P25 TiO2 NPs. Environ. Sci. pollut. R. 26, 21394–21403. doi: 10.1007/s11356-019-05332-6
Umbría-Salinas K., Valero A., Martins S. E., Wallner-Kersanach M. (2021). Copper ecological risk assessment using DGT technique and PNEC: A case study in the Brazilian coast. J. Hazard. Mater. 403, 123918. doi: 10.1016/j.jhazmat.2020.123918
USEPA (1998). Guidelines for ecological risk assessment, EPA/630/R-95/002F. (Washington, DC, USA:U.S. Environmental Protection Agency).
Vicario-Parés U., Castañaga L., Lacave J. M., Oron M., Reip P., Berhanu D., et al. (2014). Comparative toxicity of metal oxide nanoparticles (CuO, ZnO and TiO2) to developing zebrafish embryos. J. Nano. Res. 16, 2550. doi: 10.1007/s11051-014-2550-8
Wang J., Wang W.-X. (2014). Low bioavailability of silver nanoparticles presents trophic toxicity to marine medaka (Oryzias melastigma). Environ. Sci. Technol. 48, 8152–8161. doi: 10.1021/es500655z
Wang Y., Zhou X., Engel B. (2018). Water environment carrying capacity in bosten lake basin. J. Clean. Prod. 199, 574–583. doi: 10.1016/j.jclepro.2018.07.202
Wang Y., Zhu X., Lao Y., Lv X., Tao Y., Huang B., et al. (2016). TiO2 nanoparticles in the marine environment: Physical effects responsible for the toxicity on algae Phaeodactylum tricornutum. Sci. Tot. Environ. 565, 818–826. doi: 10.1016/j.scitotenv.2016.03.164
Wheeler J. R., Leung K. M. Y., Morritt D., Sorokin N., Rogers H., Toy R., et al. (2002). Freshwater to saltwater toxicity extrapolation using species sensitivity distributions. Environ. Toxicol. Chem. 21, 2459–2467. doi: 10.1002/etc.5620211127
Wu F., Sokolov E. P., Khomich A., Fettkenhauer C., Schnell G., Seitz H., et al. (2022). Interactive effects of ZnO nanoparticles and temperature on molecular and cellular stress responses of the blue mussel mytilus edulis. Sci. Tot. Environ. 818, 151785. doi: 10.1016/j.scitotenv.2021.151785
Xia B., Chen B., Sun X., Qu K., Ma F., Du M. (2015). Interaction of TiO2 nanoparticles with the marine microalga nitzschia closterium: growth inhibition, oxidative stress and internalization. Sci. Tot. Environ. 508, 525–533. doi: 10.1016/j.scitotenv.2014.11.066
Xia B., Sui Q., Sun X., Han Q., Chen B., Zhu L., et al. (2018). Ocean acidification increases the toxic effects of TiO2 nanoparticles on the marine microalga chlorella vulgaris. J. Hazard. Mater. 346, 1–9. doi: 10.1016/j.jhazmat.2017.12.017
Xu L., Wang Z., Zhao J., Lin M., Xing B. (2020). Accumulation of metal-based nanoparticles in marine bivalve mollusks from offshore aquaculture as detected by single particle ICP-MS. Environ. pollut. 260, 114043. doi: 10.1016/j.envpol.2020.114043
Yang Y., Chen T., Liu X., Wang S., Wang K., Xiao R., et al. (2022). Ecological risk assessment and environment carrying capacity of soil pesticide residues in vegetable ecosystem in the three gorges reservoir area. J. Hazard. Mater. 435, 128987. doi: 10.1016/j.jhazmat.2022.128987
Zhao J., Cao X., Liu X., Wang Z., Zhang C., White J., et al. (2016). Interactions of CuO nanoparticles with the algae Chlorella pyrenoidosa: adhesion, uptake, and toxicity. Nanotoxicology 10, 1297–1305. doi: 10.1080/17435390.2016.1206149
Zhao J., Lin M., Wang Z., Cao X., Xing B. (2020). Engineered nanomaterials in the environment: are they safe? Crit. Rev. Env. Sci. Tec. 51, 1443–1478. doi: 10.1080/10643389.2020.1764279
Zha S., Rong J., Guan X., Tang Y., Han Y., Liu G. (2019). Immunotoxicity of four nanoparticles to a marine bivalve species, Tegillarca granosa. J. Hazard. Mater. 377, 237–248. doi: 10.1016/j.jhazmat.2019.05.071
Zha S., Tang Y., Shi W., Liu H., Sun C., Bao Y., et al. (2022). Impacts of four commonly used nanoparticles on the metabolism of a marine bivalve species, Tegillarca granosa. Chemosphere 296, 134079. doi: 10.1016/j.chemosphere.2022.134079
Zheng Y., Nowack B. (2021). Size-specific, dynamic, probabilistic material flow analysis of titanium dioxide releases into the environment. Environ. Sci. Technol. 55, 2392–2402. doi: 10.1021/acs.est.0c07446
Zhou X.-Y., Zheng B., Khu S.-T. (2019). Validation of the hypothesis on carrying capacity limits using the water environment carrying capacity. Sci. Tot. Environ. 665, 774–784. doi: 10.1016/j.scitotenv.2019.02.146
Zhu L., Booth A. M., Feng S., Shang C., Xiao H., Tang X., et al. (2022). UV-B radiation enhances the toxicity of TiO2 nanoparticles to the marine microalga Chlorella pyrenoidosa by disrupting the protection function of extracellular polymeric substances. Environ. Sci. Nano. 9, 12254–12261. doi: 10.1039/d1en01198g
Keywords: Nanoparticles, marine species, species sensitivity distribution, risk assessment, environment carrying capacity
Citation: Feng S, Zhu L, Zhao X, Sui Q, Sun X, Chen B, Qu K and Xia B (2022) Ecological risk assessment of metallic nanoparticles on the marine environments: Species sensitivity distributions analysis. Front. Mar. Sci. 9:985195. doi: 10.3389/fmars.2022.985195
Received: 03 July 2022; Accepted: 25 July 2022;
Published: 12 August 2022.
Edited by:
Ke Pan, Shenzhen University, ChinaReviewed by:
Guangxu Liu, Zhejiang University, ChinaYouji Wang, Shanghai Ocean University, China
Zhihua Feng, Jiangsu Ocean Universiity, China
Copyright © 2022 Feng, Zhu, Zhao, Sui, Sun, Chen, Qu and Xia. This is an open-access article distributed under the terms of the Creative Commons Attribution License (CC BY). The use, distribution or reproduction in other forums is permitted, provided the original author(s) and the copyright owner(s) are credited and that the original publication in this journal is cited, in accordance with accepted academic practice. No use, distribution or reproduction is permitted which does not comply with these terms.
*Correspondence: Lin Zhu, emh1bGluQHlzZnJpLmFjLmNu; Bin Xia, eGlhYmluQHlzZnJpLmFjLmNu