- 1School of Earth Sciences and Environmental Engineering, Gwangju Institute of Science and Technology, Gwangju, South Korea
- 2Department of Oceanography, College of Natural Science, Chonnam National University, Gwangju, South Korea
- 3Resources Enhancement Division, East Sea Branch, Korea Fisheries Resources Agency, Pohang, South Korea
- 4Research Institute for Coastal Environment and Fishery-Policy Center, Gwangju, South Korea
Knowledge of physiological responses of important shellfish species to rising temperatures is crucial in assessing the impacts of climate change on marine aquaculture production. The physiological components of energy balance that support growth performance were measured seasonally at different exposure temperatures in the ark clams (Anadara kagoshimensis) cultured in the shallow muddy bottom sediment in Yeoja Bay, Korea. We tested the effects of winter minimum (3–8°C) and summer maximum (23–28°C) temperature elevations on individual physiological processes (ingestion, respiration, egestion, and excretion) and the combined energetic physiology (scope for growth [SFG] and net growth efficiency [K2] measures). The seasonal cycle of dry flesh tissue weight (DW) was also investigated from January 2016 to November 2018, to compare its variation at contrasting cold vs. warm regimes. The rates of physiological components were related to DW, generating significant allometric equations. The weight exponents of the equations for ingestion rate and respiration rate were low at the winter minimum compared with the remaining season temperatures, indicating a higher thermal sensitivity in larger individuals. The physiological rates that were re-calculated for individual components based on estimates of the slope and intercept of the equations increased with increasing temperature, revealing an incapability of thermal acclimation and a temperature effect at seasonally different endogenous conditions. The thermal sensitivity (Q10) of the ingestion rate and respiration rate was reversed between the winter minimum and the summer maximum temperature elevations, yielding negative SFG and K2 values at 3 and 28°C. Furthermore, the interannual difference in the seasonal cycle of clam DW displayed variations in the period of increment prior to spawning and the post-spawning loss/recovery in association with its energy balance status in the winter and summer temperature conditions. Overall, these results indicate that warming is projected to affect physiological performance and the seasonal DW cycle of clams in different manners between winter and summer: physiological benefits and advanced weight gain vs. heat stress and progressive weight loss, respectively. The mechanistic adjustment of the clam energy balance across thermal conditions seems to explain the recent advancement in its seasonal biological cycle, as well as the failure in spat collection and the mass summer mortality observed at this culturing site.
Introduction
In recent decades, coastal marine ecosystems have undergone modifications in long-term and seasonal thermal regimes in response to climate change (Lima and Wethey, 2012). Marine ectotherms have a capacity for physiological adjustment in response to thermal stress (Somero, 2012). These organisms show physiological plasticity to meet their energy requirements associated with the physiological stress response (Seebarcher et al., 2015). The resulting physiological responses can be integrated by determining the energy available for somatic and gametic production, i.e., scope for growth (SFG) (Bayne and Newell, 1983). Moreover, changes in the thermal regime may have a fundamental effect on biological processes (i.e., growth, reproductive phenology, development, and distributional range), as most physiological processes are temperature dependent (Bayne and Newell, 1983; Bopp et al., 2013; Woodin et al., 2013; Philippart et al., 2014).
Sedentary clams inhabiting temperate coastal zones are subject to changes in the prevailing thermal regime and seasonal thermal variations in their habitats associated with warming of the sea (Jung, 2008; Dunstan et al., 2018). The ark shell (Anadara kagoshimensis Tokunaga, 1906) inhabits the soft muddy bottom of the shallow (depth, 5–10 m) subtidal zone along the northwestern Pacific coasts, including those of Korea, Japan, and China (Nakamura, 2005; Feng et al., 2009). This clam shows a clear biological cycle regarding growth and reproduction, in which gametogenesis initiates in winter and spawning takes place mainly during the late spring and summer, reflecting seasonal thermal variations (Lee, 1998; Kim et al., 2008). As natural seed spat collection was developed in the 1970s, aquaculture of the ark shell was pioneered in Yeoja Bay off the southern coast of Korea and now accounts for 90% of domestic production in Korea. According to its phenology, the seeds are collected from shallow (depth,<5 m) subtidal spawning grounds from June to August. The spats are transplanted to shallow muddy bottom sediment (30%–40% silt and ~65% clay; mean grain size, 9–9.5 ϕ), in which natural settlement of larvae occurs, and grown for 12–18 months until they reach a marketable size (MOF (Ministry of Oceans and Fisheries, Korea), 2019). The total annual production in Korea was estimated to be 5,000–10,000 metric tons in the 1990s and 2000s, but gradually declined to approximately 3,000 metric tons in the 2010s (MIFAFF (Ministry of Food, Agriculture, Forestry and Fisheries, Korea), 2010). Recently, clam production has fluctuated yearly (e.g., 2,369 metric tons in 2017 and 4,439 metric tons in 2018) (KOSIS (Korea Statistical Information Service), 2019). The recent decline in the annual production of ark clams cultured on the coast of Korea has been attributed to a failure of larval production, poor larval survival, and/or mass mortality of adult clams in spring and summer associated with the elevated temperature (Park et al., 2011; Kim et al., 2019).
Based on long-term data of sea surface temperatures collected daily at a monitoring station located on the bay mouth by the National Institute of Fisheries Science (available at: www.nifs.go.kr/kodc/), the average winter minimum temperatures have increased from 4.2 (± 1.5, 1 SD) °C in 1963–1990 to 6.0 (± 1.0) °C in 1991–2018 and 7.2°C in 2019; whereas the average summer maximum temperatures have increased from 25.9 (± 1.1) °C in 1963–2015 to 27.5 (± 1.1) °C in 2016–2019 (unpublished data, H.Y. Kang). An increase in water temperature in winter due to climate change and the resultant advancement of warmer spring conditions may alter the physiological and reproductive processes of clams reared in the natural subtidal zone, as supported by an advancement in gametogenic development and spawning (Park et al., 2011). When exposed to high temperatures, thermal changes (17–32°C) cause physiological modifications in the ark shell, with the impact of the heat stress being accompanied by an increase in mortality in response to prolonged exposure to the stress (Zhao et al., 2016; Jiang et al., 2020). Understanding the physiological processes underlying the adjustment to the energy balance of the ark shell to varying thermal conditions would help predict its growth and further phenology, and contribute to aquaculture activities. Although the interannual variation in the energy reserves and reproductive cycles of A. kagoshimensis was found to be closely associated with changes in seasonal thermal regimes (Park et al., 2011), its physiological responses that regulate energy balance to apparent temperature changes during the critical periods (i.e., winter initiation of gametogenesis and summer post-spawning recovery) remain poorly understood.
The thermal sensitivity of biological reactions is defined by the temperature coefficient (Q10), which has been traditionally used as an approach to measure the temperature dependence of physiological processes (Schmidt-Nielsen, 1983; Schipper et al., 2014). The Q10 provides useful information regarding the attributes of individual physiological components in organisms (Nespolo et al., 2003; Wang et al., 2018). Identifying the responses to environmental changes at an individual level further requires the interpretation of energy dynamics based on the integration of the overall physiological measures of energy balance (Newell and Bayne, 1980; Ibarrola et al., 2008; Kang et al., 2015). There is a broad consensus on the temperature dependence of feeding and metabolism, which are major physiological components of energy balance, in marine ectotherms. However, the species-specific responses to thermal changes deviate from a typical exponential curve, provoking inconsistencies between the expected and the general trends (Giomi and Pörtner, 2013). Metabolic rates increase with increasing temperature in some marine ectotherms (Jasen et al., 2007; Giomi and Pörtner, 2013), but remain constant at different temperatures in others (Newell and Kofoed, 1977). The increased feeding rates that occur to offset the metabolic cost allow animals to maintain a positive energy balance (i.e., SFG) across environmental conditions (Wilbur and Hilbish, 1989; Sarà et al., 2008; Kang et al., 2016). A lack of such compensation processes may induce a negative energy balance under unfavorable conditions (Bayne and Newell, 1983; Bougrier et al., 1995). Therefore, the interspecific differences regarding the presence/absence of the capacity for thermal acclimation may prevent the generalization of physiological responses to thermal changes (Van der Have and De Jong, 1996; Seebarcher et al., 2015). The rates of the physiological components of energy balance have been commonly expressed as a function of body size (i.e., weight) because of the allometric relationships between them (Bayne and Newell, 1983; Ibarrola et al., 2008). Based on the allometric equation, the exponents scale physiological rates to body size, which characterizes the species-specific growth effect on the physiological processes of organisms. In addition, probabilistic estimates of the rates allow comparisons of physiological performance in different thermal conditions. Conversely, because seasonal variation in the endogenous factors of organisms (e.g., growth, reproductive conditions, and energy-reserve levels) influence their physiological processes, seasonal measurements may provide a more realistic indication of the relationship between growth performance and a combination of physiological processes (Newell and Bayne, 1980; Iglesias and Navarro, 1991; Ibarrola et al., 2008; Shin et al., 2011).
The present study aimed to measure the seasonal variation of the physiological components (e.g., ingestion, egestion, excretion, and respiration) of energy balance in A. kagoshimensis, examine its physiological adjustment processes under a wide thermal range, and estimate SFG to evaluate its capacity of net production. Specifically, we tested (1) the effect of rising minimum temperature on winter energy dynamism, which would be responsible for the advancement of the accumulation of energy reserves in spring and, thus, the reproductive phenology of this species; and (2) the effect of an extremely high temperature on summer energy dynamism, which would determine the recovery of the somatic condition after spawning, with a particular emphasis on their physiological responses to changes in thermal regimes. Our final goal was to highlight the manner in which the recent warming of this sea area (which is changing the thermal regime) affects the seasonal energy dynamism that supports the growth performance of this clam species. Our hypothesis was that physiological responses of the clam to winter and summer warming would alter their seasonal energy dynamism and finally patterns in fitness.
Materials and methods
Sample collection and experimental design
The suspension-feeding ark shell Anadara kagoshimensis (Tokunaga, 1906) was collected at a culturing site (34°79′ N, 127°52′ E) in Yeoja Bay on the southern coast of the Korean Peninsula. The bay is shallow (mean depth, 5.4 m) and semi-enclosed, encompassing a total area of about 320 km2. To test the physiological responses of A. kagoshimensis to rising winter minimum and summer maximum temperatures within the wide annual thermal range, we collected specimens in February 2018 (winter) and August 2017 (summer), respectively. We set the thermal conditions used in physiological measurements to 3°C and 8°C in February and 23°C and 28°C in August, respectively, to test the thermal effects by making comparisons of physiological rates between cold and warm temperatures under the identical endogenous conditions (e.g., spent, resting, initial, and ripe stages in gametogenic stage in August, November, February, and May, respectively: Table 1) in each season. Here, 3°C and 23°C represent the cold conditions in February and August, respectively; and 8°C and 28°C represent the elevated winter-minimum and summer-maximum temperature (i.e., warmer) conditions, respectively, in the same months. We also collected specimens in November 2017 (autumn) and May 2018 (spring) and conducted measurements at 13°C and 18°C, respectively. These experimental thermal conditions were chosen according to the seasonal thermal conditions and long-term increasing trends in minimum and maximum temperatures, representing an interval of 5°C.
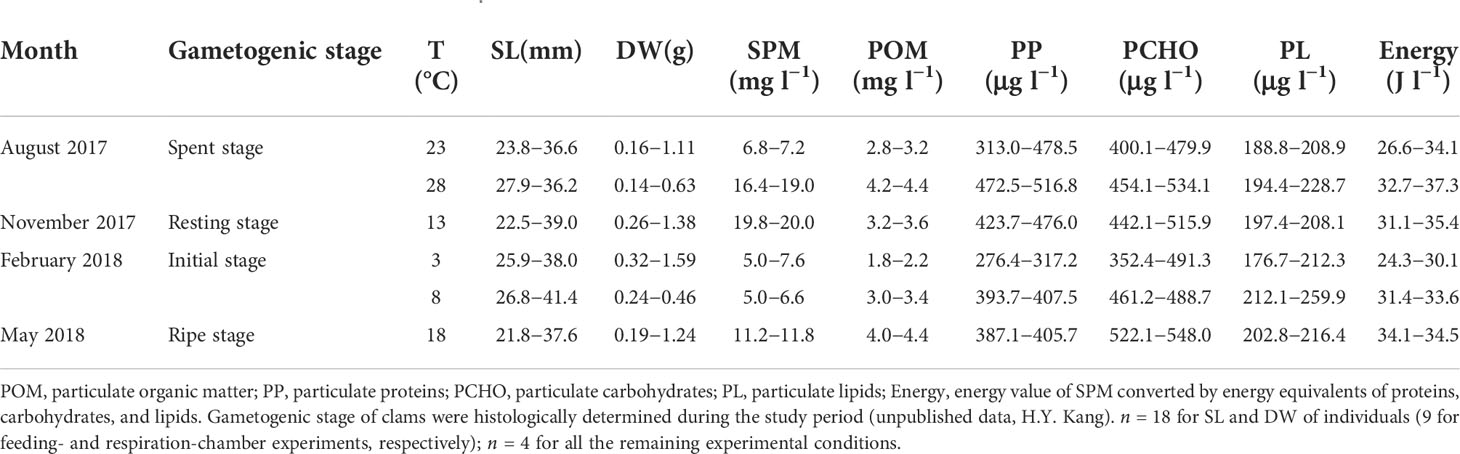
Table 1 Shell length (SL) and dry tissue weight (DW) of experimental individuals, and composition of suspended particulate matter (SPM) supplied to the ark shell Anadara kagoshimensis.
For physiological measurements, about 100 specimens were collected using a clam-harvesting dredge at the same rearing ground in each season. Groups of clams of varying sizes were randomly collected (Table 1), placed in filtered seawater (temperature, 4.5–26.8 °C; salinity, 30.1–33.7) with aeration, and transported to a 200-L water tank that was equipped with a continuous seawater flow system and aeration in the laboratory. On each sampling occasion, 40 individuals were then acclimated to the experimental temperatures to secure sufficient specimens for physiological measurements. The range of those temperatures covered the long-term, interannual, and seasonal variations in the bay. Finally, 18 individuals (9 for feeding- and respiration-chamber experiments, respectively) were used for physiological measurements.
The water temperature in the container gradually increased or decreased by 1 °C/day to minimize the effect of acute thermal variation on physiological activity. After reaching the desired temperature, the specimens were kept under the constant temperatures to acclimate to tank water conditions for 14 days before measurements of physiological activities were performed (Figure 1). During the acclimation period, the ark shells were fed daily with a mixed diet (2 × 106 cells L−1, Shellfish Diet 1800®; Reed Mariculture, Campbell, CA, USA) composed of four marine microalgae (40% Isochrysis sp., 15% Pavlova sp., 20% Thalassiosira weissflogii, and 25% Tetraselmis sp.); subsequently, the feeding was halted for 48 h before the initiation of the measurements, to minimize the relevant physiological activities. After being transferred to the experimental chambers, the specimens were acclimated again to chamber conditions of water-flow rate and temperature for 6 h before the beginning of the measurements.
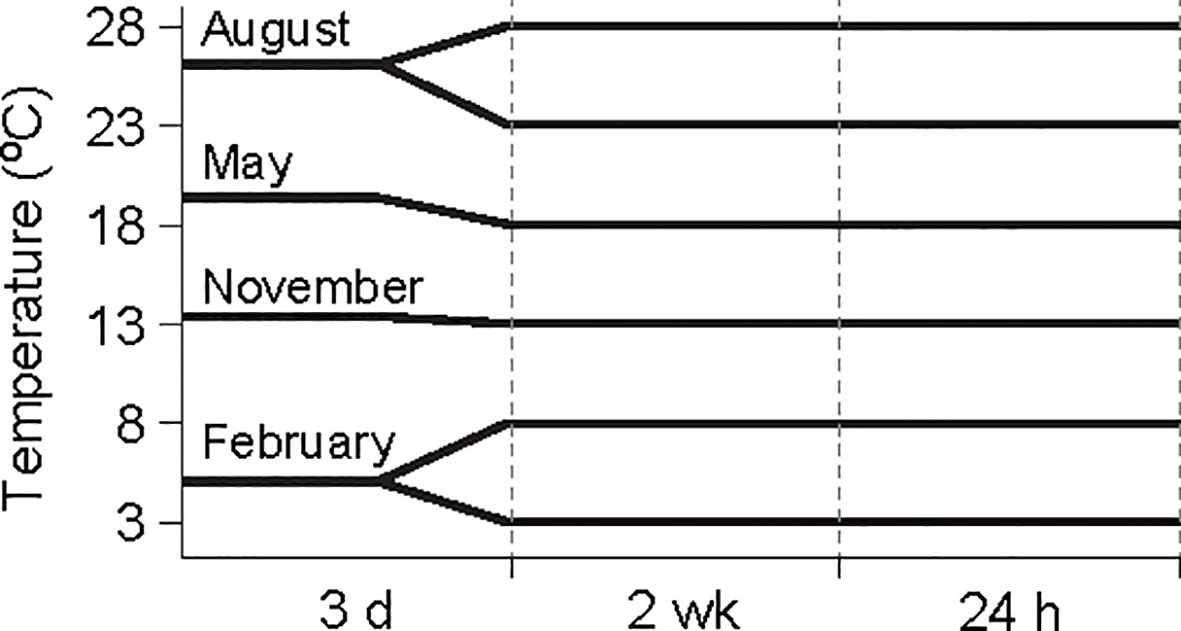
Figure 1 Experimental design and water temperature regimes in different seasons. Water temperatures were adjusted to 3°C and 8°C for February conditions to test the effect of winter warming on physiological activities in Anadara kagoshimensis, and 23°C and 28°C for August conditions to test the effect of summer warming. 13°C and 18°C represent November (autumn) and May (spring) conditions.
All measurements were carried out over 24 h in seasonal field conditions in terms of suspended particulate matter (SPM) concentration and temperature, to exclude confounding effects of the changes in diet concentration and acclimation to experimental temperatures. The feeding rate of the ark shells was determined using 300-mL flow-through open cylindrical chambers, and the respiration rate was assessed using 350-mL closed chambers equipped with a magnetic stirrer. Among the 10 chambers used to assess each component, nine experimental chambers were occupied by a single individual each and one chamber was left empty, as a control. The chambers were immersed in a water bath to keep the water temperature constant for each experimental condition. Aerated water was continuously supplied into the individual chambers by a peristaltic pump equipped with a 10-channel pump head (BT 100-1 L; Longer Precision Pump Co. Ltd, Baoding, China). The concentration and composition of the SPM in the experimental water that was supplied for feeding were adjusted, to minimize variations in the experimental conditions (Table 1). The flow rates in each chamber were adjusted to approximately 20 mL min−1 to maintain the percentage of particles cleared from the inflow of the chamber at around 20% (Filgueira et al., 2006). The oxygen concentration in the individual chamber was kept at a saturation level above 80% (Bougrier et al., 1995).
Furthermore, monthly clam collections were carried out from January 2016 to November 2018, for the interannual comparison of the seasonal cycles of flesh weight under rising winter minimum and summer maximum temperatures. The samplings were conducted at the end of each month. For monthly biometric measurements, 20–40 clam individuals were collected at two neighboring sites (one, collection site of specimens for physiological measurements; another, rearing ground about 1 km away from that site within the bay). They were kept alive in filtered seawater during transport to the laboratory and were starved overnight to evacuate gut materials. Subsequently, the biometric parameters (shell length, height and width, and flesh dry weight) of clams were recorded to test for seasonal variations in growth patterns in cool vs. warm thermal conditions. On each sampling occasion, the water temperature was determined in situ using a YSI Model 85 probe (YSI Inc., Yellow Springs, OH, USA). Water samples for the assessment of the suspended particulate matter (SPM) and phytoplankton biomass (chlorophyll a) were collected at 1 m above the sea floor using a van Dorn water sampler and screened through a 180-μm Nitex mesh to remove large particles and zooplankton on board. The water collected was placed in acid-washed plastic bottles and kept on ice in the dark during the transportation to the laboratory.
Physiological measurements
Ingestion rate
The ingestion rate of the ark shells was determined by quantifying the differences in particles in the outflow of the control chamber compared with each of the experimental chambers. All measurements were carried out over 24 h in seasonal field conditions in terms of suspended particulate matter (SPM) concentration and temperature, to exclude confounding effects of the changes in diet concentration and acclimation to experimental temperatures. Water from the outflow of the chambers was collected every 6 h over a 24 h period and individual quantification data of particle components were summed. Subsequently, 500 mL of seawater was filtered onto precombusted Whatman GF/F filters (diameter, 47 mm; mesh, 0.7 μm). The filters were lyophilized. Proteins were hydrolyzed with 0.5 N sodium hydroxide and then determined using a colorimetric method (Lowry et al., 1951). Carbohydrates were extracted in 15% trichloroacetic acid and determined according to the phenol–sulfuric acid method (Dubois et al., 1956). Lipids were extracted in a mixture of chloroform and methanol (Bligh and Dyer, 1959) and measured using a colorimetric method (Marsh and Weinstein, 1966). The weights of biochemical components were converted and summed to their energy equivalents (J d−1) using the energy conversion factors of 24.0, 17.5, and 39.5 J per 1 mg of proteins, carbohydrates, and lipids, respectively (Gnaiger, 1983). Pseudofecal materials were expected during the experiments; however, they were excluded from this analysis because of the difficulty in collecting them. Finally, the ingestion rate was used instead of the filtration rate in the present study.
Feces production rate
Fecal materials produced by ark shells were collected several times (every 3–4 h) using a micropipette during the 24-h experiment and transferred into 15-mL precombusted glass tubes. The total feces collected per clam were summed over the 24-h period. After centrifugation, the fecal pellets were rinsed with Milli Q water to eliminate salt contents, lyophilized, and weighed. The egestion (i.e., feces production) rate was calculated from the biochemical composition of the fecal materials and their energy equivalents (J d−1), according to the procedures described above for ingestion rate analysis.
Ammonium excretion rate
For ammonium excretion measurements, water samples were collected every 6 h from the outflow of the chambers during the experiments. The total amount of ammonia excreted per clam was summed over the 24-h period. Ammonium concentrations were spectrophotometrically analyzed using the phenol–hypochlorite reaction (Parsons et al., 1984). The excretion rate was calculated from the differences in ammonium concentration between the control and the experimental chambers, and was converted into the energy equivalent (J d−1) using a conversion factor (24.83 J for 1 mg of NH4-N) (Elliot and Davison, 1975).
Respiration rate
The oxygen concentration inside the closed chambers was continuously recorded for 24 h according to a measuring protocol (Bougrier et al., 1998) using oximetric probes (Oxy-10 micro; PreSens-Precision Sensing GmbH, Regensburg, Germany). The respiration rate was calculated from the difference in oxygen concentration between the control and the experimental chambers. The flow rate of water to keep a saturation level above of 80% was acquired based on a preliminary experiment and correct your oxygen concentrations and saturation level were corrected for temperature, salinity, and barometric pressure. The energy conversion factor of oxygen consumption was 14.0 J for 1 mg of O2 (Gnaiger, 1983). The O:N ratio was calculated by dividing the oxygen consumption in atoms by the ammonium excretion in atoms (Bayne and Newell, 1983).
Thermal sensitivity
The thermal sensitivity (Q10) of the rates of individual physiological components was calculated for the winter minimum (3–8°C) and the summer maximum (23–28°C) temperature elevations using the following formula: Q10 = (R2 / R1)10 / (T2 – T1), where R represents the rate of the parameter at temperature (T) 1 and 2.
Scope for growth
SFG was calculated based on the energy balance equation in an individual using the energy equivalents of physiological components (Winberg, 1956; Bayne and Newell, 1983): SFG = A – ( U + R) , where A is the absorbed energy calculated from the difference between the ingested energy (C) and the egested energy by feces production (F), U is the excretion energy, and R is the respiration energy. The assimilation efficiency (AE) was then assessed using the formula AE=(R+SFG)/C. Finally, the net growth efficiency (K2) was calculated using the formula K2=SFG/A.
Biometric measurements
After physiological measurements and monthly treatment in the laboratory, the shell length (SL) of individual specimens was measured using Vernier calipers. Soft flesh tissues of the ark shells were carefully dissected from their shells (Newell and Bayne, 1980). Shells were dried at 60°C for 72 h in a drying oven and weighed to determine the dry shell weight. Flesh tissues were lyophilized for 72 h and weighed to determine the dry flesh tissue weight (DW).
Food availability measures
Duplicate water (1 L) samples for the SPM and chlorophyll a measurements were filtered onto precombusted Whatman GF/F glass fiber filters (47 mm; pore size, 0.7 μm). The filters used for SPM assessment were dried at 60°C for 48 h, placed in a vacuum desiccator, and weighed after cooling to room temperature. The SPM concentration was determined based on the difference in the mass recorded before and after filtration. The filters were then ignited at 450°C for 4 h and weighed, to obtain the particulate organic matter (POM). Chlorophyll a was extracted by soaking the filters in 90% acetone for 24 h in the dark at −20°C. Its concentration was determined using a fluorometer (Turner Designs Model 10 AU 005, Sunnyvale, CA, USA) according to Holm-Hansen et al. (1965). Monthly mean values were used in the present study.
Size standardization and statistics of physiological measures
We transformed the physiological data and DW of the ark shells logarithmically (base 10). We then performed a least-squares regression analysis between both variances for each experimental condition according to the following allometric equation: Y = aWb where Y is the physiological rate, W is the DW, and a and b are the fitted constants that represent intercepts and slopes, respectively. We tested the significance of differences between regression equations using an analysis of covariance (ANCOVA). In cases of absence of significant differences among the fitted slopes (P > 0.05), we calculated a common slope (, representing a weight exponent) and re-estimated the intercepts () using the value. Subsequently, we tested the difference among the intercepts of the various sets of regressions using a Bonferroni post hoc multiple-comparison test. Otherwise, we adjusted the physiological rates to the value of a standard-weight animal, which represents a grand mean of the covariate (0.6 g DW in the present study) of all the experimental ark shells, according to the following equation (Packard and Boardman, 1987): Ys = (Ws / We)b × Ye , where Ys is the physiological rate of a standard individual, Ws is its DW, We is the DW of the experimental ark shell,Ye is the measured physiological rate of the experimental ark shell, and b is the corresponding allometric coeffcient (which corresponded to in the present study). We then compared group means adjusted for the effect of the covariate among the experimental groups using one-way analysis of variance (ANOVA) after testing for normality (Shapiro–Wilk test) and the homogeneity of variances (Levene’s test). ANOVA was followed by a Bonferroni post hoc multiple-comparison test. The physiological data of clam energy balance parameters are presented by the rates adjusted to a grand mean DW (0.6 g). Statistical analyses were performed using SPSS software (v. 22.0; IBM Corp., Armonk, NY, USA).
Seasonal patterns in flesh weight
To compare the seasonal patterns in the flesh-weight variation of the clam between contrasting thermal (cold vs. warm) conditions, we calculated the monthly DW of a standard individual with an SL of 34.3 mm (the grand mean SL of all specimens analyzed), to eliminate the clam-size effect on DW variation. To do this, the analysis described for physiological measurements was used to relate the DW to SL. All regressions were statistically significant (P< 0.01). A subsequent ANCOVA revealed no significant differences among the estimates of the slopes. Based on the common slope and re-estimated intercepts, we computed the monthly DW values of a standard individual by substituting the grand mean SL in the regression equations.
Results
Our physiological measurements were conducted for 2–3 years old clams with a SL range of 21.8 to 41.4 mm (Table 1). The regression analyses between the physiological rates (i.e., ingestion, respiration, feces production, and ammonium excretion) and the DW of A. kagoshimensis from Yeoja Bay revealed significant positive relationships at all seasonal experimental conditions for each variable (P< 0.05; Table 2). The physiological rates standardized to a grand mean DW (0.6 g) based on the estimates of the slope and intercept of the allometric equation demonstrated a temperature effect at seasonally different endogenous conditions. All monthly regressions between the SL and DW of the clams were also statistically significant (P< 0.001, Table 3). The monthly DWs of a standard individual (34.3 ± 3.5 mm in SL), as estimated using the DW–SL regressions, characterized seasonal cycles in flesh weight between the colder and warmer temperature regimes.
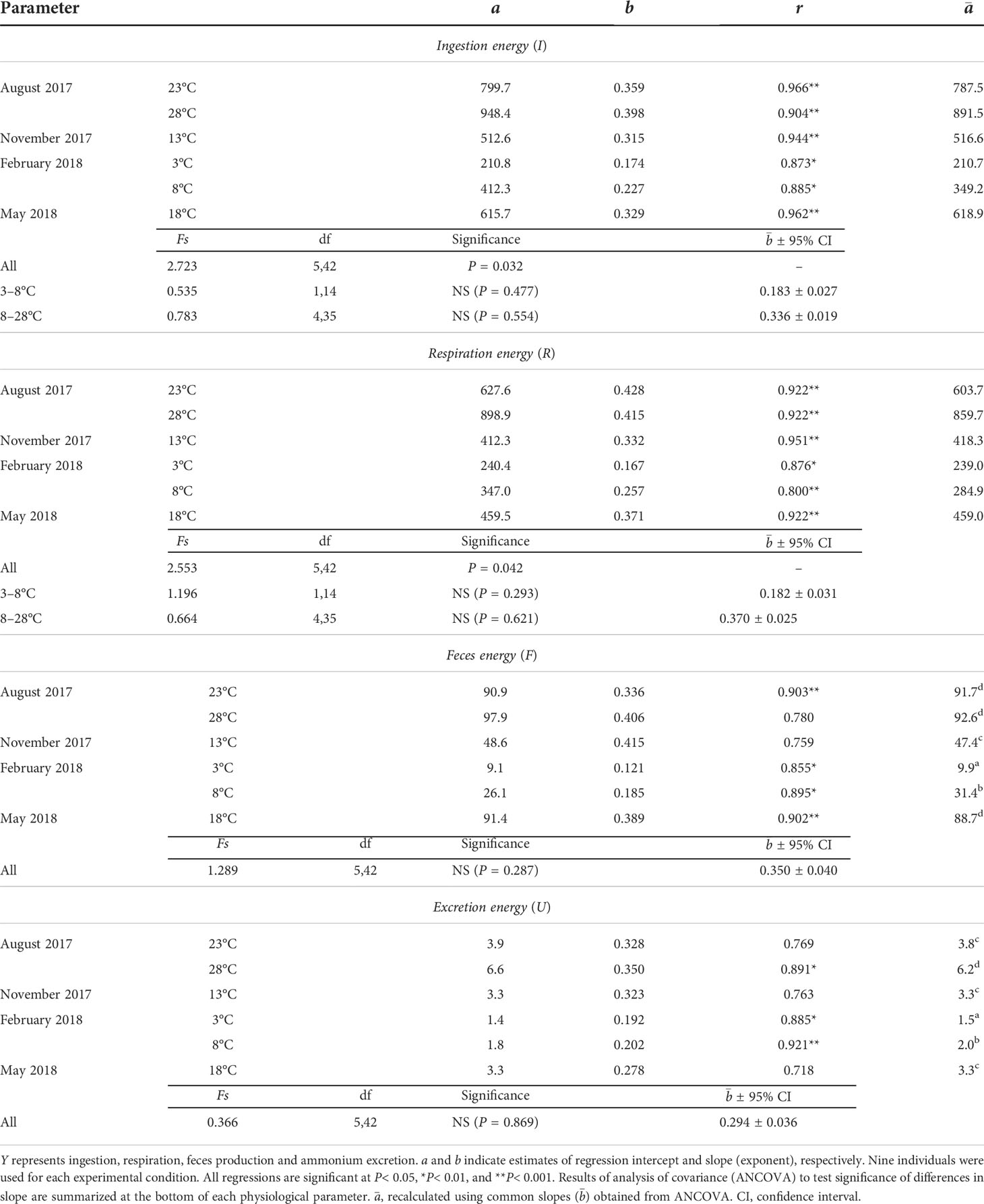
Table 2 Regression coefficients of allometric equation Y=aWb between physiological rate (Y, J d–1) and dry flesh weight (W, g) of the ark shell Anadara kagoshimensis at different temperatures.
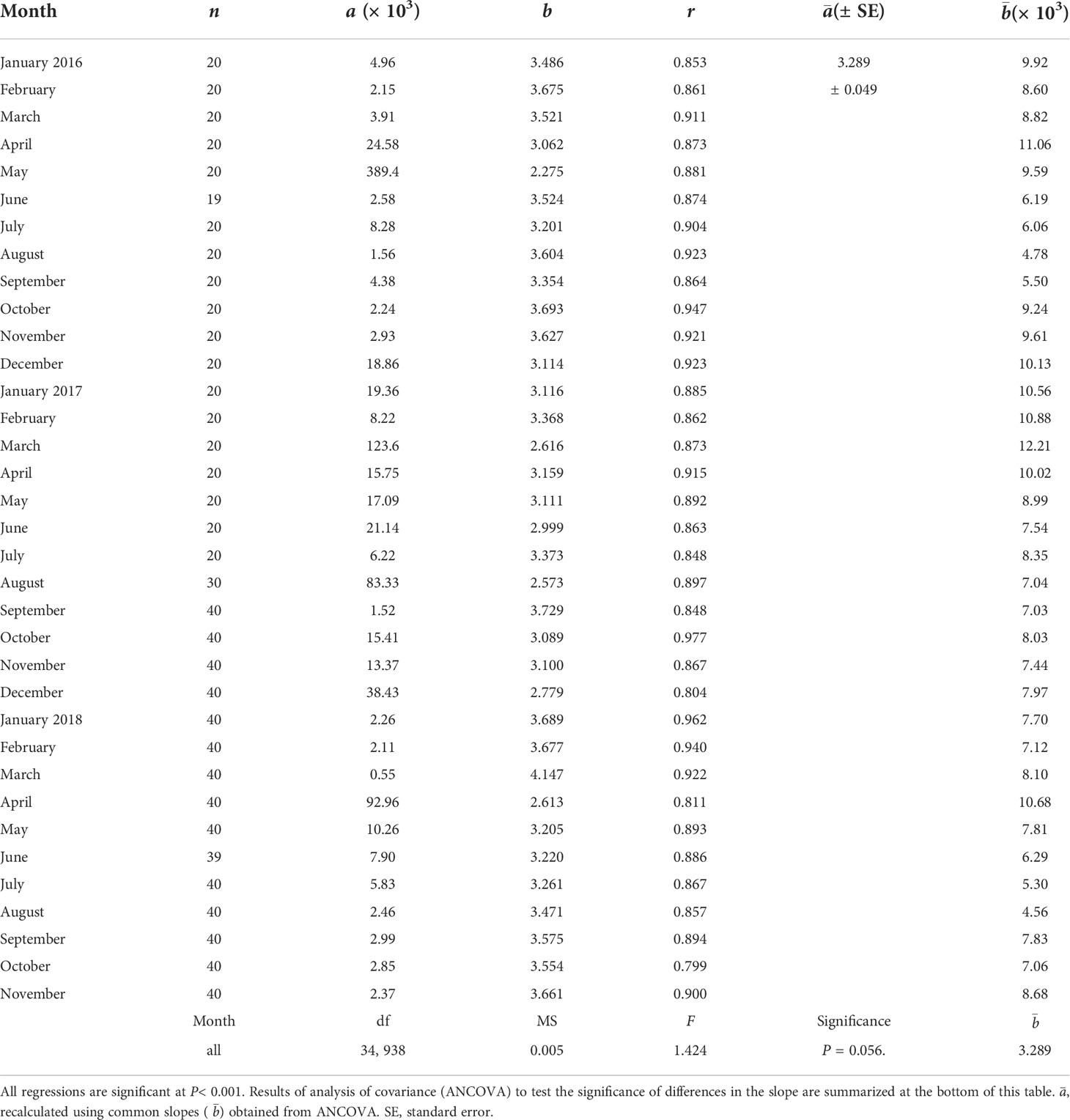
Table 3 Regression coefficients of allometric equation W = aLbbetween shell length (L, mm) and dry flesh weight (W, mg) of the ark shell Anadara kagoshimensis. a and b indicate estimates of regression intercept and slope (exponent), respectively.
Ingestion rates
ANCOVA revealed a significant difference in the estimates of the slope of the ingestion–DW regressions (F5,42 = 2.723, P = 0.032). This precluded further testing of differences among intercepts (Table 2). The ANCOVA result divided the estimates of the slope into two groups; within the winter (3–8°C) and the remaining season (8–23°C) groups, no significant differences were found between the estimates of slope (F1,14 = 0.535, P = 0.477; F4,35 = 0.783, P = 0.554, respectively), providing a common slope () for each group (0.183 ± 0.027 and 0.336 ± 0.019, respectively). In fact, the slope was steeper in the warmer vs. the colder season groups (F1,59 = 19.007, P< 0.001).
ANOVA of the ingestion rates adjusted to a grand mean DW (0.6 g) exhibited a significant difference in all experimental groups (F5,48 = 253.55, P< 0.001; Figure 2A). A subsequent Bonferroni test revealed a significant effect of temperature on ingestion, indicating a considerable increase at both the winter minimum (192 vs. 318 J d−1 at 3°C and 8°C, respectively) and the summer maximum (663 vs. 751 J d−1 at 23°C and 28°C, respectively) temperature elevations (P< 0.05). The ingestion rates were much lower at the winter minimum temperatures than those at the summer maximum temperatures, with the rates in November (435 J d−1 at 13°C) and May (521 J d−1 at 18°C) intervening between those at the winter and the summer temperatures.
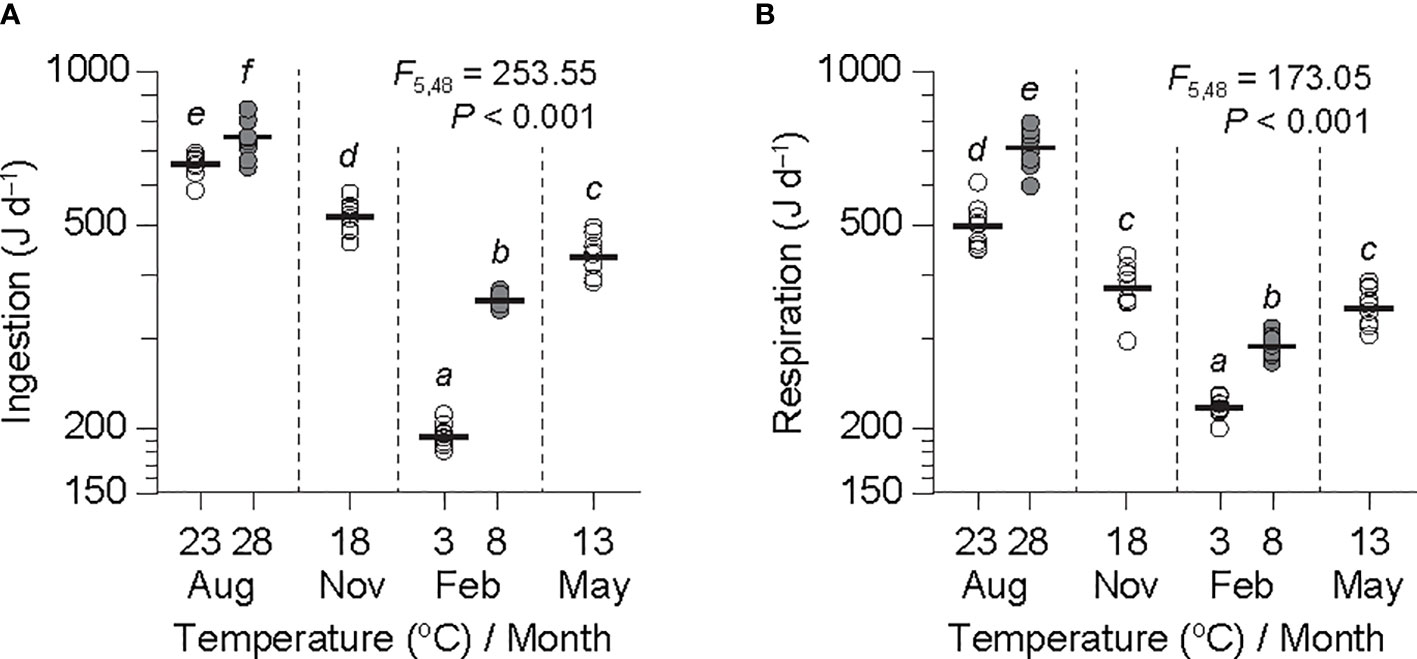
Figure 2 Rates of ingestion (A) and respiration (B) adjusted to an individual ark shell (Anadara kagoshimensis) with a dry-flesh weight of 0.6 g recorded in August and November 2017, and February and May 2018, based on the common slopes () obtained at both temperature ranges from Table 2. Sample size, n = 9 in each group. The thick horizontal lines represent the means of individual groups. The open and closed circles of August and February represent cold and warm conditions in summer and winter, respectively. The results of an analysis of variance and subsequent Bonferroni post hoc test on the adjusted values are embedded in the figures. The same letters indicate the absence of significant differences between means.
Respiration rates
ANCOVA revealed a significant difference (F5,42 = 2.553, P = 0.042) among the slopes of the oxygen consumption–DW regressions at six experimental conditions (Table 2), thus preventing a direct comparison of the differences among intercepts. There were no significant differences among the estimates of the slope of the regressions within the groups incubated at winter (3–8°C) and the remaining season (8–28°C) temperature ranges (F1,14 = 1.196, P = 0.293; F4,35 = 0.664, P = 0.621, respectively). ANCOVA further revealed a significant difference in the slopes between the two groups (F1,59 = 5.928, P = 0.018), with warmer season groups exhibiting a steeper common slope () of 0.370 ± 0.025, vs. 0.182 ± 0.031 for colder season groups.
ANOVA of respiration rates, when adjusted to a grand mean DW (0.6 g), indicated a significant difference among the means of the experimental groups (F5,48 = 173.05, P< 0.001; Figure 2B). A subsequent Bonferroni test indicated a significant temperature effect with an abrupt increase in respiration for both the winter minimum (217 vs. 260 J d−1 at 3°C and 8°C, respectively) and the summer maximum (509 vs. 724 at 23°C and 28°C, respectively) temperature elevations (P< 0.05). The respiration rates were also much higher at the winter minimum temperatures that those at the summer maximum temperatures. The rates in November (352 J d−1 at 13°C) and May (259 J d−1 at 18°C) fell between those at the winter and the summer temperatures. Interestingly, the increasing trend in respiration rate was much more pronounced at the summer than at the winter temperatures.
Feces production rate
ANCOVA performed on the feces production–DW regressions revealed no significant differences (F5,42 = 1.289, P = 0.287) and generated a common slope () of 0.350 ± 0.040 (Table 2). Moreover, ANCOVA showed significant differences between the intercepts of feces production–DW regressions (F5,47 = 267.78, P< 0.001). A Bonferroni test further showed a significant effect of temperature on feces production at the winter (8.3 vs. 26.3 J d−1 at 3°C and 8°C, respectively) temperature elevation (P< 0.05), but not at the summer (76.7 vs. 77.4 J d−1 at 23°C and 28°C, respectively) elevation, for which the highest rates were recorded (Figure 3A). The feces production rates in November (39.6 J d−1 at 13°C) and May (74.2 J d−1 at 18°C) intervened between those at the winter and the summer temperatures.
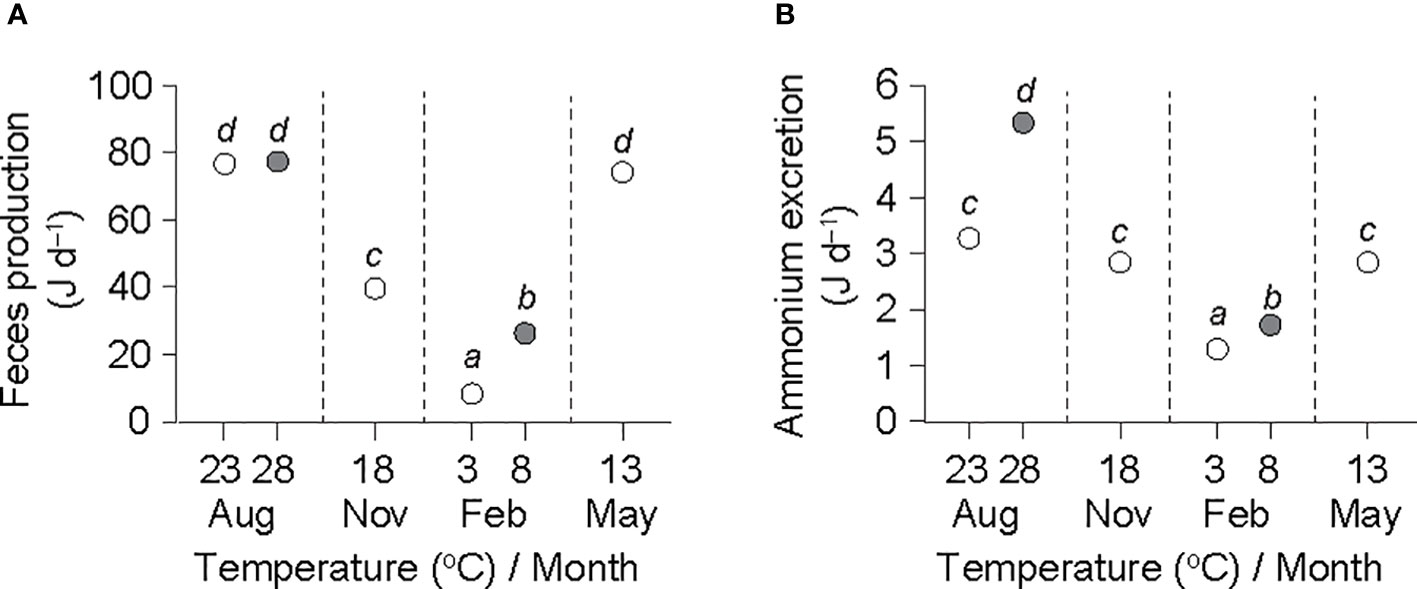
Figure 3 Rates of feces production (A) and ammonium excretion (B) in an individual ark shell (Anadara kagoshimensis) with a dry-flesh weight of 0.6 g recorded in all six experimental groups in August and November 2017, and February and May 2018. The rates were re-calculated using common and re-estimated () values from Table 2. The open and closed circles of August and February represent cold and warm conditions in summer and winter, respectively. Sample size, n = 9 in each group. The same letters indicate the absence of significant differences between means (Bonferroni test, P< 0.05).
Ammonium excretion rate
ANCOVA revealed no significant differences among the slopes of the ammonium excretion–DW regressions (F5,42 = 0.366, P = 0.869), yielding a common slope () of 0.294 ± 0.036 (Table 2). ANCOVA performed on the intercepts of the regressions denoted significant differences in the rate of ammonium excretion among the seasonal experimental conditions (F5,47 = 108.47, P< 0.001). A Bonferroni test identified a significant increase in ammonium excretion at both the winter minimum (1.3 vs. 1.7 J d−1 at 3°C and 8°C, respectively) and the summer maximum (3.3 vs. 5.3 J d−1 at 23°C and 28°C, respectively) temperature elevations (P< 0.05; Figure 3B). The ammonium excretion rates in November (2.8 J d−1 at 13°C) and May (2.8 J d−1 at 18°C) fell between those at the winter and the summer temperatures.
The physiological rates of clam energy balance parameters (i.e., ingestion, respiration, feces production, and ammonium excretion) to a grand mean DW (0.6 g) were lowest in February (3°C) and highest in August (28°C) and indicated an increasing trend with increasing temperature at seasonally different endogenous conditions.
Assimilation efficiency (AE) and O:N ratio
The AE values obtained based on physiological rates adjusted to a grand mean DW (0.6 g) fell within a narrow range, from 85% in November (18°C) to 95% in February (3°C) (Figure 4A). In the same manner, the atomic ratio of oxygen consumed to nitrogen excreted (O:N ratio) was sustained at high levels (>120) in all experimental groups (Figure 4B).
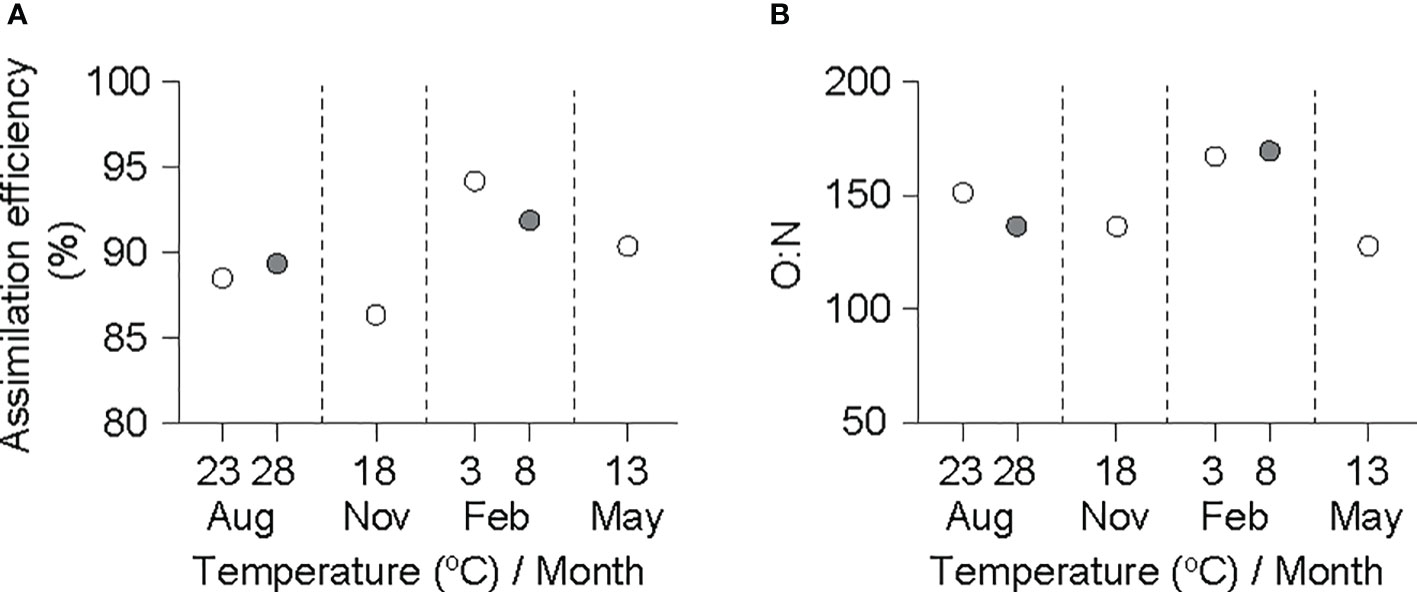
Figure 4 Assimilation efficiency (A) and O:N ratio (B) in an individual ark shell (Anadara kagoshimensis) with a dry-flesh weight of 0.6 g recorded in all six experimental groups in August and November 2017, and February and May 2018. The open and closed circles of August and February represent cold and warm conditions in summer and winter, respectively.
Temperature coefficient (Q10)
The Q10 values denoted a pronounced thermal sensitivity in the physiological rates (>1) with increasing temperature at the summer maximum and winter minimum temperature conditions (Table 4). The Q10 for filtration rates showed a drastic change at the winter minimum temperature elevation (3–8°C) and a reduced thermal sensitivity at the summer maximum temperature elevation (23–28°C). The Q10 for respiration rates exhibited a slightly elevated thermal sensitivity at the summer (23–28°C) compared with the winter (3–8°C) temperature elevations. The Q10 for feces production rates displayed a similar trend to that of ingestion rates, with higher values recorded for the winter (3–8°C) compared with the summer (23–28°C) temperature elevations. Ammonium excretion exhibited high Q10 values at the summer temperature range (23–28°C).

Table 4 Q10 values for physiological rates of ingestion, respiration, feces production and ammonia excretion of the ark shell Anadara kagoshimensis at different temperatures.
SFG and K2
The SFG, based on physiological rates adjusted to a grand mean DW (0.6 g), exhibited a clear effect of temperature at both the summer maximum and winter minimum temperatures (Figure 5A). The SFG was negative at the winter minimum (−35.5 J d−1 at 3°C) and summer maximum (−56.0 J d−1 at 28°C) temperatures, and was positive at the remaining experimental conditions (30.4–74.9 J d−1 at 8–23°C). K2 showed a similar pattern to the SFG variation (Figure 5B); the K2 values were negative at the winter minimum (−0.19 at 3°C) and summer maximum (−0.08 at 28°C) temperatures, and were positive at the remaining experimental conditions (0.10–0.13 at 8–23°C).
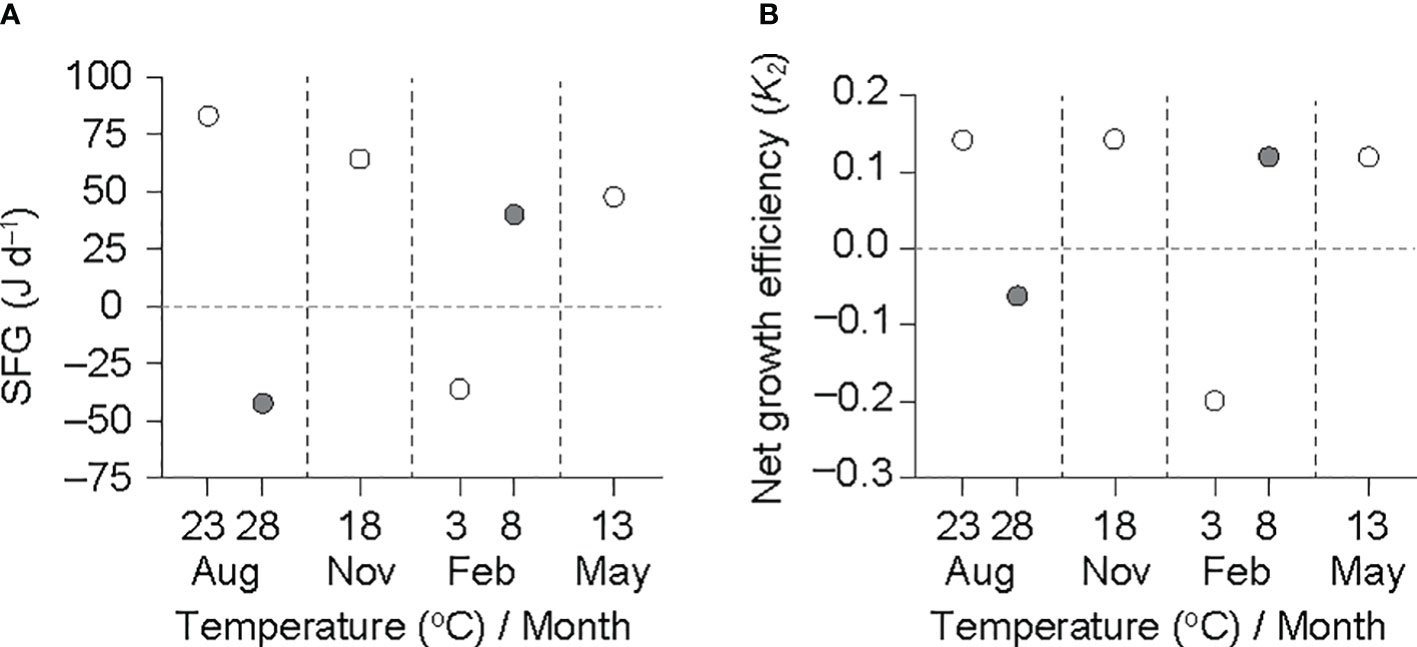
Figure 5 Scope for growth (SFG) (A) and net growth efficiency (K2) (B) in an individual ark shell (Anadara kagoshimensis) with a dry-flesh weight of 0.6 g recorded in all six experimental groups in August and November 2017, and February and May 2018. The open and closed circles of August and February represent cold and warm conditions in summer and winter, respectively.
Seasonal patterns in flesh weight, water temperature, and food availability
All specimens of 2- to 3-year-old clams analyzed here displayed an overall mean SL of 34.3 (± 3.5) mm. The ANCOVA revealed no significant differences between the estimates of the slopes of the monthly DW–SL regressions (F34,938 = 1.424, P = 0.056; Table 2). Based on the common slope () and recalculated intercepts () of the allometric equations, the flesh DW of a standard individual (SL = 34.3 mm) exhibited a clear seasonal variation within each year, peaking in early spring (March or April) (Figure 6). These DW peaks were followed by slimming during spring–summer until the time of minima, which were recorded in August. The minimal DW in summer (June to August) exhibited a significant interannual difference (857 mg ind−1 in 2017 vs. 620 mg ind−1 in 2016 and 2018; Bonferroni test, P< 0.05). A gradual increase in DW after the time of minima occurred through autumn (from September) 2016 and winter (to March) 2017. A flesh-weight increment did not take place from the autumn of 2017 until February of 2018, and was delayed to March–April 2018, displaying further interannual trends (Bonferroni test, P< 0.05).
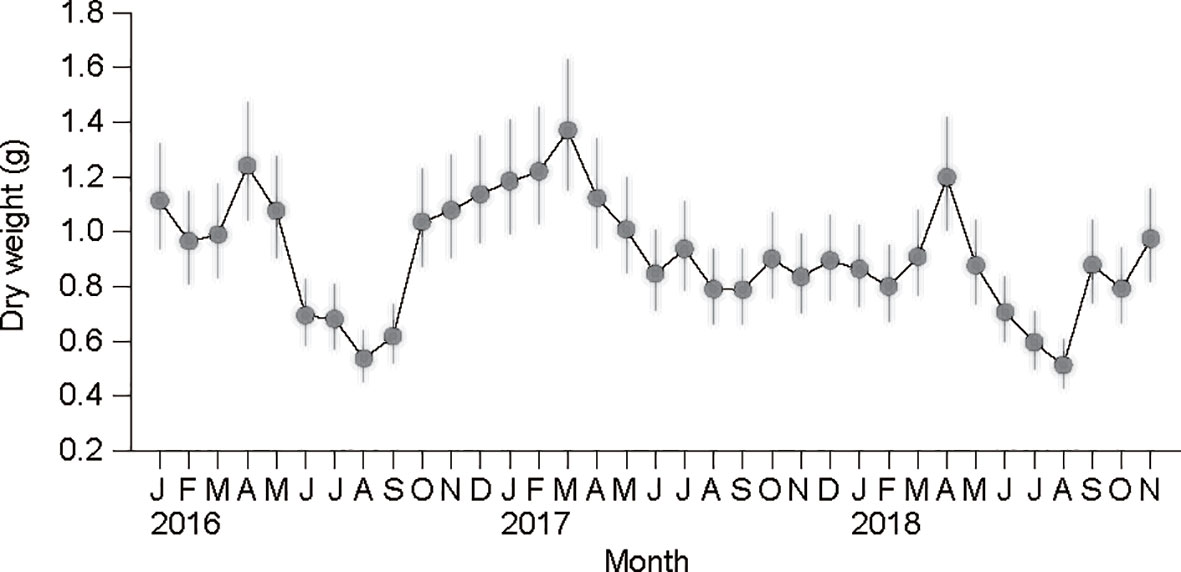
Figure 6 Seasonal variation in dry-flesh weight in a standard individual with a shell length of 34.3 mm recorded from January 2016 to November 2018. The vertical bars represent 95% confidence intervals.
Water temperature exhibited the strong seasonal fluctuation that is typical of the temperate zone during the study period (Figure 7). Both the winter minimum and summer maximum temperatures also showed strong interannual variations: 6.8°C (February 2016) and 7.0°C (February 2017) vs. 2.9°C (January 2018); and 27.7°C (August 2016) and 26.8°C (August 2017) vs. 28.4°C (July 2018), respectively. These colder minimum and warmer maximum temperatures persisted for 2–3 months. Salinity fell within a narrow range between 29.2 and 34.2. The SPM concentration was relatively low, fluctuating irregularly from 12.6 mg L−1 (June 2017) to 52.1 mg L−1 (July 2016). The organic component (POM) of the SPM showed minimal concentrations (1.9–4.4 mg L−1) during the winter, and maximal concentrations (7.0–9.2 mg L−1) during the summer. The chlorophyll a concentration in the water column varied greatly from 0.3 μg L−1 (April 2016) to 8.1 μg L−1 (August 2017), peaking each summer.
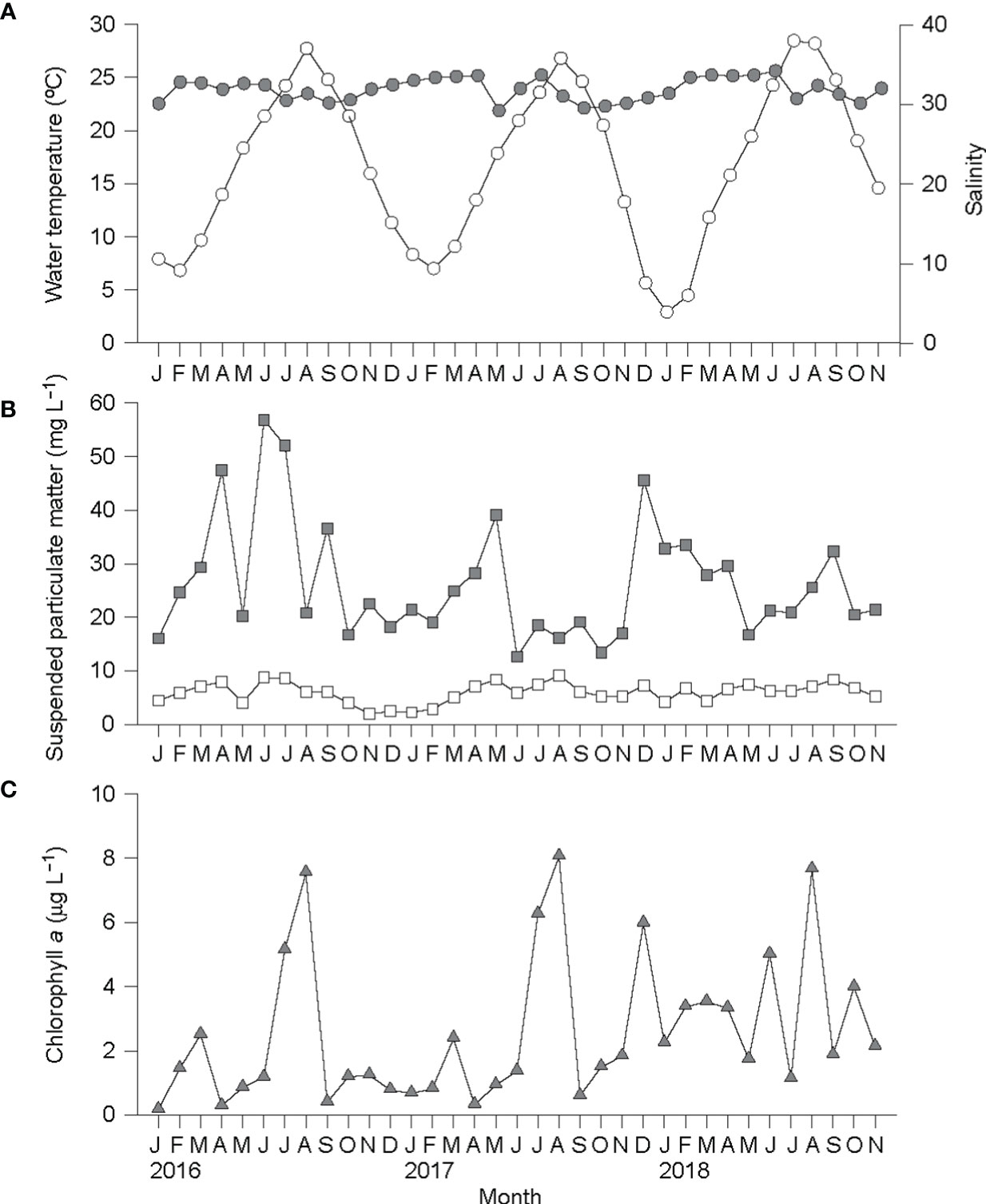
Figure 7 Seasonal variations in water temperature (open circle) and salinity (gray circle) (A), total suspended particulate matter (gray square) and suspended particulate organic matter (open square) concentrations (B), and chlorophyll a concentration (C) in a water column located at the ark shell culturing site in Yeoja Bay, Korea.
Discussion
Yeoja Bay is located in the northeast Asian marginal seas, where the rate of warming is about 2–4 times faster than the world ocean average warming over the last decades (Belkin, 2009). The bay was designated as a Marine Protected Area by the Korean government. Increasing nutrients, decreasing pH, hypoxia, and toxic chemical contamination are absent in the bay (NFRDI (National Fisheries Research and Development Institute), 2010; KOEM (Korea Marine Environment Management Corporation), 2019). Thus, this long-term warming trend has been considered one of the most prominent environmental changes, and seems to be responsible for the recent drop in aquaculture yield (Park et al., 2011; MOF (Ministry of Oceans and Fisheries, Korea), 2019). The rising trend of sea surface temperature observed in the southern sea of Korea (northern part of the East China Sea) is seasonally asymmetric, being more rapid in winter than in summer (Lima and Wethey, 2012; Bao and Ren, 2014), thereby reducing the annual amplitude slightly (Kang, 2000; Min and Kim, 2006). In accordance with this warming trend, our measurements of energy-balance components in clams suggest that both the winter and the summer temperature elevations may have a significant impact on their physiological performance. The physiological rates of A. kagoshimensis were size (i.e., weight) dependent; weight-exponent values () for the ingestion and respiration rates varied with season (i.e., thermal condition). The physiological rates of clam energy balance increased with increasing temperature at both the winter minimum and the summer maximum temperature conditions. Consistent with the reversed trends in thermal sensitivity (Q10) observed between the feeding and metabolic rates at cold and warm conditions, the winter and summer temperature elevations generated a positive and a negative SFG, respectively. The interannual variation in seasonal DW of a standard individual seemed to reflect its physiological performance in varying thermal conditions.
Size dependence of physiological rates
Our physiological measurements of clam energy balance were performed at similar ranges of food availability proxies (Table 1) and at long-term seasonal temperature ranges at the study area (Figure 7), allowing us to evaluate the patterns of thermal acclimation. The size dependence (expressed by the weight exponent in the present study) of the ingestion rate and respiration rate of the ark shells exhibited differentiated patterns between the winter minimum and the summer maximum temperatures (3–8°C and 23–28°C, respectively). This result indicates thermal effects on the size dependence of the physiological processes. In fact, the common weight-exponent value () for the ingestion rate was very much lower (0.183) at the winter minimum temperatures than that (0.336) recorded at the summer maximum temperatures, suggesting the presence of varying degrees of thermal effects between large and small individuals. No comparable data are available for the values of physiological rates in A. kagoshimensis. The exponent values for filtration of suspension-feeding bivalves vary, with a relatively broad range of 0.310–0.820 (overall mean, 0.616; Bayne and Newell, 1983). A similar variation in filtration rate has been observed even within species (Gosling, 2015; MacDonald et al., 2016). The value (0.183) obtained for ingestion in A. kagoshimensis at the minimum temperatures (3–8°C) fell outside the range of other suspension feeders, whereas the value (0.336) obtained at higher temperatures (23–28°C) corresponded to the lower limit of the range.
It has long been documented that the filtration rates (as reported using the ingestion rate in the present study) of suspension-feeding bivalves are highly susceptible to changes in diet quality and quantity (Bayne and Newell, 1983; MacDonald et al., 2016). The measurement of filtration rates in the presence of cultivated microalgal diets yields consistently overestimated rates compared with those obtained using natural seston (Bayne and Newell, 1983; Jørgensen, 1996; Lee et al., 2018). The low exponent values found for A. kagoshimensis suggest that the relative suppression of feeding activity in larger individuals plays a part in lowering the exponent value in the experimental conditions of natural seston diets (Hatton et al., 2005). Those authors found that the filtration rates were constant in small mussels (Perna canaliculus), whereas they varied according to food concentration or quality in large mussels. Conversely, infaunal bivalves, such as A. kagoshimensis, may reduce their filtration rates in the flow-through experiment outside their normal sediment substratum (Riisgård, 1988; Jørgensen, 1996). Therefore, a possible reduction in the ingestion rates measured in the present study caused by suboptimal conditions cannot be excluded completely.
The values for respiration rate in A. kagoshimensis varied from 0.182 at the winter minimum temperatures (3–8°C) to 0.370 at the summer maximum temperatures (23–28°C), in a manner similar to that observed for the ingestion rate. The values in different suspension-feeding bivalves varied between 0.438 and 1.090, with a mean of 0.727 (Bayne and Newell, 1983). The values obtained at the summer maximum temperatures (23–28°C) in the present study were close to the lower limits of this range. Our respiratory measurements represented the routine metabolic rates associated with physiological costs, including feeding, digestion, excretion, and biosynthesis. Given that the values for respiration rate in ectothermic animals can vary among species according to activity level (Glazier, 2009), the observed scaling exponents may reflect diversified responses, such as clam size or the limited food supply provided in the present study compared with the abovementioned measurements. The extremely low value for the respiration rate observed at the winter minimum temperatures (3–8°C) is comparable to the consistently low value obtained for ingestion rate, possibly because of declined feeding among larger individuals at similar cold conditions. This result may support a temperature-dependent modification of the size-dependent pattern of respiration rate in clams.
Interestingly, the size dependence of the egestion rate (as measured based on feces production) and ammonium excretion rate was consistent between the winter minimum and the summer maximum temperatures. The value (0.350) obtained for egestion was very close to that of the ingestion rate at high temperatures (8–28°C) in seasonally different endogenous conditions. This may be explained by high absorption (also assimilation) efficiency detected at all experimental conditions, as discussed later. The value (0.294) observed for the excretion rates was low compared with the other physiological components, suggesting a limited protein metabolism in larger clams, as indicated by the high O:N ratios reported here.
Seasonal energetic physiology
The effect of temperature on feeding activity in temperate regions categorizes bivalves into two groups (Kittner and Riisgård, 2005; Gosling, 2015; MacDonald et al., 2016). Those authors reported that, although some bivalves possess temperature-independent or fairly constant filtration rates over the broad temperature range of 10–26°C, the majority exhibit filtration rates that increase linearly with temperature (Winter, 1978). In either case, filtration declines markedly and even ceases at low temperatures (5°C or below). Consistent with that observed for other bivalves, ingestion in A. kagoshimensis was markedly reduced at 3°C, albeit with no complete cessation. The extremely low value found at the winter minimum temperatures (3–8°C) indicates a more pronounced suppression of feeding activity in larger individuals in cold conditions. Given that our experiments were conducted in a similar range of food concentrations, the progressive increase in the regression intercept (a) values with rising temperature detected over the seasonal range (8–28°C), within which a common weight exponent was warranted, reveals a clear thermal effect on the ingestion rate of the ark shell. The increase in the ingestion rate of A. kagoshimensis with rising temperature is compatible with previous findings (Nakamura, 2005). The lack of temperature acclimation of the filtration rate in bivalves has been considered an evolutionary adaptation that enables them to offset the metabolic energy loss and consequently maintain a potential for growth (Jørgensen, 1990; Kittner and Riisgård, 2005). The dramatically increased Q10 recorded at the winter temperature elevation (3.44, 3–8°C) compared with that (1.29, 23–28°C) recorded at the summer elevation indicates that ingestion may be more sensitive to winter warming.
The metabolic rate (both respiration and ammonium excretion) is a key component that represents the energy expenditure and thereby determines the growth rates of an individual. The comparisons of respiration rate and ammonium excretion rate over the temperature elevations (3–8 and 23–28°C) highlighted the presence of a significant thermal effect on metabolic rate. Metabolic energy expenditure can be adjusted to thermal acclimation in some molluscs, such as Mytilus edulis (Widdows and Bayne, 1971) or Crepidula fornicata (Newell and Kofoed, 1977), or can be temperature dependent in others, such as Ostrea edulis (Newell et al., 1977) or Ruditapes philippinarum (Kang et al., 2016). As mentioned previously, our measurements were performed seasonally to extract thermal effects on physiological rates in a broad temperature range (Table 1). Consistent with the latter bivalve group, the broad range in metabolic cost of A. kagoshimensis and its increase with increasing temperature denote an incapability of acclimation to seasonal temperatures (Zhao et al., 2016; Jiang et al., 2020). This result emphasizes the fact that, although the clam can acclimate its metabolic rate to a narrow range of relatively low temperatures, as observed in a 10°C change (8–17.5°C) for Cardium edule (Newell and Bayne, 1980), the winter minimum and the summer maximum temperature elevations may affect its seasonal metabolism greatly. Considering the temperature-dependent ingestion rate, our results further indicate the increased metabolic costs of feeding during the warm summer season. However, the increased Q10 value obtained for metabolic rate, as opposed to ingestion rate, between 23 and 28°C supports the relatively enhanced thermal effect on metabolic rate observed at 28°C.
As re-calculated using the present allometric equations, the fecal energy loss in A. kagoshimensis was closely associated with the energy acquired by ingestion (r2 = 0.90), corresponding to 5%–15% of the energy consumed. Furthermore, the constant assimilation efficiency (85%–95%) observed for diets offered to feeding trials indicates a temperature-independent assimilation activity (Shin et al., 2011). In a similar manner, despite the effect of rising temperature on the excretion energy loss of A. kagoshimensis, the energy expenditure by excretion was negligible (<1%) among the total energy losses in terms of the energy balance in the present study. Despite its minor role in determining the energy budget, its measurement is useful as an indicator of changes in the catabolism of substrates occurring along the seasonal cycle (Bayne and Newell, 1983). The consistently high O:N values (range, 120–170) detected over the experimental temperature range indicate that A. kagoshimensis catabolizes carbohydrates and lipids predominantly, relative to proteins (<30 for considerable protein catabolism, Bayne and Newell, 1983; Gosling, 2015), for maintenance (and growth) metabolism. Therefore, our results suggest that the observed size- and temperature-dependent trends (b and a values, respectively) in the egestion and excretion rates reflect a temperature-dependent ingestion efficiency in different-sized individuals.
In A. kagoshimensis, the regulation of the SFG and K2 values was attained by adaptive adjustments of the physiological processes that determine the energy budget over a wide temperature range, thus summarizing the thermal impact on energy balance. A maximum and nearly constant positive SFG (and K2) value was observed between 8 and 23°C, respectively, providing seasonally optimal thermal conditions for production. Both the SFG and K2 values were independent of temperature over that temperature range. This positive energy balance reflected a synchronous increment in the ingestion and respiration rates (Wilbur and Hilbish, 1989; Sarà et al., 2008; Kang et al., 2016). In contrast, SFG and K2 dropped drastically to negative values at the winter minimum (3°C) and the summer maximum (28°C) temperatures, indicating the presence of thermal (both cold and heat) stresses. The Q10 of the respiration rate was high (2.02) compared with that (1.29) of the ingestion rate at the temperature elevation of 23–28°C, suggesting that the metabolic rate is more sensitive to temperatures above the optimal value. This indicates that the feeding rate did not increase as much as it compensated for the increased metabolic cost at 28°C (Bougrier et al., 1995; Kang et al., 2015). Conversely, the Q10 of the ingestion rate was maximized by 3.44 at low temperatures of 3–8°C, indicating a more sensitive response than that (1.74) of the respiration rate to temperature variation. As a result, although a more pronounced feeding suppression restricts the compensation even for the lowered metabolic cost at 3°C, the increase in energy acquisition (i.e., ingestion rate) at the elevated temperature (8°C) in winter induces a positive SFG, which may support the winter growth of clams.
Interannual variation in seasonal cycle of flesh weight
The seasonal cycle of flesh tissues in A. kagoshimensis is characterized by a fast storage of energy reserves after weight loss in winter and concomitant gonadal development and somatic growth during spring, as well as a rapid exhaustion of reserves and weight loss during summer (Park et al., 2011). Because the accumulation of energy reserves is synchronized with gametogenic development, the physiological optimization of the energy balance to meet energy requirements for reproductive activity during spring and for synthesis of reserves during summer–autumn, which may be used for maintenance in the quiescent period of winter, is of particular importance to the life-history strategy of the clam, as in other bivalves (Pouvreau et al., 2006; Filgueira et al., 2011; Park et al., 2011). In addition to the seasonal variation, the observed interannual difference in the seasonal cycle of clam weight indicated variations in the period of growth (i.e., weight increment) prior to spawning and the post-spawning weight loss/recovery of a standard individual.
During the growth period from winter to spring (January–April), clams had much higher flesh weight in 2016/2017 vs. 2018. This difference was well associated with a temporal change in thermal conditions. In fact, over the past six decades, the winter minimum temperature fell below 3 °C before 1990, but gradually increased up to 7 °C during the subsequent 30 years (Kang, 2000; Min and Kim, 2006). At the beginning of 2018, the clams experienced a period of extremely low minimum temperature (<3 °C), which resulted in lowered ingestion and metabolic rates and generated a negative SFG. Under this unusual thermal condition recorded in recent years, clam growth was surprisingly slow and delayed to March, allowing us to unravel the physiological regulation behind their growth performance in colder winters. In contrast, the warmer (≥7°C) conditions recorded in the winter of 2016/2017, which is a common observation in recent years, might provide physiological benefits that support a positive SFG and, thereby, the accumulation of energy reserves during winter–early spring (Park et al., 2011), as indicated by the SFG and K2 values calculated at 3 and 8 °C, respectively.
Our observations further provide empirical evidence that physiological stresses and, therefore, an energy imbalance in A. kagoshimensis during the warmer summer after spawning might severely threaten their survival, as hypothesized based on their high summer mortality (MOF (Ministry of Oceans and Fisheries, Korea), 2019). Spawning in marine bivalves is a great energy-demanding process, and it often results in lethal and sublethal stresses during the post-spawning period. Thus, spawned cockles may become more susceptible to secondary stresses such as infection or high temperature during the post-spawning period (summer and autumn) (Hong et al., 2014; Hong et al., 2020; Kim et al., 2022). Because our sampling efforts were made at the end of each month, the abrupt fall in weight indicated that their massive spawning took place in May–June. Despite the improved food availability with regard to the chlorophyll a peak recorded each summer, the clams continued losing weight progressively during July–August in 2016 and 2018, but exhibited a slight recovery during the same months in 2017. Such an interannual discrepancy in clam weight during summer seemed to be linked to a physiological disruption, according to the elevation and longer duration of the maximum temperature detected just after spawning in July–August 2016 and 2018 (Figure 7). This explanation may be supported by the negative SFG because of the lack of compensatory adjustment (i.e., increased metabolic energy loss compared with lowered ingestion rate) in A. kagoshimensis at the temperature elevation of 23–28°C.
Conclusion
Our results suggest that the warming of the sea is reflected in the physiological performance and ultimately the seasonal cycle in flesh weight of the ark shell in different manners between winter and summer: physiological benefits and advanced weight gain vs. heat stress and progressive weight loss (Ning et al., 2021; Zou et al., 2021), respectively. Our measurements revealed the absence of seasonal temperature acclimation in the physiological processes of A. kagoshimensis. The resulting mechanistic adjustment of energy balance based on feeding, assimilation, excretion, and metabolism across thermal conditions is likely to explain the recently observed advancement in its seasonal biological cycle (growth, gametogenic development, and spawning) (Park et al., 2011), further supporting the failure in spat collection for culturing due to the advancement and thereby the expansion of spawning period, and the summer mass mortality observed at the culturing sites. A similar pattern of interannual variation in the seasonal weight cycle has been reported in other bivalves (Cerastoderma edule, Navarro et al., 1989; Crassostrea gigas, Ren et al., 2003; Mizuhopecten yessoensis, Baba et al., 2009), reflecting changes in food availability. Food availability (both quantity and quality) has been considered another major environmental factor contributing to physiological adjustment of bivalves (Bayne and Newell, 1983; Gosling, 2015). In the present study, relatively low concentrations and less variable composition of suspended particulate matter in the subtidal waters throughout the experimental occasions compared to adjacent intertidal area allowed us to evaluate seasonal variation of temperature-dependent physiological traits in relation to food acquisition and energy expenditure in clams (Ibarrola et al., 2008). Further information on the combined effects of temperature and food availability on the physiological mechanisms of the clam is needed to understand better their energy dynamism in relation to climate forcing in a warming world (Kang et al., 2016).
Data availability statement
The raw data supporting the conclusions of this article will be made available by the authors, without undue reservation.
Author contributions
HK and C-KK designed the experiments and identified overarching research goals. HK, BL, and C-KK wrote the manuscript. JS and IL arranged specimen collection. HK, JS, and CK conducted field observations and designed and performed physiological measurements. HK, JS, and CK contributed to laboratory analyses. HK, BL, and C-KK analyzed data and performed statistics. IL contributed to specimen collection and aquarium facilities and involved in funding acquisition. All authors reviewed the first draft and approved the submitted version.
Funding
This work was supported by the National Research Foundation of Korea (NRF) grant funded by the Korea government (MSIT) (No. 2021R1C1C2006581) and by the Korea Institute of Marine Science & Technology (KIMST) Promotion [grant no. 20200504].
Acknowledgments
Special thanks should be given to Southeast Sea Fisheries Research Institute, National Institute of Fisheries Science, Korea, for providing the facilities for ecophysiological measurements.
Conflict of interest
The authors declare that the research was conducted in the absence of any commercial or financial relationships that could be construed as a potential conflict of interest.
Publisher’s note
All claims expressed in this article are solely those of the authors and do not necessarily represent those of their affiliated organizations, or those of the publisher, the editors and the reviewers. Any product that may be evaluated in this article, or claim that may be made by its manufacturer, is not guaranteed or endorsed by the publisher.
References
Baba K., Sugawara R., Nitta H., Endou K., Miyazono A. (2009). Relationship between spat density, food availability, and growth of spawners in cultured Mizuhopecten yessoensis in funka bay: concurrence with El niño southern oscillation. Can. J. Fish. Aquat. Sci. 66, 6–17. doi: 10.1139/F08-183
Bao B., Ren G. (2014). Climatological characteristics and long-term change of SST over the marginal seas of China. Cont. Shelf. Res. 77, 96–106. doi: 10.1016/j.csr.2014.01.013
Bayne B. L., Newell R. C. (1983). “Physiological energetics of marine Mollusca,” in The Mollusca, vol. 4. Eds. Saleuddin A. S. M., Wilbur K. M. (New York: Academic Press), 407–515.
Belkin I. M. (2009). Rapid warming of large marine ecosystem. Prog. Oceanogr. 81, 207–213. doi: 10.1016/j.pocean.2009.04.011
Bligh E. G., Dyer W. F. (1959). A rapid method of total lipid extraction and purification. Can. J. Biochem. Physiol. 37, 911–917. doi: 10.1139/y59-099
Bopp L., Resplandy L., Orr J. C., Doney S. C., Dunne J. P., Gehlen M., et al. (2013). Multiple stressors of ocean ecosystems in the 21st century: projections with CMIP5 models. Biogeosciences 10, 6225–6245. doi: 10.5194/bg-10-6225-2013
Bougrier S., Collet B., Geairon P., Geffard O., Héral M., Deslous-Paoli J. M. (1998). Respiratory time activity of the Japanese oyster Crassostrea gigas (Thunberg). J. Exp. Mar. Biol. Ecol. 219, 205–216. doi: 10.1016/S0022-0981(97)00181-0
Bougrier S., Geairon P., Deslous-Paoli J. M., Bacher C., Jonquières G. (1995). Allometric relationships and effects of temperature on clearance and oxygen consumption rates of Crassostrea gigas (Thunberg). Aquaculture 134, 143–154. doi: 10.1016/0044-8486(95)00036-2
Dubois M., Gilles K. A., Hamilton J. K., Rebecs P. A., Smith F. (1956). Colorimetric method for the determination of sugars and related substances. Anal. Chem. 28, 350–356. doi: 10.1021/ac60111a017
Dunstan P. K., Foster S. D., King E., Risbey J., O’kane T. J., Monselesan D., et al. (2018). Global patterns of change and variation in sea surface temperature and chlorophyll a. Sci. Rep. 8, 14624. doi: 10.1038/s41598-018-33057-y
Elliot J. M., Davison W. (1975). Energy equivalents of oxygen consumption in animal energetics. Oecologia. (Berl.). 19, 195–201. doi: 10.1007/BF00345305
Feng Y. W., Li Q., Kong L. F. (2009). Isolation and characterization of 14 polymorphic microsatellite loci in the ark shell Scapharca subcrenata (Bivalvia: Arcidae). Conserv. Genet. 10, 1125–1127. doi: 10.1007/s10592-008-9725-8
Filgueira R., Labarta U., Fernandez-Reiriz M. J. (2006). Flow-through chamber method for clearance rate measurements in bivalves: design and validation of individual chambers and mesocosm. Limnol. Oceanogr. Methods 4, 284–292. doi: 10.4319/lom.2006.4.284
Filgueira R., Roslan R., Grant J. (2011). A comparison of scope for growth (SFG) and dynamic energy budget (DEB) models applied to the blue mussel (Mytilus edulis). J. Sea. Res. 66, 403–410. doi: 10.1016/j.seares.2011.04.006
Giomi F., Pörtner H. O. (2013). A role for haemolymph oxygen capacity in heat tolerance of eurythermal crabs. Front. Physiol. 4. doi: 10.3389/fphys.2013.00110
Glazier D. S. (2009). Activity affects intraspecific body-size scaling of metabolic rate in ectothermic animals. J. Comp. Physiol. B. 179, 821–828. doi: 10.1007/s00360-009-0363-3
Gnaiger E. (1983). “Calculation of energetic and biochemical equivalents of respiratory oxygen consumption,” in Polarographic oxygen sensors. Eds. Gnaiger E., Forstner H. (Belin: Springer-Verlag), 337–345. Appendix C.
Hatton S., Hayden B. J., James M. R. (2005). The effects of food concentration and quality on the feeding rates of three size classes of the GreenshellTM mussel, Perna canaliculus. Hydrobiologia 548, 23–32. doi: 10.1007/s10750-005-2185-y
Holm-Hansen O., Lorenzen C. J., Holmes R. W., Strickland J. D. (1965). Fluorometric determination of chlorophyll. J. Conseil. 30, 3–15. doi: 10.1093/icesjms/30.1.3
Hong H. K., Donaghy L., Park H.-S., Choi K.,.-S. (2014). Influence of reproductive condition of the Manila clam ruditapes philippinarum on hemocyte parameters during early post-spawning period. Aquaculture 434, 241–248. doi: 10.1016/j.aquaculture.2014.08.019
Hong H. K., Jeung H.-D., Kang H.-S., Choi K.,.-S. (2020). Seasonal variations in the hemocyte parameters, gonad development, energy storage and utilization of the giant honeycomb oyster Hyotissa hyotis (Linnaeus 1758) in jeju island off the south coast of Korea. Aquacult. Rep. 17, 100299. doi: 10.1016/j.aqrep.2020.100299
Ibarrola I., Larretxea X., Navarro E., Iglesias J. I. P., Urrutia M. B. (2008). Effects of body-size and season on digestive organ size and the energy balance of cockles fed with a constant diet of phytoplankton. J. Comp. Physiol. B. 178, 501–514. doi: 10.1007/s00360-007-0243-7
Iglesias J. I. P., Navarro E. (1991). Energetics of growth and reproduction in cockles (Cerastoderma edule): seasonal and age dependent variations. Mar. Biol. 111, 359–368. doi: 10.1007/BF01319407
Jørgensen C. B. (1990). Bivalve filter feeding: Hydrodynamics, bioenergetics, physiology and ecology (Fredensborg: Olsen & Olsen).
Jørgensen C. B. (1996). Bivalve filter feeding revisited. Mar. Ecol. Prog. Ser. 142, 287–302. doi: 10.3354/meps142287
Jasen J. M., Pronker A. E., Kube S., Sokolowski A., Sola J. C., Marquiegui M. A., et al. (2007). Geographic and seasonal patterns and limits on the adaptive response to temperature of European mytilus spp. and Macoma balthica populations. Oecologia 154, 23–34. doi: 10.1007/s00442-007-0808-x
Jiang Y., Jiao H., Sun P., Yin F., Tang B. (2020). Metabolic response of Scapharca subcrenata to heat stress using GC/MS-based metabolomics. PeerJ 8, e8445. doi: 10.7717/peerj.8445
Jung S. (2008). Spatial variability in long-term changes of climate and oceanographic conditions in Korea. J. Environ. Biol. 29, 519–529.
Kang Y.-Q. (2000). Warming trend of coastal waters of Korea during recent 60 years, (1936–1995). J. Fish. Sci. Tech. 3, 173–179.
Kang H. Y., Lee Y. J., Choi K. S., Park H. J., Yun S. G., Kang C. K. (2016). Combined effects of temperature and seston concentration on the physiological energetics of the Manila clam Ruditapes philippinarum. PloS One 11, e0152427. doi: 10.1371/journal.pone.0152427
Kang C. K., Lee Y. J., Han E., Park H. J., Yun S. G., Lee W. C. (2015). Effects of temperature and body size on the physiological energetics of the stalked sea squirt Styela clava. J. Exp. Mar. Biol. Ecol. 462, 105–112. doi: 10.1016/j.jembe.2014.10.026
Kim H. J., Kang J. S., Jung S. W., Park Y. J. (2019). Changes in planktonic bivalve larvae of Tegillarca granosa and Anadara kagoshimensis in the boseong coastal waters of south Korea. Korean. J. Environ. Biol. 37, 351–361. doi: 10.11626/KJEB.2019.37.3.351
Kim J.-H., Lee H.-M., Cho Y. G., Shin J.-S., Yoo J.-S., Hong H.-K., et al. (2022). Effects of spawning stress on the immune capacity of blood cockle Tegillarca granosa occurring on the south coast of Korea. Fish. Shellfish. Immunol. 120, 15–22. doi: 10.1016/J.FSI.2021.11.013
Kim S. Y., Shin Y. K., Lim H. K., Lee W. C. (2008). Gonadal development and reproductive cycle of the ark shell, Scapharca subcrenata (bivalvia: arcidae) from yeoja bay. J. Aquacult. 21, 252–258.
Kittner C., Riisgård H. U. (2005). Effect of temperature on filtration rate in the mussel Mytilus edulis: no evidence for temperature compensation. Mar. Ecol. Prog. Ser. 305, 147–152. doi: 10.3354/MEPS305147
KOEM (Korea Marine Environment Management Corporation) (2019). Annual report of marine ecosystem research in Korean waters (Sejong-si, Republic of Korea: Ministry of Oceans and Fisheries).
KOSIS (Korea Statistical Information Service) (2019). Fishery. url:http://kosis.kr. Statistics Korea, Daejeon, Republic of Korea.
Lee J. H. (1998). A study on sexual maturation of the ark shell, Scapharca subcrenata lischke. Korean. J. Malacol. 14, 91–102.
Lee Y.-J., Han E., Wilberg M. J., Lee W. C., Choi K.-S., Kang C. K. (2018). Physiological processes and gross energy budget of the submerged longline-cultured pacific oyster Crassostrea gigas in a temperate bay of Korea. PloS One 13, e0199752. doi: 10.1371/journal.pone.0199752
Lima F. P., Wethey D. S. (2012). Three decades of high-resolution coastal sea surface temperatures reveal more than warming. Nat. Commun. 3, 704. doi: 10.1038/ncomms1713
Lowry O. M., Roseborough N. I., Farrand A. L., Randall R. J. (1951). Protein measurement with folin phenol reagent. J. Biol. Chem. 193, 263–275.
MacDonald B. A., Bricelj V. M., Shumway S. E. (2016). Physiology: energy acquisition and utilization, in scallops biology, ecology, aquaculture, and fisheries. 3rd ed. Eds. Shumway S. E., Parsons G. J. (Amsterdam: Elsevier), 301–353.
Marsh J. B., Weinstein D. B. (1966). Simple charring method for determination of lipid. J. Lipid Res. 7, 574–576. doi: 10.1016/S0022-2275(20)39274-9
MIFAFF (Ministry of Food, Agriculture, Forestry and Fisheries, Korea) (2010). Food, agriculture, forestry and fisheries statistical yearbook. MIFAFF, Sejong, Republic of Korea. 417–421.
Min H.-S., Kim C.-H. (2006). Interannual variability and long-term trend of coastal sea surface temperature in Korea. Ocean. Polar. Res. 28, 415–423. doi: 10.4217/OPR.2006.28.4.415
MOF (Ministry of Oceans and Fisheries, Korea) (2019). Final reports: Investigation of the cause of the decline in ark shell aquaculture production (Report no. 11-1192000-001210-01). MOF, Sejong, Republic of Korea.
Nakamura Y. (2005). Suspension feeding of the ark shell Scapharca subcrenata as a function of environmental and biological variables. Fish. Sci. 71, 875–883. doi: 10.1111/j.1444-2906.2005.01040.x
Navarro E., Iglesias J. I. P., Larrañaga A. (1989). Interannual variation in the reproductive cycle and biochemical composition of the cockle Cerastoderma edule from mundaca estuary (Biscay, north Spain). Mar. Biol. 101, 503–511. doi: 10.1111/j.1444-2906.2005.01040.x
Nespolo R. F., Lardies M. A., Bozinovic F. (2003). Intrapopulational variation in the standard metabolic rate of insects: repeatability, thermal dependence and sensitivity (Q10) of oxygen consumption in a cricket. J. Exp. Biol. 206, 4309–4315. doi: 10.1242/jeb.00687
Newell R. I. E., Bayne B. L. (1980). Seasonal changes in the physiology, reproductive condition and carbohydrate content of the cockle Cardium (=Cerastoderma) edule (Bivalvia: Cardiidae). Mar. Biol. 56, 11–19. doi: 10.1007/BF00390589
Newell R. C., Johnson L. G., Kofoed L. H. (1977). Adjustment of the components of energy balance in response to temperature change in Ostrea edulis. Oecologia. (Berlin). 30, 97–110. doi: 10.1007/BF00345414
Newell R. C., Kofoed L. H. (1977). Adjustment of the components of energy balance in the gastropod Crepidula fornicata in response to thermal acclimation. Mar. Biol. 44, 275–286. doi: 10.1007/BF00387708
NFRDI (National Fisheries Research and Development Institute) (2010). Long-term survey of environmental conditions of fishing ground (Seoul, Republic of Korea: Ministry for Food, Agriculture, Forestry and Fisheries).
Ning J., Zou D., Lu X., Cao W., Chen M., Liu B., et al. (2021). Transcriptomic analyses provide insights into the adaptive responses to heat stress in the ark shells, scapharca subcrenata. Comp. Biochem. Physiol. Part D. Genomics Proteomics 38, 100813. doi: 10.1016/j.cbd.2021.100813
Packard G. C., Boardman T. J. (1987). “The misuse of ratios to scale physiological data that vary allometrically with body size,” in New directions in ecological physiology. Eds. Feder M. E., Bennett A. F., Burggren W. W., Huey R. B. (Cambridge: Cambridge University Press), 216–239.
Park H. J., Lee W. C., Choy E. J., Choi K.-S., Kang C. K. (2011). Reproductive cycle and gross biochemical composition of the ark shell Scapharca subcrenata (Lischke 1869) reared on subtidal mudflats in a temperate bay of Korea. Aquaculture 322–323, 149–157. doi: 10.1016/j.aquaculture.2011.10.015
Parsons T. R., Maita Y., Lalli C. M. (1984). A manual of biological and chemical methods for seawater analysis (Oxford: Pergamon Press).
Philippart C. J. M., van Belijswijk J. D. L., Kromkamp J. C., Zuur A. F. (2014). Reproductive phenology of coastal marine bivalves in a seasonal environment. J. Plankton. Res. 36, 1512–1527. doi: 10.1093/plankt/fbu073
Pouvreau S., Bourles Y., Lefebvre S., Gangnery A., Alunno-Bruscia A. (2006). Application of a dynamic energy budget model to the pacific oyster, Crassostrea gigas, reared under various environmental conditions. J. Sea. Res. 56, 156–167. doi: 10.1016/j.seares.2006.03.007
Ren J. S., Marsden I. D., Ross A. H., Schiel D. R. (2003). Seasonal variation in the reproductive activity and biochemical composition of the pacific oyster (Crassostrea gigas) from the Marlborough sounds, new Zealand. N. Z. J. Mar. Freshw. Res. 37, 171–182. doi: 10.1080/00288330.2003.9517155
Riisgård H. U. (1988). Efficiency of particle retention and filtration rate in 6 species of northeast American bivalves. Mar. Ecol. Prog. Ser. 45, 217–223. doi: 10.3354/meps045217
Sarà G., Romano C., Widdows J., Staff F. J. (2008). Effect of salinity and temperature on feeding physiology and scope for growth of an invasive species (Brachidontes pharaonis - Mollusca: Bivalvia) within the Mediterranean Sea. J. Exp. Mar. Biol. Ecol. 363, 130–136. doi: 10.1016/j.jembe.2008.06.030
Schipper L. A., Hobbs J. K., Rutledge S., Arcus V. L. (2014). Thermodynamic theory explains the temperature optima of soil microbial processes and high Q10 values at low temperatures. Glob. Change Biol. 20, 3578–3586. doi: 10.1111/gcb.12596
Schmidt-Nielsen K. (1983). Animal physiology: Adaptation and environment (Cambridge: Cambridge University Press).
Seebarcher F., White C. R., Franklin C. E. (2015). Physiological plasticity increases resilience of ectothermic animals to climate change. Nat. Clim. Change 5, 61–66. doi: 10.1038/nclimate2457
Shin Y. K., Lee W. C., Kim S. Y., Jun J. C., Kim E. O. (2011). Variation in physiological energetics of blood cockle Scapharca subcrenata (Bivalvia: Arcidae) from yeoja bay, south coast of Korea. Korean. J. Malacol. 27, 205–211. doi: 10.9710/kjm.2011.27.3.205
Somero G. N. (2012). The physiology of global change: linking patterns to mechanisms. Annu. Rev. Mar. Sci. 4, 39–61. doi: 10.1146/annurev-marine-120710-100935
Van der Have T. M., De Jong G. (1996). Adult size in ectotherms: temperature effects on growth and differentiation. J. Theor. Biol. 183, 329–340. doi: 10.1006/jtbi.1996.0224
Wang J., Russell B. D., Ding M. W., Dong Y. W. (2018). Ocean acidification increases the sensitivity of and variability in physiological responses of an intertidal limpet to thermal stress. Biogeosciences 15, 2803–2817. doi: 10.5194/bg-15-2803-2018
Widdows J., Bayne B. L. (1971). Temperature acclimation of Mytilus edulis with reference to its energy budget. J. Mar. Biol. Ass. U.K. 51, 827–843. doi: 10.1017/S0025315400018002
Wilbur A. E., Hilbish T. J. (1989). Physiological energetics of the ribbed mussel Geukensia demissa (Dillwyn) in response to increased temperature. J. Exp. Mar. Biol. Eco. 131, 161–170. doi: 10.1016/0022-0981(89)90005-1
Winberg G. G. (1956). Rate of metabolism and food requirements of fishes. belorussian state university, Minsk. Fish. Res. Board. Can. Transl. Ser. No. 194. 251
Winter J. E. (1978). A review on the knowledge of suspension-feeding in lamellibranchiate bivalves, with special reference to artificial aquaculture systems. Aquaculture 13, 1–33. doi: 10.1016/0044-8486(78)90124-2
Woodin S. A., Hilbish T. J., Helmuth B., Jones S. J., Wethey D. S. (2013). Climate change, species distribution models, and physiological performance metrics: predicting when biogeographic models are likely to fail. Ecol. Evol. 3, 3334–3346. doi: 10.1002/ece3.680
Zhao W., Han T., Wei J., Pu H., Wang S., Yuan X. (2016). Effect of acute temperature change on antioxidant enzyme activities and lipid peroxidation in the ark shell Scapharca subcrenata (Lischke 1869). J. Shellfish. Res. 35, 399–403. doi: 10.2983/035.035.0214
Keywords: Scapharca subcrenata, energetic physiology, thermal effect, temperature acclimation, coastal warming, Korean coast
Citation: Kang HY, Seong J, Kim C, Lee B G, Lee IT and Kang C-K (2022) Seasonal energetic physiology in the ark shell Anadara kagoshimensis in response to rising temperature. Front. Mar. Sci. 9:981504. doi: 10.3389/fmars.2022.981504
Received: 29 June 2022; Accepted: 19 August 2022;
Published: 13 September 2022.
Edited by:
Mark Botton, Fordham University, United StatesReviewed by:
Maria Christina Vasquez, Loyola Marymount University, United StatesHyun-Ki Hong, Jeju National University, South Korea
Copyright © 2022 Kang, Seong, Kim, Lee, Lee and Kang. This is an open-access article distributed under the terms of the Creative Commons Attribution License (CC BY). The use, distribution or reproduction in other forums is permitted, provided the original author(s) and the copyright owner(s) are credited and that the original publication in this journal is cited, in accordance with accepted academic practice. No use, distribution or reproduction is permitted which does not comply with these terms.
*Correspondence: Chang-Keun Kang, Y2trYW5nQGdpc3QuYWMua3I=