- 1Dipartmento di Scienze della Vita e dell’Ambiente, Università Politecnica delle Marche, Ancona, Italy
- 2Istituto per i Polimeri Compositi e Biomateriali (IPCB), Consiglio Nazionale delle Ricerche (CNR), Pozzuoli, Italy
Textile microfibers (MFs) have natural (e.g. cotton, wool and silk) or synthetic origin (e.g. polyester and polyamide), and are increasingly documented in the marine environment. Knowledge on their biological effects in marine organisms is still limited, and virtually unexplored is their capability to modulate the responsiveness toward other stressors, including those of emerging relevance under global changes scenario. With such background, the aims of this study were to i) determine the ingestion and biological effects of MFs, discriminating between synthetic and natural ones, and ii) elucidate the possibility that MFs alter the responsiveness toward additional stressors occurring at a later stage, after exposure. Adult mussels Mytilus galloprovincialis were exposed for 14 days to a high but still environmentally realistic concentration of 50 MFs L-1 of either polyester (618 ± 367 µm length, 13 ± 1 µm diameter), polyamide (566 ± 500 µm length, 11 ± 1 µm in diameter) or cotton (412 ± 342 µm length, 16 ± 4 µm diameter). After the exposure, mussels were left for 7 days to recover at control temperature (23°C) or exposed to a heatwave condition (27°C). At the end of each phase (exposure – recovery – heat stress), MFs ingestion-elimination was evaluated, along with a wide panel of biological responses, including neuro-immune and antioxidant systems alterations, lipid metabolism and onset of cellular damages. Results were elaborated through a Weight of Evidence approach to provide synthetic hazard indices based on both the magnitude and toxicological relevance of observed variations. Beside limited differences in retention and elimination of MFs, biological analyses highlighted disturbance of the immune system and demand of protection toward oxidative insult, particularly evident in mussels exposed to synthetic-MFs. Carry-over effects were observed after 7 days of recovery: organisms that had been previously exposed to MFs showed a higher susceptibility of the neuroendocrine-immune system and lipid metabolism to thermal stress compared to un-exposed mussels. Overall, this study provided evidence of direct cellular effects of MFs, emphasizing differences between synthetic and natural ones, and highlighted their capability to modulate organisms’ susceptibility toward additional stressors, as those predicted for future changes in marine ecosystems.
Introduction
Textile microfibers (MFs) are receiving increased attention as pollutants of emerging concern, being widely distributed in all terrestrial and aquatic ecosystems (Kwak et al., 2022).
Textile fabrics for clothes, agricultural, industrial and domestic use are estimated to be the main sources of MFs which can be released into the environment through their entire lifecycle, i.e. during manufacturing, use, and after disposal (Liu et al., 2019).
Approximately 0.48-4.28 million metric tonnes of MFs enter the environment every year and, similarly to the trend of global textiles production, this input could increase by 54% within 2030 (Sustainable Investment Institute, 2022). MFs end-up in marine environment via air or water transport, through wastewater treatment plants effluents, rain, riverine and agricultural run-offs (Liu et al., 2021), which are the main factors affecting MFs levels in coastal areas while ocean currents drive their distribution and accumulation in specific convergence zones. Overall, MFs are detected in surface layer at levels ranging from less than one item L-1 up to few tens items L-1 (Bagaev et al., 2017; Gago et al., 2018; Suaria et al., 2020; Herzke et al., 2021; Di Mauro et al., 2022), but substantial amounts are probably suspended in the water column or exported to the seafloor (Suaria et al., 2020; Di Mauro et al., 2022).
Almost two-thirds of all textiles are made from petroleum (synthetic-based fibers), with polyester dominating over other polymers, as polyamide (also named nylon), acrylic and polypropylene; the remaining fibers are processed from cellulose or animal sources such as cotton, silk and wool (natural-based fibers) (Textile Exchange, 2021). Despite the marked prevalence in production of synthetic vs natural fibers, 60–80% of MFs in the world’s oceans are of cellulosic nature (Suaria et al., 2020), including those mostly ingested by marine organisms (Avio et al., 2020). Different reasons could contribute to explain this discrepancy, including a higher shedding rate observed for natural fibers compared to synthetic ones during both laundering and daily use of textiles (De Falco et al., 2019; De Falco et al., 2020). In addition, despite natural and semi-synthetic fibers (regenerated cellulose like rayon and viscose) are known to degrade faster than synthetic ones in well-controlled experiments (Gaylarde et al., 2021), the limited data available under marine realistic conditions suggest a “longer-than-expected” environmental persistence (Suaria et al., 2020). Degradation time might be further influenced by chemical additives used in production and textiles processing (Sørensen et al., 2021), contributing to additional concerns on the possible toxicity of MFs to biota (Athey and Erdle, 2022).
The number and frequency of ingestion in marine biota is much greater for MFs than for the other anthropogenic microplastics, like fragments, films or pellets (Avio et al., 2020). Due to their length-to-diameter ratio coupled to the possible formation of fibrous aggregates within the digestive systems, specific detrimental effects were observed in aquatic species such as gastrointestinal injuries, mechanical disturbance, altered feeding and filtering activity, growth and survival (reviewed in Singh et al., 2020). Despite their emerging widespread abundance and predominance in biota, our knowledge on the molecular and cellular effects of MFs is still limited; indeed, a great effort has been carried out in the context of microplastics (i.e. Avio et al., 2015; Paul-Pont et al., 2016; Ribeiro et al., 2017; Barboza et al., 2018; Pittura et al., 2018; Tang et al., 2020; Missawi et al., 2021; Shang et al., 2021; Trestrail et al., 2021; Romdhani et al., 2022), but typically without considering microfibers, certainly not the natural and semi-synthetic ones. Among the available literature, dimensional characteristics of MFs have shown to influence their ingestion and biological effects: a preferential ingestion of MFs of 100-250µm was observed in the Asian clam exposed to PEST MFs for 2 days at a concentration of 1000 items L-1 (Li et al., 2019), and length dependent effects were observed in Mytilus galloprovincialis with harsher genotoxic damages caused by 100 µm long PET-MFs compared to smaller ones (Choi et al., 2021). Accordingly, 106.5 ± 74.8 μm PET-MFs caused digestive tubules atrophy, sex hormones and gonad development alterations, and oxidative imbalance in M. galloprovincialis in a long-term experiment (Choi et al., 2022); a transient oxidative disturbance was caused also by PA-MFs but without any effects on immune system and DNA integrity of mussels (Cole et al., 2020), thus suggesting some polymeric specificities in observed effects.
In this respect, a better understanding of the specific biological effects of MFs is urgent, focusing on possible differences between synthetic and natural fibers, as well as the possibility that the effects caused by MFs ingestion trigger vulnerability and susceptibility of organisms toward additional stressors, including those occurring at a later stage after MFs exposure. Indeed, organisms’ health status is challenged by multiple stressors, not necessarily acting in synchronous mode, but rather through additive, synergistic or antagonistic effects (Crain et al., 2008): even a limited disturbance provoked by a single factor may interfere in a more complex network of biological responsiveness (Benedetti et al., 2022).
In actual scenarios, marine ecosystems are increasingly subjected to extreme heatwaves, among the most relevant emerging climate-related stressors: these represent acute increases of seawater temperature, which act as pulsed and transient events lasting from a few days up to several months (Frölicher et al., 2018; Oliver et al., 2018). The impacts of marine heatwaves may be particularly relevant in coastal ecosystems, where the anthropogenic footprint is significant, and the loads of contaminants are prominent (Lu et al., 2018): indeed, it is now well documented that the effects of traditional and emerging contaminants, as trace metals, PAHs and pharmaceuticals, may be inflated by heat stress, with adverse outcomes on neuro-immune and antioxidant systems of bivalves (Kamel et al., 2012; Bagwe et al., 2015; Coppola et al., 2017; Nardi et al., 2022), deriving from stress-defense mechanisms impairment and higher energy demand to sustain these.
In this context, the aim of the present study was to compare the effects of synthetic (polyester and polyamide) and natural (cotton) microfibers in the Mediterranean mussel Mytilus galloprovincialis integrating results on ingestion and elimination of MFs with the measurement of a wide spectrum of cellular responses reflecting the possible biological targets of MFs: similarly to MPs, also MFs could be recognized by immunocytes as foreign particles, altering their phagocytic activity and subpopulations ratio, and promoting pro-inflammatory pathways which trigger ROS production and oxidative pressure, which may result in cellular and genotoxic damages (Avio et al., 2015; Pittura et al., 2018; Benedetti et al., 2022). In mussels, the activation of stress-defense mechanisms is mediated, among others, by the cholinergic system (Liu et al., 2018a) and sustained by energy reserves like neutral lipids deriving from beta-oxidation of fatty acids and stored in the digestive gland (Bocchetti and Regoli, 2006). Based on such hypotheses and mechanisms of disturbance, biological processes analyzed in this study included immunocytes alterations (granulocytes on hyalinocytes ratio, phagocytosis rate, haemocytes lysosomal membrane stability and micronuclei frequency), cholinergic function (acetylcholinesterase activity in haemolymph and gills), antioxidant defenses (total oxyradical scavenging capacity toward peroxyl and hydroxyl radical, TOSC ROO• and TOSC HO•, content of total glutathione), accumulation of peroxidation products (lipofuscin) and lipid metabolism (acyl-CoA oxidase activity and neutral lipids content) in the digestive gland.
As additional objective of this study, the onset or persistence of delayed effects, as well as the possibility of MFs to influence sensitivity to other non-synchronous stressors were investigated: after exposure to MFs, mussels were either allowed to recover or subjected to a heatwave simulation, chosen as model secondary stressor. The obtained results were integrated through a quantitative Weight of Evidence approach providing synthetic hazard indices for each experimental condition based on weighted elaboration of toxicological relevance and magnitude of variations observed for all the analyzed biological endpoints (d'Errico et al., 2021). This study was expected to provide novel insights on direct and indirect effects of MFs, the comparison of synthetic and natural fibers, and the relevance of observed biological effects in a long-term perspective under realistic environmental conditions of multiple stressors.
Materials and methods
Microfibers preparation
Microfibers made of polyester (PEST-MFs), polyamide (PA-MFs) and cotton (CO-MFs) were prepared from coloring- and surface treatments-free standard fabrics using a Retsch mill equipped with a 500 µm grid. The length and diameter of MFs were calculated under an optical microscope by averaging the dimensions of 100 MFs of each typology: PEST-MFs were 618 ± 367 µm in length and 13 ± 1 µm in diameter, PA-MFs were 566 ± 500 µm in length and 11 ± 1 µm in diameter and CO-MFs were 412 ± 342 µm in length and 16 ± 4 µm in diameter. PEST-MFs and PA-MFs have the usual morphology of synthetic fibers, with the shape of long thin cylinders, uniform and regular in thickness and with by a smooth surface (Cook, 2001); CO-MFs present the typical convolutions of cotton fibers, characterized by a twisted ribbon form (Houck, 2009).
To confirm the chemical nature of produced MFs, Fourier Transform Infrared (FTIR) spectra were acquired by means of a PerkinElmer Frontier spectrometer, equipped with the Universal ATR accessory, using 16 scans and a resolution of 4 cm-1, over the range 4000-650 cm-1 (Figure 1D–F and detailed in Supplementary Materials). Additionally, thermal properties of MFs were evaluated by Differential Scanning Calorimetry (DSC) using a TA-Q2000 differential scanning calorimeter equipped with an RCS-90 cooling unit (TA Instruments) and by Thermogravimetric Analysis (TGA) carried out on a Pyris 1 TGA analyzer (PerkinElmer), ( and detailed in Supplementary Materials). PEST-, PA- and CO-MFs optical micrographs, FTIR spectra and TGA traces are shown in Figure 1 and analyses detailed in Supplementary Materials.
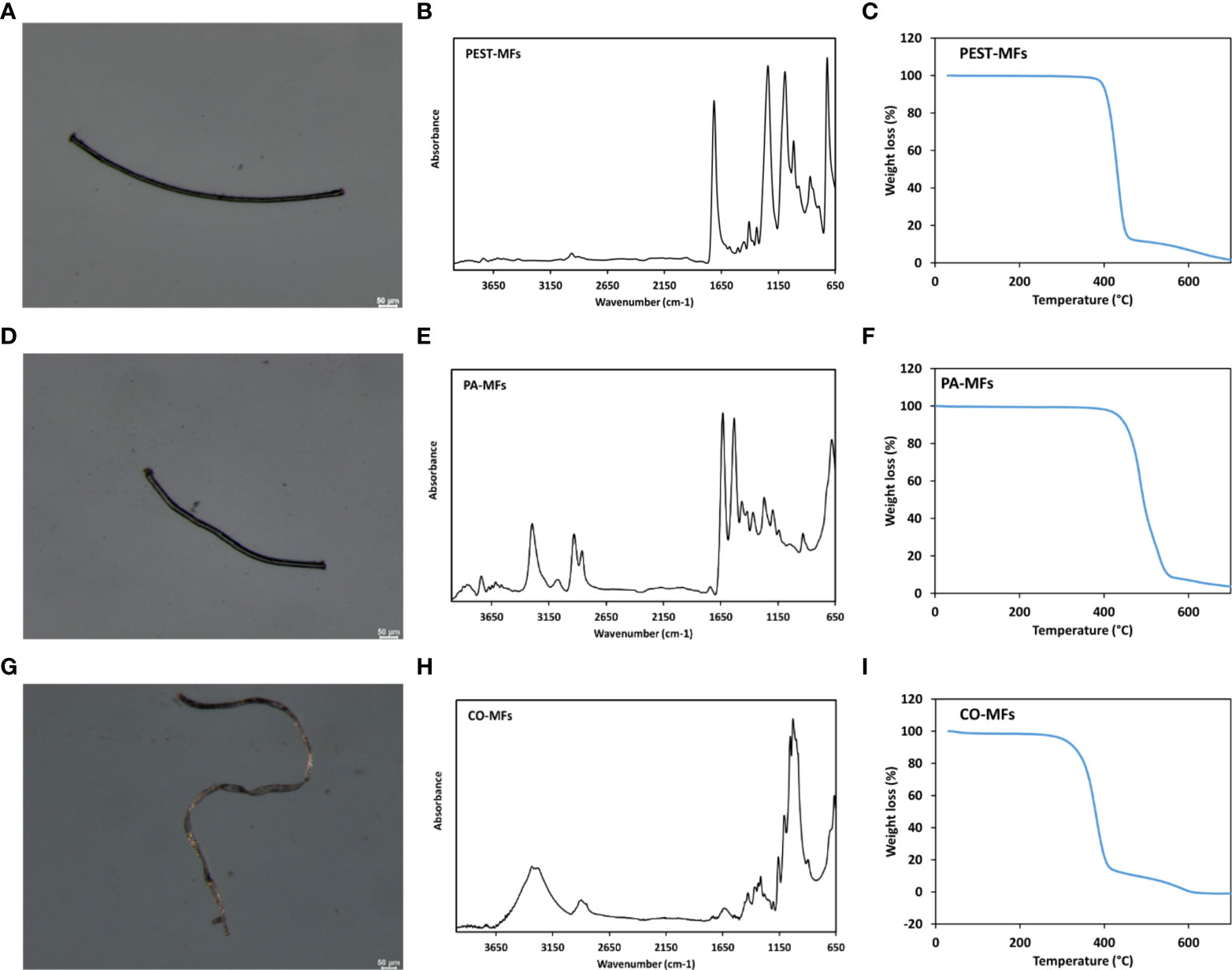
Figure 1 Optical micrographs, FTIR spectra and TGA traces of PEST-MFs (A–C), PA-MFs (D–F) and CO-MFs (G–I) produced for the study.
For each polymer typology, MFs were manually isolated from the stock on aluminum foils using tweezers under a stereomicroscope (GZ 808 Optech) and counted to obtain the number of MFs required for the exposure concentration for mussels at 50 MFs L-1. This value is at the upper end of the of environmental range of MFs extrapolated from available literature (Bagaev et al., 2017; Gago et al., 2018; Suaria et al., 2020; Herzke et al., 2021; Di Mauro et al., 2022), but still environmentally realistic of polluted scenarios and accumulation areas as coastal waters or convergence zones (Bagaev et al., 2017; Herzke et al., 2021; Di Mauro et al., 2022; Queiroz et al., 2022).
Animal collection and experimental setup
Adult Mediterranean mussels Mytilus galloprovincialis (6.6 ± 0.6, mean valves length and standard deviation) were obtained from a shellfish farm in central Adriatic Sea in late June 2020, and immediately transported to laboratory facilities for acclimation in aerated artificial seawater (ASW) at local seasonal environmental conditions of temperature 23°C, pH 8.1, salinity 35 and density 1.028 g cm-3 at 20°C. ASW was prepared by dissolving InstanOcean® salt in ultrapure 0.22µm-filtered water until the desired salinity was reached: this was chosen to avoid natural variability in organic matter content and presence of contaminants that could have been detected in natural seawater (including others than microplastics and microfibers, as e.g. trace metals and PAHs) thus excluding confounding factors and ensuring a higher reproducibility of the experiment. During acclimation, and throughout the study, organisms were fed daily with a commercial mixture of zooplankton for filter-feeding organisms (Brightwell Zooplanktos-S, size range 50–300 μm).
After 10 days of acclimation (T0), a total of 240 mussels were randomly distributed in eight aerated 20L acid-washed glass tanks and exposed for 14 days, in duplicate, to polyester (PEST), polyamide (PA) and cotton (CO) microfibers at 50 items L-1; control organisms were maintained in clean filtered ASW. At the end of the exposure, for the following 7 days organisms were either left to recover in MFs-free ASW at 23°C (recovery hereafter) or exposed to a marine heatwave scenario in MFs-free ASW (27°C, heat stress hereafter), subjecting to heatwave also experimental organisms not previously exposed to MFs (CTL) for comparison. The experiments were designed to highlight the recovery or the onset/persistence of effects after MFs exposure or the possibility that MFs influence responsiveness toward delayed, non-synchronous stressors, as marine heatwaves. The latter was reproduced based on events occurred in Adriatic Sea during summer 2018 and 2019 (www.marineheatwaves.org/tracker, coordinates: 43.625, 13.625, event 104 and 110; more details are given in Supplementary Figure S1). In each tank, 2-4 organisms (2 during the exposure and 4 during the recovery phases) were held in a petri dish to allow for an easy collection of produced biodeposits (pseudofeces and/or feces). Every three days, water was completely changed and, after washing tanks twice with filtered ASW, microfibers were re-dosed and organisms fed; during this procedure, mussels’s biodeposits were collected from Petri dishes with a glass Pasteur pipette and transferred in acid-washed glass containers until processed for evaluating MFs. Mortality was daily checked throughout the acclimation and experimental phase, evaluating responsiveness of organisms to external stimuli, and no dead organism was recorded throughout the whole experiment.
At T0, and after 14 and 21 days, from each experimental condition 20 organisms were sampled and processed for the analyses of MFs and cellular responses. Whole digestive glands of 4 organisms were carefully rinsed with ultrapure water (filtered at 0.22 µm) and individually stored at -20°C until extraction and characterization of MFs. For cellular responses, the digestive gland, gills and haemolymph of 12 organisms were pooled to prepare 4 samples each constituted by tissues of 3 specimens: digestive gland and gills were flash frozen in liquid nitrogen and then stored at -80°C; for haemolymph, withdrawn from the posterior adductor muscle, an aliquot of each sample (~200 µL) was immediately tested for in-vivo immune parameters, while the remaining was flash frozen in liquid nitrogen and stored at -80°C for further analyses of AChE activity. Digestive glands of 4 additional organisms were excised, individually flash frozen and maintained at -80°C for histological analyses.
MFs extraction and characterization
Digestive glands and biodeposits collected during the exposure, recovery and heat stress phases were digested in glass Petri dishes using 15% H2O2 solution for 24h at 40°C (Avio et al., 2020). Petri dishes were microscopically inspected using a stereomicroscope, and microfibers were isolated based on their shape (Figure 1) and color (i.e. uncolored fibers appearing translucent). To confirm PEST, PA and CO nature of extracted MFs, μATR-FTIR characterization was always performed on all isolated MFs throughout the experiment using a Perkin Elmer Spotlight 200i FTIR microscope system, equipped with Spectrum Two and driven by Spectrum 10 software.
Since the use of oxidative agents as hydrogen peroxide has been recently suggested to be destructive toward some typologies of microplastics and microfibers (Athey and Erdle, 2022), the suitability of the proposed methodology was tested by placing known amounts of microfibers of each typology in Petri dishes and treating these according to Avio et al. (2020) with slight modifications (as previously described); before and after the treatment MFs were observed under the stereomicroscope, and IR-spectra acquired. These investigations confirmed that both synthetic (polyester and polyamide) and cotton microfibers were not negatively affected in terms of length and integrity by the method.
Contamination control
Several practices were applied to prevent and check microfibers contamination by external sources and cross-contamination of exposure treatments.
All the procedures for the preparation of MFs, dissection of mussels, MFs extraction and characterization, were performed in a dedicated laboratory with no windows and access allowed only to a single operator. Before and after the operations, the work benches and equipment were cleaned with pure grade ethanol, tweezers and aluminum foils were observed under the stereomicroscope before the use, and experimental tanks were always covered with lids. The use of white lab coats was avoided during the experimental set-up and analyses, to easily highlight potential contamination by colored MFs. ASW and 15% H2O2 solutions were prepared with ultra-pure water and additionally filtered with nitrate acetate membrane with pore size of 0.22 μm before use. In addition, control organisms (mussels not exposed to MFs) were used also to validate results on quantification of MFs ingestion and elimination in exposed organisms: no MFs matching characteristics of those used for the exposure were detected in T0 and control mussels, allowing to exclude cross-contamination.
Biological analyses
The following cellular responses were analyzed in collected tissues through validated protocols, as detailed in Supplementary Materials: immunocytes alterations (granulocytes on hyalinocytes ratio, phagocytosis rate, haemocytes lysosomal membrane stability and micronuclei frequency), cholinergic function (acetylcholinesterase activity in haemolymph and gills), antioxidant defenses (total oxyradical scavenging capacity toward peroxyl and hydroxyl radical, TOSC ROO• and TOSC HO•, content of total glutathione), accumulation of peroxidation products (lipofuscin), lipid metabolism (acyl-CoA oxidase activity and neutral lipids content) in the digestive gland.
Statistical analyses and weighted elaboration
Two-way ANOVA was applied to evaluate effects of the factors “Treatment”, with 4 levels (control, polyester, polyamide and cotton), “Exposure phase”, with 3 levels (exposure, recovery, heat stress) and their interaction (Table 1), after testing for normal distribution (Shapiro-Wilk test) and homogeneity of variances (Levene’s Test). Tukey HSD post-hoc test was used to compare the means of interest.
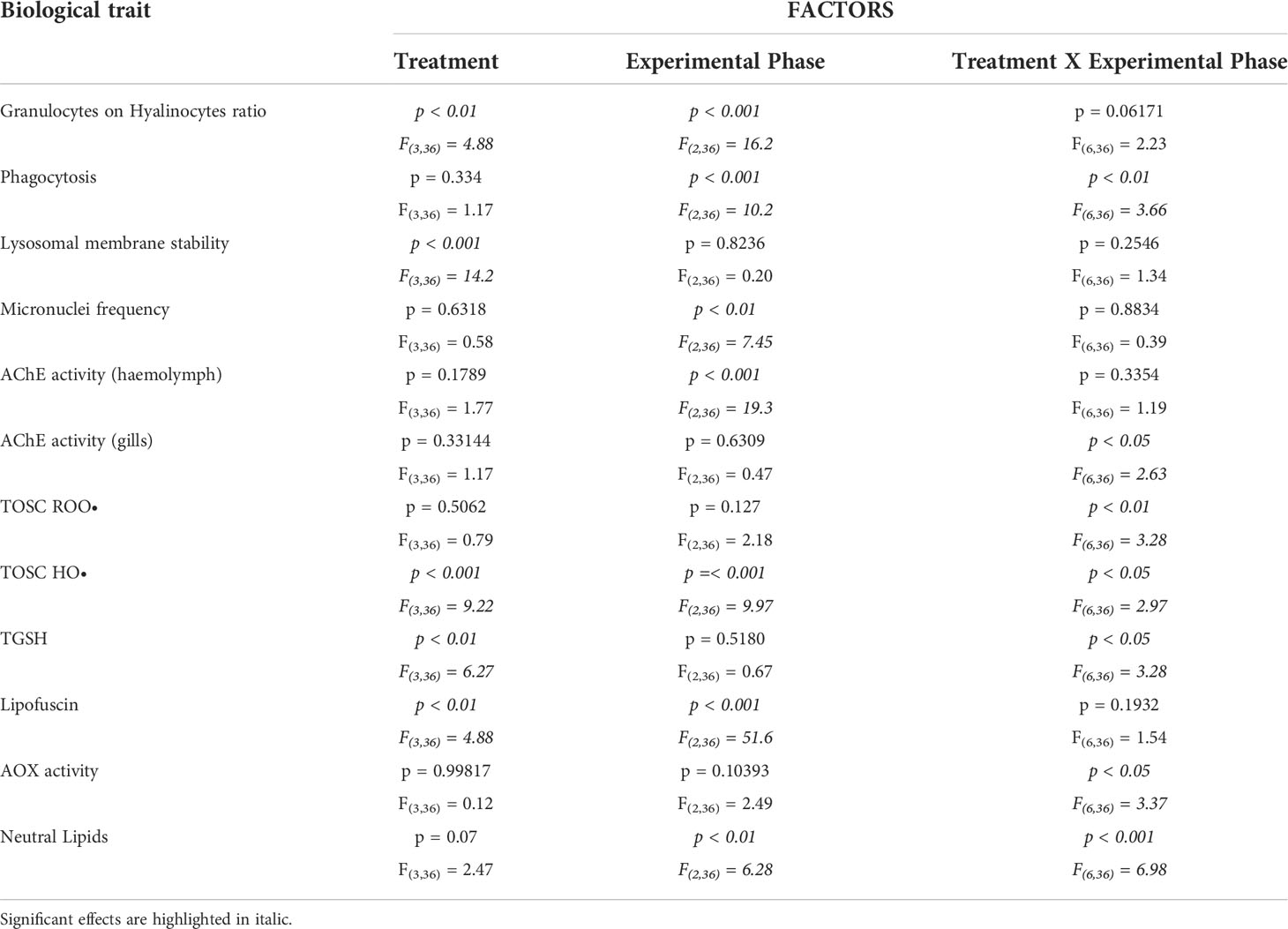
Table 1 Two-way ANOVA results for single factors (Treatment, Experimental Phase) and their interactions; F-values with degrees of freedom and p-values are reported for each factor and interaction for each analyzed biological trait.
The dataset of biological responses was further elaborated through a quantitative Weight Of Evidence (WOE) model to provide synthetic hazard indices for each typology of MF in various experimental phases. Briefly, Hazard Quotients (HQs) are calculated on the basis of number and toxicological relevance of affected responses, statistical significance and magnitude of variation compared to thresholds specific for each analyzed parameter: variations lower or equal to their threshold are not considered, while averaging or adding the summation (Σ) for those responses with variations up to 2-fold or more than 2-fold greater than the specific threshold, respectively (Avio et al., 2015; Pittura et al., 2018; Regoli et al., 2019; Mezzelani et al., 2021; Nardi et al., 2022). The model finally assigns the elaborated HQ to one of five classes of hazard, from Absent to Severe: whole calculations and assumptions are detailed in Supplementary Materials.
Results
Quantification of MFs ingestion and elimination
Analyses on digestive gland collected at the end of the exposure (Figure 2A) revealed that all mussels ingested MFs, but a higher frequency of ingestion was obtained after PA-exposure (75% of organisms containing at least 1 MF), followed by CO (50%) and PEST (25%). After 7 days of recovery or heat stress, MFs were still detected in exposed mussels (25% of ingestion frequency) without differences between the typologies of MFs or exposure to heat stress. Worthy to note, regardless of polymer typology and exposure phase, no more than 1 microfiber was detected in digestive glands of mussels, which thus differed among treatments only in terms of frequency of ingestion. MFs were also detected in biodeposits, with lower levels in CO-treatment compared to other polymer typologies in all experimental phases, as well as in heat stress exposed mussels compared to those recovered at control temperature (Figure 2B).
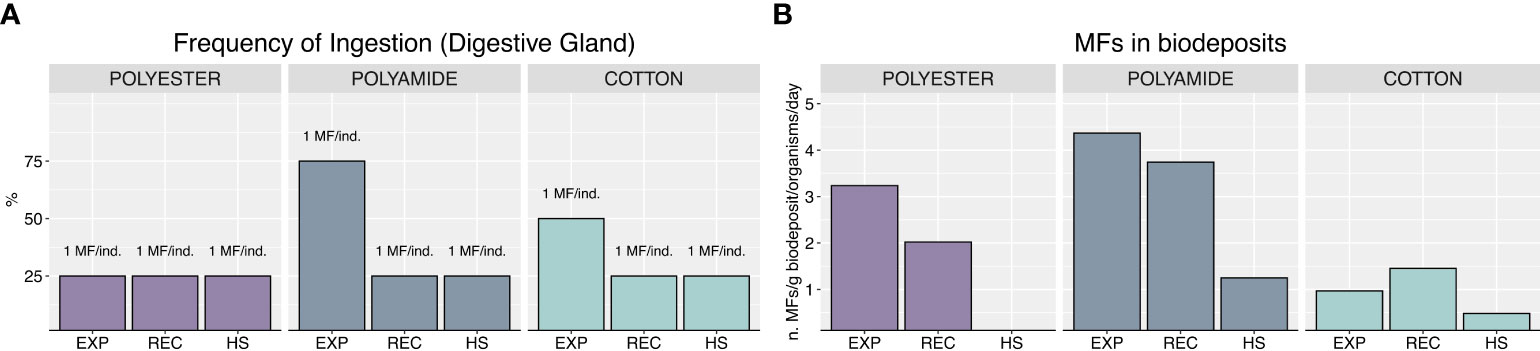
Figure 2 Frequency of ingestion and number of MFs found in samples (A), MFs in biodeposits, expressed as number of MFs per g of biodeposit per organism per day (B).
Immunocytes sub-populations and functional parameters
Granulocytes on hyalinocytes ratio increased under heat stress in all MFs-exposed organisms compared to mussels exposed to HS alone, particularly for PEST and CO (Figure 3A; Table 1).
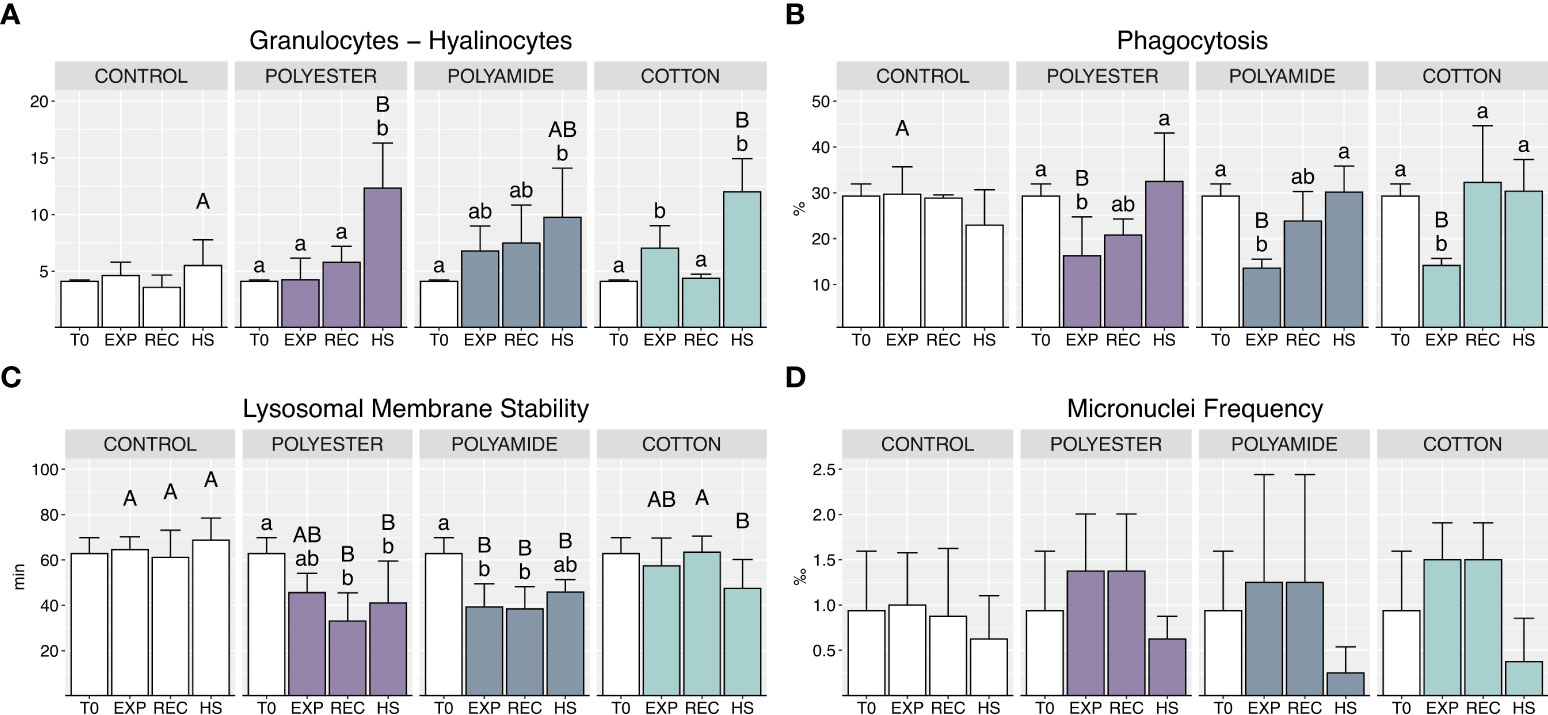
Figure 3 Haemocytes sub-populations and functional parameters: granulocytes – hyalinocytes ratio (A), phagocytosis (B), lysosomal membrane stability (C), micronuclei frequency (D) in control and polyester, polyamide or cotton-exposed mussels measured at T0 and at the end of each experimental phase (EXP, end of MFs-exposure; REC, recovery after MFs-exposure; HS, heat-stress after the MFs-exposure). Upper case letters highlight differences among treatments within the same experimental phase; lower case letters highlight differences among different experimental phases of the same treatment. Values are given as mean ± SD (n=4).
Phagocytosis was significantly reduced during MFs-exposure, independently of polymer typology (Figure 3B; Table 1), while it was efficiently restored during the recovery phase and the heat stress exposure.
A reduction of lysosomal membranes stability (LMS) was caused by PA-exposure (Figure 3C; Table 1), persisting during the recovery period and under heat stress; a delayed alteration of this parameter was observed in PEST-exposed organisms during the recovery phase with no additional effect of heat stress. CO-treated organisms did not exhibit variations of LMS during the exposure and recovery phases but appeared more sensitive during heat stress (HS) with a significant reduction of this parameter compared to mussels not previously exposed to such MFs.
No significant differences were caused in terms of micronuclei frequency by different polymer typologies or exposure conditions (Figure 3D; Table 1).
Cholinergic function in hemolymph and gills
Acetylcholinesterase (AChE) activity in hemolymph was not affected during MFs-exposure (Figure 4A; Table 1), while a slight significant difference was observed in the recovery phase between PA-exposed and control mussels; a generalized decrease of AChE activity appeared under heat stress, but without differences between mussels previously exposed or not to MFs.
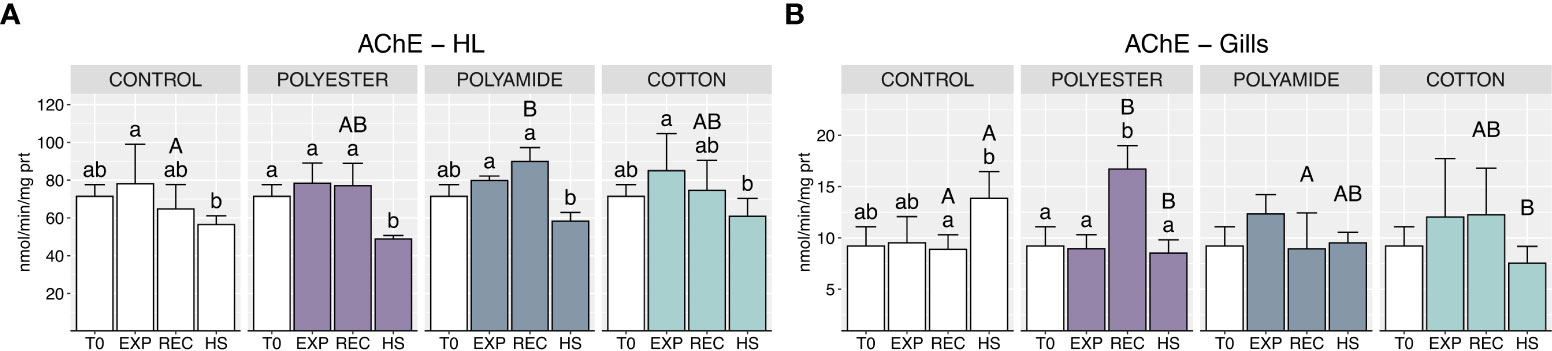
Figure 4 Acetylcholinesterase activity in haemolymph (A) and gills (B) in control and polyester, polyamide or cotton-exposed mussels measured at T0 and at the end of each experimental phase (EXP, end of MFs-exposure; REC, recovery after MFs-exposure; HS, heat-stress after the MFs-exposure). Upper case letters highlight differences among treatments within the same experimental phase; lower case letters highlight differences among different experimental phases of the same treatment. Values are given as mean ± SD (n=4).
Likewise, MFs did not elicit effects on gills AChE activity at the end of the exposure phase (Figure 4B; Table 1), but delayed outcomes were suggested by the significant induction of this enzyme in PEST-exposed organisms after the recovery; heat stress resulted in a lower AChE activity in MFs-exposed organisms compared to mussels exposed to this stressor alone (CONTROL-HS).
Antioxidant defenses and accumulation of peroxidation products
PA-exposed organisms showed an increased oxyradical scavenging capacity toward both peroxyl and hydroxyl radicals at the end of the exposure (Figures 5A, B; Table 1), which returned to control groups levels after the recovery and under heat stress phases. PEST- and CO-exposed organisms did not exhibit variations of TOSC values during both exposure and recovery phases (Figure 5B): heat stress determined a significant decrease of TOSC HO• in PEST-exposed mussels but this effect was comparable to what observed in mussels exposed only to HS. On the other hand, TOSC HO• in CO-treated mussels was higher following heat stress compared to control mussels (Figure 5B).
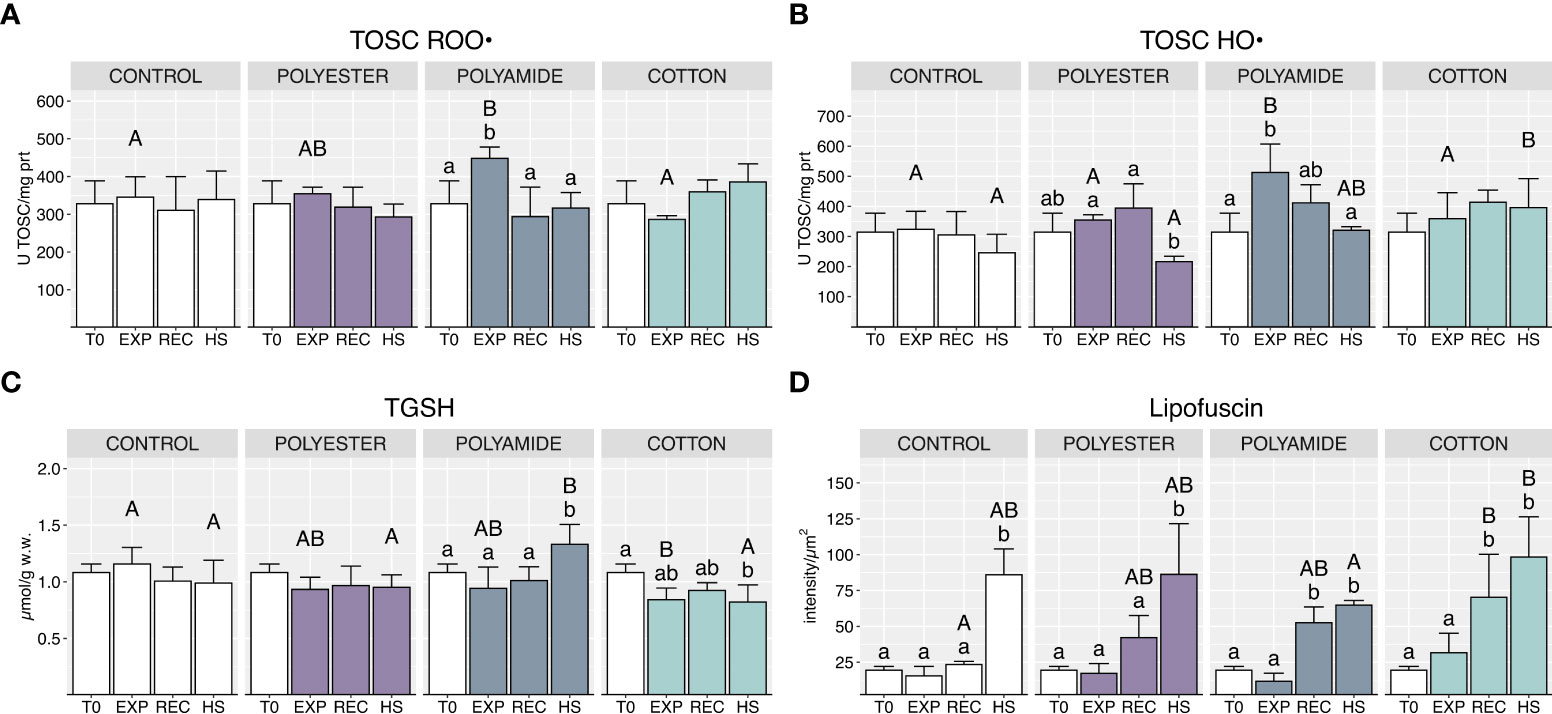
Figure 5 Total oxyradical scavenging capacity toward peroxyl radical (A) and hydroxyl radical (B), total glutathione (C) and lipofuscin content (D) in control and polyester, polyamide or cotton-exposed mussels measured at T0 and at the end of each experimental phase (EXP, end of MFs-exposure; REC, recovery after MFs-exposure; HS, heat-stress after the MFs-exposure). Upper case letters highlight differences among treatments within the same experimental phase; lower case letters highlight differences among different experimental phases of the same treatment. Values are given as mean ± SD (n=4).
Total glutathione was significantly lowered at the end of the exposure to CO microfibers while it was increased by heat stress in PA-exposed mussels (Figure 5C; Table 1).
Delayed effects in MFs-treated mussels were observed in terms of lipofuscin content (Figure 5D; Table 1), which increased in the recovery phase, with significant effects in PA and CO treatments. Lipofuscin furtherly increased under heat stress, with a generalized pattern in all experimental conditions.
Acyl-CoA oxidase (AOX) and neutral lipids content
MFs exposure caused a generalized decrease of AOX activity (Figure 6A; Table 1), which occurred during the exposure phase for synthetic-MFs treatments (PEST and PA) and during the recovery in mussels exposed to CO; heat stress caused a significant decrease of this enzyme activity in control organisms, which was not paralleled by the same effect in mussels previously exposed to MFs.
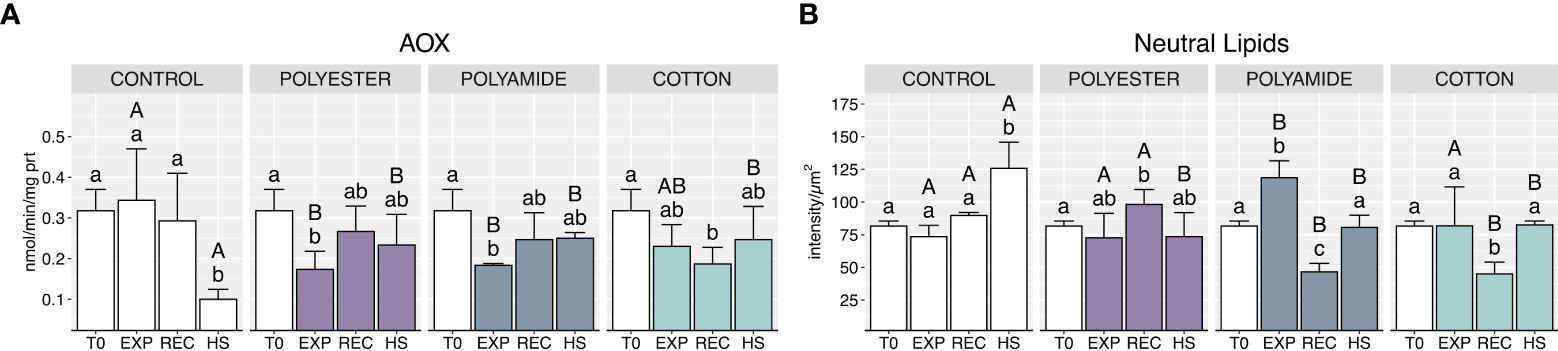
Figure 6 Acyl-CoA oxidase activity (A) and neutral lipids content (B) in control and polyester, polyamide or cotton-exposed mussels measured at T0 and at the end of each experimental phase (EXP, end of MFs-exposure; REC, recovery after MFs-exposure; HS, heat-stress after the MFs-exposure). Upper case letters highlight differences among treatments within the same experimental phase; lower case letters highlight differences among different experimental phases of the same treatment. Values are given as mean ± SD (n=4).
Neutral lipids content significantly increased in PA-exposed organisms at the end of the exposure (Figure 6B; Table 1), followed by a marked decrease after the recovery phase, also observed for CO-exposed. Conversely, heat stress resulted in an increased neutral lipids content in control organisms compared to mussels previously exposed to PEST, PA and CO microfibers.
Weighted elaboration
Weighted elaboration of biological responses provided a synthetic classification of hazard for each typology of microfiber and experimental phase (Figure 7).
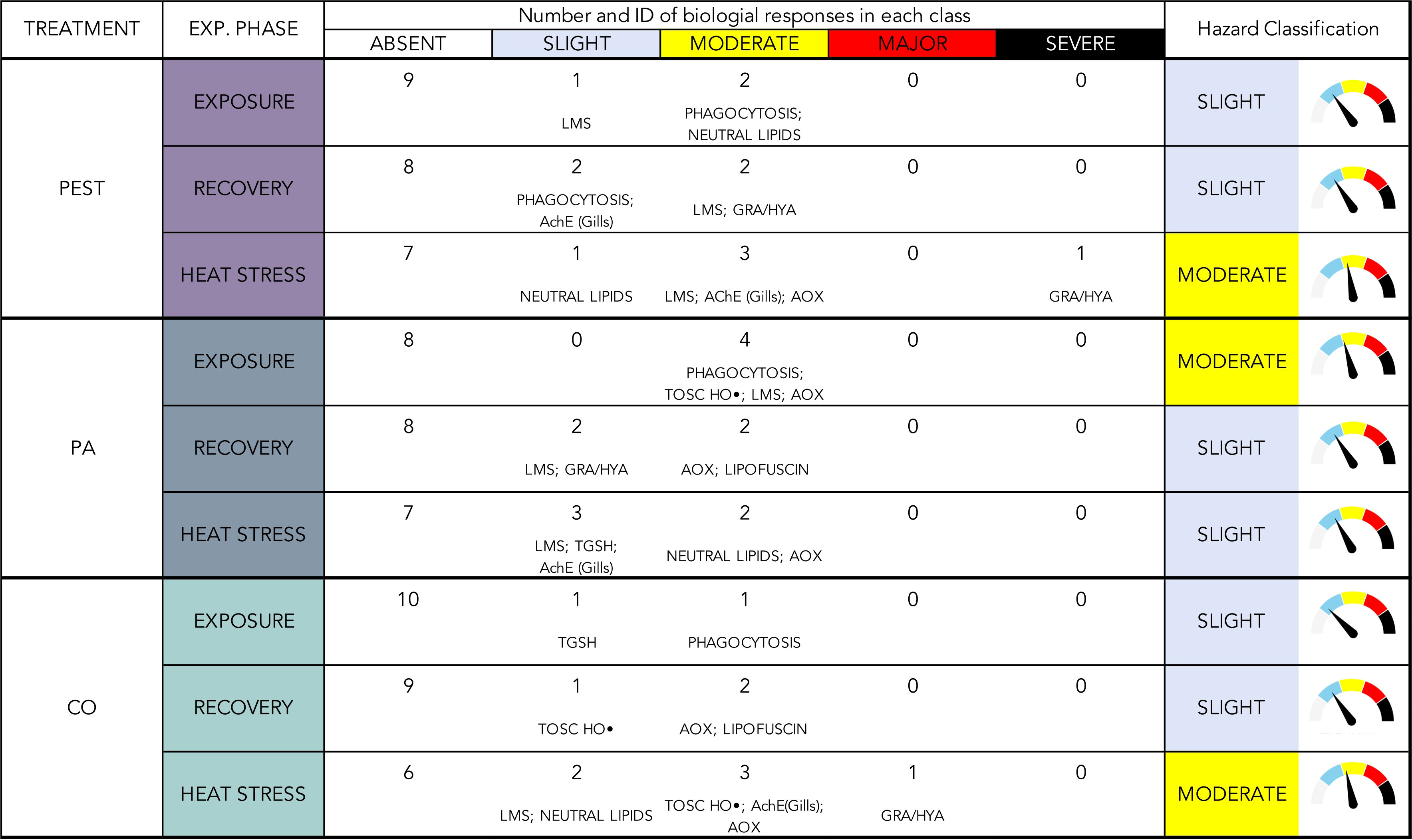
Figure 7 Weighted elaboration of collected results for each experimental treatment in each experimental phase. Number and identification (ID) of biological responses in each class are given, together with the overall hazard classification.
Among different microfibers, PA determined the greatest cellular hazard, classified as “Moderate” at the end of the exposure, and deriving from variations of phagocytosis, lysosomal membranes stability, antioxidant efficiency against HO• radical and AOX activity; the overall effects of PEST and CO were summarized in a “Slight” hazard after the exposure. Overall, this elaboration allowed to quantify the main contribution of phagocytosis in response to all typologies of MFs, while a certain disturbance of lipid metabolism was also relevant during exposure to synthetic MFs (neutral lipids in PEST-treatment and AOX activity in PA treatment).
The weighted elaboration after the recovery phase synthetized the capability of mussels to recover from PA-effects, with a hazard level decreasing from “Moderate” to “Slight”, the same classification observed for PEST- and CO-exposed organisms. A shift of targeted biological processes was evident when analyzing the parameters mainly contributing to this classification: the level of phagocytosis impairment decreased in all treatments remaining slight only in mussels exposed to PEST where (in addition to branchial AChE), immune responses gave the greater contribution to the elaborated hazard with haemocytes lysosomal membranes stability and granulocytes on hyalinocytes ratio (Figure 7); after the recovery from PA exposure, lipid metabolism and peroxidation were predominant over a persisting haemocytes sensitivity (lysosomal membranes stability and granulocytes on hyalinocytes ratio), while CO-exposed organisms showed delayed onset of effects in terms of antioxidant efficiency against HO• radical, lipid metabolism (AOX activity) and peroxidation (lipofuscin).
The weighted elaboration of results in organisms subjected to heat stress after the exposure to MFs showed an increased hazard (“Moderate”) for PEST- and CO-exposed organisms; heat stress amplified previous effects in terms of neuro-immune alterations (granulocytes on hyalinocytes ratio, AChE activity in gills, lysosomal membranes stability) and lipid metabolism (AOX activity and neutral lipids) in both treatments and altered antioxidant efficiency against HO• radical in CO-exposed organisms; the “Slight” classification of hazard observed after HS in organisms previously exposed to PA was attributed mostly to persistence of alterations affecting lipid metabolism and, to a lower extent, neuro-immune responses.
Discussions
MFs exposure and carry-over effects
Among the general objectives, this study was aimed to compare the biological reactivity and sub-lethal effects of synthetic and natural MFs, assessing the potential onset of carry-over outcomes after a recovery period.
The overall results provided a clear laboratory-evidence of cellular disturbance in M. galloprovincialis triggered by the ingestion of the most frequently detected MFs in marine environment (polyester, polyamide, and cotton), with specificities of targeted biological traits related to polymeric nature of fibers, different timing of effects and modulation of sensitivity toward additional stressors.
PA resulted to be preferentially ingested by mussels compared to other microfibers typologies, as indicated by the frequencies of ingestion at the end of the exposure period. Considering only synthetic MFs, it could be hypothesized that ingestion was affected by the chemistry of the polymer rather than by other intrinsic characteristics of microfibers: beside comparable dimensions (PEST-MFs, 618 ± 367 µm length, 13 ± 1 µm diameter; PA-MFs, 566 ± 500 µm length, 11 ± 1 µm in diameter), PEST should have had a higher likelihood than PA to sink in the exposure tanks and be more easily intercepted by organisms given its higher density (1.38-1.41 g cm-3 compared to 1.12-1.15 g cm-3 for PEST and PA, respectively, and 1.028 g cm-3 ASW used in the experiment). Conversely, experimental results showed a preferential ingestion of PA, that more than driven by density characteristics could be explained by the possible presence or release from the polymeric matrix of phagostimulants acting as feeding cue (Allen et al., 2017; Axworthy and Padilla-Gamiño, 2019). Evidence of preferential ingestion of PA over other synthetic MFs (polyester and polypropylene) has already been observed also in anemones and ascribed to a higher palatability (Romanó de Orte et al., 2019). In addition, the higher frequency of ingestion of CO over PEST-MFs obtained in this study supports field observations of a conspicuous presence of natural MFs among those ingested by wild organisms: once again, palatability characteristics of cellulose or its density (CO: 1.5-1.55 g cm-3) are possible explanatory factors favoring the interaction with sessile filter-feeding organisms, rather than their dimensions (CO-MFs, 412 ± 342 µm length, 16 ± 4 µm diameter). Regardless of polymer typology and frequency of ingestion, the numbers of detected items (generally 1 item/individual) indicated that the ingestion of anthropogenic microparticles, including microfibers, does not lead to accumulation, since organisms can eliminate them via feces and pseudofeces (Bour et al., 2020), as also observed in biodeposits investigated in our study. Retention time of microfibers in digestive tissues was shown to vary from a few hours to up to 3 weeks in crabs and some fish species, and from a few hours to a couple of days in small invertebrates, like daphnids, gammarids, sea anemones, and shrimps (Bour et al., 2020). Mussels are known for a certain capability of particle-selection and fast (~3h) rejection-egestion mechanisms (Woods et al., 2018; Ward et al., 2019): the evidence of MFs in biodeposits of exposed organisms support the robustness of our findings on limited accumulation of MFs over time in mussels, in agreement with the low numbers of these particles (1-4/individual) typically detected in wild bivalves (Lourenço et al., 2017; Catarino et al., 2018; Li et al., 2018; Avio et al., 2020; Ding et al., 2021).
Despite the limited retention of ingested items, MFs showed a significant biological reactivity with different magnitude and timing according to polymer typology. Phagocytosis was reduced regardless of synthetic or natural origin of MFs, allowing to exclude a chemical-related effect but rather a consequence of the mechanical disturbance and cell-mediated immune response of heamocytes (Balbi et al., 2021). The result observed for MFs support a similar physical disturbance already described for other microplastics on the immune system of bivalves (Avio et al., 2015; Pittura et al., 2018; Tang et al., 2020; Capolupo et al., 2021, among others). The effects observed in our study on phagocytosis were transient and limited to the exposure phase, with restored values at the end of the recovery. A slight increase of granulocytes on hyalinocytes ratio was measured in mussels after exposure to PA- and CO-microfibers, which may suggest granulocytosis as a mechanism to increase the number of phagocyting cells, already described for Mytilus spp. after short-term exposure to PA-MFs (Cole et al., 2020). The physical disturbance caused by MFs on immunocytes subpopulations and functioning might have been worsened through polymer-specificities, given that synthetic-MFs impaired haemocytes lysosomal membrane stability both during the exposure and after the recovery phase: sub-cellular cascade-effects on haemocytes may have resulted in lysosomal membranes damages and stimulation of ROS production, as described for other typologies of MPs (Von Moss et al., 2012; Avio et al., 2015; Romdhani et al., 2022). Since granules of granulocytes have lysosomal origin (Carballal et al., 1997), the reduced lysosomal membrane stability could be, at least partially, linked to the impairment of phagocytosis.
The delayed induction of AChE at the end of the recovery may represent a biochemical to overcome cellular effects related to phagocytosis efficiency, rather than being symptomatic of MFs neurotoxicity. Neurotoxic effects of microplastics have been reported as AChE inhibition (Ribeiro et a., 2017; Barboza et al., 2018; Prüst et al., 2020; Missawi et al., 2021), but is now also known that this enzyme plays a fundamental role in regulating immune function by preventing phagocytosis block caused by ACh accumulation (Liu et al., 2018b; Du et al., 2020; Nardi et al., 2021).
The hypothesized pro-oxidant action of synthetic MFs was confirmed by a transient activation of oxidative-stress responses in PA-exposed mussels and by a delayed and generalized accumulation of lipofuscin after the recovery period. This oxidative modulation may derive from the onset of inflammation caused by the presence of MFs in the digestive tissue and their interaction with epithelia, the same mechanism previously suggested for MPs by other authors (von Moss et al., 2012; Paul-Pont et al., 2016; Cole et al., 2020).
In addition to oxidative disturbance, the ingestion of MFs contributed to alteration of peroxisomal fatty acids metabolism with a different timing between synthetic- and natural-MFs; the inhibition of AOX activity in digestive tissues was observed after the exposure to PEST and PA, while the onset of this effect was delayed after the recovery phase for CO-exposed organisms. These results are consistent with previous observations on the effects of polystyrene (PS) microplastics on AOX activity in mussels (von Hellfeld et al., 2022), and on disturbance of PS-nanoplastics on acetyl-CoA metabolism in razor clam Sinonovacula constricta (Jiang and Zhang, 2021). The lower activity of this key enzyme for beta-oxidation of fatty acids may be prognostic of metabolism disorders and energy reserves mobilization: while limited effects were caused by PEST-MFs, both PA and CO determined a relevant decrease of neutral lipids after the recovery phase, with a particularly relevant magnitude of variation in PA-treatment compared to the exposure phase. In this respect, since neutral lipids are one of the main energy reserve in bivalves (Bocchetti and Regoli, 2006), the observed decrease may reflect a demand to sustain the activated stress-defense mechanisms (Shang et al., 2021), or an inefficient nutrients assimilation deriving from MFs presence in the digestive tissue, as previously hypothesized for MPs (Wright et al., 2013; Trestrail et al., 2021).
Effects of MFs on thermal stress susceptibility
A relevant aim of this study was to assess if effects of MFs could influence susceptibility of organisms to additional stressor occurring at a later stage after MFs exposure, such as a sudden increase of temperature. Given the increasing occurrence of short-term and acute heat stress events like marine heatwaves (Oliver et al., 2018), this scenario was tested on mussels previously exposed to MFs, gaining environmental relevance considering that despite ubiquitous, MFs (and MPs) have a deeply variable distribution in space and time (Adamopoulou et al., 2021).
The disturbance on immunocytes was markedly exacerbated by heat stress after MFs exposure: the increased granulocytes on hyalinocytes ratio coupled to the lack of variations on phagocytosis, allows to hypothesize granulocytosis as a compensatory mechanism to maintain the functional performance of moderately stressed immunocytes additionally affected by heat stress. A negative effect of prolonged heat stress (7-28 days) was previously described on phagocytosis efficiency of bivalves immunocytes (Monari et al., 2007; Nardi et al., 2017; Nardi et al., 2018; Rahman et al., 2019; Amorim et al., 2020), and evidence of granulocytes proliferation was associated to transient and dynamic heat stress under simulated marine heatwaves in laboratory experiments on Scorbicularia plana and M. galloprovincialis (Amorim et al., 2020; Nardi et al., 2022). Despite heat stress has been often associated to increased labilization of haemocytes lysosomal membranes (Yao and Somero, 2012; Negri et al., 2013; Nardi et al., 2017; Nardi et al., 2018), no alteration was observed in mussels exposed only to temperature increase (CONTROL-HS); additive effects of heat stress and MFs on lysosomal stability were evident only for CO-treated organisms, thus highlighting the delayed onset of a biological response after the exposure period, and suggesting a MFs polymer-specificity in determining differential responsiveness to non-synchronous additional stressors.
Interactive effects of heat stress and MFs-disturbance were highlighted on AChE activity, with tissue differences. The slight inhibition provoked by heat stress on AChE in haemolymph was not further influenced by previous exposure to various typologies of MFs; on the contrary, the branchial induction of AChE caused by temperature on control organisms was not paralleled in mussels previously treated with MFs. The latter result would suggest a higher demand of cholinergic activity in the gills of organisms subjected to heat stress alone, probably related to increased filtration and respiration (Pfeifer et al., 2005; Freitas et al., 2019; Costa et al., 2020), or some antagonistic effects or carry-over drawbacks of MFs exposure on cholinergic system of mussels.
The exposure to heat stress did not modulate an evident pro-oxidant challenge neither in control nor in previously MFs exposed mussels, despite the well-known capacity of temperature to promote ROS production, activation of antioxidant defenses and oxidative stress in marine bivalves (Benedetti et al., 2022 and references therein). The only exception was the significant accumulation of lipofuscin in mussels after heat stress regardless of previous exposure to MFs. These results, beyond confirming the sensitivity of M. galloprovincialis to sudden increases of temperature near the upper limits of its thermal window (Georgoulis et al., 2021; Nardi et al., 2022), allows to exclude that pre-exposure to MFs can enhance oxidative susceptibility and damages in a multiple stressors scenario.
Interestingly, a different responsiveness to thermal stress was observed on lipid metabolism between control and MFs-exposed mussels. In control organisms, temperature increase caused a lowered AOX activity and neutral lipids accumulation, a relationship already described in mussels and suggesting accumulation of energy reserves to sustain cellular homeostasis under heat stress (Cancio et al., 1999). On the other hand, mussels previously exposed to MFs did not exhibit a further modulation of lipid metabolism compared to organisms exposed only to MFs: whether this result should be interpreted as a negative drawback of MFs exposure affecting energy homeostasis or an increased stress-tolerance mechanism remains to be explored.
Concluding remarks based on weighted elaboration of synthetic indices
This study provided clear evidence of the biological disturbance caused by both synthetic and natural MFs. Overall, immunocytes subpopulations ratio, functional activity and organelles integrity were sensitive to all investigated MFs, confirming the susceptibility of this system to microlitter components. At the same time, the weighted elaboration of results, based on the magnitude of observed variations and on the biological relevance of analyzed parameters, highlighted different degrees of reactivity and the involvement of other cellular districts and biological processes among investigated typologies of polymers. Polyamide resulted to be the more reactive after the exposure phase, but the alterations were partially restored after 7 days of recovery; on the other hand, the detrimental consequences in organisms exposed to polyester and cotton, despite less evident, lasted longer and appeared to exacerbate the biological effects of a delayed heat stress, suggesting synergistic disturbance from non-synchronous multi-stressor conditions which affected lipid metabolism due to sustained activation of stress defense mechanisms.
These results suggest that despite the limited short-term effects, MFs represent a real hazard for marine organisms, disturbing their immune response, activating prooxidative mechanisms and affecting lipid metabolism which may ultimately lead to lower energetic investment in other crucial biological processes, as growth and reproduction among others.
In conclusion, our results revealed similarities but also differences in biological reactivity of synthetic or natural microfibers, highlighting the need to also consider their role as factors contributing to possible negative outcomes of other environmental stressors. Given the limited short-term biological reactivity and effects of microfibers, future steps should further investigate their impact in target species on longer-term perspectives and under multiple stressors and climate change scenarios, possibly establishing links between biological effects of MFs and their physic-chemical characteristics such as polymer typology, length and diameter, to elucidate potentially specific forms of hazard.
Data availability statement
The raw data supporting the conclusions of this article will be made available by the authors, without undue reservation.
Author contributions
LP, AN, SG and FR, conceptualization and design of the study. LP, AN, MC and FF, resources and methodology. LP, AN, CM and FM, data collection. LP, AN and GD’E, data curation, statistical analyses and data elaboration. LP and AN, supervision, writing – original draft. LP, AN, MC, MB, SG, MA and FR, writing – review and editing. SG, MB and FR, funding acquisition and project administration. All authors contributed to the article and approved the submitted version.
Funding
This work was conducted under the framework of EMME PRIN-2017 project (“Exploring the fate of Mediterranean microplastics: from distribution pathways to biological effects”) funded by MUR Italian Agency (Ministero dell’Università e della Ricerca) and RESPONSE project (“Toward a risk-based assessment of microplastic pollution in marine ecosystems”) funded by JPI Oceans (2020-2023). CUP I54I20000220001 for PRIN-2017 project. CUP I35F18000760005 for RESPONSE project.
Conflict of interest
The authors declare that the research was conducted in the absence of any commercial or financial relationships that could be construed as a potential conflict of interest.
Publisher’s note
All claims expressed in this article are solely those of the authors and do not necessarily represent those of their affiliated organizations, or those of the publisher, the editors and the reviewers. Any product that may be evaluated in this article, or claim that may be made by its manufacturer, is not guaranteed or endorsed by the publisher.
Supplementary material
The Supplementary Material for this article can be found online at: https://www.frontiersin.org/articles/10.3389/fmars.2022.981365/full#supplementary-material
References
Adamopoulou A., Zeri C., Garaventa F., Gambardella C., Ioakeimidis C., Pitta E. (2021). Distribution patterns of floating microplastics in open and coastal waters of the Eastern Mediterranean Sea (Ionian, Aegean, and levantine seas). Front. Mar. Sci. 8. doi: 10.3389/fmars.2021.699000
Allen A. S., Seymour A. C., Rittschof D. (2017). Chemoreception drives plastic consumption in a hard coral. Mar. pollut. Bull. 124 (1), 198–205. doi: 10.1016/j.marpolbul.2017.07.030
Amorim V. E., Gonçalves O., Capela R., Fernández-Boo S., Oliveira M., Dolbeth M., et al. (2020). Immunological and oxidative stress responses of the bivalve scrobicularia plana to distinct patterns of heatwaves. Fish Shellfish Immunol. 106, 1067–1077. doi: 10.1016/j.fsi.2020.09.024
Athey S. N., Erdle L. M. (2022). Are we underestimating anthropogenic microfiber pollution? a critical review of occurrence, methods, and reporting. Environ. Toxicol. Chem. 41 (4), 822–837. doi: 10.1002/etc.5173
Avio C. G., Gorbi S., Milan M., Benedetti M., Fattorini D., D'Errico G., et al. (2015). Pollutants bioavailability and toxicological risk from microplastics to marine mussels. Environ. pollut. 198, 211–222. doi: 10.1016/j.envpol.2014.12.021
Avio C. G., Pittura L., d'Errico G., Abel S., Amorello S., Marino G., et al. (2020). Distribution and characterization of microplastic particles and textile microfibers in Adriatic food webs: General insights for biomonitoring strategies. Environ. pollut. 258, 113766. doi: 10.1016/j.envpol.2019.113766
Axworthy J. B., Padilla-Gamiño J. L. (2019). Microplastics ingestion and heterotrophy in thermally stressed corals. Sci. Rep. 9, 18193. doi: 10.1038/s41598-019-54698-7
Bagaev A., Mizyuk A., Khatmullina L., Isachenko I., Chubarenko I. (2017). Anthropogenic fibres in the Baltic Sea water column: Field data, laboratory and numerical testing of their motion. Sci. Total Environ. 599, 560–571. doi: 10.1016/j.scitotenv.2017.04.185
Bagwe R., Beniash E., Sokolova I. M. (2015). Effects of cadmium exposure on critical temperatures of aerobic metabolism in eastern oysters crassostrea virginica (Gmeli). Aquat. Toxicol. 167, 77–89. doi: 10.1016/j.aquatox.2015.07.012
Balbi T., Auguste M., Ciacci C., Canesi L. (2021). Immunological responses of marine bivalves to contaminant exposure: Contribution of the -omics approach. Front. Immunol. 12, 618726. doi: 10.3389/fimmu.2021.618726
Barboza L. G. A., Vieira L. R., Branco V., Figueiredo N., Carvalho F., Carvalho C., et al. (2018). Microplastics cause neurotoxicity, oxidative damage and energy-related changes and interact with the bioaccumulation of mercury in the European seabass, Dicentrarchus labrax (Linnaeu). Aquat. Toxicol. 195, 49–57. doi: 10.1016/j.aquatox.2017.12.008
Benedetti M., Giuliani M. E., Mezzelani M., Nardi A., Pittura L., Gorbi S., et al. (2022). Emerging environmental stressors and oxidative pathways in marine organisms: Current knowledge on regulation mechanisms and functional effects. Biocell 46 (1), 37. doi: 10.1007/s11356-019-06265-w
Bocchetti R., Regoli F. (2006). Seasonal variability of oxidative biomarkers, lysosomal parameters, metallothioneins and peroxisomal enzymes in the Mediterranean mussel mytilus galloprovincialis from Adriatic Sea. Chemosphere 65 (6), 913–921. doi: 10.1016/j.chemosphere.2006.03.049
Bour A., Hossain S., Taylor M., Sumner M., Carney Almroth B. (2020). Synthetic microfiber and microbead exposure and retention time in model aquatic species under different exposure scenarios. Front. Environ. Sci. 8. doi: 10.3389/fenvs.2020.00083
Cancio I., Ibabe A., Cajaraville M. P. (1999). Seasonal variation of peroxisomal enzyme activities and peroxisomal structure in mussels mytilus galloprovincialis and its relationship with the lipid content. Comp. Biochem. Physiol. - C Pharmacol. Toxicol. Endocrinol. 123 (2), 135–144. doi: 10.1016/S0742-8413(99)00019-5
Capolupo M., Valbonesi P., Fabbri E. (2021). A comparative assessment of the chronic effects of micro-and nano-plastics on the physiology of the mediterranean mussel mytilus galloprovincialis. Nanomaterials 11 (3), 1–17, 649. doi: 10.3390/nano11030649
Carballal M. J., Carmen López M., Azevedo C., Villalba A. (1997). Hemolymph cell types of the mussel mytilus galloprovincialis. Dis. Aquat. Organisms 29 (2), 127–135. doi: 10.3354/dao029127
Catarino A. I., Macchia V., Sanderson W. G., Thompson R. C., Henry T. B. (2018). Low levels of microplastics (MP) in wild mussels indicate that MP ingestion by humans is minimal compared to exposure via household fibres fallout during a meal. Environ. pollut. 237, 675–684. doi: 10.1016/j.envpol.2018.02.069
Choi J. S., Kim K., Hong S. H., Park K.-I., Park J.-W. (2021). Impact of polyethylene terephthalate microfiber length on cellular responses in the Mediterranean mussel mytilus galloprovincialis. Mar. Environ. Res. 168, 105320. doi: 10.1016/j.marenvres.2021.105320
Choi J. S., Kim K., Park K., Park J.-W. (2022). Long-term exposure of the Mediterranean mussels, mytilus galloprovincialis to polyethylene terephthalate microfibers: Implication for reproductive and neurotoxic effects. Chemosphere 299, 134317. doi: 10.1016/j.chemosphere.2022.134317
Cole M., Liddle C., Consolandi G., Drago C., Hird C., Lindeque P. K., et al. (2020). Microplastics, microfibres and nanoplastics cause variable sub-lethal responses in mussels (Mytilus spp.). Mar. pollut. Bull. 160, 111552. doi: 10.1016/j.marpolbul.2020.111552
Cook. G. J. (2001). Handbook of textile fibres, vol II. man-made fibres (Cambridge, England: Woodhead Publishing Series in Textiles), pp 9–78.
Coppola F., Almeida Â., Henriques B., Soares A. M. V. M., Figueira E., Pereira E., et al. (2017). Biochemical impacts of Hg in mytilus galloprovincialis under present and predicted warming scenarios. Sci. Total Environ., 601–602, 1129–1138. doi: 10.1016/j.scitotenv.2017.05.201
Costa S., Coppola F., Pretti C., Intorre L., Meucci V., Soares A. M. V. M., et al. (2020). The influence of climate change related factors on the response of two clam species to diclofenac. Ecotoxicol. Environ. Saf. 189, 109899. doi: 10.1016/j.ecoenv.2019.109899
Crain C. M., Kroeker K., Halpern B. S. (2008). Interactive and cumulative effects of multiple human stressors in marine systems. Ecol. Lett. 11 (12), 1304–1315. doi: 10.1111/j.1461-0248.2008.01253.x
d'Errico G., Nardi A., Benedetti M., Mezzelani M., Fattorini D., Di Carlo M., et al. (2021). Application of a multidisciplinary weight of evidence approach as a tool for monitoring the ecological risk of dredging activities. Front. Mar. Sci. 8, 765256. doi: 10.3389/fmars.2021.765256
De Falco F., Cocca M., Avella M., Thompson R. C. (2020). Microfiber release to water, via laundering, and to air, via everyday use: a comparison between polyester clothing with differing textile parameters. Environ. Sci. Technol. 54 (6), 3288–3296. doi: 10.1021/acs.est.9b06892
De Falco F., Di Pace E., Cocca M., Avella M. (2019). The contribution of washing processes of synthetic clothes to microplastic pollution. Sci. Rep. 9 (1), 1–11. doi: 10.1038/s41598-019-43023-x
Di Mauro R., Castillo S., Pérez A., Iachetti C. M., Silva L., Tomba J. P., et al. (2022). Anthropogenic microfibers are highly abundant at the burdwood bank seamount, a protected sub-Antarctic environment in the southwestern Atlantic ocean. Environ. Pollution 306 art. no. 119364. doi: 10.1016/j.envpol.2022.119364
Ding J., Sun C., He C., Li J., Ju P., Li F. (2021). Microplastics in four bivalve species and basis for using bivalves as bioindicators of microplastic pollution. Sci. Total Environ. 782, 146830. doi: 10.1016/j.scitotenv.2021.146830
Du X., Tang Y., Han Y., Ri S., Kim T., Ju K., et al. (2020). Acetylcholine suppresses phagocytosis via binding to muscarinic- and nicotinic-acetylcholine receptors and subsequently interfering Ca2+- and NFκB-signaling pathways in blood clam. Fish Shellfish Immunol. 102, 152–160. doi: 10.1016/j.fsi.2020.04.030
Freitas R., Leite C., Pinto J., Costa M., Monteiro R., Henriques B., et al. (2019). The influence of temperature and salinity on the impacts of lead in mytilus galloprovincialis. Chemosphere 235, 403–412. doi: 10.1016/j.chemosphere.2019.05.221
Frölicher T. L., Fischer E. M., Gruber N. (2018). Marine heatwaves under global warming. Nature 560 (7718), 360–364. doi: 10.1038/s41586-018-0383-9
Gago J., Carretero O., Filgueiras A. V., Viñas L. (2018). Synthetic microfibers in the marine environment: A review on their occurrence in seawater and sediments. Mar. pollut. Bull. 127, 365–376. doi: 10.1016/j.marpolbul.2017.11.070
Gaylarde C., Baptista-Neto J. A., da Fonseca E. M. (2021). Plastic microfibre pollution: how important is clothes’ laundering? Heliyon 7 (5), e07105. doi: 10.1016/j.heliyon.2021.e07105
Georgoulis I., Feidantsis K., Giantsis I. A., Kakale A., Bock C., Pörtner H. O., et al. (2021). Heat hardening enhances mitochondrial potential for respiration and oxidative defence capacity in the mantle of thermally stressed mytilus galloprovincialis. Sci. Rep. 11 (1), 17098. doi: 10.1038/s41598-021-96617-9
Herzke D., Ghaffari P., Sundet J. H., Tranang C. A., Halsband C. (2021). Microplastic fiber emissions from wastewater effluents: Abundance, transport behavior and exposure risk for biota in an Arctic fjord. Front. Environ. Sci. 9, 662168. doi: 10.3389/fenvs.2021.662168
Houck M. M. (2009). Identification of textile fibres (London: Woodhead Publishing Limited and CRC Press LLC).
Jiang Q., Zhang W. (2021). Gradual effects of gradient concentrations of polystyrene nanoplastics on metabolic processes of the razor clams. Environ. pollut. 287, 117631. doi: 10.1016/j.envpol.2021.117631
Kamel N., Attig H., Dagnino A., Boussetta H., Banni M. (2012). increased temperatures affect oxidative stress markers and detoxification response to benzo[a]pyrene exposure in mussel mytilus galloprovincialis. Arch. Environ. Contamination Toxicol. 63 (4), 534–543. doi: 10.1007/s00244-012-9790-3
Kwak J. I., Liu H., Wang D., Lee Y. H., Lee J. S., An Y. J. (2022). Critical review of environmental impacts of microfibers in different environmental matrices. Comp. Biochem. Physiol. Part C: Toxicol. Pharmacol. 251, 109196. doi: 10.1016/j.cbpc.2021.109196
Li J., Green C., Reynolds A., Shi H., Rotchell J. M. (2018). Microplastics in mussels sampled from coastal waters and supermarkets in the united kingdom. Environ. pollut. 241, 35–44. doi: 10.1016/j.envpol.2018.05.038
Li L., Su L., Cai H., Rochman C.M., Li Q., Kolandhasamy P., Peng J., Shi H. (2019). The uptake of microfibers by freshwater Asian clams (Corbicula fluminea) varies based upon physicochemical properties. Chemosphere 221, 107–114. doi: 10.1016/j.chemosphere.2019.01.024
Liu J., Liang J., Ding J., Zhang G., Zeng X., Yang Q., et al. (2021). Microfiber pollution: an ongoing major environmental issue related to the sustainable development of textile and clothing industry. Environment Dev. Sustainability 23 (8), 11240–11256. doi: 10.1007/s10668-020-01173-3
Liu Z., Li M., Yi Q., Wang L., Song L. (2018a). The neuroendocrine-immune regulation in response to environmental stress in marine bivalves. Front. Physiol. 9 (NOV). doi: 10.3389/fphys.2018.01456
Liu Z., Wang L., Lv Z., Zhou Z., Wang W., Li M., et al. (2018b). The cholinergic and adrenergic autocrine signaling pathway mediates immunomodulation in oyster crassostrea gigas. Front. Immunol. 9. doi: 10.3389/fimmu.2018.00284
Liu J., Yang Y., Ding J., Zhu B., Gao W. (2019). Microfibers: a preliminary discussion on their definition and sources. Environ. Sci. pollut. Res. 26 (28), 29497–29501. doi: 10.1007/s11356-019-06265-w
Lourenço P. M., Serra-Gonçalves C., Ferreira J. L., Catry T., Granadeiro J. P. (2017). Plastic and other microfibers in sediments, macroinvertebrates and shorebirds from three intertidal wetlands of southern Europe and west Africa. Environ. pollut. 231, 123–133. doi: 10.1016/j.envpol.2017.07.103
Lu Y., Yuan J., Lu X., Su C., Zhang Y., Wang C., et al. (2018). Major threats of pollution and climate change to global coastal ecosystems and enhanced management for sustainability. Environ. Pollution 239 pp, 670–680. doi: 10.1016/j.envpol.2018.04.016
Mezzelani M., Nardi A., Bernardini I., Milan M., Peruzza L., d'Errico G., et al. (2021). Environmental pharmaceuticals and climate change: The case study of carbamazepine in m. galloprovincialis under ocean acidification scenario. Environ. Int. 146, 106269. doi: 10.1016/j.envint.2020.106269
Missawi O., Bousserrhine N., Zitouni N., Maisano M., Boughattas I., Marco De, et al. (2021). Uptake, accumulation and associated cellular alterations of environmental samples of microplastics in the seaworm hediste diversicolor. J. Hazardous Materials 406, 124287. doi: 10.1016/j.jhazmat.2020.124287
Monari M., Matozzo V., Foschi J., Cattani O., Serrazanetti G. P., Marin M. G. (2007). Effects of high temperatures on functional responses of haemocytes in the clam chamelea gallina. Fish Shellfish Immunol. 22 (1), 98–114. doi: 10.1016/j.fsi.2006.03.016
Nardi A., Benedetti M., d'Errico G., Fattorini D., Regoli F. (2018). Effects of ocean warming and acidification on accumulation and cellular responsiveness to cadmium in mussels mytilus galloprovincialis: Importance of the seasonal status. Aquat. Toxicol. 204, 171–179. doi: 10.1016/j.aquatox.2018.09.009
Nardi A., Benedetti M., Gorbi S., Regoli F. (2021). Interactive immunomodulation in the Mediterranean mussel mytilus galloprovincialis under thermal stress and cadmium exposure. Front. Mar. Sci. 8. doi: 10.3389/fmars.2021.751983
Nardi A., Mezzelani M., Costa S., d'Errico G., Benedetti M., Gorbi S., et al. (2022). Marine heatwaves hamper neuro-immune and oxidative tolerance toward carbamazepine in mytilus galloprovincialis. Environ. pollut. 300. doi: 10.1016/j.envpol.2022.118970
Nardi A., Mincarelli L. F., Benedetti M., Fattorini D., d'Errico G., Regoli F. (2017). Indirect effects of climate changes on cadmium bioavailability and biological effects in the Mediterranean mussel mytilus galloprovincialis. Chemosphere 169, 493–502. doi: 10.1016/j.chemosphere.2016.11.093
Negri A., Oliveri C., Sforzini S., Mignione F., Viarengo A., Banni M. (2013). Transcriptional response of the mussel mytilus galloprovincialis (Lam.) following exposure to heat stress and copper. PloS One 8 (6), e66802. doi: 10.1371/journal.pone.0066802
Oliver E. C. J., Donat M. G., Burrows M. T., Moore P. J., Smale D. A., Alexander L. V., et al. (2018). Longer and more frequent marine heatwaves over the past century. Nat. Commun. 9 (1), 1324. doi: 10.1038/s41467-018-03732-9
Paul-Pont I., Lacroix C., González Fernández C., Hégaret H., Lambert C., Le Goïc N., et al. (2016). Exposure of marine mussels mytilus spp. to polystyrene microplastics: Toxicity and influence on fluoranthene bioaccumulation. Environ. pollut. 216, 724–737. doi: 10.1016/j.envpol.2016.06.039
Pfeifer S., Schiedek D., Dippner J. W. (2005). Effect of temperature and salinity on acetylcholinesterase activity, a common pollution biomarker, in mytilus sp. from the south-western Baltic Sea. J. Exp. Mar. Biol. Ecol. 320 (1), 93–103. doi: 10.1016/j.jembe.2004.12.020
Pittura L., Avio C. G., Giuliani M. E., d'Errico G., Keiter S. H., Cormier B., et al. (2018). Microplastics as vehicles of environmental PAHs to marine organisms: Combined chemical and physical hazards to the mediterranean mussels, mytilus galloprovincialis. Front. Mar. Sci. 5. doi: 10.3389/fmars.2018.00103
Prüst M., Meijer J., Westerink R. H. S. (2020). The plastic brain: Neurotoxicity of micro- and nanoplastics. Particle Fibre Toxicol. 17 (1), 24. doi: 10.1186/s12989-020-00358-y
Queiroz A. F. D. S., da Conceição A. S., Chelazzi D., Rollnic M., Cincinelli A., Giarrizzo T., et al. (2022). First assessment of microplastic and artificial microfiber contamination in surface waters of the Amazon continental shelf. Sci. Total Environ. 839, 156259. doi: 10.1016/j.scitotenv.2022.156259
Rahman M. A., Henderson S., Miller-Ezzy P., Li X. X., Qin J. G. (2019). Immune response to temperature stress in three bivalve species: Pacific oyster crassostrea gigas, Mediterranean mussel mytilus galloprovincialis and mud cockle katelysia rhytiphora. Fish Shellfish Immunol. 86, 868–874. doi: 10.1016/j.fsi.2018.12.017
Regoli F., d'Errico G., Nardi A., Mezzelani M., Fattorini D., Benedetti M., et al. (2019). Application of a weight of evidence approach for monitoring complex environmental scenarios: The case-study of off-shore platforms. Front. Mar. Sci. 6 (JUL), 377. doi: 10.3389/fmars.2019.00377
Ribeiro F., Garcia A. R., Pereira B. P., Fonseca M., Mestre N. C., Fonseca T. G., et al. (2017). Microplastics effects in scrobicularia plana. Mar. pollut. Bull. 122 pp (1-2), 379–391. doi: 10.1016/j.marpolbul.2017.06.078
Romanó de Orte M., Clowez S., Caldeira K. (2019). Response of bleached and symbiotic sea anemones to plastic microfiber exposure. Environ. pollut. 249, 512–517. doi: 10.1016/j.envpol.2019.02.100
Romdhani I., De Marco G., Cappello T., Ibala S., Zitouni N., Boughattas I., et al. (2022). Impact of environmental microplastics alone and mixed with benzo[a]pyrene on cellular and molecular responses of mytilus galloprovincialis. J. Hazardous Materials 435, 128952. doi: 10.1016/j.jhazmat.2022.128952
Sørensen L., Groven A. S., Hovsbakken I. A., Del Puerto O., Krause D. F., Sarno A., et al. (2021). UV Degradation of natural and synthetic microfibers causes fragmentation and release of polymer degradation products and chemical additives. Sci. Total Environ. 755, 143170. doi: 10.1016/j.scitotenv.2020.143170
Shang Y., Wang X., Chang X., Sokolova I. M., Wei S., Liu W., et al. (2021). The effect of microplastics on the bioenergetics of the mussel mytilus coruscus assessed by cellular energy allocation approach. Front. Mar. Sci. 8. doi: 10.3389/fmars.2021.754789
Singh R. P., Mishra S., Das A. P. (2020). Synthetic microfibers: Pollution toxicity and remediation. Chemosphere 257, 127199. doi: 10.1016/j.chemosphere.2020.127199
Suaria G., Achtypi A., Perold V., Lee J. R., Pierucci A., Bornman T. G., et al. (2020). Microfibers in oceanic surface waters: A global characterization. Sci. Adv. 6 (23), EAAY8493. doi: 10.1126/sciadv.aay8493
Sustainable Investment Institute (2022). Microfibres: the invisible pollution from textiles first sentier MUFG sustainable investment institute sources, distribution and interventions. Report 2022, 1–34. Available at: https://www.firstsentier-mufg-sustainability.com/content/dam/sustainabilityinstitute/assets/research/FSI-Sustainability-Investment-Institute-Report-January2022-final.pdf.
Tang Y., Zhou W., Sun S., Du X., Han Y., Shi W., et al. (2020). Immunotoxicity and neurotoxicity of bisphenol a and microplastics alone or in combination to a bivalve species, tegillarca granosa. Environ. pollut. 265, 115115. doi: 10.1016/j.envpol.2020.115115
Textile Exchange (2021). Preferred fiber & materials market report 2021. 1–118. Available at: https://textileexchange.org/wp-content/uploads/2021/08/Textile-Exchange_Preferred-Fiber-and-Materials-Market-Report_2021.pdf.
Trestrail C., Walpitagama M., Miranda A., Nugegoda D., Shimeta J. (2021). Microplastics alter digestive enzyme activities in the marine bivalve, mytilus galloprovincialis. Sci. Total Environ. 779, 146418. doi: 10.1016/j.scitotenv.2021.146418
von Hellfeld R., Zarzuelo M., Zaldibar B., Cajaraville M. P., Orbea A. (2022). Accumulation, depuration, and biological effects of polystyrene microplastic spheres and adsorbed cadmium and benzo(a)pyrene on the mussel mytilus galloprovincialis. Toxics 10, (1). doi: 10.3390/toxics10010018
Von Moos N., Burkhardt-Holm P., Köhler A. (2012). Uptake and effects of microplastics on cells and tissue of the blue mussel mytilus edulis l. after an experimental exposure. Environ. Sci. Technol. 46 (20), 11327–11335. doi: 10.1021/es302332w
Ward J. E., Zhao S., Holohan B. A., Mladinich K. M., Griffin T. W., Wozniak J., et al. (2019). Selective ingestion and egestion of plastic particles by the blue mussel (Mytilus edulis) and eastern oyster (Crassostrea virginica): implications for using bivalves as bioindicators of microplastic pollution. Environ. Sci. Technol. 53 (15), 8776–8784. doi: 10.1021/acs.est.9b02073
Woods M. N., Stack M. E., Fields D. M., Shaw S. D., Matrai P. A. (2018). Microplastic fiber uptake, ingestion, and egestion rates in the blue mussel (Mytilus edulis). Mar. pollut. Bull. 137, 638–645. doi: 10.1016/j.marpolbul.2018.10.061
Wright S. L., Rowe D., Thompson R. C., Galloway T. S. (2013). Microplastic ingestion decreases energy reserves in marine worms. Curr. Biol. 23 (23), R1031–R1033. doi: 10.1016/j.cub.2013.10.068
Yao C.-L., Somero G. N. (2012). Research article: The impact of acute temperature stress on hemocytes of invasive and native mussels (Mytilus galloprovincialis and mytilus californianus): DNA damage, membrane integrity, apoptosis and signaling pathways. J. Exp. Biol. 215 (24), 4267–4277. doi: 10.1242/jeb.07357
Keywords: microplastics, microfibers, thermal stress, oxidative stress, lipid metabolism, immune system, multiple stressors, mussels
Citation: Pittura L, Nardi A, Cocca M, De Falco F, d’Errico G, Mazzoli C, Mongera F, Benedetti M, Gorbi S, Avella M and Regoli F (2022) Cellular disturbance and thermal stress response in mussels exposed to synthetic and natural microfibers. Front. Mar. Sci. 9:981365. doi: 10.3389/fmars.2022.981365
Received: 29 June 2022; Accepted: 03 August 2022;
Published: 18 August 2022.
Edited by:
Chiara Gambardella, National Research Council (CNR), ItalyReviewed by:
Ilaria Corsi, University of Siena, ItalyR Rathinamoorthy, PSG College of Technology, India
Copyright © 2022 Pittura, Nardi, Cocca, De Falco, d’Errico, Mazzoli, Mongera, Benedetti, Gorbi, Avella and Regoli. This is an open-access article distributed under the terms of the Creative Commons Attribution License (CC BY). The use, distribution or reproduction in other forums is permitted, provided the original author(s) and the copyright owner(s) are credited and that the original publication in this journal is cited, in accordance with accepted academic practice. No use, distribution or reproduction is permitted which does not comply with these terms.
*Correspondence: Francesco Regoli, Zi5yZWdvbGlAdW5pdnBtLml0
†These authors have contributed equally to this work and share first authorship