- 1Department of Life and Environmental Sciences, Polytechnic University of Marche, Ancona, Italy
- 2Department of Materials, Environmental Sciences and Urban Planning, Polytechnic University of Marche, Ancona, Italy
An increasing number of methods for extracting microplastic particles from marine sediments have been published but without evaluating the extraction efficiency. Furthermore, while most of the procedures developed have been applied to sandy sediments from shallow water habitats, specific and standardized procedures for deep-water sediments (> 200 meters deep) are limited. In this study, we describe a specific protocol for extracting microplastics (2- 1000 µm) from deep-sea sediments and for quantifying and identifying them. We also assessed its extraction efficiency, which resulted in a high recovery (on average ca. 60%, and up to 80%) particularly, for polyethylene, polypropylene, and polystyrene. This method can be applied to all fine-grained/muddy sediments and allows the extraction of even the smallest fraction of microplastics (<20 µm), which are expected to have the most severe effects on marine biodiversity and ecosystem functioning and ultimately also have implications for human health.
Introduction
In the last years, several studies have investigated microplastic contaminations (< 5 mm, Hartmann et al., 2019) in different marine environments from beaches to deep-sea systems (Uddin et al., 2021 references therein). Such studies have been conducted by using different methods to separate, identify and determine the abundance of microplastics depending on the marine matrix investigated (e.g., seawater, sediments; Cashman et al., 2020; Kavya et al., 2020).
Marine sediments are considered microplastic sinks, with abundances even higher than in the water column, and previous studies have shown that deep-sea ecosystems are not exempt from these contaminants (Barrett et al., 2020; Courtene-Jones et al., 2020; Tekman et al., 2020; Abel et al., 2021), which may have negative effects on deep-sea biodiversity and food-web functioning (Ma et al., 2020).
Available methods concerning the extraction of (micro)plastics from marine sediments typically include different steps: 1) sieving of wet or dry sediment samples to separate microplastics in different granulometric fractions (Song et al., 2015; Qiu et al., 2016); 2) floatation (consisting of a density separation of the microplastics with specific high-density-salt solution; Imhof et al., 2012; Van Cauwenbeghe et al., 2015; Quinn et al., 2017; Ruggero et al., 2020) or elutriation (i.e., based on density separation in a column with air or water inflow at the bottom of the column to favor flotation or deposition of the particles according to their density; Claessens et al., 2013; Kedzierski et al., 2016; Ruggero et al., 2020); 3) digestion which can be performed using acid or alkaline solutions or oxidant agents and/or enzymes as well as the Fenton reaction (Ruggero et al., 2020 and references therein), 4) visual sorting (through stereo- or light microscopy for fragments > 500 µm; Fischer et al., 2015) and chemical identification (i.e., gas chromatography mass spectrometry (Py-GC/MS), thermogravimetric analysis (TGA) with: thermos-extraction and desorption coupled with gas chromatography-mass spectroscopy (TED-GC–MS), or thermal desorption gas chromatography mass spectrometry (TDS-GC–MS), Raman and FTIR Spectroscopy, Mai et al., 2018).
Currently, some recommendations for microplastic extraction from marine sediments are available (Frias et al., 2018) but there are no standardized procedures and these generally differ according to the different sampling devices used, size of target plastic fragments, granulometry, and characteristics of the sedimentary matrix investigated (e.g., carbonate, muddy, sandy; Phuong et al., 2016; Lindeque et al., 2020; Corinaldesi et al., 2021). These discrepancies and the lack of protocol standardization hinder the comparison of microplastic contaminations among different environments and estimates of their impact on a global scale (Coffin et al., 2021).
Most studies are based on a fractionated sieving of the sediments and microplastics using different mesh sizes, which allow the microplastic classification into different size categories (McDermid and McDermid and McMullen, 2004; Vianello et al., 2013). To further separate microplastics from the sedimentary matrix, flotation is generally carried out by exploiting the different density of the sediments (i.e., 1.8 – 2.2 g cm-3 for muddy and sandy sediments, respectively; Hamilton, 1976) and microplastic particles (i.e., from 0.9 to 2.3 g cm-3 for polyethylene and polyester respectively; Hidalgo-Ruz et al., 2012). Typically, a saturated salt solution, such as NaCl, ZnCl2, NaI, CaCl2, ZnBr2 is used (Stolte et al., 2015; Kedzierski et al., 2017; Ruggero et al., 2020). The step of identifying the particles, which are separated from the sediments, is essential to confirm whether they are plastics or not. Visual detection alone (by stereo- or light microscope) has been especially used in pioneering studies and to investigate microplastic contamination in beaches and coastal environments (Dekiff et al., 2014, references therein; Shim et al., 2017). However, such an approach only allows rapid screening of potential microplastics but can introduce various biases such as misidentification of particles or underestimation of those that are too small to be detected (Dekiff et al., 2014; Filella, 2015; Lavers et al., 2016). To date, spectroscopy is the most used method for identifying and characterizing (micro)plastics in benthic ecosystems (Ruggero et al., 2020). In particular, Fourier Transformed Infrared Spectroscopy (FTIR) has been applied to more than 60% of studies available in the literature (Miller et al., 2017) and different marine benthic matrices (Thompson et al., 2004; Li et al., 2020; Khoironi et al., 2020; Zhang et al., 2020). Also, Raman Spectroscopy has been widely used to investigate microplastic contamination in marine sediments (Lenz et al., 2015; Shim et al., 2016; Imhof et al., 2017; Peng et al., 2018) but this technology is more specific for the identification of inorganic compounds, and the detection of microplastics can be hindered by the interference of additives and pigments present in the samples (Van Cauwenberghe et al., 2013; Tagg et al., 2015; Kavya et al., 2020).
Procedures used to analyze microplastic contaminations in deep-sea soft bottoms have been generally adapted from those applied to shallow-water sediments (Van Cauwenberghe et al., 2013; Woodall et al., 2014; Bergmann et al., 2017) despite deep-sea sediments can have different characteristics (e.g. mineralogical composition, granulometry, organic matter composition, porosity, and water content; Hamilton, 1976) from the coastal ones, which can influence the extraction procedures of plastics. Most of the available studies on microplastic contamination of deep-sea sediments have reported the use of high density saturated salt solutions to extract microplastics from sediment samples such as NaI, NaCl, ZnCl2 or Ludox and FT-IR or Raman spectroscopy to identify the extracted fragments (Van Cauwenberghe et al., 2013; Woodall et al., 2014; Bergmann et al., 2017), documenting abundances of polymers ranging approximately from 3 to more than 103 particles per kg of sediment (Kane et al., 2020; Tekman et al., 2020; Abel et al., 2021)
A key aspect in microplastic pollution studies is their target size range of polymers, which is generally dependent on the method used and that can influence microplastic estimates. In deep-sea sediments, investigations have been largely focused on the analysis of microplastics with a > 50 µm size (Van Cauwenberghe et al., 2013; Peng et al., 2018; La Daana et al., 2019; Cunningham et al., 2020; Peng et al., 2020; Zhang et al., 2020). However, the ecological impacts of fragments smaller of this size could be even stronger than those of larger fragments due to the potential negative effects on processes and mechanisms acting at the cellular level, and consequently on food webs (Galloway et al., 2017).
Another crucial issue to provide reliable estimates of microplastics in deep-sea sediments is the extraction efficiency, which might be reduced compared to coastal sediments as deep-sea sediments are generally characterized by finer grain size and higher content of refractory organic matter, which could trap organic particles (Ghsoub et al., 2020). However, to date, the available protocols applied to the deep-sea sedimentary matrix, have not been tested for their efficiency in recovering microplastics.
In the present study, we describe a procedure for the extraction of microplastics (size: 20-1000 µm) from a deep-sea canyon of the Central Mediterranean Sea and assessed the recovery efficiency of the five polymers most frequently reported in the marine environment (Paul-Pont et al., 2018; Corinaldesi et al., 2021b). We also present here new insights into microplastic abundances of particles smaller than 20 µm (down to 2 µm), whose chemical identification was conducted for the first time by using microanalysis combined with SEM.
Materials and methods
Microplastic mixture preparation
The microplastic mixture was prepared using five different plastic polymers from everyday life (i.e. containers, bottles, cups, pipes) with different densities: polyethylene (PE, 0.89-0.95 g cm-3), polypropylene (PP, 0.85-0.92 g cm-3), polystyrene (PS, 1.04-1.09 g cm-3), polyethylene terephthalate (PET, 1.38 g cm-3) and polyvinylchloride (PVC, 1.16-1.41 g cm-3) (Figure 1) according to the procedure also described in Corinaldesi et al., 2021. These polymers were selected because they account for 80% of plastic production in Europe (PlasticEurope, 2020) and are some of the most frequently reported plastics in marine environments (Imhof et al., 2012; Phuong et al., 2016; Paul-Pont et al., 2018; Corinaldesi et al., 2021). To obtain microplastic particles, of various sizes and shapes, plastic objects from everyday life (i.e. containers, bottles, cups, pipes) were milled. After milling plastic particles, each polymer was sorted by sieving it onto 1000 µm and 2 µm filters, and an amount of 2 mg for each polymer was weighed using a high precision analytical balance (Gibertini E42-B) to obtain a total of 10 mg of a mixture of microplastics. The FT-IR spectroscopy analyses (PerkinElmer FTIR Spectrometer Spectrum GX1) performed on a subsample of the microplastic mixture showed that the chemical characteristics of the polymers remained identical comparing the samples before and after milling.
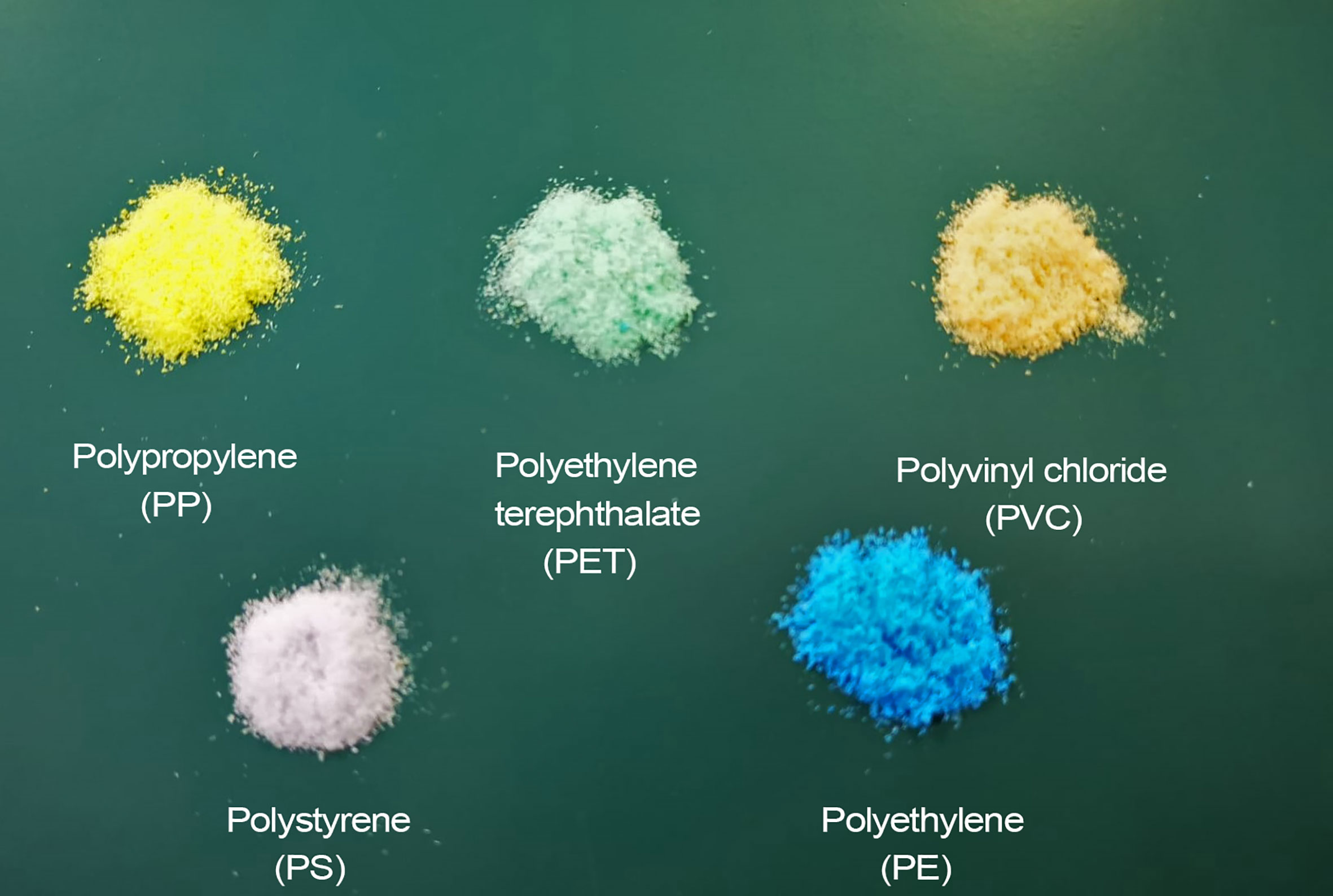
Figure 1 Plastic polymers used in the present study to assess the extraction efficiency of microplastics from deep-sea sediments.
The number of microplastics for each weighted polymer was determined by placing it into a cellulose filter and counting the particles in 60 optical fields using a stereomicroscope at 50× magnification (Zeiss Stami). An optical field coefficient (OFC) was obtained by dividing the filtration area (962 mm2) by the area of the filter containing microplastic particles (4 mm2) and multiplying OFC by the average number of microplastic particles. This approach was already used for the enumeration of benthic bacteria and viruses by epifluorescence microscopy (Danovaro, 2010).
The chemical nature of the polymers was checked by FT-IR.
Additional details are reported in the Supplementary Materials.
Deep-sea sediment collection and recovery efficiency of microplastic particles
Deep-sea sediment was collected at three different depths (at 221, 304, and 910 m), within the San Gregorio Canyon in the Central Mediterranean Sea (38° 03.69553’ N, 15° 35.06056’ E) using a box corer. Surface sediment samples (the top 10 cm, n=3 replicates) were collected at each depth using metal manual corers of 4 cm diameter and once recovered on board, they were sliced and immediately frozen at -20°C. The sediment was characterized by a silt-clay fraction higher than 50% as typically observed in deep-sea sediments (Smith et al., 2008; Danovaro et al., 2010) and organic matter (in terms of biopolymeric C, sensu Danovaro et al., 2001) was on average 0.43 mg g-1 and 1.39 mg g-1 at 910 m and 221-m depth.
To assess the extraction efficiency of microplastics from the deep-sea sediment, it was dried in an oven at 50°C for 4-5 days to avoid overheating of the samples and the formation of flocculates due to chemical reactions between ZnCl2 and seawater (see details in Supplementary Materials). During desiccation, the samples were covered with aluminum foil to avoid external contamination. First, to optimize and assess the procedure with the highest extraction efficiency of microplastics (2-1000 µm) from deep-sea sediments we compared two different approaches based on the addition of:
1. 10 mg of the microplastic mixture (see details in the paragraph "Microplastic mixture preparation) to bulk sediments (three replicates of 250 g each of dry sediment) hereafter defined BS; and
2. 10 mg of the microplastic mixture to partitioned sediments (five aliquots of 50 g of sediment each dry sediment) hereafter defined PS.
These approaches were compared to verify whether the recovery efficiency of microplastics changed when the deep-sea sediment was processed as a whole or subdivided in aliquots as already observed for the extraction of other sediment components (Corinaldesi et al., 2017).
The extraction efficiency of microplastic polymers from deep-sea sediments was determined by FT-IR (for the fraction 20-1000 µm) and SEM (for the fraction 2-20 µm) considering the number of polymers added to the sediments and those recovered after the extraction procedure.
The extraction efficiency from deep-sea sediments of the microplastic particles with a size of 20-1000 µm was determined for each polymer of the microplastic mixture while for the 2-20 µm fraction of the microplastic particles, the extraction efficiency was determined without distinguishing by polymer typology. The coefficient of variation of the extraction efficiency of microplastics from deep-sea sediments by FT-IR is on average 12.9%.
Extraction of microplastic particles from deep-sea sediments and their quantification
The procedure was developed by modifying available protocols for the extraction of microplastic particles from sandy and also muddy sediments (Imhof et al., 2012; Claessens et al., 2013 and Vianello et al., 2013).
A solution of ZnCl2 (1.6 - 1.7 kg L-1) was added both to the bulk sediment (BS) and the partitioned sediment (PS) samples. All the sediments were stirred for 12 h. To favor the detachment of the microplastics from the sediment particles, the samples were subjected to an ultrasound treatment three times of a 1-minute cycle each, with 30 seconds of stirring after each cycle (frequency: 40 kHz; Bransonic Branson 3510). Then, sediment samples were maintained at room temperature for 3 days so that the denser sediment particles could settle down. Subsequently, since the plastic particles tend to float, supernatants were transferred into centrifuge tubes (17,000 ×g for five minutes; Avanti J-30I) to allow the separation of the sediment particles from microplastics. Subsequently, supernatants were collected and filtered onto 20 µm filters (SEFAR NITEX) with a vacuum filtration apparatus while the eluates were held for the analyses of the 2-20 µm microplastic fraction (described below).
To eliminate any organic matter trace and facilitate microplastic counting, the filters were washed three times with 10 ml of H2O2 (30%, Carlo Erba), holding it for 5 minutes (for each cycle) before switching on the vacuum filtration system. After each cycle with H2O2, the filter and filtration column were washed twice with Milli-Q (pre-filtered onto 0.2 µm filters and autoclaved).
Finally, particles with a size of 20-1000 µm were observed under a stereomicroscope at 50×magnification (Zeiss, Stemi). Filters were dried at 30°C and maintained in a glass petri dish at room temperature under a laminar flux hood before counting microplastic particles. Sixty optical fields (n=60) were observed randomly for each filter and counted as previously described.
The eluates obtained by filtration onto 20 µm filters, were in turn filtered onto 2 µm filters (Polycarbonate, Whatman Nucleopore). To eliminate any organic matter trace and facilitate microplastic counting, the filters were subjected to three cycles of treatment with 10 ml of H2O2 (30%, Carlo Erba), holding it for 5 minutes (for each cycle) before switching on the vacuum filtration system. After each cycle with H2O2, the filter and filtration column were washed twice with Milli-Q (pre-filtered onto 0.2 µm filters and autoclaved). Finally, the filters were dried at 30°C and maintained in a glass petri dish at room temperature under a laminar flux hood before the investigation with Scanning Electron Microscopy (SEM).
Thirty optical fields (n=30) were observed in three randomly selected areas of each filter (n=10 optical random fields for each area).
We did not consider a homogeneous distribution of the microplastic particles in the filter but only those detected in the optical fields analyzed, therefore these estimates are to be considered conservative estimates.
Chemical identification of polymers extracted from sediments
To confirm the chemical composition of plastic polymers used during the extraction protocol (size ranging from 20 to 1000 µm), we used a Perkin Elmer FT-IR Spectrometer Spectrum GX1, interfaced with a Perkin–Elmer Autoimage microscope and equipped with a photoconductive 0.25 mm Hg-Cd-Te (MCT) array detector operating at liquid nitrogen temperature and covering the entire IR spectral range from 4000 to 700 cm-1. The Spectrum Autoimage 5.1.0 software package (Perkin Elmer, Waltham, MA, USA) was used. Spectra were acquired in transmission mode on CaF2 support. Background spectra were acquired on clean portions of the CaF2 optical windows and compared against samples spectra. The output spectra were subsequently subjected to a spectral search against reference libraries of polymer spectra contained by PerkinElmer database.
To have a more accurate analysis of the natural samples we used µFT-IR microscope (Spotlight i200, PerkinElmer) coupled with a spectrometer (Spectrum Two, PerkinElmer). The potential microplastics extracted from the deep-sea sediment samples have been manually transferred onto nitrocellulose filters (Sartori), and spectra were acquired using the µATR mode. Following back-ground scans, 32 scans were performed for each particle, with a resolution of 4 cm-1. Background spectra were acquired on clean portions of filters. Spectrum 10 software was used to compare spectra and the identification of polymers was performed by comparison with commercial libraries of standard spectra both (PerkinElmer®) and custom-made libraries.
The detection limit of FT-IR is ca. 10 µm but we set it to 20 µm to have more reliable data.
The microplastic particles collected on 2-µm filters were identified by using Scanning Electron Microscopy (Zeiss SUPRA 40, magnification of 7.26 KX). The very fine granulometry of the sediments created a strong background in the filters therefore, an (energy dispersive x-ray) EDX compositional map (Bruker Quantax Z200) was produced to distinguish the sediment grains (i.e., mapping of silicon) from the microplastic particles in each filter area considered. Once the X-ray map was obtained, the non-silicon particles were subjected to microanalysis to assess the presence of a large amount of carbon atoms (large intensity of the C peak in the EDX spectra), which indicated the presence of the microplastics (Figure 2). In more detail, all the investigated polymers are mainly composed of C and H, but hydrogen cannot be detected by EDX. O and Cl are also present in PET and PVC, respectively, but oxygen is always detected in all samples exposed in air, and chlorine EDX signal is usually hidden by the carbon peak. Therefore, a large presence of C atoms is used to identify the microplastics (Ríos et al., 2020). We set the lowest limit of the measurements performed with SEM to 2 µm.
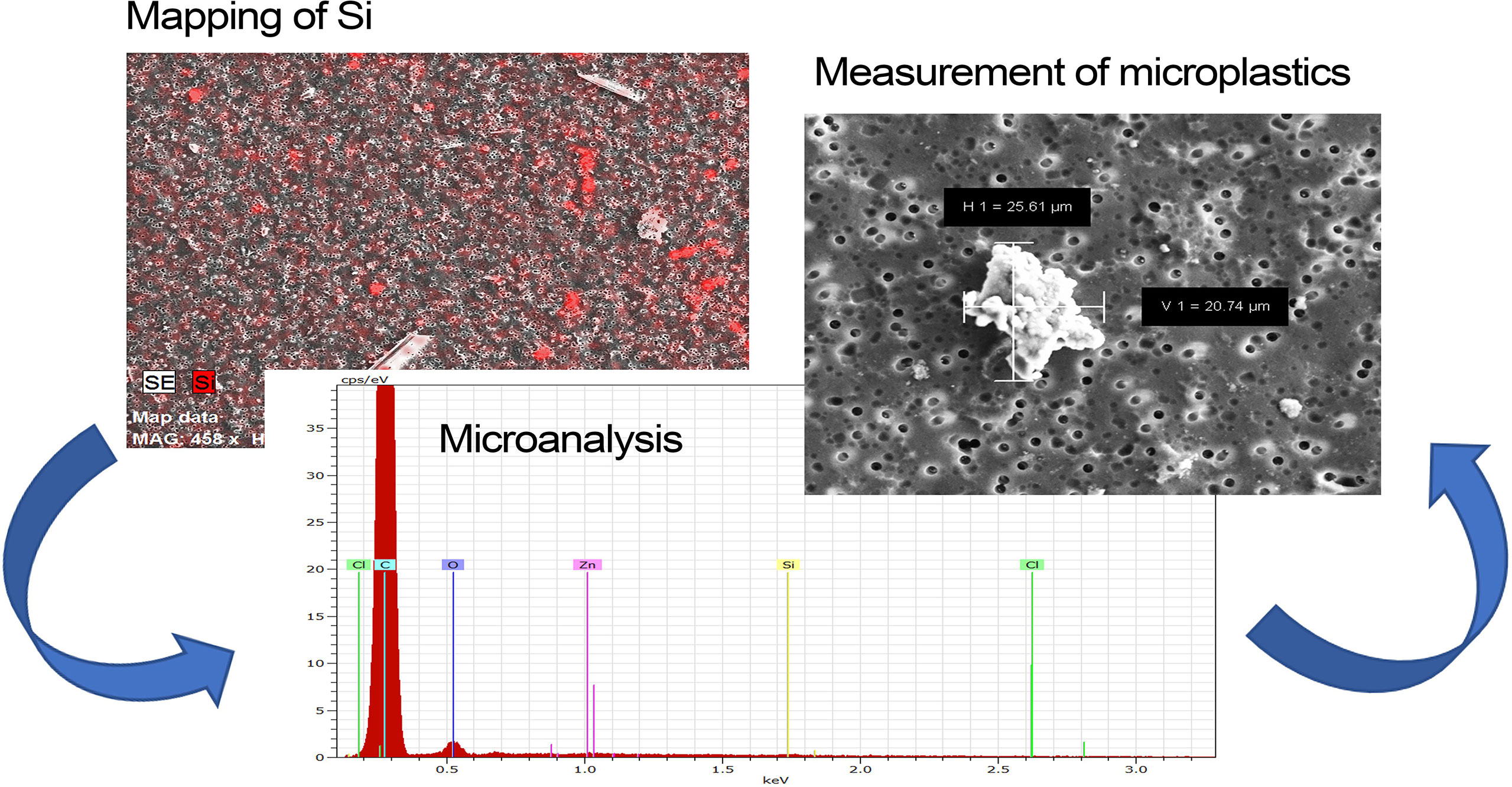
Figure 2 Workflow of microplastic identification by Scanning Electron Microscopy (SEM). Reported are the silicon EDX map (Mapping of Si), which is used to distinguish the sediment grains from the other inorganic particles, and the EDX microanalysis (i.e., Microanalysis) of the Si-free inorganic particles (i.e., Measurement of microplastics) used to verify the large presence of C, which is the specific element of the microplastics (H cannot be detected by EDX).
Microplastics (2-1000 µm) in deep-sea sediments
The analysis of the microplastic contamination in the deep-sea sediments was carried out using the procedure that resulted in the highest efficient extraction (i.e., based on the sediment partitioning, PS). It was applied to the same deep-sea sediment used for the determination of the extraction efficiency of the method, without the addition of the microplastic mixture.
Abundance and chemical identity (only for the fraction 20-1000 µm) of the polymers “naturally” present in the samples were determined. The abundances of the microplastic particles determined in the deep-sea sediments from the San Gregorio canyon were corrected considering the extraction efficiency of the method (on average, 60%).
Quality controls
Reduction of cross-contamination risks was carried out according to Frias et al., 2018. Lab staff wore cotton lab coats to reduce contamination from synthetic fabrics. Extraction procedures were carried out in a clean room and all the solutions used were filtered in a nitrocellulose filter of 0.2 µm (Sartori) to avoid the introduction of external contamination during the analysis. All products were opened exclusively under a laminar flow hood. None of the laboratory ware was made of plastic, we used only glass and metal laboratory ware previously washed and rinsed with ultrapure Milli-Q (Millipore) formerly filtered on nitrocellulose filters 0.2 µm and autoclaved.
All the solutions used in the analysis have been purchased from accredited companies and are easily available on the market (ZnCl2, Sigma-Aldrich CAS number: 7646-85-7; H2O2, Sigma-Aldrich CAS number 7722-84-1). To obtain the solution of Zinc Chloride (1.6-1.7 kg L-1), 900 g of ZnCl2 were weighed, then they were dissolved in 700 ml of ultrapure Milli-Q (Millipore).
Procedural blanks were performed during the analysis (including the counting phase by stereomicroscope). They consisted of three filters soaked in ZnCl2, H2O2, and Milli Q to evaluate both the presence of contamination in the working solutions, and in the laboratory airborne. The blanks contained (only the one soaked with Milli Q) very low contamination from cotton fibers as confirmed by FT-IR and were not included in the final amount of microplastics found in the samples.
Statistical analyses
Since data comply with the assumptions of normality (checked by Excel package), differences in terms of efficiency between the two approaches (BS and PS) were tested by ANOVA one-way tests with Bonferroni correction whereas differences in the abundances of different microplastic polymers and their size were analyzed through PERMANOVA (Anderson et al., 2008) according to a one-way experimental design and the analyses were carried out on Euclidean distance-based resemblance matrixes of untransformed data. For the one-way test, a P-value was provided using unrestricted permutation of raw data. Level of significance for the rejection of the null-hypothesis was set at α < 0.05. When low unique values in the permutation distribution were available, asymptotical Monte Carlo P-values were used instead of permutational P-values. All the analyses were performed using PRIMER 6 and PERMANOVA+ (Anderson et al., 2008).
Results and discussion
The present procedure has been specifically optimized for the extraction of microplastic particles from deep-sea sediments, for which ad hoc protocols have not yet been developed although these habitats are characterized by different characteristics from coastal sediments, such as a dominant silt-clay fraction (Smith et al., 2008; Danovaro et al., 2010), which can hamper the efficient recovery of microplastic particles. In this regard, we assessed for the first time the extraction efficiency of microplastics from deep-sea sediments, by comparing two different approaches, in order to maximize their recovery.
The procedure is essentially based on the use of ZnCl2 as a density separation solution that is useful to recover also high-density polymers (potentially present in benthic systems), and on H2O2 used to eliminate organic matter (Imhof et al., 2012). A new ultracentrifuge step (17,000 ×g) was added to increase the efficiency of separation of microplastics from fine-grained sediments, typically found in deep-sea environments (Stow, 1985; Smith et al., 2008; Danovaro et al., 2010). Furthermore, we modified the 3,500 ×g centrifugation step applied by Van Cauwenberghe et al., 2013 to coastal sediments to tailor it to abyssal sediments. In particular, we extended the gravity time (3,500 ×g to 17,000 ×g) to increase the precipitation of finer sediment particles.
Previous studies highlighted possible drawbacks associated with the use of ultracentrifugation, which could alter the sample by forming aggregates or damaging plastic particles (Schwaferts et al., 2019). However, this step was essential to carry out the identification of microplastics through FT-IR and SEM, due to the high background caused by the fine-grained size of our samples.
The extraction efficiency of microplastic particles from biological (i.e., marine organisms and their tissues) or abiotic (e.g., seawater and sediment samples) matrices is a key step in the development of a reliable procedure and to determine the extent of their unrecovered part. In this regard, two approaches were assessed in the present study: the first applied to bulk sediment (BS), and the second one to partitioned sediment in different aliquots (PS), which had been shown to be more efficient in extracting microscopic biological particles from deep-sea sediments (Corinaldesi et al., 2017).
Since the detection limit of chemical identification of microplastics by FT-IR is 10-20 µm (Löder and Gerdts, 2015), the fraction of microplastics smaller than 20 µm was analyzed by Scanning Electron Microscopy (SEM), which to our knowledge has never been applied so far for this purpose. Our method based on the use of SEM to quantify microplastic particles smaller than 20 µm in marine sediments, has been for the first time applied to deep-sea sediment samples, although previous investigations have used a similar approach to identify larger plastics in other marine habitats/samples (Tiwari et al., 2019; Duan et al., 2020). This procedure is also based on a pre-screening through the silicon mapping before analyzing the elemental composition of the microplastic particles, allowing us to identify them without scanning every single particle of the optical field: a procedure that would be extremely difficult and slow.
From the comparison of the BS and PS approaches we observed that the extraction efficiency of all the five polymers (size: 20-1000 µm) from the partitioned sediment samples was double compared to the extraction efficiency obtained from the bulk sediment (62% vs. 29%, respectively; P<0.001).
The most efficient approach (PS), when applied to the extraction procedure of microplastics of sizes from 2 to 20 µm resulted in a recovery efficiency of 57%, which is similar to that obtained for the extraction of 20-1000 µm polymers, suggesting that this procedure is suitable also for the recovery of the most abundant fraction of microplastics in the marine environment (Brandon et al., 2020).
These findings are consistent with previous studies for the extraction of biological particles even smaller than the microplastic particles (i.e. prokaryotes and viruses) from deep-sea sediment samples (Ellery and Schleyer, 1984; Danovaro et al., 2001b), which reported greater recovery efficiency when sediments were partitioned rather than when they were processed as a whole (Corinaldesi et al., 2017). Therefore, we can conclude that the partitioning of the sediment into aliquots favors the extraction of microplastics in the size range of 2-1000 µm from deep-sea sediments or other fine-grained sediments.
The extraction efficiency of microplastic particles obtained here from deep-sea sediments falls within the range of values obtained in previous studies on shallow-water sediments based on the density separation methods and other approaches such as the Munich Plastic Sediment Separator (ca. 40-95%, Imhof et al., 2012; Zobkov and Esiukova, 2017). Conversely, to our knowledge, only a single investigation evaluated the extraction efficiency of microplastics from deep-sea sediments, but the target polymers had a much larger size than that analyzed in our study (Barrett et al., 2020), therefore it does not allow us to make adequate and consistent comparisons.
In our investigation, we observed that the extraction efficiency changed depending on the polymer types (Figure 3) and their size range (Figure 4). Both approaches resulted in more efficient extraction of polyethylene, propylene, and polystyrene (BS: 32-40% and PS: 50-80%) than for PET and PVC particles (although the differences in polystyrene, PET and PVC abundances were not significant).
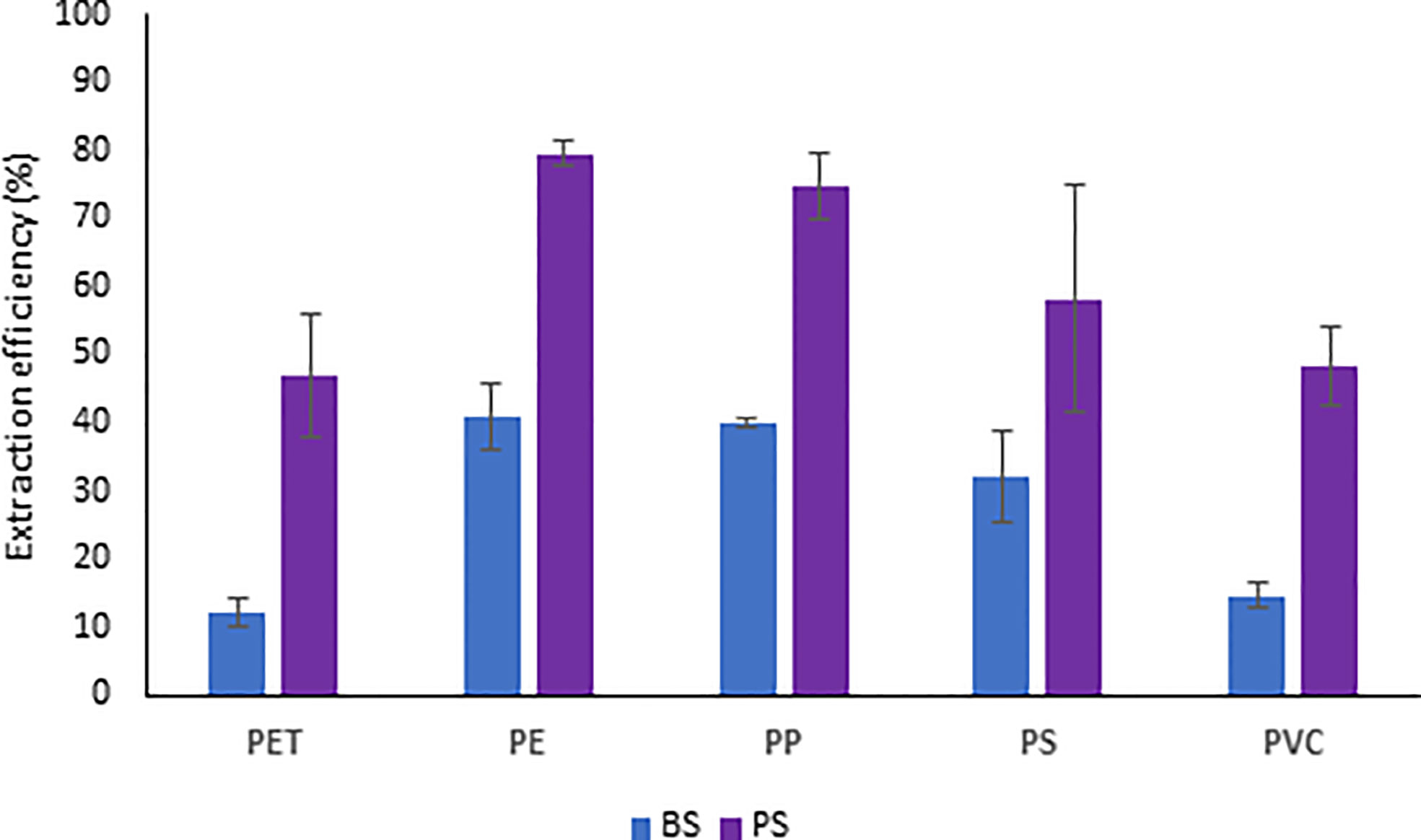
Figure 3 Extraction efficiency of microplastics (20-1000 µm) from deep-sea sediments based on two different approaches applied to bulk (BS) and partitioned (PS) sediments.
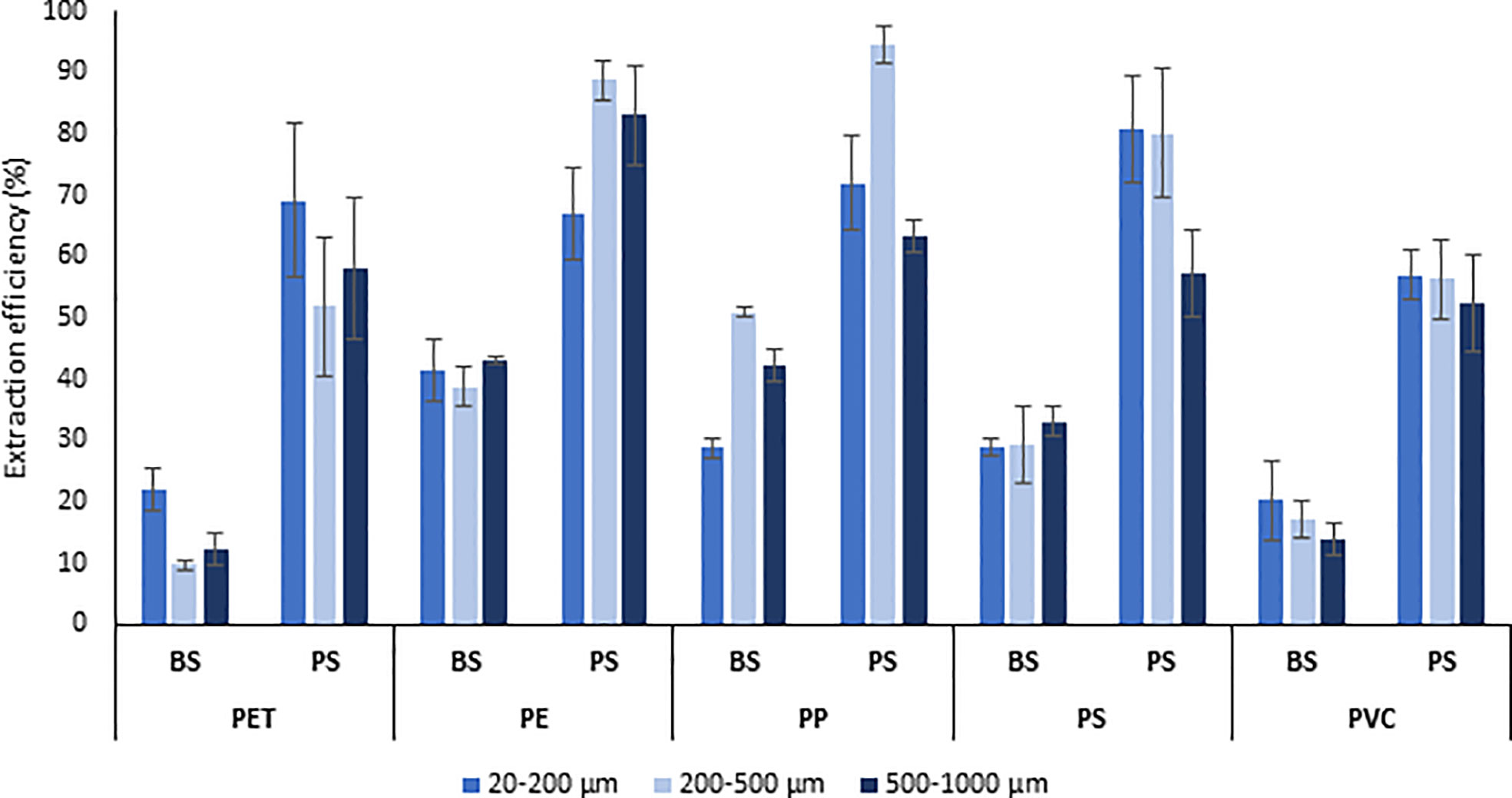
Figure 4 Extraction efficiency (%) of different microplastic polymers and size ranges (500-1000 µm, 200-500 µm, and 20-200 µm) from deep-sea sediments comparing the two different approaches applied to bulk (BS) and partitioned (PS) sediments. PET, Polyethylene terephthalate; PE, Polyethylene; PP, polypropylene; PS, polystyrene; PVC, Polyvinyl chloride.
Previous investigations conducted on coastal sediments have revealed that some polymers such as PE and PA were extracted more efficiently than PET (Rivoira et al., 2020) whereas other studies reported a similar extraction efficiency among different polymers (Imhof et al., 2012; Nuelle et al., 2014). However, this discrepancy might be due to the different sedimentary granulometry and organic matter content/composition, as well as the different sizes of the target microplastic and polarity and the procedures used for extraction (Bellasi et al., 2021; Halbach et al., 2021).
The extraction efficiency of the different polymers was quite similar among the different size ranges considered (20-200, 200-500, and 500-1000 µm), except for PP, which regardless of the type of approach used (BS or PS) was characterized by a more variable recovery efficiency, and PET, which was extracted less efficiently in the size range 200-500 µm than in the 20-200 µm (with significant differences only for the approach BS). However, comparing the extraction efficiency of polymers included in the same size range, this was always higher when the PS approach, rather than BS (P <0.05), was used, confirming the maximum effectiveness of the sediment partitioning approach for all the different sizes of the analyzed microplastics (20-1000µm).
The extraction efficiency of the microplastic particles smaller than 20 µm was determined using the samples processed with the approach PS. However, in this case, we couldn’t provide the extraction efficiency for each polymer but the whole mixture since the EDX microanalysis is unable to distinguish among the different polymers (Figure 5).
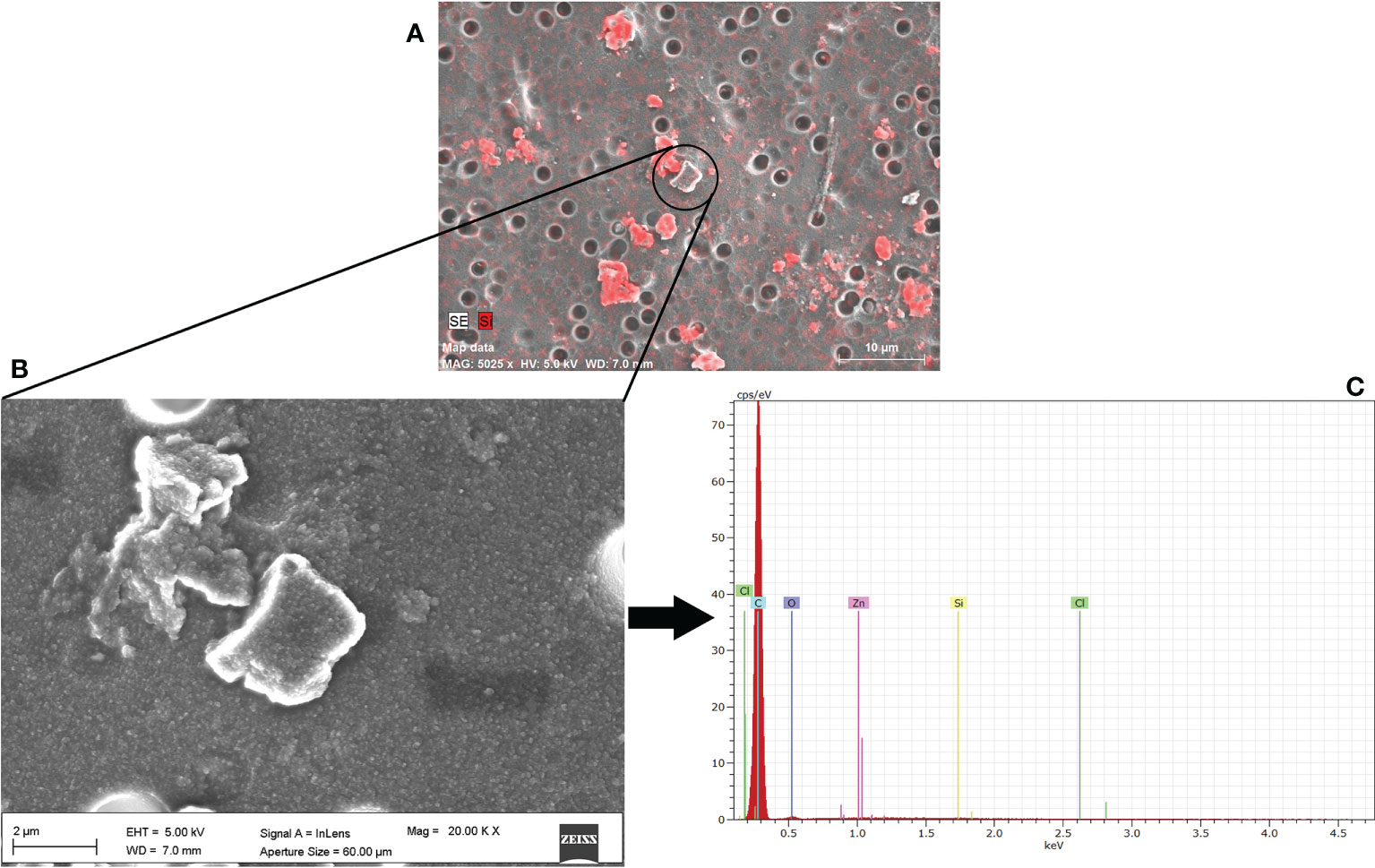
Figure 5 Identification of microplastic particles (2-20 µm) extracted from deep-sea sediments by Scanning Electron Microscopy. (A) Silicon EDX map (mapping Si mineral) used to distinguish sediments from the other inorganic particles, (B) close-up of microplastic particles (C) EDX spectrum confirming the large presence of C atoms (the highest peak). Carbon is present in all the investigated plastics and therefore cannot be used for recognizing the different polymers.
When the most efficient procedure (PS) was used to determine microplastic contamination in the sediments of the Mediterranean canyon, we found an abundance of particles of 20-1000 µm ranging, on average, from 420 ± 73.48 to 1080 ± 374.70 particles m-2 at 910 and 304-m depth, respectively (i.e., 47-120 particles kg-1, Figure 6), which showed a different partitioning among the different size ranges (20-200, 200-500, and 500-1000 µm) along the bathymetric gradient (Figure 7). These abundances were up to four times higher than those found in the deep-sea sediments of the Eastern (Nile Deep-Sea Fan, 1176-m depth; Van Cauwenberghe et al., 2013; 40 particles kg-1) and Western Mediterranean (Tyrrhenian Sea, 200-1344-m depth; Kane et al., 2020, 20 particles kg-1) considering samples at similar water depths and target microplastic ranges. However, further studies on a wider spatial scale will be needed to assess the microplastic contamination in deep-sea ecosystems.
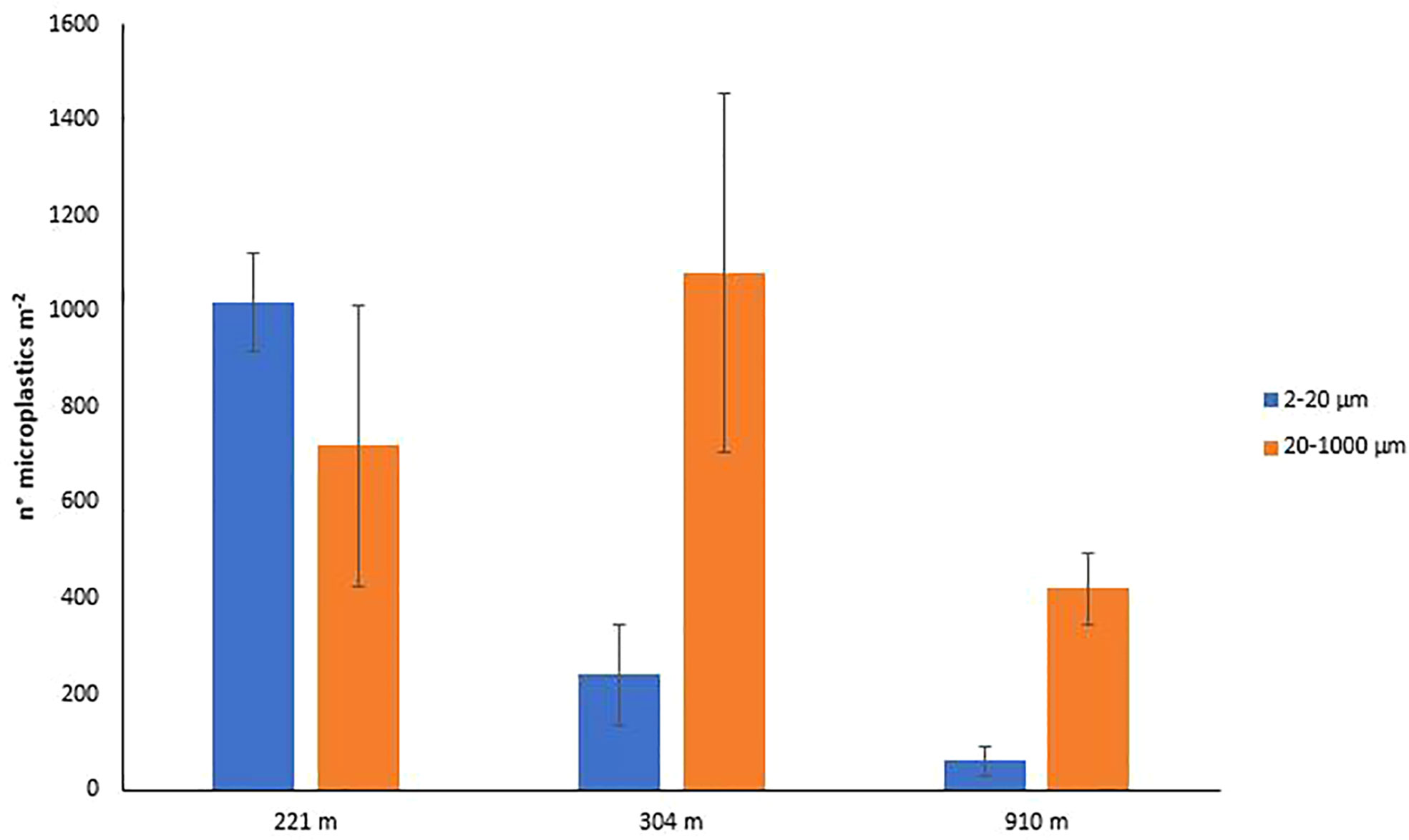
Figure 6 Microplastic abundance (size ranges: 2-20 and 20-1000 µm) in the deep-sea sediments of the San Gregorio canyon.
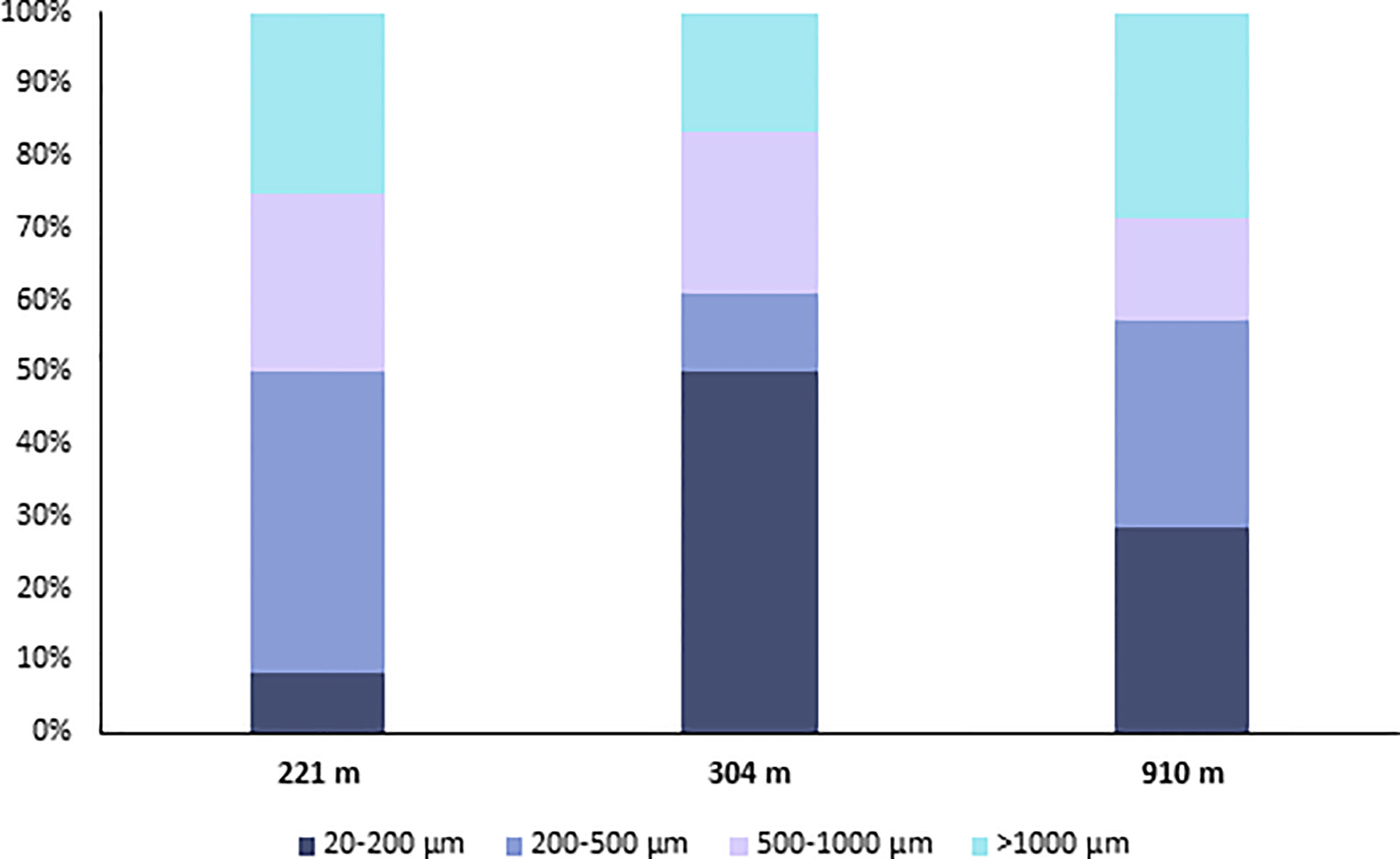
Figure 7 Partitioning of microplastics among the different size ranges in the deep-sea sediments of the San Gregorio canyon.
The protocol presented here, allowed us to quantify for the first time the abundance of microplastics in the 2-20 µm size range in the deep-sea sediments of the San Gregorio canyon (Central Mediterranean). In particular, the most conservative estimates obtained ranged, on average, from 60 ± 31.80 to 1020 ± 103.93 particles m-2 (at 910 and 221-m depth, respectively; Figure 6), thus contributing from ca. 13% to ca. 60% of the microplastic fraction 2-1000 µm. Our results allow us to hypothesize that the relevance of microplastics smaller than 20 µm and, their potential negative impacts on benthic organisms, could decrease with increasing water depth. This hypothesis, however, should be confirmed through the analysis of a larger set of samples and deep-sea habitats.
In the canyon sediments, we found a wide diversity of plastic polymers such as polystyrene, nylon, polyethylene terephthalate, polyester, polytetrafluoroethylene, polypropylene, polyurethane, polyamide, polyvinyl chloride, polyethylene, SIS (styrene-isoprene block copolymer), epoxy resin, EVA (ethylene-vinyl acetate), acrylates, and polyvinyl chloride with a high contribution of phthalic plasticizer, putative KEVLAR, PE+EVA (polyethylene + ethylene-vinyl acetate) (Figure 8; Figures S1- S4). In particular, a higher number of polymers (10 different typologies) were observed in the sediments located at the intermediate depth (304 m) where polyethylene and acrylates were the most abundant (40% each). Conversely, the polyvinyl chloride + phthalic plasticizer prevailed at 221-m depth (80%), while SIS was the most represented at ca. 900-m depth (33%). Despite a large portion of plastic polymers, at ca. 900-m depth, showed a relatively high density (ranging from 1.04 to 2.2 g cm-3, for polystyrene and polytetrafluoroethylene, respectively) we found no relationship between water depth and the density of the plastic polymers, indicating that biological and physical processes can interfere with the sinking of the plastic (Galloway et al., 2017; Kane et al., 2020).
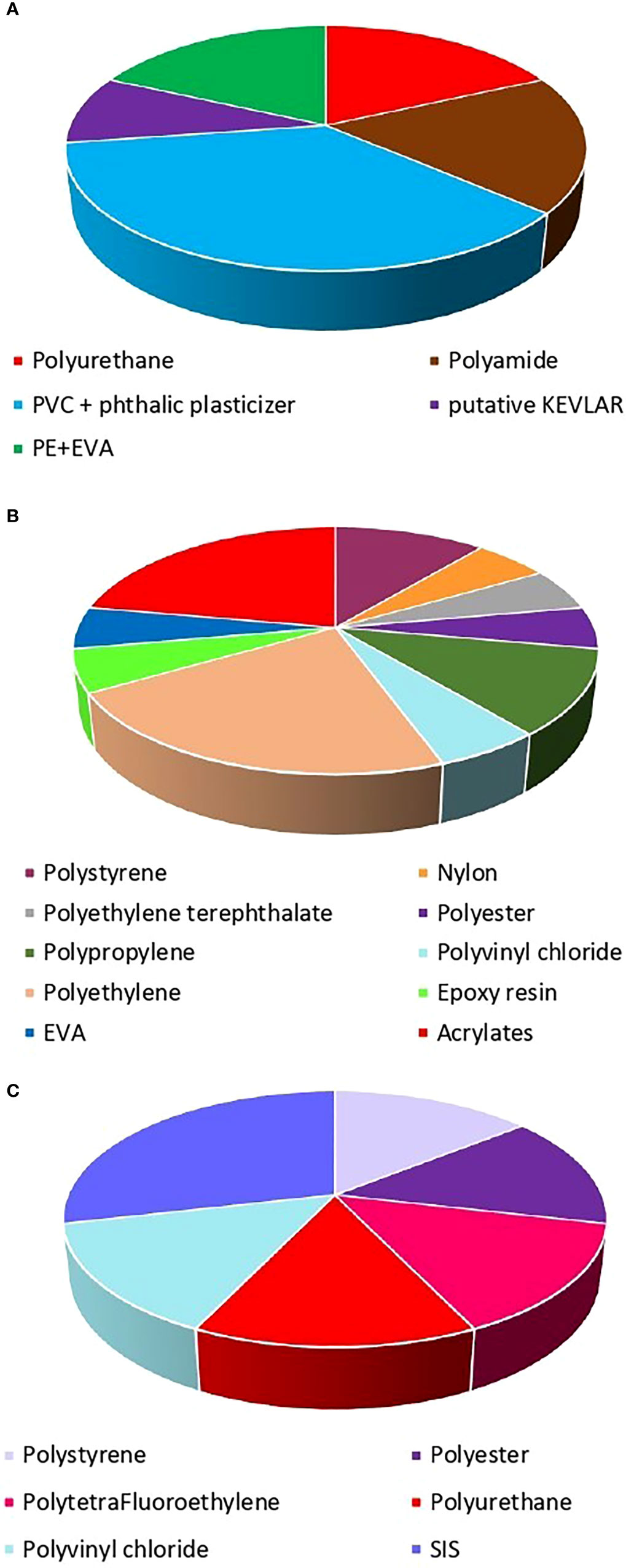
Figure 8 Polymeric composition of microplastics in the deep-sediments at (A) 221 m, (B) 304 m and (C) 910 m in San Gregorio canyon.
While polyethylene’s impact on marine benthic organisms, has been already documented (Corinaldesi et al., 2021; Corinaldesi et al., 2022), the effects of acrylate polymers are still poorly known.
The risk to aquatic organisms associated with phthalic acids, which are the most common plasticizers used in polyvinyl chloride products, is also well known (Baloyi et al., 2021). These compounds have been indeed reported to act as endocrine disruptors raising also concerns for human health (Bi et al., 2021).
The most abundant polymers found at the deepest site investigated here, the SIS, is a copolymer employed as an impact modifier and toughener in plastics and (structural) adhesives (https://polymerdatabase.com) and is widely used in sealants, gasket materials, rubber bands, toy products, shoe soles, bitumen products for road paving and antifouling paints. Although SIS has been found in the intestines of deep-sea benthic organisms, suggesting its potential elimination from the organism (Cau et al., 2020), at the same time the negative effects of styrene on marine organisms have been documented (Mamaca et al., 2005; Wathsala et al., 2018).
The identification of plastic polymers in deep-sea sediments, including some polymers already reported to be harmful to marine organisms, confirms that deep-sea canyons can act as a final reservoir for the accumulation of microplastics, representing a potential risk for benthic communities and trophic webs.
Concluding the protocol here optimized for the extraction of microplastics from deep-sea sediments appears to be efficient in recovering even microplastic particles smaller than 20 µm. Unfortunately, the analysis of the chemical composition and identification of the smallest polymers (< 20 µm) by SEM is still laborious, time-consuming, and with high costs associated. Furthermore, despite Raman Spectroscopy has been also used to investigate microplastic particles < 20 µm in environmental samples (Anger et al., 2018; Schwaferts et al., 2019) it has limitations associated with misidentification due to the presence of concurrent fluorescence interference, which may be intrinsic to the main constituent of the plastic fragment or due to impurities such as dyes, degradation products, and biological material (Kavya et al., 2020; Nava et al., 2021). Due to these limitations, including those associated with the most widely used FT-IR-based technology (i.e., detection limit: 10-20 µm), the analysis of microplastics < 20 µm remains a challenge. Further studies should be directed to the use and optimization of complementary technologies, such as X-ray diffraction and X-ray photoelectron spectroscopy, to identify nano- and microplastics and to understand their distribution in the marine environment (Mai et al., 2018).
Conclusions
The protocol presented here is suitable for the extraction of the most relevant plastic polymers found in a marine environment especially polystyrene, polypropylene, and polyvinyl chloride which are reported to be the most abundant in deep-sea environments and with multiple negative effects on marine organisms.
This procedure allows the efficient extraction of microplastics (on average ca. 60% and up to ca. 80% for polyethylene) with dimensions smaller than 1000 µm. Due to the relevant expected impact of these microplastic fractions also on deep-sea trophic webs, the protocol presented here can represent a reference point for the extraction of microplastics from deep-sea sediments.
Data availability statement
The original contributions presented in the study are included in the article/Supplementary Material. Further inquiries can be directed to the corresponding author.
Author contributions
CC conceived the study. SC performed laboratory analyses and data elaboration. GB supervised SEM analyses. SC drafted the first version of the manuscript. All authors contributed to the article and approved the submitted version.
Funding
This study has been conducted in the framework of the National Flag Project RITMARE (Marine Italian Research).
Acknowledgments
We thank Adriano Di Cristoforo for the support in the Scanning Electron Microscopy analyses and Carlo Giacomo Avio, Stefania Gorbi, Lucia Pittura and Simona Sabbatini for the support in the FT-IR analyses.
Conflict of interest
The authors declare that the research was conducted in the absence of any commercial or financial relationships that could be construed as a potential conflict of interest.
Publisher’s note
All claims expressed in this article are solely those of the authors and do not necessarily represent those of their affiliated organizations, or those of the publisher, the editors and the reviewers. Any product that may be evaluated in this article, or claim that may be made by its manufacturer, is not guaranteed or endorsed by the publisher.
Supplementary material
The Supplementary Material for this article can be found online at: https://www.frontiersin.org/articles/10.3389/fmars.2022.975875/full#supplementary-material
References
Abel S. M., Primpke S., Int-Veen I., Brandt A., Gerdts G. (2021). Systematic identification of microplastics in abyssal and hadal sediments of the kuril kamchatka trench. Environ. Pollut. 269, 116095. doi: 10.1016/j.envpol.2020.116095
Anderson M., Gorley R., Clarke K. P. (2008). For PRIMER: guide to software and statistical methods (Plymouth, UK: Primer-e).
Anger P. M., von der Esch E., Baumann T., Elsner M., Niessner R., Ivleva N. P. (2018). Raman microspectroscopy as a tool for microplastic particle analysis. Trends Analyt. Chem. 109, 214–226. doi: 10.1016/j.trac.2018.10.010
Baloyi N. D., Tekere M., Maphangwa K. W., Masindi V. (2021). Insights into the prevalence and impacts of phthalate esters in aquatic ecosystems. Front. Environ. Sci. 9. doi: 10.3389/fenvs.2021.684190
Barrett J., Chase Z., Zhang J., Holl M. M. B., Willis K., Williams A., et al. (2020). Microplastic pollution in deep-Sea sediments from the great Australian bight. Front. Mar. Sci. 7. doi: 10.3389/fmars.2020.576170
Bellasi A., Binda G., Pozzi A., Boldrocchi G., Bettinetti R. (2021). The extraction of microplastics from sediments: An overview of existing methods and the proposal of a new and green alternative. Chemosphere 278, 130357. doi: 10.1016/j.chemosphere.2021.130357
Bergmann M., Wirzberger V., Krumpen T., Lorenz C., Primpke S., Tekman M. B., et al. (2017). High quantities of microplastic in Arctic deep-sea sediments from the HAUSGARTEN observatory. Environ. Sci. Technol. 51, 11000–11010. doi: 10.1021/acs.est.7b03331
Bi M., Liu W., Luan X., Li M., Liu M., Liu W., et al. (2021). Production, use, and fate of phthalic acid esters for polyvinyl chloride products in China. Environ. Sci. Technol. 55 (20), 13980–13989. doi: 10.1021/acs.est.1c02374
Brandon J. A., Freibott A., Sala L. M. (2020). Patterns of suspended and salp- ingested microplastic debris in the north pacific investigated with epifluorescence microscopy. LO Lett. 5, 46–53. doi: 10.1002/lol2.10127
Cashman M. A., Ho K. T., Boving T. B., Russo S., Robinson S., Burgess R. M. (2020). Comparison of microplastic isolation and extraction procedures from marine sediments. Mar. pollut. Bull. 159, 111507. doi: 10.1016/j.marpolbul.2020.111507
Cau A., Avio C. G., Dessì C., Moccia D., Pusceddu A., Regoli F., et al. (2020). Benthic crustacean digestion can modulate the environmental fate of microplastics in the deep sea. Environ. Sci. Technol. 54 (8), 4886–4892. doi: 10.1021/acs.est.9b07705
Claessens M., Cauwenberghe L. V., B. Vandegehuchte B., Janssen C. R. (2013). New techniques for the detection of microplastics in sediments and field collected organisms. Mar. Pollut. Bull. 70, 227–233. doi: 10.1016/j.marpolbul.2013.03.009
Coffin S., Wyer H., Leapman J. C. (2021). Addressing the environmental and health impacts of microplastics requires open collaboration between diverse sectors. PloS Biol. 19 (3), e3000932. doi: 10.1371/journal.pbio.3000932
Corinaldesi C., Canensi S., Carugati L., Martire M. L., Marcellini F., Nepote E., et al (2022). Organic enrichment can increase the impact of microplastics on meiofaunal assemblages in tropical beach systems. Enviro. Pollut. 292, 118415.
Corinaldesi C., Canensi S., Dell’Anno A., Tangherlini M., Di Capua I., Varrella S., et al. (2021). Multiple impacts of microplastics can threaten marine habitat-forming species. Commun. Biol. 4 (1), 1–13. doi: 10.1038/s42003-021-01961-1
Corinaldesi C., Canensi S., Carugati L., Martire M. L., Marcellini F., Nepote E., et al. (2021b). Organic enrichment can increase the impact of microplastics on meiofaunal assemblages in tropical beach systems. Environ. Pollut. 292, 118415. doi: 10.1016/j.envpol.2021.118415
Corinaldesi C., Tangherlini M., Dell’Anno A. (2017). From virus isolation to metagenome generation for investigating viral diversity in deep-sea sediments. Sci. Rep. 7 (1), 1–12. doi: 10.1038/s41598-017-08783-4
Courtene-Jones W., Quinn B., Ewins C., Gary S. F., Narayanaswamy B. E. (2020). Microplastic accumulation in deep-sea sediments from the rockall trough. Mar. pollut. Bull. 154, 111092. doi: 10.1016/j.marpolbul.2020.111092
Courtene-Jones W., Quinn B., Murphy F., Gary S. F., Narayanaswamy B. E. (2017). Optimisation of enzymatic digestion and validation of specimen preservation methods for the analysis of ingested microplastics. Anal. Methods 9, 1437–1445. doi: 10.1039/c6ay02343f
Cunningham E. M., Ehlers S. M., Dick J. T., Sigwart J. D., Linse K., Dick J. J., et al. (2020). High abundances of microplastic pollution in deep-sea sediments: evidence from Antarctica and the southern ocean. Environ. Sci. Technol. 54 (21), 13661–13671. doi: 10.1021/acs.est.0c03441
Danovaro R. (2010). Methods for the study of deep-Sea sediments, their functioning and biodiversity (Boca Raton, FL: CRC Press).
Danovaro R., Company J. B., Corinaldesi C., D’Onghia G., Galil B., Gambi C., et al. (2010). Deep-sea biodiversity in the Mediterranean Sea: The known, the unknown, and the unknowable. PloS One 5 (8), e11832. doi: 10.1371/journal.pone.0011832
Danovaro R., Dell Anno A., Fabiano M. (2001). Bioavailability of organic matter in the sediments of the porcupine abyssal plain, northeastern Atlantic. Mar. Ecol. Prog. Ser. 220, 25–32. doi: 10.3354/meps220025
Danovaro R., Dell’Anno A., Trucco A., Serresi M., Vanucci S. (2001b). Determination of virus abundance in marine sediments. AEM 67 (3), 1384–1387. doi: 10.1128/AEM.67.3.1384-1387.2001
Dekiff J. H., Remy D., Klasmeier J., Fries E. (2014). Occurrence and spatial distribution of microplastics in sediments from norderney. Environ. Pollut. 186, 248–256. doi: 10.1016/j.envpol.2013.11.019
Duan J., Han J., Zhou H., Lau Y. L., An W., Wei P., et al. (2020). Development of a digestion method for determining microplastic pollution in vegetal-rich clayey mangrove sediments. Sci. Total Environ. 707, 136030. doi: 10.1016/j.scitotenv.2019.136030
Ellery W. N., Schleyer M. H. (1984). Comparison of homogenization and ultrasonication as techniques in extracting attached sedimentary bacteria. Mar. Ecol. Prog. Ser. Oldendorf 15 (3), 247–250.
Filella M. (2015). Question of size and numbers in environmental research on microplastics: methodological and conceptual aspects. Environ. Chem. 12, 527–538. doi: 10.1071/EN15012
Fischer V., Elsner N., Brenke N., Schwabe E., Brandt A. (2015). Plastic pollution of the kuril–kamchatka trench area (NW pacific). Deep-Sea Res. Pt II. 111, 399–405. doi: 10.1016/j.dsr2.2014.08.012
Frias J., Pagter E., Nash R., O’Connor I., Carretero O., Filgueiras A., et al. (2018). Standardised protocol for monitoring microplastics in sediments. (JPI-oceans BASEMAN project). doi: 10.13140/RG.2.2.36256.89601/1
Galloway T. S., Cole M., Lewis C. (2017). Interactions of microplastic debris throughout the marine ecosystem. Nat. Ecol. Evol. 1, 1–8. doi: 10.1038/s41559-017-0116
Ghsoub M., Fakhri M., Courp T., Khalaf G., Buscail R., Ludwig W. (2020). River signature over coastal area (Eastern mediterranean): grain size and geochemical analyses of sediments. Reg. Stud. Mar. Sci. 35, 101169. doi: 10.1016/j.rsma.2020.101169
Halbach M., Baensch C., Dirksen S., Scholz-Böttcher B. M. (2021). Microplastic extraction from sediments established? – a critical evaluation from a trace recovery experiment with a custom-made density separator. Analytical Methods. 13(44), 5299–5308. doi: 10.1039/D1AY00983D
Hamilton E. L. (1976). Variations of density and porosity with depth in deep-sea sediments. J. Se. Res. 46 (2), 280–300. doi: 10.1306/212F6F3C-2B24-11D7-8648000102C1865D
Hartmann N. B., Huffer T., Thompson R. C., Hassellöv M., Verschoor A., Daugaard A. (2019). Are we speaking the same language? Recommendations for a definition and categorization framework for plastic debris. Environ. Sci. Technol. 53 (3), 1039–1047. doi: 10.1021/acs.est.8b05297
Hidalgo-Ruz V., Gutow L., Thompson R. C., Thiel M. (2012). Microplastics in the marine environment: a review of the methods used for identification and quantification. Environ. Sci. Technol. 46, 3060–3075. doi: 10.1021/es2031505
Imhof H. K., Schmid J., Niessner R., Ivleva N. P., Laforsch C. (2012). A novel, highly efficient method for the separation and quantification of plastic particles in sediments of aquatic environments. Limnol. Oceanogr. Meth. 10, 524–537. doi: 10.4319/lom.2012.10.524
Imhof H. K., Sigl R., Brauer E., Feyl S., Giesemann P., Klink S., et al. (2017). Spatial and temporal variation of macro-, meso-and microplastic abundance on a remote coral island of the Maldives, Indian ocean. Mar. Pollut. Bull. 116 (1-2), 340–347. doi: 10.1016/j.marpolbul.2017.01.010
Kane I. A., Clare M. A., Miramontes E., Wogelius R., Rothwell J. J., Garreau P., et al. (2020). Seafloor microplastic hotspots controlled by deep-sea circulation. Science 368 (6495), 1140–1145. doi: 10.1126/science.aba5899
Kavya A. N. L., Sundarrajan S., Ramakrishna S. (2020). Identification and characterization of micro-plastics in the marine environment: A mini review. Mar. Pollut. Bull. 160, 111704. doi: 10.1016/j.marpolbul.2020.111704
Kedzierski M., Le Tilly V., Bourseau P., Bellegou H., César G., Sire O., et al. (2016). Microplastics elutriation from sandy sediments: A granulometric approach. Mar. Pollut. Bull. 107, 315–323. doi: 10.1016/j.marpolbul.2016.03.041
Kedzierski M., Le Tilly V., César G., Sire O., Bruzaud S. (2017). Efficient microplastics extraction from sand. a cost effective methodology based on sodium iodide recycling. Mar. Pollut. Bull. 115 (1), 120–129. doi: 10.1016/j.marpolbul.2016.12.002
Khoironi A., Hadiyanto H., Anggoro S., Sudarno S. (2020). Evaluation of polypropylene plastic degradation and microplastic identification in sediments at tambak lorok coastal area, semarang, Indonesia. Mar. Pollut. Bull. 151, 110868. doi: 10.1016/j.marpolbul.2019.110868
La Daana K. K., Johansson C., Frias J. P. G. L., Gardfeldt K., Thompson R. C., O’Connor I. (2019). Deep sea sediments of the Arctic central basin: A potential sink for microplastics. Deep-Sea Res. I: Oceanogr. Res. Pap. 145, 137–142. doi: 10.1016/j.dsr.2019.03.003
Lavers J. L., Oppel S., Bond A. L. (2016). Factors influencing the detection of beach plastic debris. Mar. Environ. Res. 119, 245–251. doi: 10.1016/j.marenvres.2016.06.009
Lenz R., Enders K., Stedmon C. A., Mackenzie D. M. A., Nielsen T. G. (2015). A critical assessment of visual identification of marine microplastic using raman spectroscopy for analysis improvement. Mar. pollut. Bull. 100 (1), 82–91. doi: 10.1016/j.marpolbul.2015.09.026
Li W., Lo H. S., Wong H. M., Zhou M., Wong C. Y., Tam N. F. Y., et al. (2020). Heavy metals contamination of sedimentary microplastics in Hong Kong. Mar. pollut. Bull. 153, 110977. doi: 10.1016/j.marpolbul.2020.110977
Lindeque P. K., Cole M., Coppock R. L., Lewis C. N., Miller R. Z., Watts A. J., et al. (2020). Are we underestimating microplastic abundance in the marine environment? a comparison of microplastic capture with nets of different mesh-size. Environ. Pollut. 265, 114721. doi: 10.1016/j.envpol.2020.114721
Löder M. G., Gerdts G. (2015). “Methodology used for the detection and identification of microplastics_A critical appraisal,” in In marine anthropogenic litter (Cham: Springer), 201–227. Available at: 10.1007/978-3-319-16510-3_8.
Mai L., Bao L. J., Shi L., Wong C. S., Zeng E. Y. (2018). A review of methods for measuring microplastics in aquatic environments. Environ. Sci. pollut. Res. 25 (12), 11319–11332. doi: 10.1007/s11356-018-1692-0
Mamaca E., Bechmann R. K., Torgrimsen S., Aas E., Bjørnstad A., Baussant T., et al. (2005). The neutral red lysosomal retention assay and comet assay on haemolymph cells from mussels (Mytilus edulis) and fish (Symphodus melops) exposed to styrene. Aquat. Toxicol. 75 (3), 191–201. doi: 10.1016/j.aquatox.2005.08.001
Ma H., Pu S., Liu S., Bai Y., Mandal S., Xing B. (2020). Microplastics in aquatic environments: toxicity to trigger ecological consequences. Environ. pollut. 261, 114089. doi: 10.1016/j.envpol.2020.114089
McDermid K. J., McMullen T. L. (2004). Quantitative analysis of small-plastic debris on beaches in the Hawaiian archipelago. Mar. pollut. Bull. 48 (7-8), 790–794. doi: 10.1016/j.marpolbul.2003.10.017
Miller M. E., Kroon F. J., Motti C. A. (2017). Recovering microplastics from marine samples: A review of current practices. Mar. pollut. Bull. 123 (1-2), 6–18. doi: 10.1016/j.marpolbul.2017.08.058
Nava V., Frezzotti M. L., Leoni B. (2021). Raman spectroscopy for the analysis of microplastics in aquatic systems. J. Appl. Spectrosc. 75 (11), 1341–1357. doi: 10.1364/AS.75.001341
Nuelle M., Dekiff J. H., Remy D., Fries E. (2014). A new analytical approach for monitoring microplastics in marine sediments. Environ. Pollut. 184, 161–169. doi: 10.1016/j.envpol.2013.07.027
Paul-Pont I., Tallec K., Gonzalez-Fernandez C., Lambert C., Vincent D., Mazurais D., et al. (2018). Constraints and priorities for conducting experimental exposures of marine organisms to microplastics. Front. Mar. Sci. 5. doi: 10.3389/fmars.2018.00252
Peng X., Chen M., Chen S., Dasgupta S., Xu H., Ta K., et al. (2018). Microplastics contaminate the deepest part of the world’s ocean. Geochem. Perspect. Lett. 9 (1), 1–5. doi: 10.7185/geochemlet.1829
Peng L., Fu D., Qi H., Lan C. Q., Yu H., Ge C. (2020). Micro-and nano-plastics in marine environment: Source, distribution and threats–a review. Sci. Total Environ. 698, 134254. doi: 10.1016/j.scitotenv.2019.134254
Phuong N. N., Zalouk-Vergnoux A., Poirier L., Kamari A., Châtel A., Mouneyrac C. (2016). Is there any consistency between the microplastics found in the field and those used in laboratory experiments? Environ. Pollut. 211, 111–123. doi: 10.1016/j.envpol.2015.12.035
PlasticsEurope (2020). “Plastics - the facts 2020,” in An analysis of European latest plastics production (Frankfurt: Demand and Waste Data).
Qiu Q., Tan Z., Wang J., Peng J., Li M., Zhan Z. (2016). Extraction, enumeration and identification methods for monitoring microplastics in the environment. Estuar. Coast. Shelf. Sci. 176, 102–109. doi: 10.1016/j.ecss.2016.04.012
Quinn B., Murphy F., Ewins C. (2017). Validation of density separation for the rapid recovery of microplastics from sediment. Anal. Methods. 9(9), 1491–1498. doi: 10.1039/c6ay02542k
Ríos M. F., Hernández-Moresino R. D., Galván D. E. (2020). Assessing urban microplastic pollution in a benthic habitat of Patagonia Argentina. Mar. pollut. Bull. 159, 111491. doi: 10.1016/j.marpolbul.2020.111491
Rivoira L., Castiglioni M., Rodrigues S. M., Freitas V., Bruzzoniti M. C., Ramos S., et al. (2020). Microplastic in marine environment: Reworking and optimisation of two analytical protocols for the extraction of microplastics from sediments and oysters. MethodsX 7, 101116. doi: 10.1016/j.mex.2020.101116
Ruggero F., Gori R., Lubello C. (2020). Methodologies for microplastics recovery and identification in heterogeneous solid matrices: A review. J. Polym. Environ. 28, 1–10. doi: 10.1007/s10924-019-01644-3
Schwaferts C., Niessner R., Elsner M., Ivleva N. P. (2019). Methods for the analysis of submicrometer-and nanoplastic particles in the environment. Trends Analyt. Chem. 112, 52–65. doi: 10.1016/j.trac.2018.12.014
Shim W. J., Hong S. H., Eo S. E. (2017). Identification methods in microplastic analysis: a review. Analytical Methods 9 (9), 1384–1391. doi: 10.1039/C6AY02558G
Shim W. J., Song Y. K., Hong S. H., Jang M. (2016). Identification and quantification of microplastics using Nile red staining. Mar. pollut. Bull. 113, 469–476. doi: 10.1016/j.marpolbul.2016.10.049
Smith C. R., De Leo F. C., Bernardino A. F., Sweetman A. K., Arbizu P. M. (2008). Abyssal food limitation, ecosystem structure and climate change. Trends Ecol. Evol. 23 (9), 518–528. doi: 10.1016/j.tree.2008.05.002
Song Y. K., Hong S. H., Jang M., Han G. M., Rani M., Lee J., et al. (2015). A comparison of microscopic and spectroscopic identification methods for analysis of microplastics in environmental samples. Mar. Pollut. Bull. 93, 202–209. doi: 10.1016/j.marpolbul.2015.01.015
Stolte A., Forster S., Gerdts G., Schubert H. (2015). Microplastic concentrations in beach sediments along the German Baltic coast. Mar. Pollut. Bull. 99, 216–229. doi: 10.1016/j.marpolbul.2015.07.022
Stow D. A. V. (1985). Deep-sea clastics: where are we and where are we going? Geological Society London Special Publications 18 (1), 67–93. doi: 10.1144/GSL.SP.1985.018.01.05
Tagg A. S., Sapp M., Harrison J. P., Ojeda J. J. (2015). Identification and quantification of microplastics in wastewater using focal plane array-based reflectance micro-FT-IR imaging. J. Anal. Chem. 87 (12), 6032–6040. doi: 10.1144/GSL.SP.1985.018.01.0
Tekman M. B., Wekerle C., Lorenz C., Primpke S., Hasemann C., Gerdts G., et al. (2020). Tying up loose ends of microplastic pollution in the Arctic: Distribution from the Sea surface through the water column to deep-Sea sediments at the HAUSGARTEN observatory. J. Environ. Sci. Technol. 54 (7), 4079–4090. doi: 10.1021/acs.est.9b06981
Thompson R. C., Olsen Y., Mitchell R. P., Davis A., Rowland S. J., John A. W., et al. (2004). Lost at sea: where is all the plastic? Science 304 (5672), 838–838. doi: 10.1126/science.1094559
Tiwari M., Rathod T. D., Ajmal P. Y., Bhangare R. C., Sahu S. K. (2019). Distribution and characterization of microplastics in beach sand from three different Indian coastal environments. Mar. pollut. Bull. 140, 262–273. doi: 10.1016/j.marpolbul.2019.01.055
Uddin S., Fowler S. W., Uddin M. F., Behbehani M., Naji A. (2021). A review of microplastic distribution in sediment profiles. Mar. Pollut. Bull. 163, 111973. doi: 10.1016/j.marpolbul.2021.111973
Van Cauwenberghe L., Devriese L., Galgani F., Robbens J., Janssen C. R. (2015). Microplastics in sediments: a review of techniques, occurrence and effects. Mar. Environ. Res. 111, 5–17. doi: 10.1016/j.marenvres.2015.06.007
Van Cauwenberghe L., Vanreusel A., Mees J., Janssen C. R. (2013). Microplastic pollution in deep-sea sediments. Environ. Pollut. 182, 495–499. doi: 10.1016/j.envpol.2013.08.013
Vianello A., Boldrin B., Guerriero P., Mochino V., Rella R., Sturaro A., et al. (2013). Microplastic particles in sediments of lagoon of Venice, Italy: First observations on occurrence, spatial patterns and identification. Estuar. Coast. Shelf Sci. 130, 54–61. doi: 10.1016/j.ecss.2013.03.022
Wathsala R. H. G. R., Franzellitti S., Scaglione M., Fabbri E. (2018). Styrene impairs normal embryo development in the Mediterranean mussel (Mytilus galloprovincialis). Aquat. Toxicol. 201, 58–65. doi: 10.1016/j.aquatox.2018.05.026
Woodall L. C., Sanchez-Vidal A., Canals M., Paterson G. L. J., Coppock R., Sleight V., et al. (2014). The deep sea is a major sink for microplastic debris. R. Soc. 1, 140317. doi: 10.1098/rsos.140317
Zhang D., Liu X., Huang W., Li J., Wang C., Zhang D., et al. (2020). Microplastic pollution in deep-sea sediments and organisms of the Western pacific ocean. Environ. pollut. 259, 113948. doi: 10.1016/j.envpol.2020.113948
Keywords: microplastics, deep-sea sediments, FT-IR spectroscopy, scanning electron microscopy, marine pollution
Citation: Canensi S, Barucca G and Corinaldesi C (2022) Extraction efficiency of different microplastic polymers from deep-sea sediments and their quantitative relevance. Front. Mar. Sci. 9:975875. doi: 10.3389/fmars.2022.975875
Received: 22 June 2022; Accepted: 22 September 2022;
Published: 07 October 2022.
Edited by:
Stefano Aliani, National Research Council (CNR), ItalyReviewed by:
Claudia Lorenz, Aalborg University, DenmarkTania Martellini, University of Florence, Italy
Copyright © 2022 Canensi, Barucca and Corinaldesi. This is an open-access article distributed under the terms of the Creative Commons Attribution License (CC BY). The use, distribution or reproduction in other forums is permitted, provided the original author(s) and the copyright owner(s) are credited and that the original publication in this journal is cited, in accordance with accepted academic practice. No use, distribution or reproduction is permitted which does not comply with these terms.
*Correspondence: Cinzia Corinaldesi, Yy5jb3JpbmFsZGVzaUB1bml2cG0uaXQ=