- 1Laboratorio de Microbiología Ambiental, Centro de Investigaciones Biológicas del Noroeste, Acuicultura, La Paz, BS, Mexico
- 2Laboratorio de Genómica y Bioinformática, Centro de Investigaciones Biológicas del Noroeste, Acuicultura, La Paz, BS, Mexico
- 3Centro de Investigaciones Biológicas del Noroeste, Acuicultura, La Paz, BS, Mexico
- 4Departamento de Oceanografía Biológica, Centro de Investigación Científica y de Educación Superior de Ensenada, Ensenada, BC, Mexico
- 5Microbiome Informatics Team, European Molecular Biology Laboratory, European Bioinformatics Institute (EMBL-EBI), Hinxton, United Kingdom
The aquaculture industry faces many challenges regarding the intensification of shrimp rearing systems. One of these challenges is the release of excessive amounts of nitrogen and phosphorus into coastal areas, causing disruption in nutrient cycling and microbial equilibrium, which are important for coastal productivity. Biosecurity within the shrimp rearing systems can also be compromised by disruption to the nutrient fluxes, and as consequence the microbiome of the system. In certain conditions, these changes could lead to the blooming of potentially pathogenic bacteria. These changes in the external microbiome of the system and the constant fluctuations of nutrients can affect the intestinal microbiome of shrimp, which is involved in the growth and development of the host, affecting nutrient absorption, regulating metabolic processes, synthesising vitamins, modulating the immune response and preventing growth of pathogenic bacteria. It has been suggested that specific changes in the intestinal microbiome of Litopenaeus vannamei may be an avenue through which to overcome some of the problems that this industry faces, in terms of health, growth and waste. Recent research, however, has focussed mainly on changes in the intestinal microbiome. Researchers have overlooked the relevance of other aspects of the system, such as the microbiome from the benthic biofilms; zooplankton, plankton and bacterioplankton; and other sources of microorganisms that can directly affect the microbial status of the intestinal and epiphytic communities, especially in rearing systems that are based on intensification and microbial maturation processes, such as a biofloc system. It is therefore necessary to place holobiome studies into context, including the ‘holobiome of the aquaculture system’ (microbiomes that make up the culture system and their interactions) and not only the intestinal microbiome. Thus, we describe factors that affect the shrimp microbiome, the methodology of study, from sampling to bioinformatic workflows, and introduce the concept of the ‘holobiome of the aquaculture system’ and how this enables us to promote the intensification, biosafety and eco-efficiency of shrimp farming. The holobiome perspective implies a greater investment of resources and time for research, but it will accelerate the development of technology that will benefit the development and sustainability of the aquaculture industry.
1 Introduction
Shrimp has become one of the most economically important seafoods worldwide. The most important and in demand species in this industry is the white leg shrimp (L. vannamei). Its production in aquaculture systems has increased to be even more important in economic and volume terms than fishing production (FAO, 2020; García-López et al., 2020).
High demand for shrimp production and stagnation in fisheries has favoured the expansion of shrimp culture systems, and therefore its intensification has become necessary (Crab et al., 2007; Zhang et al., 2021b). This intensification process has pushed the industry to develop novel technologies such as recirculation systems (RAS) and biofloc (Dauda, 2020), with an excellent ecofriendly process, not only for higher productivity but also for sustainable development beside other benefits revised recently (Jamal et al., 2020). The development of this technology has faced other challenges as developing new pathogen-resistant genetic strains (Alday-Sanz et al., 2020), improving feed formulation (Kuhn et al., 2016; McLean et al., 2020), dietary supplements (Ringø and Song, 2016), and the use of microbial consortia to improve water quality (Kumar et al., 2017). All these innovations have improved the shrimp production, however, it is still necessary to deal with problems related to the environmental impact of intensified rearing systems, such as the deterioration of local water quality, biosecurity (such as antibiotic resistant bacteria), disease emergence, water acidification and eutrophication. All these problems result in unpredictable culture efficiency and mean that the industry is less sustainable (Henriksson et al., 2018; Suantika et al., 2018; Dauda, 2020; Davis et al., 2021), making it harder to assess investment, determine financial return and/or to include circular economy strategies. These challenges are all related to the system of microbial management (microbiome), which is not only important, but intrinsically linked to the sustainability and productivity of intense rearing systems.
The microbiome can be described as the bacteria, archaea, viruses and microbial eukaryotes that occupy a physical, chemical and biologically defined habitat, and involves their genomes and metabolic activity (Whipps et al., 1988; Pereira-Marques et al., 2019; Berg et al., 2020). In the context of biosecurity, two main issues have been explored within microbiome studies, the resistome (set of antibiotics resistant genes), and virulence factors (tools that pathogenic microorganisms use to avoid host immune defences and to improve survival) (Guevarra et al., 2021). From a wider perspective, an ecological approach allows us to understand the importance of the intestinal microbiome, as this is the link between the organism and the whole system.
The intestinal microbiome is composed of a great number and diversity of microorganisms. Some microbial groups are present in the gut in the long-term, and others are transient, but even the transient groups could play a significant ecological role in the system (especially during larval development). Host-microbiome interaction also has a direct impact on the development and physiology of the host via a mutualist interaction through metabolic collaborations, affecting nutrient absorption, regulating metabolic processes, synthesising vitamins, modulating the immune response and limiting the colonisation of pathogenic bacteria (Buford, 2017; Fetissov, 2017; Read and Holmes, 2017; García-López et al., 2020). This host-microbiome interaction cannot be seen as stationary, as it is highly dynamic, in part because the gut microbiome composition is continuously affected by both biotic and abiotic factors (Bentzon‐Tilia et al., 2016; Butt and Volkoff, 2019). Under intensive conditions, the level of nutrients increases and changes depending on the type of intensive system (RAS or biofloc), modifying the microbial communities present in the water, feed or in farming organisms, and increasing the biotic pressure in the system (Dittmann et al., 2017; Bass et al., 2019; Guo et al., 2020). These modifications to the microbial equilibrium could turn out to be positive in terms of sustainability, biosecurity or productivity, however the opposite may be true if the system is not resilient, or the load of nutrients surpasses the capacity of the microbial community (microbiome) to metabolise those nutrients (Schryver and Vadstein, 2014). As a consequence, understanding the microbiome, and in particular the host-associated microbiota, could lead to the better microbial management of the system, which could help to solve some of the problems in aquaculture (Dittmann et al., 2017; Landsman et al., 2019a), such as reducing the presence of specific pathogens (Cheng et al., 2020; Mazón-Suástegui et al., 2020), optimising growth (Fan et al., 2019a; Guo et al., 2020) and reducing the amount of feed and waste by optimising nutrient utilisation (Hamidoghli et al., 2020; Zhang et al., 2021a). Recent research on L. vannamei microbiome has been focused on the optimisation of the intestinal microbiome and its recognition as a vital organ responsible for functions such as digestion, nutrient absorption and immune response (Li et al., 2018).
In order to achieve the proper microbial management of the system and to use the microbiome ecological concept as a platform from which to improve shrimp production, it is necessary to study the aquaculture system as a whole, including all the elements integrated within it. This integrative vision and/or perspective of the system is based on the “holobiome” concept; under this concept it is necessary to consider that each element of the system not only directly affects target culture species, but also the micro/macro-habitats that function as the nuclei of the different microbiomes, which by themselves retain a level of interaction and influence between them. In other words, the holobiome includes all the genomic information within the system, and this concept recognises that the genomic information will be displayed depending on the micro/macro habitat landscapes available at a certain point in time, under specific environmental conditions, therefore, acknowledging that the system is highly dynamic rather than static, and that all the genomic information that will be expressed under certain conditions belongs to a specific systemic momentum. As a consequence, merely studying the intestinal microbiome (traditional research approach) inside any intensive system could overlook what is really happening in a highly dynamic system. We therefore want to bring a critical analysis to the gut microbiome approach, and explain how this vision may not enable a more holistic approximation of the system. Finally, we will try to discuss how the holobiome concept in the aquaculture industry could contribute to a better understanding of how intensified systems work, so that in the future we could better approximate the productivity, biosecurity and eco-efficiency of shrimp rearing systems.
2 The L.vannamei gut microbiome studies as an approach to understanding the complexity of rearing systems
Keeping a stable and functional microbiome in the digestive system is important for the host’s health and optimal growth. The host microbiome can be affected by intrinsic and extrinsic factors, which alter composition, diversity and function, and affect health, feeding, energy storage and adult size (Zhang et al., 2016; Butt and Volkoff, 2019). Extrinsic factors modulate the microbiomes of aquatic organisms with greater ease than in terrestrial organisms. This is due to the direct interactions of aquatic organisms with the surrounding environment, composed of water, food, and faeces (Schryver and Vadstein, 2014; Sun et al., 2019). At the same time, the environment microbiome is affected by changing physical-chemical conditions which in turn will modify the host microbiome as well as their interactions. We could therefore expect that any change in a closed-system, such as intensive aquaculture rearing systems, will affect the host microbiome, but how each variable will affect the host microbiome is hard to assess, and therefore this approach faces certain challenges.
In general, the study of the microbiome can be divided into four basic components: microbial composition (microbiota), determining genomic features, microbial metabolic activity and interactions (Dai et al., 2017; Butt and Volkoff, 2019; Huang et al., 2020b). Other approaches that also involve part of the microbiome have been added in newer studies, however, especially in aquaculture studies, such as the resistome (the whole set of antibiotic resistance genes) and genetic virulence factors (Figure 1). Specifically, L. vannamei microbiome studies have been focused almost exclusively on the description of changes in the bacterial composition of the digestive system through the use of amplicon sequencing. These types of studies have contributed to connecting certain environmental factors to changes in the microbial community in the gut of L. vannamei, including stress by sulphide (Suo et al., 2017; Duan et al., 2019a; Jiang et al., 2019b), different pH in the rearing water (Duan et al., 2019b; Yu et al., 2020), stress by ammonium and nitrite (Duan et al., 2018; Jiang et al., 2019b), water salinity (Zhang et al., 2016; Fan et al., 2019b), stress due to copper (Qian et al., 2020), water temperature (Jun-xia et al., 2004), feed composition and the use of supplements (Duan et al., 2017; He et al., 2017; Qiao et al., 2017; Vargas-Albores et al., 2017; Fan et al., 2019a; Cheng et al., 2020; Elizondo-González et al., 2020; Gainza and Romero, 2020), disease (Rungrassamee et al., 2016; Wang et al., 2019b; Zhou et al., 2019a; Dai et al., 2020; Liang et al., 2020), and the culture system type (Hostins et al., 2017; Deng et al., 2019; Landsman et al., 2019a; Omont et al., 2020); whereas the intrinsic factors involved include the genetic strain (Landsman et al., 2019b; Liu et al., 2019b), ontological development stage (Xiong et al., 2018b; Garibay-Valdez et al., 2020), and the physiological condition of the host (Dai et al., 2017; Xiong et al., 2017).
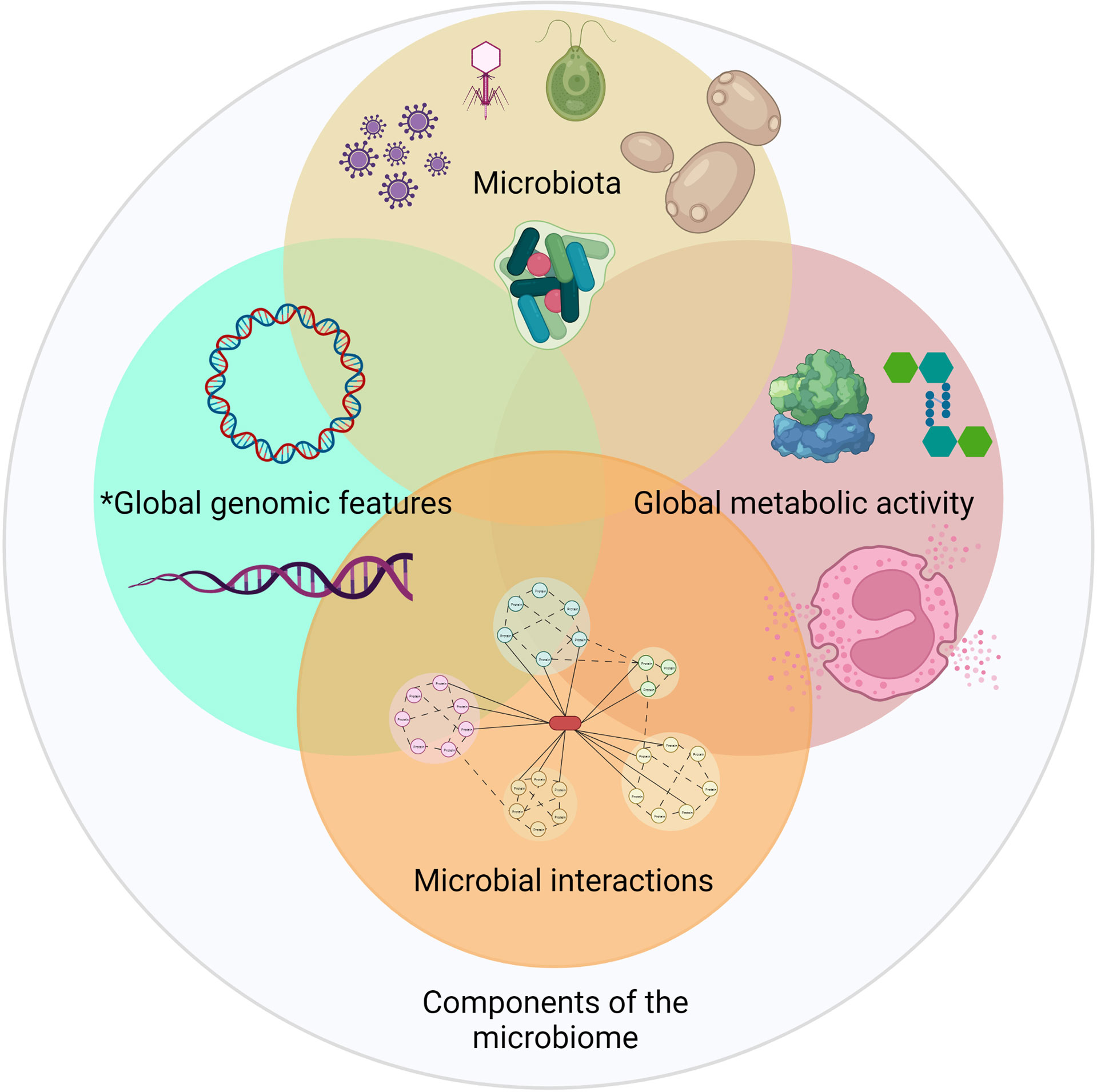
Figure 1 Perspectives for the study of a microbiome.*The resistome and virulence factors are focused studies within the genetic characteristics of the microbiota.
To date there has been little information on the study of micro eukaryotic communities in the gastrointestinal tract of L. vannamei. This group is diverse and important, since it is positively related to digestive enzyme activity, and positively affects shrimp growth (Dai et al., 2017). It has also been shown that they are closely related to host immune modulation pathways (Li et al., 2019), and their composition is shaped by host disease conditions (Xiong et al., 2018b; Dai et al., 2019).
The integration of multiple omic data such as metagenomics (He et al., 2020; Huang et al., 2020b), meta-transcriptomics (Duan et al., 2019a), metabolomics (Guo et al., 2020; Huang et al., 2020b) and metaproteomics is necessary to gain a full understanding of the shrimp microbiome and its implications in aquaculture optimisation. We will thus be able to elucidate the nature of microbial interaction networks between microbial species and the host in different conditions (Sergaki et al., 2018; Rise et al., 2019).
2.1 Technical challenges in the current state of analysis of L. vannamei microbiome
2.1.1 Sample collection, manipulation and storage
It is necessary to integrate new data with current knowledge to homogenise and highlight the available results on the L. vannamei microbiome, reducing biases. First, the term ‘microbiota’ refers to the complete group of prokaryotic and eukaryotic microorganisms in a specific niche (e.g., midgut) (Pereira-Marques et al., 2019; Berg et al., 2020), and this is important as most research is focused only on bacteria, and misuses the term ‘microbiota’. Other things that need to be considered in gut microbiome analyses are where the samples were taken from and what this represents. On the other hand, it must be considered that penaeid intestines change throughout the ontological development until the mature stage is reached, and comprise sections varying in morphology and function, (Lovett and Felder, 1989), so that each section has a distinctive selective pressure on the respective microbiota (Xiong et al., 2015; Li et al., 2019). The digestive system of adult penaeids such as L. vannamei involves three sections: the anterior intestine is subdivided into the oesophagus and anterior (cardiac) and posterior (pyloric) stomach chambers (the latter is connected with the hepatopancreas); the midgut starts in the union with the hepatopancreas, crossing most of the abdomen; and lastly, the hindgut includes the rectum and anus (Dall, 1967; Ceccaldi, 1989). A separate study of these structures can provide better information about the effect of a variable of interest. This could be impractical, however, especially for younger specimens. A potential solution is to identify where the sample is taken, and then always use that section for future studies. This is particularly helpful for the conjunction between the intestinal tract and the hepatopancreas, because the intestinal and hepatopancreas microbiomes have been described as differing in composition (Cornejo-Granados et al., 2017; Li et al., 2019; Wang et al., 2020b). Another aspect to consider is whether the gut has intestinal content or not, given that transitory non-adhered microbiota may affect the composition of the associated microbiota (Vargas-Albores et al., 2017; Wang et al., 2020a).
The pooling of samples is common in studies of the intestinal microbiota of L. vannamei, where two or more dissected intestine samples are combined, mainly to increase microbial nucleic acid extraction yield (Rungrassamee et al., 2016; Xiong et al., 2018b), to minimise biological variation among specimens (Xiong et al., 2015), and to increase the opportunity to observe differences across treatments (Schleder et al., 2020). Although this strategy may be useful and practical, it could reduce the accuracy of conclusions. It is necessary to understand that these groupings are not a substitute for biological representability, given that a single sample has low statistical power (Creer et al., 2016). Conversely, in other studies, the tissue is subsampled to conduct diverse analyses in the same sample (Chen et al., 2017), including the study of the microbiota.
True biological variation can be affected by technical variation, for example due to the sample collection technique or DNA extraction methods (Debelius et al., 2016). Obtaining an accurate profile of the microbiota therefore requires an initial evaluation of the variation caused by technical sources (Rosenthal et al., 2014). When tissue subsampling is performed, it must be considered that microbial populations have a functional adaptation to particular niches (Lloyd-Price et al., 2017), making it unlikely that bacterial communities are uniformly distributed within the volume from a faecal sample (Wesolowska-Andersen et al., 2014). On the other hand, the DNA extraction method used in studies of the shrimp microbiome is not usually justified or validated for marine organisms, and several of these techniques are reported in studies of the human intestinal microbiome but applied to stool samples (Yuan et al., 2012) and not tissue, as is the case in shrimp. These differences in sample types affect extraction yields, can produce bias, and affect reproducibility (Xue et al., 2018). In order to efficiently extract microbial DNA from the shrimp gut, its components must therefore be lysed: muscle, connective tissue, cylindrical epithelium (Dall, 1967), peritrophic membrane that includes proteins and chitin (Wang et al., 2012), and the structural composition of microbial cells. Sensitive information on the sample should be recorded (before, during and after sampling), as well as the experimental procedures, all this information conforming the sample metadata (covariates), which are used in combination with sequencing data to conduct statistical modelling and correlation analyses that allow the microbiome structure and function under particular settings to be explained (Goodrich et al., 2014; Jovel et al., 2016; Nayfach and Pollard, 2016). Moreover, the dynamism of aquaculture systems due to seasonal or environmental factors means that a proportion of the microbiome will remain in a changing state, which creates the need to conduct longitudinal instead of cross-sectional studies to obtain direct information about the causal covariates of microbiome dynamics (Quince et al., 2017).
Microbial DNA of high quality and integrity is required for high-throughput sequencing. The collection and preservation of samples is a critical step in this process (Bellali et al., 2019). Good practices include the cleansing of specimens (with sterile water and 70% alcohol) previous to collection to minimise contamination by the surface of the organism (Hou et al., 2018b; He et al., 2020); and continuing with standardised tissue dissection protocols, such as those described by Landsman et al. (2019b). In the case of RNA, preservation of the nucleic acids within the sample is recommended in liquid nitrogen or at -80°C in combination with a stabiliser such as RNA-later ® (Creer et al., 2016; García-López et al., 2020). The most common practice to preserve DNA is direct storage at (or less than) -20°C, as DNA is less labile than RNA or the use of absolute ethanol. When genetic material cannot be extracted in a three month window, lyophilisation previous to frozen storage has allowed the long term preservation of human faeces with later good DNA quality for studies based on amplicons (Kia et al., 2016). Finally, glycerol could be used in frozen samples as a cryoprotectant agent to avoid the loss of susceptible taxa such as Bacteroidetes, as has been observed in sheep rumen samples (McKain et al., 2013).
2.1.2 High-throughput sequencing in the assessment of microbial communities
Studying the taxonomic profile of microbial communities by molecular methods based on high-throughput sequencing requires the extraction of high-quality metagenomic DNA (Handelsman J., 2004). DNA can be extracted using standard molecular biology protocols (Huang et al., 2016a; Pilotto et al., 2018; Mazón-Suástegui et al., 2020), extraction kits (Chai et al., 2016; Dai et al., 2018; Shao et al., 2019) or a combination of both (Gainza et al., 2018; Gainza and Romero, 2020). The selection of DNA extraction method is important, as no method will be optimal in all types of samples. This has been true for processing meconium samples (Stinson et al., 2018), human faeces (McOrist et al., 2002; Claassen et al., 2013) and mouse faeces (Ferrand et al., 2014). The selection must be based on the subjacent composition of the cells present in the sample in order to achieve the efficient extraction of most of the present taxa. Works by Jiang et al. (2019a) and Xue et al. (2018) are the first approaches to consider screening for optimal extraction protocols in the study of bacterial biota in L. vannamei. These studies suggest using the QIamp fast DNA stool minikit (Qiagen), E.Z.N.A. ® Bacterial DNA kit (Omega Bio-tek) and E.Z.N.A. ® stool DNA kit (Omega Bio-tek). The two works only tested six methods not widely used in the literature. Optimisation should thus be considered during the incorporation of additional treatments with chitinase, given that chitin is a component present in shrimp samples. It may be worth testing the efficiency of the “repeated bead beating plus column” method (Yu and Morrison, 2004) or similar methods adapting the composition of the sample. It has been reported that adding a bead-beating step will improve the yield and representativity of the microbial profiles (Quince et al., 2017; Yang et al., 2020a). Differentiation between live and death microbial cells is another important challenge due to DNA persistence in the environment after a cell has lost its viability, which usually leads to the misestimation of the microbial composition (Nocker et al., 2007). Strategies to overcome this problem include the use of propidium monoazide (Nocker et al., 2006) prior to DNA/RNA extraction.
During the extraction of nucleic acids, it is common to obtain DNA from both the host and microbiome, due to the high abundance of the host DNA in the sample. This affects the yield of microbial DNA recovery during extraction, masking the microbial signal during sequencing and interfering with the interpretation of results (Lim et al., 2014; Heravi et al., 2020). This problem can be particularly important in the detection of low abundant taxa. According to Pereira-Marques et al. (2019), a proportion of host DNA as high as 60% in the initial extraction is enough to allow the detection of taxa with a relative abundance as low as 0.2% through shotgun sequencing. The host DNA issue may be solved by the enrichment of microbial DNA prior to implementing background (eukaryotic) depletion techniques (Quince et al., 2017). Methods include hypotonic lysis (Lim et al., 2014), NEBNext microbiome DNA enrichment kit (New England Biolabs Inc.), Molzym ultra-deep microbiome prep (Molzym), QIAamp DNA microbiome kit (Qiagen) and the HostZERO microbial DNA kit (Zymo Research) (Heravi et al., 2020). In the particular case of shrimp samples, an easy way to avoid extracting host DNA in high quantity is using shrimp faeces, which contains ~10% of host DNA (Pereira-Marques et al., 2019). The peritrophic membrane protects the faecal microbiome from contamination with the environment. Faeces can then be recovered using the methodology described by Córdova-Murueta et al. (2003).
Amplicon sequencing of hypervariable regions of the ribosomal operon has been widely used in the identification of microbial taxonomic groups in all sorts of environments, and the L. vannamei microbiome is no exception. This is because these regions are universally distributed in prokaryotic and eukaryotic organisms, and have hypervariable regions that allow taxonomic labelling, as well as conserved regions that allow the design of primers (Barb et al., 2016; Martínez‐Porchas and Vargas‐Albores, 2017). Genes that code for specific functions can also be used when the goal is to characterise microbial communities with a particular metabolic capacity. The preparation of the amplicon sequencing libraries and the sequencing should use a high-fidelity polymerase and a low number of amplification cycles (Goodrich et al., 2014).
The hypervariable regions V1-V9 of the 16S rRNA gene have been evaluated to identify bacterial taxa in various environments (Wang and Qian, 2009). Cornejo-Granados et al. (2018) compared non-overlapping amplicons from seven hypervariable regions (V2, V3, V4, V6-V7, V8, and V9) to study the bacterial biota of the hepatopancreas and intestine in cultures of L. vannamei, reporting that at the family level Vibrionaceae (35.6%), Enterobacteriaceae (20.1%), Pseudoalteromonadaceae (5.9%), Pseudomonadaceae (3.3%) and Rhodobacteraceae (1.3%) were the most abundant taxa in the intestine of healthy shrimp and Enterobacteriaceae (70.2%), Pseudomonadaceae (18.1%), Vibrionaceae (6.9%), Pseudoalteromonadaceae (0.6%) and Moraxellaceae (0.5%) were the most abundant in the hepatopancreas of healthy shrimp. The most commonly used amplicon is that covering the V3-V4 regions (Cheng et al., 2020; García-López et al., 2020; Holt et al., 2020; Liang et al., 2020; Zhang et al., 2021b), which has been reported to better recover the original diversity of the sample (García-López et al., 2020). It is important to mention that using the V3-V4 region of 16S rRNA gene is highly convenient, because it has low intragenomic heterogeneity in bacteria, reducing the overestimation of taxa with a higher gene copy number, and decreasing the error in diversity metrics (Sun et al., 2013). The length of the V3-V4 amplicon (~460 bp) is convenient for the Illumina MiSeq sequencing platform, and taxonomic annotation using the Ribosomal Database Project (Cheng et al., 2020; Elizondo-González et al., 2020), Greengenes (Huang et al., 2020b; Yu et al., 2020) or Silva (Wang et al., 2020a; Wang et al., 2020b) databases are the most commonly used in the study of the bacterial microbiota of L. vannamei.
The eukaryotic biota in L. vannamei intestines has been identified with the V2-V3 and V4 regions of the 18S rDNA gene, using the Illumina MiSeq platform and SILVA database (Dai et al., 2017; Xiong et al., 2018b; Dai et al., 2019). With these settings the microeukaryotes were characterised (Santos et al., 2010) and a fraction of the mycobiota using the region ITS1 of rDNA with the platform Illumina HiSeq and the UNITE database to identify only the mycobiota in L. vannamei, reporting that the phyla Ascomycota and Basidiomycota are the most abundant in intestine and hepatopancreas, and that there is a greater abundance of the genera Didymella and Filobasidium in the gut, and Pyrenochaetopsis in the hepatopancreas in healthy shrimp (Li et al., 2019).
Different programs and specialised algorithms are needed to obtain biologically meaningful information from amplicon sequencing data (Goodrich et al., 2014). Together these are the “bioinformatic workflow”. Currently, most bioinformatic workflows include a step grouping sequences into operational taxonomic units (OTUs) or amplicon sequence variants (ASVs) (Schloss, 2021). The latter strategy has better differentiation of biological sequences, is reproducible, represents the biological variation in the sample, provides better taxonomic resolution, decreases diversity bias and has a higher predictive ability for health biomarkers compared to OTUs (Callahan et al., 2017; Prodan et al., 2020). However, the ASV approach has limitations, such as in the comparison of independent data from sequencing different loci, the separation of one genome into several ASVs due to intragenomic variation of multiple copies of the targeted locus (commonly observed in the 16S rRNA gene) and its intragenomic heterogeneity, as well as the formation of ASVs containing sequences from multiple species, which is more common in the OTU approach (Sun et al., 2013; Callahan et al., 2017; Schloss, 2021). Most of the bioinformatic workflows used in studies of L. vannamei microbiota are based on OTU analysis, which makes comparison between studies difficult. The availability and constant improvement of programs and algorithms results in the heterogeneity of their implementations, with the possibility of consequently introducing limitations and biases. The DADA2 program is recommended for studies requiring the finest taxonomic resolution (e.g., to differentiate closely related strains) and the USEARCH-UNOISE3 program because it provides better overall performance combining high sensitivity and specificity (Prodan et al., 2020).
Although amplicon sequencing is the most commonly used strategy for studying microbiota composition, it has certain limitations and biases in the reconstruction of the community structure. Some caveats that have to be kept in mind include: differential affinity of primers to conserved regions, the resolution of each variable region among taxa and even exclusion of certain members of the microbial community, amplification artefacts such as the formation of chimeric sequences, and overestimated abundance of certain taxa by multicopy marker genes (Carlos et al., 2012; Thomas et al., 2012; Koslicki et al., 2014). These limitations can be addressed by the use of a whole metagenome shotgun approach, which allows the genetic content of archaea, bacteria, protists, virus, phages and eukaryotes to be simultaneously obtained. A good representation of the community structure and the metabolic potential of the most abundant organisms could be obtained if the sample is sequenced with enough coverage (Jovel et al., 2016; Ortiz‐Estrada et al., 2019). Implementing this technique to study the microbiome of L. vannamei has shown that the genera Shewanella, Chitinibacter, Rhodobacter, Gemmobacter, Bacillus and Roseburia are more abundant in the intestines of healthy shrimp than those with WFS; that metabolic pathways such as mineral absorption, aminobenzoate degradation, lipoic acid metabolism and phenylpropanoid biosynthesis significantly decrease in shrimp with WFS; and when complemented by metabolome studies, that there are 35 compounds which are significantly different between the two conditions described (Huang et al., 2020b). However, this approach also has some drawbacks, such as the higher cost, the analysis of higher data volume, hardware requirements for data processing and problems optimising the genomic assembly of low abundant taxa (Carlos et al., 2012; Ortiz‐Estrada et al., 2019). The taxonomic assignment and the annotation of genes is limited by the information contained in the databases, so it is also necessary to continue the characterisation and assignment of functions to poorly described and neglected microorganisms (Sergaki et al., 2018; Rise et al., 2019).
While bioinformatic workflows used for amplicon sequencing data commonly involve quality control, read merging (when paired-end strategy is used), OTU or ASV formation, identification and removal of chimeric sequences, taxonomic assignment and diversity analysis (Goodrich et al., 2014), the bioinformatic workflow for analysing metagenomic data involves steps for the quality control of reads and host sequence removal, assembly (optional), taxonomic and functional annotation and statistical analysis (Nayfach and Pollard, 2016; Martínez‐Porchas and Vargas‐Albores, 2017). Currently only He et al. (2020) and Huang et al. (2020b) have analysed metagenomic data to study the intestinal microbiome of L. vannamei. We recommend Thomas et al. (2012) and Quince et al. (2017) for more details about the processing of metagenomic data, considerations, software and algorithms. Bioinformatic tools such as PICRUSt (Douglas et al., 2018), Tax4Fun (Aßhauer et al., 2015) and Vikodak (Nagpal et al., 2016) have been developed to generate information on the metabolic potential of microbial communities from amplicon profiles, however, this functionality is assigned by inference and does not accurately represent the function inherent in the sample, as limitations inherent in taxonomic labelling affect functional inference. Implementing these tools to study the bacterial biota of L. vannamei revealed that although the intestinal microbiota of shrimp and sediment have a similar profile in the dominant bacterial genera, they show significant differences in functional pathways (Huang et al., 2018). Another study concluded that the abundances of genes involved in metabolism were significantly lower in shrimps with white faeces syndrome (WFS) than in the healthy shrimp (Hou et al., 2018a) and results by Xiong et al. (2018a) showed that the predicted functional composition of gut microbiota changes over the shrimp developmental stages.
3 The holobiome as a necessary concept for studying the aquaculture system
We define the “holobiome of the aquaculture system” as all the microbiomes interacting in a culture system: the planktonic microbiome, sediment microbiome, feed microbiome and the host microbiome. This network of microbiomes and their interactions affects the eco-efficiency and production of the aquacultured species which are affected by the system’s endogenous and exogenous factors (Figure 2). As the result of this definition, the first step in studying the holobiome of an aquaculture system is to develop an integrative experimental design that considers the recovery of the most representative microbiomes of every one of the components of the system, as well as relevant information (metadata) associated with the environmental conditions when those components were collected. The subsequent steps include to build a sampling program to collect the necessary samples, undertake the corresponding analyses for the study of microbiomes, obtain the corresponding data, analyse it, and finally establish the meaning of the interactions of the microbiomes within the system.
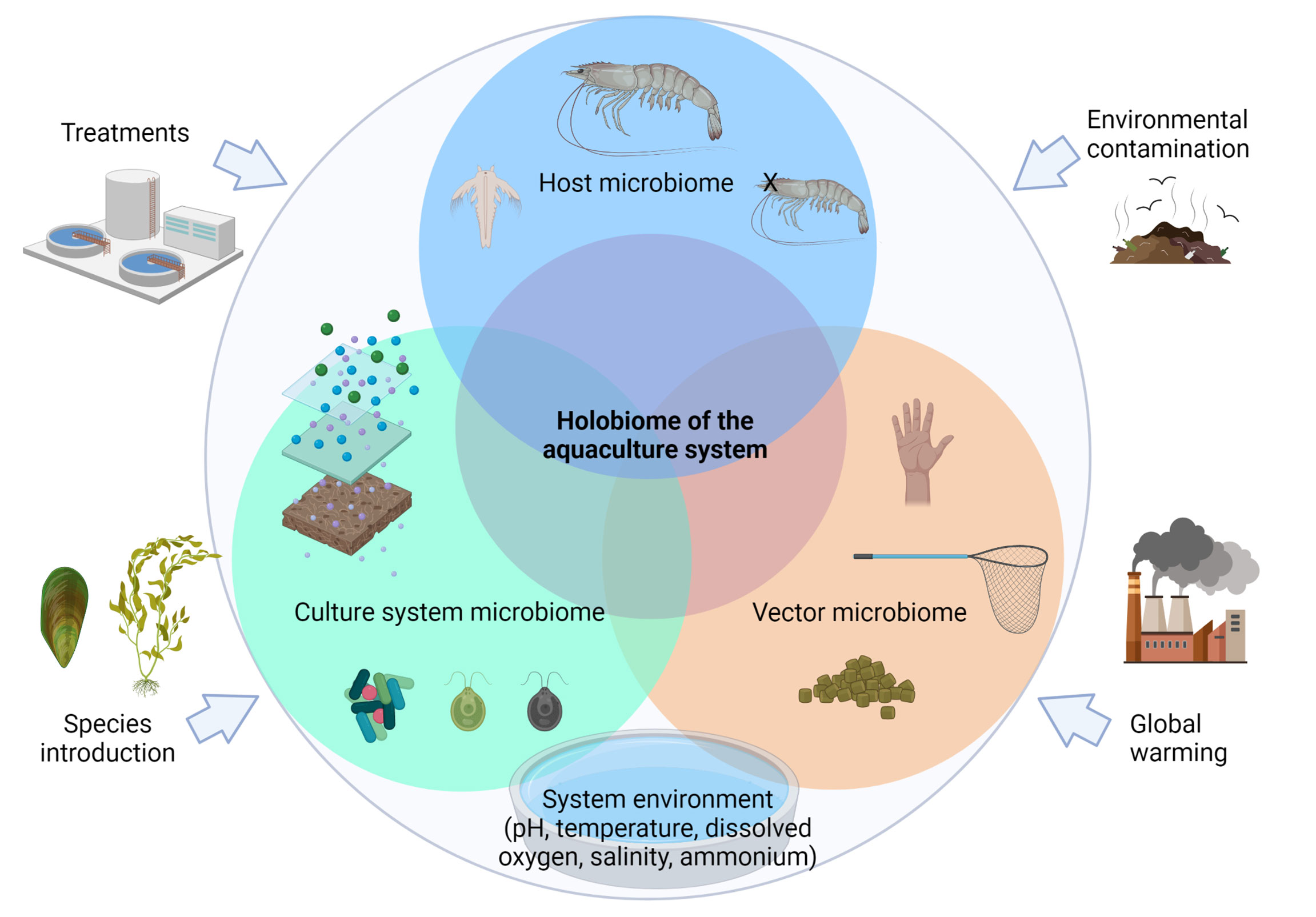
Figure 2 Components of the “holobiome of the aquaculture system”. “Culture system microbiome” involves the planktonic microbiome, sediment microbiome and biofloc microbiome; in “Host microbiome” can be included the skin, hepatopáncreas and midgut microbiome among others; “Vectors microbiome” involve those components that can actively introduce exotic microbiomes; “Holobiome of the aquaculture system” are intended to identify beneficial microorganisms, commensals, symbionts, pathogens and potential pathogens.
This approach will identify the components of the system whose microbiomes have a closer interaction and effect on the intestinal microbiome of the cultured organism, and the associated covariates. With this information, strategies can be developed to optimise cultivation systems through the management of microbiomes.
3.1 How the holobiome of the aquaculture system concept is related to intensification
The economical and productive performance of shrimp farming systems depends mostly on the system type and the production strategies. Aquaculture systems can be classified depending on the organism density, from low to high as: extensive, semi-intensive, intensive and hyperintensive (Pillay, 1997). Fishery stagnancy and human population growth (Crab et al., 2007) has led to the need for efficient resource utilisation, leading to a tendency for aquaculture production intensification (Pomeroy et al., 2014). In the L. vannamei case, its fast growth, good survival in high density seeding, and high tolerance to diseases make it a good candidate for intensive systems (Liu et al., 2017). However, its production has been limited to semi-intensive systems due to the increased risk of viral and/or bacterial infections which has damaged the industry in certain locations of the world (Araneda et al., 2008; Dauda, 2020; Zhang et al., 2021b) with great implications for the economic development of coastal environments.
How can the concept of “the holobiome of the aquaculture system” affect and improve the intensification process of L. vannamei farming? First, we have to recognise that the aquaculture system is per se an ecosystem that interacts intrinsically with the environment, and the microbial biota. By ruling out a group (microalgae and/or yeast or another) we can therefore easily get lost in trying to measure the effect of different intensification variables, such as the density of organisms, the use of probiotics, prebiotics, symbiotics, and functional feeds, on global shrimp productivity and its economy. It is thus necessary from this holistic perspective of the system to first understand how the system works as a whole, and not just the parts. To do so, we need to consider more practical aspects of the holobiome concept, such as how to determine diet supplements, how the holobiome is affected by different nutrients that enter the system via the feeds, and how to formulate feeds that will improve the performance of the holobiome in favour of system productivity. Different studies have proven that diets supplemented with bacteria, such as Bacillus PC465 (Chai et al., 2016) or Streptomyces sp. RL8 (Mazón‐Suástegui et al., 2020), or supplemented with prebiotics, such as Sargassum filipendula and Undaria pinnatifida (Schleder et al., 2020) or different organic acids (He et al., 2017) produce changes in the gut microbiome of shrimp that reduces mortality by white spot syndrome virus and/or infection by V. parahaemolyticus. Other intensification practices such as the use of natural microbial consortia and the use of biofloc culture systems (Guo et al., 2020) have shown similar results, in contrast to expensive microbial approaches. As an example, Zhang et al. (2021a) studied the effect of fermented feed, using different amounts of a microbial consortia. They obtained higher growth parameters, smaller feed conversion ratios and significant differences in the structure of shrimp’s intestinal bacterial biota. It is therefore necessary to understand that affecting the gut microbiome of shrimp relies not only on specific supplements and/or strains, but should be considered as a whole complex system.
One of the main factors of intensive systems is stock density (number of shrimps per m2). The density of shrimp also plays a relevant role because it directly affects not only the microbial load of the system as total biomass, but also its diversity. From this perspective each shrimp counts as a potential niche for the microbial biota that forms part of the holobiome. At a low density of farming ~25-200 shrimp•m-², the composition of microbial communities in the water poorly affects intestinal communities and their metabolic profiles (Xiong et al., 2015; Cardona et al., 2016; Huang et al., 2016a; Chen et al., 2017; Huang et al., 2018). By using biofloc technology (by changing the C/N ratio) when high seeding densities are used, it opens an opportunity to shape the intestinal microbiome structure towards a highly diverse community, reducing the niches that are available for pathogenic bacteria. Under biofloc conditions, Hou et al. (2018b) reported that the intestinal bacterial biota shares the same composition with that in the water column and in the sediment, but with different taxa proportions. The value of this work lies in the recognition that other microbial components of the system (those in water and sediment) are related to the shrimp gut microbiome, closing the gap between the inner biota and that found in the environment. Similarly, Deng et al. (2019) reported the effect of the culture density (400 m-2, 600 m-2, and 800 m-2) in biofloc systems on the microbial community, and observed that by increasing stock density it is possible to affect the biofloc microbial community, which could affect its functionality, and that in return the microbial composition in the bioflocs could significantly modify the gut microbiome of shrimp. We can only conclude that water, bioflocs, feed, sediment and shrimp microbiota are more closely interlinked in intensified systems, not only by specific taxa, but by diversity and richness. In support of that, Huang et al. (2020a) reported that different sizes of biofloc (related to age) affected the microbiota of shrimp differently. They found that the larger bioflocs (> 20 μm), which are older, were more similar to the intestinal bacterial biota of the shrimp. Those bioflocs are easier to grab by the shrimp, and they can therefore feed on them, compared with the small ones, and as a consequence, those bioflocs were more similar to the gut microbiota of shrimp. A good example of how different parts of the system will have an effect on the holobiome, is the fact that the microbiota of bioflocs is affected not only by the stock density and the type of biofloc system, but also by the C/N ratio. These two variables depend strongly on the biofloc microbial composition, which will change the holobiome.
Guo et al. (2020) studied the effect of different C/N ratios over the shrimp’s intestinal bacterial biota, reporting that C/N ratios of 15:1 changed the microbial structure of gut shrimp at the same time as the biofloc volume was also increased due to the carbon stimulation of microbial biomass. Those changes produced a significant increase in the relative abundance of beneficial bacteria, shrimp size and biomass. These results indicate that a high C:N ratio drives the hyperintensive systems (high stock density) into a more diverse ecosystem with higher microbial biomass that directly affects the gut microbiome, especially because shrimp is a well-known species that actively grazes biofloc particles (Ekasari et al., 2014a), further research is needed to understand if different C sources would have the same effect. BFT (biofloc technology) systems seem to be better intensified systems for shrimp, and are based on controlling the microbial biomass through the C:N ratio. This ratio can be modified depending on the C source. Tinh and colleagues (2021) demonstrated that by using cornstarch as a carbon source it was possible to significantly increase certain factors, such as survival, feed conversion ratio and weight gain. Other factors that can also modify the microbiome structure of the system under BFT are the use of microbial aggregation booster/inhibitor molecules (Fatimah et al., 2019), the addition of microbial consortia to culture systems (Hostins et al., 2017; Huerta-Rábago et al., 2019), different carbon sources and C/N ratios for biofloc development (Liu et al., 2019a; Wei et al., 2020), and different biofloc nucleators (Vargas-Albores et al., 2019). Understanding how all these variables affect the microbial composition from a systemic perspective is necessary not only because it is possible to increase productivity goals under intensive systems, but also because by using the concept of the holobiome on those studies, it could be possible to more accurately assess the relevance of adding different types of C, microbes and microbial boosters, in order to make better decisions regarding productivity, biosecurity and eco-efficiency, which are the three pillars of a sustainable aquaculture.
Another system that allows an intensification process is the Recirculating Aquaculture System (RAS). This type of technology relies on using microbial biofilters to remove the toxic nitrogen species in a rearing system. Although these systems are more expensive and less commonly used by the main aquaculture industry, they have been used to produce indoor cultivated shrimp in colder zones of Europe. This type of system is an alternative that involves the use of recirculating systems and a bioreactor to process nitrogen (Kuhn et al., 2010). Under RAS conditions, the biofloc production can be achieved in bioreactors in order to use them as feeds that will promote a healthy intestinal microbiome (Kuhn et al., 2016). In another approach Mangott et al. (2020) observed that the addition of Ulva lactuca macroalgae as feed for shrimp and as a bioremediation agent inside a recirculating aquaculture system (RAS) promoted the establishment of potentially beneficial bacteria in the water and in the shrimp hepatopancreas, which is reflected in the productivity variables (growth) as well as in the counts of potential pathogens.
As mentioned before, diverse factors generate changes in the shrimp’s intestinal microbiome and the information available does not allow to develop strategies for achieving a target microbial structure to be established (Bass et al., 2019). For instance, there are differences in the bacterial biota between retarded growth shrimp, normal/standard growth and overgrowth (Xiong et al., 2017), suggesting that shrimp’s microbiome composition could be manipulated to impact on the productivity, and the biome could actually be classified using this criteria as deficient, standard or optimal (Figure 3). Studying the system from the perspective of the holobiome would reveal the conditions and microbial networks within the culture system tos develop strategies for the active manipulation of the intestinal microbiome that favours the productive systems. Building a public database of different holobiomes under hyperintensive conditions, rearing systems, stock density, environmental features, C/N management, and feed additives/feed formulation would be a good start to understand the patterns associated with a better intensification process, eco-eficiency and biosecurity.
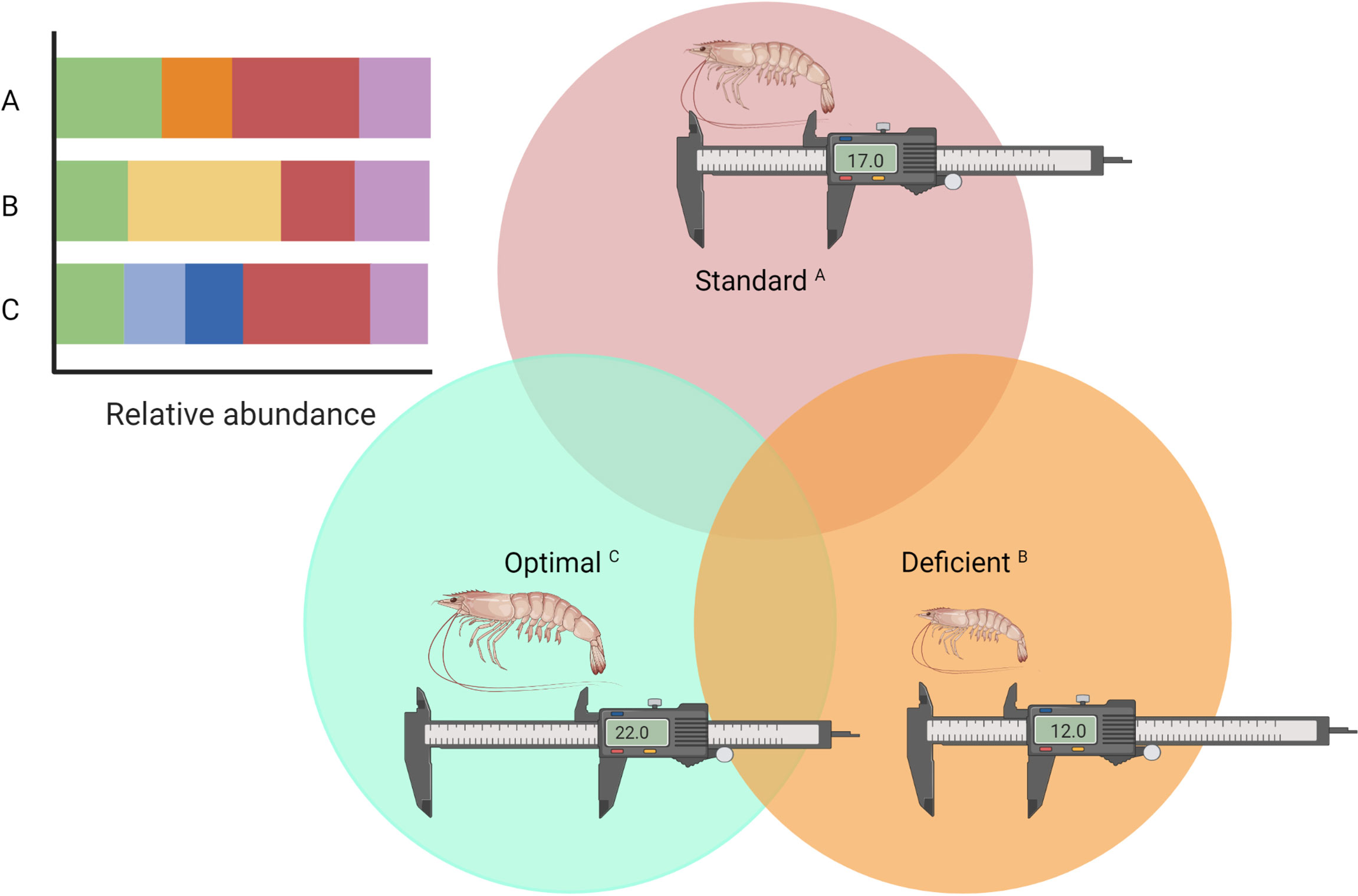
Figure 3 Classification of the intestinal microbiome of L. vannamei according to its benefits in aquaculture production. Standard: microbiome in the natural environment (undisturbed); Deficient: microbiome presenting with retarded growth, low survival rates, or pathology; Optimal: microbiome that provides benefits over the standard to the host, favoring productivity, biosecurity and/or eco-efficiency of the aquaculture system. Relative abundance can refer to phylotypes, transcripts or metabolic pathways. The letters A–C are used to relate and illustrate that each classification has different relative abundances.
3.2 The holobiome of an aquaculture system concept is necessary to achieve biosecurity in rearing systems
Biosecurity in aquaculture consists of practices that minimise the risk of introducing an infectious disease and spreading it to the animals at a facility, and the risk that diseased animals or infectious agents will leave a facility and spread to other sites and to other susceptible species. These practices also reduce stress to the animals, thus making them less susceptible to disease (Yanong and Erlacher-Reid, 2012). A disease can be defined as any harmful deviation from the normal functional state of an organism (Scarpelli and Burrows, 2020). Diseases in aquaculture systems generally develop quickly, and are detectable only when critical points have been reached, generally by an increase in the mortality rate and/or by visual symptoms. This is because the water system is an excellent carrier of pathogenic bacteria and viruses. The outcome of disease outbreaks will depend on specific factors such as species (immunological state, development stage, susceptibility), environmental factors (physical-chemical and microbiological status of the water), features of the potential pathogens (biology, life cycle, approved options for treatment), husbandry practices, and the correct implementation of standard operating procedures and biosecurity protocols by workers (Scarfe at al., 2008; Yanong and Erlacher-Reid, 2012; Hernández‐Cabanyero and Amaro, 2020).
The main strategies used to solve problems with potential pathogens in shrimp farming involve the use of high-health larvae, organisms with low pathogen content, genetic enhancement for disease resistance, biosecurity protocols and reduced water replacement ratios given that water is one of the most important factors in introducing pathogens (Pruder, 2004; Moss et al., 2012; Alday‐Sanz et al., 2020). Great efforts have thus been made to improve the gut health of shrimp, either by the addition of microbial consortia (Kumar et al., 2017), synbiotics (Ringø and Song, 2016) or the use of biofloc systems (Ekasari et al., 2014b; Dauda, 2020). Another approach to increasing biosecurity in rearing systems is the identification and/or the characterisation of potential pathogens. In general, in order to identify the cause of a disease, it is usually necessary to isolate a single pathogen from host tissues in which signs of disease are present, however, this approach is time consuming and fails to provide a real understanding of the niche of the pathogen inside the system and their ecological interaction with the rest of the holobiome: this approach could generate bias not only in the identification of a causative agent but also in the information provided to understand the disease development in a multimicrobial universe (Dai et al., 2018). New research has demonstrated that some diseases result from interactions of multi-specific/strain pathogens (Peters et al., 2012; Dai et al., 2018; Yao et al., 2018; Huang et al., 2020b). In order to better integrate the information that we can obtain from a rearing system, it is therefore necessary to change the way we study diseases. By using the concept of the holobiome we can consider the whole microbial equilibrium as one, and we cannot isolate one microbiome from another, because all those microbes are connected in one big closed or semi-closed system. This situation is even more important in BFT systems (hyperintensive), which are closed systems that could be seen as bioreactors. As a consequence, if a disease appears we would expect that the pathogen, though interacting with a host, also plays an ecological role and has an effect on the rest of the microbial community (holobiome).
Moreover, due to a pathogen-specific approach, most biosecurity strategies are focused on pathogen elimination from the culture, for example, by using antibiotics, which are often insufficient (Bass et al., 2019), and/or may to eradicate entire bacterial genera that are common members of the microbiome in the absence of disease, such as the Vibrio genus, without the understanding the ecological and functional roles they play in the host. One of the most important points exemplifying the need for a shift in the model of one host-one disease involves syndromic conditions such as WFS in L. vannamei. This disease cannot be explained by one single pathogen, and studies have shown that during this disease, key taxa of the gut are modified and/or altered, as are the networks of some of the nodes (taxa), promoting a complex interaction in the gut microbiome that makes it more difficult to treat this disease (Dai et al., 2020). Studies of well-known pathogens could be re-evaluated under the lens of the holobiome concept, and by doing so, we could achieve a better understanding of how diseases from bacterial and/or viral origins operate, so that in the future biosecurity strategies could be more assertive (Vayssier-Taussat et al., 2014). Mazón-Suástegui et al. (2020) reported that by changing the gut microbiome of L. vannamei via new microbial formulations in the feed, the infection of V. parahaemolyticus was reduced. On the other hand, understanding the pathological microbiomes involves adaptation to the microbiome level of the Koch and Hill postulates, as proposed by Huang et al. (2020b) to validate the causality of the WFS. Genomic and metagenomic analyses of the intestinal microbiome have shown that several pathogens live and interact with other microorganisms as bacteria, protists, fungi, viruses or phages, all generating and participating in complex interactions (Berg et al., 2020). Studies on the healthy or diseased states of the holobiome must focus on the nature of the biotic and abiotic interactions in the whole system in an ecological, chemical, physical, genetic and immunological context, and they also have to consider that these states are dynamic (Bass et al., 2019). Longitudinal studies will be necessary for the establishment of biosecurity protocols that avoid a certain disease outbreak, as exemplified by Chen et al. (2017) and Rungrassamee et al. (2016) in V. parahaemolyticus and V. harveyi infections respectively.
Given that disease progression and dispersion in aquaculture systems is fast, and that it is necessary to be proactive instead of reactive, new studies are needed to understand what happens from a holobiome perspective before a disease reaches its peak, and how microbial diversity is related to this. In general, studies show that a less diverse microbiome is related to a higher risk of diseases (Berg et al., 2020), however, Liang et al. (2020) and Zhou et al. (2019a) for blue body syndrome and “cotton shrimp-like” disease respectively in L. vannamei, report no difference in intestinal bacterial diversity between sick and healthy organisms, contrary to the greater diversity in sick L. vannamei animals with acute hepatopancreatic necrosis disease reported by Cornejo-Granados et al. (2017). The former studies reinforce the need to study more than one microbiome and the disease conditions given that the holobiome will provide more complete information about the conditions present in this state, highlighting the fact that research must not only focus on the bacterial component.
Antibiotics in aquaculture systems have been used to control infectious diseases caused by bacteria, but their misuse, such as the use of non-authorised antibiotics or their use in excess, has created antibiotic resistant bacteria (ARBs) (Crab et al., 2012) and is a human health risk, as the resistance can be transmitted to human pathogens, making it a public health problem (Zaheer et al., 2018). Bacteria may acquire resistance through a variety of mechanisms such as the modification of extant genes, horizontal gene transfer and the presence of low-levels of antibiotics in the environment that promote the transfer of mobile genetic elements (integrons, insertion sequences and plasmids) (Martínez‐Porchas and Vargas‐Albores, 2017; Zhou et al., 2019b) that might contain antibiotic resistant genes (ARGs). ARGs have been identified in sediment, water from lakes, basins and rivers, livestock manure (used in aquaculture as fertiliser), wastewater treatment plants, drinking water and aquaculture operations (Zhao et al., 2018). Given that aquaculture takes water from rivers, estuaries or coastal areas, it is sensitive to external contamination with ARGs and antibiotics released to the environment by anthropogenic activities (Pomeroy et al., 2014; Su et al., 2018). We also need to consider the presence of insecticides such as imidacloprid, which is used in agriculture, given that it also affects crustaceans by reducing their growth, health status and favours a pathogenic microbiome in shrimp intestines (Fu et al., 2022). Moreover, the tendency to intensify aquaculture systems means that a high bacterial density is promoted due to nutrient availability, together with the antibiotics, providing adequate conditions to facilitate horizontal gene transfer between bacteria (Liu et al., 2019d). Studies in this area have shown that diverse ARGs remain in commercial shrimp available at supermarkets (Liu et al., 2019c), and have been detected in water, sediment and shrimp intestines in aquaculture systems (Zhao et al., 2018), and ARG-bearing human-pathogenic bacteria have been detected in shrimp farming operations (water, sediment and intestines) where antibiotics were not used (Zhou et al., 2019b). These points highlight the importance of studying the resistome in the microbiome of the aquaculture systems, from effluent water and in the intestines of organisms for good decision making about antibiotic treatments, and searching for better strategies for the microbiological management of cultures. On the other hand, bacteria may become pathogenic by acquiring and using DNA from other bacteria through plasmids, genomic islands, transposons, insertion sequence elements and bacteriophages (Bass et al., 2019). These acquisitions may involve virulence factors, which are the tools that pathogenic microorganisms use to avoid host defences and improve their survival. These include pathogenicity islands, ARGs, toxins, and adhesins among others (Ellul et al., 2021). A virulence factor approach is therefore key to understanding pathologies, and can be useful in disease risk assessment, as well as to elaborate regulation strategies in farming operations and to identify new drug-targets (Wu et al., 2008; Zhang and Li, 2021). To understand the ecological dynamics of the virulence factors in aquaculture systems it is necessary to study them in the holobiome of the aquaculture system, and they should be evaluated in all L. vannamei genetic strains given that, as mentioned before, they involve differences in their microbiome.
3.3 The holobiome of the aquaculture system and eco-efficiency
The goal of efficient aquaculture systems is to maximise benefits and services, and to provide appropriate economical coastal development, and therefore, the industry has made big efforts to reduce waste such as wastewater, feed, soil, and energy in order to attain sustainability. Feeds are a key factor because they are the main component that introduces a source of nitrogen, phosphorus and other microelements inside the system. From this perspective it is important to connect the use of feeds and their effect on eco-efficiency of the system, and even more important to consider how these affect the holobiome. The holobiome works as a super metabolism that goes beyond the target culture species, and therefore the use of adequate feeds could not only provide nutrition to shrimp but could also affect certain groups that will improve eco-efficiency, which is crucial. One example of this is the C:N ratio; by changing it either in the water or in feeds we will affect the microbial groups represented in a system (either RAS or BFT), as the organic matter breaks down inside the system forming new nutritional niches and allowing different groups to build a complex ecological network between them, and as consequence, the nutrient fluxes will follow these changes. Formulated feeds can increase the presence of certain microbial groups, such as nitrifiers, denitrifiers, ammonia oxidisers, phosphorus solubilising/immobilising bacteria and microalgae, along with other relevant groups that will use any residual to avoid bottlenecks of nutrient recycling that can cause the generation of new niches for the establishment of potential pathogenic bacteria.
In order to understand the importance of the holobiome of the aquaculture system in the eco-efficiency we will use the feed used in L. vannamei farming as a good example. Intensification is characterised by high population densities in the cultures and higher feed rations, which can represent up to 60% of production costs (Hu et al., 2019). This creates challenges, such as higher nutrient loads in the system and discharges to the environment (da Silva et al., 2013). Approximately 36% of the feed is disposed of as organic waste, and nearly 75% of N and P is not utilised by the organisms (Crab et al., 2007; Martínez‐Córdova et al., 2015). The most common protein source in aquaculture feed is fishmeal, as it is highly digestible and contains essential amino acids and necessary nutrients to fulfil the dietetic requirements of the cultures (Shiu et al., 2015). More economical and sustainable approaches, however, mean that the industry has been searching for alternative substitutes, such as plant-based proteins, such as soya. However, their use under certain conditions results in a decrease of digestibility and the lower growth of organisms, because of the deficiency in essential amino acids, high fibre content and anti-nutrients (tripsin inhibitors, phytic acid, tannins, saponins and lectins) that also affects the palatability of the food (Hua et al., 2019; Hu et al., 2019; McLean et al., 2020). The use of microbial fermentation has been proposed to solve this issue, as it improves the nutritional quality of the plant-based proteins, their digestibility and the profile of essential amino acids, and the anti-nutrients decrease (Jannathulla et al., 2017; Jannathulla et al., 2018) which contributes to better use. Fermentation conditions and the different types of microorganisms used for this purpose affect the nutritional quality and digestibility of foods (Kang et al., 2010; Hamidoghli et al., 2020), which can reduce inputs and waste (Jannathulla et al., 2018). This feed fermentation process incorporates two important components that will affect “the holobiome of the aquaculture system”, the first is a microbial load and the second is the by-products derived from fermentation.
These two components can produce changes in the intestinal microbiome of organisms, in the same way as probiotics (Mazón‐Suástegui et al., 2020), additives (He et al., 2017) or changes in the composition of diets (Qiao et al., 2017). Such disturbances in the microbiomes of the culture system are induced by niches generation via metabolic by-products or antimicrobial compounds accumulation (Stanton et al., 2005), affecting the recycling of system nutrients. The benefits of the fermented feed in shrimp farming are: (i) economical-environmental, (ii) nutritional, (iii) production, and (iv) health. Fermented foods allow the full replacement of fishmeal in the feed (McLean et al., 2020) and the use of organic waste (Kang et al., 2010; McLean et al., 2020) to obtain microbially-based protein that is nutritionally better than unfermented plant-based protein sources (Shiu et al., 2015; Jannathulla et al., 2017). Studies have shown that the bio-processes of the feeds allow the maintenance and improvement of the weight, growth, FCA and survival rates, compared to feed with fishmeal (Jannathulla et al., 2018; Hamidoghli et al., 2020); nut also improves the nutritional status of the organisms, improving the intestinal micro ecological environment, increases the activity of antioxidant and digestive enzymes (Liñan-Vidriales et al., 2021; Zhang et al., 2021a) and also improves survival after infection with pathogens such as V. parahaemolyticus (Cheng et al., 2017; Hamidoghli et al., 2020).
Animal-based proteins can also be fermented to improve their properties and benefits to cultures (Dawood and Koshio, 2020). In addition to the benefits due to the fermentation of the protein sources, this process also has an effect on the microbial composition in L. vannamei gut (Cheng et al., 2020; Zhang et al., 2021a), and therefore also represents a strategy based on modulating the function of the holobiome of the aquaculture system. Despite the input of the fermented feed to eco-efficiency it does not solve the nitrogenated waste accumulation issue, such as ammonia and nitrite, which affects the water quality and is toxic for cultured organisms (Duan et al., 2018; Jiang et al., 2019b). To solve this problem, Hamidoghli et al. (2020) evaluated the implementation of diets with fermented-based proteins and also the addition of microbial consortia for water treatment, reporting that the treatments with soy protein-corn gluten and concentrated soy protein, fermented by Bacillus subtilis and added microbial consortia, presented a significantly lower concentration of ammonia and nitrite, compared with the control. Other alternatives are the use of biofloc systems (Hostins et al., 2017) which removes toxic nitrogen compounds through bacterial heterotrophic assimilation, oxidative chemoautotrophy or photoautotrophic assimilation (Huang et al., 2020a; Martins et al., 2020). RAS uses biofilters for metabolic refuse and ammonia removal from aquaculture systems (Tidwell, 2012; Huang et al., 2016b), avoiding the waste discharge to the environment. Additionally, other nutrients/waste can be used to generate biofloc that can be incorporated as food (Khatoon et al., 2016) optimising the use of nutrients. This is an example of the multitrophic aquaculture, where the waste of a species in residual water is captured and assimilated by species of lower trophic levels, which is a more sustainable option in traditional monoculture operations, seizing resources and allowing a wide variety of products (Liu et al., 2014; Omont et al., 2020). A derivation from this technology is the aquaponic systems that comprise an aquaculture system and hydroponic units, where the water enriched by nutrients generated by the former is redirected to the latter, providing nutrients for plant growth (Goddek et al., 2016), or the decoupled multi-loop aquaponics systems (Goddek and Körner, 2019). As an example of the advances in aquaponic systems, Fierro-Sañudo et al. (2020) reviewed the co-culture of shrimp with plants of economic importance. All alternatives presented to improve the eco-efficiency of the systems have a microbiological component and can be improved by assessing the best conditions that provide microbiological compositions to seize/reduce waste, but we need to consider the effect of other microbiomes that comprise the system, as the interaction among species in a community is critical and determines its functions (Dai et al., 2017) and their response to perturbations (Dai et al., 2019). In this scenario where the aquaculture expansion will also be restricted by land, cost and water availability, the systems described may allow a better use of all resources (Gooley and Gavine, 2003; Emerenciano et al., 2013; Boyd et al., 2020). Nevertheless, it is also necessary to consider the pros and cons of the productive systems from an environmental impact perspective, including the study of water and carbon footprints, analyses of the organism life cycle (Fiore, 2017; Henriksson et al., 2018), and data envelopment analysis (Cortés et al., 2021), as well as financial indicators (Rego et al., 2017), with the goal of selecting the best practices for production.
4 What it is the relevance of the eukaryotic microbiome in Penaeus aquaculture? And moreover, what is its role in the intensification systems such as biofloc?
So far, the bacterial component in intensive aquaculture systems has been studied more than their eukaryotic counterpart. The development of wet-lab protocols as well as the improvement of computational methods dedicated to the analysis of 16S rRNA amplicon sequences has biased the field towards the study of the prokaryotic component of microbial communities in all kinds of biomes. In aquaculture systems, the eukaryotic microbiota has been poorly analysed, making it too difficult to approach in applicative research. In addition, there is a historical misconception of the role of microalgae and protozoa in hyperintensive shrimp systems, leading to a common practice of reducing their abundance through the addition of high concentrations of carbohydrates (high C:N ratio) to enhance heterotrophic bacteria growth.
On the other hand, studies that consider the role of microeukaryotes in the system have revealed that the stability of a biofloc system with zero or minimal water exchange depends on the dynamic interaction between bacteria, fungi, protozoans, nematodes and rotifers, where phytoplankton and heterotrophic and nitrifying bacteria have the most crucial role in N and C utilisation (Emerenciano et al., 2017). In addition, microalgae are relevant for N and P absorption and provide greater nutritional value to the bioflocs, especially in integrated intensive aquaculture systems (Silva et al., 2022).
Phytoplankton plays an essential role in the biogeochemical reactions in aquatic systems, recycling important elements for life such as nitrogen, sulphur, and carbon. Researchers have described that carbon release into the system is possible due to microenvironments generated in the immediate region surrounding microalgal cells. The production of secondary metabolites favours the interaction between algae and certain bacteria in a sophisticated metabolic cooperative process (Rolland et al., 2016).
External factors like exposure to light impact the structure of phytoplankton and the rest of the microbial community. Exposure of hyperintensive systems to low light intensity promotes the development of a healthier microbial community compared with light-deprived systems. This results in higher survival and biomass of L. vannamei, whereas the occurrence of Vibrio spp. and heterotrophic bacteria decrease (Khoa et al., 2020). Supporting these observations, Yang and co-workers (2020b) reported that factors such as bacterioplankton fluctuations, temperature, dissolved oxygen, salinity and pH impact the phytoplankton community structure. The taxa most associated with rearing systems are Chlorophyta, Bacillariophyta and Pyrrophyta. During shrimp cultivation, the process goes from a highly diverse microbial state to a diatom bloom state.
In a recent work, Zhu and co-workers (2022) studied the sustainability of a multitrophic aquaculture system (rice-crayfish-culture or RCFP) through a multi-kingdom analysis. The authors observed fewer horizontal gene transfer events across kingdoms (particularly of antibiotic-resistance genes) and concluded that the system is a sustainable model more resilient to changes in certain environmental factors such as pH, oxidation-reduction potential, temperature and total N. Furthermore, they authors found that the RCFP system was dominated by positive multi-kingdom interactions in water, sediment and crayfish gut habitats.
The role of microbial eukaryotes in L. vannamei culture has not been widely explored despite their importance in the intensification of aquaculture systems. Whole metagenomics is a powerful technique that could be used to fill the gap in exploring the microbial composition and dynamics of complex communities in aquaculture models.
5 Diversity and potential function of viral communities in aquaculture systems
Although viral diversity in aquatic ecosystems has been well studied, our understanding of the role of virus populations in aquaculture systems is rather limited. Viruses are the most numerous biological entities on Earth, having a great impact on the microbial dynamics, metabolism and biogeochemical cycles in the aquatic ecosystems (Suttle, 2007; Chow and Suttle, 2015). In aquaculture, a virus can impact the holobiome in three ways: 1) infect the host as a pathogenic virus, like white spot syndrome; 2) regulate the proliferation of specific bacterial populations when the virus is active in the lytic phase; and 3) contribute auxiliary metabolic genes (AMGs) that supplement host metabolism when the virus is in the lysogenic phase (Chu et al., 2022). Most of the studies on viruses associated with aquaculture species have focussed on pathogenic viruses (Orosco and Lluisma, 2017), and only a few studies have aimed to understand viral diversity in prawn-culture environments.
Chu et al. (2022) reported that the order Caudovirales (i.e. viruses infecting bacteria and archaea) was the most represented in pond sediments (49% relative abundance). Using a predictive host analysis, they found viruses linked to 25 bacterial and archaeal phyla, with the most frequent being Proteobacteria, Bacteroidetes and Chloroflexi. In the same study, the authors reported up to 99 putative viruses encoding AMGs including genes involved in photosynthesis, carbohydrate metabolism, sulphur cycling and energy metabolism. Viral AMGs may help the host to respond to environmental gradients and to increase metabolic plasticity to better survive in their habitats.
To better understand the tight association between the virome and the prokaryotic fraction of the microbiota in a particular habitat, one may also consider their concerted contribution to modulate the ecosystem by carrying genetic elements such as virulence factors and antibiotic-resistance genes (García-López et al., 2019). The important role of viruses as genetic engineers is reflected by the great amount of DNA that is continuously transduced by phages across bacterial cells. However, the contributions of viral communities in aquaculture systems and related environments remain to be investigated. Due to the lack of universal gene markers, metagenomics will be required to study viral diversity and the function. The major challenges to this endeavour are a lack of information on the dynamics and biogeography of viral communities and their potential biogeochemical impacts. Moreover, a significant fraction of the virome remains to be characterised, the so-called dark matter of biological diversity (Chow and Suttle, 2015; Roux et al., 2015).
6 Discussion
The intestinal microbiome of aquatic organisms is intimately linked to the environment that surrounds them. Accordingly, to develop microbiological management strategies with a positive impact on the productive systems, the intestinal microbiome of culture species should be analysed from the ‘holobiome of the aquaculture system’ perspective. In this sense, we have to start gathering as much information as we can about the microbial communities associated with the system, including the prokaryotic, micro-eukaryotic and virome fractions. The non-culturable species are the most challenging to describe using conventional methods of microbiology. However, advances in DNA sequencing technologies have improved our understanding of the extent of microbial diversity in nature. The use of deep sequencing to reconstruct metagenome assembled genomes of uncultured species and the development of methods like single-cell sequencing, are examples of tools being explored to elucidate the potential function and taxonomy profiles of the microbial community as a whole, and to decipher particular syntrophic processes (Lasken and McLean, 2014; Parks et al., 2017; Zhou et al., 2021).
The study of the ‘holobiome of the aquaculture system’ in the culture of L. vannamei focuses primarily on the identification of the functional and taxonomic characteristics of the intestinal microbiome in cultures where some benefit in production is observed. Knowledge of intestinal microbial composition or the key taxa (taxonomic signatures) allow to test its ex situ replication, manipulating the species composition in the lab, and validating through inoculation in the intestine of shrimp, similarly to faecal transplantation (Wang et al., 2019a). However, this approach tends to simplify the role of low-abundance species and its contribution as reservoirs of functions in adverse conditions (Wang et al., 2017). To incorporate to the analysis every single piece of information associated with the system require the use of computational methods such as machine learning and artificial intelligence, applied in data modelling and condition predictions (Wirbel et al., 2021). These kinds of algorithms work better with large amounts of data, allowing the incorporation of physicochemical data, nutritional conditions of the system, functional and taxonomic profiles obtained using diverse omic approaches, microbial interactions predicted and/or tested, etc.
To get the most of these technologies in the improvement of production in aquaculture systems, it is necessary the development of ad-hoc computational methods, the enrichment of the databases to improve predictions and contextualise the data, and to continue testing and validating in the lab. For instance, results from prediction models could be replicated in biofloc bioreactors maintaining conditions consistent with aquaculture production parameters (i.e., pH, temperature, dissolved oxygen). The validation of the structures could be carried out by supplying the synthetic biofloc to the water of the culture systems or incorporating it into the food, to finally evaluate if the resulting intestinal microbiome is stable and beneficial for production.
The holobiome perspective implies a greater investment of resources and time for research, but it will accelerate the development of technology that will benefit the development of the aquaculture industry, leading to its sustainability.
Author contributions
EG-P: conceptualization, resources, writing—original draft preparation, review and editing. PM-S: conceptualization, visualization, supervision, writing—review and editing. RV-J: conceptualization, visualization, supervision, writing—review and editing. FM-B: conceptualization, visualization, supervision, writing—review and editing. MM-M: conceptualization, visualization, supervision, writing—review and editing. AE-Z: conceptualization, visualization, supervision, writing—review and editing. All authors have read and agreed to the published version of this manuscript.
Acknowledgments
The authors are grateful for the CONACYT scholarship awarded to EG-P; to BioRender.com for the images created on its platform.
Conflict of interest
The authors declare that the research was conducted in the absence of any commercial or financial relationships that could be construed as a potential conflict of interest.
Publisher’s note
All claims expressed in this article are solely those of the authors and do not necessarily represent those of their affiliated organizations, or those of the publisher, the editors and the reviewers. Any product that may be evaluated in this article, or claim that may be made by its manufacturer, is not guaranteed or endorsed by the publisher.
References
Aßhauer K. P., Wemheuer B., Daniel R., Meinicke P. (2015). Tax4Fun: predicting functional profiles from metagenomic 16S rRNA data. Bioinformatics 31, 2882–2884. doi: 10.1093/bioinformatics/btv287
Alday-Sanz V., Brock J., Flegel T. W., McIntosh R., Bondad-Reantaso M. G., Salazar M., et al. (2020). Facts, truths and myths about SPF shrimp in aquaculture. Rev. Aquacult. 12, 76–84. doi: 10.1111/raq.12305
Araneda M., Pérez E. P., Gasca-Leyva E. (2008). White shrimp Penaeus vannamei culture in freshwater at three densities: condition state based on length and weight. Aquaculture 283, 13–18. doi: 10.1016/j.aquaculture.2008.06.030
Barb J. J., Oler A. J., Kim H. S., Chalmers N., Wallen G. R., Cashion A., et al. (2016). Development of an analysis pipeline characterizing multiple hypervariable regions of 16S rRNA using mock samples. PLoS One 11, e0148047. doi: 10.1371/journal.pone.0148047
Bass D., Stentiford G. D., Wang H. C., Koskella B., Tyler C. R. (2019). The pathobiome in animal and plant diseases. Trends Ecol. Evol. 34, 996–1008. doi: 10.1016/j.tree.2019.07.012
Bellali S., Lagier J. C., Raoult D., Bou Khalil J. (2019). Among live and dead bacteria, the optimization of sample collection and processing remains essential in recovering gut microbiota components. Front. Microbiol. 10. doi: 10.3389/fmicb.2019.01606
Bentzon-Tilia M., Sonnenschein E. C., Gram L. (2016). Monitoring and managing microbes in aquaculture–towards a sustainable industry. Microb. Biotechnol. 9, 576–584. doi: 10.1111/1751-7915.12392
Berg G., Rybakova D., Fischer D., Cernava T., Vergès M. C. C., Charles T., et al. (2020). Microbiome definition re-visited: old concepts and new challenges. Microbiome 8, 103. doi: 10.1186/s40168-020-00875-0
Boyd C. E., D’Abramo L. R., Glencross B. D., Huyben D. C., Juarez L. M., Lockwood G. S., et al. (2020). Achieving sustainable aquaculture: Historical and current perspectives and future needs and challenges. J. World Aquac. Soc 51, 578–633. doi: 10.1111/jwas.12714
Buford T. W. (2017). (Dis) trust your gut: the gut microbiome in age-related inflammation, health, and disease. Microbiome 5, 80. doi: 10.1186/s40168-017-0296-0
Butt R. L., Volkoff H. (2019). Gut microbiota and energy homeostasis in fish. Front. Endocrinol. 10. doi: 10.3389/fendo.2019.00009
Callahan B. J., McMurdie P. J., Holmes S. P. (2017). Exact sequence variants should replace operational taxonomic units in marker-gene data analysis. ISME J. 11, 2639–2643. doi: 10.1038/ismej.2017.119
Cardona E., Gueguen Y., Magré K., Lorgeoux B., Piquemal D., Pierrat F., et al. (2016). Bacterial community characterization of water and intestine of the shrimp Litopenaeus stylirostris in a biofloc system. BMC Microbiol. 16, 157. doi: 10.1186/s12866-016-0770-z
Carlos N., Tang Y.-W., Pei Z. (2012). Pearls and pitfalls of genomics-based microbiome analysis. Emerg. Microbes Infect. 1, e45–e45. doi: 10.1038/emi.2012.41
Ceccaldi H. (1989). “Anatomy and physiology of digestive tract of crustaceans decapods reared in aquaculture,” in Actes de colloques ifremer, vol. 26. (Tahiti, French Polynesia), pp. 243–259.
Chai P. C., Song X. L., Chen G. F., Xu H., Huang J. (2016). Dietary supplementation of probiotic Bacillus PC465 isolated from the gut of Fenneropenaeus chinensis improves the health status and resistance of Litopenaeus vannamei against white spot syndrome virus. Fish Shellfish Immunol. 54, 602–611. doi: 10.1016/j.fsi.2016.05.011
Cheng A. C., Lin H. L., Shiu Y. L., Tyan Y. C., Liu C. H. (2017). Isolation and characterization of antimicrobial peptides derived from Bacillus subtilis E20-fermented soybean meal and its use for preventing Vibrio infection in shrimp aquaculture. Fish Shellfish Immunol. 67, 270–279. doi: 10.1016/j.fsi.2017.06.006
Cheng A. C., Yeh S. P., Hu S. Y., Lin H. L., Liu C. H. (2020). Intestinal microbiota of white shrimp, Litopenaeus vannamei, fed diets containing Bacillus subtilis E20-fermented soybean meal (FSBM) or an antimicrobial peptide derived from B. subtilis E20-FSBM. Aquac. Res. 51, 41–50. doi: 10.1111/are.14345
Chen W. Y., Ng T. H., Wu J. H., Chen J. W., Wang H. C. (2017). Microbiome dynamics in a shrimp grow-out pond with possible outbreak of acute hepatopancreatic necrosis disease. Sci. Rep. 7, 9395. doi: 10.1038/s41598-017-09923-6
Chow C. E. T., Suttle C. A. (2015). Biogeography of viruses in the sea. Annu. Rev. Virol. 2, 41–66. doi: 10.1146/annurev-virology-031413-085540
Chu Y., Zhao Z., Cai L., Zhang G. (2022). Viral diversity and biogeochemical potential revealed in different prawn-culture sediments by virus-enriched metagenome analysis. Environ. Res. 210, 112901. doi: 10.1016/j.envres.2022.112901
Claassen S., du Toit E., Kaba M., Moodley C., Zar H. J., Nicol M. P. (2013). A comparison of the efficiency of five different commercial DNA extraction kits for extraction of DNA from faecal samples. J. Microbiol. Methods 94, 103–110. doi: 10.1016/j.mimet.2013.05.008
Córdova-Murueta J. H., Garcıa-Carreno F. L., Navarrete-del M. A. (2003). Digestive enzymes present in crustacean feces as a tool for biochemical, physiological, and ecological studies. J. Exp. Mar. Biol. Ecol. 297, 43–56. doi: 10.1016/S0022-0981(03)00355-1
Cornejo-Granados F., Gallardo-Becerra L., Leonardo-Reza M., Ochoa-Romo J. P., Ochoa-Leyva A. (2018). A meta-analysis reveals the environmental and host factors shaping the structure and function of the shrimp microbiota. PeerJ 6, e5382. doi: 10.7717/peerj.5382
Cornejo-Granados F., Lopez-Zavala A. A., Gallardo-Becerra L., Mendoza-Vargas A., Sánchez F., Vichido R., et al. (2017). Microbiome of pacific whiteleg shrimp reveals differential bacterial community composition between wild, aquacultured and AHPND/EMS outbreak conditions. Sci. Rep. 7, 11783. doi: 10.1038/s41598-017-11805-w
Cortés A., Casillas-Hernández R., Cambeses-Franco C., Bórquez-López R., Magallón-Barajas F., Quadros-Seiffert W., et al. (2021). Eco-efficiency assessment of shrimp aquaculture production in Mexico. Aquaculture 544, 737145. doi: 10.1016/j.aquaculture.2021.737145
Crab R., Avnimelech Y., Defoirdt T., Bossier P., Verstraete W. (2007). Nitrogen removal techniques in aquaculture for a sustainable production. Aquaculture 270, 1–14. doi: 10.1016/j.aquaculture.2007.05.006
Crab R., Defoirdt T., Bossier P., Verstraete W. (2012). Biofloc technology in aquaculture: beneficial effects and future challenges. Aquaculture 356, 351–356. doi: 10.1016/j.aquaculture.2012.04.046
Creer S., Deiner K., Frey S., Porazinska D., Taberlet P., Thomas W. K., et al. (2016). The ecologist’s field guide to sequence-based identification of biodiversity. Methods Ecol. Evol. 7, 1008–1018. doi: 10.1111/2041-210X.12574
Dai W., Qiu Q., Chen J., Xiong J. (2019). Gut eukaryotic disease-discriminatory taxa are indicative of pacific white shrimp (Litopenaeus vannamei) white feces syndrome. Aquaculture 506, 154–160. doi: 10.1016/j.aquaculture.2019.03.034
Dai W., Sheng Z., Chen J., Xiong J. (2020). Shrimp disease progression increases the gut bacterial network complexity and abundances of keystone taxa. Aquaculture 517, 734802. doi: 10.1016/j.aquaculture.2019.734802
Dai W., Yu W., Xuan L., Tao Z., Xiong J. (2018). Integrating molecular and ecological approaches to identify potential polymicrobial pathogens over a shrimp disease progression. Appl. Microbiol. Biotechnol. 102, 3755–3764. doi: 10.1007/s00253-018-8891-y
Dai W., Yu W., Zhang J., Zhu J., Tao Z., Xiong J. (2017). The gut eukaryotic microbiota influences the growth performance among cohabitating shrimp. Appl. Microbiol. Biotechnol. 101, 6447–6457. doi: 10.1007/s00253-017-8388-0
Dall W. (1967). The functional anatomy of the digestive tract of a shrimp Metapenaeus bennettae racek and dall (Crustacea: Decapoda: Penaeidae). Aust. J. Zool. 15, 699–714. doi: 10.1071/ZO9670699
da Silva K. R., Wasielesky W. Jr., Abreu P. C. (2013). ). nitrogen and phosphorus dynamics in the biofloc production of the pacific white shrimp, Litopenaeus vannamei. J. World Aquac. Soc 44, 30–41. doi: 10.1111/jwas.12009
Dauda A. B. (2020). Biofloc technology: a review on the microbial interactions, operational parameters and implications to disease and health management of cultured aquatic animals. Rev. Aquacult. 12, 1193–1210. doi: 10.1111/raq.12379
Davis R. P., Boyd C. E., Davis D. A. (2021). Resource sharing and resource sparing, understanding the role of production intensity and farm practices in resource use in shrimp aquaculture. Ocean Coast. Manage. 207, 105595. doi: 10.1016/j.ocecoaman.2021.105595
Dawood M. A., Koshio S. (2020). Application of fermentation strategy in aquafeed for sustainable aquaculture. Rev. Aquacult. 12, 987–1002. doi: 10.1111/raq.12368
Debelius J., Song S. J., Vazquez-Baeza Y., Xu Z. Z., Gonzalez A., Knight R. (2016). Tiny microbes, enormous impacts: what matters in gut microbiome studies? Genome Biol. 17, 217. doi: 10.1186/s13059-016-1086-x
Deng Y., Xu X., Yin X., Lu H., Chen G., Yu J., et al. (2019). Effect of stock density on the microbial community in biofloc water and pacific white shrimp (Litopenaeus vannamei) gut microbiota. Appl. Microbiol. Biotechnol. 103, 4241–4252. doi: 10.1007/s00253-019-09773-4
Dittmann K. K., Rasmussen B. B., Castex M., Gram L., Bentzon-Tilia M. (2017). The aquaculture microbiome at the centre of business creation. Microb. Biotechnol. 10, 1279–1282. doi: 10.1111/1751-7915.12877
Douglas G. M., Beiko R. G., Langille M. G. (2018). “Predicting the functional potential of the microbiome from marker genes using PICRUSt,” in Microbiome analysis. Eds. Beiko R., Hsiao W., Parkinson J. (New York: Humana Press). doi: 10.1007/978-1-4939-8728-3_11
Duan Y., Liu Q., Wang Y., Zhang J., Xiong D. (2018). Impairment of the intestine barrier function in litopenaeus vannamei exposed to ammonia and nitrite stress. Fish Shellfish Immunol. 78, 279–288. doi: 10.1016/j.fsi.2018.04.050
Duan Y., Wang Y., Liu Q., Xiong D., Zhang J. (2019a). Transcriptomic and microbiota response on Litopenaeus vannamei intestine subjected to acute sulfide exposure. Fish Shellfish Immunol. 88, 335–343. doi: 10.1016/j.fsi.2019.02.021
Duan Y., Wang Y., Liu Q., Zhang J., Xiong D. (2019b). Changes in the intestine barrier function of Litopenaeus vannamei in response to pH stress. Fish Shellfish Immunol. 88, 142–149. doi: 10.1016/j.fsi.2019.02.047
Duan Y., Zhang Y., Dong H., Wang Y., Zhang J. (2017). Effects of dietary poly-β-hydroxybutyrate (PHB) on microbiota composition and the mTOR signaling pathway in the intestines of. Litopenaeus vannamei. J. Microbiol. 55, 946–954. doi: 10.1007/s12275-017-7273-y
Ekasari J., Angela D., Waluyo S. H., Bachtiar T., Surawidjaja E. H., Bossier P., et al. (2014a). The size of biofloc determines the nutritional composition and the nitrogen recovery by aquaculture animals. Aquaculture 426, 105–111. doi: 10.1016/j.aquaculture.2014.01.023
Ekasari J., Azhar M. H., Surawidjaja E. H., Nuryati S., De Schryver P., Bossier P. (2014b). Immune response and disease resistance of shrimp fed biofloc grown on different carbon sources. Fish Shellfish Immunol. 41, 332–339. doi: 10.1016/j.fsi.2014.09.004
Elizondo-González R., Quiroz-Guzmán E., Howe A., Yang F., Flater J., Gemin M., et al. (2020). Changes on the intestinal bacterial community of white shrimp Penaeus vannamei fed with green seaweeds. J. Appl. Phycol. 32, 2061–2070. doi: 10.1007/s10811-020-02072-w
Ellul R. M., Kalatzis P. G., Frantzen C., Haugland G. T., Gulla S., Colquhoun D. J., et al. (2021). Genomic analysis of Pasteurella atlantica provides insight on its virulence factors and phylogeny and highlights the potential of reverse vaccinology in aquaculture. Microorganisms 9, 1215. doi: 10.3390/Microorganisms9061215
Emerenciano M., Gaxiola G., Cuzon G. (2013). “Biofloc technology (BFT): a review for aquaculture application and animal food industry,” in Biomass now - cultivation and utilization. Ed. Matovic M. D. (Rijeka: IntechOpen, London), 301–328. InTech. doi: 10.5772/53902
Emerenciano M. G. C., Martínez-Córdova L. R., Martínez-Porchas M., Miranda-Baeza A. (2017). “Biofloc technology (BFT): a tool for water quality management in aquaculture,” in Water quality. Ed. Tutu H. (Croatia: IntechOpen, London), 92–109. InTech. doi: 10.5772/66416
Fan J., Chen L., Mai G., Zhang H., Yang J., Deng D., et al. (2019a). Dynamics of the gut microbiota in developmental stages of Litopenaeus vannamei reveal its association with body weight. Sci. Rep. 9, 734. doi: 10.1038/s41598-018-37042-3
Fan L., Wang Z., Chen M., Qu Y., Li J., Zhou A., et al. (2019b). Microbiota comparison of Pacific white shrimp intestine and sediment at freshwater and marine cultured environment. Sci. Total Environ. 657, 1194–1204. doi: 10.1016/j.scitotenv.2018.12.069
FAO (2020). “The state of world fisheries and aquaculture. sustainability in action,” The state of world fisheries and aquaculture 2020 (Rome: Food and Agriculture Organization of the United Nations (FAO)). doi: 10.4060/ca9229en
Fatimah N., Pande G. S. J., Natrah F. M. I., Meritha W. W., Sucipto A., Ekasari J. (2019). The role of microbial quorum sensing on the characteristics and functionality of bioflocs in aquaculture systems. Aquaculture 504, 420–426. doi: 10.1016/j.aquaculture.2019.02.022
Ferrand J., Patron K., Legrand-Frossi C., Frippiat J. P., Merlin C., Alauzet C., et al. (2014). Comparison of seven methods for extraction of bacterial DNA from fecal and cecal samples of mice. J. Microbiol. Methods 105, 180–185. doi: 10.1016/j.mimet.2014.07.029
Fetissov S. O. (2017). Role of the gut microbiota in host appetite control: bacterial growth to animal feeding behaviour. Nat. Rev. Endocrinol. 13, 11–25. doi: 10.1038/nrendo.2016.150
Fierro-Sañudo J. F., Rodríguez-Montes de Oca G. A., Páez-Osuna F. (2020). Co-Culture of shrimp with commercially important plants: a review. Rev. Aquacult. 12, 2411–2428. doi: 10.1111/raq.12441
Fiore G. (2017). Farming and food security: an assessment of animal productions and environmental impact, EUR 28792 EN (Luxembourg: Publications Office of the European Union). doi: 10.2760/935752
Fu Z., Han F., Huang K., Zhang J., Qin J. G., Chen L., et al. (2022). Impact of imidacloprid exposure on the biochemical responses, transcriptome, gut microbiota and growth performance of the pacific white shrimp Litopenaeus vannamei. J. Hazard. Mater. 424, 127513. doi: 10.1016/j.jhazmat.2021.127513
Gainza O., Ramírez C., Ramos A. S., Romero J. (2018). Intestinal microbiota of white shrimp Penaeus vannamei under intensive cultivation conditions in Ecuador. Microb. Ecol. 75, 562–568. doi: 10.1007/s00248-017-1066-z
Gainza O., Romero J. (2020). Effect of mannan oligosaccharides on the microbiota and productivity parameters of Litopenaeus vannamei shrimp under intensive cultivation in Ecuador. Sci. Rep. 10, 2719. doi: 10.1038/s41598-020-59587-y
García-López R., Cornejo-Granados F., Lopez-Zavala A., Sánchez-López F., Cota-Huízar A., Sotelo-Mundo R., et al. (2020). Doing more with less: A comparison of 16S hypervariable regions in search of defining the shrimp microbiota. Microorganisms 8, 134. doi: 10.3390/microorganisms8010134
García-López R., Pérez-Brocal V., Moya A. (2019). Beyond cells–the virome in the human holobiont. Microb. Cell. 6, 373–396. doi: 10.15698/mic2019.09.689
Garibay-Valdez E., Martínez-Porchas M., Calderón K., Vargas-Albores F., Gollas-Galván T., Martínez-Córdova L. (2020). Taxonomic and functional changes in the microbiota of the white shrimp (Litopenaeus vannamei) associated with postlarval ontogenetic development. Aquaculture 518, 734842. doi: 10.1016/j.aquaculture.2019.734842
Goddek S., Espinal C. A., Delaide B., Jijakli M. H., Schmautz Z., Wuertz S., et al. (2016). Navigating towards decoupled aquaponic systems: a system dynamics design approach. Water 8, 303. doi: 10.3390/w8070303
Goddek S., Körner O. (2019). A fully integrated simulation model of multi-loop aquaponics: a case study for system sizing in different environments. Agric. Syst. 171, 143–154. doi: 10.1016/j.agsy.2019.01.010
Goodrich J. K., Di Rienzi S. C., Poole A. C., Koren O., Walters W. A., Caporaso J. G., et al. (2014). Conducting a microbiome study. Cell 158, 250–262. doi: 10.1016/j.cell.2014.06.037
Gooley G., Gavine F. (2003). ). introduction to integrated agri-aquaculture systems (Barton: RIRDC).
Guevarra R. B., Magez S., Peeters E., Chung M. S., Kim K. H., Radwanska M. (2021). Comprehensive genomic analysis reveals virulence factors and antibiotic resistance genes in Pantoea agglomerans KM1, a potential opportunistic pathogen. PLoS One 16, e0239792. doi: 10.1371/journal.pone.0239792
Guo H., Huang L., Hu S., Chen C., Huang X., Liu W., et al. (2020). Effects of carbon/nitrogen ratio on growth, intestinal microbiota and metabolome of shrimp (Litopenaeus vannamei). Front. Microbiol. 11. doi: 10.3389/fmicb.2020.00652
Hamidoghli A., Won S., Farris N. W., Bae J., Choi W., Yun H., et al. (2020). Solid state fermented plant protein sources as fish meal replacers in whiteleg shrimp Litopenaeus vannamei. Anim. Feed Sci. Technol. 264, 114474. doi: 10.1016/j.anifeedsci.2020.114474
Handelsman J. (2004). Metagenomics: application of genomics to uncultured microorganisms. Microbiol. Mol. Biol. Rev. 68, 669–685. doi: 10.1128/MMBR.68.4.669-685.2004
Henriksson P. J. G., Belton B., Murshed-e-Jahan K., Rico A. (2018). Measuring the potential for sustainable intensification of aquaculture in Bangladesh using life cycle assessment. PNAS 115, 2958–2963. doi: 10.1073/pnas.1716530115
He Z., Pan L., Zhang M., Zhang M., Huang F., Gao S. (2020). Metagenomic comparison of structure and function of microbial community between water, effluent and shrimp intestine of higher place Litopenaeus vannamei ponds. J. Appl. Microbiol. 129, 243–255. doi: 10.1111/jam.14610
He W., Rahimnejad S., Wang L., Song K., Lu K., Zhang C. (2017). Effects of organic acids and essential oils blend on growth, gut microbiota, immune response and disease resistance of pacific white shrimp (Litopenaeus vannamei) against Vibrio parahaemolyticus. Fish Shellfish Immunol. 70, 164–173. doi: 10.1016/j.fsi.2017.09.007
Heravi F. S., Zakrzewski M., Vickery K., Hu H. (2020). Host DNA depletion efficiency of microbiome DNA enrichment methods in infected tissue samples. J. Microbiol. Methods 170, 105856. doi: 10.1016/j.mimet.2020.105856
Hernández-Cabanyero C., Amaro C. (2020). Phylogeny and life cycle of the zoonotic pathogen Vibrio vulnificus. Environ. Microbiol. 22, 4133–4148. doi: 10.1111/1462-2920.15137
Holt C. C., Bass D., Stentiford G. D., van der Giezen M. (2020). Understanding the role of the shrimp gut microbiome in health and disease. J. Invertebr. Pathol. 186, 107387. doi: 10.1016/j.jip.2020.107387
Hostins B., Lara G., Decamp O., Cesar D. E., Wasielesky J. W. (2017). Efficacy and variations in bacterial density in the gut of litopenaeus vannamei reared in a BFT system and in clear water supplemented with a commercial probiotic mixture. Aquaculture 480, 58–64. doi: 10.1016/j.aquaculture.2017.07.036
Hou D., Huang Z., Zeng S., Liu J., Wei D., Deng X., et al. (2018a). Intestinal bacterial signatures of white feces syndrome in shrimp. Appl. Microbiol. Biotechnol. 102, 3701–3709. doi: 10.1007/s00253-018-8855-2
Hou D., Huang Z., Zeng S., Liu J., Weng S., He J. (2018b). Comparative analysis of the bacterial community compositions of the shrimp intestine, surrounding water and sediment. J. Appl. Microbiol. 125, 792–799. doi: 10.1111/jam.13919
Hua K., Cobcroft J. M., Cole A., Condon K., Jerry D. R., Mangott A., et al. (2019). The future of aquatic protein: implications for protein sources in aquaculture diets. One Earth 1, 316–329. doi: 10.1016/j.oneear.2019.10.018
Huang L., Guo H., Chen C., Huang X., Chen W., Bao F., et al. (2020a). The bacteria from large-sized bioflocs are more associated with the shrimp gut microbiota in culture system. Aquaculture 523, 735159. doi: 10.1016/j.aquaculture.2020.735159
Huang Z., Li X., Wang L., Shao Z. (2016a). Changes in the intestinal bacterial community during the growth of white shrimp, Litopenaeus vannamei. Aquac. Res. 47, 1737–1746. doi: 10.1111/are.12628
Huang F., Pan L., Song M., Tian C., Gao S. (2018). Microbiota assemblages of water, sediment, and intestine and their associations with environmental factors and shrimp physiological health. Appl. Microbiol. Biotechnol. 102, 8585–8598. doi: 10.1007/s00253-018-9229-5
Huang Z., Wan R., Song X., Liu Y., Hallerman E., Dong D., et al. (2016b). Metagenomic analysis shows diverse, distinct bacterial communities in biofilters among different marine recirculating aquaculture systems. Aquacult. Int. 24, 1393–1408. doi: 10.1007/s10499-016-9997-9
Huang Z., Zeng S., Xiong J., Hou D., Zhou R., Xing C., et al. (2020b). Microecological koch’s postulates reveal that intestinal microbiota dysbiosis contributes to shrimp white feces syndrome. Microbiome 8, 32. doi: 10.1186/s40168-020-00802-3
Huerta-Rábago J. A., Martínez-Porchas M., Miranda-Baeza A., Nieves-Soto M., Rivas-Vega M. E., Martínez-Córdova L. R. (2019). Addition of commercial probiotic in a biofloc shrimp farm of Litopenaeus vannamei during the nursery phase: effect on bacterial diversity using massive sequencing 16S rRNA. Aquaculture 502, 391–399. doi: 10.1016/j.aquaculture.2018.12.055
Hu X., Yang H. L., Yan Y. Y., Zhang C. X., Ye J. D., Lu K. L., et al. (2019). Effects of fructooligosaccharide on growth, immunity and intestinal microbiota of shrimp (Litopenaeus vannamei) fed diets with fish meal partially replaced by soybean meal. Aquac. Nutr. 25, 194–204. doi: 10.1111/anu.12843
Jamal M. T., Broom M., Al-Mur B. A., Al Harbi M., Ghandourah M., Al Otaibi A., et al. (2020). Biofloc technology: Emerging microbial biotechnology for the improvement of aquaculture productivity. Pol. J. Microbiol. 69, 401–409. doi: 10.33073/pjm-2020-049
Jannathulla R., Dayal J. S., Vasanthakumar D., Ambasankar K., Muralidhar M. (2017). Effect of fermentation methods on amino acids, fiber fractions and anti-nutritional factors in different plant protein sources and essential amino acid index for Penaeus vannamei Boone 1931. Indian J. Fish. 64, 40–47. doi: 10.21077/ijf.2017.64.2.60341-07
Jannathulla R., Dayal J. S., Vasanthakumar D., Ambasankar K., Muralidhar M. (2018). Effect of fungal fermentation on apparent digestibility coefficient for dry matter, crude protein and amino acids of various plant protein sources in Penaeus vannamei. Aquac. Nutr. 24, 1318–1329. doi: 10.1111/anu.12669
Jiang L., Feng J., Ying R., Yin F., Pei S., Lu J., et al. (2019b). Individual and combined effects of ammonia-n and sulfide on the immune function and intestinal microbiota of pacific white shrimp Litopenaeus vannamei. Fish Shellfish Immunol. 92, 230–240. doi: 10.1016/j.fsi.2019.06.020
Jiang B., Sun J., Lv A., Hu X., Shi H., Sung Y., et al. (2019a). Impact of DNA extraction methods on the observed microbial communities from the intestinal flora of the penaeid shrimp Litopenaeus vannamei. FEMS Microbiol. Lett. 366, fnz099. doi: 10.1093/femsle/fnz099
Jovel J., Patterson J., Wang W., Hotte N., O’Keefe S., Mitchel T., et al. (2016). Characterization of the gut microbiome using 16S or shotgun metagenomics. Front. Microbiol. 7. doi: 10.3389/fmicb.2016.00459
Jun-xia Y., Wen-ying S., Ping L. (2004). Study on the influence of water temperature on the intestinal microflora of Penaeus vannamei. Mar. Sci. 28, 33–36.
Kang H. Y., Yang P. Y., Dominy W. G., Lee C. S. (2010). Bioprocessing papaya processing waste for potential aquaculture feed supplement–economic and nutrient analysis with shrimp feeding trial. Bioresour. Technol. 101, 7973–7979. doi: 10.1016/j.biortech.2010.05.058
Khatoon H., Banerjee S., Yuan G. T. G., Haris N., Ikhwanuddin M., Ambak M. A., et al. (2016). Biofloc as a potential natural feed for shrimp postlarvae. Int. Biodeterior. Biodegradation 113, 304–309. doi: 10.1016/j.ibiod.2016.04.006
Khoa T. N. D., Tao C. T., Khanh L. V., Hai T. N. (2020). Super-intensive culture of white leg shrimp (Litopenaeus vannamei) in outdoor biofloc systems with different sunlight exposure levels: Emphasis on commercial applications. Aquaculture 524, 735277. doi: 10.1016/j.aquaculture.2020.735277
Kia E., Wagner Mackenzie B., Middleton D., Lau A., Waite D. W., Lewis G., et al. (2016). Integrity of the human faecal microbiota following long-term sample storage. PLoS One 11, e0163666. doi: 10.1371/journal.pone.0163666
Koslicki D., Foucart S., Rosen G. (2014). WGSQuikr: fast whole-genome shotgun metagenomic classification. PLoS One 9, e91784. doi: 10.1371/journal.pone.0091784
Kuhn D. D., Lawrence A. L., Boardman G. D., Patnaik S., Marsh L., Flick J. G.J. (2010). Evaluation of two types of bioflocs derived from biological treatment of fish effluent as feed ingredients for pacific white shrimp, Litopenaeus vannamei. Aquaculture 303, 28–33. doi: 10.1016/j.aquaculture.2010.03.001
Kuhn D. D., Lawrence A. L., Crockett J., Taylor D. (2016). Evaluation of bioflocs derived from confectionary food effluent water as a replacement feed ingredient for fishmeal or soy meal for shrimp. Aquaculture 454, 66–71. doi: 10.1016/j.aquaculture.2015.12.009
Kumar V. S., Pandey P. K., Anand T., Bhuvaneswari R., Kumar S. (2017). Effect of periphyton (aquamat) on water quality, nitrogen budget, microbial ecology, and growth parameters of Litopenaeus vannamei in a semi-intensive culture system. Aquaculture 479, 240–249. doi: 10.1016/j.aquaculture.2017.05.048
Landsman A., St-Pierre B., Rosales-Leija M., Brown M., Gibbons W. (2019a). Impact of aquaculture practices on intestinal bacterial profiles of pacific whiteleg shrimp Litopenaeus vannamei. Microorganisms 7, 93. doi: 10.3390/microorganisms7040093
Landsman A., St-Pierre B., Rosales-Leija M., Brown M., Gibbons W. (2019b). Investigation of the potential effects of host genetics and probiotic treatment on the gut bacterial community composition of aquaculture-raised pacific whiteleg shrimp, Litopenaeus vannamei. Microorganisms 7, 217. doi: 10.3390/microorganisms7080217
Lasken R. S., McLean J. S. (2014). Recent advances in genomic DNA sequencing of microbial species from single cells. Nat. Rev. Genet. 15, 577–584. doi: 10.1038/nrg3785
Liang Q., Li Z., Ou M., Wu X., Qiao X., Wei W., et al. (2020). Hypoimmunity and intestinal bacterial imbalance are closely associated with blue body syndrome in cultured Penaeus vannamei. Aquaculture 522, 735118. doi: 10.1016/j.aquaculture.2020.735118
Li J., Jiang H., Li L., Zhang X., Chen J. (2019). The effect of disease and season to hepatopancreas and intestinal mycobiota of Litopenaeus vannamei. Front. Microbiol. 10. doi: 10.3389/fmicb.2019.00889
Lim Y. W., Haynes M., Furlan M., Robertson C. E., Harris J. K., Rohwer F. (2014). Purifying the impure: sequencing metagenomes and metatranscriptomes from complex animal-associated samples. J. Vis. Exp. 94, e52117. doi: 10.3791/52117
Liñan-Vidriales M. A., Peña-Rodríguez A., Tovar-Ramírez D., Elizondo-González R., Barajas-Sandoval D. R., Ponce-Gracía E. I., et al. (2021). Effect of rice bran fermented with Bacillus and Lysinibacillus species on dynamic microbial activity of pacific white shrimp (Penaeus vannamei). Aquaculture 531, 735958. doi: 10.1016/j.aquaculture.2020.735958
Liu G., Deng Y., Verdegem M., Ye Z., Zhu S. (2019a). Using poly (β-hydroxybutyrate-β-hydroxyvalerate) as carbon source in biofloc-systems: Nitrogen dynamics and shift of Oreochromis niloticus gut microbiota. Sci. Total Environ. 694, 133664. doi: 10.1016/j.scitotenv.2019.133664
Liu K., Han J., Li S., Liu L., Lin W., Luo J. (2019c). Insight into the diversity of antibiotic resistance genes in the intestinal bacteria of shrimp Penaeus vannamei by culture-dependent and independent approaches. Ecotoxicol. Environ. Saf. 172, 451–459. doi: 10.1016/j.ecoenv.2019.01.109
Liu L., Hu Z., Dai X., Avnimelech Y. (2014). Effects of addition of maize starch on the yield, water quality and formation of bioflocs in an integrated shrimp culture system. Aquaculture 418, 79–86. doi: 10.1016/j.aquaculture.2013.10.005
Liu Z., Klümper U., Liu Y., Yang Y., Wei Q., Lin J. G., et al. (2019d). Metagenomic and metatranscriptomic analyses reveal activity and hosts of antibiotic resistance genes in activated sludge. Environ. Int. 129, 208–220. doi: 10.1016/j.envint.2019.05.036
Liu J., Wang K., Wang Y., Chen W., Jin Z., Yao Z., et al. (2019b). Strain-specific changes in the gut microbiota profiles of the white shrimp Litopenaeus vannamei in response to cold stress. Aquaculture 503, 357–366. doi: 10.1016/j.aquaculture.2019.01.026
Liu G., Zhu S., Liu D., Guo X., Ye Z. (2017). Effects of stocking density of the white shrimp Litopenaeus vannamei (Boone) on immunities, antioxidant status, and resistance against Vibrio harveyi in a biofloc system. Fish Shellfish Immunol. 67, 19–26. doi: 10.1016/j.fsi.2017.05.038
Li E., Xu C., Wang X., Wang S., Zhao Q., Zhang M., et al. (2018). Gut microbiota and its modulation for healthy farming of pacific white shrimp Litopenaeus vannamei. Rev. Fish. Sci. Aquac. 26, 381–399. doi: 10.1080/23308249.2018.1440530
Lloyd-Price J., Mahurkar A., Rahnavard G., Crabtree J., Orvis J., Hall A. B., et al. (2017). Strains, functions and dynamics in the expanded human microbiome project. Nature 550, 61–66. doi: 10.1038/nature23889
Lovett D. L., Felder D. L. (1989). Ontogeny of gut morphology in the white shrimp Penaeus setiferus (Decapoda, penaeidae). J. Morphol. 201, 253–272. doi: 10.1002/jmor.1052010305
Mangott A., Nappi J., Carini A. D. P., Goncalves P., Hua K., Domingos J. A., et al. (2020). Ulva lactuca as a functional ingredient and water bioremediator positively influences the hepatopancreas and water microbiota in the rearing of Litopenaeus vannamei. Algal Res. 51, 102040. doi: 10.1016/j.algal.2020.102040
Martínez-Córdova L. R., Emerenciano M., Miranda-Baeza A., Martínez-Porchas M. (2015). Microbial-based systems for aquaculture of fish and shrimp: an updated review. Rev. Aquacult. 7, 131–148. doi: 10.1111/raq.12058
Martínez-Porchas M., Vargas-Albores F. (2017). Microbial metagenomics in aquaculture: a potential tool for a deeper insight into the activity. Rev. Aquacult. 9, 42–56. doi: 10.1111/raq.12102
Martins M. A., Poli M. A., Legarda E. C., Pinheiro I. C., Carneiro R. F. S., Pereira S. A., et al. (2020). Heterotrophic and mature biofloc systems in the integrated culture of pacific white shrimp and Nile tilapia. Aquaculture 514, 734517. doi: 10.1016/j.aquaculture.2019.734517
Mazón-Suástegui J. M., Salas-Leiva J. S., Medina-Marrero R., Medina-García R., García-Bernal M. (2020). Effect of Streptomyces probiotics on the gut microbiota of Litopenaeus vannamei challenged with Vibrio parahaemolyticus. MicrobiologyOpen 9, e967. doi: 10.1002/mbo3.967
McKain N., Genc B., Snelling T. J., Wallace R. J. (2013). Differential recovery of bacterial and archaeal 16S rRNA genes from ruminal digesta in response to glycerol as cryoprotectant. J. Microbiol. Methods 95, 381–383. doi: 10.1016/j.mimet.2013.10.009
McLean E., Barrows F. T., Craig S. R., Alfrey K., Tran L. (2020). Complete replacement of fishmeal by soybean and poultry meals in pacific whiteleg shrimp feeds: Growth and tolerance to EMS/AHPND and WSSV challenge. Aquaculture 527, 735383. doi: 10.1016/j.aquaculture.2020.735383
McOrist A. L., Jackson M., Bird A. R. (2002). A comparison of five methods for extraction of bacterial DNA from human faecal samples. J. Microbiol. Methods 50, 131–139. doi: 10.1016/S0167-7012(02)00018-0
Moss S. M., Moss D. R., Arce S. M., Lightner D. V., Lotz J. M. (2012). The role of selective breeding and biosecurity in the prevention of disease in penaeid shrimp aquaculture. J. Invertebr. Pathol. 110, 247–250. doi: 10.1016/j.jip.2012.01.013
Nagpal S., Haque M. M., Mande S. S. (2016). Vikodak-a modular framework for inferring functional potential of microbial communities from 16S metagenomic datasets. PLoS One 11, e0148347. doi: 10.1371/journal.pone.0148347
Nayfach S., Pollard K. S. (2016). Toward accurate and quantitative comparative metagenomics. Cell 166, 1103–1116. doi: 10.1016/j.cell.2016.08.007
Nocker A., Cheung C. Y., Camper A. K. (2006). Comparison of propidium monoazide with ethidium monoazide for differentiation of live vs. dead bacteria by selective removal of DNA from dead cells. J. Microbiol. Methods 67, 310–320. doi: 10.1016/j.mimet.2006.04.015
Nocker A., Sossa-Fernandez P., Burr M. D., Camper A. K. (2007). Use of propidium monoazide for live/dead distinction in microbial ecology. Appl. Environ. Microbiol. 73, 5111–5117. doi: 10.1128/AEM.02987-06
Omont A., Elizondo-González R., Quiroz-Guzmán E., Escobedo-Fregoso C., Hernández-Herrera R., Peña-Rodríguez A. (2020). Digestive microbiota of shrimp Penaeus vannamei and oyster Crassostrea gigas co-cultured in integrated multi-trophic aquaculture system. Aquaculture 521, 735059. doi: 10.1016/j.aquaculture.2020.735059
Orosco F. L., Lluisma A. O. (2017). Variation in virome diversity in wild populations of Penaeus monodon (Fabricius 1798) with emphasis on pathogenic viruses. VirusDis 28, 262–271. doi: 10.1007/s13337-017-0389-1
Ortiz-Estrada Á.M., Gollas-Galván T., Martínez-Córdova L. R., Martínez-Porchas M. (2019). Predictive functional profiles using metagenomic 16S rRNA data: a novel approach to understanding the microbial ecology of aquaculture systems. Rev. Aquacult. 11, 234–245. doi: 10.1111/raq.12237
Parks D. H., Rinke C., Chuvochina M., Chaumeil P. A., Woodcroft B. J., Evans P. N., et al. (2017). Recovery of nearly 8,000 metagenome-assembled genomes substantially expands the tree of life. Nat. Microbiol. 2, 1533–1542. doi: 10.1038/s41564-017-0012-7
Pereira-Marques J., Hout A., Ferreira R. M., Weber M., Pinto-Ribeiro I., van Doorn L. J., et al. (2019). Impact of host DNA and sequencing depth on the taxonomic resolution of whole metagenome sequencing for microbiome analysis. Front. Microbiol. 10. doi: 10.3389/fmicb.2019.01277
Peters B. M., Jabra-Rizk M. A., O’May G. A., Costerton J. W., Shirtliff M. E. (2012). Polymicrobial interactions: impact on pathogenesis and human disease. Clin. Microbiol. Rev. 25, 193–213. doi: 10.1128/CMR.00013-11
Pillay T. V. R. (1997). Economic and social dimensions of aquaculture management. Aquac. Econ. Manage. 1, 3–11. doi: 10.1080/13657309709380199
Pilotto M. R., Goncalves A. N., Vieira F. N., Seifert W. Q., Bachère E., Rosa R. D., et al. (2018). Exploring the impact of the biofloc rearing system and an oral WSSV challenge on the intestinal bacteriome of Litopenaeus vannamei. Microorganisms 6, 83. doi: 10.3390/microorganisms6030083
Pomeroy R., Dey M. M., Plesha N. (2014). The social and economic impacts of semi-intensive aquaculture on biodiversity. Aquac. Econ. Manage. 18, 303–324. doi: 10.1080/13657305.2014.926467
Prodan A., Tremaroli V., Brolin H., Zwinderman A. H., Nieuwdorp M., Levin E. (2020). Comparing bioinformatic pipelines for microbial 16S rRNA amplicon sequencing. PLoS One 15, e0227434. doi: 10.1371/journal.pone.0227434
Pruder G. D. (2004). Biosecurity: application in aquaculture. Aquac. Eng. 32, 3–10. doi: 10.1016/j.aquaeng.2004.05.002
Qian D., Xu C., Chen C., Qin J. G., Chen L., Li E. (2020). Toxic effect of chronic waterborne copper exposure on growth, immunity, anti-oxidative capacity and gut microbiota of pacific white shrimp Litopenaeus vannamei. Fish Shellfish Immunol. 100, 445–455. doi: 10.1016/j.fsi.2020.03.018
Qiao F., Liu Y. K., Sun Y. H., Wang X. D., Chen K., Li T. Y., et al. (2017). Influence of different dietary carbohydrate sources on the growth and intestinal microbiota of Litopenaeus vannamei at low salinity. Aquac. Nutr. 23, 444–452. doi: 10.1111/anu.12412
Quince C., Walker A. W., Simpson J. T., Loman N. J., Segata N. (2017). Shotgun metagenomics, from sampling to analysis. Nat. Biotechnol. 35, 833–844. doi: 10.1038/nbt.3935
Read M. N., Holmes A. J. (2017). Towards an integrative understanding of diet–host–gut microbiome interactions. Front. Immunol. 8. doi: 10.3389/fimmu.2017.00538
Rego M. A. S., Sabbag O. J., Soares R., Peixoto S. (2017). Risk analysis of the insertion of biofloc technology in a marine shrimp Litopenaeus vannamei production in a farm in pernambuco, Brazil: A case study. Aquaculture 469, 67–71. doi: 10.1016/j.aquaculture.2016.12.006
Ringø E., Song S. K. (2016). Application of dietary supplements (synbiotics and probiotics in combination with plant products and β-glucans) in aquaculture. Aquac. Nutr. 22, 4–24. doi: 10.1111/anu.12349
Rise M. L., Martyniuk C. J., Chen M. (2019). Comparative physiology and aquaculture: Toward omics-enabled improvement of aquatic animal health and sustainable production. Comp. biochem. physiol. - d. Genom. Proteom. 31, 100603. doi: 10.1016/j.cbd.2019.100603
Rolland J. L., Stien D., Sanchez-Ferandin S., Lami R. (2016). Quorum sensing and quorum quenching in the phycosphere of phytoplankton: a case of chemical interactions in ecology. J. Chem. Ecol. 42, 1201–1211. doi: 10.1007/s10886-016-0791-y
Rosenthal M., Aiello A. E., Chenoweth C., Goldberg D., Larson E., Gloor G., et al. (2014). Impact of technical sources of variation on the hand microbiome dynamics of healthcare workers. PLoS One 9, e88999. doi: 10.1371/journal.pone.0088999
Roux S., Hallam S. J., Woyke T., Sullivan M. B. (2015). Viral dark matter and virus–host interactions resolved from publicly available microbial genomes. eLIFE 4, e08490. doi: 10.7554/eLife.08490
Rungrassamee W., Klanchui A., Maibunkaew S., Karoonuthaisiri N. (2016). Bacterial dynamics in intestines of the black tiger shrimp and the pacific white shrimp during Vibrio harveyi exposure. J. Invertebr. Pathol. 133, 12–19. doi: 10.1016/j.jip.2015.11.004
Santos H. F., Cury J. C., Carmo F. L., Rosado A. S., Peixoto R. S (2010). 18S rDNA sequences from microeukaryotes reveal oil indicators in mangrove sediment.PLoS One 5, e12437. doi: 10.1371/journal.pone.0012437
Scarfe A. D., Lee C. S., O’Bryen P. J. (2008). Aquaculture biosecurity: prevention, control, and eradication of aquatic animal disease (Iowa: John Wiley & Sons).
Scarpelli D. G., Burrows W. (2020)Disease. In: Encyclopedia Britannica. Available at: https://www.britannica.com/science/disease (Accessed April 12, 2022).
Schleder D. D., Blank M., Peruch L. G. B., Poli M. A., Goncalves P., Rosa K. V., et al. (2020). Impact of combinations of brown seaweeds on shrimp gut microbiota and response to thermal shock and white spot disease. Aquaculture 519, 734779. doi: 10.1016/j.aquaculture.2019.734779
Schloss P. D. (2021). Amplicon sequence variants artificially split bacterial genomes into separate clusters. mSphere 6, e00191–e00121. doi: 10.1128/mSphere.00191-21
Schryver P., Vadstein O. (2014). Ecological theory as a foundation to control pathogenic invasion in aquaculture. ISME J. 8, 2360–2368. doi: 10.1038/ismej.2014.84
Sergaki C., Lagunas B., Lidbury I., Gifford M. L., Schäfer P. (2018). Challenges and approaches in microbiome research: from fundamental to applied. Front. Plant Sci. 9. doi: 10.3389/fpls.2018.01205
Shao J., Wang B., Liu M., Jiang K., Wang L., Wang M. (2019). Replacement of fishmeal by fermented soybean meal could enhance the growth performance but not significantly influence the intestinal microbiota of white shrimp Litopenaeus vannamei. Aquaculture 504, 354–360. doi: 10.1016/j.aquaculture.2019.02.011
Shiu Y. L., Wong S. L., Guei W. C., Shin Y. C., Liu C. H. (2015). Increase in the plant protein ratio in the diet of white shrimp, Litopenaeus vannamei (Boone), using Bacillus subtilis E20-fermented soybean meal as a replacement. Aquac. Res. 46, 382–394. doi: 10.1111/are.12186
Silva V. F., Pereira P. K. M., Martins M. A., Lorenzo M. A. D., Cella H., Lopes R. G., et al. (2022). Effects of microalgae addition and fish feed supplementation in the integrated rearing of pacific white shrimp and Nile tilapia using biofloc technology. Anim., 12, 1527. doi: 10.3390/ani12121527
Stanton C., Ross R. P., Fitzgerald G. F., Van Sinderen D. (2005). Fermented functional foods based on probiotics and their biogenic metabolites. Curr. Opin. Biotechnol. 16, 198–203. doi: 10.1016/j.copbio.2005.02.008
Stinson L. F., Keelan J. A., Payne M. S. (2018). Comparison of meconium DNA extraction methods for use in microbiome studies. Front. Microbiol. 9. doi: 10.3389/fmicb.2018.00270
Suantika G., Situmorang M. L., Kurniawan J. B., Pratiwi S. A., Aditiawati P., Astuti D. I., et al. (2018). Development of a zero water discharge (ZWD)–recirculating aquaculture system (RAS) hybrid system for super intensive white shrimp (Litopenaeus vannamei) culture under low salinity conditions and its industrial trial in commercial shrimp urban farming in gresik, East Java, Indonesia. Aquac. Eng. 82, 12–24. doi: 10.1016/j.aquaeng.2018.04.002
Su H., Hu X., Xu Y., Xu W., Huang X., Wen G., et al. (2018). Persistence and spatial variation of antibiotic resistance genes and bacterial populations change in reared shrimp in south China. Environ. Int. 119, 327–333. doi: 10.1016/j.envint.2018.07.007
Sun D. L., Jiang X., Wu Q. L., Zhou N. Y. (2013). Intragenomic heterogeneity of 16S rRNA genes causes overestimation of prokaryotic diversity. Appl. Environ. Microbiol. 79, 5962–5969. doi: 10.1128/AEM.01282-13
Sun F., Wang Y., Wang C., Zhang L., Tu K., Zheng Z. (2019). Insights into the intestinal microbiota of several aquatic organisms and association with the surrounding environment. Aquaculture 507, 196–202. doi: 10.1016/j.aquaculture.2019.04.026
Suo Y., Li E., Li T., Jia Y., Qin J. G., Gu Z., et al. (2017). Response of gut health and microbiota to sulfide exposure in pacific white shrimp Litopenaeus vannamei. Fish Shellfish Immunol. 63, 87–96. doi: 10.1016/j.fsi.2017.02.008
Suttle C. A. (2007). Marine viruses–major players in the global ecosystem. Nat. Rev. Microbiol. 5, 801–812. doi: 10.1038/nrmicro1750
Thomas T., Gilbert J., Meyer F. (2012). Metagenomics-a guide from sampling to data analysis. Microb. Informatics. Exp. 2, 3. doi: 10.1186/2042-5783-2-3
Tinh T. H., Koppenol T., Hai T. N., Verreth J. A., Verdegem M. C. (2021). Effects of carbohydrate sources on a biofloc nursery system for whiteleg shrimp (Litopenaeus vannamei). Aquaculture 531, 735795. doi: 10.1016/j.aquaculture.2020.735795
Vargas-Albores F., Martínez-Córdova L. R., Gollas-Galván T., Garibay-Valdez E., Emerenciano M. G. C., Lago-Leston A., et al. (2019). Inferring the functional properties of bacterial communities in shrimp-culture bioflocs produced with amaranth and wheat seeds as fouler promoters. Aquaculture 500, 107–117. doi: 10.1016/j.aquaculture.2018.10.005
Vargas-Albores F., Porchas-Cornejo M. A., Martínez-Porchas M., Villalpando-Canchola E., Gollas-Galván T., Martínez-Córdova L. R. (2017). Bacterial biota of shrimp intestine is significantly modified by the use of a probiotic mixture: a high throughput sequencing approach. Helgol. Mar. Res. 71, 5. doi: 10.1186/s10152-017-0485-z
Vayssier-Taussat M., Albina E., Citti C., Cosson J. F., Jacques M. A., Lebrun M. H., et al. (2014). Shifting the paradigm from pathogens to pathobiome: new concepts in the light of meta-omics. Front. Cell. Infect. Microbiol. 4. doi: 10.3389/fcimb.2014.00029
Wang Y., Hatt J. K., Tsementzi D., Rodriguez-R L. M., Ruiz-Pérez C. A., Weigand M. R., et al. (2017). Quantifying the importance of the rare biosphere for microbial community response to organic pollutants in a freshwater ecosystem. Appl. Environ. Microbiol. 83, e03321–e03316. doi: 10.1128/AEM.03321-16
Wang H., Huang J., Wang P., Li T. (2020a). Insights into the microbiota of larval and postlarval pacific white shrimp (Penaeus vannamei) along early developmental stages: a case in pond level. Mol. Genet. Genomics 295, 1517–1528. doi: 10.1007/s00438-020-01717-2
Wang J., Huang Y., Xu K., Zhang X., Sun H., Fan L., et al. (2019b). White spot syndrome virus (WSSV) infection impacts intestinal microbiota composition and function in Litopenaeus vannamei. Fish Shellfish Immunol. 84, 130–137. doi: 10.1016/j.fsi.2018.09.076
Wang J. W., Kuo C. H., Kuo F. C., Wang Y. K., Hsu W. H., Yu F. J., et al. (2019a). Fecal microbiota transplantation: Review and update. J. Formos. Med. Assoc. 118, S23–S31. doi: 10.1016/j.jfma.2018.08.011
Wang L., Li F., Wang B., Xiang J. (2012). Structure and partial protein profiles of the peritrophic membrane (PM) from the gut of the shrimp Litopenaeus vannamei. Fish Shellfish Immunol. 33, 1285–1291. doi: 10.1016/j.fsi.2012.09.014
Wang Y., Qian, P. Y. (2009). Conservative fragments in bacterial 16S rRNA genes and primer design for 16S ribosomal DNA amplicons in metagenomic studies. PLoS One 4, e7401. doi: 10.1371/journal.pone.0007401
Wang H., Wan X., Xie G., Dong X., Wang X., Huang J. (2020b). Insights into the histopathology and microbiome of pacific white shrimp, Penaeus vannamei, suffering from white feces syndrome. Aquaculture 527, 735447. doi: 10.1016/j.aquaculture.2020.735447
Wei Y. F., Wang A. L., Liao S. A. (2020). Effect of different carbon sources on microbial community structure and composition of ex-situ biofloc formation. Aquaculture 515, 734492. doi: 10.1016/j.aquaculture.2019.734492
Wesolowska-Andersen A., Bahl M. I., Carvalho V., Kristiansen K., Sicheritz-Pontén T., Gupta R., et al. (2014). Choice of bacterial DNA extraction method from fecal material influences community structure as evaluated by metagenomic analysis. Microbiome 2, 19. doi: 10.1186/2049-2618-2-19
Whipps J. M., Lewis K., Cooke R. C. (1988). “Mycoparasitism and plant disease control,” in Fungi in biological control systems. Ed. Burge M. N. (Manchester and New York: Manchester University Press), 161–187.
Wirbel J., Zych K., Essex M., Karcher N., Kartal E., Salazar G., et al. (2021). Microbiome meta-analysis and cross-disease comparison enabled by the SIAMCAT machine learning toolbox. Genome. Biol. 22, 93. doi: 10.1186/s13059-021-02306-1
Wu H. J., Wang A. H., Jennings M. P. (2008). Discovery of virulence factors of pathogenic bacteria. Curr. Opin. Chem. Biol. 12, 93–101. doi: 10.1016/j.cbpa.2008.01.023
Xiong J., Dai W., Qiu Q., Zhu J., Yang W., Li C. (2018a). Response of host–bacterial colonization in shrimp to developmental stage, environment and disease. Mol. Ecol. 27, 3686–3699. doi: 10.1111/mec.14822
Xiong J., Dai W., Zhu J., Liu K., Dong C., Qiu Q. (2017). The underlying ecological processes of gut microbiota among cohabitating retarded, overgrown and normal shrimp. Microb. Ecol. 73, 988–999. doi: 10.1007/s00248-016-0910-x
Xiong J., Wang K., Wu J., Qiuqian L., Yang K., Qian Y., et al. (2015). Changes in intestinal bacterial communities are closely associated with shrimp disease severity. Appl. Microbiol. Biotechnol. 99, 6911–6919. doi: 10.1007/s00253-015-6632-z
Xiong J., Yu W., Dai W., Zhang J., Qiu Q., Ou C. (2018b). Quantitative prediction of shrimp disease incidence via the profiles of gut eukaryotic microbiota. Appl. Microbiol. Biotechnol. 102, 3315–3326. doi: 10.1007/s00253-018-8874-z
Xue M., Wu L., He Y., Liang H., Wen C. (2018). Biases during DNA extraction affect characterization of the microbiota associated with larvae of the pacific white shrimp, Litopenaeus vannamei. PeerJ 6, e5257. doi: 10.7717/peerj.5257
Yang F., Sun J., Luo H., Ren H., Zhou H., Lin Y., et al. (2020a). Assessment of fecal DNA extraction protocols for metagenomic studies. GigaScience 9, giaa071. doi: 10.1093/gigascience/giaa071
Yang W., Zhu J., Zheng C., Lukwambe B., Nicholaus R., Lu K., et al. (2020b). Succession of phytoplankton community during intensive shrimp (Litopenaeus vannamei) cultivation and its effects on cultivation systems. Aquaculture 520, 734733. doi: 10.1016/j.aquaculture.2019.734733
Yanong R. P., Erlacher-Reid C. (2012). Biosecurity in aquaculture, part 1: an overview (Stoneville Mississippi: Southern Regional Aquaculture Center (SRAC)). Pub. No.4707.
Yao Z., Yang K., Huang L., Huang X., Qiuqian L., Wang K., et al. (2018). Disease outbreak accompanies the dispersive structure of shrimp gut bacterial community with a simple core microbiota. AMB Expr. 8, 120. doi: 10.1186/s13568-018-0644-x
Yuan S., Cohen D. B., Ravel J., Abdo Z., Forney L. J. (2012). Evaluation of methods for the extraction and purification of DNA from the human microbiome. PLoS One 7, e33865. doi: 10.1371/journal.pone.0033865
Yu Z., Morrison M. (2004). Improved extraction of PCR-quality community DNA from digesta and fecal samples. Biotechniques 36, 808–812. doi: 10.2144/04365ST04
Yu Q., Xie J., Huang M., Chen C., Qian D., Qin J. G., et al. (2020). Growth and health responses to a long-term pH stress in pacific white shrimp Litopenaeus vannamei. Aquac. Rep. 16, 100280. doi: 10.1016/j.aqrep.2020.100280
Zaheer R., Noyes N., Ortega Polo R., Cook S. R., Marinier E., Van Domselaar G., et al. (2018). Impact of sequencing depth on the characterization of the microbiome and resistome. Sci. Rep. 8, 5890. doi: 10.1038/s41598-018-24280-8
Zhang W., Li C. (2021). Virulence mechanisms of vibrios belonging to the splendidus clade as aquaculture pathogens, from case studies and genome data. Rev. Aquacult. 13, 2004–2026. doi: 10.1111/raq.12555
Zhang X., Li X., Lu J., Qiu Q., Chen J., Xiong J. (2021b). Quantifying the importance of external and internal sources to the gut microbiota in juvenile and adult shrimp. Aquaculture 531, 735910. doi: 10.1016/j.aquaculture.2020.735910
Zhang M., Pan L., Fan D., He J., Su C., Gao S., et al. (2021a). Study of fermented feed by mixed strains and their effects on the survival, growth, digestive enzyme activity and intestinal flora of Penaeus vannamei. Aquaculture 530, 735703. doi: 10.1016/j.aquaculture.2020.735703
Zhang M., Sun Y., Liu Y., Qiao F., Chen L., Liu W.-T., et al. (2016). Response of gut microbiota to salinity change in two euryhaline aquatic animals with reverse salinity preference. Aquaculture 454, 72–80. doi: 10.1016/j.aquaculture.2015.12.014
Zhao Y., Zhang X. X., Zhao Z., Duan C., Chen H., Wang M., et al. (2018). Metagenomic analysis revealed the prevalence of antibiotic resistance genes in the gut and living environment of freshwater shrimp. J. Hazard. Mater. 350, 10–18. doi: 10.1016/j.jhazmat.2018.02.004
Zhou L., Chen C., Xie J., Xu C., Zhao Q., Qin J. G., et al. (2019a). Intestinal bacterial signatures of the “cotton shrimp-like” disease explain the change of growth performance and immune responses in pacific white shrimp (Litopenaeus vannamei). Fish Shellfish Immunol. 92, 629–636. doi: 10.1016/j.fsi.2019.06.054
Zhou Y., Jia E., Qiao Y., Shi H., Liu Z., Pan M., et al. (2021). Low bias multiple displacement amplification with confinement effect based on agarose gel. Anal. Bioanal. Chem. 413, 4397–4405. doi: 10.1007/s00216-021-03415-3
Zhou R., Zeng S., Hou D., Liu J., Weng S., He J., et al. (2019b). Occurrence of human pathogenic bacteria carrying antibiotic resistance genes revealed by metagenomic approach: A case study from an aquatic environment. J. Environ. Sci. 80, 248–256. doi: 10.1016/j.jes.2019.01.001
Keywords: litopenaeus vannamei, microbiome, intensification, biofloc, holobiome of aquaculture systems
Citation: Gutiérrez-Pérez ED, Vázquez-Juárez R, Magallón-Barajas FJ, Martínez-Mercado MÁ, Escobar-Zepeda A and Magallón-Servín P (2022) How a holobiome perspective could promote intensification, biosecurity and eco-efficiency in the shrimp aquaculture industry. Front. Mar. Sci. 9:975042. doi: 10.3389/fmars.2022.975042
Received: 21 June 2022; Accepted: 05 September 2022;
Published: 23 September 2022.
Edited by:
Bin Xia, Qingdao Agricultural University, ChinaReviewed by:
Yafei Duan, South China Sea Fisheries Research Institute, ChinaMoslem Sharifinia, Iranian Fisheries Science Research Institute, Iran
Copyright © 2022 Gutiérrez-Pérez, Vázquez-Juárez, Magallón-Barajas, Martínez-Mercado, Escobar-Zepeda and Magallón-Servín. This is an open-access article distributed under the terms of the Creative Commons Attribution License (CC BY). The use, distribution or reproduction in other forums is permitted, provided the original author(s) and the copyright owner(s) are credited and that the original publication in this journal is cited, in accordance with accepted academic practice. No use, distribution or reproduction is permitted which does not comply with these terms.
*Correspondence: Paola Magallón-Servín, cG1hZ2FsbG9uQGNpYm5vci5teA==; Ricardo Vázquez-Juárez, cnZhenF1ZXowNEBjaWJub3IubXg=