- 1Departamento de Ciencias, Facultad de Artes Liberales, Universidad Adolfo Ibañez, Santiago, Chile
- 2Instituto Milenio de Socio-Ecología Costera SECOS, Santiago, Chile
- 3Centro de Investigación e Innovación en Cambio Climático, Facultad de Ciencias, Universidad Santo Tomás, Santiago, Chile
- 4Departamento de Ecología y Biodiversidad, Facultad de Ciencias de la Vida, Universidad Andrés Bello, Santiago, Chile
- 5Centro de Investigación Marina Quintay (CIMARQ), Facultad de Ciencias de la Vida, Universidad Andrés Bello, Santiago, Chile
- 6Facultad de Ingeniería y Ciencias, Universidad Adolfo Ibáñez, Viña del Mar, Chile
- 7Departamento de Ingeniería Mecánica, Universidad de Santiago de Chile, Santiago, Chile
Warming and ocean acidification are currently critical global change drivers for marine ecosystems due to their complex and irreversible effects on the ecology and evolution of marine communities. Changes in the chemistry and the temperature of the ocean impact the biological performance of marine resources by affecting their energy budget and thus imposing energetic restrictions and trade-offs on their survival, growth, and reproduction. In this study, we evaluated the interplaying effects of increased pCO2 levels and temperature on the economically relevant clam Ameghinomya antiqua, an infaunal bivalve inhabiting a wide distributional range along the coast of Chile. Juvenile clams collected from southern Chile were exposed to a 90-day experimental set-up emulating the current and a future scenario projeced to the end of the current century for both high pCO2/low-pH and temperature (10 and 15°C) projected for the Chilean coast. Clams showed physiological plasticity to different projected environmental scenarios without mortality. In addition, our results showed that the specimens under low-pH conditions were not able to meet the energetic requirements when increased temperature imposed high maintenance costs, consequently showing metabolic depression. Indeed, although the calcification rate was negative in the high-pCO2 scenario, it was the temperature that determined the amount of shell loss. These results indicate that the studied clam can face environmental changes for short-term periods modifying energetic allocation on maintenance and growth processes, but with possible long-term population costs, endangering the sustainability of an important benthic artisanal fisheries resource.
Introduction
Small-scale fisheries and aquaculture systems currently play a critical role in providing both livelihood and food security for millions of people worldwide (Short et al., 2021). Concomitant with the rapid growth of the human population, the demand for “blue foods” (food originated in marine or freshwater systems, both captured or cultivated) as an affordable and sustainable source of protein, has experienced a significant rise in the last years (Blanchard et al., 2017; Naylor et al., 2021). However, simultaneously with the realization of the future potential of blue foods, global-scale human activities are indirectly imposing new environmental stressors on oceans, changing their physical and chemical properties, and thus affecting the ecosystem services they provide (Bindoff et al., 2019). Indeed, it is expected that these changes in the ocean’s properties, will negatively affect the biology of marine species. As some of them are important food resources, the security of the current and future supply of blue foods could be seriously threatened (Blanchard et al., 2017; Bindoff et al., 2019).
Among the main global change drivers disturbing marine ecosystems, ocean acidification (OA) and ocean warming (OW) are currently of critical concern due to their ubiquity and their irreversible and complex effects on the ecological systems (Gattuso et al., 2015; Sage, 2020). Both OA and OW are mainly a consequence of the rise in atmospheric CO2 derived from the use of fossil fuels and land-use emissions (Le Quéré et al., 2018; Doney et al., 2020). In the case of OA, oceans act to moderate the rise in atmospheric CO2 concentration by up-taking between a quarter and a third of all CO2 emissions (Parker et al., 2013; Doney et al., 2020). This oceanic uptake is translated into chemical changes in the carbonate system that, in turn, produce a decrement in pH and the concentration of carbonate ion (CO32-), affecting the shell synthesis in shell-producing organisms and the organism’s physiology (Orr et al., 2005; Doney et al., 2020; Cornwall et al., 2021; Vargas et al., 2022). On the other hand, oceans also act as regulators of atmospheric temperature having captured more than 90% of the Earth’s additional heat since the 1970s, consequently, increasing global oceanic temperature (Gattuso et al., 2015; Doney et al., 2020). Since many marine valuable species, such as blue foods, are ectothermic-calcifying organisms, the changes in temperature and the chemistry of the carbonate system will impact their biological performance and their capacity to produce their shells (Spalding et al., 2017; Leung et al., 2020). Moreover, the impact of both drivers can be critical for their survival, growth, reproduction, and phenology (Baag and Mandal, 2022).
Changes in pH and temperature are usual natural processes that can be more or less acute and periodic, especially in coastal and estuarine systems (see Vargas et al., 2017). At an individual level, both OA and OW affect the organism’s energy budget in the short term, thus having effects on all their biology and imposing intra-individual energetic constraints and trade-offs (Calosi et al., 2017; Baltar et al., 2019; Leung et al., 2020). For instance, it has been shown that calcifying species exhibit effects in several attributes including morphology, biomechanics, or behavior (Harvey et al., 2013; Kroeker et al., 2013; Barton et al., 2015; Leung et al., 2017; Lemasson and Knights, 2021; Jahnsen-Guzmán et al., 2022). However, the first and most affected traits are the physiological ones (Bozinovic and Pörtner, 2015; Doney et al., 2020). Indeed, much physiological research has been done in the last years using these two drivers individually or in combination (i.e. OA and OW) with contrasting outcomes depending on the species analyzed, suggesting these effects can be species-specific (Harvey et al., 2013; Baag and Mandal, 2022). Furthermore, OA and OW pose potential impacts on ecosystems through the combination of responses, affecting several levels of biological organization (Poore et al., 2013; Gattuso et al., 2015; Baltar et al., 2019; Lemasson and Knights, 2021; Jahnsen-Guzmán et al., 2022; Vargas et al., 2022).
Currently, there is a critical need to understand the possible synergistic or antagonistic responses of the interplay between OA and OW on socio-ecologically relevant species to predict their sustainability and future supply (Sage, 2020). For instance, in the southern hemisphere, Chile has more than 6000 km of coastline and is among the top 5 global producers of shellfish, a trend that has been supported by the rapid development of bivalve aquaculture since the 1990s (Yáñez et al., 2017). Paradoxically, the harvesting of most of the shellfish resources is still artisanal and relies on the production of natural stocks under current environmental conditions. This potential vulnerability led to recent efforts to focus on studies aimed at evaluating the effects of global change drivers on economically relevant species (Navarro et al., 2013; Duarte et al., 2014b; Osores et al., 2017; Yáñez et al., 2017; Benítez et al., 2018). Additionally, as the responses of marine species to OA, OW, and other global drivers are species-specific, there is a necessity to understand the possible biological responses of several important “blue food” species using medium and long-term experimental set-ups accounting for these possible challenges (Boyd et al., 2018).
One of the most important free-living (not cultured) marine resources in the Southern Cone of South America, and especially in Chile, is the clam Ameghinomya antiqua (King, 1832), a species inhabiting soft bottoms along the coasts of the South Pacific Ocean. This infaunal species (Clements and Hunt, 2017) exhibits extremely high calcification rates given the thickness and resistance of its shell compared with other species of bivalves, which has even allowed shell preservation through thousands of years (Clasing et al., 1994; Gordillo et al., 2008; Watson et al., 2017). These features, together with ecological value, make A. antiqua an excellent biological model to study the interplaying effect of OA and OW. Even though the economic value of A. antiqua is not so elevated, this species is relevant for artisanal fisheries, and its study has been promoted by governmental offices to improve the culture of this species under field and hatchery conditions (SERNAPESCA, 2021). Indeed, it is recognized as an ecologically and economically relevant species on the coast of Chile (Miranda and Stotz, 2021). Nevertheless, their marked energy expenditure in juvenile stages (Stead and Clasing, 1997) and their high somatic growth compared with morphologically similar bivalves (Clasing et al., 1994) can be affected by the new environmental conditions that projected OA and OW conditions will impose. Considering the observed strong seasonal pattern of growth in A. antiqua and the minimum size of the capture of this species (55 mm), any factor affecting their energy balance will impact not only their ecology but will also affect the annual fisheries activities associated with the species, thus scaling up to economic and social levels.
In this study, we evaluated, from an ecophysiological perspective, the interplaying effects of acidification and temperature on the infaunal clam A. antiqua. We exposed clam juvenile specimens (≤32 mm) to a 90-day experimental mesocosm set-up emulating the current and a projected future scenario of increased pCO2 and temperature levels. Due to the high energy demand to produce shells under acidic or high-pCO2 conditions, shell production could not occur if an increment in temperature imposed extra costs on the organism maintenance. Thus, the interaction between pH/pCO2 and temperature would result in an energetic trade-off between maintenance and growth, affecting its ecology and its associated fisheries.
Materials and methods
Animal sampling and mesocosm set-up
Juvenile specimens of the clam A. antiqua (≤32 mm) were collected at the beginning of the austral spring of 2019 from the soft bottom at Coihuin tidal flat, southern Chile (41°29’ S; 72°54’ W), where the mean water temperature is ca. 9°C during winter (Urrutia et al., 2001; Alarcón et al., 2015). The specimens were transported to the Bioengineering laboratory at Adolfo Ibáñez University (Santiago, Chile), in wet conditions and acclimatized for 10 days in a “common garden” aquarium with controlled environmental conditions (T° = 10°C, pH = 7.9, salinity ~30 PSU) to avoid any stressful response generated by the transport process. After this time, each individual was marked with non-toxic bee markers to identify and recognize possible deaths during the experiment. Groups of five specimens were randomly assigned to 9 L aquariums filled up to a third of its volume with quartz sand (0.4 – 0.6 mm) and the rest with filtered and UV-sterilized seawater (salinity ~30 PSU), to simulate their natural environmental conditions. As clams are common infaunal species, thus showing burial behavior (Lardies et al., 2001), and because the sediment from their natural habitat can change the chemical properties of the surrounding water (e.g., pH, alkalinity, see Brenner et al. (2016), quartz sand, an inert material, was used to avoid any possible effect of the substrate on the experimental set-up, previous evaluation of ΔpH(in situ) inside and outside the quartz (ΔpH range = 0 – 0.03 for the four treatments, unpublished data). Thus, each aquarium was assigned to one of four treatments (n = 4 aquariums per treatment, 80 specimens in total) in an orthogonal design simulating a current and future acidification scenario (pH = 7.9 and 7.5 respectively) and current and ocean warming conditions projected for the coast of central-southern Chile (T° = 10 and 15°C, respectively, see Duarte et al., 2022). To recreate a realistic scenario (Asnicar and Marin, 2022) the environmental change (T° and pH) was calculated to the end of the current century (2100) based on the current conditions near the study site. For the case of temperature, current values measured in the austral winter months can achieve ~13°C following Jahnsen-Guzmán et al. (2021), so ~1.5°C was added following the projections of the RCP2.6 under low CO2 emissions (see Gattuso et al., 2015). For the case of pH, we follow previous works on marine invertebrates, in part summarized in Vargas et al. (2017), using the highest pCO2 condition, accounting for data obtained near the study site where values ~1500 µatm have been registered already. During the experimental period, clams were fed daily with Reef Blizzard-O (Brightwell®Aquatics) suspension, calculating approximately 5% of the buoyant body weight to ensure ad libitum food. Every aquarium was monitored daily to account for mortality (no replacement in case of death). Seawater was renewed carefully every two days. The total duration of the experiment was 90 days.
Seawater pCO2 and temperature manipulation
To obtain the two levels of the pH treatment (7.9 and 7.5, see above), a mix of dry air with pure CO2 (partial pressure of CO2 [pCO2] = 500 and 1500 μatm respectively) was added to each aquarium containing seawater in a continuous regular flux using mass flow controllers (Aalborg Instruments & Controls, Inc., Orangeburg, NY, USA) following Torres et al. (2013). Measurements of pH, temperature, and salinity were obtained every three days to ensure the stability of experimental conditions. The pH (NBS scale) was measured using a pH-meter (Mobile 826, Metrohm, Herisau, Switzerland), connected to a combined electrode (double juncture), and calibrated using Metrohm buffers (pH = 4.0, 7.0, and 9.0) at 25°C in a temperature-controlled water bath. Salinity (PSU) and temperature (°C) were monitored daily during the experimental period using a portable Salinometer (Salt6+, Oakton; accuracy: ± 0.1 PSU and ± 0.5°C, respectively). Additionally, total alkalinity (AT) of the water was sampled every 3 days using 50 mL of seawater from each aquarium fixed with a saturated solution of HgCl2 and stored in 250 ml polypropylene bottles in the dark at room temperature until the analysis in the laboratory at the end of the experiment using potentiometric titration (Titrando 888, Metrohm, Herisau, Switzerland), in accordance with Torres et al. (2013). Temperature, salinity, pH, and AT data were used to calculate the carbonate system parameters shown in Table 1 (partial pressure of CO2 [pCO2] and saturation states of calcite [Ωca] and aragonite [Ωar]). Analyses were performed using CO2SYS software in MS Excel (Pierrot et al., 2006) set with Mehrbach solubility constants and refitted by Dickson and Millero (1987).
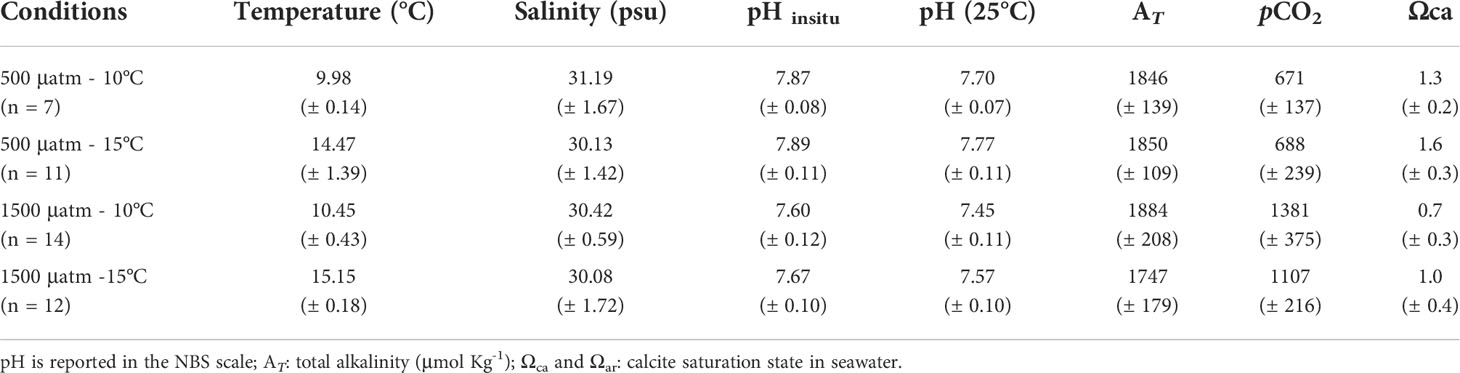
Table 1 Average conditions (± SE) of carbonate system parameters for each experimental treatment: pCO2 (500 and 1500 μatm) and temperature (10 and 15°C).
Biological traits measurements
To obtain a measure of growth rate and morphological changes during the experiment, buoyant weight, length, width, and height of each experimental individual were measured using an electronic caliper (Mitutoyo, Sakado, Japan). Buoyant weight, a non-invasive estimator of calcification rate and thus mollusc growth (Palmer, 1982; Duarte et al., 2014a; Lardies et al., 2017) was measured at the beginning and at the end of the experiment using an analytical balance (± 0.1 mg, AUX 220, Shimadzu, Kioto, Japan) with the whole animal immersed in seawater and under air conditions.
At the end of the experiment (after 90 days), metabolic rate was measured in the same conditions of each acclimation treatment to obtain a value of energy expenditure in each environment. To estimate the clams’ metabolic rate for each treatment, clams were previously left in fasting conditions for 24h. Measurement of oxygen consumption (mgO2 h− 1 ind− 1) was obtained using glass respirometry chambers (804 mL) with a PreSens Mini Oxy-4 respirometer (PreSens GmbH, Regensburg, Germany). The quantification of the standard metabolic rate was measured for at least 2 individuals randomly selected from each aquarium (n ≥ 10). Respirometric chambers were filled with seawater at ~30 PSU. The dissolved oxygen was quantified every 15 s for a period of ~ 1 h. The sensors were previously calibrated in anoxic water, using a Na2SO3 solution for 0% oxygen and water saturated with air bubbles for 100% oxygen. The obtained measurement was standardized per gram of weight to posterior comparisons (no weight differences between treatments were previously evaluated by diagnostic analyses). Additionally, in a unique aquarium per treatment 2 to 3 empty valves were left alone to obtain an approximate the net dissolution rate for each experimental treatment without biological regulation.
Statistical analyses
First, basic visual diagnostics to evaluate normality and homoscedasticity of each measured trait were performed, followed by regular linear models that were run to explore the data. Subsequently, and following the data structure (individuals sharing aquariums), we employed hierarchical generalized linear mixed models (GLMM) to evaluate changes in physiological (growth and metabolic rate) and morphological (length, width, and height) traits, including pCO2 (pH) and temperature levels as fixed factors, and using the ‘aquarium’ as the replicate unit (n = 4). As the individuals sharing the same aquarium could be influenced by its condition, the individuals were nested within each ‘aquarium’ as a random factor. To estimate the explanatory importance of fixed effects, we estimated the relative support of hierarchical models with versus without specific fixed factors, including their interaction, employing Akaike Information Criterion for small samples (AICc) and Akaike weights (wi), and assessed their statistical significance with an F-ratio test when necessary. In addition, we used the “lmerTest” package (Kuznetsova et al., 2017) to obtain approximated d.f, F-values, and p-values for the selected model using the Satterthwaite’s method due to “the exact null distributions for the parameter estimates and test statistics are unknown” (Kuznetsova et al., 2017). All data were analyzed using the R statistical environment (R Core Team, 2020) using the package “lme4” (Bates et al., 2015) and “performance” (Lüdecke et al., 2021). The hierarchical models obtained for the two kinds of variables (physiological and morphological) are listed in Tables 2, 3.
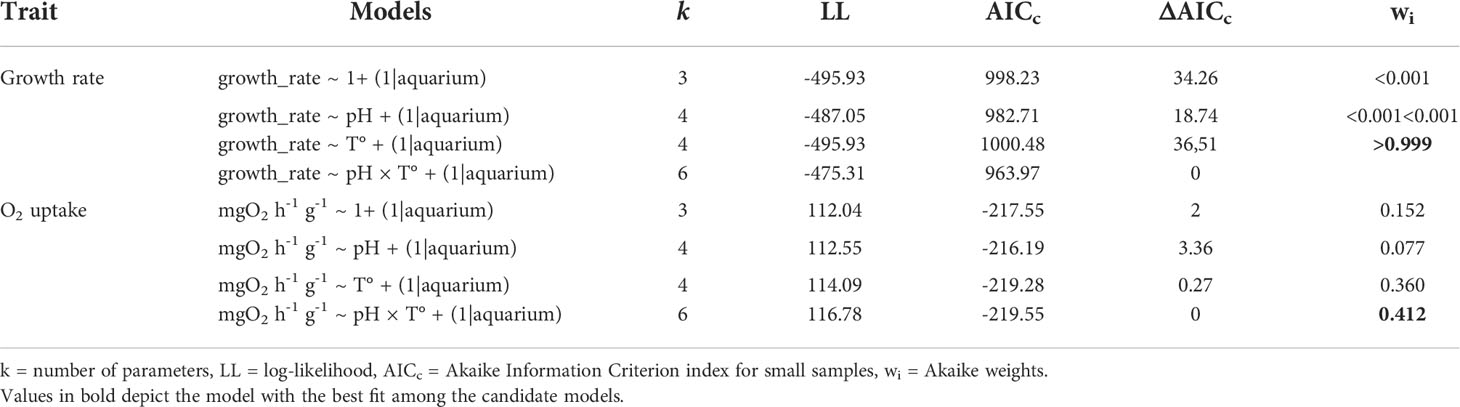
Table 2 Hierarchical general linear mixed models for physiological traits of Ameghinomya antiqua juvenile clams.
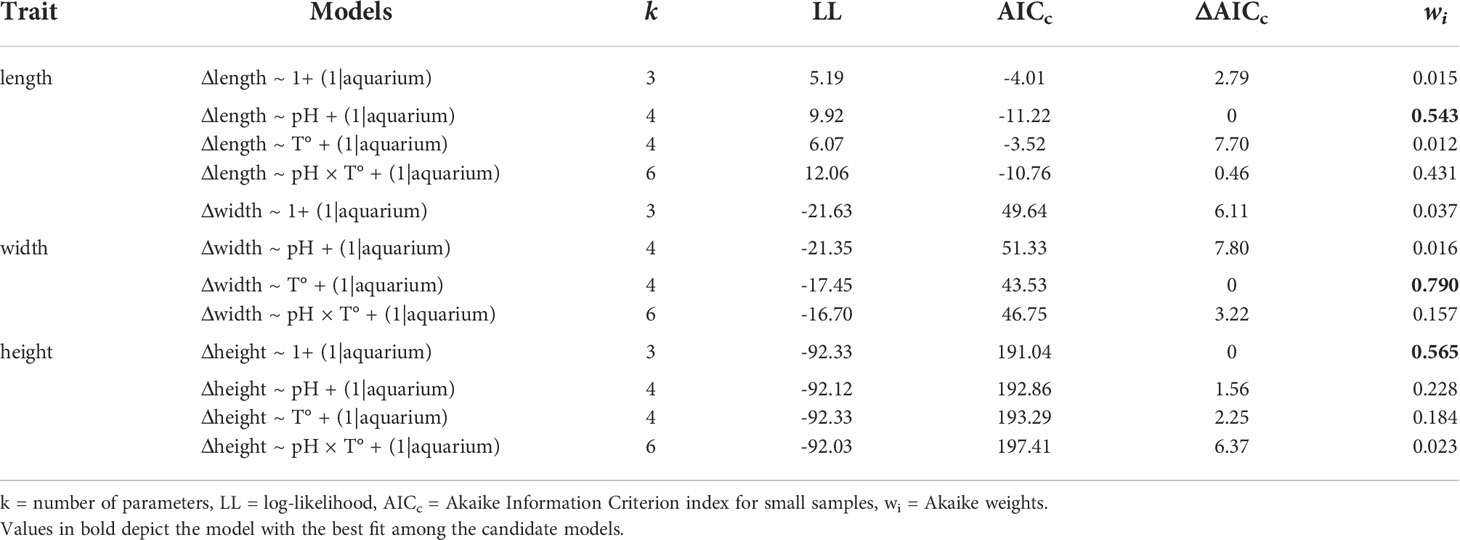
Table 3 Hierarchical general linear mixed models for morphological changes of Ameghinomya antiqua juvenile clams.
Results
During the experimental period, all of the four treatments were quite stable in their set parameters with low variations in pH and temperature (see Table 1). After the experimental period, shell dissolution recorded for empty shells of A. antiqua showed a higher rate in the high pCO2/low-pH treatment compared with the control (low pCO2/high-pH) treatment, regardless of the water temperature ( = 264.92 µg d-1, SE = 97.28, N = 7, at pCO2 = 1500 μatm; = 170.92 µg d-1, SE = 27.23, N = 6, at pCO2 = 500 μatm, Figure 1A). Concomitantly, the net calcification (changes in buoyant weight) increased in living A. antiqua clams exposed to the control pCO2/pH and high temperature treatment (pCO2 = 500 μatm, T° = 15°C, Figure 1B). However, decalcification was evident for individuals exposed to acidification conditions (pCO2 = 1500 μatm) regardless of the temperature (Figure 1B). Nevertheless, the higher the temperature, the greater the shell loss, as indicates in the GLMM model, including the interaction between factors (ΔAICc = -18.74, wi >0.999; d.f. = 69, F = 49.12, p< 0.001; Figure 1B, Table 2). Interestingly, accounting for the fact that the weight loss is for two valves per individual, the total weight loss (decalcification) in the case of the empty valve (Figure 1A), would be duplicated in living individuals, something that can be appreciated comparing the values from Figures 1A, B, as buoyant weight is directly related with the calcification (or decalcification) rate (see Duarte et al., 2014a). Finally, the interplaying effect of temperature and acidification upon the metabolic rate indicates that the oxygen uptake of clams was similar in a non-acidified environment regardless of the temperature. However, oxygen uptake increased significantly under acidified conditions at the control temperatures, but decreased again in the high temperature treatment (Figure 1C, pCO2 = 1500 μatm, T° = 15°C treatment). This is supported by the GLMM, including the interaction between pCO2 × T° (ΔAICc = -0.27, wi = 0.412; d.f. = 49, F = 4.95, p = 0.030; Table 2). Regarding the morphological adjustment of studied clams, the best fitted GLMM explaining the change in each of the three measured traits (length, width and height) was case-dependent: pH in the case of length (d.f. = 15.67, F = 12.93, p = 0.002), T° for width (d.f. = 16.62, F = 10.58, p = 0.004) and no attributable effect of the studied variables for height (Figure 2, GLMM parameters in Table 3).
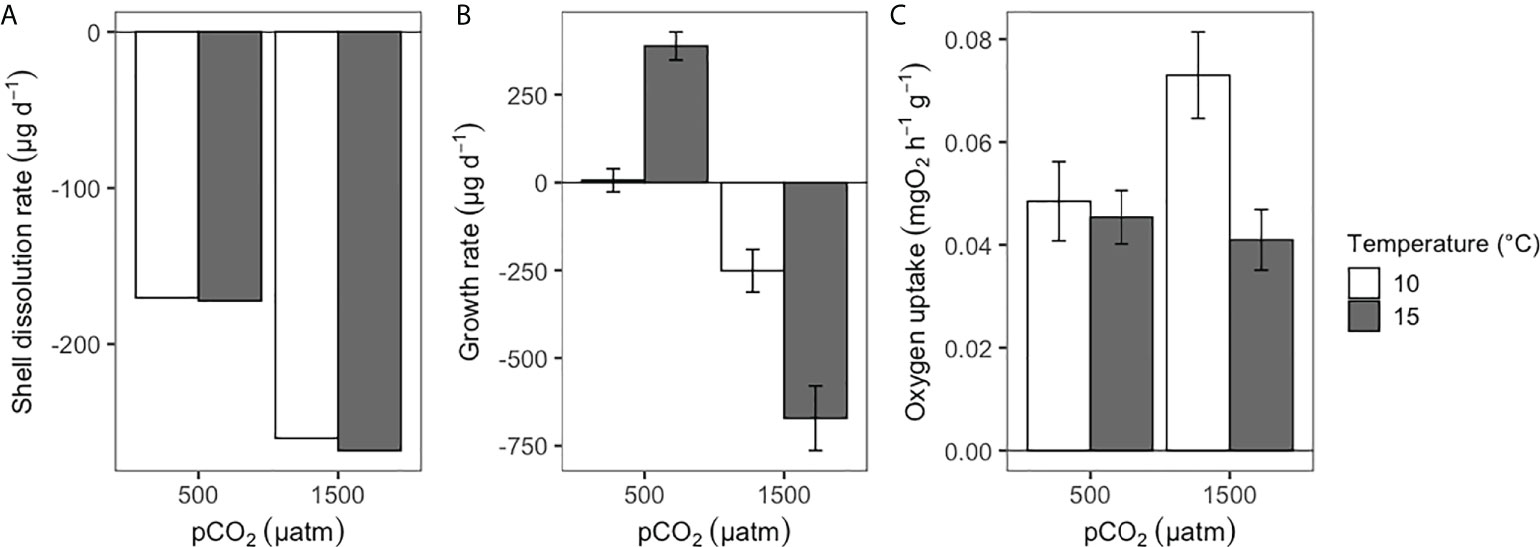
Figure 1 Ameghinomya antiqua: Shell dissolution rate (A) and physiological adjustments including growth rate (calcification rate, (B), and metabolic rate as oxygen uptake (C) for clams in each experimental condition.
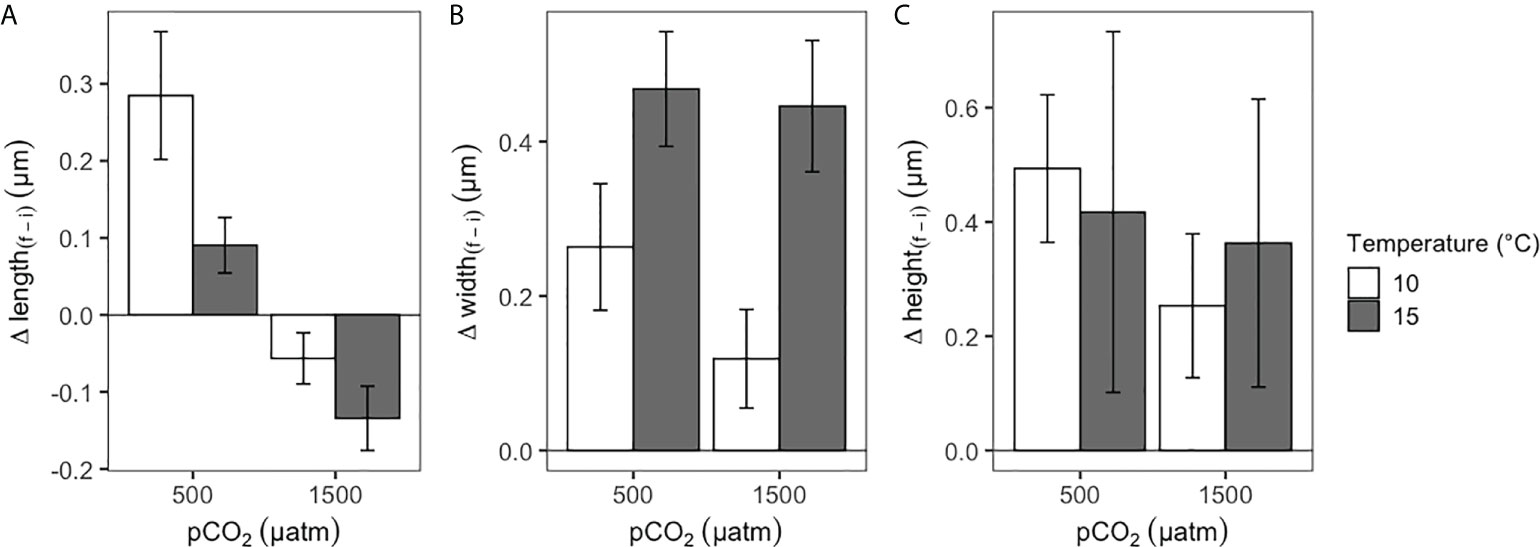
Figure 2 Morphological changes in (A) length, (B) width and (C) height of Ameghinomya antiqua after 90 days of experimental conditions.
Discussion
The combined results obtained in the present work suggest an energetic constraint to growth in the clam A. antiqua after experiencing the combined effects of acidification and a temperature of 5°C above the mean conditions experienced in its natural environment (see Urrutia et al., 2001; Alarcón et al., 2015, and materials and methods). As a consequence, limited available energy (i.e. metabolic depression) was translated into negative growth (i.e. shell loss by decalcification, = -671.52 µg d-1; SE = 92.26, Figure 1A). Whereas a lower level of shell loss, but associated with high metabolic rates, was exhibited by clams exposed to the same acidification at current temperatures, suggesting their attempts to achieve the energetic requirements of maintenance in this bivalve ( = -251.17 µg d-1, SE = 60.8, Figure 1B). Nevertheless, despite the negative energetic balance, all the experimental individuals were able to survive regardless of the environmental conditions after 90 days of experimental conditions.
Energy acquisition and allocation is a critical process for organisms, determining their survival, growth, reproduction, and the performance of any biological function (Weiner, 1992; Pörtner and Farrell, 2008; Bartheld et al., 2015). Hence, the efficiency of organisms to manage their energy budget will allow their persistence under current, novel, and changing environments (Bozinovic and Pörtner, 2015). In this context, the effect of ocean acidification producing decalcification on bivalve shells without biological regulation is depicted in Figure 1A. However, individuals exposed to the high pCO2/low-pH and current temperature treatment (pCO2 = 500 µatm, T° = 10°C) showed shell growth. Nevertheless, the capacity to produce shell decreases with acidification, a trend that can be exacerbated by increased temperature. This would suggest an incapacity to gather enough energy per unit of time to achieve maintenance demands, and thus reducing the rate of oxygen uptake in individuals experiencing a high energetic demand under these environmental conditions. Thus, in undersaturated conditions for calcite (Ωca< 1, see Table 1), it is expected that the mineral phase of the clam shell would be eroded, and hence more energy would be required to maintain shell integrity and functionality (e.g Fitzer et al., 2015). Indeed, morphological changes showed a relatively low total amount of change in size compared with changes in buoyant weight. Indeed, the loss in buoyant weight experienced by individuals in low pH conditions can be translated into important changes in shell density that cannot be correlated with morphological changes, an aspect previously reported (Lagos et al., 2021). However, although warming may diminish the negative impact of acidification on calcification in some bivalve species (Byrne et al., 2011; Lardies et al., 2017), we found that metabolic depression, and probably the low capacity of this clam to compensate for disturbances in extracellular pH, could explain the reduction in growth and calcification (see Pörtner and Farrell, 2008).
Indeed, the population of A. antiqua used here inhabits a wide tidal flat located in southern Chile that experiences extreme environmental variability on a daily basis (Lardies et al., 2001; Urrutia et al., 2001). Nevertheless, its burial behavior (Lardies et al., 2001) and the stable micro-environmental ge, possibly explaining the lack of strong physiological conditions in the mud where infauna lives (Clements and Hunt, 2017), can be enough to avoid extreme environmental change, possibly explaining the lack of strong physiological strategies to deal with sustained and stable environmental changes.
In this context, aerobically challenging biological functions, in addition with environmental conditions, can profoundly affect an organism’s energy budget since the energy allocated to one function cannot be transferred to other ones (Weiner, 1992; Hendriks et al., 2015). The biological function of calcification is a chemical reaction that does not only rely on the availability and concentration of its components in the surrounding environment (CO32- and Ca+). On the contrary, calcifying organisms regulate their intra- and extra-cellular environment to reduce their dependence on external chemical conditions (Reipschläger and Pörtner, 1996; Hendriks et al., 2015; Spalding et al., 2017). This capacity is energetically demanding and involves a chemical alteration of the surrounding seawater by ion pumping at a biochemical level (Palmer, 1992; Spalding et al., 2017). In addition, synthesizing the organic matrix covering the inorganic shell elevates the total energetic cost of the CaCO3 precipitation, resulting in high energetic costs that account for 10 to 60% of the energy allocated to somatic growth (see Palmer, 1992). Ocean acidification is characterized by changes in the availability of carbonate ions, thus, representing an additional cost for calcifying organisms to achieve the required concentrations of it to produce shells.
The biological responses of calcifying organisms experimentally exposed to high pCO2 conditions (i.e. low pH) and/or high temperatures are mostly negative (Pörtner et al., 2004; Jakubowska and Normant-Saremba, 2015). Indeed, the biological effects are variable and usually complex, and seem to be mostly specie-specific. For instance, decreases and increases in metabolic rate, or alteration in other functions such as calcification, development, and reproduction have been reported (Parker et al., 2013). In bivalve species, in terms of energetics, Benítez et al. (2018) found an elevated metabolic rate in the bivalve Choromytilus chorus, another species inhabiting Chile’s southern coast, without changes in any other trait after being exposed to high pCO2 levels in a medium-term laboratory experiment (30 days of duration). These results were in accordance with the work published by Navarro et al. (2022) in a long-term experiment (6 months) using the snail Concholepas concho Lepas, where significant effects on physiological variables including oxygen uptake were registered. Moreover, Duarte et al. (2014a) and Navarro et al. (2016) showed a reduced growth rate and reduced energy expenditure for Mytilus chilensis after the exposition to high pCO2 values, and the same for Mytilus galloprovincialis (Michaelidis et al., 2005). However, as a rule of thumb, the capacity of organisms to face environmental stress seems to go together with their capacity to manage their energy budget including food acquisition, physiological constraints, thermal breadth, and probably most important, metabolic scope (Bozinovic and Pörtner, 2015; Verberk et al., 2016). As an example, Thomsen et al. (2013) showed that M. edulis may be able to cope with high pCO2 levels when food supply is ensured.
Marine calcifying organisms contemplate two alternatives for energy expenditure depending on their metabolic response to hypercapnia (Jakubowska and Normant-Saremba, 2015). On one hand, the metabolic rate can increase with decreasing pH, possibly as compensation due to the additional cost of maintaining an extracellular acid-base regulation and the overexpression of calcifying enzymes. This response is translated into a high resilience to acidification but contemplates an energetic trade-off for other functions (Parker et al., 2013). However, on the other hand, for other species, a significant decrease in oxygen uptake occurs with decreasing pH, explained in principle by the same extracellular acid-base regulation, but this time related with a poor capacity to compensate it (Reipschläger and Pörtner, 1996; Michaelidis et al., 2005; Parker et al., 2013). This response, namely metabolic depression, has been explained as a short-term strategy to reduce the energy expenditure to the minimum when organisms are facing adverse environmental conditions (Guppy and Withers, 1999). In this way, the individuals can extend their survival during a challenging period by allocating most of their energy to maintenance, but with a generalized trade-off of other functions. For the case of the present study, only clams exposed to high pCO2 levels combined with a high temperature (i.e. pCO2 = 1500 μatm, T° = 15°C) showed metabolic depression, without mortality, but with a severe cost of the extensive dissolution of their shells at a high rate (Figure 2). In other words, their capacity to allocate the available energy was not enough to compensate for the challenge. In agreement with that, at the same low pH but at current temperature (10°C), the energy budget is almost sufficient to maintain the shell (tissue production), but with no growth.
Previously, metabolic increase and depression have been related to the expression of the Na+/K+ATPase, an enzyme that can consume up to 77% of the metabolic rate in the larvae of sea urchins (Leong and Manahan, 1997; Stumpp et al., 2011; Jakubowska and Normant-Saremba, 2015). Thus, these physiological responses can be seen as an adaptative strategy allowing short- and medium-term survival of calcifying species in their natural conditions where environmental variation is common (Vargas et al., 2017). This physiological output has been seen in several studies in different species of marine invertebrates and in response to both environmental factors (Pörtner et al., 2004; Lannig et al., 2010; Parker et al., 2013). For example, on the coast of southern Chile, Navarro et al. (2013) reported metabolic depression in the mussel M. chilensis, another economically relevant species inhabiting the region, after its exposure to high pCO2 levels.
Quantification and modelling of the energetic demands of organisms under predicted stressful environments can help us to understand the effects of environmental change on species and ecosystem functioning (Kooijman, 2010). For instance, using both laboratory and field data on natural seasonal variability in the field, Maynou et al. (2020) developed a theoretical dynamic energy budget model for the clam Ruditapes philippinarum, which can be used to predict life-history traits such as growth or reproduction considering its environmental conditions (e.g., OA and OW). They show that although higher growth rates are expected as the temperature increases, the acidification would have a deleterious effect on growth (decrements of 2 to 5% in weight or length) and a reduction in the reproductive potential in acidified conditions (pH< 7.7). Nevertheless, this model suggests that temperature increases could compensate tissue and function losses due to acidification. However, this model did not include the possibility of metabolic depression. Contrastingly, our experimental data shows metabolic depression in A. antiqua making the possible biological compensation more complex. This is the first study reporting this physiological response in this clam species after being exposed to stressful conditions (but see Han et al., 2008; Range et al., 2014; Xu et al., 2016 for other clam species).
On a broader scale, the specific metabolic depression response of A. antiqua to acidification could affect the harvestable biomass and the maximum sustainable yield of this species, impacting the social and economic interests of the country. Accounting for the massive extractive activities associated with this species (2,964 Mt by 219 ships dedicated to its recollection, only in the first trimester of 2021 in the locality of this study, SERNAPESCA, 2021), the commercial size above 55 mm, and considering a loss between 2-5% of the length, following the model developed by Maynou et al. (2020), the harvest loss could be translated into important economic losses per year. Indeed, since it is expected that this trend (elevated OA and OW) will only increase in the future, there is a call to refine the public policies and evaluate the species-specific response to global change drivers as the blue foods will become essential to meet the future demands of proteins and the global nutritional security (Troell et al., 2014; Gattuso et al., 2015; Brodie Rudolph et al., 2020; Lubchenco et al., 2020; Tittensor et al., 2021; Ward et al., 2022).
Data availability statement
The raw data supporting the conclusions of this article will be made available by the authors, without undue reservation.
Author contributions
ML, CD, and NL conceived the ideas and designed the methodology; ML, CF, and NL collected the data; SM analyzed the data; SM and ML wrote the first draft and led the writing of the manuscript. All authors contributed to the article and approved the submitted version.
Funding
This study was funded by PIA ANID ACT 172037 for international collaborative research among Chile (NAL, MAL, JV, CG-H, CD). Authors also acknowledge support from FONDECYT 1190444 (MAL), and ANID – Millennium Science Initiative Program – ICN2019_015 (SECOS) (NAL and MAL).
Acknowledgments
We thank J Lagos for his laboratory assistance and technical support.
Conflict of interest
The authors declare that the research was conducted in the absence of any commercial or financial relationships that could be construed as a potential conflict of interest.
Publisher’s note
All claims expressed in this article are solely those of the authors and do not necessarily represent those of their affiliated organizations, or those of the publisher, the editors and the reviewers. Any product that may be evaluated in this article, or claim that may be made by its manufacturer, is not guaranteed or endorsed by the publisher.
References
Alarcón E., Valdeíz N., Torres R. (2015). Calcium carbonate saturation state in an area of mussels culture in the Reloncavií sound, northern Patagonia, Chile. Latin Americamn J. Aquat. Resesarch 43 (2), 277–281. doi: 10.3856/vol43-issue2-fulltext-1
Asnicar D., Marin M. G. (2022). Effects of seawater acidification on echinoid adult stage: A review. J. Mar. Sci. Eng. 10, 477. doi: 10.3390/jmse10040477
Baag S., Mandal S. (2022). Combined effects of ocean warming and acidification on marine fish and shellfish: A molecule to ecosystem perspective. Sci. Total Environ. 802, 149807. doi: 10.1016/j.scitotenv.2021.149807
Baltar F., Bayer B., Bednarsek N., Deppeler S., Escribano R., Gonzalez C. E., et al. (2019). Towards integrating evolution, metabolism, and climate change studies of marine ecosystems. Trends Ecol. Evol. 34, 1022–1033. doi: 10.1016/j.tree.2019.07.003
Bartheld J. L., Gaitán-Espitia J. D., Artacho P., Salgado-Luarte C., Gianoli E., Nespolo R. F. (2015). Energy expenditure and body size are targets of natural selection across a wide geographic range, in a terrestrial invertebrate. Funct. Ecol. 29, 1463–1474. doi: 10.1111/1365-2435.12451
Barton A., Waldbusser G. G., Feely R. A., Weisberg S. B., Newton J. A., Hales B., et al. (2015). Impacts of coastal acidification on the pacific Northwest shellfish industry and adaptation strategies implemented in response. Oceanography 28, 146–159. doi: 10.5670/oceanog.2015.38
Bates D., Mächler M., Bolker B., Walker S. (2015). Fitting linear mixed effects models using lme4. Journal of Statistical Software 67, 1–48. doi: 10.18637/jss.v067.i01
Benítez S., Lagos N. A., Osores S., Opitz T., Duarte C., Navarro J. M., et al. (2018). High pCO2 levels affect metabolic rate, but not feeding behavior and fitness, of farmed giant mussel ChoroMytilus chorus. Aquaculture Environ. Interact. 10, 267–278. doi: 10.3354/aei00271
Bindoff N. L., Cheung W. W., Kairo J. G., Arístegui J., Guinder V. A., Hallberg R., et al. (2019). Changing ocean, marine ecosystems, and dependent communities. IPCC special Rep. ocean cryosphere changing Climate 477–587.
Blanchard J. L., Watson R. A., Fulton E. A., Cottrell R. S., Nash K. L., Bryndum-Buchholz A., et al. (2017). Linked sustainability challenges and trade-offs among fisheries, aquaculture and agriculture. Nat. Ecol. Evol. 1, 1240–1249. doi: 10.1038/s41559-017-0258-8
Boyd P. W., Collins S., Dupont S., Fabricius K., Gattuso J.-P., Havenhand J., et al. (2018). Experimental strategies to assess the biological ramifications of multiple drivers of global ocean change–a review. Global Change Biol. 24, 2239–2261. doi: 10.1111/gcb.14102
Bozinovic F., Pörtner H.-O. (2015). Physiological ecology meets climate change. Ecol. Evol. 5, 1025–1030. doi: 10.1002/ece3.1403
Brenner H., Braeckman U., Le Guitton M., Meysman F. (2016). The impact of sedimentary alkalinity release on the water column CO2 system in the north Sea. Biogeosciences 13, 841–863. doi: 10.5194/bg-13-841-2016
Brodie Rudolph T., Ruckelshaus M., Swilling M., Allison E. H., Österblom H., Gelcich S., et al. (2020). A transition to sustainable ocean governance. Nat. Commun. 11, 3600. doi: 10.1038/s41467-020-17410-2
Byrne M., Ho M., Wong E., Soars N. A., Selvakumaraswamy P., Shepard-Brennand H., et al. (2011). Unshelled abalone and corrupted urchins: development of marine calcifiers in a changing ocean. Proc. R. Soc. B: Biol. Sci. 278, 2376–2383. doi: 10.1098/rspb.2010.2404
Calosi P., Melatunan S., Turner L. M., Artioli Y., Davidson R. L., Byrne J. J., et al. (2017). Regional adaptation defines sensitivity to future ocean acidification. Nat. Commun. 8, 13994. doi: 10.1038/ncomms13994
Clasing E., Brey T., Stead R., Navarro J., Asencio G. (1994). Population dynamics of Venus antiqua (Bivalvia: Veneracea) in the bahía de yaldad, isla de chiloé, southern Chile. J. Exp. Mar. Biol. Ecol. 177, 171–186. doi: 10.1016/0022-0981(94)90235-6
Clements J. C., Hunt H. L. (2017). Effects of CO2-driven sediment acidification on infaunal marine bivalves: A synthesis. Mar. Pollut. Bull. 117, 6–16. doi: 10.1016/j.marpolbul.2017.01.053
Cornwall C. E., Comeau S., Kornder N. A., Perry C. T., Van Hooidonk R., Decarlo T. M., et al. (2021). Global declines in coral reef calcium carbonate production under ocean acidification and warming. Proc. Natl. Acad. Sci. 118 (21), e2015265118. doi: 10.1073/pnas.2015265118
Dickson A., Millero F. J. (1987). A comparison of the equilibrium constants for the dissociation of carbonic acid in seawater media. Deep Sea Res. Part A. Oceanographic Res. Papers 34, 1733–1743. doi: 10.1016/0198-0149(87)90021-5
Doney S. C., Busch D. S., Cooley S. R., Kroeker K. J. (2020). The impacts of ocean acidification on marine ecosystems and reliant human communities. Annu. Rev. Environ. Resour. 45, 83–112. doi: 10.1146/annurev-environ-012320-083019
Duarte C., Jahnsen-Guzmán N., Quijón P. A., Manríquez P. H., Lardies M. A., Fernández C., et al. (2022). Morphological, physiological and behavioral responses of an intertidal snail, Acanthina monodon (Pallas), to projected ocean acidification and cooling water conditions in upwelling ecosystems. Environ. pollut. 293, 118481. doi: 10.1016/j.envpol.2021.118481
Duarte C., Navarro J., Acuña K., Torres R., Manríquez P., Lardies M., et al. (2014a). Combined effects of temperature and ocean acidification on the juvenile individuals of the Mussel Mytilus chilensis. J. Sea Res. 85, 308–314. doi: 10.1016/j.seares.2013.06.002
Duarte C., Navarro J., Acuña K., Torres R., Manríquez P., Lardies M., et al. (2014b). Intraspecific variability in the response of the edible mussel Mytilus chilensis (Hupe) to ocean acidification. Estuaries Coasts 38, 590–598. doi: 10.1007/s12237-014-9845-y
Fitzer S. C., Zhu W., Tanner K. E., Phoenix V. R., Kamenos N. A., Cusack M. (2015). Ocean acidification alters the material properties of Mytilus edulis shells. J. R. Soc. Interface 12, 20141227. doi: 10.1098/rsif.2014.1227
Gattuso J.-P., Magnan A., Billé R., Cheung W. W. L., Howes E. L., Joos F., et al. (2015). Contrasting futures for ocean and society from different anthropogenic CO2 emissions scenarios. Science 349, aac4722. doi: 10.1126/science.aac4722
Gordillo S., Rabassa J., Coronato A. (2008). Paleoecology and paleobiogeographic patterns of mid-Holocene mollusks from the Beagle channel (southern tierra del fuego, Argentina). Rev. Geologica Chile 35, 321.333. doi: 10.5027/andgeoV35n2-a07
Guppy M., Withers P. (1999). Metabolic depression in animals: physiological perspectives and biochemical generalizations. Biol. Rev. Camb Philos. Soc. 74, 1–40. doi: 10.1017/S0006323198005258
Han K. N., Lee S. W., Wang S. Y. (2008). The effect of temperature on the energy budget of the Manila clam, Ruditapes philippinarum. Aquaculture Int. 16, 143–152. doi: 10.1007/s10499-007-9133-y
Harvey B. P., Gwynn-Jones D., Moore P. J. (2013). Meta-analysis reveals complex marine biological responses to the interactive effects of ocean acidification and warming. Ecol. Evol. 3, 1016–1030. doi: 10.1002/ece3.516
Hendriks I. E., Duarte C. M., Olsen Y. S., Steckbauer A., Ramajo L., Moore T. S., et al. (2015). Biological mechanisms supporting adaptation to ocean acidification in coastal ecosystems. Estuarine Coast. Shelf Sci. 152, A1–A8. doi: 10.1016/j.ecss.2014.07.019
Jahnsen-Guzmán N., Lagos N. A., Lardies M. A., Vargas C. A., Fernández C., San Martín V., et al. (2021). Environmental refuges increase perfomance of juvenile mussels Mytilus chilensis: Implications for mussel seedling and farming strategies. Sci. Total Environm. 751, 141723.
Jahnsen-Guzmán N., Lagos N. A., Quijón P. A., Manríquez P. H., Lardies M. A., Fernández C., et al. (2022). Ocean acidification alters anti-predator responses in a competitive dominant intertidal mussel. Chemosphere 288, 132410. doi: 10.1016/j.chemosphere.2021.132410
Jakubowska M., Normant-Saremba M. (2015). The effect of CO 2 -induced seawater acidification on the behaviour and metabolic rate of the Baltic clam Macoma balthica. Annales Zoologici Fennici 52, 353–367. doi: 10.5735/086.052.0509
Kooijman B. (2010). Dynamic energy budget theory for metabolic organisation (New York: Cambridge university press).
Kroeker K. J., Kordas R. L., Crim R., Hendriks I. E., Ramajo L., Singh G. S., et al. (2013). Impacts of ocean acidification on marine organisms: quantifying sensitivities and interaction with warming. Global Change Biol. 19, 1884–1896. doi: 10.1111/gcb.12179
Kuznetsova A., Brockhoff P. B., Christensen R. H. B. (2017). lmerTest package: Tests in linear mixed effects models. J. Stat. Software 82, 1–26. doi: 10.18637/jss.v082.i13
Lagos N. A., Benítez S., Grenier C., Rodriguez-Navarro A. B., García-Herrera C., Abarca-Ortega A., et al. (2021). Plasticity in organic composition maintains biomechanical performance in shells of juvenile scallops exposed to altered temperature and pH conditions. Sci. Rep. 11, 24201. doi: 10.1038/s41598-021-03532-0
Lannig G., Eilers S., Pörtner H. O., Sokolova I. M., Bock C. (2010). Impact of ocean acidification on energy metabolism of oyster, crassostrea gigas–changes in metabolic pathways and thermal response. Mar. Drugs 8, 2318–2339. doi: 10.3390/md8082318
Lardies M. A., Benitez S., Osores S., Vargas C. A., Duarte C., Lohrmann K. B., et al. (2017). Physiological and histopathological impacts of increased carbon dioxide and temperature on the scallops Argopecten purpuratus cultured under upwelling influences in northern Chile. Aquaculture 479, 455–466. doi: 10.1016/j.aquaculture.2017.06.008
Lardies M. A., Clasing E., Navarro J. M., Stead R. (2001). Effects of environmental variables on burial depth of two infaunal bivalves inhabiting a tidal flat in southern Chile. J. Mar. Biol. Assoc. United Kingdom 81, 809–816. doi: 10.1017/S0025315401004635
Lemasson A. J., Knights A. M. (2021). Differential responses in anti-predation traits of the native oyster Ostrea edulis and invasive magallana gigas to ocean acidification and warming. Mar. Ecol. Prog. Ser. 665, 87–102. doi: 10.3354/meps13687
Leong P., Manahan D. (1997). Metabolic importance of Na+/K+-ATPase activity during sea urchin development. J. Exp. Biol. 200, 2881–2892. doi: 10.1242/jeb.200.22.2881
Le Quéré C., Andrew R. M., Friedlingstein P., Sitch S., Hauck J., Pongratz J., et al. (2018). Global carbon budget 2018. Earth System Sci. Data 10, 2141–2194. doi: 10.5194/essd-10-2141-2018
Leung J. Y., Connell S. D., Nagelkerken I., Russell B. D. (2017). Impacts of near-future ocean acidification and warming on the shell mechanical and geochemical properties of gastropods from intertidal to subtidal zones. Environ. Sci. Technol. 51, 12097–12103. doi: 10.1021/acs.est.7b02359
Leung J. Y., Russell B. D., Connell S. D. (2020). Linking energy budget to physiological adaptation: How a calcifying gastropod adjusts or succumbs to ocean acidification and warming. Sci. Total Environ. 715, 136939. doi: 10.1016/j.scitotenv.2020.136939
Lubchenco J., Haugan P. M., Pangestu M. E. (2020). Five priorities for a sustainable ocean economy. Nature 588, 30–32. doi: 10.1038/d41586-020-03303-3
Lüdecke D., Ben-Shachar M. S., Patil I., Waggoner P., Makowski D. (2021). Performance: An r package for assesment, comparison and testing of statistical models. J. Open Source Software 6, 3139. doi: 10.21105/joss.03139
Maynou F., Galimany E., Ramón M., Solé M. (2020). Impact of temperature increase and acidification on growth and the reproductive potential of the clam Ruditapes philippinarum using DEB. Estuarine Coast. Shelf Sci. 247, 107099. doi: 10.1016/j.ecss.2020.107099
Michaelidis B., Ouzounis C., Paleras A., Pörtner H.-O. (2005). Effects of long-term moderate hypercapnia on acid-base balance and growth rate in marine mussels Mytilus galloprovincialis. Mar. Ecol. Prog. Ser. 293, 109–118. doi: 10.3354/meps293109
Miranda F. V., Stotz W. B. (2021). Why do state interventions in artisanal fisheries often fail in Chile? misalignment to the way of life of fishers. Mar. Policy 132, 104693. doi: 10.1016/j.marpol.2021.104693
Navarro J. M., Andrade-Villagrán P. V., Manríquez P. H., Duarte C., Chaparro O. R. (2022). Long-term effects of contrasting pCO2 levels on the scope for growth in the carnivorous gastropod Concholepas concholepas. Mar. Environ. Res. 175, 105586. doi: 10.1016/j.marenvres.2022.105586
Navarro J. M., Duarte C., Manriquez P. H., Lardies M., Torres R., Acuña K., et al. (2016). Ocean warming and elevated carbon dioxide: Multiple stressor impacts on juvenile mussels from southern Chile. ICES Journal of Marine Science. doi: 10.1093/icesjms/fsv249
Navarro J. M., Torres R., Acuña K., Duarte C., Manriquez P. H., Lardies M., et al. (2013). Impact of medium-term exposure to elevated pCO2 levels on the physiological energetics of the mussel Mytilus chilensis. Chemosphere 90, 1242–1248. doi: 10.1016/j.chemosphere.2012.09.063
Naylor R. L., Hardy R. W., Buschmann A. H., Bush S. R., Cao L., Klinger D. H., et al. (2021). A 20-year retrospective review of global aquaculture. Nature 591, 551–563. doi: 10.1038/s41586-021-03308-6
Orr J. C., Fabry V. J., Aumont O., Bopp L., Doney S. C., Feely R. A., et al. (2005). Anthropogenic ocean acidification over the twenty-first century and its impact on calcifying organisms. Nature 437, 681–686. doi: 10.1038/nature04095
Osores S., Lagos N., San Martin V., Manríquez P., Vargas C., Torres R., et al. (2017). Plasticity and inter-population variability in physiological and life-history traits of the mussel Mytilus chilensis: A reciprocal transplant experiment. J. Exp. Mar. Biol. Ecol. 490, 1–12. doi: 10.1016/j.jembe.2017.02.005
Palmer R. A. (1982). Growth in marine gastropods: a non-destructive technique for independently measuring shell and body weight. Malacologia 23, 63–73.
Palmer A. R. (1992). Calcification in marine molluscs: how costly is it? Proc. Natl. Acad. Sci. United States America 89, 1379–1382. doi: 10.1073/pnas.89.4.1379
Parker L. M., Ross P. M., O’connor W. A., Pörtner H. O., Scanes E., Wright J. M. (2013). Predicting the response of molluscs to the impact of ocean acidification. Biol. (Basel) 2, 651–692. doi: 10.3390/biology2020651
Pierrot D., Lewis E., Wallace D. W. R. (2006). MS excel program developed for CO2 system calculations. ORNL/CDIAC-105a.
Poore A. G., Graba-Landry A., Favret M., Sheppard Brennand H., Byrne M., Dworjanyn S. A. (2013). Direct and indirect effects of ocean acidification and warming on a marine plant–herbivore interaction. Oecologia 173, 1113–1124. doi: 10.1007/s00442-013-2683-y
Pörtner H. O., Farrell A. P. (2008). Physiology and climate change. Science 322, 690–692. doi: 10.1126/science.1163156
Pörtner H. O., Langenbuch M., Reipschläger A. (2004). Biological impact of elevated ocean CO2 concentrations: lessons from animal physiology and earth history. J. oceanography 60, 705–718. doi: 10.1007/s10872-004-5763-0
Range P., Chícharo M., Ben-Hamadou R., Piló D., Fernandez-Reiriz M., Labarta U., et al. (2014). Impacts of CO2-induced seawater acidification on coastal Mediterranean bivalves and interactions with other climatic stressors. Regional Environ. Change 14, 19–30. doi: 10.1007/s10113-013-0478-7
R Core Team (2020). “R: A language and environment for statistical computing,” in R foundation for statistical computing(Vienna, Austria). Available at: https://www.R-project.org/.
Reipschläger A., Pörtner H.-O. (1996). Metabolic depression during environmental stress: the role of extracellular versus intracellular pH in Sipunculus nudus. J. Exp. Biol. 199, 1801–1807. doi: 10.1242/jeb.199.8.1801
Sage R. F. (2020). Global change biology: A primer. Global Change Biol. 26, 3–30. doi: 10.1111/gcb.14893
SERNAPESCA (2021). “Boletines informativos regionales, tercer trimestre 2021,” in Ministerio de economía, fomento y turismo. (Chile: Servicio Nacional de Pesca y Acuicultura).
Short R. E., Gelcich S., Little D. C., Micheli F., Allison E. H., Basurto X., et al. (2021). Harnessing the diversity of small-scale actors is key to the future of aquatic food systems. Nat. Food 2, 733–741. doi: 10.1038/s43016-021-00363-0
Spalding C., Finnegan S., Fischer W. W. (2017). Energetic costs of calcification under ocean acidification. Global Biogeochemical Cycles 31, 866–877. doi: 10.1002/2016GB005597
Stead R. A., Clasing E. (1997). Reproductive cycle and cohort formation of Venus antiqua (Bivalvia: Veneridae). Rev. Chil. Hist. Natural 70, 181–190.
Stumpp M., Dupont S., Thorndyke M. C., Melzner F. (2011). CO2 induced seawater acidification impacts sea urchin larval development II: Gene expression patterns in pluteus larvae. Comp. Biochem. Physiol. A Mol. Integr. Physiol. 160, 320–330. doi: 10.1016/j.cbpa.2011.06.023
Thomsen J., Casties I., Pansch C., Körtzinger A., Melzner F. (2013). Food availability outweighs ocean acidification effects in juvenile Mytilus edulis: Laboratory and field experiments. Global Change Biol. 19, 1017–1027. doi: 10.1111/gcb.12109
Tittensor D. P., Novaglio C., Harrison C. S., Heneghan R. F., Barrier N., Bianchi D., et al. (2021). Next-generation ensemble projections reveal higher climate risks for marine ecosystems. Nat. Climate Change 11, 973–981. doi: 10.1038/s41558-021-01173-9
Torres R., Manríquez P., Duarte C., Navarro J., Lagos N., Vargas C., et al. (2013). Evaluation of a semi-automatic system for long-term seawater carbonate chemistry manipulation. Rev. Chil. Hist. Natura 86, 443–451. doi: 10.4067/S0716-078X2013000400006
Troell M., Naylor R. L., Metian M., Beveridge M., Tyedmers P. H., Folke C., et al. (2014). Does aquaculture add resilience to the global food system? Proc. Natl. Acad. Sci. 111, 13257–13263. doi: 10.1073/pnas.1404067111
Urrutia G. X., Navarro J., Clasing E., Stead R. (2001). The effects of environmental factors on the biochemical composition of the bivalve Tagelus dombeii (Lamarck 1818) (Tellinacea: Solecurtidae) from the intertidal flat of coihuín, Puerto montt, Chile. J. Shellfish Res. 20, 1077–1088.
Vargas C. A., Cuevas L. A., Broitman B. R., San Martin V. A., Lagos N. A., Gaitán-Espitia J. D., et al. (2022). Upper environmental pCO2 drives sensitivity to ocean acidification in marine invertebrates. Nat. Climate Change 12, 200–207. doi: 10.1038/s41558-021-01269-2
Vargas C. A., Lagos N. A., Lardies M. A., Duarte C., Manríquez P. H., Aguilera V. M., et al. (2017). Species-specific responses to ocean acidification should account for local adaptation and adaptive plasticity. Nat. Ecol. Evol. 1, 0084. doi: 10.1038/s41559-017-0084
Verberk W. C., Overgaard J., Ern R., Bayley M., Wang T., Boardman L., et al. (2016). Does oxygen limit thermal tolerance in arthropods? A critical review of current evidence. Comp. Biochem. Physiol. Part A: Mol. Integr. Physiol. 192, 64–78. doi: 10.1016/j.cbpa.2015.10.020
Ward D., Melbourne-Thomas J., Pecl G. T., Evans K., Green M., Mccormack P. C., et al. (2022). Safeguarding marine life: conservation of biodiversity and ecosystems. Rev. Fish Biol. Fisheries 32, 65–100. doi: 10.1007/s11160-022-09700-3
Watson S.-A., Morley S. A., Peck L. S. (2017). Latitudinal trends in shell production cost from the tropics to the poles. Sci. Adv. 3, e1701362. doi: 10.1126/sciadv.1701362
Weiner J. (1992). Physiological limits to sustainable energy budgets in birds and mammals: Ecological implications. Trends Ecol. Evol. 7, 384–388. doi: 10.1016/0169-5347(92)90009-Z
Xu X., Yang F., Zhao L., Yan X. (2016). Seawater acidification affects the physiological energetics and spawning capacity of the Manila clam Ruditapes philippinarum during gonadal maturation. Comp. Biochem. Physiol. Part A: Mol. Integr. Physiol. 196, 20–29. doi: 10.1016/j.cbpa.2016.02.014
Keywords: ecophysiology, ocean warming, metabolic depression, decalcification, artisanal fisheries, infaunal bivalves
Citation: Martel SI, Fernández C, Lagos NA, Labra FA, Duarte C, Vivanco JF, García-Herrera C and Lardies MA (2022) Acidification and high-temperature impacts on energetics and shell production of the edible clam Ameghinomya antiqua. Front. Mar. Sci. 9:972135. doi: 10.3389/fmars.2022.972135
Received: 17 June 2022; Accepted: 25 August 2022;
Published: 12 September 2022.
Edited by:
Marco Munari, Anton Dohrn Zoological Station, ItalyReviewed by:
Davide Asnicar, University of Padua, ItalyMatteo Nannini, Stazione Zoologica Anton Dohrn Napoli, Italy
Copyright © 2022 Martel, Fernández, Lagos, Labra, Duarte, Vivanco, García-Herrera and Lardies. This is an open-access article distributed under the terms of the Creative Commons Attribution License (CC BY). The use, distribution or reproduction in other forums is permitted, provided the original author(s) and the copyright owner(s) are credited and that the original publication in this journal is cited, in accordance with accepted academic practice. No use, distribution or reproduction is permitted which does not comply with these terms.
*Correspondence: Marco A. Lardies, bWFyY28ubGFyZGllc0B1YWkuY2w=