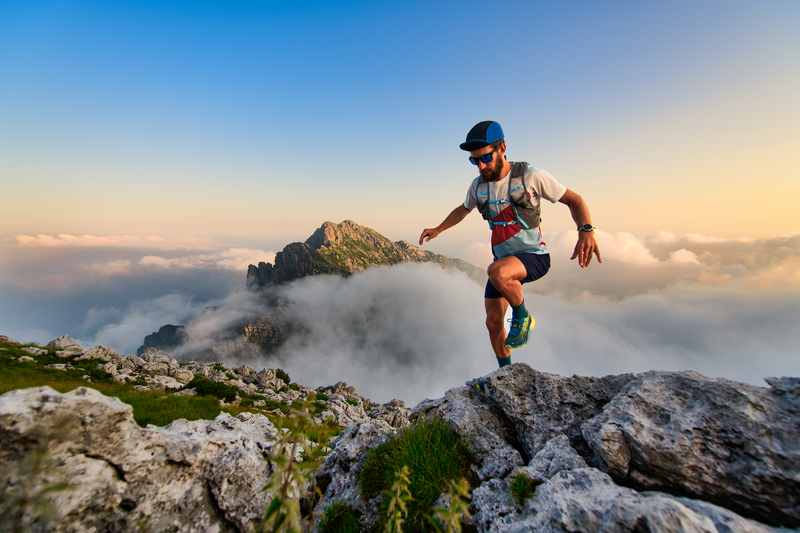
95% of researchers rate our articles as excellent or good
Learn more about the work of our research integrity team to safeguard the quality of each article we publish.
Find out more
ORIGINAL RESEARCH article
Front. Mar. Sci. , 11 August 2022
Sec. Marine Biogeochemistry
Volume 9 - 2022 | https://doi.org/10.3389/fmars.2022.970678
This article is part of the Research Topic Carbon Dynamics in Freshwater, Coastal and Oceanic Ecosystems in Response to the SDG Goals View all 12 articles
To determine the effects of mesoscale eddies on sea-air CO2 flux, we investigated the surface fugacity of CO2 (surface fCO2) distribution in the northern Philippine Sea, where mesoscale eddies are common. Surface fCO2 showed large spatial variations, such that values were high in the non-eddy and cyclonic eddy regions, while they were low within the anticyclonic eddy. The maximum fCO2 was observed in the non-eddy region; higher fCO2 values were observed in the area surrounding the cyclonic eddy than at the center of the cyclonic eddy. Within the cyclonic eddy, the contribution of dissolved inorganic carbon (DIC) enrichment because of upwelling was considerably offset by cooling. In the non-eddy region, the contribution of DIC enrichment from upwelling was rarely offset by cooling; thus, the maximum fCO2 was observed in the non-eddy region. Surface fCO2 showed a robust correlation with sea surface temperature (SST) within the cyclonic and anticyclonic eddies, but it did not display any correlation in the non-eddy region. Temperature was a major factor that controlled surface fCO2 in the anticyclonic eddy, but this effect was absent in the cyclonic eddy. Temperature-normalized fCO2 exhibited a clear negative relationship with SST in the cyclonic eddy and the non-eddy region, indicating that surface fCO2 was considerably affected by the upwelling of high-fCO2 deep water in both regions. Sea-air CO2 fluxes ranged from 0.011 to 9.92 mmol m-2 day-1 and all values were positive, indicating that the entire study area acted as a CO2 source during the research period. The estimated mean sea-air CO2 fluxes in the cyclonic eddy, anticyclonic eddy, and non-eddy region were 1.10 ± 0.75, 0.64 ± 0.66, and 1.42 ± 1.12 mmol m-2 day-1, respectively. The sea-air CO2 fluxes considerably varied according to eddy type; they were almost twofold higher in the cyclonic eddy than in the anticyclonic eddy. In the cyclonic eddy and non-eddy regions, upwelling caused surface fCO2 to increase, thereby increasing sea-air CO2 flux.
The Philippine Sea is a marginal sea bounded by the Philippines and Taiwan to the west, the Ryukyu Island arc to the north, and the Izu–Mariana Ridge to the east (Ramp et al., 2017). This sea is oceanographically bounded by the North Equatorial Current to the south, Kuroshio Current to the west, and Ryukyu Current to the north (Yaremchuk and Qu, 2004; Andres et al., 2008; Ramp et al., 2017). The Subtropical Counter Current (STCC) flows eastward in the Philippine Sea; it is present at shallow depths (~100 m) around 17–27°N latitude and 130–180°E longitude (Chang and Oey, 2014). The STCC region contains cyclonic and anticyclonic eddies with diameters of ~300 km that propagate westward at approximately 8–10 km day-1 (Chelton et al., 2011). These STCC eddies have large impacts on ocean circulation in the western North Pacific Ocean (Zhang et al., 2001; Miyazawa et al., 2008; Sheu et al., 2010).
In the open ocean, mesoscale eddies have considerable impacts on surface fugacity of CO2 (fCO2) and sea-air CO2 flux (Mahadevan et al., 2004; Gonzalez-Davila et al., 2006; Chen et al., 2007; Song et al., 2016; Moreau et al., 2017; Orselli et al., 2019). In cyclonic eddies, the uplift of subsurface waters may initially increase surface fCO2, but this increase is largely offset by the lower temperature and biological productivity of the uplifted waters (Mahadevan et al., 2004); they have suggested that the vertical transport of dissolved inorganic carbon (DIC), temperature, and nitrate, as well as their contributions to surface fCO2, mainly depend on their vertical gradients below the mixed layer. In the subtropical North Pacific Ocean, a negative relationship between sea surface temperature (SST) and surface fCO2 is observed in a cold cyclonic eddy; the magnitude of the CO2 sink decreases by 17% with the passage of a mesoscale cyclonic eddy (Chen et al., 2007). In the subtropical North Atlantic Ocean, surface fCO2 is 15 µatm lower inside the cyclonic eddy than in surrounding waters (Gonzalez-Davila et al., 2006). In the South Atlantic Ocean, anticyclonic eddies serve as CO2 sinks relative to the surrounding waters (Orselli et al., 2019). In the Southern Ocean, eddy effects on sea-air CO2 fluxes are rather complex; compared with cyclonic eddies, anticyclonic eddies take up more CO2 during summer (this relationship is reversed in winter), and anticyclonic eddies exhibit more outgassing than do cyclonic eddies (Song et al., 2016). In the open ocean, therefore, the impacts of mesoscale eddies on sea-air CO2 fluxes are not simple; the overall sign and magnitude depend on a complex balance of physical and biological processes.
Several researchers have reported that surface fCO2 is nearly equilibrated with atmospheric CO2 in the Philippine Sea; therefore, the sea-air CO2 flux is near zero (Inoue et al., 1995; Ishii et al., 2001; Takahashi et al., 2009; Ishii et al., 2014; Yasunaka et al., 2019). No zonal survey of surface fCO2 has been conducted in the STCC region of the Philippine Sea where both cyclonic and anticyclonic eddies are abundant. These mesoscale eddies can affect the zonal distribution of surface fCO2 and sea-air CO2 flux in the Philippine Sea. In this study, we conducted a zonal survey of surface fCO2 in the northern Philippine Sea where cyclonic and anticyclonic eddies frequently occur. We identified the major factors that control surface fCO2 in the northern Philippine Sea. In addition, we explored how surface fCO2 was affected by changes in SST, sea surface salinity (SSS), DIC, and total alkalinity (TA). Finally, we characterized the sea-air CO2 flux in cyclonic eddy, anticyclonic eddy, and non-eddy regions to elucidate the effects of mesoscale eddies on sea-air CO2 flux in the northern Philippine Sea.
This study was conducted on the R/V ISABU in the northern Philippine Sea from September 14th to 23rd, 2017 (Figure 1). Underway samples were collected using the flow-through system onboard R/V ISABU. Surface fCO2 was obtained from a commercial underway pCO2 system (Model 8050, General Oceanics Inc., FL, USA) equipped on the vessel; the system consisted of a non-dispersive infrared analyzer (Li-7000, LI-COR Inc., NE, USA) connected through an 8-port switching valve to three types of gases (standard gases, ambient air, and air equilibrated with flowing seawater). The analyzer was calibrated at 14-h intervals using three standard gases with different CO2 concentrations (202, 350, and 447 ppm in dry air) after it had been zeroed and spanned using ultra-high-purity standard N2 gas (zero-CO2 gas) and 447-ppm CO2 standard gas, respectively. The system was operated on the following cycle: 3 standard gases, 10 measurements of ambient air, 40 measurements of air equilibrated with seawater, and 3 repeats of all previous measurements except the standard gases. CO2 measurement was conducted in mole fraction in dry air (xCO2) mode at 4-min intervals, then converted into fCO2 through correction for non-ideality and water vapor fraction based on the method established by Pierrot et al. (2009). Because atmospheric and seawater CO2 measurements were not conducted concurrently, the atmospheric CO2 value associated with each seawater measurement was linearly interpolated from the two nearest atmospheric measurement sets. Underway SST and SSS data were obtained alongside CO2 measurements using a digital thermometer (SBE-38, Sea-Bird Scientific, WA, USA) and a thermosalinograph (SBE-45, Sea-Bird Scientific), respectively, installed near the seawater intake of the vessel.
Figure 1 Map of the study area and locations of sampling stations overlaid on mean sea surface height anomaly and geostrophic velocity in the northern Philippine Sea from September 14th to 23rd, 2017. Red color indicates an anticyclonic eddy, and blue color indicates a cyclonic eddy.
Vertical profiles of temperature and salinity were measured with a calibrated conductivity–temperature–depth/pressure recorder (SBE-911; Sea-Bird Electronics Inc., WA, USA) at 29 stations (Figure 1). Seawater samples were collected for the measurement of DIC, TA, nitrate+nitrite (hereafter referred to as nitrate), and chlorophyll-a using a rosette sampler with 10-L Niskin bottles mounted on the conductivity–temperature–depth/pressure assembly. DIC concentrations were determined using the VINDTA 3D system (Marianda, Kiel, Germany) coupled to a CO2 coulometric titrator (Model 5011: UIC, Inc., Joliet, IL, USA). TA concentrations were determined with a potentiometric titration system (AS-ALK2, Apollo SciTech LLC, DE, USA). The measurement uncertainty of DIC and TA was evaluated on a daily basis using certified reference materials provided by the Scripps Institution of Oceanography (University of California, San Diego, CA, USA). The precisions of DIC and TA were ± 2 μmol kg-1 and ± 3 μmol kg-1, respectively. Nitrate concentrations were measured using a four-channel continuous auto-analyzer (QuAAtro 39, Seal Analytical Inc., Germany). Reference materials for nitrate in seawater provided by “KANSO Technos” (Lot. No. “BV”) were measured alongside standards in each batch. The analytical error for nitrate was ± 0.14 µmol kg-1. Water samples for chlorophyll-a analysis were filtered through GF/F filters (47 mm, Whatman). Chlorophyll-a concentrations were determined using extracted filtrate mixed with 90% acetone for 24 h shipboard with a fluorometer (Trilogy; Turner Designs, USA) that had been previously calibrated against pure chlorophyll-a (Sigma, USA).
Temperature-normalized surface fCO2 values were calculated by normalizing surface fCO2 based on temperature to examine the effects of SST on the distribution of surface fCO2, as suggested by Takahashi et al. (1993), using the following equation:
where 0.0423 is the thermodynamic coefficient (Takahashi et al., 1993), SSTaver is the average of all underway SST measurements within the study area (30.1°C), and SSTmeas is the observed value for each CO2 measurement.
Sea-air CO2 flux was calculated using the following equations:
where k and K0 are the gas transfer velocity and the solubility coefficient of CO2 in seawater, respectively (Weiss, 1974). For the coefficient k, we used the constant value (0.251) from the formulation of Wanninkhof (2014). U10 and Sc indicate wind speed collected at 10 m altitude and the Schmidt number, respectively. Wind speed data were obtained from an automatic weather station installed on R/V ISABU during underway CO2 measurement.
Daily mean satellite altimeter gridded sea level anomaly data with 0.25° × 0.25° spatial resolution were used to examine the appearance and movement of eddies; they were obtained from a data product estimated through the optimal interpolation method, which merged available measurements from multiple altimeter missions. These data are available online from the Copernicus Marine Service (https://marine.copernicus.eu).
In the study area, two eddies (cyclonic and anticyclonic) were identified on the basis of sea surface height anomaly (SSHA) during the research period (Figure 1). SSHA is negative for a cyclonic eddy, whereas it is positive for an anticyclonic eddy. The cyclonic eddy propagated westward at a rate of 8 km day-1, while the anticyclonic eddy propagated southwestward at a rate of 6 km day-1 during the research period. The widths of the anticyclonic and cyclonic eddies had ranges of 400–440 km and 120–160 km, respectively, during the research period (Figure 1). The anticyclonic eddy was stronger than the cyclonic eddy. Surface waters in the cyclonic eddy were typically colder and more saline, while they were warmer and fresher in the anticyclonic eddy (Figures 2A, B). Surface fCO2 ranged from 384.2 to 406.7 µatm, with a mean of 393.2 µatm; atmospheric fCO2 varied from 379.2 to 383.1 µatm, with a mean of 381.2 µatm. Lower surface fCO2 was observed in the anticyclonic eddy containing fresher and warmer waters (Figure 2C). The cyclonic eddy, with saltier and colder waters, showed moderate surface fCO2 (395–400 µatm). Higher surface fCO2 (> 400 µatm) was found in the area surrounding the cyclonic eddy (Figure 2C). Surface DIC concentrations ranged from 1902 to 1963 μmol kg-1, with higher values in the cyclonic eddy and lower values in the anticyclonic eddy (Figure 2D). Surface TA displayed a distribution pattern similar to DIC, with a concentration range of 2237–2288 μmol kg-1 (Figure 2E).
Figure 2 Surface distributions of temperature (A), salinity (B), fCO2 (C), DIC (D), TA (E), and salinity-normalized TA (nTA, F) in the study area. Underway and discrete measurements are shown in white lines (A–C) and black dots (D, E), respectively.
The vertical distributions of temperature, salinity, seawater density, DIC, TA, nitrate, and chlorophyll-a were plotted along the 19.5°N transect, where both cyclonic and anticyclonic eddies were observed (Figure 3). The upper layer from the surface to 200 m was warmer and less salty in the anticyclonic eddy than in the cyclonic eddy (Figures 3A, B). The uplifted structure of the cyclonic eddy was clearly observed from the vertical distributions of temperature, salinity, and seawater density, whereas a downwelled structure was observed in the anticyclonic eddy. DIC concentrations generally showed low values (< 1940 μmol kg-1) within the upper 50 m of the anticyclonic eddy; these low values were associated with low salinity in this layer (Figure 3D). The DIC concentration was approximately 40 μmol kg-1 higher at 50 m of water depth in the cyclonic eddy than at the same depth in the anticyclonic eddy. DIC exhibited a distinct downwelling pattern within the 300 m water depth of the anticyclonic eddy. TA displayed a vertical distribution similar to DIC, with a downwelling pattern within the upper 300 m of water in the anticyclonic eddy (Figure 3F). Nitrate was completely depleted within the upper 100 m of the water column in the study area, with extremely low concentrations (< 1.0 μmol kg-1, Figure 3G). Nitrate showed a downwelling pattern below 150 m in depth within the anticyclonic eddy. Chlorophyll-a concentrations were rather low (< 0.1 mg m-3) and uniform within the upper 80 m of water (Figure 3H). A subsurface chlorophyll maximum was observed at the depth of 130 m in the anticyclonic eddy and at 100 m in the cyclonic eddy.
Figure 3 Vertical distributions of temperature (A), salinity (B), seawater density (C), DIC (D), TA (E), fCO2 (F), nitrate (G), and chlorophyll-a (H) along the 19.5°N transect. Open diamonds indicate sampling depths.
In the open ocean, surface fCO2 generally exhibits a significant correlation with SST (Stephens et al., 1995; Bates et al., 1998; Nelson et al., 2001; Takahashi et al., 2002; Olsen et al., 2004; Chen et al., 2007; Parard et al., 2016). However, in the study area, surface fCO2 was not strongly correlated with SST; there was considerable variation in the data (Figure 4A). We divided the study area into three regions on the basis of SSHA: cyclonic eddy (SSHA ≤ −0.1 m), anticyclonic eddy (SSHA ≥ 0.1 m), and non-eddy (−0.1 m < SSHA < 0.1 m). Surface fCO2 exhibited a significant correlation (r = 0.75, p < 0.001) with SST within the cyclonic eddy (Figure 4B) and a relatively strong correlation (r = 0.61, p < 0.001) within the anticyclonic eddy; it did not exhibit a correlation in the non-eddy region (Figure 4B). These results substantially differ from findings in the subtropical northeastern Pacific, where surface fCO2 showed a positive linear relationship with SST outside of a cyclonic eddy and a negative relationship with SST within the cyclonic eddy (Chen et al., 2007). This discrepancy was presumably related to different vertical gradients of temperature and DIC below the mixed layer in these two areas; such gradients drive the vertical transport of temperature and DIC (Mahadevan et al., 2004).
Figure 4 Plots of fCO2 vs. SST in the study region (A) and three subregions (B); cyclonic eddy, anticyclonic eddy, and non-eddy. Blue dots indicate a cyclonic eddy, green dots are non-eddy region, and red dots are an anticyclonic eddy. Dotted lines indicate linear regressions in the cyclonic and anticyclonic eddies.
Surface fCO2 was positively linearly correlated with SST within the anticyclonic eddy, such that its values were positioned along two lines (Figure 5). These two lines were separated by approximately 5 µatm of surface fCO2 at a given SST. The slopes (∂lnfCO2/∂T) of those two lines were identical (0.0358), which was similar to the isochemical trend (0.0423) identified by Takahashi et al. (1993); the findings suggested that temperature was a major factor controlling surface fCO2 within the anticyclonic eddy. The two lines were separated according to latitude; the upper line was located along the 20.5°N transect, while the lower line was located at the 18.5 and 19.5°N transects. SSS did not considerably differ between the two transects, but surface DIC concentrations were slightly higher along the 20.5°N transect than along the 18.5 and 19.5°N transects (Figure 2D); thus, fCO2 of the upper line was 5 µatm higher at a given SST, compared with fCO2 of the lower line.
Figure 5 Plots of fCO2 vs. SST in the anticyclonic eddy. Solid lines indicate linear regressions at 20.5°N and 18.5°N-19.5°N transects.
Surface fCO2 was also positively correlated with SST within the cyclonic eddy (Figure 4B), with a slope (∂lnfCO2/∂T) of 0.0128, which was much lower than the isochemical trend (0.0423); this finding suggested that temperature was not a major factor controlling surface fCO2 within the cyclonic eddy. In the plot of temperature-normalized fCO2 at 30.1°C vs. SST, a negative relationship was clearly present within the cyclonic eddy (Figure 6A). This negative relationship within the cyclonic eddy may be attributed to the upwelling of high-fCO2 deep water. The highest temperature-normalized fCO2 value (428.4 µatm) was observed at the minimum temperature (28.2°C), which was found at the center of the cyclonic eddy. The temperature-normalized fCO2 value increased by 30.4 µatm from a mean value of 398.0 µatm in the non-eddy region to the maximum value of 428.4 µatm within the cyclonic eddy because of the upwelling of cold high-fCO2 deep water. When this cold high-fCO2 deep water reached the surface and flowed away from the eddy center, it mixed with warm low-fCO2 surface water; thus, temperature-normalized fCO2 decreased with increasing SST. In addition, temperature-normalized fCO2 decreased because of CO2 efflux and biological uptake (Takahashi et al., 2002; Chen et al., 2007). The cyclonic eddy showed positive ΔfCO2 values (surface fCO2 – atmospheric fCO2), with a range of 5.2–24 µatm (Figure 7A); this finding indicated that CO2 was outgassed in the study area in September 2017. Thus, the temperature-normalized fCO2 might be reduced because of CO2 efflux. Biological uptake is an important driver of decreased surface fCO2 in the ocean. The upwelling of nitrate-enriched deep water may enhance biological production because nitrate is completely depleted in the surface water (Figure 3G) and DIC is consumed during biological uptake fueled by upwelled nitrate, which causes surface fCO2 to decrease. In the study area, nitrate concentrations were extremely low (< 1.0 μmol kg-1) within the upper 100 m of the water column and showed no uplifted structure (Figure 3G). In addition, chlorophyll-a concentrations were rather low (< 0.1 mg m-3) and uniform within the upper 80 m of the water column (Figure 3H). Thus, biological uptake may not considerably influence the temperature-normalized fCO2 decrease in the study area.
Figure 6 Plots of temperature-normalized fCO2 vs. SST in the cyclonic eddy (A), non-eddy region (B), and anticyclonic eddy (C). Solid lines indicate linear regressions.
Figure 7 Spatial distributions of ΔfCO2(A), wind speed (B), and sea-air CO2 fluxes (C) in the northern Philippine Sea from September 14th to 23rd, 2017.
In the non-eddy region, temperature-normalized fCO2 also showed a negative relationship with SST (Figure 6B). This negative relationship indicated that the non-eddy region was affected by the upwelling of high-fCO2 deep water. Higher temperature-normalized fCO2 values were found in the area surrounding the cyclonic eddy. Chen et al. (2007) observed that temperature-normalized pCO2 values were nearly independent of the temperature outside the cyclonic eddy in the subtropical northeastern Pacific Ocean; they suggested that temperature was a major factor controlling surface pCO2 in that region. Thus, surface pCO2 behavior in the subtropical northeastern Pacific Ocean markedly differed from surface pCO2 behavior in our study area (the northern Philippine Sea), where temperature was not a major factor controlling surface fCO2 outside of the cyclonic eddy. In our study, we found that the upwelling of high-fCO2 deep water considerably influenced surface fCO2 in the area surrounding the cyclonic eddy. The cyclonic eddy propagated westward at a rate of 8 km day-1. The area surrounding the cyclonic eddy may be affected by the upwelling of high-fCO2 deep water; this possibility was corroborated by the observation of elevated temperature-normalized fCO2 values only in the area surrounding the cyclonic eddy. In the the anticyclonic eddy, most temperature-normalized fCO2 values were present within the narrow SST range of 30–31°C, and a weak negative relationship with SST was observed (Figure 6C).
Surface fCO2 showed high spatial variability, such that values were high in the non-eddy region and anticyclonic eddy, while they were low within the cyclonic eddy (Figure 2C). The maximum fCO2 was observed in the non-eddy region, which was located at the center of the study area (Figure 2C). Indeed, elevated surface fCO2 was observed in the area surrounding the cyclonic eddy, but it was absent from the center of the cyclonic eddy (Figure 2C). The upwelled water enriched in DIC had a high fCO2 value, but its impact was offset by the low temperature of the upwelled water. To determine the effect of upwelling on surface fCO2 in the cyclonic eddy and non-eddy regions, we explored the effects of changes in SST, SSS, DIC, and TA on surface fCO2 by using the following equation (Takahashi et al., 1993; Sarmiento and Gruber, 2006);
The equation used by authors (p. 7) is valid only along the water pathway (Lagrangian approach). The presented equation takes into account the changes in the seawater pCO2 due to evaporation/precipitation, warming/cooling, and photosynthesis/organic matter degradation. But it does not take into account the changes in the seawater pCO2 due to mixing (horizontal and vertical) with the water masses of different origin and different initial (pre-formed) values of pCO2 (T, S, TA, DIC). It’s necessarily to add some contents on cyclone and anticyclone water origin.
Anaswer: The changes in the seawater pCO2 due to mixing (horizontal and vertical) with the water masses make the changes in the DIC concentrations, and thereby, we can calculate the pCO2 changes due to mixing from the DIC changes by using the Revelle factor. Thus, this equation takes into account the changes in the seawater pCO2 due to mixing (horizontal and vertical) with the water masses.
where β is approximately 0.0423°C-1, α is 1.0, γDIC is 9.5, and γTA is −8.9 (Sarmiento and Gruber, 2006). Assuming that upwelling was not active in the anticyclonic eddy, that eddy would be served as a reference point for the comparison of δfCO2 values between the cyclonic eddy and non-eddy regions. Thus, the observed δfCO2 values in the cyclonic eddy were fCO2 differences between the cyclonic eddy and the anticyclonic eddy (Table 1). The observed δfCO2 values in the non-eddy region were fCO2 differences between the non-eddy region and anticyclonic eddy (Table 2). The observed δfCO2 values closely aligned with the δfCO2 values calculated from δSST, δSSS, δDIC, and δTA in both the cyclonic eddy and non-eddy regions (Tables 1, 2). The differences between the observed δfCO2 and calculated δfCO2 values were < 10% (Tables 1, 2).
Table 1 Observed δfCO2, δSST, δSSS, δDIC, and δTA in the cyclonic eddy, maximum and minimum fCO2 sites. Calculated δfCO2 values from δSST, δSSS, δDIC, and δTA are shown in brackets.
Table 2 Observed δfCO2, δSST, δSSS, δDIC, and δTA in the non-eddy region, maximum and minimum fCO2 sites. Calculated δfCO2 from δSST, δSSS, δDIC, and δTA are shown in brackets.
Mean surface fCO2 values were 12.2 µatm higher in the cyclonic eddy than in the anticyclonic eddy (Table 1). δfCO2 increased by 65.6 µatm because of DIC enrichment driven by upwelling in the cyclonic eddy, while it decreased by 37.2 µatm because of the TA increase (Table 1). Thus, the δfCO2 value increased by 28.4 µatm because of the combined effects of DIC and TA enrichment with upwelling in the cyclonic eddy. SST contributed a δfCO2 decrease of 19.9 µatm, while SSS contributed a δfCO2 increase of 4.4 µatm (Table 1).
Mean surface fCO2 values were 4.8 µatm higher in the non-eddy region than in the anticyclonic eddy (Table 2). δfCO2 increased by 22.7 µatm because of the DIC increase, while it decreased by 12.4 µatm because of the TA increase (Table 2). Thus, the δfCO2 value increased by 10.3 µatm overall because of DIC and TA increases in the non-eddy region. SST contributed a δfCO2 decrease of 6.7 µatm, while SSS contributed a δfCO2 increase of 1.3 µatm (Table 2).
The maximum δfCO2 value observed in the cyclonic eddy was 16.8 µatm (Table 1). The DIC and TA enrichment by upwelling contributed the δfCO2 increase of 41.7 µatm, while SST and SSS contributed the δfCO2 decrease of 24.4 µatm (Table 1). In contrast, the maximum δfCO2 value observed in the non-eddy region was 25.8 µatm (Table 2). The DIC and TA enrichment contributed the δfCO2 increase of 27.2 µatm, while SST and SSS contributed the δfCO2 decrease of 0.3 µatm (Table 2). In the cyclonic eddy, the contribution of DIC enrichment by upwelling was largely balanced by cooling. In the non-eddy region, however, the contribution of DIC enrichment by upwelling was rarely balanced by cooling; this was the main reason for maximum fCO2 observation in the non-eddy region. DIC enrichment in the non-eddy region was presumably associated with the upwelling because it was observed in the area surrounding the cyclonic eddy.
ΔfCO2 (surface fCO2 – atmospheric fCO2) varied from 5.2 to 25.7 µatm; it had consistently positive values. ΔfCO2 exhibited high spatial variability, such that values were high within the anticyclonic eddy and low within the cyclonic eddy (Figure 7A). Wind speed obtained from the R/V ISABU automatic weather station had a range of 0.6–15.8 m s-1 and exhibited high spatial variability, such that values were elevated in the non-eddy region (Figure 7B). Sea-air CO2 fluxes were calculated from ΔfCO2 and wind speed. In the study area, sea-air CO2 fluxes ranged from 0.011 to 9.92 mmol m-2 day-1 and all values were positive, indicating that the entire study area acted as a CO2 source during the research period (Figure 7C). The estimated mean sea-air CO2 fluxes in the cyclonic eddy, anticyclonic eddy, and non-eddy region were 1.10 ± 0.75, 0.64 ± 0.66, and 1.42 ± 1.12 mmol m-2 day-1, respectively. The sea-air CO2 fluxes considerably varied according to eddy type; they were almost twofold higher in the cyclonic eddy than in the anticyclonic eddy. In the cyclonic eddy and non-eddy regions, upwelling caused surface fCO2 to increase, thereby increasing sea-air CO2 flux. However, the CO2 flux was slightly greater in the non-eddy region than in the cyclonic eddy. The mean ΔfCO2 was higher in the cyclonic eddy than in the non-eddy region, but CO2 flux was higher in the non-eddy region because of the high wind speed (Figure 7). In addition, maximum ΔfCO2 was observed in the non-eddy region because the DIC enrichment caused by upwelling was rarely offset by cooling in this region.
The study area was located in the northern Philippine Sea, where surface fCO2 was nearly equilibrated with atmospheric CO2, and the sea-air CO2 flux was therefore rather small (< 0.6 mmol m-2 day-1; Takahashi et al., 2002; Takahashi et al., 2009; Ishii et al., 2014; Yasunaka et al., 2019). In this study, CO2 flux in the anticyclonic eddy was similar to previously reported results. However, CO2 fluxes in the cyclonic eddy and non-eddy region were approximately twofold greater than in previous studies. The cyclonic eddy caused the CO2 flux to increase because of the upwelling of high-fCO2 deep water. In the non-eddy region, high CO2 fluxes were observed in the area surrounding the cyclonic eddy, indicating that the non-eddy region was affected by the upwelling of high-fCO2 deep water. In the study area, a cyclonic eddy with a width of 120–160 km propagated westward at a rate of 8 km day-1. After the cyclonic eddy had passed, the waters surrounding the cyclonic eddy had high DIC concentrations, which caused surface fCO2 to increase during the warming of cold upwelled water. Indeed, the waters surrounding the cyclonic eddy showed higher CO2 fluxes compared with the area around the anticyclonic eddy (Figure 7C).
This study examined how mesoscale eddies affect surface fCO2 and sea-air CO2 flux in the northern Philippine Sea. Surface fCO2 showed high spatial variability, such that values were high in the non-eddy region and cyclonic eddy, while they were low within the anticyclonic eddy. Temperature was a major factor controlling surface fCO2 in the anticyclonic eddy, but such an effect was not observed in the cyclonic eddy. Surface fCO2 was influenced by the upwelling of high-fCO2 deep water in the non-eddy region and cyclonic eddy. In the northern Philippine Sea, surface fCO2 was strongly positively correlated with SST within cyclonic and anticyclonic eddies, while it showed no correlation in the non-eddy region. In the subtropical northeastern Pacific, in contrast, surface fCO2 showed a positive linear relationship with SST outside of a cyclonic eddy and a negative relationship with SST within the cyclonic eddy (Chen et al., 2007). This discrepancy was presumably related to different vertical gradients of temperature and DIC below the mixed layer in these two areas; such gradients drive the vertical transport of temperature and DIC. Therefore, the effects of mesoscale eddies on surface fCO2 were highly variable in the open ocean. To overcome this problem, more intensive surveys must be conducted across broad areas of the open ocean.
In the northern Philippine Sea, a cyclonic eddy caused surface fCO2 to increase because of the upwelling of high-fCO2 deep water, thereby increasing local sea-air CO2 flux, which was nearly twofold greater than the flux within an anticyclonic eddy. However, surface fCO2 and sea-air CO2 flux were not strongly affected by the anticyclonic eddy. Numerous researchers have attempted to estimate surface fCO2 and sea-air CO2 flux in the open ocean using the general surface fCO2 and SST relationship (Olsen et al., 2004; Park and Wanninkhof, 2012). However, in open-ocean areas where multiple eddies are present, sea-air CO2 flux estimated in this manner might be considerably underestimated within cyclonic eddies and the surrounding areas because cyclonic eddies increase CO2 flux due to the upwelling of high-fCO2 deep water. Therefore, estimation of sea-air CO2 flux using the general surface fCO2 and SST relationship should be avoided in the open ocean where cyclonic eddies occur.
The datasets presented in this study can be found in online repositories. The names of the repository/repositories and accession number(s) can be found below: https://doi.org/10.22711/idr/941.
DK, S-EL, and SC were responsible for data analysis and writing the manuscript. G-HP contributed with discussions and data analysis. D-JK and SSK performed a field survey and analyzed the data set. All authors contributed to the article and approved the submitted version.
This work was supported by the project PEA0012 of the Korea Institute of Ocean Science and Technology, and in part by the project entitled ‘Study on Northwestern Pacific Warming and Genesis and Rapid Intensification of Typhoon’, funded by the Ministry of Oceans and Fisheries (20220566)
The authors declare that the research was conducted in the absence of any commercial or financial relationships that could be construed as a potential conflict of interest.
All claims expressed in this article are solely those of the authors and do not necessarily represent those of their affiliated organizations, or those of the publisher, the editors and the reviewers. Any product that may be evaluated in this article, or claim that may be made by its manufacturer, is not guaranteed or endorsed by the publisher.
Andres M., Park J. H., Winibush M., Zhu X. H., Chang K. I., Ichikawa H. (2008). Study of the Kuroshio/Ryukyu current system based on satellite-altimeter and in situ measurements. J. Oceanogr 64 (6), 937–950. doi: 10.1007/s10872-008-0077-2
Bates N. R., Takahashi T., Chipman D. W., Knap A. H. (1998). Variability of pCO2 on diel to seasonal timescales in the Sargasso Sea near Bermuda. J. Geophys Res-Ocean 103 (C8), 15567–15585. doi: 10.1029/98jc00247
Chang Y. L., Oey L. Y. (2014). Instability of the north pacific subtropical countercurrent. J. Phys. Oceanogr 44 (3), 818–833. doi: 10.1175/jpo-d-13-0162.1
Chelton D. B., Schlax M. G., Samelson R. M. (2011). Global observations of nonlinear mesoscale eddies. Prog. Oceanogr 91 (2), 167–216. doi: 10.1016/j.pocean.2011.01.002
Chen F. Z., Cai W. J., Benitez-Nelson C., Wang Y. C. (2007). Sea Surface pCO2-SST relationships across a cold-core cyclonic eddy: Implications for understanding regional variability and air-sea gas exchange. Geophys Res. Lett. 34 (10). doi: 10.1029/2006gl028058
Gonzalez-Davila M., Santana-Casiano J. M., de Armas D., Escanez J., Suarez-Tangil M. (2006). The influence of island generated eddies on the carbon dioxide system, south of the canary islands. Mar. Chem. 99 (1-4), 177–190. doi: 10.1016/j.marchem.2005.11.004
Inoue H. Y., Matsueda H., Ishii M., Fushimi K., Hirota M., Asanuma I., et al. (1995). Long-term trend of the partial pressure of carbon dioxide (pCO2) in surface waters of the western north pacific 1984-1993. Tell Ser. B-Chem Phys. Meteorol 47 (4), 391–413. doi: 10.1034/j.1600-0889.47.issue4.2.x
Ishii M., Feely R. A., Rodgers K. B., Park G. H., Wanninkhof R., Sasano D., et al. (2014). Air-sea CO2 flux in the pacific ocean for the period 1990-2009. Biogeosciences 11 (3), 709–734. doi: 10.5194/bg-11-709-2014
Ishii M., Inoue H. Y., Matsueda H., Saito S., Fushimi K., Nemoto K., et al. (2001). Seasonal variation in total inorganic carbon and its controlling processes in surface waters of the western north pacific subtropical gyre. Mar. Chem. 75 (1-2), 17–32. doi: 10.1016/s0304-4203(01)00023-8
Mahadevan A., Levy M., Memery L. (2004). Mesoscale variability of sea surface pCO2: What does it respond to? Global Biogeochem Cycle 18 (1). doi: 10.1029/2003gb002102
Miyazawa Y., Kagimoto T., Guo X. Y., Sakuma H. (2008). The kuroshio large meander formation in 2004 analyzed by an eddy-resolving ocean forecast system. J. Geophys Res-Ocean 113 (C10). doi: 10.1029/2007jc004226
Moreau S., Della Penna A., Llort J., Patel R., Langlais C., Boyd P. W., et al. (2017). Eddy-induced carbon transport across the Antarctic circumpolar current. Global Biogeochem Cycle 31 (9), 1368–1386. doi: 10.1002/2017gb005669
Nelson N. B., Bates N. R., Siegel D. A., Michaels A. F. (2001). Spatial variability of the CO2 sink in the Sargasso Sea. Deep-Sea Res. Part Ii-Top Stud. Oceanogr 48 (8-9), 1801–1821. doi: 10.1016/s0967-0645(00)00162-4
Olsen A., Trinanes J. A., Wanninkhof R. (2004). Sea-Air flux of CO2 in the Caribbean Sea estimated using in situ and remote sensing data. Remote Sens. Environ. 89 (3), 309–325. doi: 10.1016/j.rse.2003.10.011
Orselli I. B. M., Kerr R., de Azevedo J. L. L., Galdino F., Araujo M., Garcia C. A. E. (2019). The sea-air CO2 net fluxes in the south Atlantic ocean and the role played by agulhas eddies. Prog. Oceanogr 170, 40–52. doi: 10.1016/j.pocean.2018.10.006
Parard G., Charantonis A. A., Rutgersson A. (2016). Using satellite data to estimate partial pressure of CO2 in the Baltic Sea. J. Geophys Res-Biogeosci 121 (3), 1002–1015. doi: 10.1002/2015jg003064
Park G. H., Wanninkhof R. (2012). A large increase of the CO2 sink in the western tropical north Atlantic from 2002 to 2009. J. Geophys Res-Ocean 117. doi: 10.1029/2011jc007803
Pierrot D., Neill C., Sullivan K., Castle R., Wanninkhof R., Luger H., et al. (2009). Recommendations for autonomous underway pCO2 measuring systems and data-reduction routines. Deep-Sea Res. Part Ii-Top Stud. Oceanogr 56 (8-10), 512–522. doi: 10.1016/j.dsr2.2008.12.005
Ramp S. R., Colosi J. A., Worcester P. F., Bahr F. L., Heaney K. D., Mercer J. A., et al. (2017). Eddy properties in the subtropical countercurrent, Western Philippine Sea. Deep-Sea Res. Part I-Oceanogr Res. Pap 125, 11–25. doi: 10.1016/j.dsr.2017.03.010
Sarmiento J. L., Gruber N. (2006). Ocean biogeochemical dynamics (New Jersey: Princeton University Press).
Sheu W. J., Wu C. R., Oey L. Y. (2010). Blocking and Westward passage of eddies in the Luzon strait. Deep-Sea Res. Part Ii-Top Stud. Oceanogr 57 (19-20), 1783–1791. doi: 10.1016/j.dsr2.2010.04.004
Song H., Marshall J., Munro D. R., Dutkiewicz S., Sweeney C., McGillicuddy D. J., et al. (2016). Mesoscale modulation of air-sea CO2 flux in drake passage. J. Geophys Res-Ocean 121 (9), 6635–6649. doi: 10.1002/2016jc011714
Stephens M. P., Samuels G., Olson D. B., Fine R. A. (1995). Sea-Air flux of CO2 in the north pacific using shipboard and satellite data. J. Geophys Res-Ocean 100 (C7), 13571–13583. doi: 10.1029/95jc00901
Takahashi T., Olafsson J., Goddard J. G., Chipman D. W., Sutherland S. C. (1993). Seasonal variation of CO2 and nutrients in the high-latitude surface oceans: A comparative study. Global Biogeochem Cycle 7 (4), 843–878. doi: 10.1029/93gb02263
Takahashi T., Sutherland S. C., Sweeney C., Poisson A., Metzl N., Tilbrook B., et al. (2002). Global sea-air CO2 flux based on climatological surface ocean pCO2, and seasonal biological and temperature effects. Deep-Sea Res. Part Ii-Top Stud. Oceanogr 49 (9-10), 1601–1622. doi: 10.1016/s0967-0645(02)00003-6
Takahashi T., Sutherland S. C., Wanninkhof R., Sweeney C., Feely R. A., Chipman D. W., et al. (2009). Climatological mean and decadal change in surface ocean pCO2, and net sea-air CO2 flux over the global oceans. Deep-Sea Res. Part Ii-Top Stud. Oceanogr 56 (8-10), 554–577. doi: 10.1016/j.dsr2.2008.12.009
Wanninkhof R. (2014). Relationship between wind speed and gas exchange over the ocean revisited. Limnol Oceanogr: Methods 12, 351–362. doi: 10.4319/lom.2014.12.351
Weiss R. F. (1974). Carbon dioxide in water and seawater: the solubility of a non-ideal gas. Mar. Chem. 2 (3), 203–215. doi: 10.1016/0304-4203(74)90015-2
Yaremchuk M., Qu T. D. (2004). Seasonal variability of the large-scale currents near the coast of the Philippines. J. Phys. Oceanogr 34 (4), 844–855. doi: 10.1175/1520-0485(2004)034<0844:Svotlc>2.0.Co;2
Yasunaka S., Kouketsu S., Strutton P. G., Sutton A. J., Murata A., Nakaoka S., et al. (2019). Spatio-temporal variability of surface water pCO2 and nutrients in the tropical pacific from 1981 to 2015. Deep-Sea Res. Part Ii-Top Stud. Oceanogr 169. doi: 10.1016/j.dsr2.2019.104680
Keywords: cyclonic eddy, anticyclonic eddy, sea-air CO2 flux, surface fCO2, philippine sea
Citation: Kim D, Lee S-E, Cho S, Kang D-J, Park G-H and Kang SK (2022) Mesoscale eddy effects on sea-air CO2 fluxes in the northern Philippine Sea. Front. Mar. Sci. 9:970678. doi: 10.3389/fmars.2022.970678
Received: 16 June 2022; Accepted: 25 July 2022;
Published: 11 August 2022.
Edited by:
Keisuke Nakayama, Kobe University, JapanReviewed by:
Andreey Andreev, V.I. Il’ichev Pacific Oceanological Institute (RAS), RussiaCopyright © 2022 Kim, Lee, Cho, Kang, Park and Kang. This is an open-access article distributed under the terms of the Creative Commons Attribution License (CC BY). The use, distribution or reproduction in other forums is permitted, provided the original author(s) and the copyright owner(s) are credited and that the original publication in this journal is cited, in accordance with accepted academic practice. No use, distribution or reproduction is permitted which does not comply with these terms.
*Correspondence: Dongseon Kim, ZGtpbUBraW9zdC5hYy5rcg==
Disclaimer: All claims expressed in this article are solely those of the authors and do not necessarily represent those of their affiliated organizations, or those of the publisher, the editors and the reviewers. Any product that may be evaluated in this article or claim that may be made by its manufacturer is not guaranteed or endorsed by the publisher.
Research integrity at Frontiers
Learn more about the work of our research integrity team to safeguard the quality of each article we publish.