- 1Center for Global Discovery and Conservation Science, Arizona State University, Tempe, AZ and Hilo, HI, United States
- 2Hopkins Marine Station and Emmett Interdisciplinary Program for Environment and Resources, Stanford University, Stanford, CA, United States
Turbidity from land-based runoff has been identified as a possible driver of coral bleaching refugia, as particulate matter in turbid habitats may block excessive irradiance and alter the food supply to corals during ocean heatwaves. However, negative effects of turbidity have also been documented worldwide, and high-resolution data across reef regions are required to identify habitats where turbidity is harmful or helpful during heatwaves. We monitored turbidity across five Main Hawaiian Islands during a 2019 ocean heatwave using Planet Dove satellites (3.7 m spatial resolution, extracted at 100 m resolution) to examine relationships between turbidity and coral bleaching occurrence and severity. We also attributed turbidity to urban and non-urban land uses to determine how turbidity associated with different human activities affects coral bleaching. We found that, while turbidity had a negative relationship with coral bleaching severity, this effect weakened at higher temperatures, especially in rural areas. However, turbidity maintained a slightly negative effect on bleaching severity in urban settings. In addition, while thermal stress was the predominant driver of bleaching occurrence, interactions between temperature and local variables like turbidity and wave energy were the most significant drivers of bleaching severity. This study represents one of the first high-resolution analyses of turbid refugia at large spatial scales. We show how new data sources from remote sensing can provide scalable yet context-specific information to coastal managers addressing land-sea runoff.
Introduction
Coral reefs are experiencing increased bleaching events worldwide, jeopardizing marine biodiversity, coastal infrastructure, and livelihoods in fishing and tourism (Burke et al., 2011; Osborne et al., 2017; Hughes et al., 2018). As ocean heatwaves intensify, additional research is needed to identify areas where corals can survive thermal stress. At the land-sea interface, nearshore runoff has been identified as a possible driver of coral refugia (Camp et al., 2018; Sully and van Woesik, 2020). The deposition of soils, sewage, and other particulate and dissolved matter from land to sea can alter water properties and impact coral bleaching in several important ways. First, suspended sediment may serve as a sunshade for light entering the water column, reducing ultraviolet light exposure and photoinhibition of corals during bleaching events (Morgan et al., 2017). In addition, turbidity is an indicator of dissolved organic matter, particulate organic matter, and zooplankton in the water column, which can provide corals with an alternative, heterotrophic pool of fixed carbon during bleaching events, compensating for their loss of photosynthesis (Grottoli et al., 2006; Anthony et al., 2007; Houlbrèque and Ferrier-Pagès, 2009). Given that turbidity has long been considered a coastal pollutant associated with sediment deposition and coral degradation from smothering, light limitation, water chemistry alterations, and other effects, further research is needed to determine if and where enabling conditions for refugia occur.
Numerous studies provide early evidence of turbidity effects on coral bleaching. A recent case study on the Great Barrier Reef found low bleaching in one turbid reef relative to regional bleaching means (Morgan et al., 2017). In a global study of turbidity patterns, Sully and van Woesik (2020) found that regions with remotely sensed turbidity of 0.080-0.127 Kd490 (attenuation coefficient at 490 nanometers observed from satellite sensors) underwent less bleaching during thermal events than areas with minimal turbidity. Cacciapaglia and van Woesik (2016) likewise estimated that turbidity offsets bleaching in 9% of reef habitat threatened by ocean warming in the Indian and Pacific Oceans. In the Florida Keys, reefs in turbid, inshore waters exhibited higher coral cover, higher growth rates, and lower partial mortality rates than offshore reefs (Lirman and Fong, 2007). A bleaching survey in Palau showed lower bleaching incidence inshore compared to offshore, perhaps due to coral shading from land-based runoff inshore (van Woesik et al., 2012); however, this study treated coral proximity to shore as a proxy for turbidity, and other confounding variables may protect corals from bleaching nearshore such as variable temperature at shallow depths (Safaie et al., 2018).
There is disagreement on the effects of turbidity, with some case studies and experiments showing severe bleaching in highly sedimented reefs. Surveys in northwestern Australia and Okinawa Island, Japan demonstrated steep declines in coral cover due to thermal stress on nearshore reefs with heavy runoff (Hongo and Yamano, 2013; Lafratta et al., 2017). On Palmyra Atoll, turbidity was the strongest predictor of bleaching across all taxa, outweighing thermal anomalies (Williams et al. (2010). Paling and bleaching has also been observed in corals exposed to low light under realistic dredging conditions (<0.1 mol photons m−2 d−1 for 10 d) (Bessell-Browne et al., 2017a). Sediment deposition after bleaching (e.g., from dredging) may exacerbate mortality, as corals have reduced sediment-rejection ability after thermal stress (Bessell-Browne et al., 2017c), and sediment cleaning and/or low light can also reduce coral lipid stores needed to survive bleaching (Fisher et al., 2019). Sediment may also inhibit heterotrophy if excessive sediment causes polyps to retract or otherwise reject particulates (Mills and Sebens, 1997; Anthony, 2000; Jones et al., 2016). In addition, the nutritive benefits of turbidity during bleaching may be limited to certain species like Montipora capitata and Goniastrea retiformis, which show higher heterotrophy than Porites compressa in tank experiments (Grottoli et al., 2006), and heterotrophy may decline with repeat bleaching (Levas et al., 2016).
Suspended sediment is a known driver of reef degradation. Effects of suspended sediments include, but are not limited to, polyp smothering, excessive light limitation, increased coral competitors like macroalgae and filter feeders, and changes in the oxygenation and chemical environment of corals (Fabricius, 2005; Brodie et al., 2012; Jones et al., 2016; Jones et al., 2020; Carlson et al., 2021). Foliose and encrusting corals may be more susceptible to sediment smothering than other, branching species (Jones et al., 2019) as depressions in the colony structure accumulate sediment, and pocilloporid species are sensitive to low light conditions (Jones et al., 2020a; Luter et al., 2021). Sediment may also induce a suite of coral reproductive impairments including disruption of light-based spawning cues, failed surface ascension of egg-sperm bundles due to sediment blocking, reduced coral recruitment, and increased post-settlement mortality (Jones et al., 2015; Brunner et al., 2022). Recent studies indicate that light reduction, relative to sediment itself, is a strong driver of coral loss; in one experiment, corals treated with suspended sediment absent light mitigation displayed no significant change, whereas corals treated with low light exhibited decreased chlorophyll a and increased bleaching (Bessell-Browne et al., 2017b). In addition, turbidity often covaries with high nitrate input, which has been found to exacerbate bleaching severity at low levels of temperature stress (Fabricius, 2005; Donovan et al., 2020).
Inconsistencies among past studies on turbidity effects may also arise from inconsistent sources of turbidity, which is a light-based rather than biochemical metric. Turbidity can arise from multiple sources, ranging from dissolved organic matter to silt and marine snow. Particulate matter varies by grain size, color, organic content, and other properties which could have differential impacts on corals. For example, Storlazzi et al. (2015) found that light attenuation is highest when sediment has a fine grain size and darker color; however, fine sediment is also most persistent and likely to create a “light rain” of particles in the water column that is harmful to corals (Erftemeijer et al., 2012). Turbidity may be comprised of different fractions of dissolved organic matter, detrital particulate organic matter, zooplankton, phytoplankton, and pico- or nano-plankton, which provide variable food quality to corals (Ferrier, 1991; Hoegh-Guldberg and Williamson, 1999; Houlbrèque and Ferrier-Pagès, 2009). For example, pico- and nano-plankton provide three times more nitrogen to Stylophora pistillata, relative to dissolved nitrogen (Grover et al., 2002, 2003), and corals have shown preferential feeding on zooplankton in tank experiments (Palardy et al., 2008). Finally, turbidity may be associated with different levels of toxins, where agrochemicals (fertilizers, pesticides, herbicides) are more likely to originate from rural areas and golf courses, and petroleum and medical waste from urban areas. Urban environments can give rise to distinctive “marginal reefs” where corals display unique species assemblages, habitat preferences, reproductive strategies, heterotrophic behavior, and bleaching response, perhaps reflecting specific interactions between urban runoff and local taxa (Camp et al., 2018; Heery et al., 2018).
Past research has been limited in scale, based primarily on case studies that may not represent coral reefs in other regions. A few, global studies have used NASA MODerate-resolution Imaging Spectroradiometer (MODIS) Aqua satellite data to measure the link between turbidity and bleaching (Cacciapaglia and van Woesik, 2016; Sully and van Woesik, 2020); however, these data are too coarse (4 km resolution) to capture many shallow, nearshore features that carry turbidity onto reefs, particularly as 4 km pixels overlapping the coast contain signal from both ocean and land and therefore cannot be used to detect turbidity. In fact, 4 km represents the maximum transport edge for very fine sediment (maximum 3-5 km offshore in previous literature) (Bartley et al., 2014), and this resolution is likely inadequate to characterize variable coastal flux. As a result of these limitations, past studies have not compared different sources of turbidity to determine if certain types of turbidity are more favorable to corals in terms of bleaching than others. Coastal managers require a more detailed understanding of potential turbid refugia in order to address harmful and helpful sources of runoff.
We addressed these gaps by using turbidity data extracted from high-resolution Planet Dove satellite imagery (3.7 m source imagery extracted at 100 m) to measure the effect of turbidity on coral bleaching during a moderate bleaching event in the Hawaiian Islands. We also investigated whether turbidity from predominantly urban versus undeveloped/rural areas have a differential effect on coral bleaching. We applied an algorithm specifically designed to track turbidity in shallow, nearshore waters (Li et al., 2022), demonstrating how advances in the spatial and temporal resolution of satellite data can help managers access actionable information.
Materials and methods
The Hawaiian archipelago is the longest contiguous island chain in the world, arising from periodic volcanic activity on the Pacific lithospheric plate over the past 27.7 million years (Juvik and Juvik, 1998). Due in part to stratification in volcanic substrate, Hawaiʻi represents a “model ecosystem” where a range of habitat conditions can be studied across a relatively small area (Vitousek, 1995). Coral reefs in Hawaiʻi exist along gradients of temperature, solar radiation, nutrients, oceanography, substrate ages, bathymetry, and wind and wave shelter (Grigg, 1983) and experience variable human development and access. Hawaiʻi therefore offers an opportune context for comparing coral health across taxonomic, light, and turbidity regimes, in waters marked by different levels of human sewage and volcanic silt.
We compared remotely sensed turbidity to coral bleaching occurrence (presence/absence of bleaching) and severity (% bleached of live coral cover) across five Main Hawaiian Islands (Hawaiʻi, Maui, Oʻahu, Lānaʻi, Molokaʻi) during a prolonged heat stress event from August through December 2019. During this event, sea-surface temperature anomalies (SSTa) exceeded 2 degrees Celsius and SST surpassed 29 degrees Celsius (Figure 1), causing mild to severe bleaching in over 50% of corals statewide (Winston et al., 2020).
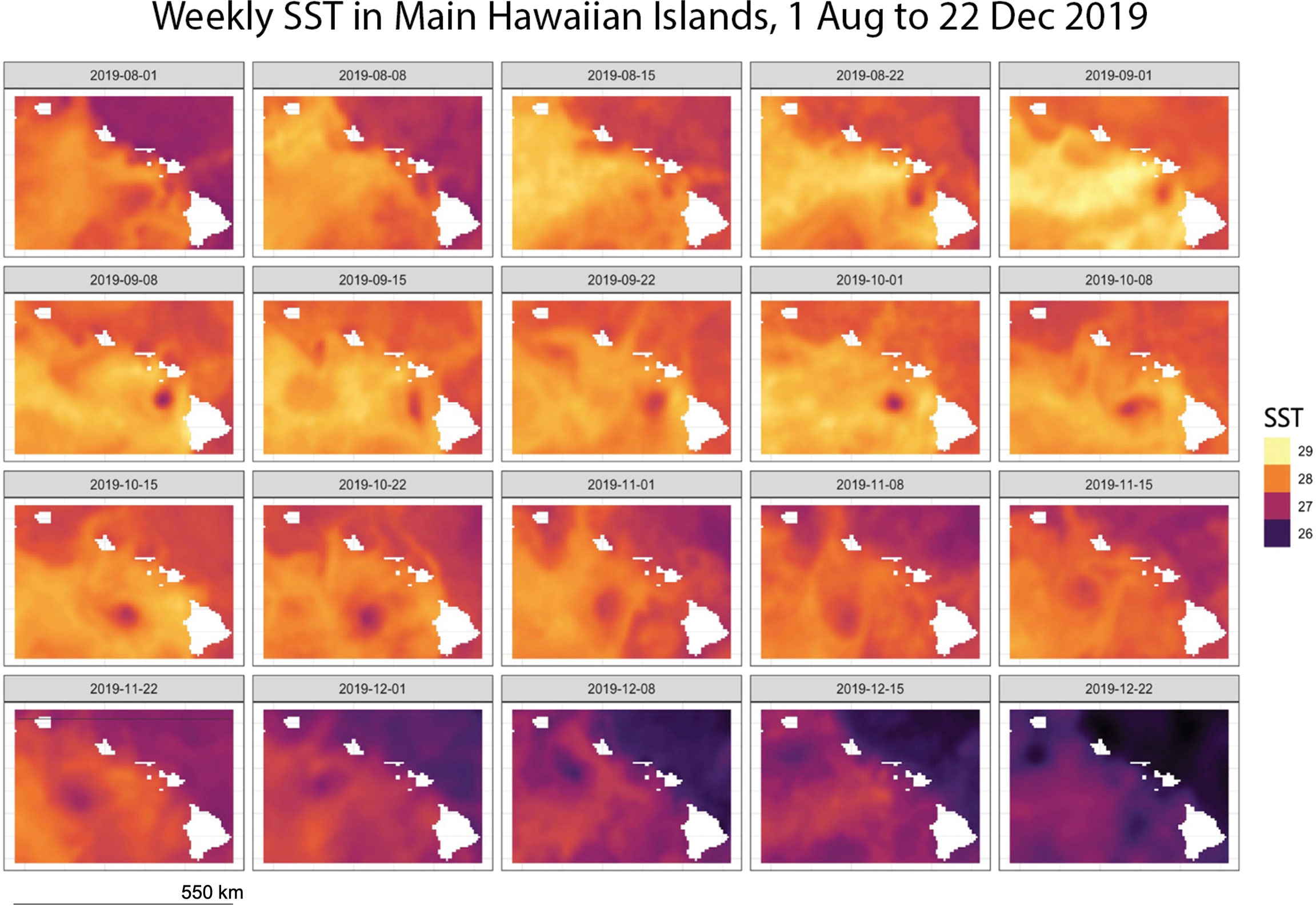
Figure 1 SST (°C), Main Hawaiian Islands, 01 August 2019 – 22 December 2019 aggregated from NOAA Coral Reef Watch (2019).
Coral surveys
Data from 1,024 bleaching surveys across the Main Hawaiian Islands were obtained from the Hawaiʻi Coral Bleaching Collaborative (Hawaiʻi Coral Bleaching Collaborative, 2021), a monitoring partnership created in response to the 2019 Hawaiʻi coral bleaching event and comprised of NOAA Fisheries Ecosystem Sciences Division; State of Hawaiʻi Department of Land and Natural Resources, Division of Aquatic Resources; Scripps Institution of Oceanography; Arizona State University; National Park Service, Pacific Island Inventory & Monitoring Network; Hawaiʻi Institute of Marine Biology; and The Nature Conservancy of Hawaiʻi. Survey and site selection methods differed slightly across organizations, and thus we included organization as a random factor in our analysis (see “Data Analysis” below). Coral bleaching (% bleaching of live coral cover) and % live coral cover were measured using either a rapid visual assessment or line transects (10, 25, or 30 m depending on the organization), and transects were quantified using non-overlapping photoquadrats analyzed through CoralNet, or a line-intercept method. Some islands were monitored using ordinal bins (bleaching levels 1-5), but we elected to use only continuous (% bleaching) data to achieve a more detailed metric, which limited our analysis to five islands: Hawaiʻi, Maui, Oʻahu, Lānaʻi, and Molokaʻi (Figure 2A). Each survey represents a single visit to a distinct location (“site”); some locations were spaced closer together than others (see discussion of spatial autocorrelation below). While surveys included some taxon-level data, these data were not available for all locations, and we therefore did not analyze bleaching by taxon. We also decided not to analyze taxa because when several different survey techniques are used, as in this dataset, species records can vary widely between survey techniques, while % coral cover is more comparable between survey methods (Jokiel et al., 2015). Depth was recorded for all sites but was missing for 10 observations; in these cases, we imputed depth from aircraft-based bathymetry from the Global Airborne Observatory (Asner et al., 2020a).
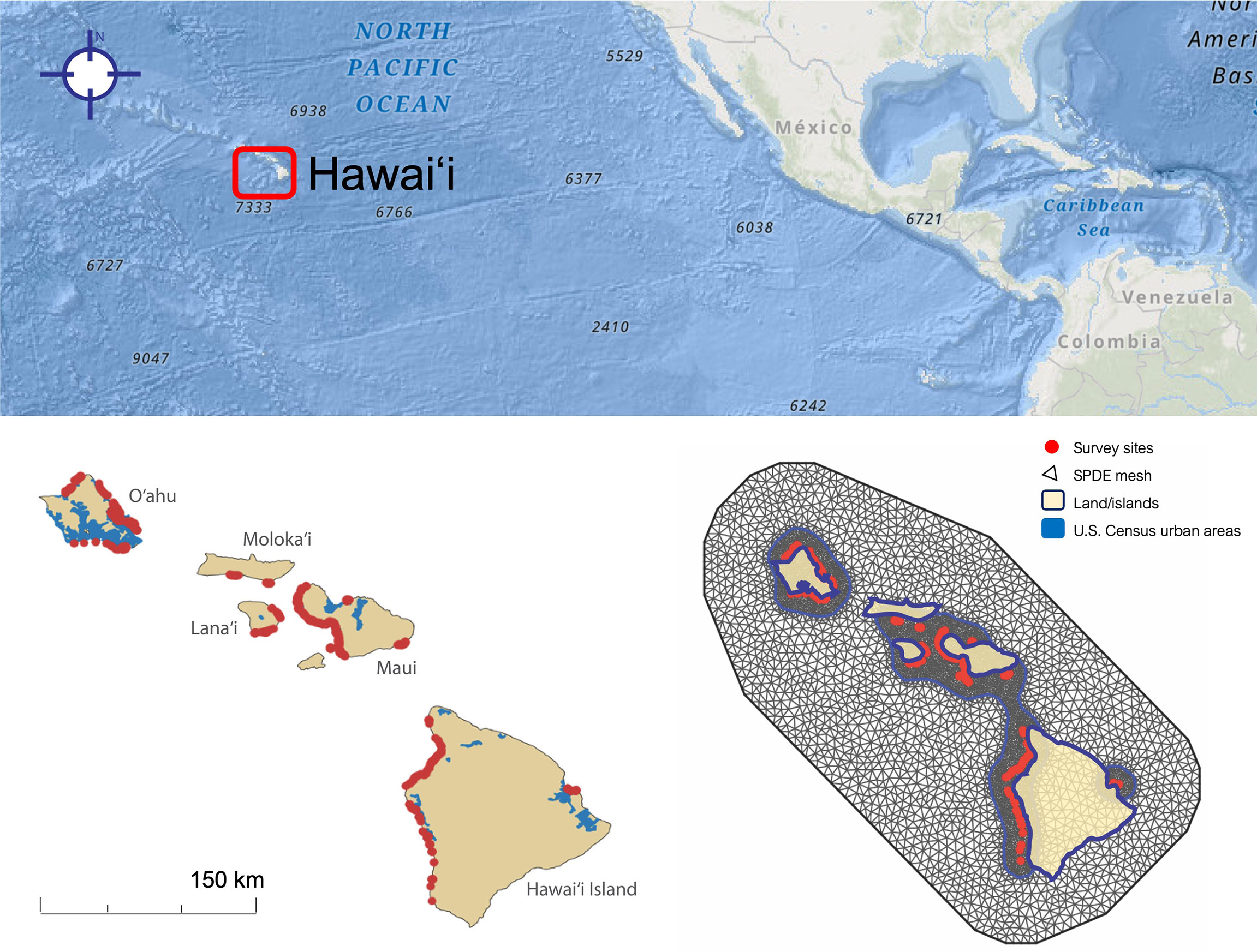
Figure 2 Coral bleaching site distribution (n = 1024) in five Main Hawaiian Islands. (Left) Observation sites for coral bleaching surveys (red) shown alongside urban areas (blue). (Right) Spatial mesh for modeling site spatial structure and spatial autocorrelation.
Turbidity
We extracted turbidity values from each site using a recently developed Shallow Water Turbidity (SWaT) method, which is designed to detect turbidity in nearshore shallow waters using the Planet Dove satellite constellation (3.7 m spatial resolution) (Li et al., 2022). SWaT accounts for bottom reflectance in total water-leaving radiance, and therefore improves upon historical remote-sensing turbidity algorithms, which do not address bottom reflectance and therefore may overestimate turbidity values in clear, shallow, nearshore waters where bottom reflectance is high (Lee et al., 2012). SWaT was previously validated against field turbidity monitoring data from the Pacific Islands Ocean Observing System and United States Geological Service, exhibiting an accuracy of R2 = 0.83 and RMSE = 0.90 FNU (Li et al., 2022). We extracted weekly mosaics of Planet imagery for all weeks during the bleaching event (28 July 2019 to 1 December 2019) and used bathymetry processed from Sentinel-2 data (Li et al., 2021) to subtract depth-dependent bottom reflectance from water-leaving radiance. This produced water column reflectance, i.e., the in-water turbidity signal. We then used the red band of water column reflectance to derive turbidity in Formazin Nephelometric Units (FNU) based on Dogliotti et al. (2015).
We found the maximum turbidity value within a buffer of 100 m of each survey site, which was the lowest buffer that both maximized data availability while remaining appropriate to the scale of particle mixing. We used turbidity maxima because sediment runoff often occurs in sporadic events and, therefore, turbidity regimes are well-characterized by peaks (De Carlo et al., 2007). In addition, because the SWaT algorithm subtracts bottom effects it can over-correct for bottom reflectance (underestimate turbidity) in extremely shallow areas directly abutting the coast, and therefore, nearshore surveys were more accurately represented by maxima. A visual inspection showed limited evidence of artificial outliers in imagery (discrete, extreme turbidity values) near survey points, and we found high (> 0.70) Pearson's correlation between turbidity calculated from maximum and mean values, so determined that using maxima was appropriate. All analysis was conducted using Python and the gdal library on Linux command line.
To attribute turbidity to urban sources, we created a binary variable for urban coastline using a dataset of ‘urbanized areas and urban clusters’ from the U.S. Census Bureau (2015) TIGER database. Survey points were snapped to the nearest coastline and considered urban if listed as ‘urban’ by the Census; each survey point was then manually checked against true-color satellite imagery to ensure that classification based on 2015 Census data was still accurate.
Environmental covariates
We controlled for additional bleaching drivers determined from prior literature: thermal stress, sea-surface irradiance (hereafter “irradiance”), wave power, total effluent, nitrogen runoff, depth, and distance from shore. We also examined % live coral cover as a possible confounding variable, given that high coral cover may indicate low prior bleaching and, perhaps, low vulnerability.
We derived thermal stress as both 1) SSTa and 2) Degree-Heating Weeks (DHW) from NOAA Coral Reef Watch Operational Daily Near-Real-Time Global 5-km Satellite Coral Bleaching Monitoring data (2019), obtained via ERDDAP (Simons, 2020). Since coral survey dates were inconsistent, ranging from 20 August 2019 to 7 December 2019, we extracted daily thermal data between a start date of 1 August 2019 (1 week before the Main Hawaiian Islands mean SST surpassed the regional bleaching threshold of 28.5° C) and each unique survey date. SSTa was measured as the mean daily SSTa for that period, and DHW as cumulative DHW at the survey date. SSTa and DHW were linearly related, so we selected only SSTa for analysis. We measured irradiance as the maximum Photosynthetically Available Radiation (PAR, mol m-2 day-1) at each site during the bleaching event, derived from the MODIS Aqua satellite (NASA, 2019), which has a maximum spatial resolution of 4 km. As with temperature, we accounted for variable survey dates by fetching data between 1 August 2019 and each individual survey date. We then calculated PAR as the maximum pixel value at each point from eight-day PAR composites.
‘Wave power’ (kW m-2) was the long-term mean of wave power (2000-2013) at each site, obtained from Kappel et al. (2017a). In this dataset, long-term means were based on hourly wave data from a SWAN (Simulating WAves Nearshore) model, which were converted to maximum daily wave power, then averaged by month from 2000-2013. While these data precede 2019, they represent the best available data for our study region and serve as a relative index of wave exposure between sites. ‘Total effluent’ (gal km-2 d-1) was likewise derived from Kappel et al. (2017b), based on effluent flux from Onsite Sewage Disposal Systems (OSDS). In this dataset, OSDS flux was attributed to Tax Map Key (TMK) parcels (Whittier and El-Kadi, 2014), which were aggregated into 500-m pixels and added as total flux within a 1.5-km radius of each cell. ‘Nitrogen runoff’ (gal km-2 d-1) was obtained from the same dataset.
Finally, we found ‘distance from shore’ as the orthogonal distance between each point and the nearest landmass. All geospatial processing was conducted using the sf package in R (Pebesma, 2018) and visualized in QGIS. Final independent variables for analysis were turbidity, urban area (binary), SSTa, distance from shore, depth, total effluent, nitrogen runoff, live coral cover, wave power, and irradiance (Table 1). We also tested interactions between all variables and SSTa, and the three-way interaction of turbidity, urban area, and SSTa given our interest in the source (urban v. non-urban) of turbidity.
Data analysis
We used a Bayesian hierarchical spatial model to estimate the effect of turbidity and other environmental variables on coral bleaching. Given that our dataset contained a high number of zeros (18% of data showed no bleaching), we used a Zero-Altered approach (Zuur and Ieno, 2016) where bleaching was modeled first as a binary outcome of ‘bleaching occurrence’ (whether bleaching was present/absent in the survey), and data was then subset to only nonzero bleaching surveys to assess ‘bleaching severity’ (% bleaching of live coral cover). A Bernoulli distribution was used to fit bleaching occurrence and a beta distribution for bleaching severity, as these distributions are best suited to, respectively, presence-absence and proportional data (Zuur and Ieno, 2016). Bleaching was modeled using logit link functions as:
where and are bleaching occurrence and severity, E denotes fitted bleaching values, Xi is a covariate matrix of independent variables, β is a vector of regression parameters with α intercepts, μ is the mean of the beta component, π is the probability of bleaching absence vs. presence, ϕ summarizes shape parameters for the beta distribution, and Wi represents the models’ spatial structure, described below.
We standardized all continuous independent variables by subtracting the mean from each observation and dividing by the standard deviation. Beta distributions only take proportional data < 1, so we converted our response variable where Yi = 1 to negligibly lower values using Yi = (Yi * (N-1) + 0.5)/N, following Zuur and Ieno (2016). We assessed all variables for collinearity using Pearson’s correlation index; a correlation higher than 0.70 existed between ‘nitrogen’ and ‘total effluent’ variables (p = 0.98, P < 0.001) so we retained only ‘total effluent’ for analysis (Figure S1). We also used Point Biserial Correlation to test for correlation between categorical and continuous independent variables and confirmed that all p < 0.70. Preliminary plots of independent variables (Figure S2) indicated high positive skewness in distance from shore, depth, and wave power. Distance from shore was log-transformed, which is advised when distributions display extreme positive skewness, and wave power and depth (milder skew) were square-root-transformed. We also removed two outliers in total effluent, which represented exceptionally high values (Figure S2). Outlier removal and variable transformations had no impact on model fit > 2 DIC but improved homoskedasticity in residual-covariate plots (Figure S3).
A variogram of Pearson’s residuals in initial models indicated that spatial autocorrelation existed in our dataset. We therefore modeled a spatial random effect using Stochastic Partial Differential Equations (SPDE) based on a Matern variance-covariance matrix (Lindgren and Rue, 2015; Zuur et al., 2017). To drive SPDE, we created a mesh (Figure 2B) with a nonconvex hull (convex = -0.05, concave = 0.05), which is best suited to well-separated islands, and specified maximum node distance between 1 and 10 km. We used a histogram of between-plot distances to determine range and sigma priors of, respectively, 5 km and 1 km, at 0.05 probability. We also conducted a sensitivity analysis of node distances, ranges, and sigmas, and found that variable influence was not substantially changed by priors.
To account for slight differences in survey method by organization, we included ‘organization name’ (identity of the organization collecting data) as a random factor in our analysis. Random effects are appropriate when correlation is expected between observations in the same group (organization), but when the relationship between independent and dependent variables is still expected to carry across all group levels (Harrison et al., 2018). Since we did not expect ‘organization’ to change the environment-bleaching relationship, but produce variable intercepts, a random factor was appropriate. We also included island as a fixed effect, given that islands represent different oceanographic, geomorphological, and human development regimes on reefs.
Final variables in the model were determined through backward stepwise selection, where we removed variables that did not increase model fit by 2 DIC, i.e., when only influential variables remained (Table S1-S2). We tested for homogeneity of variance by comparing residuals to all covariates, and to fitted values (Figure S3A–H). We also tested for normality of residuals (Figure S3I), and compared fitted to observed values, visually determining that the relationship was linear but not overfitted. Following Donovan et al., 2020; 2021), we considered variables influential if their 80% credible interval did not cross zero, but also assessed the 95% credible interval to identify highly influential variables (note: frequentist p-values do not apply in Bayesian statistics, but we will use the term “significant” to refer to these influential variables, for the sake of interpretability). We used penalized complexity priors for random effects following Fuglstad et al. (2019); default vague Gaussian priors for fixed effects (mean = 0, precision = 0.001), and log-gamma priors for hyperparameters (mean = 1, precision = 0.1). All analysis was conducted using the R-INLA (Rue et al., 2009).
Results
Bleaching levels varied widely between surveys, with 18% of surveys (184 of 1024) showing no bleaching, and 25% of sites showing between 30-100% bleaching. Mean SSTa experienced by corals before survey date ranged between 0.6 and 1.4° C (Table 1). Turbidity values were evenly distributed across rural and urban areas, ranging from 0.1 FNU to 21.3 FNU (Figure 3). In addition, turbidity was not correlated with distance from shore (ρ = 0.23, P < 0.001), likely because 1) most surveys occurred within a short distance from shore (50th percentile of surveys < 200 m from shore) where strong sediment advection/mixing is possible, 2) resuspension of bottom sediments may be variable nearshore (Jokiel et al., 2014), and/or 3) our turbidity metric was derived from a 100-meter buffer, so points 200 m apart may have had similar turbidity values. Survey regions with the highest turbidity included Honolulu and Kāneʻohe Bay, South Molokaʻi, and Maui from Olowalu to Lāhainā and Kahului (Figure 3), while the region with the lowest turbidity was West Hawaiʻi. However, turbidity varied over fine (< 500 m) scales and was generally highest near harbors, airports, and refineries; wastewater outfalls; erosive roadsides; and steep hillsides.
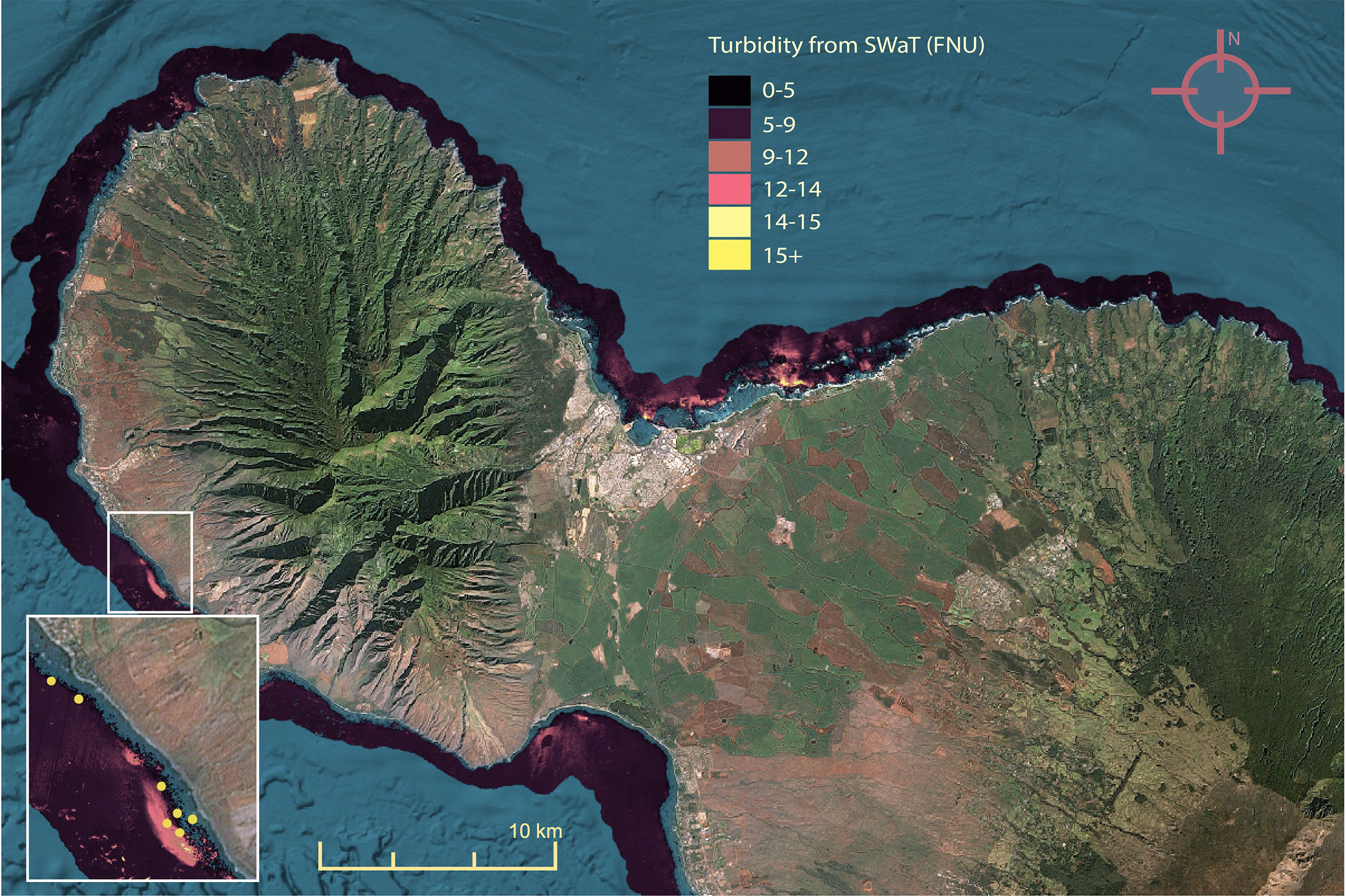
Figure 3 Maximum weekly turbidity (FNU) measured by Planet Dove satellites for bleaching period (28 July 2019 to 1 December 2019), magnified to Maui for detail. Inset: turbidity plume near Olowalu, HI overlaid with survey locations (yellow).
The model of best fit for bleaching occurrence included SSTa, live coral cover, turbidity, urban area, distance from shore, and the interactions between SSTa-live coral cover, SSTa-urban area, SSTa-turbidity, and SSTa-urban area-turbidity (three-way interaction). For bleaching severity, the model of best fit included SSTa, turbidity, urban area, distance from shore, depth, wave power, live coral cover, and the interactions between SSTa-wave power, SSTa-urban area, SSTa-turbidity, and SSTa-urban area-turbidity (three-way interaction). All final models also included island.
Turbidity
Turbidity was a negative driver of bleaching severity (80% credible interval -0.195 < β < -0.049), and thus, turbidity decreased percent bleaching in live coral cover (Figure 4B). In contrast, turbidity did not affect bleaching occurrence (binary variable of bleaching presence/absence) (Figure 4A). The interaction between turbidity and heat stress (SSTa) was positive for both bleaching occurrence (0.098 < β < 1.121) and severity (0.139 < β < 0.373). Thus, thermal stress suppressed the protective effect of turbidity on bleaching severity, i.e., turbidity had a weaker effect at higher temperatures (Figure 5). In urban areas, turbidity had a largely flat effect on bleaching severity, though in non-urban areas, the relationship between turbidity and bleaching severity reversed to weakly positive (interactive effect of urban turbidity -0.480 < β < -0.143). Urban turbidity also had a negative effect on bleaching occurrence (-1.137 < β < 0.462), but this effect was not significant (Figure 4A). Urban areas, when treated in isolation without interaction, had a highly positive effect on bleaching occurrence (0.499 < β < 1.894) and no effect on bleaching severity. Therefore, the urban environment alone did not create a protective effect on corals during the bleaching event, but the presence of urban turbidity may have indicated higher coral resistance or resilience to high temperatures (note: covariates were standardized; see Table S3 for β relative to standard deviations).
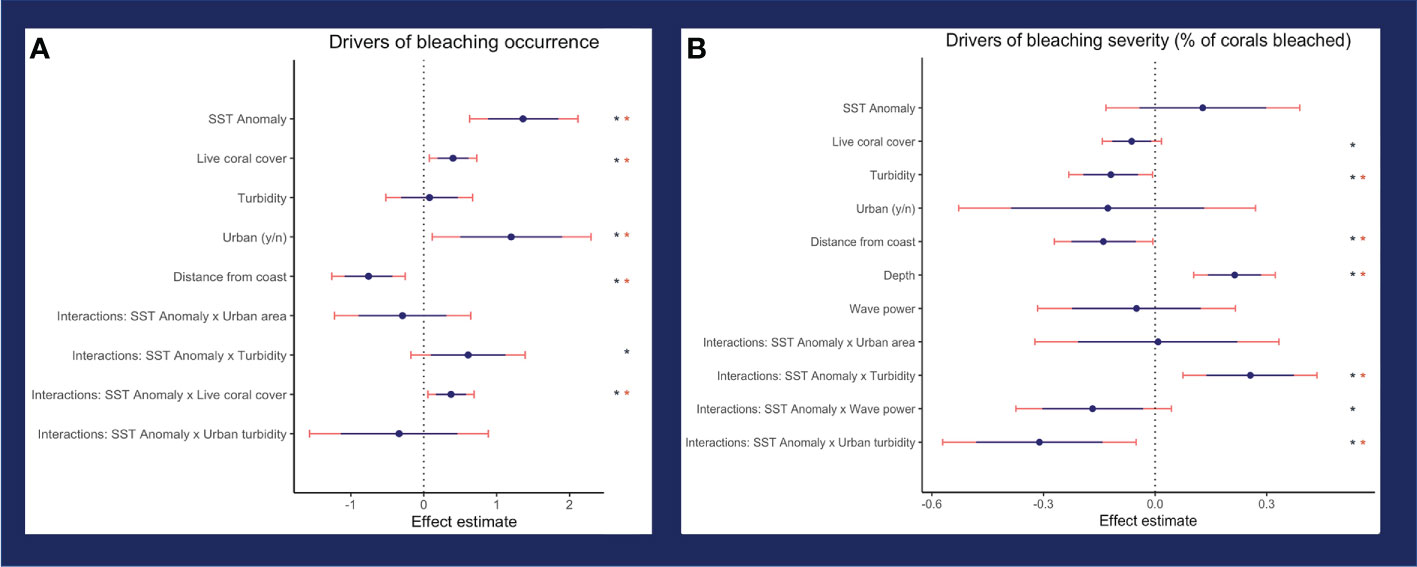
Figure 4 Effect of independent variables on (A) bleaching occurrence (binary variable for whether bleaching is present or absent) and (B) bleaching severity (% bleaching cover where bleaching is nonzero). Thin, pink lines show the 95% credible interval, and thick, blue lines show the 80% credible interval. One asterisk indicates significant variables (80% interval does not cross zero) and two asterisks indicate highly significant variables (95% interval does not cross zero).
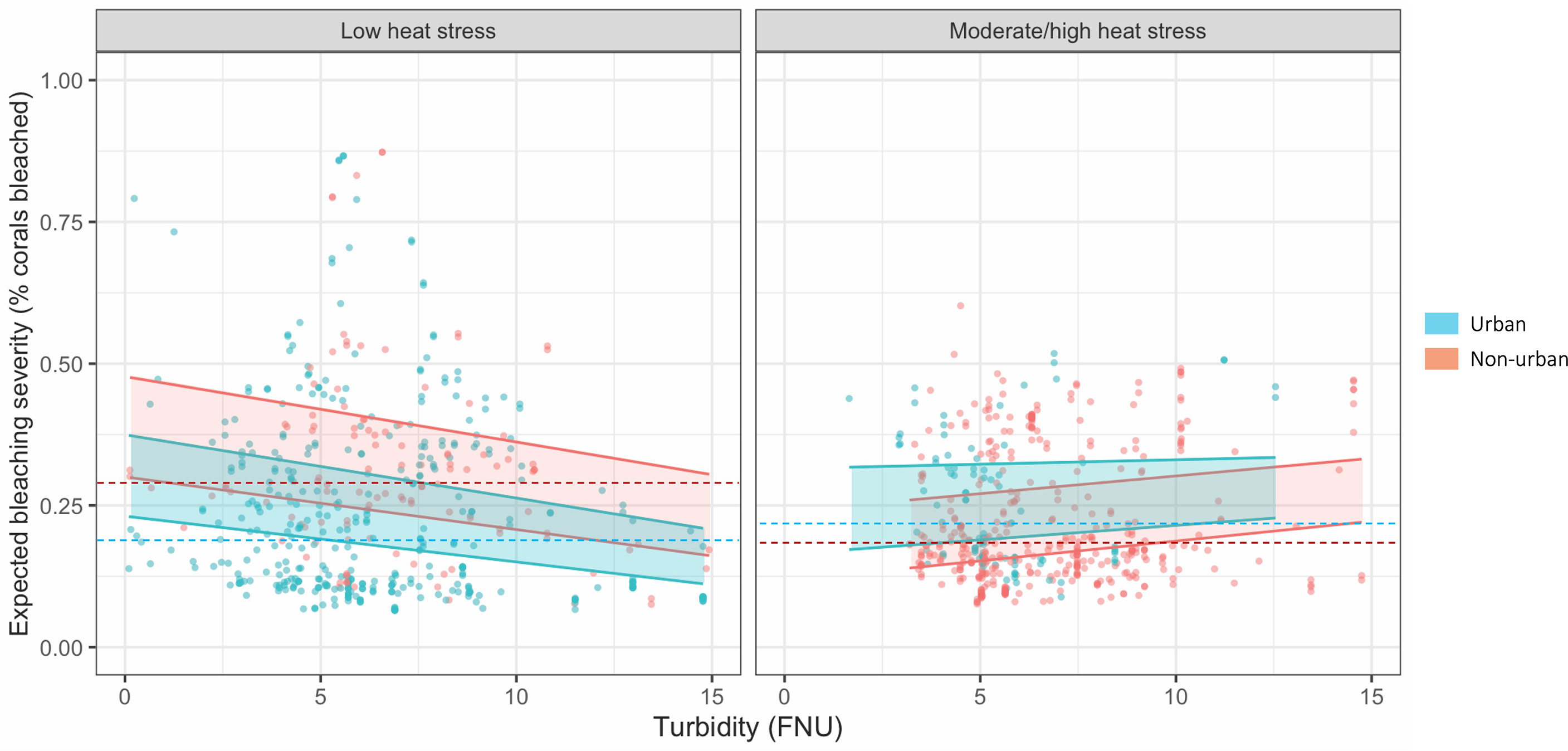
Figure 5 Change in bleaching severity with increasing turbidity (unstandardized) under low and high heat stress. Heat stress is divided as low <= 1.15° C SSTa and high >1.15° C SSTa, based on the 50th percentile of SSTa data. Points represent each observation in the dataset with turbidity mapped against the mean posterior estimate of bleaching severity. Bands represent fitted 80% credible interval posterior estimates for (blue) urban sites, i.e., U.S. Census-listed urban coastline, and (red) non-urban sites. Dotted lines represent median observed bleaching severity for each category. Note: In low heat stress areas, there are n = 118 non-urban and n = 408 urban sites; in moderate/high heat stress areas, there are n = 405 non-urban and n = 91 urban sites; urban areas and heat stress are not correlated > p = 0.70 (see Supplementary Material).
Other environmental drivers
SSTa had a positive effect on bleaching occurrence and severity, though this effect was highly significant (0.886 < β < 1.848) for occurrence only. Therefore, SSTa was a major driver of whether corals bleached, but not specific levels of bleaching. In contrast, bleaching severity showed a significant dependence on the interactions between temperature and other environmental covariates such as turbidity, urban turbidity, and wave power (Figure 6; Figure S4). The interaction between wave power and SSTa had a negative effect on bleaching severity (-0.282 < β < -0.016) and therefore wave action reduced bleaching levels under increasing thermal stress.
Beyond temperature, variables associated with bleaching occurrence were % live coral cover (0.189 < β < 0.613) and distance from shore (-1.087 < β < -0.430). Areas with higher live coral cover showed a higher propensity to bleach, though this effect was weak. Corals located further from the coast were more likely to show low to no bleaching. In contrast, % live coral cover had a weakly negative relationship with bleaching severity (-0.113 < β - 0.009), as increasing live coral cover was correlated with a slight decrease in bleaching severity. Bleaching severity was higher in deeper reefs (0.145 < β < 0.286). Sea-surface irradiance was not a significant variable for either bleaching occurrence or severity.
Including island as a fixed effect dramatically reduced model DIC values, and therefore island had a strong influence on bleaching occurrence and severity. With the Big Island as a reference value, all islands showed higher bleaching occurrence and severity, although Oʻahu showed the strongest, positive effect, and Lānaʻi the weakest effect on both bleaching metrics (Figure 6). In addition, including organization name (identifier for the organization conducting surveys, which was a proxy for survey method) as a random effect substantially reduced model DIC values (-40 DIC) and thus increased model fit (Table S1–2).
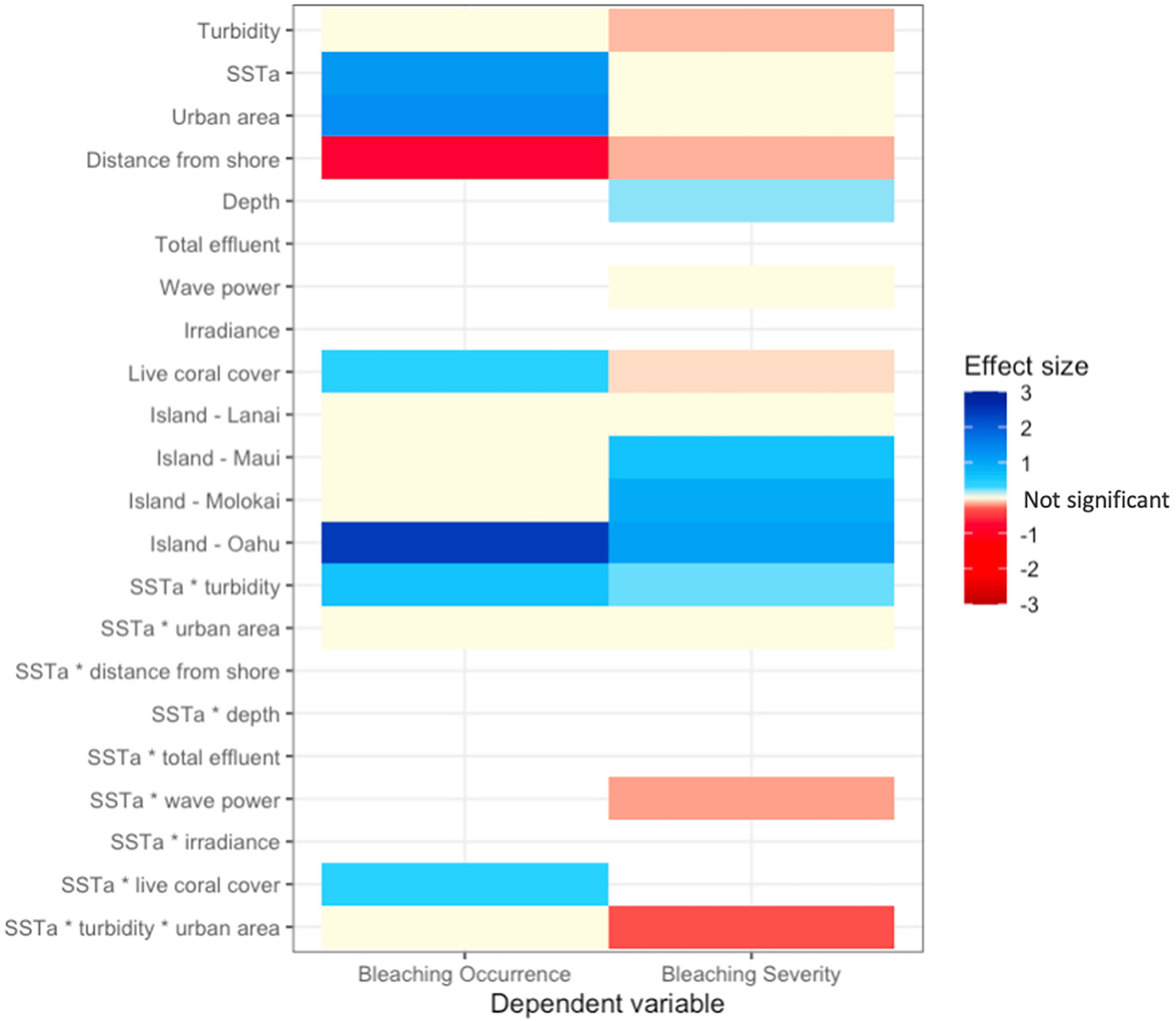
Figure 6 Heatmap of effect sizes for independent variables in final models for bleaching occurrence (left column) and severity (right column).
Discussion
Turbidity
Turbid coral bleaching refugia have been documented worldwide, but in contrast, so have heavily damaged reefs under severe sedimentation, toxin exposure, effluent, dredging, and other turbid-area stressors (Brodie et al., 2012; Cacciapaglia and van Woesik, 2016; Carlson et al., 2019; Sully and van Woesik, 2020). By coupling high-resolution remote sensing with in-situ field observation, we demonstrate that turbidity may provide protection from bleaching severity but increasing temperature stress may weaken or reverse this effect, particularly in rural areas. Across the Main Hawaiian Islands, turbidity ameliorated bleaching severity overall, but thermal stress counteracted this effect, as indicated by a positive turbidity-SSTa interaction. In rural areas in particular, turbidity failed to improve coral bleaching under increased SSTa, while in urban environments, turbidity still mildly suppressed bleaching despite climbing temperatures (Figure 5). This pattern persisted when bleaching was measured as bleaching occurrence (presence/absence): turbidity decreased bleaching occurrence, though this relationship weakened as temperatures rose, especially in rural areas. Unlike for bleaching severity, these effects were not significant (80% credible interval crossed 0), likely because bleaching occurrence was overdetermined by SSTa, which was by far its most significant driver.
These findings suggest that temperature can override the protective benefits of turbidity, but that marginal reefs (chronically silty, urban areas) may have unique features that confer resilience. One possible reason for this pattern is that urban, turbid areas in Hawaiʻi may contain stress-tolerant corals. Urban hubs in Hawaiʻi have experienced prolonged disturbance, where surviving corals may have been selected over decades of intensive human contact. Stress-tolerant communities are common in urban, turbid areas, and have been observed in Singapore, Brazil, and Australia (Mora, 2008; Guest et al., 2016a; Heery et al., 2018; Loiola et al., 2019). In these regions, weedy or stress-tolerant communities have survived and/or adapted to urban trends like early grazer removal, heavy metal deposition, macroalgal proliferation, artificial habitat from urban structures, and genetic isolation (or, conversely, novel corridors) along the built coastal form (Goodkin et al., 2011; Darling et al., 2012; Heery et al., 2018). Rural areas, in contrast, represent the new development margin in Hawaiʻi, where earthmoving is recent and increasing. In these developing but not yet “urban” areas, coral community shifts may not have taken full course and stress-tolerant species may be uncommon or absent. Therefore, the combination of turbidity and heatwaves in rural areas may be particularly damaging. This aligns with findings by Asner et al. (2022), which linked coral mortality during Hawaiʻi’s 2019 bleaching event to new development (conversion of “natural landscape to human-built environments” over five recent years).
Coral taxa associated with urban landscapes in Hawaiʻi also may have experienced more sustained pressure that begets trophic plasticity over time. Heterotrophy has been noted in turbid reefs worldwide (e.g., De'ath and Fabricius, 2010; Morgan et al., 2016), conferring resistance to bleaching through higher photosynthate translocation during heatwaves (Tremblay et al., 2016) and carbon subsidies when symbionts are lost (Grottoli et al., 2006). However, heterotrophic taxa have been recorded in remote and urban areas alike (Zweifler et al., 2021), and further research is required to delineate the trait space of corals surviving in urban versus rural, sedimented habitats, as well as linkages between these traits and repeat bleaching susceptibility. Importantly, our results show that living in an urban environment, absent turbidity, does not confer bleaching resilience, suggesting that bleaching tolerance is limited to taxa thriving in murky segments of the urban landscape.
Previous research shows there are considerable coarse- and fine-scale morphological differences between species and phenotypes that impact coral response to sediment (Jones et al., 2016). For example, branching morphologies have been linked to higher sediment tolerance due to high surface inclination (Jones et al., 2019) but lower bleaching tolerance (Darling et al., 2012). Sediment clearing mechanisms are diverse and complex, as are morphologies designed to maximize photosynthesis (Stafford-Smith and Ormond, 1992; Guest et al., 2016b). Traits of corals surviving under low light, high sediment, and high temperature conditions along gradients of remote, urban, and developing coastlines is a critical area of research. A large portion of sites considered in our analysis did not record taxonomic data, and therefore, we were unable to evaluate taxonomic explanations for our results. Future studies should use innovative methods like rapid Structure-from-Motion photogrammetry to capture geospatial patterns in morphology over large areas, and over time (Fukunaga et al., 2020).
Another possible reason for urban refugia is that urban and rural sources of turbidity differ. The types of dissolved and particulate matter associated with urban areas may be, counterintuitively, less harmful to corals than rural runoff at high temperatures. Urban turbidity is often associated with point-sources of pollution like wastewater treatment plants and stormwater outfalls (Carlson et al., 2019), whereas rural areas are linked to more diffuse, nonpoint sources of pollution like unpaved roads (Ramos-Scharron and MacDonald, 2005), agricultural and golf course runoff (Delevaux et al., 2018), erosion from scalded hillsides (Bartley et al., 2014), soil disturbed by feral ungulates (Tribble et al., 2016), and new construction areas, i.e., future urban sprawl (Maragos, 1993). Mills and Sebens (1997) showed that corals reject “clean” sediments. “Natural’, terrigenous sources of turbidity may be most damaging to corals in Hawaiʻi, where lateritic, volcanic soils have small grain sizes (Storlazzi et al., 2015) that are energetically expensive for coral polyps to clear, reducing energy stores that are severely needed during thermal stress (Erftemeijer et al., 2012); however, these particles also take longer to settle out of suspension (Bartley et al., 2014). While soil runoff and fine siltation certainly occurs in urban areas, it might represent a more dominant size fraction in steep, erosive watersheds with few retaining structures, and where new construction is accelerating. Future research in Hawaiʻi should investigate sediment constituents and settling rates alongside bleaching severity to determine which sedimentation profiles are most associated with coral bleaching, mortality, and resilience.
Point sources of pollution, which concentrate in urban areas, are better regulated than nonpoint sources in the United States, the country of our study region (Humenik et al., 1987). Several case studies demonstrate the benefits of legally mandated, infrastructural upgrades on reef health in turbid urban waters like wastewater outfalls zones in Hawaiʻi. For example, after a municipal sewage system in "Māmala" Bay, Hawaiʻi received treatment upgrades in 1977, reefs in the bay showed no significant evidence of sewage impact (Grigg, 1995). Likewise, sewage upgrades in 1978 in Kāneʻohe Bay resulted in a doubling of coral cover from 12% in 1971 to 26% in 1983 (Hunter and Evans, 1995). If rural areas are more difficult to regulate, or last in line for infrastructural improvements, they may drive a higher toxic load on reefs from facilities like leaking cesspools/underground storage tanks (Yoshioka et al., 2016; Abaya et al., 2018). Finally, our results align with a recent meta-analysis on bleaching susceptibility in human-dominated and isolated reefs (e.g., atolls), which provide limited evidence for “remote refugia” but indicate some resilience in human-dominated habitats (Baumann et al., 2022). In particular, our results suggest that regions that are not yet developed but show symptoms of new development (non-urban turbidity) may be particularly susceptible to coral bleaching.
Our results do not imply that urban turbidity creates a ‘safe haven’ for coral reefs. We did not evaluate coral disease, diversity, fecundity, size classes, calcification rate, or other elements of coral health in our analysis, and it is possible that corals in silty, urban areas are stress-tolerant but sparse, homogenous, or otherwise represent ecosystem phase shifts. However, our results support past case studies (e.g., Guest et al., 2016b) by indicating that urbanization can counterbalance thermal stress during bleaching events. We further add that, in rural areas, turbidity does not counteract heatwaves, and runoff from new development, agriculture, unpaved roads, and nonpoint sources of pollution may still pose a critical threat. In Hawaiʻi, rural areas may represent a combination of unchecked earthmoving, new dredging, and aging infrastructure, which require urgent intervention.
A recent study indicated that mortality is highest when thermal stress and dredging conditions (high suspended sediment, low light) occur together (Fisher et al., 2019). While corals bleached less under low/moderate light reduction, severe light limitation acted synergistically with thermal stress to increase mortality. We likewise find that turbidity only acts antagonistically against bleaching under certain conditions (low heat stress). More research is needed on the nuanced thresholds of light, irradiance, and heat that alter interactions between stressors for various coral species.
Our findings are inconsistent with one hypothesis of turbid refugia—that sediment shades corals from excessive sunlight (Anthony et al., 2007). Equivalent levels of rural and urban turbidity should have the same effect on coral bleaching if protection comes from light-shading alone. Instead, in our study, turbidity associated with different land uses affects bleaching differently. At low levels of SSTa, rural areas show a slightly steeper decline in bleaching severity than urban areas. Conversely, at high SSTa, an equivalent increase in turbidity provides less bleaching protection in rural areas compared to urban areas (Figure 5). However, turbidity levels may be relatively moderate in the Hawaiian Islands compared to other regions where turbid refugia have been studied. For example, Morgan et al. (2016) found that turbidity in Paluma Shoals, Australia caused 100% attenuation of Photosynthetically Active Radiation (PAR) at 4 m water depth, relative to turbid reefs in Hawaiʻi like Puhi Bay where we previously measured ~72% attenuation of PAR at 5 m depth in preliminary experiments. Rural turbidity may still protect corals from excessive irradiance during bleaching events in regions where turbidity levels are high (van Woesik et al., 2012; Cacciapaglia and van Woesik, 2016; Morgan et al., 2017) though, as discussed, suspended sediment and excessively low light can also induce bleaching and mortality in corals (Fisher et al., 2019).
Other environmental drivers
When estimating the impacts of SSTa alone, we found that SSTa was an extremely strong predictor of bleaching occurrence, but not severity. However, the interactions between SSTa and local environmental variables (turbidity, urban turbidity, and wave power) had a very strong effect on bleaching severity. Specifically, under increasing SSTa, turbidity exacerbated bleaching severity, but urban turbidity and wave power reduced bleaching severity (Figure 4B). Overall, our results show that temperature, expectedly, is the most important factor determining whether corals bleach, but SSTa interactions with local environmental variables determine whether bleaching is minor or severe. Often, studies do not differentiate between bleaching occurrence and severity, and our results highlight additional insights gained through this approach. Irradiance showed no significant relationship to coral bleaching occurrence or severity, perhaps because our remotely sensed irradiance metric captured light on the ocean surface but not at depth, and corals in our analysis experienced a wide range of in-water light-scattering conditions. Indeed, since turbidity is a light-based metric, turbidity likely captured some variability in irradiance at depth. In addition, the effect of SSTa, irradiance, and other environmental variables may have been moderated by the lower resolution of those data (e.g., 5 km for SSTa, 4 km for irradiance, 500 meters for total effluent) relative to turbidity; nonetheless, these were the best data available.
Our results highlight the importance of SSTa-environment interactions, which is consistent with growing literature on the impact of local drivers on coral reef health (Graham et al., 2015; Cinner et al., 2016; Ellis et al., 2019; Donovan et al., 2021). Local variables (e.g., wave action, upwelling, internal waves, tides, mangrove refugia, and other features) alter coral exposure to thermal stress and ability to cope with and recover from bleaching (West and Salm, 2003). In two global analyses using Reef Check data, interactions between SSTa and local conditions including turbidity, wave power, and macroalgae were found to mediate bleaching severity (Sully and van Woesik, 2020; Donovan et al., 2021). In our analysis, wave power negatively influenced SSTa effects on bleaching severity, perhaps because waves increase mass transfer of oxygen radicals from coral tissues (Nakamura and Van Woesik, 2001), thereby reducing host stress. Waves may also have caused ocean cooling at finer scales than the thermal signal detected by satellites. Live coral cover was linked to increased bleaching occurrence but decreased bleaching severity. One possible explanation is that high coral cover increases the probability of observing any (even minor) bleaching on a survey, but also indicates areas where corals survived prior bleaching in Hawaiʻi (high bleaching resilience). Overall, interactions between local environment and regional heatwaves is a critical area of ongoing research, and advances in remote sensing can enable a granular, scalable understanding of these dynamics.
Including island as a fixed effect increased model fit, and in particular, three islands (Maui, Molokaʻi, and Oʻahu) showed increased bleaching severity relative to Hawaiʻi. Molokaʻi is largely undeveloped but exhibited the highest turbidity of any island (Table 1), likely because numerous surveys were located on the heavily sedimented south shore of the island where terrigenous sediment is deposited from several drainage basins (Ogston et al., 2004; Jokiel et al., 2014). Oʻahu, in contrast, is highly urbanized with variable turbidity and low coral cover proportional to substrate area (Asner et al., 2020b), though Oʻahu surveys displayed a mean 43% live coral cover and surveys may therefore have focused on higher-coral areas. Maui represents a mixture of urban and rural areas experiencing sewage discharge and bottom sediment resuspension in urban centers (Laws et al., 2004) and increasing development and agriculture in remote regions (Oleson et al., 2017). Given this complex array of factors, it is difficult to interpret island-based results without additional investigation, but it is possible that site selection may have played a role in island influence (e.g., excluding the north and west sides of Molokaʻi).
Finally, it should be noted that including ‘organization name’ as a proxy for survey method substantially increased model fit in all cases, pointing to the need to control for survey biases in coral research. Nonetheless, when using broad metrics like percent bleaching, past research has shown that coral survey techniques yield comparable results (Jokiel et al., 2015).
Implications for management and research
This study is an early step in using high-resolution satellite monitoring to characterize possible turbid refugia. We considered broad categories of turbid landscapes (urban and rural), which should be refined in the future to include a full spectrum of land-use classes such as agriculture, forestland, grassland/scrub, suburban and residential areas, and others. In addition, recent research points to the importance of temporality in turbidity regimes (persistent, fluctuating, and transitional turbidity) in dictating coral health (Zweifler et al., 2021). Planet Dove satellites, which offer a daily revisit frequency, can help characterize differences in the timing of turbidity, and identify which temporal regimes are linked to bleaching protection or harm. Importantly, the methods we highlight here may be highly useful to local managers. High-resolution satellite data can help coastal decision-makers identify specific plumes, resuspension areas, and other sources of turbidity to address runoff on a case-by-case basis. For example, where heavy plumes are linked to bleaching refugia, managers may prioritize coral protection by addressing persistent stressors like pathogens and disease, while frequently monitoring for algal overgrowth. Turbid areas associated with coral decline, on the other hand, should be prioritized for complete remediation. High-resolution satellite data can also be used to help refine existing models for predictive purposes, and scaled to local suspended sediment concentrations to monitor sediment against known coral health thresholds.
Data availability statement
The original contributions presented in the study are included in the article/Supplementary Material. Further inquiries can be directed to the corresponding author.
Author contributions
RC conceived the study, conducted the analysis, and wrote the manuscript. GA conceived and funded the study and contributed to the analysis and writing of the manuscript. All authors contributed to the article and approved the submitted version.
Acknowledgments
This study was supported by Paul G. Allen’s Vulcan Inc. under contract to Arizona State University and the Lenfest Ocean Program, and by the National Science Foundation Graduate Research Fellowship Program (Grant Number DGE-1656518). The authors declare that they have no known competing financial interests or personal relationships that could have appeared to influence the work reported in this paper. We thank Shawna Foo for helpful comments on the manuscript.
Conflict of interest
The authors declare that the research was conducted in the absence of any commercial or financial relationships that could be construed as a potential conflict of interest.
Publisher’s note
All claims expressed in this article are solely those of the authors and do not necessarily represent those of their affiliated organizations, or those of the publisher, the editors and the reviewers. Any product that may be evaluated in this article, or claim that may be made by its manufacturer, is not guaranteed or endorsed by the publisher.
Supplementary material
The Supplementary Material for this article can be found online at: https://www.frontiersin.org/articles/10.3389/fmars.2022.969472/full#supplementary-material
References
Abaya L. M., Wiegner T. N., Beets J. P., Colbert S. L., Kaile'a M. C., Kramer K. L. (2018). Spatial distribution of sewage pollution on a Hawaiian coral reef. Mar. pollut. Bul 130, 335–347. doi: 10.1016/j.marpolbul.2018.03.028
Anthony K. R. N. (2000). Enhanced particle-feeding capacity of corals on turbid reefs (Great barrier reef, Australia). Coral Reefs 19 (1), 59–67. doi: 10.1007/s003380050227
Anthony K. R. N., Connolly S. R., Hoegh-Guldberg O. (2007). Bleaching, energetics, and coral mortality risk: Effects of temperature, light, and sediment regime. Limnol Oceanogr 52, 716–726. doi: 10.4319/lo.2007.52.2.0716
Asner G. P., Vaughn N. R., Balzotti C., Brodrick P. G., Heckler J. (2020a). High-resolution reef bathymetry and coral habitat complexity from airborne imaging spectroscopy. Remote Sens. 12, 310. doi: 10.3390/rs12020310
Asner G. P., Vaughn N. R., Heckler J., Knapp D. E., Balzotti C., Shafron E., et al. (2020b). Large-Scale mapping of live corals to guide reef conservation. Proc. Natl. Acad. Sci. 117 (52), 33711–33718. doi: 10.1073/pnas.2017628117
Asner G. P., Vaughn N. R., Martin R. E., Foo S. A., Heckler J., Neilson B. J., et al. (2022). Mapped coral mortality and refugia in an archipelago-scale marine heat wave. Proc. Natl. Acad. Sci. 119 (19), e2123331119. doi: 10.1073/pnas.2123331119
(2019). NASA Goddard Space flight center, ocean ecology laboratory, ocean biology processing group. moderate-resolution imaging spectroradiometer (MODIS) aqua. Available at: https://oceancolor.gsfc.nasa.gov
Photosynthetically available radiation. data; (Greenbelt, MD, USA: NASA OB.DAAC). Available at: https://oceancolor.gsfc.nasa.gov/l3 (Accessed 11 November 2020).
Bartley R., Bainbridge Z. T., Lewis S. E., Kroon F. J., Wilkinson S. N., Brodie J. E., et al. (2014). Relating sediment impacts on coral reefs to watershed sources, processes and management: A review. Sci. Tot Environ. 468, 1138–1153. doi: 10.1016/j.scitotenv.2013.09.030
Baumann J. H., Zhao L. Z., Stier A. C., Bruno J. F. (2022). Remoteness does not enhance coral reef resilience. Glob Chang Biol. 28 (2), 417–428. doi: 10.1111/gcb.15904
Bessell-Browne P., Negri A. P., Fisher R., Clode P. L., Duckworth A., Jones R. (2017b). Impacts of turbidity on corals: The relative importance of light limitation and suspended sediments. Mar. pollut. Bul 117 (1-2), 161–170. doi: 10.1016/j.marpolbul.2017.01.050
Bessell-Browne P., Negri A. P., Fisher R., Clode P. L., Jones R. (2017). Cumulative impacts: thermally bleached corals have reduced capacity to clear deposited sediment. Sci. Rep. 7 (1), 1–14. doi: 10.1038/s41598-017-02810-0
Bessell-Browne P., Negri A. P., Fisher R., Clode P. L., Jones R. (2017a). Impacts of light limitation on corals and crustose coralline algae. Sci. Rep. 7 (1), 1–12. doi: 10.1038/s41598-017-11783-z
Brodie J. E., Kroon F. J., Schaffelke B., Wolanski E. C., Lewis S. E., Davis A. M. (2012). Terrestrial pollutant runoff to the great barrier reef: An update of issues, priorities and management responses. Mar. pollut. Bul 65, 81–100. doi: 10.1016/j.marpolbul.2011.12.012
Brunner C. A., Ricardo G. F., Uthicke S., Negri A. P., Hoogenboom M. O. (2022). Effects of climate change and light limitation on coral recruits. Mar. Ecol. Prog. Ser. 690, 65–82. doi: 10.3354/meps14055
Burke L., Reytar K., Spalding M., Perry A. (2011). Reefs at risk revisited (Washington, DC: World Resources Institute).
Cacciapaglia C., van Woesik R. (2016). Climate-change refugia: Shading reef corals by turbidity. Glob Chang Biol. 22 (3), 1145–1154. doi: 10.1111/gcb.13166
Camp E. F., Schoepf V., Mumby P. J., Hardtke L. A., Rodolfo-Metalpa R., Smith D. J., et al. (2018). The future of coral reefs subject to rapid climate change: Lessons from natural extreme environments. Front. Mar. Sci. 5. doi: 10.3389/fmars.2018.00004
Carlson R. R., Evans L. J., Foo S. A., Grady B. W., Li J., Seeley M., et al. (2021). Synergistic benefits of conserving land-Sea ecosystems. Glob Ecol. Conserv., e01684. doi: 10.1016/j.gecco.2021.e01684
Carlson R. R., Foo S. A., Asner G. P. (2019). Land use impacts on coral reef health: A ridge-to-reef perspective. Front. Mar. Sci. 6. doi: 10.3389/fmars.2019.00562
Cinner J. E., Huchery C., MacNeil M. A., Graham N. A. J., McClanahan T. R., Maina J., et al. (2016). Bright spots among the world’s coral reefs. Nature 535, 416–419. doi: 10.1038/nature18607
Coles S. L., Jokiel P. L. (1978). Synergistic effects of temperature, salinity and light on the hermatypic coral montipora verrucosa. Mar. Biol. 49, 187–195. doi: 10.1007/BF00391130
Darling E. S., Alvarez-Filip L., Oliver T. A., McClanahan T. R., Côté I. M. (2012). Evaluating life-history strategies of reef corals from species traits. Ecol. Lett. 15, 1378–1386. doi: 10.1111/j.1461-0248.2012.01861.x
De'ath G., Fabricius K. (2010). Water quality as a regional driver of coral biodiversity and macroalgae on the great barrier reef. Ecol. Appl. 20, 840–850. doi: 10.1890/08-2023.1
De Carlo E. H., Hoover D. J., Young C. W., Hoover R. S., Mackenzie F. T. (2007). Impact of storm runoff from tropical watersheds on coastal water quality and productivity. Appl. Geochem 22 (8), 1777–1797. doi: 10.1016/j.apgeochem.2007.03.034
Delevaux J. M., Whittier R., Stamoulis K. A., Bremer L. L., Jupiter S., Friedlander A. M., et al. (2018). A linked land-sea modeling framework to inform ridge-to-reef management in high oceanic islands. PloS One 13, e0193230. doi: 10.1371/journal.pone.0193230
Dogliotti A. I., Ruddick K. G., Nechad B., Doxaran D., Knaeps E. (2015). A single algorithm to retrieve turbidity from remotely-sensed data in all coastal and estuarine waters. Remote Sens Environ. 156, 57–168. doi: 10.1016/j.rse.2014.09.020
Donovan M. K., Adam T. C., Shantz A. A., Speare K. E., Munsterman K. S., Rice M. M., et al. (2020). Nitrogen pollution interacts with heat stress to increase coral bleaching across the seascape. Proc. Natl. Acad. Sci. U.S.A. 117, 5351–5357. doi: 10.1073/pnas.1915395117
Donovan M. K., Burkepile D. E., Kratochwill C., Shlesinger T., Sully S., Oliver T. A., et al. (2021). Local conditions magnify coral loss after marine heatwaves. Science 372, 977–980. doi: 10.1126/science.abd9464
Ellis J. I., Jamil T., Anlauf H., Coker D. J., Curdia J., Hewitt J., et al. (2019). Multiple stressor effects on coral reef ecosystems. Glob Chang Biol. 25, 4131–4146. doi: 10.1111/gcb.14819
Erftemeijer P. L., Riegl B., Hoeksema B. W., Todd P. A. (2012). Environmental impacts of dredging and other sediment disturbances on corals: a review. Mar. pollut. Bul 64, 1737–1765. doi: 10.1016/j.marpolbul.2012.05.008
Fabricius K. E. (2005). Effects of terrestrial runoff on the ecology of corals and coral reefs: Review and synthesis. Mar. pollut. Bul 50, 125–146. doi: 10.1016/j.marpolbul.2004.11.028
Ferrier M. D. (1991). Net uptake of dissolved free amino acids by four scleractinian corals. Coral Reefs 10(4), 183–187.
Fisher R., Bessell-Browne P., Jones R. (2019). Synergistic and antagonistic impacts of suspended sediments and thermal stress on corals. Nat. Commun. 10 (1), 1–9. doi: 10.1038/s41467-019-10288-9
Fuglstad G. A., Simpson D., Lindgren F., Rue H. (2019). Constructing priors that penalize the complexity of Gaussian random fields. Jour Amer Stat. Assoc. 114, 445–452. doi: 10.48550/arXiv.1503.00256
Fukunaga A., Burns J. H., Pascoe K. H., Kosaki R. K. (2020). Associations between benthic cover and habitat complexity metrics obtained from 3D reconstruction of coral reefs at different resolutions. Remote Sens. 12 (6), 1011. doi: 10.3390/rs12061011
Goodkin N. F., Switzer A. D., McCorry D., DeVantier L., True J. D., Hughen K. A., et al. (2011). Coral communities of Hong Kong: long-lived corals in a marginal reef environment. Mar. Ecol. Prog. Ser. 426, 185–196. doi: 10.3354/meps09019
Graham N. A., Jennings S., MacNeil M. A., Mouillot D., Wilson S. K. (2015). Predicting climate-driven regime shifts versus rebound potential in coral reefs. Nature 518, 94–97. doi: 10.1038/nature14140
Grigg R. W. (1983). Community structure, succession and development of coral reefs in Hawaii. Mar. Ecol. Prog. Ser. 11, 1–14. doi: 10.3354/meps011001
Grigg R. W. (1995). Coral reefs in an urban embayment in Hawaii: a complex case history controlled by natural and anthropogenic stress. Coral Reefs 14 (4), 253–266. doi: 10.1007/BF00334349
Grottoli A. G., Rodrigues L. J., Palardy J. E. (2006). Heterotrophic plasticity and resilience in bleached corals. Nature 440, 1186–1189. doi: 10.1038/nature04565
Grover R., Maguer J. F., Reynaud-Vaganay S., Ferrier-Pages C. (2002). Uptake of ammonium by the scleractinian coral Stylophora pistillata: effect of feeding, light, and ammonium concentrations. Limnology and Oceanography 47(3), 782–790.
Grover R., Maguer J. F., Allemand D., Ferrier-Pages C. (2003). Nitrate uptake in the scleractinian coral Stylophora pistillata. Limnology and Oceanography 48(6), 2266–2274.
Guest J. R., Low J., Tun K., Wilson B., Ng. C., Raingeard D., et al. (2016b). Coral community response to bleaching on a highly disturbed reef. Sci. Rep. 6, 1–10. doi: 10.1038/srep20717
Guest J. R., Tun K., Low J., Verges A., Marzinelli E. M., Campbell A. H., et al. (2016a). 27 years of benthic and coral community dynamics on turbid, highly urbanised reefs off Singapore. Sci. Rep. 6, 1–10. doi: 10.1038/srep36260
Harrison X. A., Donaldson L., Correa-Cano M. E., Evans J., Fisher D., Goodwin C. E., et al. (2018). A brief introduction to mixed effects modelling and multi-model inference in ecology. PeerJ 6, e4794. doi: 10.7717/peerj.4794
Hawaii Coral Bleaching Collaborative (2021) . version 2021-01. Hawaii coral bleaching collaborative: surveys for percent of bleached coral cover across the Hawaiian archipelago from august 27 to December 7, 2019. Available at: https://www.fisheries.noaa.gov/inport/item/59191.
Heery E. C., Hoeksema B. W., Browne N. K., Reimer J. D., Ang P. O., Huang D., et al. (2018). Urban coral reefs: Degradation and resilience of hard coral assemblages in coastal cities of East and southeast Asia. Mar. pollut. Bul 135, 654–681. doi: 10.1016/j.marpolbul.2018.07.041
Hoegh-Guldberg O., Williamson J. (1999). Availability of two forms of dissolved nitrogen to the coral Pocillopora damicornis and its symbiotic zooxanthellae. Marine Biology 133(3), 561–570.
Hongo C., Yamano H. (2013). Species-specific responses of corals to bleaching events on anthropogenically turbid reefs on Okinawa island, Japan, over a 15-year period, (1995-2009). PloS One 8 (4), e60952. doi: 10.1371/journal.pone.0060952
Houlbrèque F., Ferrier-Pagès C. (2009). Heterotrophy in tropical scleractinian corals. Biol. Rev. 84, 1–17. doi: 10.1111/j.1469-185X.2008.00058.x
Hughes T. P., Anderson K. D., Connolly S. R., Heron S. F., Kerry J. T., Lough J. M., et al. (2018). Spatial and temporal patterns of mass bleaching of corals in the anthropocene. Science 359 (6371), 80–83. Available at: doi.org/10.1126/science.aan8048
Humenik F. J., Smolen M. D., Dressing S. A. (1987). Pollution from nonpoint sources. Environ. Sci. Technol. (United States) 21 (8), 737–742. doi: 10.1021/es00162a600
Hunter C. L., Evans C. W. (1995). Coral reefs in kaneohe bay, Hawaii: two centuries of western influence and two decades of data. Bul Mar. Sci. 57, 501–515.
Jokiel P. L., Rodgers K. S., Brown E. K., Kenyon J. C., Aeby G., Smith W. R., et al. (2015). Comparison of methods used to estimate coral cover in the Hawaiian islands. PeerJ. 3, e954. doi: 10.7717/peerj.954
Jokiel P. L., Rodgers K. S., Storlazzi C. D., Field M. E., Lager C. V., Lager D. (2014). Response of reef corals on a fringing reef flat to elevated suspended-sediment concentrations: Molokaʻi, hawaiʻi. PeerJ. 2, e699. doi: 10.7717/peerj.699
Jones R., Bessell-Browne P., Fisher R., Klonowski W., Slivkoff M. (2016). Assessing the impacts of sediments from dredging on corals. Mar. pollut. Bull. 102 (1), 9–29. doi: 10.1016/j.marpolbul.2015.10.049
Jones R., Fisher R., Bessell-Browne P. (2019). Sediment deposition and coral smothering. PloS One 14 (6), e0216248. doi: 10.1371/journal.pone.0216248
Jones R., Giofre N., Luter H. M., Neoh T. L., Fisher R., Duckworth A. (2020). Responses of corals to chronic turbidity. Sci. Rep. 10, 1–13. doi: 10.1038/s41598-020-61712-w
Jones R., Ricardo G. F., Negri A. P. (2015). Effects of sediments on the reproductive cycle of corals. Mar. pollut. Bull. 100 (1), 13–33. doi: 10.1016/j.marpolbul.2015.08.021
Kappel C. V., Selkoe K. A., Ocean Tipping Points (OTP) (2017a) Wave power long-term mean 2000-2013 - (Hawaii: Distributed by the Pacific Islands Ocean Observing System (PacIOOS). Available at: http://pacioos.org/metadata/hi_otp_all_wave_avg.html (Accessed 11 November 2020).
Kappel C. V., Wedding L. M., Lecky J., Ocean Tipping Points (OTP) (2017b) Nitrogen flux from onsite sewage disposal systems (OSDS) (Hawaii: Distributed by the Pacific Islands Ocean Observing System (PacIOOS). Available at: http://pacioos.org/metadata/hi_otp_all_osds_nitrogen.html (Accessed 11 November 2020).
Lafratta A., Fromont J., Speare P., Schonberg C. H. L. (2017). Coral bleaching in turbid waters of north-western Australia. Mar. Freshw. Res. 68, 65–75. doi: 10.1071/MF15314
Laws E., Brown D., Peace C. (2004). Coastal water quality in the kihei and lahaina districts of the island of Maui, Hawaiian islands. impacts from physical habitat and groundwater seepage: implications for water quality standards. Int. J. Environ. pollut. 22 (5), 531–546. doi: 10.1504/IJEP.2004.005908
Lee Z., Weidemann A., Arnone R. (2012). Combined effect of reduced band number and increased bandwidth on shallow water remote sensing: The case of worldview 2. IEEE Trans. Geosci. Remote Sens. 51, 2577–2586. doi: 10.1109/TGRS.2012.2218818
Levas S., Grottoli A. G., Schoepf V., Aschaffenburg M. D. (2016). Can heterotrophic uptake of dissolved organic carbon and zooplankton mitigate carbon budget deficits in annually bleached corals? Coral Reefs 35, 495–506. doi: 10.1007/s00338-015-1390-z
Li J., Carlson R. R., Knapp D. E., Asner G. P. (2022). Shallow coastal water turbidity monitoring using planet dove satellites. Remote Sens Ecol. Conserv 8 (4), 521–535. doi: 10.1002/rse2.259
Li J., Knapp D. E., Lyons M., Roelfsema C., Phinn S., Schill S. R., et al. (2021). Automated global shallow water bathymetry mapping using Google earth engine. Remote Sens 13, 1469. doi: 10.3390/rs13081469
Lindgren F., Rue H. (2015). Bayesian Spatial modelling with r-INLA. J. Stat. Softw 63, 1–25. doi: 10.18637/jss.v063.i19
Lirman D., Fong P. (2007). Is proximity to land-based sources of coral stressors an appropriate measure of risk to coral reefs? an example from the Florida reef tract. Mar. pollut. Bul 54, 779–791. doi: 10.1016/j.marpolbul.2006.12.014
Loiola M., Cruz I. C., Lisboa D. S., Mariano-Neto E., Leão Z. M. A. N., Oliveira M. D. M., et al. (2019). Structure of marginal coral reef assemblages under different turbidity regime. Mar. Environ. Res. 147, 138–148. doi: 10.1016/j.marenvres.2019.03.013
Luter H. M., Pineda M. C., Ricardo G., Francis D. S., Fisher R., Jones R. (2021). Assessing the risk of light reduction from natural sediment resuspension events and dredging activities in an inshore turbid reef environment. Mar. Poll Bul 170, 112536. doi: 10.1016/j.marpolbul.2021.112536
Maragos J. E. (1993). Impact of coastal construction on coral reefs in the US-affiliated pacific islands. Coast. Manage 21, 235–269. doi: 10.1080/08920759309362207
Mills M. M., Sebens K. P. (1997). Particle ingestion efficiency of the corals siderastrea siderea and agaricia agaricites: Effects of flow speed and sediment loads. In Proc. 8th Int. Coral Reef Symp 2, 1059–1064).
Mora C. (2008). A clear human footprint in the coral reefs of the Caribbean. Proc. Biol. Sci. 275, 767–773. doi: 10.1098/rspb.2007.1472
Morgan K. M., Perry C. T., Johnson J. A., Smithers S. G. (2017). Nearshore turbid-zone corals exhibit high bleaching tolerance on the great barrier reef following the 2016 ocean warming event. Front. Mar. Sci. 4. doi: 10.3389/fmars.2017.00224
Morgan K. M., Perry C. T., Smithers S. G., Johnson J. A., Daniell J. J. (2016). Evidence of extensive reef development and high coral cover in nearshore environments: implications for understanding coral adaptation in turbid settings. Sci. Rep. 6, 1–10. doi: 10.1038/srep29616
Nakamura T., Van Woesik R. (2001). Water-flow rates and passive diffusion partially explain differential survival of corals during the 1998 bleaching event. Mar. Ecol. Prog. Ser. 212, 301–304. doi: 10.3354/meps212301
NOAA Coral Reef Watch (2019) Operational daily near-Real-Time global 5-km satellite coral bleaching monitoring products. Available at: https://coastwatch.pfeg.noaa.gov/erddap/griddap/NOAA.DHW.html (Accessed 11 November 2020).
Ogston A. S., Storlazzi C. D., Field M. E., Presto M. K. (2004). Sediment resuspension and transport patterns on a fringing reef flat, Molokai, Hawaii. Coral Reefs 23 (4), 559–569. doi: 10.1007/s00338-004-0415-9
Oleson K. L., Falinski K. A., Lecky J., Rowe C., Kappel C. V., Selkoe K. A., et al. (2017). Upstream solutions to coral reef conservation: The payoffs of smart and cooperative decision-making. J. Environ. Manage 191, 8–18. doi: 10.1016/j.jenvman.2016.12.067
Osborne K., Thompson A. A., Cheal A. J., Emslie M. J., Johns K. A., Jonker M. J., et al. (2017). Delayed coral recovery in a warming ocean. Global Change Biol. 23 (9), 3869–3881. doi: 10.1111/gcb.13707
Palardy J. E., Rodrigues L. J., Grottoli A. G. (2008). The importance of zooplankton to the daily metabolic carbon requirements of healthy and bleached corals at two depths. J. Exp. Mar. Biol. Ecol. 367, 180–188. doi: 10.1016/j.jembe.2008.09.015
Pebesma E. (2018). Simple features for r: Standardized support for spatial vector data. R J. 10, 439–446. doi: 10.32614/RJ-2018-009
Ramos-Scharrón C. E., MacDonald L. H. (2005). Measurement and prediction of sediment production from unpaved roads, St John, US virgin islands. Earth Surface Process. Landforms: J. Br. Geomorphol. Res. Group 30, 1283–1304. doi: 10.1002/esp.1201
Rue H., Martino S., Chopin N. (2009). Approximate Bayesian inference for latent Gaussian models using integrated nested Laplace approximations. J. Roy Stat. Soc. 71, 319. doi: 10.1111/j.1467-9868.2008.00700.x
Safaie A., Silbiger N. J., McClanahan T. R., Pawlak G., Barshis D. J., Hench J. L., et al. (2018). High frequency temperature variability reduces the risk of coral bleaching. Nat. Commun. 9, 1–12. doi: 10.1038/s41467-018-04074-2
Simons R. A. (2020) ERDDAP (Monterey, CA: NOAA/NMFS/SWFSC/ERD). Available at: https://coastwatch.pfeg.noaa.gov/erddap (Accessed 15 November 2020).
Stafford-Smith M. G., Ormond R. F. G. (1992). Sediment-rejection mechanisms of 42 species of Australian scleractinian corals. Mar. Freshw. Res. 43 (4), 683–705. doi: 10.1071/MF9920683
Storlazzi C. D., Norris B. K., Rosenberger K. J. (2015). The influence of grain size, grain color, and suspended-sediment concentration on light attenuation: Why fine-grained terrestrial sediment is bad for coral reef ecosystems. Coral Reefs 34, 967–975. doi: 10.1007/s00338-015-1268-0
Sully S., van Woesik R. (2020). Turbid reefs moderate coral bleaching under climate-related temperature stress. Glob Chang Biol. 26, 1367–1373. doi: 10.1111/gcb.14948
Tremblay P., Gori A., Maguer J. F., Hoogenboom M., Ferrier-Pagès C. (2016). Heterotrophy promotes the re-establishment of photosynthate translocation in a symbiotic coral after heat stress. Sci. Rep. 6, 1–14. doi: 10.1038/srep38112
Tribble G., Stock J., Jim J. (2016). “"Watershed processes from ridge to reef: consequences of feral ungulates for coral reef and effects of watershed management.",” in Proceedings of the fifth interagency conference on research in the watersheds (US Department of Agriculture Forest Service, Southern Research Station), 194–194.
U.S. Department of Commerce, U.S, Census Bureau, Geography Division (2015) TIGER/Line shapefiles. Available at: http://www2.census.gov/geo/tiger/TIGER2015/UAC10/tl_2015_us_uac10.zip (Accessed 3 July 2020).
Van Woesik R., Houk P., Isechal A. L., Idechong J. W., Victor S. And Golbuu Y.(2012). climate-change refugia in the sheltered bays of Palau: Analogs of future reefs. Ecol. Evol. 2, 2474–2484. doi: 10.1002/ece3.363
Vitousek P. M. (1995). The Hawaiian islands as a model system for ecosystem studies. Pacific Sci. 49, 2–16.
West J. M., Salm R. V. (2003). Resistance and resilience to coral bleaching: implications for coral reef conservation and management. Con Bio 17, 956–967. doi: 10.1046/j.1523-1739.2003.02055.x
Whittier R. B., El-Kadi A. I. (2014). Human health and environmental risk ranking of onsite sewage disposal systems for the Hawaiian islands of Kauai, Molokai, Maui, and Hawaii (State of Hawaii Department of Health, Safe Drinking Water Branch).
Williams G. J., Knapp I. S., Maragos J. E., Davy S. K. (2010). Modeling patterns of coral bleaching at a remote central pacific atoll. Mar. pollut. Bul 60, 1467–1476. doi: 10.1016/j.marpolbul.2010.05.009
Winston M., Couch C. S., Huntington B, Vargas-Ángel B, Suka R, Oliver T, et al. (2020). Preliminary results of patterns of 2019 thermal stress and coral bleaching across the Hawaiian archipelago. NOAA Instit. Repository ID # 23699. 1–8.
Yoshioka R. M., Kim C. J., Tracy A. M., Most R., Harvell C. D. (2016). Linking sewage pollution and water quality to spatial patterns of porites lobata growth anomalies in puako, Hawaii. Mar. pollut. Bul 104, 313–321. doi: 10.1016/j.marpolbul.2016.01.002
Zuur A. F., Ieno E. N. (2016). Beginner’s guide to zero-inflated models with r (Newburgh, UK: Highland Statistics, Ltd).
Zuur A. F., Ieno E. N., Saveliev A. A. (2017). Spatial, temporal and spatial-temporal ecological data analysis with r-INLA (Newburgh, UK: Highland Statistics, Ltd).
Keywords: turbidity, refugia, coral reefs, bleaching, land use, sediment, climate change, remote sensing
Citation: Carlson RR, Li J, Crowder LB and Asner GP (2022) Large-scale effects of turbidity on coral bleaching in the Hawaiian islands. Front. Mar. Sci. 9:969472. doi: 10.3389/fmars.2022.969472
Received: 15 June 2022; Accepted: 12 August 2022;
Published: 05 September 2022.
Edited by:
Lorenzo Mari, Politecnico di Milano, ItalyReviewed by:
Asahi Sakuma, Kisarazu College, JapanPia Bessell-Browne, Oceans and Atmosphere (CSIRO), Australia
Kuulei S. Rodgers, University of Hawaii at Manoa, United States
Copyright © 2022 Carlson, Li, Crowder and Asner. This is an open-access article distributed under the terms of the Creative Commons Attribution License (CC BY). The use, distribution or reproduction in other forums is permitted, provided the original author(s) and the copyright owner(s) are credited and that the original publication in this journal is cited, in accordance with accepted academic practice. No use, distribution or reproduction is permitted which does not comply with these terms.
*Correspondence: Rachel R. Carlson, cnJjYXJsQHN0YW5mb3JkLmVkdQ==