- 1Marine Environment and Technology Center, Instituto Superior Técnico, Universidade Técnica de Lisboa, Lisbon, Portugal
- 2Portuguese Institute for Sea and Atmosphere, Algés, Portugal
- 3Marine and Environmental Sciences Centre, Universidade Nova de Lisboa, Caparica, Portugal
- 4Barreiro School of Technology, Polytechnic Institute of Setubal, Lavradio, Portugal
- 5Centre for Functional Ecology, Universidade de Coimbra, Coimbra, Portugal
- 6Centro de Ciências do Mar (CCMAR) - Universidade do Algarve, Faro, Portugal
- 7Departamento de Ecologia, Universidade do Estado do Rio de Janeiro, Rio de Janeiro, Brazil
- 8Department of Biosciences, Swansea University, Swansea, United Kingdom
- 9Centro de Investigación en Ciencias del Mar y Limnología (CIMAR), Universidad de Costa Rica, San José, Costa Rica
Seagrasses are declining globally, in large part due to increased anthropogenic coastal nutrient loads that enhance smothering by macroalgae, attenuate light, and are toxic when in excessive concentrations of inorganic nitrogen and phosphorus. However, as sanitation is improved many seagrass meadows have been observed to recover, with a few studies suggesting that they may even benefit from mild anthropogenic nutrient additions. Monitoring seagrass demography and health has faced difficulties in establishing the adequate variables and metrics. Such uncertainty in the methods has caused uncertainty of the significance of results presented and compromised extrapolations to other seasons, areas, or species. One solution has come from within the plant self-thinning theories. During the 1980s, an interspecific boundary line (IBL) was determined as the upper limit of the combination of plant density and above-ground biomass for any stand on Earth, setting their maximum possible efficiency in space occupation. Recently, two meta-analyses to determine specific IBLs for algae and for seagrasses have been performed. The recently updated seagrass dataset comprises 5,052 observations from 78 studies on 18 species. These IBLs opened new perspectives for monitoring: the observed distance of a stand to the respective IBL (i.e., each stand’s relative efficiency of space occupation) was demonstrated to be a valuable indicator of a population’s health. Thus, this metric can be used to determine the impact of nutrients and pollutants on algae and seagrass populations. Furthermore, because the IBLs are common to all species, they may be used to compare all species from any location worldwide. This novel approach showed that Halodule wrightii, Halodule beaudettei, Halophila baillonii, Zostera marina, and Zostera noltei meadows benefit from anthropogenic additions of nitrogen and phosphorus, as long as these additions are moderate. In fact, the healthier Z. noltei meadows in Portugal (and among the healthiest meadows worldwide) were the ones exposed to effluents from wastewater treatment plants (WWTP) and a food factory. We conclude that those effluents are providing water with enough quality and that their optimal management should coordinate the technological solutions of the WWTP with the natural potential of seagrass meadows as water purifiers and biomass producers.
Introduction
Seagrass meadows are one of the most common marine coastal biotopes, representing hotspots for biodiversity and productivity (Morrison et al., 2014; Hyman et al., 2019; McHenry et al., 2021; Surugiu et al., 2021). Seagrasses are keystone species in these biotopes, providing many services to both ecosystems and human populations. The most common are nursery and shelter for fish, bivalves, arthropods, and marine invertebrates—some of them commercially exploited—food, and water purification (Morrison et al., 2014; Nordlund et al., 2016). Recently, seagrass meadows have been revealed as an important carbon sink (Bedulli et al., 2020; Ricart et al., 2020) as well as a sustainable source of renewable raw material and energy for construction and industry (Ntalos and Sideras, 2014; Plis et al., 2016; Hamdaoui et al., 2018; Jedidi and Abrough, 2020; Rammou et al., 2021), and soilless agriculture (Parente et al., 2014).
Seagrasses have declined globally during the 20th century due to increased anthropogenic impacts, especially those affecting water quality. The most common negative impact is increased nutrient load to coastal systems originating from agricultural runoff and urban effluents. In many cases, this has led to eutrophication, resulting in smothering by drift or epiphytic macroalgae, light attenuation due to increased phytoplankton and turbidity, and the toxic effects of excessive concentrations of inorganic nitrogen (N) and phosphorus (P) (Burkholder et al., 2007; Lee et al., 2007). Other negative impacts include clam harvesting (Cabaço et al., 2005; Garmendia et al., 2017), herbicides (McMahon et al., 2005; Waycott et al., 2005; Negri et al., 2015; Espel et al., 2019), pesticides (Bester, 2000; Waycott et al., 2005; Espel et al., 2019), and industrial contamination (Waycott et al., 2005; Fraser and Kendrick, 2017; Espel et al., 2019; Lafratta et al., 2019). However, evidence is accumulating on the recovery of some seagrass beds worldwide (Greening et al., 2014; Bertelli et al., 2018; Burdick et al., 2020; Orth et al., 2020). This trend is particularly true for European seagrasses, for which a reversal was determined from the 2000s onward (de los Santos et al., 2019). This improvement was likely associated with the significant European-wide efforts that took place through the latter decades to improve sanitation and protect coastal habitats. A few studies have suggested that seagrass stands may even benefit from mild anthropogenic nutrient additions (Cabaço et al., 2013; Vieira et al., 2018).
Inference about the demography and health of seagrass stands is complex as we face difficulties in finding adequate variables, metrics, and models. Often, studies have been performed exclusively on a single species, comprising only a narrow band of its geographical distribution, and sometimes over only part of the seasonal cycle (Cabaço et al., 2007, 2008; Romero et al., 2007; Garcı́a-Marín et al., 2013; Jones and Unsworth, 2016; Jones et al., 2018). This has brought great uncertainty on the significance of the results presented and compromised extrapolations to other seasons, geographical areas, or species. Broader studies still face problems with numerical methods. Examples of this are the debate between Cabaço et al. (2013) and Vieira et al. (2015), or inadequate analyses such as flawed PCA applications lacking criteria to screen the meaningful principal components and the variables significantly contributing to each of these components. Concomitantly, the efficiency of space occupation and its related phalanx and guerrilla clonal growth forms were used as indicators of the health of seagrass meadows (Sintes et al., 2005) as well as of meadows of other clonal plants in wetlands and their response to environmental stressors (Chen et al., 2011, 2014, 2017; Geremew et al., 2018). Healthier meadows adopt the phalanx strategy by simultaneously growing new shoots and increasing their biomass. Weak or unhealthy stands adopt the guerilla strategy by simultaneously losing shoots and decreasing their biomass. One aspect that has been at the core of monitoring of seagrass systems has been the widespread use of seagrass population parameters as indicators of health. Following on from that has been the attempt to establish a biomass–density relationship for seagrasses and place it on the core of the metrics and models inferring the health of seagrass stands (Cabaço et al., 2007, 2008, 2013; Romero et al., 2007; Garcı́a-Marín et al., 2013; Jones and Unsworth, 2016, 2019; Jones et al., 2018; Vieira et al., 2018).
Biomass–density relationships started to be investigated in the plant kingdom from the 1960s onward (Yoda et al., 1963; White and Harper, 1970). Some patterns emerged about how biomass and density interact through intraspecific competition and plant growth which led to some generalizations. The most outstanding aspects were the “Law of Constant Final Yield” and the theory related to self-thinning, the concurrent increase in biomass with reduction in density due to mortality through intraspecific competition. The dynamic self-thinning trajectory of plant stands, also known as the “-3/2 power law” (Hutchings, 1979; Weller, 1989), was at the time considered the only theory in ecology worthy of being named a “law” (Harper quotea in Hutchings, 1983). Alongside this, an interspecific boundary line (IBL) was determined as the upper limit for any plant stand on Earth, thus setting the maximum for efficient packing of biomass in space in plant stands (Weller, 1989; Scrosati, 2000). Recently, revised IBLs have been determined for algae (Creed et al., 2019) and for seagrasses (Vieira et al., 2018, 2019). Both the self-thinning theory and IBL determination rely on the stand biomass (B) vs. density (D) plotted on log scales, i.e., log10B=a+b×log10D. In the case of seagrasses, “density” refers to shoots∙m-2 and its original IBL had constants a = 4.569 and b = -0.438 (Vieira et al., 2018). The determination of IBL specific of algae and of seagrasses allowed updated analytical tools offering new perspectives in the research and monitoring of these taxa as well as their communities and ecosystems. Given that the IBL sets the maximum space occupation efficiency, each stand’s distance to the IBL has been used as a robust indicator of how good/healthy (small distance) or how bad/impacted (large distance) the stand is. Following this rationale, the distance to the IBL has been shown as a valuable tool to access the effects of nutrients, pollutants, seasonality, light, temperature, and depth in algae (Creed et al., 2019) and seagrass (Vieira et al., 2018; Creed et al., 2019). Furthermore, because the seagrass IBL is common to all seagrass species, using the distance to the IBL (dgrass) allows different seagrass species to be analyzed together.
Here, we use the dgrass metric as an indicator in order to examine the health of seagrass stands subject to varying concentrations of nitrogen and phosphorus as a consequence of anthropogenic additions to natural background levels. We reanalyze data from previous publications of Zostera noltei Hornemann, 1832, Zostera marina Linnaeus, 1753, Halodule wrightii Asch., Halodule beaudettei Hartog, Halophila baillonii Ascherson, 1874, and Cymodocea nodosa (Ucria) Asch and analyze our own new data from Z. noltei meadows in the Tagus and Sado estuaries, Portugal.
Methods
This study includes results of monitoring of Z. noltei in Portugal, Z. noltei and C. nodosa in Italy, Z. marina in the British Isles, H. wrightii in Brazil, and H. beaudettei and H. baillonii in Costa Rica. The monitoring of Z. noltei in the Tagus and Sado estuaries, Portugal, was an unpublished experiment performed during the summer of 2021. Therefore, below we present the methods in detail. The remaining studies with Z. noltei, Z. marina, H. wrightii, H. beaudettei, and H. baillonii have already been published. Therefore, we present only the fundamental aspects of those studies. Further details about their field sampling and laboratory procedures are provided in their respective publications.
Z. noltei was monitored in the Tagus and Sado estuaries, Portugal (Figure 1A), during the summer of 2021. Sampling took place during July because that is when meadows peak their biomass, density, efficiency of space occupation, and health (Vieira et al., 2018). The rivers Tagus and Sado have meso-tidal estuaries, with a mean tidal amplitude of 2.4 m. Detailed characterizations are available of the geography and hydrodynamics of the Tagus (Dias et al., 2013) and Sado (Biguino et al., 2021) estuaries. Roughly 3 million people live in the region, mainly around the Tagus estuary, where the Portuguese capital, Lisbon, is located. Natural sources of nutrients to these systems are riverine discharge and tidal transport of nutrients upwelled in the coastal ocean during the summer upwelling season. The main anthropogenic sources of nutrients are urban effluents and agriculture, the latter being most intense in livestock and rice fields. The Tagus monitoring included nine stations (Figure 1B). Stations “T.Al1” and “T.Al2” were inside the Tagus Estuary Natural Reserve and thus expected to be the most pristine sites. Station “T.Al3” was along the Alcochete city margin. Stations “T.Sam1,” “T.Sam2,” and “T.Sam3” were next to the Samouco locality. Station “T.Sex” was located at the Seixalinho wastewater treatment plant (WWTP) runoff. Station “T.Rib1” was located at the Ribeiralves fish factory runoff, and “T.Rib2” was approximately 300 m away. All stations were located at mid intertidal heights. The Sado monitoring included six stations (Figure 1C). Station “S.ST” was along the main channel, closest to the estuary inlet and next to the “Sol Troia” resort. It was the only station located at the lower intertidal limit, only emersed during spring tides. In the Sado estuary, stations “S.Com1,” “S.Com2,” and “S.Com3” were located along the Comporta channel whereas stations “S.Cr1” and “S.Cr2” were located in the main channel next to the Carrasqueira village. All these stations were located at mid intertidal heights. The area next to the Comporta and Carrasqueira villages, and landward for tens of km along the main channel is intensively occupied by rice fields. In each location, three cores (11 cm diameter, 20 cm length) were taken from the center of the seagrass patch. Hence, the three distinct dgrass observations corresponded to the three cores taken at each location. While immersed, each location was sampled for water properties (temperature, N, P, and Si) three times: soon after immersion, during peak high tide, and soon before emersion. The data from these three time instances were averaged, hence the nutrient concentrations common to all three data points from each location. In the laboratory, the seagrasses were cleaned from sediment and measured for counts of shoots and nodes. Above-ground and below-ground biomasses were separated, dried in the oven for 48 h at 60°C, and weighed. Samples for nutrients in the water column were filtered through a 0.45-µm membrane (Whatman cellulose acetate) to 25-ml plastic bottles and frozen until analysis in the laboratory. Ammonium and phosphates were analyzed by colorimetry in a Skalar autoanalyzer SAN+ (Hansen and Koroleff, 1999).
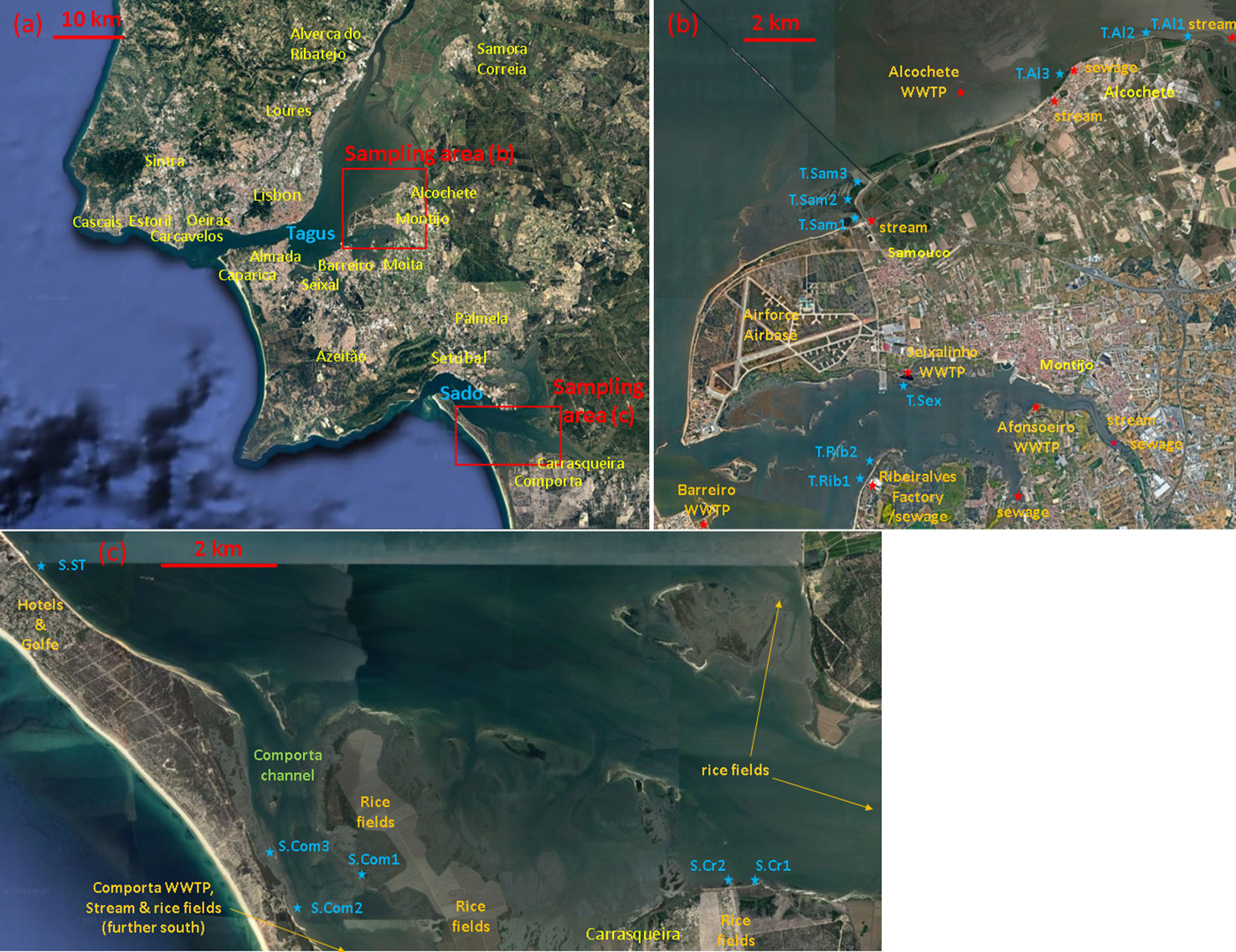
Figure 1 Region of study with the Tagus and Sado estuaries, urban areas, locations of sampling sites, and anthropogenic sources of nutrient enrichment. Aerial photos obtained from Google Maps. (A) Lisbon and Setubal metropolitan areas with Tagus and Sado estuaries, (B) sampling locations in the Tagus estuary, and (C) sampling locations in the Sado estuary.
Z. noltei was monitored in Ria Formosa, south Portugal, during 2001 and 2002, along a distance gradient from Montenegro’s WWTP (Cabaço et al., 2008). One census per season was performed in the stations S1, S2, S3, and S4, located 270, 520, 610, and 1,500 m away from WWTP, respectively. Monitoring included the above- and below-ground biomass, shoot density, and morphometrics of Z. noltei, as well as concentrations of ammonium and phosphates.
Z. marina was monitored at 11 locations along the British Isles (Jones and Unsworth, 2016; Jones et al., 2018). The monitoring during the summer season (May to August 2013) certified that the meadows were sampled during the seasonal peak of biomass, density, efficiency of space occupation, and health (Vieira et al., 2018). Monitoring included the above- and below-ground biomass and shoot density of Z. marina, as well as above-ground δ15N isotopic ratios. The seagrass biomass and density, presented by (Jones and Unsworth, 2016; Jones et al., 2018), were relative to quadrat area (0.25 m2). In this work, as well as in previous ones, we present the data standardized to unit area (1 m2).
C. nodosa and Z. noltei were monitored in an intermixed meadow at 5-m depth in a protected embayment adjacent to the Aragonese Castle on the island of Ischia in the Gulf of Naples, Italy (Kraemer and Mazella, 1999). Monitorization took place from October 1994 to October 1995. Three quadrats were sampled each month. Ammonium concentrations were measured in the water and in the sediment.
H. wrightii was monitored along southeast Brazil (Bertelli et al., 2020). The ecological and environmental characteristics were quantified in April 2017 in 10 seagrass meadows along the coast of Rio de Janeiro and São Paulo states, Brazil. At each site, single transect lines (50 -10 m depending on the size of the meadow) running parallel to the shore were placed through the middle of the seagrass meadow and along the outer (deep) edge of the meadow. Monitoring included above- and below-ground biomass and shoot density of H. wrightii, as well as above-ground concentrations of N and of the δ15N isotopic ratios.
H. beaudettei and H. baillonii were monitored along the Pacific coast of Costa Rica at Potrero during February 2016 (Samper-Villarreal et al., 2018), at Playa Refugio Animal, Playa Colibrí, Golfito, and Puerto Jiménez during May 2016 (Samper-Villarreal and Cortés, 2020); at Potrero, Jobo, and Matapalito during August 2017, December 2017, and May 2018 (Samper-Villarreal et al., 2020); and at Sámara Bay during August 2018 and March 2019 (Samper-Villarreal et al., 2022). Measured seagrass variables included, among others, above- and below-ground biomass, shoot density, and above- and below-ground concentrations of N as well as δ15N isotopic ratios.
Seagrasses couple their growth in biomass and density (Figure 2, Supplementary Material Figure S1, Cabaço et al., 2013, 2019; Vieira et al., 2018), thus allowing the use of the distance to the seagrass IBL (dgrass) as a proxy for their health (Vieira et al., 2018). Adopting the phalanx strategy, healthier meadows approach the IBL (and dgrass approaches zero) by simultaneously growing new shoots and increasing their biomass. Adopting the guerilla strategy, weak or unhealthy stands depart the IBL (and dgrass departs from zero) by simultaneously losing shoots and decreasing their biomass. The dgrass = (log10 − log10B)∙cosθ. Here, is the theoretical maximum biomass given an observed density. It is estimated from the IBL as log10 = a + b∙log10D. Coefficients are a = 4.708, b = − 0.489, θ = arctg(|b|)=0.455, and thus, cosθ = 0.898. The seagrass data compiled to estimate this IBL were here updated with 26 more studies providing 1,221 more observations. The current full data set, with 5,052 observations from 78 studies on 18 seagrass species, confirmed the seagrass clonal growth form (Figures 2, S1) but led to a re-estimation of the seagrass IBL with slightly different coefficients.
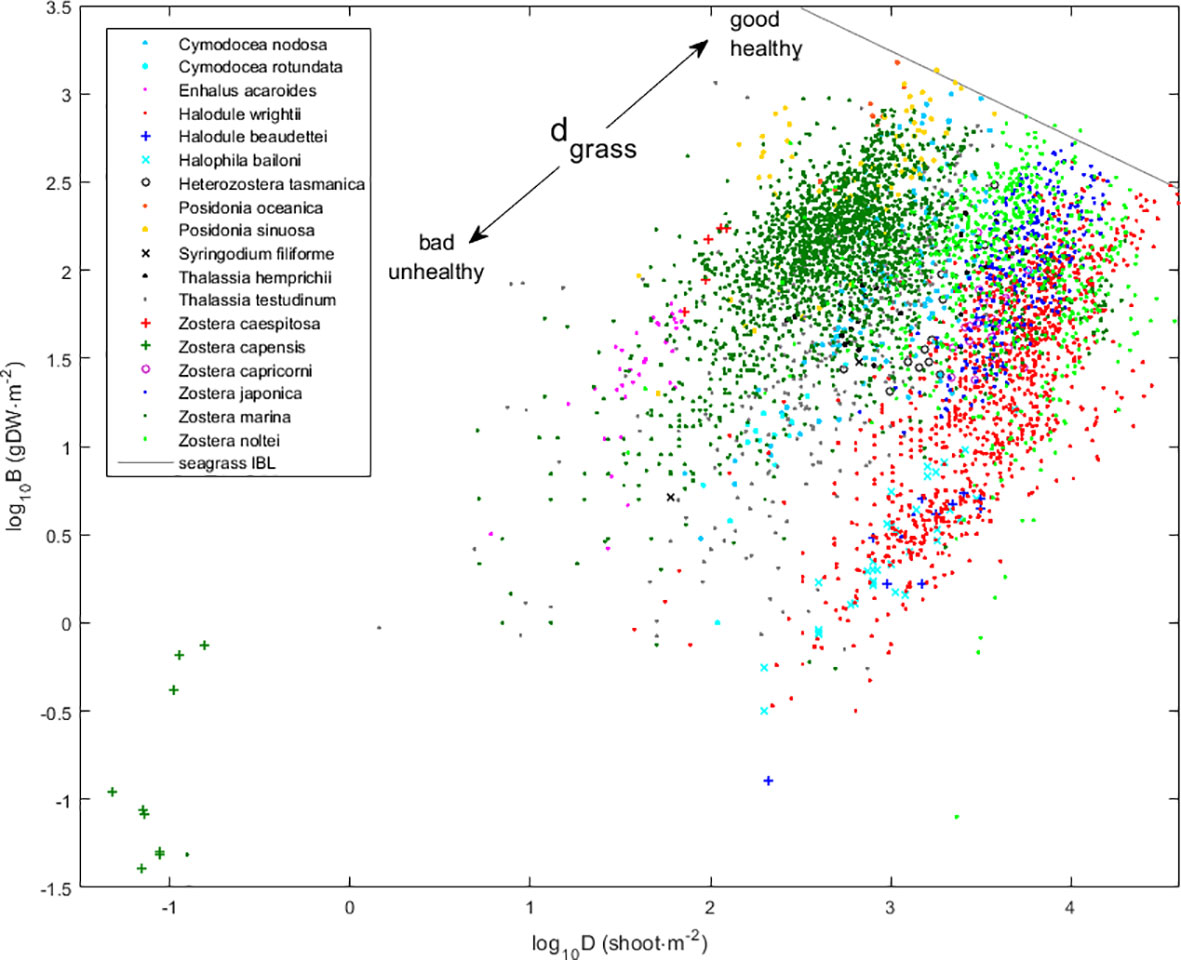
Figure 2 The meta-analysis on the biomass–density relations of 18 seagrass species and the derived seagrass IBL. B, biomass in grams of dry weight (g DW) per square meter; D, density of shoots per square meter.
The dgrass indicator was compared with the concentrations of ammonium, phosphate, and the δ15N isotope. In the studies by (Jones and Unsworth, 2016; Jones et al., 2018), Bertelli et al. (2020); Samper-Villarreal and Cortés (2020), and Samper-Villarreal et al. (2018, 2020, 2022), the proportion of the δ15N isotope to the total N in the leaf content was considered to be an indicator of the N provenience: lower proportions (below 4 ppt) usually correspond to N coming from natural sources, whereas higher proportions (above 4 ppt) usually correspond to N coming from anthropogenic sources, namely, urban sewage and livestock effluents (following Macko and Ostrom, 1994; McClelland et al., 1997; McClelland and Valiela, 1998; Kjønaas and Wright, 2007; Bruland and MacKenzie, 2010; Román et al, 2019).
Third-order polynomials were fit to the non-linear relations between dgrass (response) and nutrients (predictor). Distinct fitting methods were applied, namely, ordinary least squares (OLS), weighted least squares (WLS), iterative reweighted least squares (IRLS), linear-in-the-parameters oblique least squares (LOLS), and maximum likelihood estimation (MLE). A detailed presentation of these methods and their comparison is presented by Vieira et al. (2016). Their application was done using the Matlab software developed by Vieira et al. (2016). The best fits should be those presenting the highest R2 and the lowest root mean square deviation (RMSD, also known as root mean square error: RMSE). However, the data distribution was strongly biased (assymetrical), with many more observations on the side of lower nutrient concentrations. Consequently, the highest R2 and the lowest RMSD optimized the fit on the side of lower nutrient concentrations but at the cost of poor fits on the side of higher nutrient concentrations. This problem was overcome by attributing relative weights to the data on each side and applying an MLE using the Newton–Raphson method (Vieira et al., 2016).
Results
The Z. noltei meadows monitored in south and central Portugal, namely, in Ria Formosa and in the Tagus and Sado estuaries, had smaller dgrass (indicative of healthier meadows) under moderate nutrient concentrations in the water (Figures 3A, B). The healthier Ria Formosa meadow was S2 located 510 m away from the WWTP (Figure 3C). The only time S2 was in a poor condition was during winter under a peak of excess ammonium. In the Tagus estuary, the healthier meadows were located exactly next to the Seixalinho WWTP and the Ribeiralves factory effluent (Figure 3D), where the ammonium concentrations were similar to those observed for the Ria Formosa meadows S2 and S3 located at intermediate distances from the Montenegro WWTP (Figures 3C, D). Under lower nutrient loads, the dgrass of Z. noltei meadows increased (i.e., departed the IBL), suggesting that they were under nutrient deprivation. These lower loads occurred in the Ria Formosa and Tagus meadows furthest away from the WWTPs, and in the Sado meadows, which were all far from WWTP (Figures 3C, D). Furthermore, the Sado meadows were even less healthy than the Tagus and Ria Formosa meadows under equivalently low ammonium loads (Figure 3A), suggesting that the lack of phosphate worsened its condition (Figure 3B). Under the highest nutrient loads, Z. noltei meadows also increased dgrass (i.e., departed the IBL), suggesting that they were suffering from toxicity (Figures 3A, B). This happened in the Ria Formosa S1 meadow located closer to the Montenegro WWTP. Comparison with Z. noltei meadows from other locations and reported in other studies shows that the meadows from Ria Formosa and the Tagus estuary under moderate anthropogenic nutrient additions were among the healthier ever reported (Figure 3).
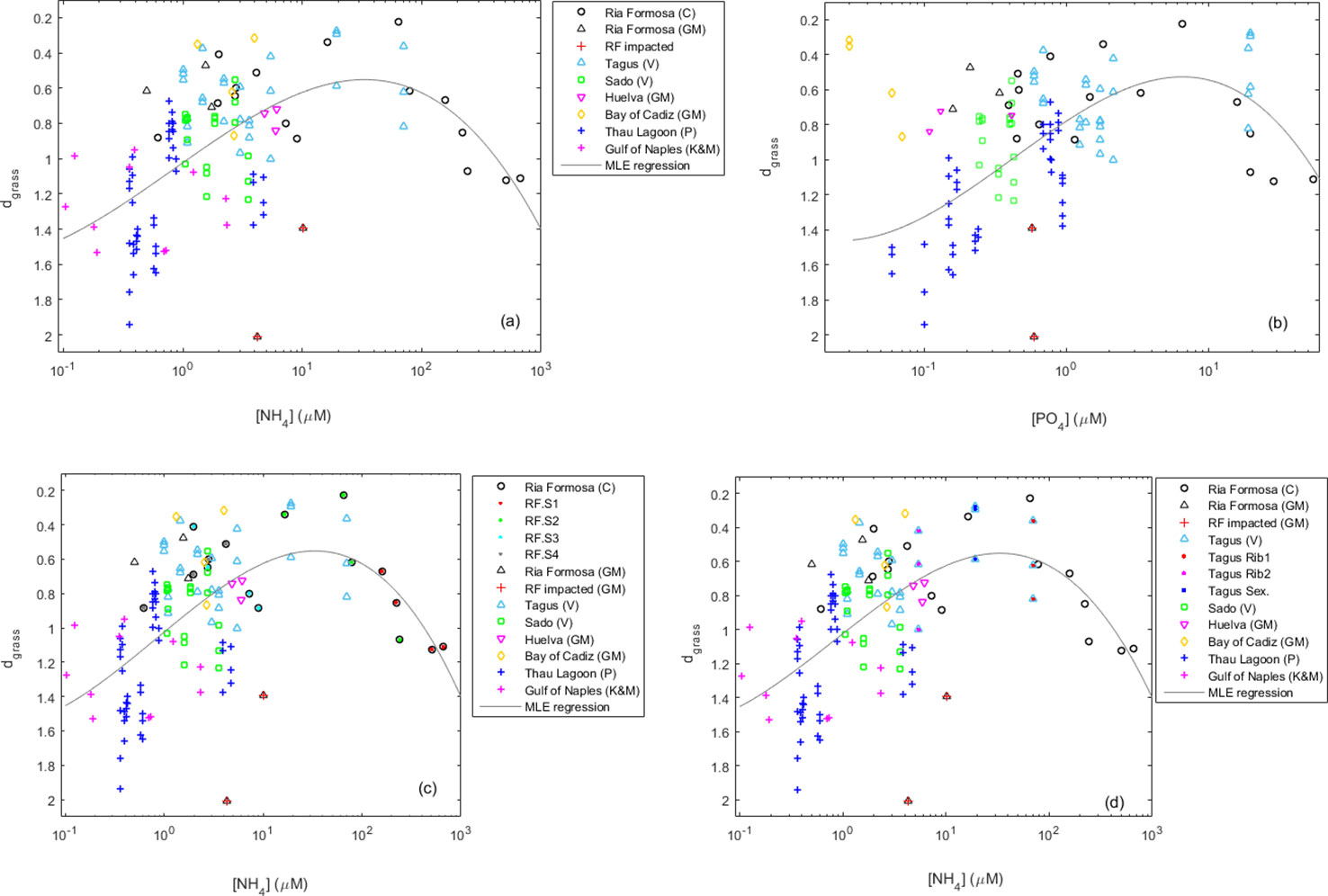
Figure 3 Health of Zostera noltei meadows in Portugal, namely, in the Ria Formosa lagoon system and the Tagus and Sado estuaries, and its dependency on ammonium (A, C, D) and phosphate (B) concentrations in the water. Z. noltei meadows in Portugal are compared with other Z. noltei meadows from elsewhere. Original data comes from this study (V), Kraemer and Mazella (1999) (K&M), Plus et al. (2001) (P), Cabaço et al. (2008) (C), and Garcı́a-Marín et al. (2013) (GM). The Z. noltei health is indicated by its efficiency of space occupation, in its turn indicated by its distance (dgrass) to the seagrass IBL. Panel (C) highlights the Ria Formosa (RF) case, with stations S1, S2, S3, and S4 sorted in increased distance from Montenegro’s WWTP. Panel (D) highlights the Tagus case. The Seixalinho station (Sex) is immediately outside Seixalinho WWTP, whereas Ribeiralves stations are immediately outside (Rib1) and 300 m away (Rib2) from Ribeiralves fish factory effluent. Trends fit by maximum likelihood estimation (MLE).
The C. nodosa and Z. noltei meadows monitored in the Bay of Naples, Italy, had smaller dgrass (indicative of healthier meadows) under intermediate nutrient concentrations in the water and in the sediment (Figure 4). It is noteworthy that both species exhibited very similar responses.
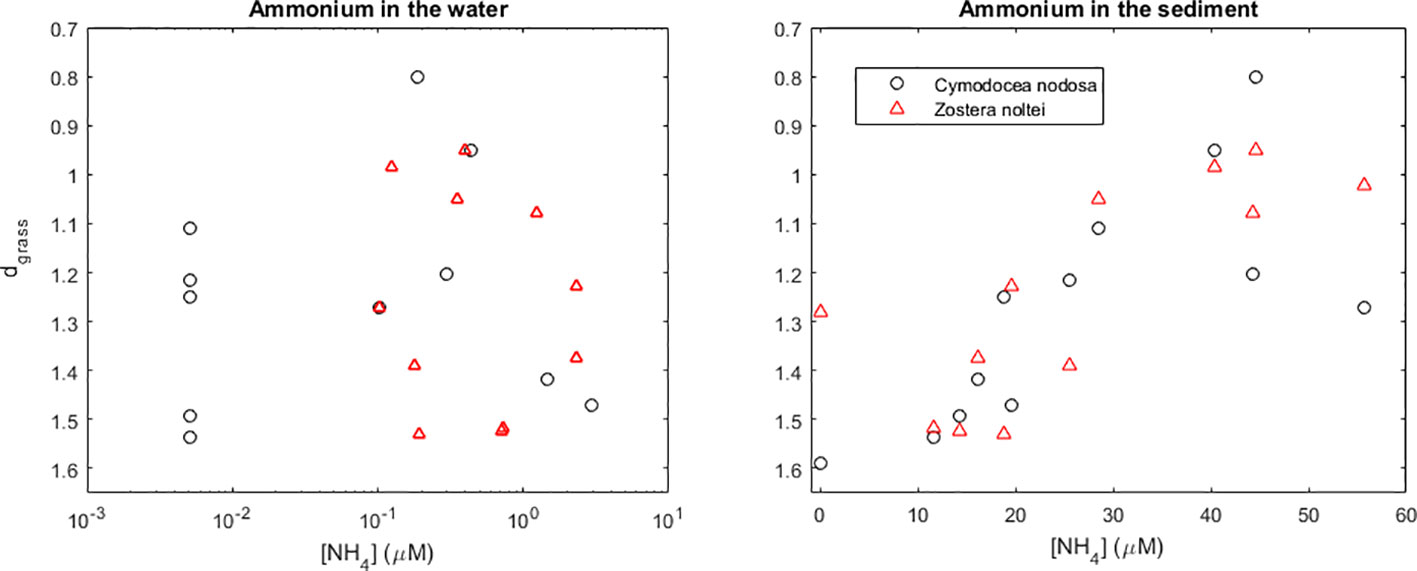
Figure 4 Health of Cymodocea nodosa and Zostera noltei meadows in the Bay of Naples, Italy, and their relation with the ammonium concentration in the water and sediment. Seagrass health is indicated by their distance (dgrass) to the seagrass IBL (i.e., their efficiency of space occupation). In some locations, samples were taken from both the middle (Middle) and the edge (Edge) of the meadow. Trends fit by maximum likelihood estimation (MLE).
In order to have a global perspective, we plotted all data showing how the health of seagrass meadows may depend on the concentrations of ammonium or phosphate, in the water or in the sediment (Figure 5). Overall, seagrasses improved their efficiency of space occupation (and thus, their health) as nutrient concentrations increased, with the healthier meadows occurring under moderate nutrient concentrations. Only under the most extreme nutrient concentrations observed in this dataset were the seagrass meadows less healthy. From all these cases, the healthier meadows, occupying almost all the space available (i.e., dgrass close to zero), were located in coastal systems subject to anthropogenic pressure, as were the cases of Z. noltei in Ria Formosa (from Cabaço et al., 2008), in the Tagus estuary (from this study), and in the Bay of Cadiz (from Garcı́a-Marín et al., 2013), and of Z. japonica in Dadae Bay (from Lee et al., 2006), in Koje Bay (from Kim et al., 2018), and in Willapa Bay (from Ruesink et al., 2009). It was also the case of T. testudinum in Laguna Madre and in Corpus Christy Bay, for both the control and the ammonium-enriched meadows (from Lee and Dunton, 2000).
The Z. marina meadows in the British Isles showing smaller dgrass (thus in better health) were in Priory Bay, Isle of Wight, Mannin Bay, Ireland, and Kircubbin Bay, Northern Ireland (Figure 6). With δ15N within 5 to 11 ppt, these meadows were clearly under anthropogenic nutrient input. They were particularly better than the meadow sampled in the pristine Isles of Sicily (with δ15N ≤5 ppt), or the heavily impacted meadows sampled in Studland Bay (with δ15N within 11 to 13 ppt) or in Southend-On-Sea (with δ15N >15 ppt). Nevertheless, there were also meadows showing δ15N signals suggesting moderate-to-large anthropogenic sources of N (5 ppt< δ15N <10 ppt) for which the dgrass was large, thus indicating unhealthy stands. Therefore, there was something else in addition to the source of N that severely affected the health of Z. marina meadows in the British Isles.
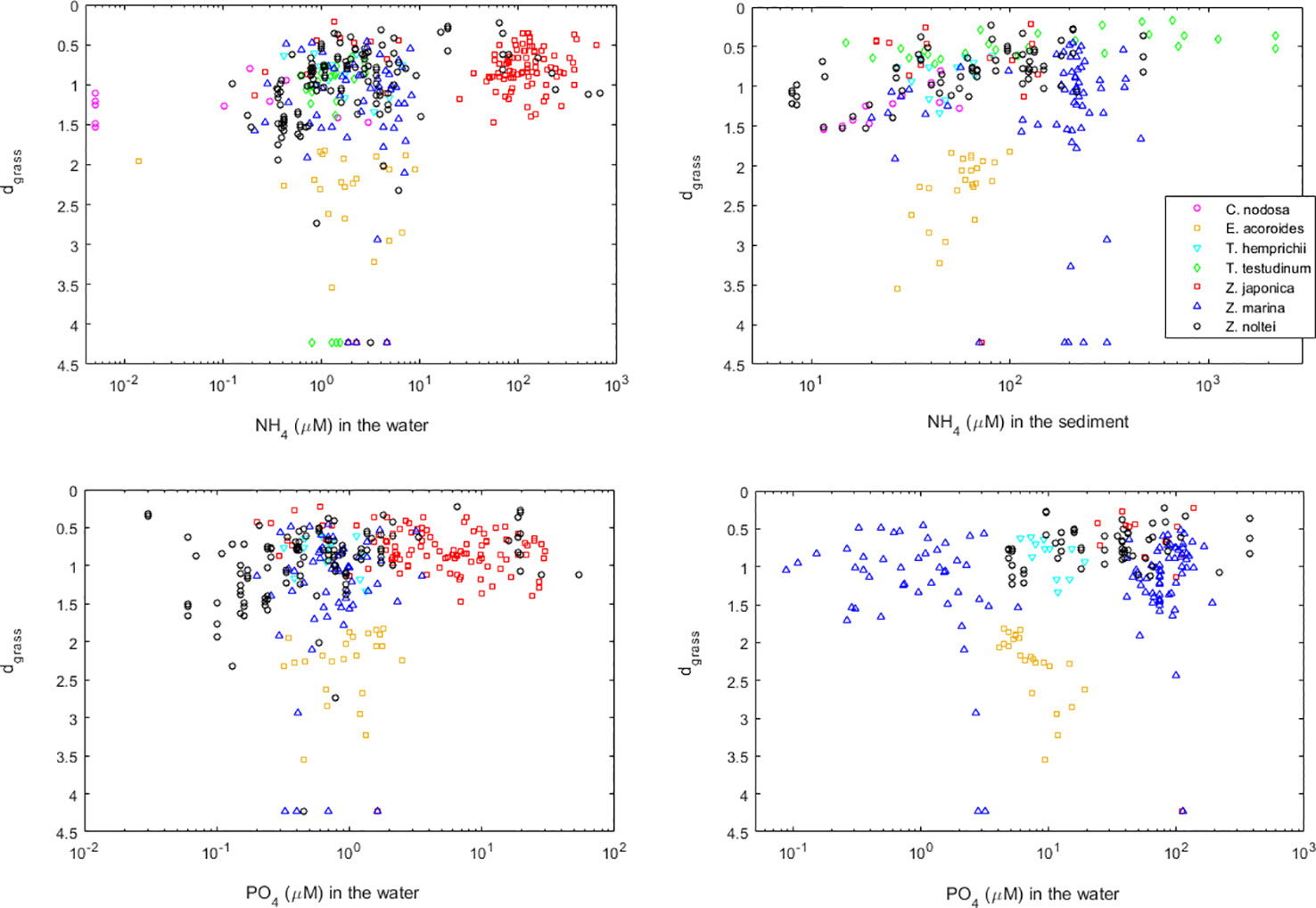
Figure 5 Health of seagrasses worldwide and their relation with the concentrations of ammonium and phosphate in the water and in the sediments. Seagrass health is indicated by their distance (dgrass) to the seagrass IBL (i.e., their efficiency of space occupation).
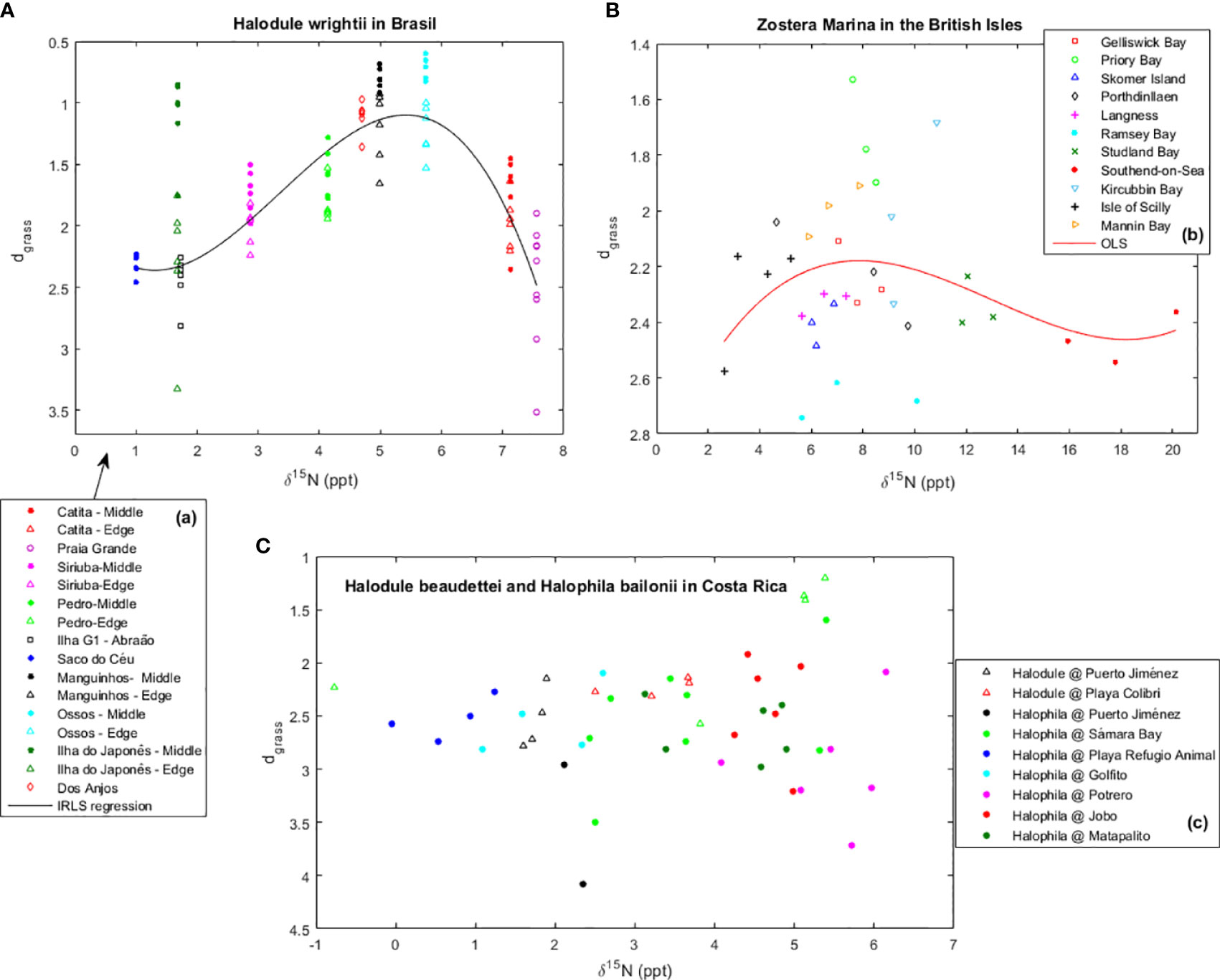
Figure 6 Health of (A) Halodule wrightii meadows in Brazil, (B) Zostera marina meadows in the British Isles, and (C) Halodule beaudettei and Halophila baillonii meadows in Costa Rica and their relation with the proportion of the δ15N isotope to total N in seagrass leaf content. Seagrass health is indicated by their distance (dgrass) to the seagrass IBL (i.e., their efficiency of space occupation). In some locations, samples were taken from both the middle (Middle) and the edge (Edge) of the meadow. Trends fit by maximum likelihood estimation (MLE).
The H. wrightii in Brazil, as well as the H. beaudettei and H. baillonii in Costa Rica, benefited from moderate nutrient enrichment (Figure 6). The meadows showing smaller dgrass were those with a δ15N proportion within 4 to 6 ppt, indicating moderate anthropogenic N enrichment. For the H. wrightii in Brazil, these meadows were in Ossos, Manguinhos, dos Anjos, and Pedro. The meadows showing δ15N proportions either above (Catita and Praia Grande) or below (Siriuba, Ilha do Japonês, Ilha G1 - Abraão and Saco do Céu) these bounds also showed larger dgrass. Furthermore, the samples taken from the middle of the meadow systematically indicated better health than the samples taken from the edge. For H. beaudettei and H. baillonii in Costa Rica, within each location sampled at different time instances, the respective meadow was generally healthier (smaller dgrass) as the proportion of δ15N in above-ground biomass increased. In order to have a global perspective, we plotted all data showing how the health of seagrass meadows may depend on anthropogenic nutrient additions as inferred from the proportion of the δ15N isotope (Figure 7). Overall, seagrasses were healthier under moderate nutrient addition. For the tropical seagrasses, the optimal δ15N was roughly around 5 to 6 ppt, whereas for temperate seagrasses, the optimal δ15N was slightly higher, around 8 ppt.
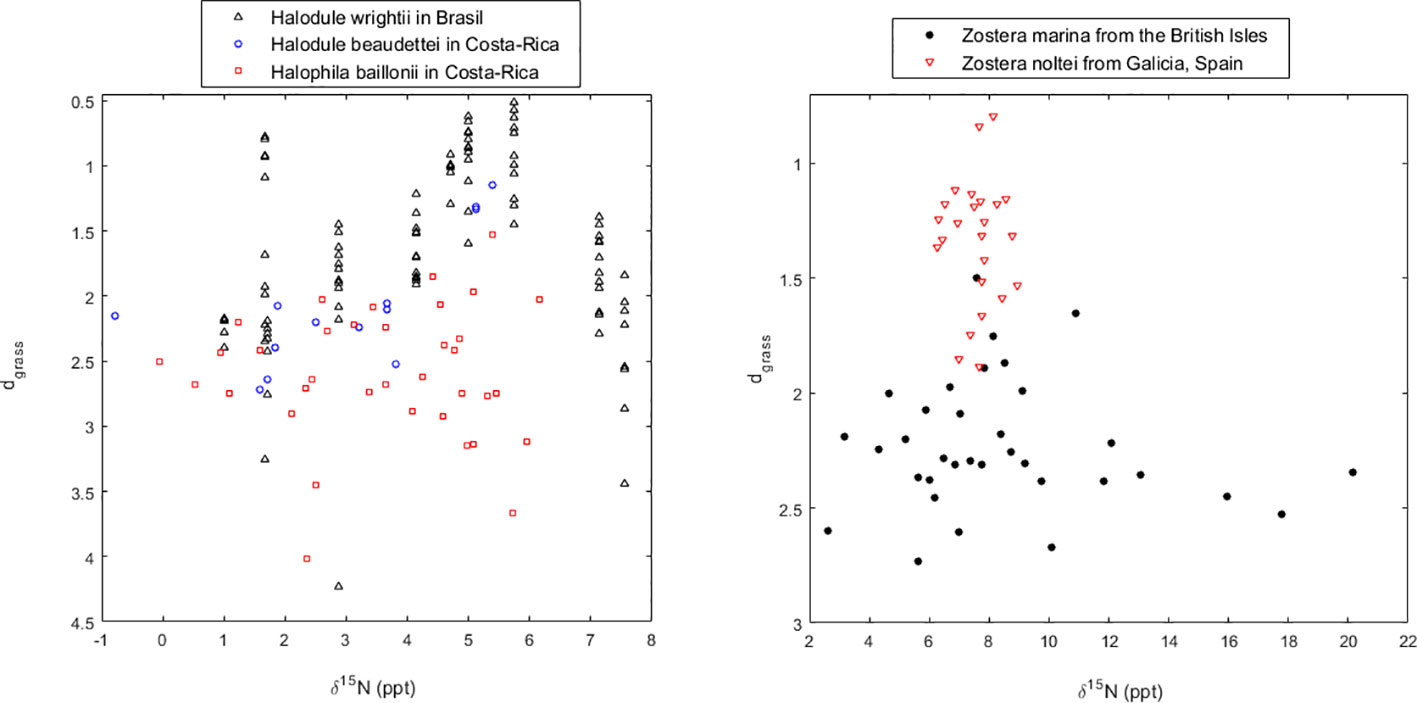
Figure 7 Health of seagrasses worldwide and their relation with the proportion of the δ15N isotope to total N in seagrass leaf content. Seagrass health is indicated by their distance (dgrass) to the seagrass IBL (i.e., their efficiency of space occupation).
Discussion
The monitoring of Z. noltei in Portugal showed that the nutrient concentrations immediately next to a WWTP and a food factory in the heavily populated Tagus estuary (outside the Natural Reserve) during the summer of 2021 were only as moderate as the nutrient concentrations 500 and 600 m away from a WWTP inside the Ria Formosa Natural Reserve during 2001 and 2002. Under these moderate nutrient concentrations were found the healthier Z. noltei meadows ever reported (considering their efficiency of space occupation). The main determinant of their health seems to have been the concentration of ammonium in the water. Concentrations too high were toxic for Z. noltei whereas concentrations too low were limiting for their growth. Nevertheless, under similarly low ammonium concentrations, the Sado meadows were seemingly less healthy than the Tagus and Ria Formosa meadows. The reason for this may have been the significantly lower phosphate concentrations in the Sado estuary. The increased vulnerability to ammonium and the light environment under phosphate deficiency had already been reported for Z. noltei in the Bay of Cadiz, Spain (Brun et al., 2008). The present contrast between Sado and the other sites, particularly regarding the phosphate concentrations, may result from the distinct types of anthropogenic inputs. Rice crops are abundant both in the Sado River and in the Tagus river and its Sorraia tributary. Rice is not cultivated anywhere within the Ria Formosa basin. However, in the Sado estuary rice crops are the main source of anthropogenic inputs, with crops abounding in the estuary and right next to the sampling sites. In the Tagus estuary, urban effluents and livestock gain preponderance, with many being located right next to the sampling sites, whereas rice crops are located many kms further upstream. Rice (Oryza sp.) growth and production have a high demand and uptake of P, with crops generally being limited by P availability and consumption (Beyrouty et al., 1994; Akinrinde and Gaizer, 2006; Julia et al., 2016; Rajaona et al., 2017; Jiang et al., 2021). It is therefore feasible that the P concentrations observed in the Sado estuary lower than the observed in the Tagus estuary and Ria Formosa are driven by P uptake by rice crops and that this relative P deficiency drives the relatively less healthy status of the Z. noltei meadows in the Sado estuary. The monitoring of Z. marina in the British Isles, of H. wrightii in Brazil, and of H. beaudettei and H. baillonii in Costa Rica showed that the meadows in better conditions were found in locations subject to mild or moderate nutrient additions to the natural background. In the cases of the tropical H. wrightii, H. beaudettei, and H. baillonii, the optimal proportion of the δ15N isotope to the total N in leaf content was roughly within 4 to 5 ppt. In the case of the temperate Z. marina and Z. noltei, the optimal range placed slightly higher around 8 ppt. The meadows under lower nitrogen enrichment were less healthy, presumably due to nitrogen deficiency, whereas meadows under higher nitrogen enrichment were also less healthy, presumably due to nitrogen toxicity. Overall, our results suggest that H. wrightii meadows in Brazil, H. beaudettei and H. baillonii in Costa Rica, Z. noltei meadows in Portugal, and Z. marina meadows in the British Isles may benefit from anthropogenic nitrogen additions, as long as these additions are moderate, and that such meadows can in fact do better than the meadows in pristine environments.
Our findings match previous studies on the physiological and demographic responses of seagrass beds to nutrient concentrations. The review by Touchette and Burkholder (2000) also determined that many seagrass species respond favorably to mild or moderate nutrient enrichment and that only when enrichment is too high do the seagrasses respond unfavorably with decreasing survival and/or growth. In this case, the negative response can be a direct physiological response to N or P toxicity or an indirect effect of eutrophication due to stimulation of algal overgrowth or smothering and associated light reduction. In the review by Waycott et al. (2005) of four seagrass species in the Great Barrier Reef, nutrients were only reported as limiting growth due to their weak supply. N or P toxicity was never found. Reported pollutants with negative impacts were only herbicides, pesticides, and other chemical contaminants. Our findings, together with the concordant previous findings reported above, contradict the former generalized negative perception that anthropogenic nutrient additions, however small these additions may be, are always harmful to seagrass beds.
A large contribution to this biased perception may have come from the lack of an adequate indicator for the health of seagrass meadows. This handicap has been solved by the distance to the seagrass IBL (dgrass), reflecting the efficiency of space occupation by the respective stand. The application of this new indicator to several species scattered worldwide showed that all monitored species benefit from moderate anthropogenic nutrient additions, which explains the recent recovery of seagrass meadows in populated coastlines where the improved management of wastewaters reduced but not eradicated the anthropogenic input of nutrients (Greening et al., 2014; Bertelli et al., 2018; de los Santos et al., 2019; Burdick et al., 2020; Orth et al., 2020). Furthermore, our results suggest that, in situations where WWTP are the main source of anthropogenic nutrient enrichment (i.e., agricultural runoff is reduced), modern wastewater treatment cleans the wastewater well enough to improve the health of nearby seagrass meadows, and their level of reduced nutrient inputs may even promote seagrass growth.
Seagrasses provide many ecosystem services, including CO2 trapping, as well as shelter and food for commercially exploited species (Nordlund et al., 2016; de los Santos et al., 2020). Furthermore, the restoration of formerly deteriorated seagrass habitat leads to rapid recovery of coastal ecosystem services (Orth et al., 2020). Here, we determined that moderate anthropogenic nutrient additions have the potential to enhance these ecosystem services in seagrass meadows. Instead of trying to minimize the nutrient load in effluents from human origin as much as possible, society might better divert its focus to identifying maximal (beneficial) nutrient loads at specific sites for different species as well as improving strategies to maximize nutrient dilution when discharged to the sea, so that the threshold concentrations for inorganic nitrogen and phosphorus to become toxic are not exceeded.
In addition to the detrimental concentrations of inorganic N and P, seagrass meadows are subject to a multitude of other stressors, acting alone or in consonance. Large nutrient inputs result in eutrophication, which, in estuarine and lagoon systems, take the form of extensive algal blooms often smothering seagrass meadows (Brun et al., 2003; Burkholder et al., 2007; Pergent et al., 2008; Cognat et al., 2018), or epiphytes and phytoplankton reducing light availability (Brun et al., 2003; Plus et al., 2003). Such was the case for Z. noltei in the Tagus estuary, with particular incidence in Samouco, where an extensive mat of mainly Ulva sp. but also some Enteromorpha sp. and Sargassum sp. covered almost all of the seafloor. During the summer of 2021 monitoring, patches with Z. noltei were sparce, and the healthy but sparse Samouco patches still showed some algae coverage (Supplementary Figure S2). During a subsequent monitoring in the summer of 2022, patches with Z. noltei in Samouco were even harder to find among the many tons of green algae, but the few patches left showed some of the best efficiencies of space occupation (dgrass) reported in the data set of seagrasses worldwide. This sparse coverage of extremely healthy Z. noltei patches mingled among an extensive green algae bloom suggests that moderate anthropogenic nutrient additions may lead seagrass meadows to their best health provided that the collateral effects of eutrophication are avoided. Otherwise, the formation of extensive algal mats covering the seagrasses, epiphytes, and/or phytoplankton becomes largely detrimental to seagrass meadows (Brun et al., 2003; Plus et al., 2003; Burkholder et al., 2007; Pergent et al., 2008; Cognat et al., 2018; and present study). Also, temperature affects and interacts with other factors that determine seagrass performance. Temperature affects the photosynthetic efficiency (P–I) curve of seagrasses (Plus et al., 2005; Lee et al., 2007; Vieira et al., 2018), their nutrient uptake and metabolic rates (Plus et al., 2001, 2003; Lee et al., 2007), and the toxicity of inorganic N and P, herbicides, and trace metals (Gamain et al., 2018). In the Tagus and Sado estuaries, during the summer, water temperature was always above 24°C and in their landward sections often above 30°C, which is considered too warm for temperate seagrasses (Lee et al., 2007). However, the largest stressor to Z. noltei in the Tagus and Sado estuaries was by far clam harvesting. During the July 2021 monitoring, the Z. noltei meadows in the Ribeiralves stations were composed of a few healthy patches interspersed within the bare sediment, with the daily pressure of tens of clandestine clam harvesters plucking their shoots and breaking their roots. By August 2021, these meadows had entirely disappeared, never to reappear thus far. The same happened in many other stations and locations in the Tagus and Sado estuaries. As examples, the Z. noltei meadow in the original Comporta 2 station had also disappeared by July 2022. The beach front along Alcochete is abundant in clandestine recreational clam harvesters daily destroying Z. noltei patches spersed within the bare sediment (Supplementary Figure S3).
Data availability statement
The original contributions presented in the study are provided online at http://dx.doi.org/10.13140/RG.2.2.32343.14240.
Author contributions
VV contributed to the conceptualization, design, field sampling, model and software development, data analysis, and writing of the manuscript. RS, DL-S, JC, CB, and JS-V contributed to the field sampling. JL-A, RS, DL-S, AV, JN, MN, JC, CB, and MP contributed to the laboratorial work. JL-A, MN, JC, CB, and JS-V contributed to reviewing the manuscript. All authors contributed to the work and approved the submitted version.
Funding
This work was funded by Portuguese National Funding through FCT - Fundação para a Ciência e Tecnologia, I. P., through project LA/P/0083/2020 (LA LARSyS - MARETEC) and project LA/P/0069/2020 granted to the Associate Laboratory ARNET. The present work was supported by the Marine and Environmental Sciences Centre (MARE) which is financed by national funds from FCT/MCTES (UIDB/04292/2020) Joana M. Neves was supported by a PhD fellowship (UI/BD/150954/2021) from FCT. This work was supported by Fundação Carlos Chagas Filho de Amparo à Pesquisa do Estado do Rio de Janeiro (JCC, FAPERJ- E26/201.286/2014) and Conselho Nacional de Desenvolvimento Científico e Tecnológico (JCC, CNPq- 307117/2014–6) and Santander Bank staff mobility funding through Swansea University, UK. Studies in Costa Rica were funded by the Vicerrectoría de Investigación at the Universidad de Costa Rica. The funders took no part in the design of the study, in the collection, analysis, and interpretation of data, and in writing the manuscript.
Acknowledgments
Our acknowledgments are given to Instituto para a Conservação da Natureza e das Florestas (ICNF), Reserva Natural do Estuario do Tejo (RNET), and Reserva Natural do Estuário do Sado (RNES) for authorizing sampling Zostera noltei in their natural parks, and to OceanAlive.org for the support in planning the Sado field campaign. To Benjamin Jones for providing additional information.
Conflict of interest
The authors declare that the research was conducted in the absence of any commercial or financial relationships that could be construed as a potential conflict of interest.
Publisher’s note
All claims expressed in this article are solely those of the authors and do not necessarily represent those of their affiliated organizations, or those of the publisher, the editors and the reviewers. Any product that may be evaluated in this article, or claim that may be made by its manufacturer, is not guaranteed or endorsed by the publisher.
Supplementary material
The Supplementary Material for this article can be found online at: https://www.frontiersin.org/articles/10.3389/fmars.2022.960249/full#supplementary-material
References
Akinrinde E., Gaizer T. (2006). Differences in the performance and phosphorus-use efficiency of some tropical rice (Oryza sativa l.) varieties. Pak. J. Nutr. 5 (3), 206–211. doi: 10.3923/pjn.2006.206.211
Bedulli C., Lavery P. S., Harvey M., Duarte C. M., Serrano O. (2020). Contribution of seagrass blue carbon toward carbon neutral policies in a touristic and environmentally-friendly island. Front. Mar. Sci. 7. doi: 10.3389/fmars.2020.00001
Bertelli C. M., Creed J. C., Nuuttila H. K., Unsworth R. K. F. (2020). The response of the seagrass halodule wrightii ascherson to environmental stressors. Est. Coast. Shelf Sci. 238, 106693. doi: 10.1016/j.ecss.2020.106693
Bertelli C. M., Robinson M. T., Mendzil A. F., Pratt L. R., Unsworth R. K. F. (2018). Finding some seagrass optimism in Wales, the case of zostera noltii. Mar. pollut. Bull. 134, 216–222. doi: 10.1016/j.marpolbul.2017.08.018
Bester K. (2000). Effects of pesticides on seagrass beds. Helgol Mar. Res. 54, 95–98. doi: 10.1007/s101520050007
Beyrouty C. A., Grigg B. C., Norman R. J., Wells B. R. (1994). Nutrient uptake by rice in response to water management. J. Plant Nutr. 17 (1), 39–55. doi: 10.1080/01904169409364708
Biguino B., Sousa F., Brito A. C. (2021). Variability of currents and water column structure in a temperate estuarine system (Sado estuary, Portugal). Water 13 (2), 187. doi: 10.3390/w13020187
Bruland G. L., MacKenzie R. A. (2010). Nitrogen source tracking with δ n content of coastal wetland plants in Hawaii. J. Environ. Qual. 39, 409–419. doi: 10.2134/jeq2009.0005
Brun F., Olivé I., Malta E., Vergara J., Hernández I., Pérez-Lloréns J. (2008). Increased vulnerability of zostera noltii to stress caused by low light and elevated ammonium levels under phosphate deficiency. Mar. Ecol.Prog. Ser. 365, 67–75. doi: 10.3354/meps07512
Brun F. G., Vergara J. J., Navarro G., Hernández I., Pérez-Lloréns J. L. (2003). Effect of shading by ulva rigida canopies on growth and carbon balance of the seagrass zostera noltii. Mar. Ecol. Prog. Ser. 265, 85–96. doi: 10.3354/meps265085
Burdick D. M., Edwardson K. J., Gregory T., Matso K., Mattera T., Paly M., et al. (2020). A case for restoration and recovery of zostera marina l. in the great bay estuary. PREP Rep. Publ. 441, 1–19.
Burkholder J. M., Tomasko D. A., Touchette B. W. (2007). Seagrasses and eutrophication. J. Exp. Mar. Biol. Ecol. 350, 46–72. doi: 10.1016/j.jembe.2007.06.024
Cabaço S., Alexandre A., Santos R. (2005). Population-level effects of clam harvesting on the seagrass zostera noltii. Mar. Ecol. Prog. Ser. 298, 123–129. doi: 10.3354/meps298123
Cabaço S., Apostolaki E. T., Garcıa-Marín P., Gruber R., Hernandez I., Martınez-Crego B., et al. (2013). Effects of nutrient enrichment on seagrass population dynamics: evidence and synthesis from the biomass–density relationships. J. Ecol. 101, 1552–1562. doi: 10.1111/1365-2745.12134
Cabaço S., Machás R., Santos R. (2007). Biomass–density relationships of the seagrass zostera noltii: A tool for monitoring anthropogenic nutrient disturbance. Estuar. Coast. Shelf. Sci. 74, 557–564. doi: 10.1016/j.ecss.2007.05.029
Cabaço S., Machás R., Vieira V., Santos R. (2008). Impacts of urban wastewater discharge on seagrass meadows (Zostera noltii). Estuar. Coast. Shelf. Sci. 78, 1–13. doi: 10.1016/j.ecss.2007.11.005
Chen X.-S., Deng Z.-M., Xie Y.-H., Li F., Hou Z.-Y., Li X. (2014). Demography of carex brevicuspis (Cyperaceae) rhizome populations: A wetland sedge that produces both elongated and shortened rhizomes. Nord. J. Bot. 32, 251–256. doi: 10.1111/j.1756-1051.2013.00094.x
Chen X., Liao Y., Xie Y., Li F., Deng Z., Hou Z., et al. (2017). Concurrent effects of sediment accretion and nutrient availability on the clonal growth strategy of carex brevicuspis – a wetland sedge that produces both spreading and clumping ramets. Front. Plant Sci. 8. doi: 10.3389/fpls.2017.01685
Chen X.-S., Xie Y.-H., Deng Z.-M., Hou Z.-Y. (2011). A change from phalanx to guerrilla growth form is an effective strategy to acclimate to sedimentation in a wetland sedge species carex brevicuspis (Cyperaceae). Flora 206 (4), 347–350. doi: 10.1016/j.flora.2010.07.006
Cognat M., Ganthy F., Auby I., Barraquand F., Rigouin L., Sottolichio A. (2018). Environmental factors controlling biomass development of seagrassmeadows of zostera noltei after a drastic decline (Arcachon bay, France). J. Sea Res. 140, 87–104. doi: 10.1016/j.seares.2018.07.005
Creed J., Vieira V.M.N.C.S., Norton T. A., Caetano D. (2019). A meta-analysis shows that seaweeds surpass plants, setting life-on-Earth’s limit for biomass packing. BMC Ecol. 19 (6), 1–11. doi: 10.1186/s12898-019-0218-z
Dias J. M., Valentim J. M., Sousa M. C. (2013). A numerical study of local variations in tidal regime of tagus estuary, Portugal. PloS One 8 (12), e80450. doi: 10.1371/journal.pone.0080450
Espel D., Diepens N. J., Boutron. O., Buffan-Dubaua E., Chéraind Y., Coulet E., et al. (2019). Dynamics of the seagrass zostera noltei in a shallow Mediterranean lagoon exposed to chemical contamination and other stressors. Est. Coast. Shelf Sci. 222, 1–12. doi: 10.1016/j.ecss.2019.03.019
Fraser M. W., Kendrick G. A. (2017). Belowground stressors and long-term seagrass declines in a historically degraded seagrass ecosystem after improved water quality. Sci. Rep. 7 (1), 2045–2322. doi: 10.1038/s41598-017-14044-1
Gamain P., Feurtet-Mazel A., Maury-Brachet R., Auby I., Pierron F., Belles A., et al. (2018). Can pesticides, copper and seasonal water temperature explain the seagrass zostera noltei decline in the arcachon bay? Mar. Poll. Bull. 134, 66–74. doi: 10.1016/j.marpolbul.2017.10.024
García-Marín P., Cabaço S., Hernández I., Vergara J. J., Silva J., Santos R. (2013) Multimetric index based on the seagrass zostera noltii (ZoNI) for ecological quality assessment of coastal and estuarine in SW Iberian peninsula. Mar. pollut. Bull. 68, 46–54. doi: 10.1016/j.marpolbul.2012.12.025
Garmendia J. M., Valle M., Borja Á., Chust G., Lee D.-J., Rodríguez J. G., et al. (2017). Effect of trampling and digging from shellfishing on zostera noltei (Zosteraceae) intertidal seagrass beds. Sci. Mar. 81 (1), 121–128. doi: 10.3989/scimar.04482.17A
Geremew A., Stiers I., Sierens T., Kefalew A., Triest L. (2018). Clonal growth strategy, diversity and structure: A spatiotemporal response to sedimentation in tropical cyperus papyrus swamps. PloS One 13 (1), e0190810. doi: 10.1371/journal.pone.0190810
Greening H., Janicki A., Sherwood E. T., Pribble R., Johansson J. O. R. (2014). Ecosystem responses to long-term nutrient management in an urban estuary: Tampa bay, Florida, USA. Estuar. Coast. Shelf. Sci. 151, A1–A16. doi: 10.1016/j.ecss.2014.10.003
Hamdaoui O., Ibos L., Mazioud A., Safi M., Limam O. (2018). Thermophysical characterization of posidonia oceanica marine fibers intended to be used as an insulation material in Mediterranean buildings. Constr. Build. Mater. 180, 68–76. doi: 10.1016/j.conbuildmat.2018.05.195
Hansen H., Koroleff F. (1999). “Determination of nutrients,” in Methods of seawater analysis. Eds. Grasshoff K., Kremling K., Ehrhardt M. (Weinheim: Wiley-VCH Verlag), 159–226.
Hutchings M. J. (1979). Weight–density relationships in ramet populations of clonal perennial herbs, with special reference to the 3/2 power law. J. Ecol. 67, 21–33. doi: 10.2307/2259334
Hyman A. C., Frazer T. K., Jacoby C. A., Frost J. R., Kowalewski M. (2019). Long-term persistence of structured habitats: seagrass meadows as enduring hotspots of biodiversity and faunal stability. Proc. R. Soc B. 286, 1912 doi: 10.1098/rspb.2019.1861
Jedidi M., Abroug A. (2020). Valorization of posidonia oceanica balls for the manufacture of an insulating and ecological material. Jordan J. Civ. Eng. 14 (3), 417–430.
Jiang B., Shen J., Sun M., Hu Y., Jiang W., Wang J., et al. (2021). Soil phosphorus availability and rice phosphorus uptake in paddy fields under various agronomic practices. Pedosphere 31 (1), 103–115. doi: 10.1016/S1002-0160(20)60053-4
Jones B. L., Cullen-Unsworth L. C., Unsworth R. F. K. (2018). Tracking nitrogen source using δ15N reveals human and agricultural drivers of seagrass degradation across the British isles. Front. Plant Sci. 9. doi: 10.3389/fpls.2018.00133
Jones B. L., Unsworth R. K. F. (2016). The perilous state of seagrass in the British isles Vol. 3 (London: Royal Society Open Science), 150596. doi: 10.1098/rsos.150596
Julia C., Wissuwa M., Kretzschmar T., Jeong K., Rose T. (2016). Phosphorus uptake, partitioning and redistribution during grain filling in rice. Ann. Bot. 118 (6), 1151–1162. doi: 10.1093/aob/mcw164
Kim S.H., Kim J.W., Kim Y.K., Lee K.-S. (2018) Growth responses of the intertidal seagrass Zosterajaponica to manipulated sea level rise conditions. Bull Mar Sci. 94 (4), 1379–1393. doi: 10.5343/bms.2017.1139
Kjønaas O. J., Wright R. F. (2007). Use of 15N-labelled nitrogen deposition to quantify the source of nitrogen in runoff at a coniferous-forested catchment at gårdsjön, Sweden. Environ. pollut. 147, 791–799. doi: 10.1016/j.envpol.2006.06.019
Kraemer G. P., Mazella L. (1999). Nitrogen acquisition, storage, and use by the co-occurring Mediterranean seagrasses cymodocea nodosa and zostera noltii. Mar. Ecol. Prog. Ser. 183, 95–103. doi: 10.3354/meps183095
Lafratta A., Serrano O., Masqué P., Mateo M. A., Fernandes M., Gaylard S., et al. (2019). Seagrass soil archives reveal centennial-scale metal smelter contamination while acting as natural filters. Sci. Tot. Environ. 649, 1381–1392. doi: 10.1016/j.scitotenv.2018.08.400
Lee K.-S., Dunton K. H. (2000). Effects of nitrogen enrichement on biomass allocation, growth, and leaf morphology of the seagrass thalassia testudinum. Mar. Ecol. Prog. Ser. 196, 39–48. doi: 10.3354/meps196039
Lee S. Y., Kim J. B., Lee S. M. (2006). Temporal dynamics of subtidal zostera marina and intertidal zostera japonica on the southern coast of Korea. Mar. Ecol. 27, 133–144. doi: 10.1111/j.1439-0485.2006.00089.x
Lee K.-S., Park S. R., Kim Y. K. (2007). Effects of irradiance, temperature, and nutrients on growth dynamics of seagrasses: a review. J. Exp. Mar. Biol. Ecol. 350, 144–175. doi: 10.1016/j.jembe.2007.06.016
Macko S. A., Ostrom N. E. (1994). “Pollution studies using stable isotopes,” in Stable isotopes in ecology and environmental science. Eds. Lajtha K., Michener R. H. (Oxford: Blackwell Scientific Publications), 45–62.
McClelland J. W., Valiela I. (1998). Linking nitrogen in estuarine producers to land-derived sources. Limnol. Oceanogr. 43, 577–585. doi: 10.4319/lo.1998.43.4.0577
McClelland J. W., Valiela I., Michener R. H. (1997). Nitrogen-stable isotope signatures in estuarine food webs: A record of increasing urbanization in coastal watersheds. Limnol. Oceanogr. 42, 930–937. doi: 10.4319/lo.1997.42.5.0930
McHenry J., Rassweiller A., Hernan G., Uejio C. K., Pau S., Dubel A. K., et al. (2021). Modelling the biodiversity enhancement value of seagrass beds. Divers. Distrib. 27 (11), 2036–2049. doi: 10.11117/ddi.13379
McMahon K., Nash S. B., Eaglesham G., Müller J. F., Duke N. C., Winderlich S. (2005). Herbicide contamination and the potential impact to seagrass meadows in hervey bay, Queensland, Australia. Mar. pollut. Bull. 51 (1-4), 325–334. doi: 10.1016/j.marpolbul.2004.10.045
Morrison M. A., Lowe M. L., Grant C., Smith P. J., Carbines G., Reed J., et al. (2014). “Seagrass meadows as biodiversity and productivity hotspots,” in New Zealand aquatic environment and biodiversity report no 137. (Wwllington, New Zealand: Ministry for Primary Industries)
Negri A. P., Flores F., Mercurio P., Mueller J. F., Collier C. J. (2015). Lethal and sub-lethal chronic effects of the herbicide diuron on seagrass. Aquat. Toxicol. 165, 73–83. doi: 10.1016/j.aquatox.2015.05.007
Nordlund L. M., Koch E. W., Barbier E. B., Creed J. C. (2016). Seagrass ecosystem services and their variability across genera and geographical regions. PloS One 11 (10), e0163091. doi: 10.1371/journal.pone.0163091
Ntalos G., Sideras A. (2014). The usage of posidonia oceanica as raw material for wood composite and thermal energy production. Mater. Methods Technol. 8, 605–611.
Orth R. J., Lefcheck J. S., McGlathery K. S., Aoki L., Luckenbach M. W., Moore K. A., et al. (2020). Restoration of seagrass habitat leads to rapid recovery of coastal ecosystem services. Sci. Adv. 6, 41. doi: 10.1126/sciadv.abc6434
Parente A., Serio F., Montesano F. F., Mininni C., Santamaria P. (2014). The compost of posidonia oceanica residues: A short review on a new component for soiless growing media. Acta Hortic. 1034, 291–298. doi: 10.17660/ActaHortic.2014.1034.36
Pergent G., Boudouresque C.-F., Dumay O., Pergent-Martini C., Wyllie-Echeverria S. (2008). Competition between the invasive macrophyte caulerpa taxifolia and the seagrass posidonia oceanica: Contrasting strategies. BMC Ecol. 8 (20), 1–13. doi: 10.1186/1472-6785-8-20
Plis A., Lasek J. A., Zuwała J., Yu C.-C., Iluk A. (2016). Combustion performance evaluation of posidonia oceanica using TGA and bubbling fluidized-bed combustor (batch reactor). J. Sustain. Min. 15 (4), 181–190. doi: 10.1016/j.jsm.2017.03.006
Plus M., Auby I., Verlaque M., Levavaseur G. (2005). Seasonal variations in photosynthetic irradiance response curves of macrophytes from a Mediterranean lagoon. Aquat. Bot. 81, 157–173. doi: 10.1016/j.aquabot.2004.10.004
Plus M., Chapelle A., Ménesguen A., Deslous-Paoli J.-M., Auby I. (2003). Modelling seasonal dynamics of biomasses and nitrogen contents in a seagrass meadow (Zostera noltii hornem.): Application to the thau lagoon (French Mediterranean coast). Ecol. Model. 161, 213–238. doi: 10.1016/S0304-3800(02)00350-2
Plus M., Deslous-Paoli J.-M., Dagault F. (2001). Factors influencing primary production of seagrass beds (Zostera noltii hornem.) in the thau lagoon (French Mediterranean coast). J. Exp. Mar. Biol. Ecol. 259, 63–84. doi: 10.1016/S0022-0981(01)00223-4
Rajaona A., Rakotoson T., Wright H., Ramarolahy J., Senthilkumar K., Saito K., et al. (2017). “Responses of grain yield and p uptake to water management and phosphorus in lowland irrigated rice (Oryza sativa l.),” in Future agriculture: Social-ecological transitions and bio-cultural shifts (Germany: Tropentag), 20–22.
Rammou E., Mitani A., Ntalos G., Koutsianitis D., Taghiyari H. R., Papadopoulos A. N. (2021). The potential use of seaweed (Posidonia oceanica) as an lternative lignocellulosic raw material for wood composites manufacture. Coatings 11 (1), 2079–6412. doi: 10.3390/coatings11010069
Ricart A. M., York P. H., Bryant C. V., Rasheed M. A., Ierodiaconou D., Macreadie P. I. (2020). High variability of blue carbon storage in seagrass meadows at the estuary scale. Sci. Rep. 10 (1), 5865. doi: 10.1038/s41598-020-62639-y
Román M., Emilio F., Zamborain-Mason J., Martínez G. (2019). Anthropogenic impact on zostera noltei seagrass meadows (NW Iberian peninsula) assessed by carbon and nitrogen stable isotopic signatures. Estuaries Coast. 42, 987–1000. doi: 10.1007/s12237-019-00549-7
Romero J., Martínez-Crego B., Alcoverro T., Pérez M. (2007). A multivariate index based on the seagrass posidonia oceanica (POMI) to assess ecological status of coastal waters under the water framework directive (WFD). Mar. pollut. Bull. 55, 196–204. doi: 10.1016/j.marpolbul.2006.08.032
Ruesink J. L., Hong J.-S., Wisehart L., Hacker S. D., Dumbauld B. R., Hessing-Lewis M., et al. (2009). Congener comparison of native (Zostera marina) and introduced (Z. japonica) eelgrass at multiple scales within a pacific Northwest estuary. Biol. Invasions. 12, 1773–1789. doi: 10.1007/s10530-009-9588-z
Samper-Villarreal J., Bolañosa R. C., Heidemeyer M., Vargas M. M., Vargas R. M. (2020). Characterization of seagrasses at two new locations in the Eastern tropical pacific (El jobo and matapalito, Costa Rica). Aquat. Bot. 165, 103237. doi: 10.1016/j.aquabot.2020.103237
Samper-Villarreal J., Cortés J. (2020). Seagrass characterization on the southern pacific coast of Costa Rica: history, vegetation, and environment. Bot. Mar. 63 (5), 429–438. doi: 10.1515/bot-2020-0022
Samper-Villarreal J., Moya-Ramírez J., Cortés J. (2022). First characterization of seagrasses at Samara bay, pacific coast of Costa Rica. Aquat. Bot. 178, 103486. doi: 10.1016/j.aquabot.2021.103486
Samper-Villarreal J., Rojas-Ortega G., Vega-Alpízar J. L., Cortés J. (2018). New sighting of seagrasses in the Eastern tropical pacific (Bahía potrero, Costa Rica). Aquat. Bot. 151, 25–29. doi: 10.1016/j.aquabot.2018.07.010
de los Santos C. B., Krause-Jensen D., Alcoverro T., Marbà N., Duarte C. M., van Katwijk M. M., et al. (2019). Recent trend reversal for declining European seagrass meadows. Nat. Commun. 10 (1), 3356. doi: 10.1038/s41467-019-11340-4
de los Santos C. B., Scott A., Arias-Ortiz A., Jones B., Kennedy H., Mazarrasa I., et al. (2020). “Out of the blue - the value of seagrasses to the environment and to people,” in Seagrass ecosystem services: assessment and scale of benefits. Eds. Potouroglou M., Grimsditch G., Weatherdon L., Lutz S., (Nairobi: UNEP) 94.
Scrosati R. A. (2000). The interspecific biomass–density relationship for terrestrial plants: where do clonal red seaweeds stand and why? Ecol. Lett. 3, 191–197. doi: 10.1046/j.1461-0248.2000.00133.x
Sintes T., Duarte C. M., Kendrick G. A. (2005). Non-linear processes in seagrass colonisation explained by simple clonal growth rules. Oikos 108 (1), 165–175. doi: 10.1111/j.0030-1299.2005.13331.x
Surugiu V., Adrian T., Ilie S., Quijón P. A. (2021). A hotspot in the Romanian black Sea: Eelgrass beds drive local biodiversity in surrounding bare sediments. Front. Mar. Sci. 8 doi: 10.3389/fmars.2021.745137
Touchette B. W., Burkholder J. M. (2000). Review of nitrogen and phosphorus metabolism in seagrasses. J. Exp. Mar. Biol. Ecol. 250 (1-2), 133–167. doi: 10.1016/s0022-0981(00)00195-7
Vieira V.M.N.C.S., Engelen A. H., Huanel O. R., Guillemin M.-L. (2016). Linear-in-the-parameters oblique least squares: a case study with the estimation of density-dependent survival in algae with isomorphic biphasic life-cycles. PloS One 11 (12), e0167418. doi: 10.1371/journal.pone.0167418
Vieira V. M., Leitão F., Mateus M. (2015). Biomass–density data analysis: a comment on cabaço et al., (2013). J. Ecol. 103, 537–540. doi: 10.1111/1365-2745.12294
Vieira V.M.N.C.S., Lopes I. E., Creed J. C. (2018). The biomass–density relationship in seagrasses and its use as an ecological indicator. BMC Ecol. 18, 44. doi: 10.1186/s12898-018-0200-1
Vieira V.M.N.C.S., Lopes I. E., Creed J. C. (2019). A model for the biomass-density dynamics of seagrasses developed and calibrated on global data. BMC Ecol. 19 (1), 4. doi: 10.1186/s12898-019-0221-4
Waycott M., Longstaff B. J., Mellors J. (2005). Seagrass population dynamics and water quality in the great barrier reef region: a review and future research directions. Mar. pollut. Bull. 51 (1-4), 343–350. doi: 10.1016/j.marpolbul.2005.01.017
Weller D. E. (1989). The interspecific size-density relationship among crowded plant stands and its implications for the -3/2 power rule of self-thinning. Am. Nat. 133, 20–41. doi: 10.1086/284899
White J., Harper J. L. (1970). Correlated change in plant size and number in plant populations. J. Ecol. 58, 467–485. doi: 10.2307/2258284
Keywords: seagrass, water quality, indicator, coastal, eutrophication, monitoring, mitigation, valuation
Citation: Vieira VMNCS, Lobo-Arteaga J, Santos R, Leitão-Silva D, Veronez A, Neves JM, Nogueira M, Creed JC, Bertelli CM, Samper-Villarreal J and Pettersen MRS (2022) Seagrasses benefit from mild anthropogenic nutrient additions. Front. Mar. Sci. 9:960249. doi: 10.3389/fmars.2022.960249
Received: 02 June 2022; Accepted: 18 October 2022;
Published: 23 November 2022.
Edited by:
Elisabetta Manea, National Research Council (CNR), ItalyReviewed by:
Loubna Boutahar, Sevilla University (Spain) and Mohammed V University (Morocco), SpainJoanne Irene Ellis, University of Waikato, New Zealand
Copyright © 2022 Vieira, Lobo-Arteaga, Santos, Leitão-Silva, Veronez, Neves, Nogueira, Creed, Bertelli, Samper-Villarreal and Pettersen. This is an open-access article distributed under the terms of the Creative Commons Attribution License (CC BY). The use, distribution or reproduction in other forums is permitted, provided the original author(s) and the copyright owner(s) are credited and that the original publication in this journal is cited, in accordance with accepted academic practice. No use, distribution or reproduction is permitted which does not comply with these terms.
*Correspondence: Vasco M. N. C. S. Vieira, dmFzY28udmllaXJhQHRlY25pY28udWxpc2JvYS5wdA==