- 1School of Life Science and Institute of Wetland Ecology, Nanjing University, Nanjing, China
- 2Nanjing University Ecology Research Institute of Changshu, Changshu, China
- 3College of Life Sciences, Shaanxi Normal University, Xi’an, China
- 4Yancheng Wetland Natural Reserve for Rare Birds, Yancheng, China
The effects of coastal embankments on nitrogen (N) cycling in the Spartina alterniflora salt marsh have been extensively reported. However, it remains unclear effects of the embankment on the sizes of diverse N subpools in the plant-soil subsystems year-round. This study examined seasonal changes in various N subpools of plant subsystems; soil subsystems [e.g., soil organic N (SON), recalcitrant organic N (RON), labile organic N (LON), dissolved organic N (DON), ammonium N(NH+4 N), and nitrate N(NO3-N)]; N mineralization [e.g., soil net ammonification (RA) and nitrification (RN) rate]; and immobilization [e.g., microbial biomass N (MBN)] in embanked and adjacent S. alterniflora natural salt marshes on the coast of Eastern China. The embankment significantly reduced the litter N storage by 62.7–71.8% over the four seasons and decreased the root N storage by 53.0% during winter. The SON, LON, RON, and N H +4 N concentrations declined significantly by 43.0–60.2%, 35.8–64.8%, 44.9–59.0%, and 20.8–42.2%, respectively, over the four seasons following the embankment construction. Furthermore, the embankment dramatically reduced the DON concentrations by 21.9% in spring, 14.6% in summer, and 10.4% in winter, while notably diminishing the NO3 N concentrations by 33.4% in autumn and 44.9% in winter, and the RA and RN in spring and summer. However, the embankment clearly increased the MBN concentrations during summer and autumn, the NO3 N concentrations in spring, and the RA and RN in winter at different levels. Due to the decreased soil N inputs from plants, the embankment decreased the organic and inorganic N subpools every season to varying degrees, except for the NO3 N concentration in spring. We suggest that the decreased soil salinity following embankment establishment might increase the uptake of ions by microbes, while stimulating the production of MBN. Ultimately, the NO3 N and DON were two vital N sources for S. alterniflora, and plants absorbed N from the soil to promote their biomass, as well as N concentration and storage. This study is conducive toward understanding the mechanisms behind the effects of coastal embankments on the N transfer among various N subpools in the plant and soil systems.
Introduction
Coastal embankments function to hold seawater back from salt marshes. Many countries spanning Europe, North America, and East Asia have established coastal embankments to promote regional economic development (Iost et al., 2007; Kirwan and Megonigal, 2013), satisfy local demands for farming and housing (Barbier et al., 2011), and control invasive plants (An et al., 2007). More than 60% of coastline is enclosed by embankments in China (Ma et al., 2014), which has led to significant alterations in local biogeochemical cycles (e.g., nitrogen (N) cycling) (Yang et al., 2016; van de Broek et al., 2019; Zhou and Bi, 2020; Feng et al., 2022). Biogeochemical cycling is complex and can be influenced by myriad factors (Purvaja et al., 2008; Aufdenkampe et al., 2011; Lin and Lin, 2022). Although the impacts of coastal embankments on biogeochemical cycling have been extensively reported (Yang et al., 2016; van de Broek et al., 2019; Feng et al., 2022), few investigation has explored the seasonal dynamics of N pools following the establishment of embankments in salt marshes.
Seasonality may directly affect N cycling via soil moisture and temperature, or indirectly through modified plant productivity and the seasonal patterns of organic matter release (Wuest, 2015; Zhao et al., 2016; Evangelou et al., 2021). For instance, the accumulation of soil organic N is generally positively related to seasonal variations in soil moisture, which influences soil oxygen levels that affect the rate at which debris is decomposed by microorganisms (Huang et al., 2013; Zhao et al., 2016). However, higher salinity levels can inhibit the activities of soil microorganisms during N cycling (Gao et al., 2012; Zhao et al., 2016). Moreover, seasonal SON in coastal ecosystems has positive correlations with the quality and quantity of plant residues (e.g., plant litter biomass), which exhibit seasonal variations (Ake-Castillo et al., 2006; Elsey-Quirk et al., 2011). Generally, the decomposition rates of plant residues are positively correlated with the average annual temperature, precipitation, etc. (Ake-Castillo et al., 2006; Battle and Golladay, 2007; Abu Hena et al., 2015), which also ultimately impact the seasonal SON. Several earlier studies also have demonstrated that higher net N mineralization rates in the soil and the N uptake of plants always occur in warmer environments, which has combined effects on the magnitudes of soil inorganic N pools (Augustine et al., 2014; Hu et al., 2015; Sanders-DeMott et al., 2018). Similarly, soil-residing inorganic N pools can be affected by the minimal activities of plants and microorganisms during autumn and/or winter (Urakawa et al., 2014; Sanders-DeMott et al., 2018). Soil microbial biomass N (MBN) comprises the mass of the living components of soil organic N, wherein soil microorganisms absorb both dissolved organic N (DON) and inorganic N (e.g., ammonium and nitrate ) and transform them to MBN (Wang et al., 2019; Evangelou et al., 2021). As recently reported that the highest concentrations of MBN are observed during the warmer season (e.g., spring and/or autumn) in coastal ecosystems (Liu et al., 2019; Chen et al., 2022). Therefore, the seasonality has important effects on the plant-soil N dynamics in temperate coastal regions (Urakawa et al., 2014; Sanders-DeMott et al., 2018; Wang et al., 2018) through dynamic N fixation (Dovrat and Sheffer, 2019), plant uptake (Socci and Templer, 2011), and N mineralization (Hu et al., 2015; Huang et al., 2021); and determining effects of coastal embankments on various N pools in different seasons may improve our understanding of how these embankments impact N pools or N cycling in salt marshes.
Jiangsu Province is home to the largest coastal wetland area (4570 km2) and longest stretches of coastal embankments (954 km) in China (Zhang et al., 2006; Zhang et al., 2013; Cui et al., 2018). The exotic Spartina alterniflora Loisel (S. alterniflora) is the dominant plant along Chinese coasts, which was introduced from North America in 1979 (Xu and Zhuo, 1985; An et al., 2007) and over the last few decades has expanded rapidly across salt marshes (An et al., 2007; Wan et al., 2009). A growing number of studies have focused on the ecological management of S. alterniflora (An et al., 2007; Patten et al., 2017), with coastal embankments being one of the more common approaches at present (An et al., 2007; Ma et al., 2014).
Our previous studies revealed that embankments significantly decreased the biomass and N storage capacities of plants, soil N pools, and soil microbial diversity, and altered the compositions of soil microbial communities in S. alterniflora salt marshes during the growing season (Yang et al., 2016; Feng et al., 2017; Feng et al., 2022). However, to the best of our knowledge, there exists very limited knowledge regarding the seasonal dynamics of N pools in natural salt marshes and those with embankments. Furthermore, seasonal variations in salt marshes following the development of coastal embankments should not be disregarded, as they may have additional deleterious effects on soil N dynamics.
Consequently, we hypothesized that embankments may reduce variant N subpools in the soil and plant subsystems in S. alterniflora salt marshes year-round. For this study, to verify our hypothesis, we compared various soil N subpools, plant N subpools, microorganismal growth (e.g., microbial biomass carbon (MBC) and MBN), soil N transformation rates (e.g., N immobilization and mineralization), and physiochemical soil properties in both embanked and adjoining natural S. alterniflora-dominated salt marshes in April, July, October 2017, and January 2018. The primary objectives of this study were to quantify and identify the (1) effects of the embankment on the sizes of various N subpools in the S. alterniflora salt marsh in four seasons, and (2) how the embankment influenced the N transfer among various N subpools in plant and soil subsystems in plant and soil subsystems. Ultimately, this study sought to provide a theoretical basis for the control and rational utilization of S. alterniflora, particularly in the coastal ecosystem where the primary productivity is conventionally thought to be limited by N (Vitousek et al., 1997).
Materials and methods
Site description
The field work for this study was conducted in the core region of the Dafeng Milu National Nature Reserve (32°59′N-33°03′N, 120°47′E-120°53′E), on the Yellow Sea coast of Jiangsu Province, China (Figure 1). This Reserve was established in 1986 and is listed as part of the World Natural Heritage in 2019, as it plays a significant role in international biodiversity conservation. It is situated such that it encompasses the transition of warm temperate and northern subtropical zones, which are primarily impacted by oceanic and continental climates (Cui et al., 2018). According to the report of China Meteorological Administration, the mean monthly temperatures were 13.1°C in April, 26.7°C in July, 16.5°C in October, and 1.6°C in January; the mean monthly precipitations were 55.2 mm in April, 251.3 mm in July, 54.1 mm in October, and 33.9 mm in January; the radiations per unit area were 135 kw‧m-2 in April, 150 kw‧m-2 in July, 98 kw‧m-2 in October, and 70 kw‧m-2 in January. A regular semidiurnal tide occurs along the coastal area, with an annual average tidal range of about 3 m (Ren, 1986; Li et al., 2018).
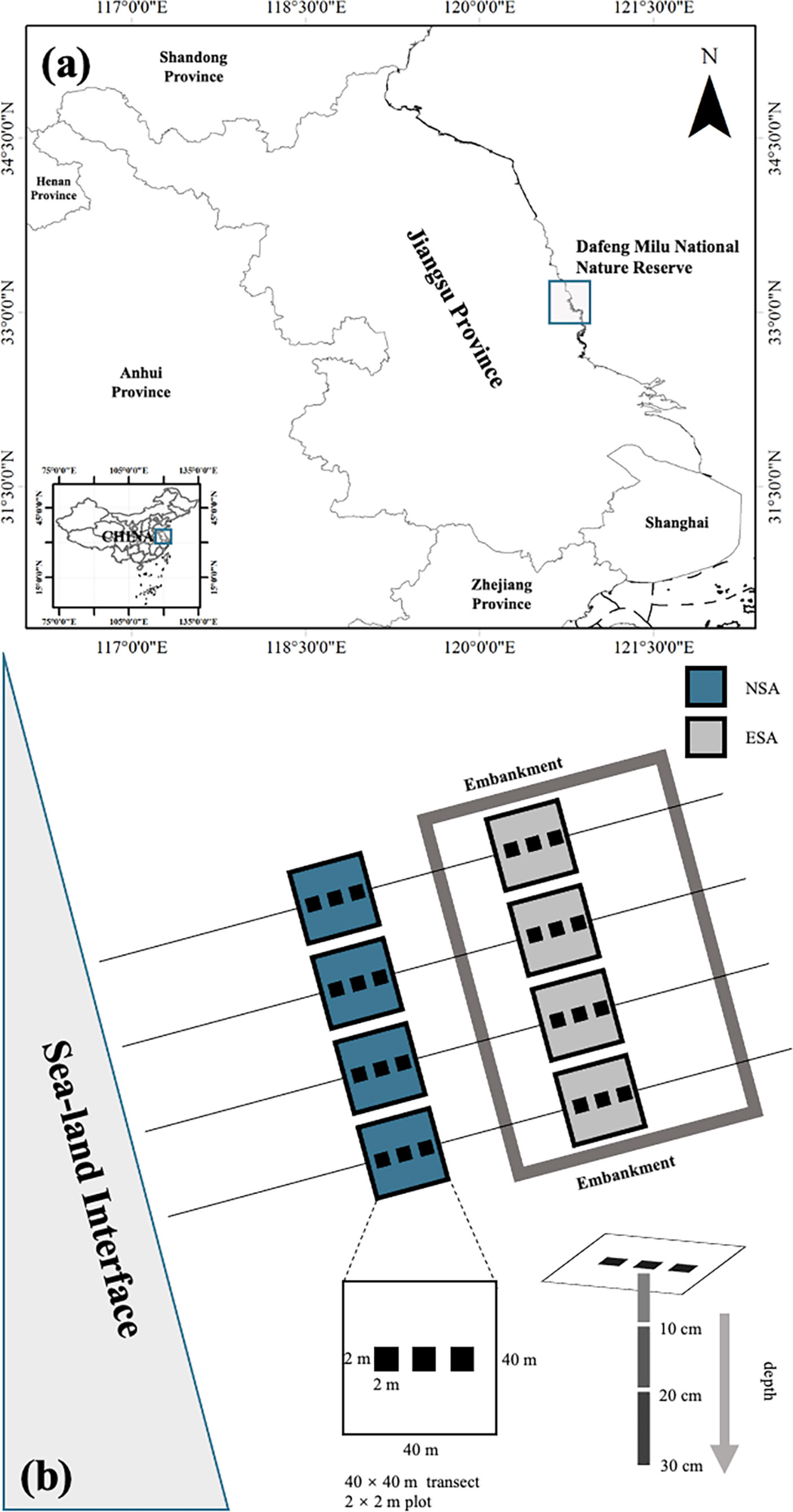
Figure 1 (A) Location of the study site and (B) schematic diagram of the study area in Dafeng Milu National Nature Reserve, Yancheng, Jiangsu Province, China. NSA, natural Spartina alterniflora salt marsh; ESA, embanked S. alterniflora salt marsh.
The coastal embankments of this region were established in 2011, with a typical length, thickness, and height of 2400 m, 2 m, and 2 m, respectively. Thus, both seawater and river water are prevented from entering the embanked range. The natural and embanked ranges have not been subjected to anthropogenic activities or other disturbances (Yang et al., 2016).
Field sample collection
In spring (April 2017), summer (July 2017), autumn (October 2017), and winter (January 2018), four parallel transects (40 × 40 m) were established in the natural and embanked S. alterniflora salt marshes (Figure 1), and three plots (2 × 2 m) in each transect were randomly selected. Three soil core samples (0-10 cm, 10-20 cm, and 20-30 cm depths) were randomly extracted from each plot, whereafter core samples from the same depths and plots were evenly mixed as the final soil samples.
Briefly, 96 soil samples (four replicates × three depths × two treatments × four seasons) were obtained. Following the removal of all belowground plant components, the soil samples were transferred to the laboratory and divided into three subsamples. The first subsample was employed to measure the soil moisture, whereas the second was air-dried and sifted through a 1-mm sieve, which was used to determine the soil pH, salinity, SOC, and SON. The third fresh subsample was sifted through a 2-mm sieve and stored at 4°C, which was used to quantify the DON, MBC, MBN, and concentrations.
The soil net ammonification (RA) and nitrification (RN) rate were quantified using the closed-top PE (polyethylene) tube incubation technique, which was based on the sequential soil coring of the undisturbed soil and in situ exposure (Raison et al., 1987). Eight PE pipes (Ø5 cm x 30 cm long) per sample were vertically inserted into the soil at random, after which they were carefully extracted. Four PE pipes were transferred to the laboratory for the quantification of the and concentrations. The other four were buried in situ for cultivation to determine the and concentrations after 30 days, where their tops and bottoms were wrapped with plastic film and gauze, respectively. Furthermore, four quadrats (50 × 50 cm) were established in each transect for the acquisition of plant tissues, including leaves, stems, litter, and roots.
Laboratory analyses
The leaves, stems, and litter were separated, whereas the roots were collected by sifting through a 100-mm sieve, which was used to remove any clods and stones. All S. alterniflora tissues were thoroughly rinsed under running water (Yang et al., 2016). Each type of tissue biomass was oven-dried at 65°C, and the N concentration was determined using an Elementar Vario Micro CHNS analyzer (Elementar Analysen system GmbH, Germany). The tissue N storage for each plant was computed using the following formula:
where SN is the plant N storage, CN is the plant N concentration, and B is the plant biomass per centiare.
The first fresh soil subsample was used to determine the soil bulk density (BD), pH, salinity, and moisture according to methods that reported by Slavich and Petterson (1990) and Yang et al. (2016).
The recalcitrant organic N (RON) was processed using the acid hydrolysis method (Rovira and Vallejo, 2002; Yang et al., 2016). The concentrations of SON and RON were measured using an Elementar Vario Micro CHNS analyser (Elementar Analysen system GmbH, Germany), whereas the labile organic N (LON) was computed using the following formula.
Additionally, DON was determined following the procedure of Jones et al. (2004). The MBC and MBN concentrations were determined following the chloroform fumigation-extraction method (Brookes et al., 1985).
A mixture of 2.5 g of fresh soil samples and 25 mL of 2 M KCl (1:10 w/v soil:solution) were placed into 50 mL PP (polypropylene) bottles and shaken at 200 rpm for 60 min using a reciprocating shaker. Subsequently, the supernatant was employed to quantify the concentrations of and with an AQ2+ Automated Discrete Analyser (SEAL, Britain, shown after the determination of RA and RN:
Where ti and ti+1are the incubation start and end times (d), respectively; and are the soil concentrations (mg/kg) at the onset and conclusion of incubation, respectively; NO2-Ni and NO2-Ni+1 are the soil NO2-N concentrations (mg/kg) at the start and end of incubation, respectively; and are the soil concentrations (mg/kg) at the beginning and end of incubation, respectively.
Statistical analyses
We employed one-way analysis of variance (ANOVA) to (1) verify the impacts of the embankment on the concentrations of SON, DON, LON, RON, , , MBC, MBN, RA, and RN, as well as the biomass of aboveground living (ALB), litter (LB), and root (RB) tissues and N storage of aboveground living (ALNS), litter (LNS), and root (RNS) tissues for the different seasons; (2) confirmed the seasonal variations in these indices for the natural and embanked salt marshes; (3) seasonal plant and soil N subpools were studied with a NMDS (Non-metric Multi-Dimensional Scaling); (4) interactions of SON with LNS and RNS in the natural and embanked salt marshes were test by using linear models. Besides, Multi-way ANOVA was employed to examine the impacts of embankment and season, as well as their interactive effects on the various N subpools in plant and soil subsystems. All above analyses were performed using R software (R Studio Inc., Massachusetts, USA).
We analyzed the relationships between plant biomass and N storage, various soil N fractions, MBC, MBN, and assorted mineralization rates for both natural and embanked S. alterniflora by using Pearson’s correlation analysis (Figure S1). Furthermore, structural equation modelling (SEM) was utilized to test the multivariate relationships between the direct effects of the coastal embankment and indirect impacts of parameters during the different seasons related to soil organic and inorganic N, MBC, MBN, RA, and RN, which was based on a conceptual a priori model (Figure S2). Using the maximum likelihood for parameter estimation (Boldea and Magnus, 2009), the best fitting model was selected through the sequential removal of nonsignificant coefficients (p < 0.05). We employed a chi-squared test (χ2) and the root-mean-square error of approximation (RMSEA; Schermelleh-Engel et al., 2003) for evaluating the model. The SEM analysis was implemented using AMOS 22.0 software (IBM, SPSS, New York).
Results
Plant biomass and N allocation
The embankment significantly decreased the ALB, LB, ALNS, and LNS of S. alterniflora throughout the year, ranging from 47.9% to 70.3%, 66.5% to 76.4%, 52.7% to 69.5%, and 62.7% to 71.8%, respectively (p < 0.05; Figure 2). Further, they reduced the RB by 57.9% and 67.7% in autumn and winter, respectively, as well as the RNS by 53.0% in winter (p < 0.05; Figure 2). Conversely, the embankment increased the ALNC remarkably by 24.8% in spring and the LNC by 20.2% in autumn, as well as the RNC by 49.7% and 80.8% in summer and autumn, respectively (p < 0.05; Figure 2). Additionally, the maximum values of RB, RNS, and ALNC occurred in spring, and the peaks of AB and ANS appeared in autumn for both the natural and embanked salt marshes (p < 0.05; Figure 2). The values of LNS and LB in spring and winter were remarkably higher than those in summer and autumn for both the natural and embanked salt marshes (p < 0.05). The LNC values in both the natural and embanked salt marshes were the lowest during winter, with the lowest RNC value in the natural salt marsh appearing in autumn (p < 0.05). However, there were no significant differences in the RNC value in the embanked salt marsh across all seasons (p ≥ 0.05, Figure 2).
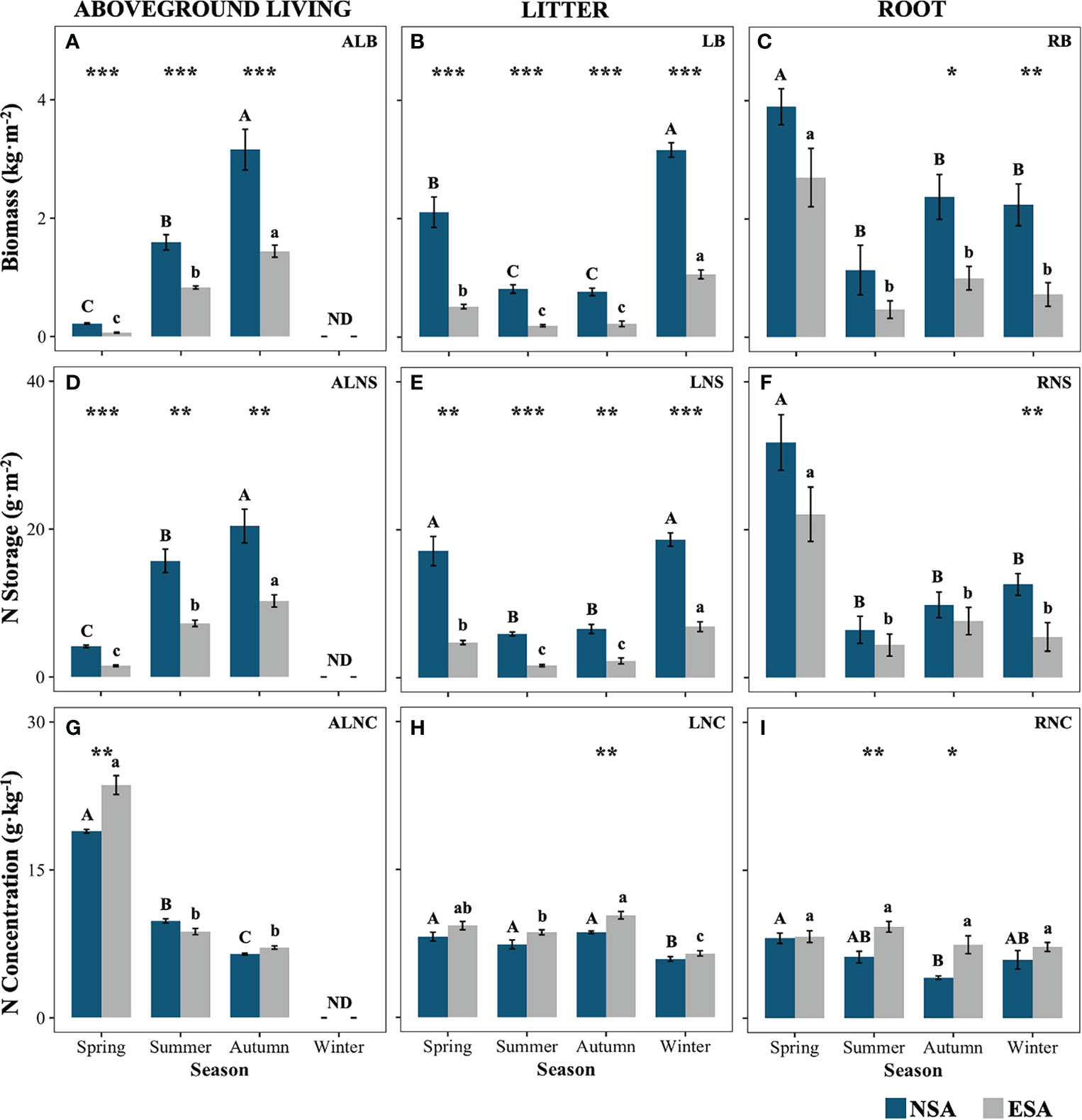
Figure 2 Values of (A–C) biomass, and nitrogen (D–F) storages and (G–I) concentrations of various Spartina alterniflora tissues in natural (NSA) and embanked (ESA) salt marshes in spring, summer, autumn, and winter. ALB, aboveground living biomass; ALNS, aboveground living nitrogen storage; ALNC, aboveground living nitrogen concentration; LB, litter biomass; LNS, litter nitrogen storage; LNC, litter nitrogen concentration; RB, root biomass; RNS, root nitrogen storage; RNC, root nitrogen concentration. The averages and standard errors of four replicates are shown. Bars labelled with different uppercase and lowercase letters denote significant differences (p < 0.05) between seasons in NSA and ESA, respectively. The differences between NSA and ESA were significant at ***p < 0.001, **p < 0.01 and *p < 0.05, and blanks indicate no significance at p ≥ 0.05.
Soil N subpools
In the soil organic N subpool, there were significant decreases in the concentrations of SON, LON, and RON in the embanked salt marsh, which ranged from 43.0% to 60.2%, 35.8% to 64.8%, and 44.9% to 59.0%, respectively, over the four seasons (p < 0.001; Figure 3). In contrast to those in the natural salt marsh, the concentrations of DON in the embanked salt marsh decreased significantly by 21.9%, 14.6%, and 10.4% in spring, summer, and winter, respectively (p < 0.05; Figure 3).
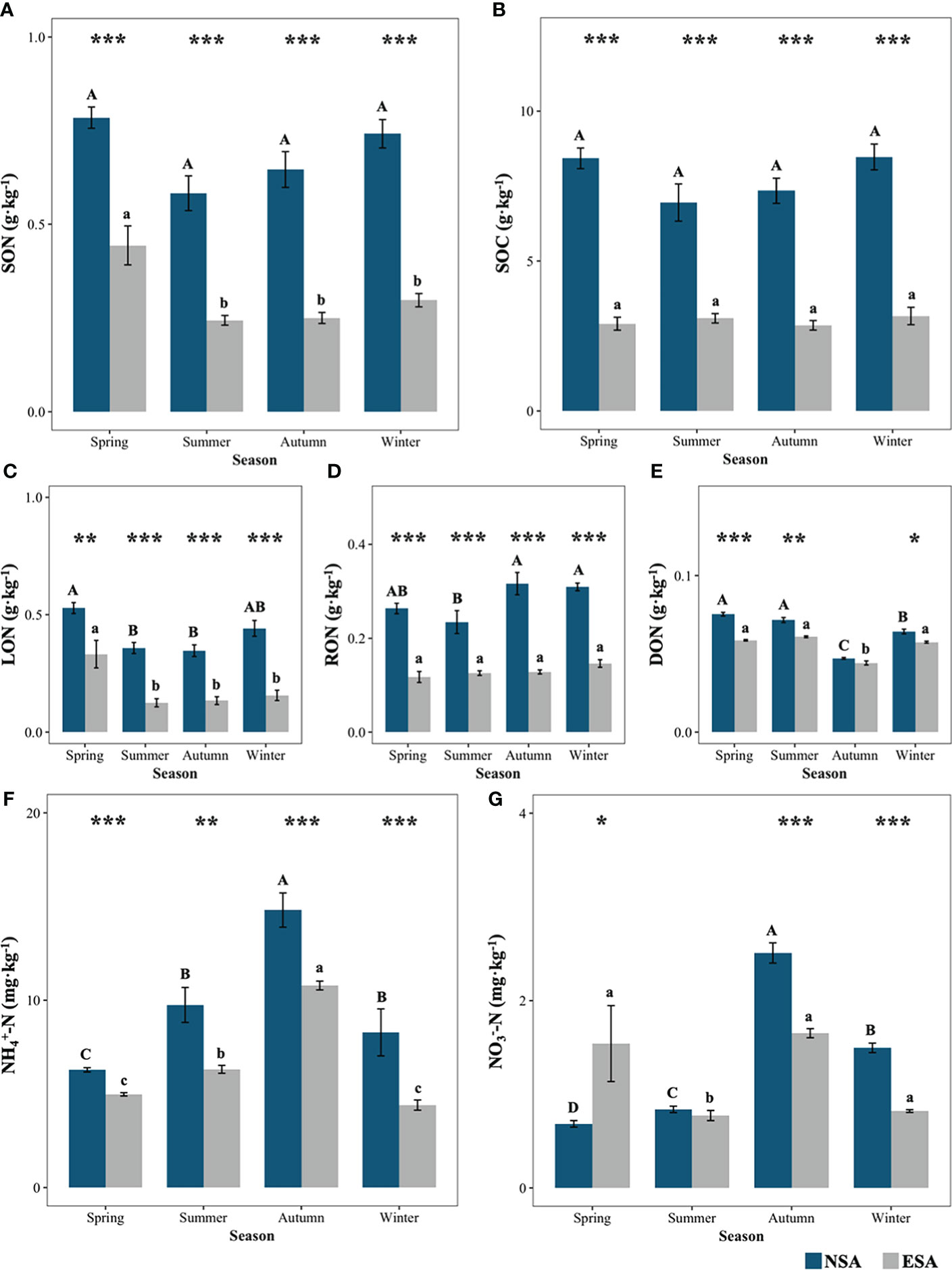
Figure 3 Values of (A) total soil organic nitrogen (SON), (B) total soil organic carbon (SOC), (C) labile organic nitrogen (LON), (D) recalcitrant organic nitrogen (RON), (E) dissolved organic nitrogen (DON), (F) ammonia nitrogen (NH+4 N), and (G) nitrite nitrogen (NO3 N) in natural (NSA) and embanked (ESA) Spartina alterniflora salt marshes in spring, summer, autumn, and winter. The averages and standard errors of four replicates are shown. Bars labelled with different uppercase and lowercase letters denote significant differences (p < 0.05) between seasons in NSA and ESA, respectively. The differences between NSA and ESA were significant at ***p < 0.001, **p < 0.01 and *p < 0.05, and blanks indicate no significance at p ≥ 0.05.
Negligible seasonal variations in the SON concentration values were observed in the natural salt marsh (p ≥ 0.05; Figure 3), with the exception of the highest being observed in spring for the embanked salt marsh (p < 0.05; Figure 3). The maximum LON concentrations were observed in spring for both the natural and embanked salt marshes (p < 0.05), but there is no difference in the LON concentrations between spring and winter in the natural salt marshes (p ≥ 0.05; Figure 3). The minimum concentration of RON was seen in spring for the natural salt marsh (p < 0.05; Figure 3), while there were no significant seasonal changes in the RON concentrations in the embanked salt marsh (p ≥ 0.05; Figure 3). The lowest DON concentrations were observed in autumn for both the natural and embanked salt marshes (Figure 3).
For the soil inorganic N subpool, the effects of the embankment significantly influenced the soil (Table 1). The embankment significantly decreased the soil concentrations (20.8 – 42.2%) through spring, summer, autumn, and winter (p < 0.05; Figure 3). The embankment also reduced the soil concentrations in autumn and winter by 33.4% and 44.9%, respectively, while they significantly increased the soil concentration by 132.6% in spring (p < 0.05; Figure 3). Moreover, the peak concentrations of soil and were observed in autumn for both the natural and embanked salt marshes (Figure 3).
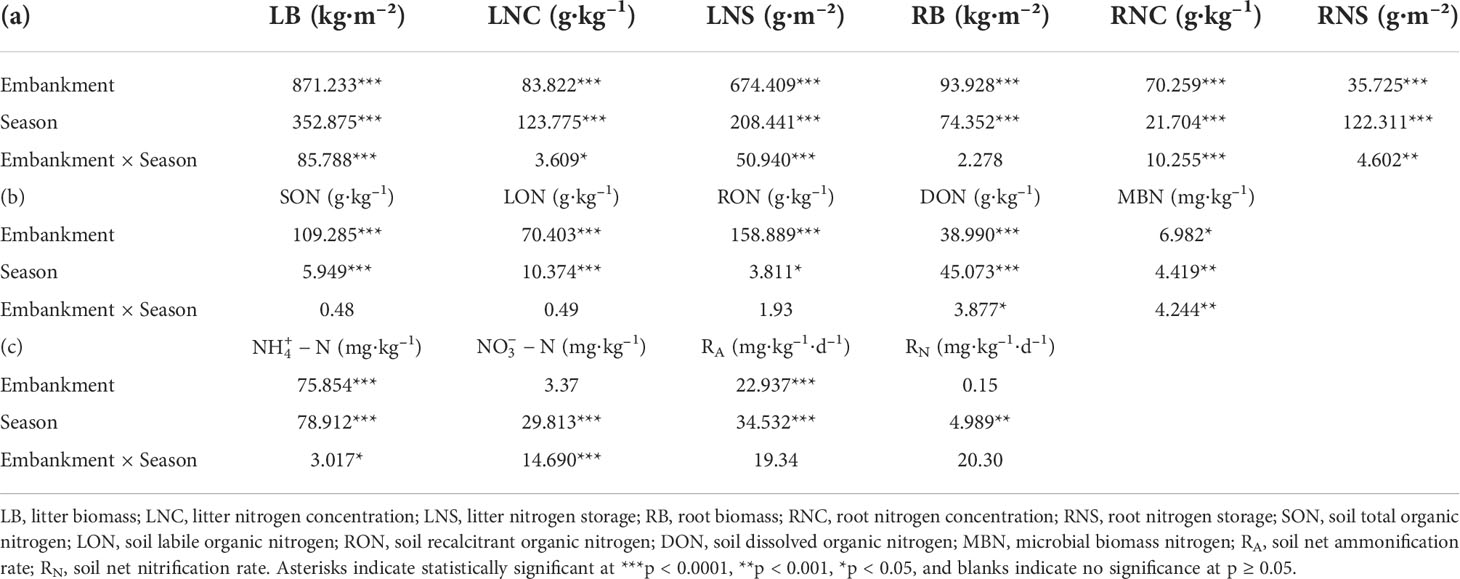
Table 1 Statistical significance of the effects of the embankment, seasons, and their interactions on (a) biomasses, N concentrations and storages of litter and roots, (b) soil organic N subpools, and (c) soil inorganic N subpools, RA and RN according to multi-way ANOVA.
Growth, N immobilization, and mineralization of microorganisms
The soil MBC concentration was significantly reduced by 52.1% in winter with the embankment, whereas on the contrary the soil MBN concentration significantly increased in summer and autumn by 189.2% and 200.8%, respectively (p < 0.05; Figure 4). The peak values of both the soil MBC and MBN concentrations were observed in winter for the natural salt marsh, whereas there were insignificant seasonal variations in the MBC and MBN following the establishment of the embankment (p < 0.05; Figure 4).
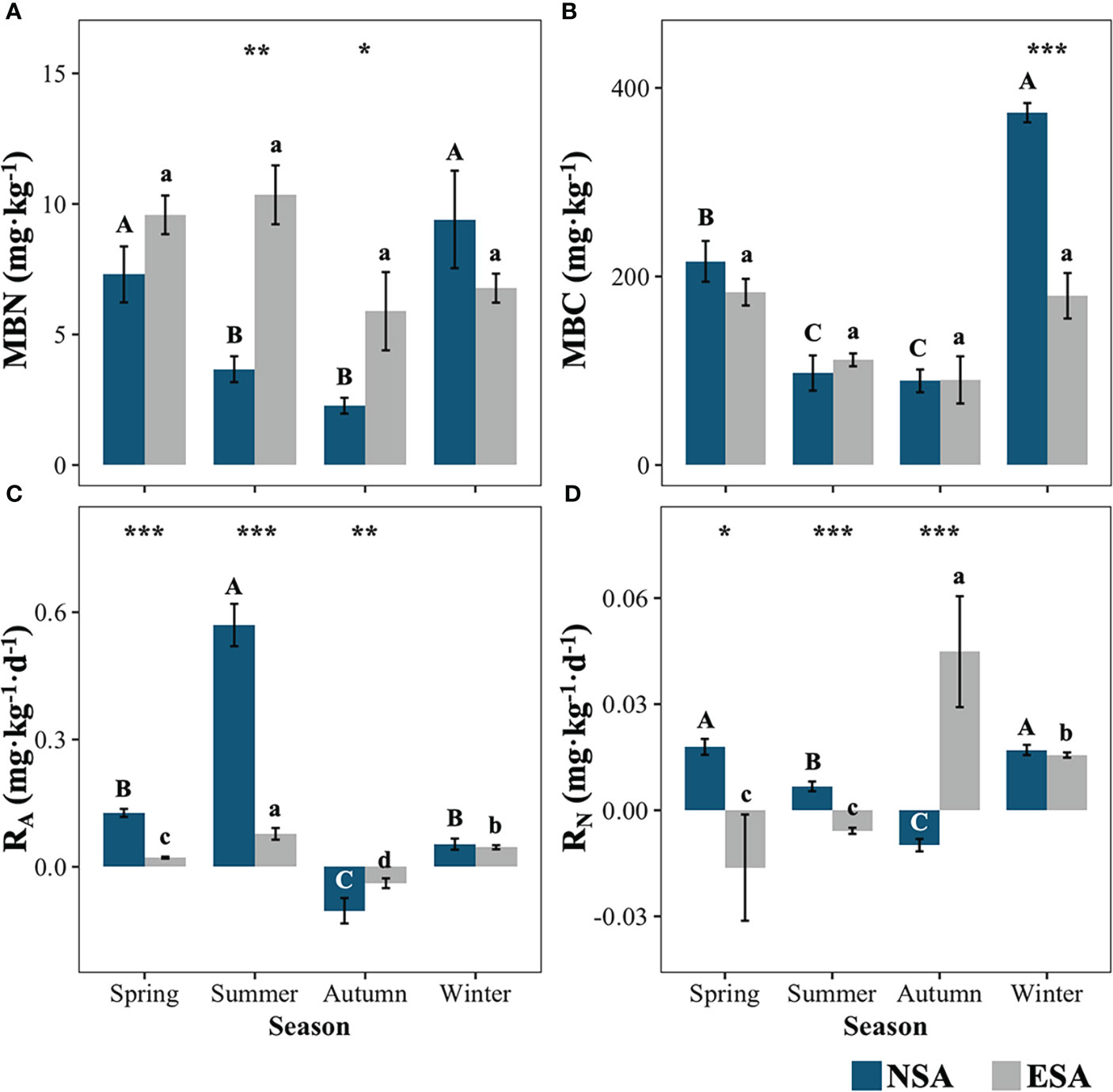
Figure 4 Values of the soil microbial biomass (A) nitrogen (MBN) and (B) carbon (MBC), and net soil (C) ammonification (RA) and (D) nitrification (RN) rate in natural (NSA) and embanked (ESA) Spartina alterniflora salt marshes in spring, summer, autumn, and winter. The averages and standard errors of four replicates are shown. Bars labelled with different uppercase and lowercase letters denote significant differences (p < 0.05) between seasons in NSA and ESA, respectively. The differences between NSA and ESA were significant at ***p < 0.001, **p < 0.01 and *p < 0.05, and blanks indicate no significance at p ≥ 0.05.
Furthermore, both the RA and RN were remarkably reduced in spring and summer, while they were significantly increased in autumn following embankment development (p < 0.01; Figure 4). The lowest RA values (< 0 mg·kg-1·d-1) were observed in autumn, while they peaked in summer for both the natural and embanked salt marshes (Figure 4). The highest and lowest RN values (p < 0.05) were observed in spring and autumn, respectively, in the natural salt marsh, while the highest and lowest RN values were observed in autumn and spring, respectively, in the embanked salt marsh (Figure 4).
Physicochemical soil properties
The soil moisture and salinity values in the embanked salt marsh were significantly lower than those in the natural salt marsh for every season (p < 0.001) (Figure S3). The soil pH and BD values in the embanked salt marsh were significantly higher than those in the natural salt marsh in every season (p < 0.001) (Figure S3). However, no significant seasonal differences in the soil moisture, salinity, pH and BD were found in both the natural and embanked salt marshes (p ≥ 0.05) (Figure S3).
Correlation analysis
The NMDS revealed that the soil N pool compositions in the embanked salt marsh were clustered and distinct from those in the natural salt marsh in every season (Stress < 0.001; Figure 5). Besides, the SON amounts highly correlated with the LNS values in natural salt marsh (p = 0.025; Figure 6), whereas the relationship between the SON amounts and the LNS values in embanked salt marsh was insignificant ((p = 0.1415; Figure 6).
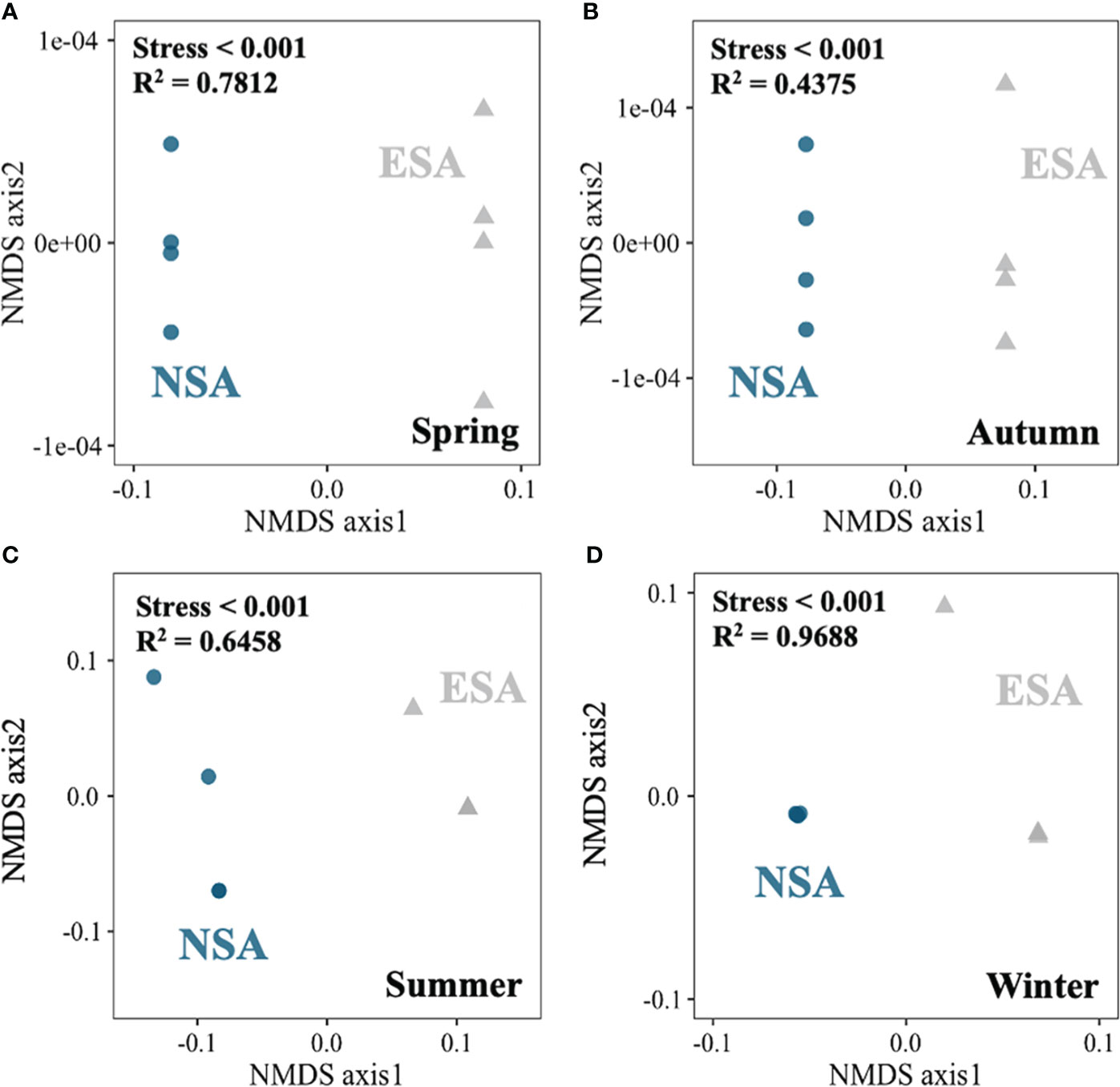
Figure 5 Non-metric multidimensional scaling (NMDS) plot of dissimilarity of the edaphic N pools between the natural (NSA) and embanked (ESA) Spartina alterniflora salt marshes in (A) spring, (B) summer, (C) autumn, and (D) winter.
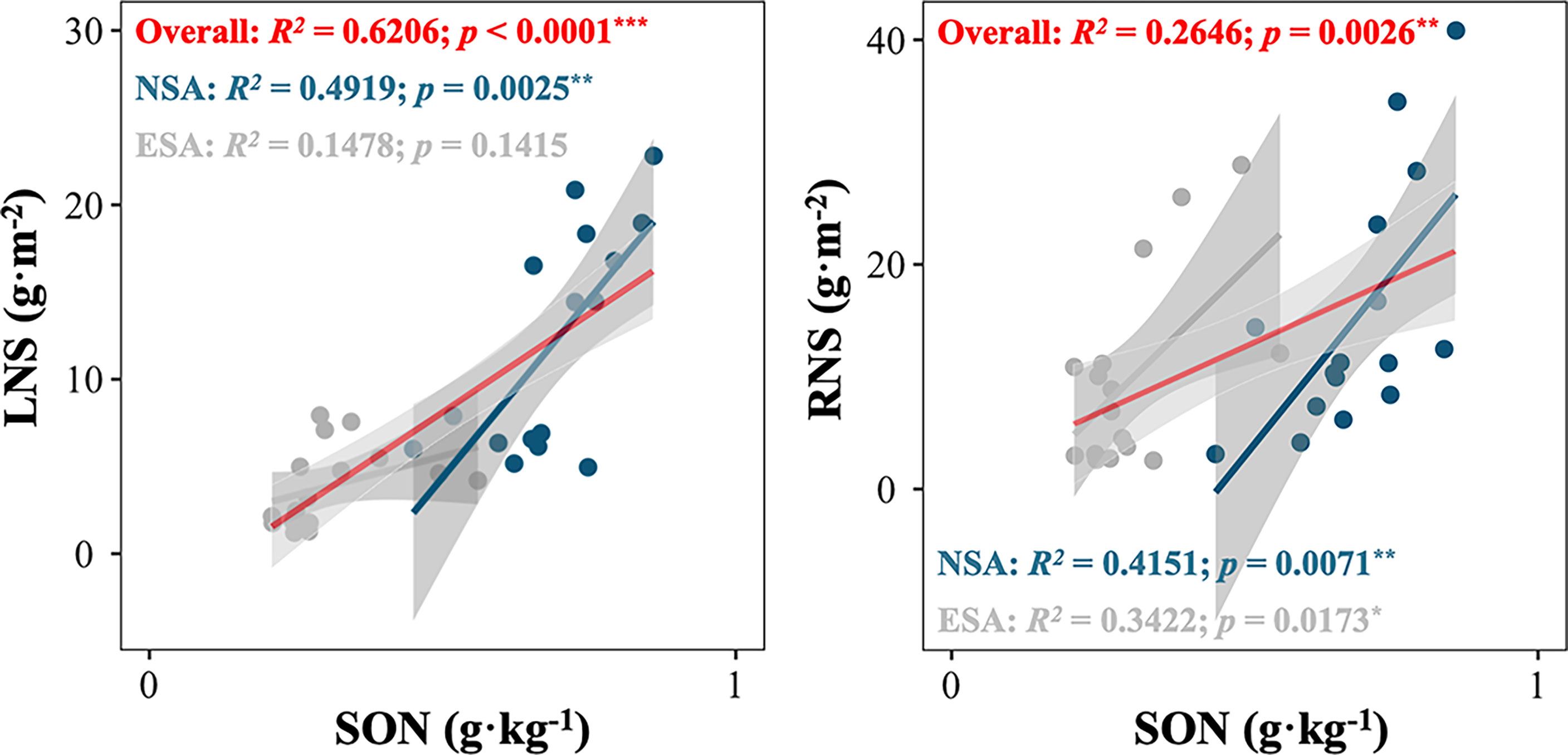
Figure 6 (A) Total soil organic nitrogen (SON) in relation to litter nitrogen storage (LNS) of Spartina alterniflora; (B) total soil organic nitrogen (SON) in relation to root nitrogen storage (RNS) of S. alterniflora in the natural (NSA) and embanked (ESA) salt marshes. Asterisks indicate statistically significant at ***p < 0.0001, **p < 0.001, and blanks indicate no significance at p ≥ 0.05.
The results of SEM analyses are shown in Figure 7. It indicated a highly positive correlation between the N storage of each plant tissue and the biomass of the corresponding tissues in both the natural and embanked salt marshes. A remarkable positive correlation was found between the LON, RON, and LNS amounts in the natural salt marsh; a significantly positive relationship was observed between the DON and LNS amounts in the embanked salt marsh. The DON concentration was significantly and positively associated with the RNS value in the natural salt marsh; the LON concentration was dramatically positive correlation with the RNS value in the embanked salt marsh. The concentrations in both the natural and embanked salt marshes were significantly and negatively associated with the DON concentrations. The concentrations were significantly positively associated with those of , but observably negative with the RNC values in both the natural and embanked salt marshes.
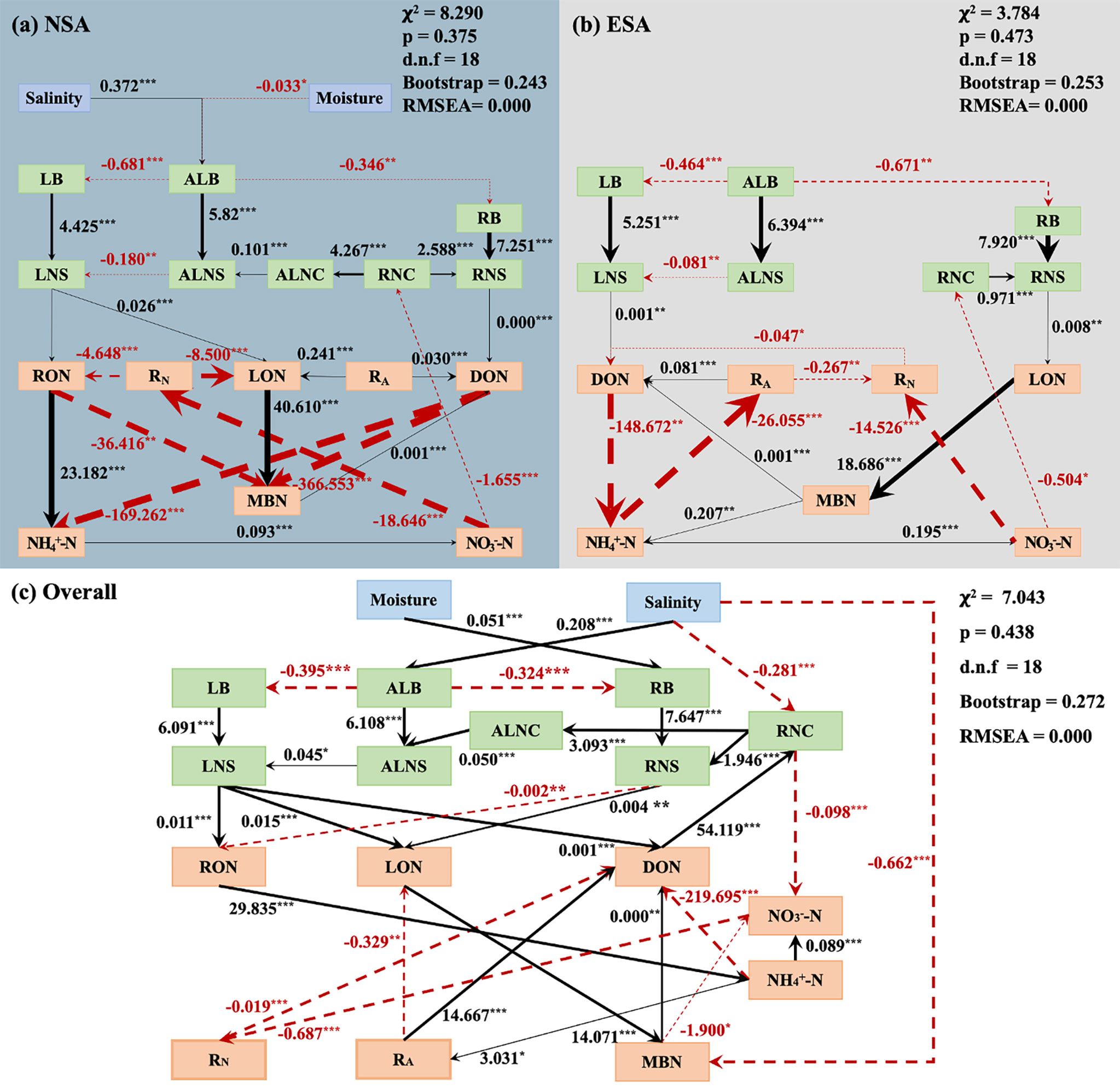
Figure 7 Nitrogen turnover among various nitrogen subpools in plant and soil subsystems in the (A) natural (NSA), and (B) embanked (ESA) salt marshes, as well as (C) their combine was examined by structural equation modelling (SEM). Solid and dashed arrows indicate positive and negative relationships, respectively. Numbers adjacent to arrows are the path coefficients (***p < 0.001, **p < 0.01 and *p < 0.05). ALB, aboveground living biomass; ALNS, aboveground living nitrogen storage; ALNC, aboveground living nitrogen concentration; LB, litter biomass; LNS, litter nitrogen storage; LNC, litter nitrogen concentration; RB, root biomass; RNS, root nitrogen storage; RNC, root nitrogen concentration; SON, total soil organic nitrogen; DON, dissolved organic nitrogen; LON, labile organic nitrogen; RON, recalcitrant organic nitrogen; NH+4 N, ammonia nitrogen; NO3 N, nitrite nitrogen; MBC, soil microbial biomass nitrogen; MBN, soil microbial biomass nitrogen; RA, soil net ammonification rate; RN, soil net nitrification rate.
Discussion
The N storages in the plant subsystem
The storage of N in plants is a vital component of ecosystem N pools (Liu et al., 2017). A recent study has reported that coastal embankments can alter the N storage of plants by influencing their growth (Feng et al., 2022). In this study, the embankment was observed to significantly reduce the N storage and biomass of aboveground living tissue year-round (Figure 2). SEM analysis indicated that low soil salinity induced low aboveground living biomass in the embanked S. alterniflora salt marsh (Figure 7), which was supported by our recent study (Feng et al., 2022). This might have been attributed to the chlorophyll content and net S. alterniflora assimilation rate that are reduced under low-salinity conditions, which decreases the generation of photosynthetic products and ultimately decreases the biomass (Ma et al., 2011). The decreased biomass of aboveground living tissues reduced their N storage (Figure 5). Furthermore, the decreased biomass and N storage of aboveground living tissues lowered the biomass and N storage of aboveground dead tissues (i.e., litter) in the embanked S. alterniflora salt marsh (Figure 7) (Jiang et al., 2009; Ma et al., 2011; Ge et al., 2015).
The embankment reduced the N storage of roots year-round, although it was insignificant in spring, summer, and autumn (Figure 2). SEM analysis suggested that low soil moisture could limit S. alterniflora root growth in the embanked salt marsh (Figure 7). This could be explained by S. alterniflora investing more nutrients for root growth when water resources were adequate, which enabled it to occupy more habitats (He et al., 2009). Further, this might reduce the tiller number and resource consumption by limiting the root growth when water was scarce (He et al., 2009). Thus, the decreased root biomass caused its N storage to be depressed (Figure 7). Besides, the temperature is generally considered as a vital factor which determines the plant growth (Elsey-Quirk et al., 2011). However, the root biomasses did not corelated with the temperature any more in the embanked salt marsh, which indicated that the embankment altered the seasonal variation of the root growth (Figure S4). This may be because the embankment altered the physiological process of S. alterniflora by changing the soil physicochemical properties, which affected its response to the temperature (Shi and Bao, 2007). In summary, since soil salinity and moisture are two critical drivers that influence plant growth in salt marshes, the coastal embankment reduced the N storage of S. alterniflora by restricting plant growth through decreased soil salinity and moisture (Figure 7).
The organic N subpool in the soil subsystem
The embankment drastically shrunk the size and modified compositions of the soil N pool in S. alterniflora salt marsh in every season (Figures 3, 5). The organic N subpool is an essential element of ecosystem N pools (Feyissa et al., 2020), while it regulates net plant primary production in most ecosystems and typically sensitive to changes in plant N storage (Jones et al., 2004; Chen et al., 2017; Feng et al., 2017). This is because soil organic matter was derived from the biodegradation of plant debris and dead roots (Figure 7; Zhou et al., 2015; Li et al., 2018). Thus, the embankment remarkably decreased the N storage of S. alterniflora litter and roots, which further reduced the size of the organic N subpools (e.g., SON, LON, RON, and DON) for every season (Figures 2, 3, 6).
The embankment also changed the N turnover from the plant subsystem to the soil subsystem in the S. alterniflora salt marsh, for example, the plant litter N storage (i.e., LNS) did not make the contribution to the size of the soil organic N subpool (i.e., SON) due to the embankment (Figures 6, 7). RON is generally composed of organics that are recalcitrant to decomposition (Lin et al., 2016; Xi et al., 2020; Xi et al., 2021). According to SEM analysis, the RON in the natural salt marsh was primarily derived from organic litter inputs (Figure 7). This was because lignin and lignocellulosic are rich in S. alterniflora litter, where the roots may not contain abundant refractory materials (Zeng et al., 2020; Feng et al., 2022). However, the effects of the litter N storages on the RON amount were negligible in the embanked salt marsh, which was possibly due to the litter N storage observably decreased due to the embankment (Figures 3, 7).
LON comprises the active component of the soil organic N subpools (Lin et al., 2016; Xi et al., 2020; Xi et al., 2021). The embankment also changed the source of LON, and the organic N inputs was from the litter in the natural salt marsh to the roots in the embanked salt marsh (Figure 7). This was probably also because the dramatically decreased litter and root N storage due to the embankment (Figure 2). However, the embankment did not alter the seasonal variation of LON (Figure 3). Generally, LON was more responsive to changes in plant N inputs compared to RON (Feyissa et al., 2020). On the coast of Jiangsu, the ALB of S. alterniflora attained a maximum in summer and autumn (Zhao et al., 2005; Zhang et al., 2010). Thus, the limited death of the aboveground S. alterniflora tissues led to a low LB in summer and autumn (Zhao et al., 2005; Zhang et al., 2010). Furthermore, the roots of S. alterniflora are nutrients storage tissues (Lawrence et al., 2013; Zhao et al., 2016). During the growing season, nutrients stored by roots were transported to support aboveground plant growth, thus the LB was low (Lytle and Hull, 1980; Lana et al., 1991; Chen et al., 2014). Therefore, lower LB and RB led to low LNS, RNS, and LON during summer and autumn for both natural and embanked S. alterniflora salt marshes (Figures 2, 3). In spring and winter, the aboveground living organs of S. alterniflora died and were transformed to litter; thus, high values of LB were observed (Figures 2, 3, 7) (Chen and Xu, 2006; Zhou et al., 2015). Additionally, S. alterniflora rapidly occupied habitats through the vegetative reproduction of its roots at the beginning of the growth season, and as a result the highest level of RB was observed in spring (Figure 2). Therefore, the elevated LB and RB resulted in the high LNS, RNS, and LON in spring and winter for both the natural and embanked S. alterniflora salt marshes (Figures 2, 3).
DON is the most active portion of the soil organic N subpool (Rentsch et al., 2007; Cott et al., 2020), which includes low-molecular weight compounds (e.g., amino acids, polypeptides, and purines) and high-molecular weight compounds (e.g., proteins and chlorophyll) (Quan et al., 2022). In this study, we found that the organic N inputs from roots was the primary source of DON in the natural salt marsh (Figure 7). This was possibly because the living roots could exude various low-molecular weight compounds (e.g., organic acids and sugar), meanwhile the decomposition of the death roots also promoted the accumulation of DON (Chen and Xu, 2006; Zhou et al., 2015). However, the DON in the embanked salt marsh mainly came from the organic N inputs from litter, which was explained by the passive leaching of soluble organic matter from litter in the soil (Figure 7; Chen and Xu, 2006; Zhou et al., 2015). Further, according to the SEM analysis, MBN was an additional important source of DON in the natural and embanked S. alterniflora salt marsh (Figure 7). This might be due to DON having the capacity to be released into the soil after microbial death (Isobe et al., 2018; Evangelou et al., 2021; Heinzle et al., 2021).
The inorganic N subpool in the soil subsystem
The embankment not only changed the process of the organic N inputs from the plant subsystem to the soil subsystem, but also altered the N turnover from the organic N subpools into the inorganic N subpools in the soil subsystem (Figure 7). Generally, the generation of inorganic N in soil is regulated by its organic N content (Cartaxana et al., 1999; Cott et al., 2020). SEM analysis indicated that the major contributor of the soil was RON in the natural salt marsh (Figure 7). Prior studies reported that bacteria and fungi, which can degrade high-molecular weight substances (e.g., RON), were rich in the S. alterniflora sediment (Yang et al., 2021; Feng et al., 2022). However, there was lack of contribution of RON to the soil in the embanked salt marsh, because there was inappreciable organic N input form the litter or roots to the soil RON (Figure 7). Additionally, according to the SEM analysis, an increased soil content can lead to decreased DON in the natural and embanked salt marshes (Figure 7). Since DON is rich in a variety of amino acids it can be mineralized to release through the microbial generation of extracellular enzymes (Berman and Bronk, 2003; Schimel and Bennett, 2004; Geisseler et al., 2009; Xing et al., 2010). Therefore, we speculated that DON is also a non-negligible source of in the soil of S. alterniflora salt marshes, where lower DON may lead to reduced in the soil following the establishment of the embankment. In summary, microorganisms may produce by decomposing the organic N; thus, the embankment significantly diminished the quantities of by shrinking the size of the organic N (e.g., RON, and DON) year-round (Figures 3, 7; Feng et al., 2022).
is another primary inorganic form of N that is intimately related to due to soil nitrification (Figure 7) (Ueda et al., 2013; Augustine et al., 2014; Nguyen et al., 2017). Thus, the concentrations of decreased under lower concentrations in summer, autumn, and winter after the establishment of the embankment (Figure 7). Nevertheless, the concentration increased significantly in spring following embankment development (Figure 3). Thus, we speculated that, (1) nitrification might continue in spring, even when the soil concentration was low (Augustine et al., 2014; Schütt et al., 2014; Heinzle et al., 2021); (2) the rapid decrease in utilization by plants at the onset of plant growth occurred in spring due to the dramatically decreased plant biomass following the establishment of the embankment (Augustine et al., 2014; Che et al., 2018; Heinzle et al., 2021).
Microbial N mineralization and immobilization
Organic soil N is converted to inorganic N via N mineralization, which is influenced by multiple factors (Zhou et al., 2015; Nguyen et al., 2017). In this study, RA in the embanked salt marsh decreased significantly in spring and summer but rapidly increased in autumn (Figure 4). Previous studies have reported that the soil N mineralization rate was determined by microbial activities, which are always highly associated with the temperatures (Augustine et al., 2014; Lozano-García et al., 2016; Heinzle et al., 2021). For example, higher temperatures can stimulate microbial activities, which directly expedite the soil N turnover rate (Lozano-García et al., 2016). In the study, we found RA was positive correlation with temperatures in the natural salt marsh, whereas the association between RA and temperatures was not observed in the embanked salt marsh (Figure S4). This result indicated that the embankment probably dramatically modified the microbial responses to the temperature changes, which ultimately altered the seasonal variation of RA (Figure 4). Simultaneously, the maximum and minimum RA values were observed in summer and autumn, respectively, in both the natural and embanked salt marshes (Figure 4). Organic soil N, particularly DON, serves as a vital mineralization substrate that drives the rate of soil N mineralization (Figure 7; Montano et al., 2007; Näsholm et al., 2009). In fact, previous studies have reported that soluble organic matter can provide energy for heterotrophic microorganisms, which is favored for higher levels of N mineralization (Montano et al., 2007; Näsholm et al., 2009). This was supported by the SEM analysis of this study (Figure 7). The highest and lowest DON amounts were observed in summer and autumn, respectively; thus, the seasonal variations in RA may be contributed to by those of the DON content (Figures 3, 4). Besides, enhanced microbial activities and accelerated soil N turnover rates under increased temperatures stimulated RA in summer and autumn either (Figures 3, 7; Augustine et al., 2014; Lozano-García et al., 2016; Heinzle et al., 2021).
Furthermore, the RN decreased significantly in spring and summer, while it increased to a considerable degree in autumn following the establishment of the embankment (Figure 4). These changes were consistent with the variations in RA following embankment development, as organic matter initially mineralized to . Subsequently, was derived from the nitrification of (Figure 4; Khalsa et al., 2020).
Microbial immobilization is also a crucial process in the soil N cycle, as organic and inorganic N can be assimilated by microbes and rapidly converted to MBN (Fahey et al., 2011; Nguyen et al., 2017). In this study, high LON concentrations promoted the accumulation of MBN in the natural and embanked salt marshes according to the SEM analysis, which suggested that soil LON was a vital N source for microorganisms (Figure 7). Nevertheless, the embankment observably decreased the soil LON, but it dramatically elevated the MBN amount in summer and autumn (Figures 3, 4). Besides, earlier studies reported that MBN was closely and positively correlated with MBC, SOC, and SON in general. Whereas in this study, MBC concentrations did not increase significantly, and concentrations of SOC and SON prominently decreased in summer or autumn (Figures 3, 4; Manirakiza et al., 2019). Therefore, the increased MBN content was not caused by lower concentrations of LON, MBC, SOC, or SON. SEM analysis revealed that the decreased soil salinity following embankment establishment raised the concentration of MBN (Figure 7). Salinity is a vital factor that drives the growth of microbes in coastal wetlands due to its being the determinant of the osmotic potential of the soil (Gao et al., 2012). Consequently, the decreased soil salinity induced an increased solution osmotic potential, which ultimately enhanced the uptake of ions (e.g., ) by microbes and stimulated the production of MBN in the embanked S. alterniflora salt marsh (Figure 7) (Mavi and Marschner, 2013).
Plants N absorption
The soil N pool is not only the N sink but also the N source for plants in salt marshes (Geisseler et al., 2009). Soil inorganic N is the primarily source of N for plants (Jones et al., 2004; Jones et al., 2005). Based on SEM analysis, increased RNC led to decreased soil but had little impact on soil (Figure 7). This indicated that S. alterniflora possessed a greater capacity to take up rather than from soil (Jones et al., 2005). This was possibly due to not being easily adsorbed by soil particles with negative charges, and its easy leaching into the soil for take up by plants (Jones et al., 2005). Besides, according to the SEM analysis, increased soil DON stimulated RNC, which indicated that DON is another essential available source of N supplies for plant uptake (Figure 7; Kerley and Read, 1995; Alexandre et al., 2015; Cott et al., 2020). Earlier studies revealed that plants can acquire a wide variety of low molecular weight N compounds from DON as the N source, via roots when they grow rapidly (e.g., amino acids, amino sugars, and peptides) (Kerley and Read, 1995; Alexandre et al., 2015; Cott et al., 2020). Soil N (e.g., and DON) can be absorbed by roots, which promoted the growth and N storage of roots (i.e., RB and RNS). Moreover, the N absorbed by roots can be transferred from root tissues to aboveground living tissues, which promote their N storage (i.e., ALNS) in the natural salt marsh (Figure 7). However, the embankment seems stop the N transfer from root tissues to aboveground living tissues (Figure 7). Therefore, the embankment also converted N absorption of plants (Figure 7).
Conclusion
The coastal embankment dramatically reduced the plant N storage and the soil N pool in the S. alterniflora salt marsh year-round, and modified the N transfer processes in the plant subsystem and soil subsystem. Firstly, the litter and root biomass of S. alterniflora were significantly decreased due to the lower soil salinity and moisture in the embanked salt marsh, compared to those in the natural salt marsh for every season. Further, the decreased litter and root biomass of S. alterniflora translated to the remarkable reduction of N storage in the embanked salt marsh year-round. Secondly, due to the decreased N inputs from plants, the soil organic N subpool (e.g., SON, RON, LON, DON) was observably reduced during every season to varying degrees. Thirdly, the embankment also significantly diminished most of the soil inorganic N subpool (i.e., and ) which was regulated by the diminution of the organic N content (e.g., RON and DON). Fourthly, although the sizes of the soil organic and inorganic N subpools were reduced, the soil MBN concentrations increased in spring, summer, and winter at different levels due to the embankment. We suggest that the decreased soil salinity following the development of the embankment induced a higher osmotic potential, which ultimately increased the uptake of ions (e.g., ) by microbes, while stimulating the production of MBN. Ultimately, the soil and DON were two vital N sources for S. alterniflora, and plants absorbed N from the soil and promote their biomass, N concentrations, and storage (e.g., RB, RNC, RNS, ALB, ALNS). Our study is conducive toward further understanding the mechanisms behind the effects of coastal embankments on the seasonal variations in plant-soil N pools.
Data availability statement
The original contributions presented in the study are included in the article/Supplementary Material. Further inquiries can be directed to the corresponding authors.
Author contributions
GQ: writing – original draft. HF: conceptualization, software, formal analysis, data curation, and writing – original draft. HZ: methodology and investigation. LX: conceptualization, methodology, software, formal analysis, data curation, writing – original draft, writing – review and editing, and visualization. WY: conceptualization and methodology. YZ: methodology, resources, and investigation. NJ: writing – review and editing. SA: conceptualization, resources, writing – review and editing, project administration, and funding acquisition. All authors contributed to the article and approved the submitted version.
Funding
This study was supported by the National Program on Key Basic Research Project (973 Program) [grant No. 2013CB430405].
Acknowledgments
The authors thank the Dafeng Milu National Nature Reserve and Yancheng National Nature Reserve for their assistants in the field investigation.
Conflict of interest
The authors declare that the research was conducted in the absence of any commercial or financial relationships that could be construed as a potential conflict of interest.
Publisher’s note
All claims expressed in this article are solely those of the authors and do not necessarily represent those of their affiliated organizations, or those of the publisher, the editors and the reviewers. Any product that may be evaluated in this article, or claim that may be made by its manufacturer, is not guaranteed or endorsed by the publisher.
Supplementary material
The Supplementary Material for this article can be found online at: https://www.frontiersin.org/articles/10.3389/fmars.2022.959144/full#supplementary-material.
Abbreviations
ALB, Aboveground living biomass; ANC, Aboveground living N concentration; ANS, Aboveground living N storage; ANOVA, Analysis of variance; BPlant, biomass per centiare; CNPlant, N concentration; DON, Dissolved organic N; ESA, Embanked Spartina alterniflora salt marshes; LB, Litter biomass; LNC, Litter N concentration; LNS, Litter N storage; LON, Labile organic N; MBC, Microbial biomass carbon; MBN, Microbial biomass N; N, Nitrogen; , Ammonium N; NMDS, Non-metric Multi-Dimensional Scaling; , Nitrate N; , Nitrite N; NSA Natural Spartina alterniflora salt marshes; PE, Polyethylene; RA Soil net, ammonification rate; RB, Root biomass; RN Soil net, nitrification rate; RNC, Root N concentration; RNS, Root N storage; RMSEA, Root-mean-square error of approximation; RON, Recalcitrant organic N; SN Plant, N storage; SON Total; soil organic N; SEM, Structural equation modelling.
References
Abu Hena M. K., Sidik B. J., Idris M. H., Nesarul M. H., Aysha A., Islam M. S., et al. (2015). Forest structure and litter production of naturally re-generated white mangrove Avicennia marina in sub-tropical estuarine coast. J. Environ. Biol. 36, 1199–1204.
Ake-Castillo J. A., Vazquez G., Lopez-Portillo J. (2006). Litterfall and decomposition of Rhizophora mangle l. in a coastal lagoon in the southern gulf of Mexico. Hydrobiologia 559, 101–111. doi: 10.1007/s10750-005-0959-x
Alexandre A., Hill P. W., Jones D. L., Santos R. (2015). Dissolved organic nitrogen: A relevant, complementary source of nitrogen for the seagrass Zostera marina. Limnol. Oceanogr. 6, 1477–1483. doi: 10.1002/lno.10084
An S. Q., Gu B. H., Zhou C. F., Wang Z. S., Deng Z. F., Zhi Y. B., et al. (2007). Spartina invasion in China: Implications for invasive species management and future research. Weed Res. 47, 183–191. doi: 10.1111/j.1365-3180.2007.00559.x
Aufdenkampe A. K., Mayorga E., Raymond P. A., Melack J. M., Doney S. C., Alin S. R., et al. (2011). Riverine coupling of biogeochemical cycles between land, oceans, and atmosphere. Front. Ecol. Environ. 9, 53–60. doi: 10.1890/100014
Augustine D. J., Brewer P., Blumenthal D. M., Derner J. D., Fischer J. C. (2014). Prescribed fire, soil inorganic nitrogen dynamics, and plant responses in a semiarid grassland. J. Arid Environ. 104, 59–66. doi: 10.1016/j.jaridenv.2014.01.022
Barbier E. B., Hacker S. D., Kennedy C., Koch E. W., Stier A. C., Silliman B. R. (2011). The value of estuarine and coastal ecosystem services. Ecol. Monogr. 81, 169–193. doi: 10.1890/10-1510.1
Battle J. M., Golladay S. W. (2007). How hydrology, habitat type, and litter quality affect leaf breakdown in wetlands on the gulf coastal plain of Georgia. Wetlands 27, 251–260. doi: 10.1672/0277-5212(2007)27[251:HHHTAL]2.0.CO;2
Berman T., Bronk D. A. (2003). Dissolved organic nitrogen: A dynamic participant in aquatic ecosystems. Aquat. Microb. Ecol. 31, 279–305. doi: 10.3354/ame031279
Boldea O., Magnus J. R. (2009). Maximum likelihood estimation of the multivariate normal mixture model. J. Am. Stat. Assoc. 104, 1539–1549. doi: 10.1198/jasa.2009.tm08273
Brookes P. C., Landman A., Pruden G., Jenkinson D. S. (1985). Chloroform fumigation and the release of soil-nitrogen - a rapid direct extraction method to measure microbial biomass nitrogen in soil. Soil Biol. Biochem. 17, 837–842. doi: 10.1016/0038-0717(85)90144-0
Cartaxana P., Cacador I., Vale C., Falcao M., Catarino F. (1999). Seasonal variation of inorganic nitrogen and net mineralization in a salt marsh ecosystem. Mangrove Salt Marshes 3, 127–134. doi: 10.1023/A:1009941219215
Chen G. Z., Lu Q. Q., Bai J. H., Wen L. X., Zhang G. L., Wang W., et al. (2022). Organic sulfur mineralization in surface soils from coastal wetlands with different flooding periods affected by the flow-sediment regulation in the yellow river delta, China. Catena 215, 106343. doi: 10.1016/j.catena.2022.106343
Chen C. R., Xu Z. H. (2006). On the nature and ecological functions of soil soluble organic nitrogen (SON) in forest ecosystems.s. J. Soil Sediment 6, 63–66. doi: 10.1065/jss2006.06.159
Chen S. Y., Yoshitake S., Iimura Y., Asai C., Ohtsuka T. (2017). Dissolved organic carbon (DOC) input to the soil: DOC fluxes and their partitions during the growing season in a cool-temperate broad-leaved deciduous forest, central Japan. Ecol. Res. 32, 713–724. doi: 10.1007/s11284-017-1488-6
Chen Y., Zeng Z. H., Qin X. J., Yang G. (2014). Monthly variation of asexual reproduction of Spartina alterniflora (Loisel). J. Biosaf. 23, 173–177. doi: 10.3969/j.issn.2095-1787.2014.03.006
Che R. X., Qin J. L., Tahmasbian I., Wang F., Zhou S. T., Xu Z. H., et al. (2018). Litter amendment rather than phosphorus can dramatically change inorganic nitrogen pools in a degraded grassland soil by affecting nitrogen-cycling microbes. Soil Biol. Biochem. 120, 145–152. doi: 10.1016/j.soilbio.2018.02.006
Cott G. M., Jansen M. A., Megonigal J. P. (2020). Uptake of organic nitrogen by coastal wetland plants under elevated CO2. Plant Soil 450, 521–535. doi: 10.1007/s11104-020-04504-5
Cui L. L., Li G. S., Ouyang N. L., Mu F. Y., Yan F., Zhang Y. T., et al. (2018). Analyzing coastal wetland degradation and its key restoration technologies in the coastal area of jiangsu, China. Wetlands 38, 525–537. doi: 10.1007/s13157-018-0997-6
Dovrat G., Sheffer E. (2019). Symbiotic dinitrogen fixation is seasonal and strongly regulated in water-limited environments. N. Phytol. 221, 1866–1877. doi: 10.1111/nph.15526
Elsey-Quirk T., Seliskar D. M., Gallagher J. L. (2011). Nitrogen pools of macrophyte species in a coastal lagoon salt marsh: implications for seasonal storage and dispersal. Estuar. Coast. 34, 470–482. doi: 10.1007/s12237-011-9379-5
Evangelou E., Tsadilas C., Giourga C. (2021). Seasonal variation of soil microbial biomass carbon and nitrogen as affected by land use in a Mediterranean agro- ecosystem. Commun. Soil Sci. Plan. 52, 222–234. doi: 10.1080/00103624.2020.1854298
Fahey T. J., Yavitt J. B., Sherman R. E., Groffman P. M., Fisk M. C., Maerz J. C. (2011). Transport of carbon and nitrogen between litter and soil organic matter in a northern hardwood forest. Ecosystems 14, 326–340. doi: 10.1007/s10021-011-9414-1
Feng H. Y., Zhao H., Xia L., Yang W., Zhao Y. Q., Jeelani N., et al. (2022). Nitrogen cycling in plant and soil subsystems is driven by changes in soil salinity following coastal embankment in typical coastal saltmarsh ecosystems of Eastern China. Ecol. Eng. 174, 106467. doi: 10.1016/j.ecoleng.2021.106467
Feng J. X., Zhou J., Wang L. M., Cui X. W., Ning C. X., Wu H., et al. (2017). Effects of short-term invasion of Spartina alterniflora and the subsequent restoration of native mangroves on the soil organic carbon, nitrogen and phosphorus stock. Chemosphere 184, 774–783. doi: 10.1016/j.chemosphere.2017.06.060
Feyissa A., Yang F., Feng J., Wu J. J., Chen Q., Cheng X. L. (2020). Soil labile and recalcitrant carbon and nitrogen dynamics in relation to functional vegetation groups along precipitation gradients in secondary grasslands of south China. Environ. Sci. Pollut. Res. 27, 10528–10540. doi: 10.1007/s11356-019-07583-9
Gao H. F., Bai J. H., Xiao R., Yan D. H., Huang L. B., Huang C. (2012). Soil net nitrogen mineralization in salt marshes with different flooding periods in the yellow river delta, China. Clean Soil Air Water 40, 1111–1117. doi: 10.1002/clen.201200031
Ge Z. M., Guo H. Q., Zhao B., Zhang L. Q. (2015). Plant invasion impacts on the gross and net primary production of the salt marsh on eastern coast of China: Insights from leaf to ecosystem. J. Geophys Res.-Biogeo. 120, 169–186. doi: 10.1002/2014JG002736
Geisseler D., Horwath W. R., Doane T. A. (2009). Significance of organic nitrogen uptake from plant residues by soil microorganisms as affected by carbon and nitrogen availability. Soil Biol. Biochem. 41, 1281–1288. doi: 10.1016/j.soilbio.2009.03.014
Heinzle J., Wanek W., Tian Y., Kengdo S. K., Borken W., Schindlbacher A., et al. (2021). No effect of long-term soil warming on diffusive soil inorganic and organic nitrogen fluxes in a temperate forest soil. Soil Biol. Biochem. 158, 108261. doi: 10.1016/j.soilbio.2021.108261
He J., Zhao C. J., Qing H., Gan L., An S. Q. (2009). Effect of soil-water condition on morphological plasticity of clonal plant Spartina alterniflora. Acta Ecol. Sin. 29, 3518–3524. doi: 10.3321/j.issn:1000-0933.2009.07.009
Huang L. B., Bai J. H., Gao H. F., Xiao R., Liu P. P., Chen B. (2013). Soil organic carbon content and storage of raised field wetlands in different functional zones of a typical shallow freshwater lake, China. Soil Res. 50, 664–671. doi: 10.1071/SR12236
Huang F., Lin X., Hu W., Zeng F., He L., Yin K. (2021). Nitrogen cycling processes in sediments of the pearl river estuary: Spatial variations, controlling factors, and environmental implications. Catena 206, 105545. doi: 10.1016/j.catena.2021.105545
Hu R., Wang X. P., Pan Y. X., Zhang Y. F., Zhang Y. F., Zhang H., et al. (2015). Seasonal variation of net n mineralization under different biological soil crusts in tengger desert, north China. Catena 127, 9–16. doi: 10.1016/j.catena.2014.12.012
Iost S., Landgraf D., Makeschin F. (2007). Chemical soil properties of reclaimed marsh soil from zhejiang province PR China. Geoderma 142, 245–250. doi: 10.1016/j.geoderma.2007.08.001
Isobe K., Oka H., Watanabe T., Tateno R., Urakawa R., Liang C., et al. (2018). High soil microbial activity in the winter season enhances nitrogen cycling in a cool-temperate deciduous forest. Soil Biol. Biochem. 124, 90–100. doi: 10.1016/j.soilbio.2018.05.028
Jiang L. F., Luo Y. Q., Chen J. K., Li B. (2009). Ecophysiological characteristics of invasive spartina alterniflora and native species in salt marshes of Yangtze river estuary, China. Estuar. Coast. Shelf Sci. 81, 74–82. doi: 10.1016/j.ecss.2008.09.018
Jones D. L., Healey J. R., Willett V. B., Farrar J. F., Hodge A. (2005). Dissolved organic nitrogen uptake by plants - An important n uptake pathway. Soil Biol. Biochem. 37, 413–423. doi: 10.1016/j.soilbio.2004.08.008
Jones D. L., Shannon D., Murphy D. V., Farrar J. (2004). Role of dissolved organic nitrogen (DON) in soil n cycling in grassland soils. Soil Biol. Biochem. 36, 749–756. doi: 10.1016/j.soilbio.2004.01.003
Kerley S. J., Read D. J. (1995). The biology of mycorrhizal in the ericaceae. XVIII. chitin degradation by Hymenoscyphus ericae and transfer of chitin-nitrogen to the host plant. N. Phytol. 131, 369–375. doi: 10.1111/j.1469-8137.1995.tb03073.x
Khalsa S. D. S., Smart D. R., Muhammad S., Armstrong C. M., Sanden B. L., Houlton B. Z., et al. (2020). Intensive fertilizer use increases orchard n cycling and lowers net global warming potential. Sci. Total Environ. 722, 137889. doi: 10.1016/j.scitotenv.2020.137889
Kirwan M. L., Megonigal J. P. (2013). Tidal wetland stability in the face of human impacts and sea-level rise. Nature 504, 53–60. doi: 10.1038/nature12856
Lana P. C., Guiss C., Disaró S. T. (1991). Seasonal-variation of biomass and production dynamics for aboveground and belowground components of a spartina alterniflora marsh in the euhaline sector of paranaguá bay. Estuar. Coast. Shelf Sci. 32, 231–241. doi: 10.1016/0272-7714(91)90017-6
Lawrence B. A., Fahey T. J., Zedler J. B. (2013). Root dynamics of Carex stricta-dominated tussock meadows. Plant Soil 364, 325–339. doi: 10.1007/s11104-012-1360-y
Lin G., Lin X. (2022). Bait input altered microbial community structure and increased greenhouse gases production in coastal wetland sediment. Water Res. 218, 118520. doi: 10.1016/j.watres.2022.118520
Lin W., Ma H., Pei G. T., Gao R., Yin Y. F., Lin Y. Y. (2016). Effects of nitrogen addition on soil carbon and nitrogen pools in mid-subtropical forest. Res. Environ. Sci. 29, 67–76. doi: 10.13198/j.issn.1001-6929.2016.01.09
Liu S., Luo D., Liu Q. L., Zhang L., Yang H. G., Shi Z. M. (2017). Carbon and nitrogen storage and distribution in different forest ecosystems in the subalpine of western sichuan. Acta Ecol. Sin. 37, 1074–1083. doi: 10.5846/stxb201604150688
Liu P., Qiu Y., Wang Y. T., Wei Z. P., Fan J. G., Cao B. H. (2019). Seasonal dynamics of soil microbial biomass in typical shelterbelts on the bohai muddy coast. Acta Ecol. Sin. 39, 363–370. doi: 10.5846/stxb201711021961
Li R. X., Yu Q., Wang Y. W., Wang Z. B., Gao S., Flemming B. (2018). The relationship between inundation duration and Spartina alterniflora growth along the jiangsu coast, China. Estuar. Coast. Shelf Sci. 213, 305–313. doi: 10.1016/j.ecss.2018.08.027
Lozano-García B., Parras-Alcántara L., Brevik E. C. (2016). Impact of topographic aspect and vegetation (native and reforested areas) on soil organic carbon and nitrogen budgets in Mediterranean natural areas. Sci. Total Environ. 544, 963–970. doi: 10.1016/j.scitotenv.2015.12.022
Lytle R. W., Hull R. J. (1980). Photoassimilate distribution in Spartina alterniflora loisel. II. autumn and winter storage and spring regrowth. Agron. J. 72, 933–938. doi: 10.2134/agronj1980.00021962007200060017x
Ma J. A., Chai M. W., Shi F. C. (2011). Effects of long-term salinity on the growth of the halophyte Spartina alterniflora loisel. Afr. J. Biotechnol. 10, 17962–17968. doi: 10.5897/AJB11.2300
Ma Z., Melville D. S., Liu J., Chen Y., Yang H., Ren W., et al. (2014). Rethinking China’s new great wall. Science 346, 912–914. doi: 10.1126/science.1257258
Manirakiza E., Ziadi N., St Luce M., Hamel C., Antoun H., Karam A. (2019). Nitrogen mineralization and microbial biomass carbon and nitrogen in response to co-application of biochar and paper mill biosolids. Appl. Soil Ecol. 142, 90–98. doi: 10.1016/j.apsoil.2019.04.025
Mavi M. S., Marschner P. (2013). Salinity affects the response of soil microbial activity and biomass to addition of carbon and nitrogen. Soil Res. 1, 68–75. doi: 10.1071/SR12191
Montano N. M., García-Oliva F., Jaramillo V. J. (2007). Dissolved organic carbon affects soil microbial activity and nitrogen dynamics in a Mexican tropical deciduous forest. Plant Soil 295, 265–277. doi: 10.1007/s11104-007-9281-x
Näsholm T., Kielland K., Ganeteg U. (2009). Uptake of organic nitrogen by plants. N. Phytol. 182, 31–48. doi: 10.1111/j.1469-8137.2008.02751.x
Nguyen T. T. N., Xu C. Y., Tahmasbian I., Che R. X., Xu Z. H., Zhou X. H., et al. (2017). Effects of biochar on soil available inorganic nitrogen: A review and meta-analysis. Geoderma 288, 79–96. doi: 10.1016/j.geoderma.2016.11.004
Patten K., O’Casey C., Metzger C. (2017). Large-Scale chemical control of smooth cordgrass (Spartina alterniflora) in willapa bay, WA: Towards eradication and ecological restoration. Invas. Plant Sci. Mana. 10, 284–292. doi: 10.1017/inp.2017.25
Purvaja R., Ramesh R., Ray A. K., Rixen T. (2008). Nitrogen cycling: A review of the processes, transformations and fluxes in coastal ecosystems. Curr. Sci. 94, 1419–1438.
Quan Z., Liu X. A., Liu D. (2022). Research progress on soil soluble organic nitrogen. Chin. J. Appl. Ecol. 33, 277–288. doi: 10.13287/j.1001-9332.202201.010
Raison R. J., Connell M. J., Khanna P. K. (1987). Methodology for studying fluxes of soil mineral-n in-situ. Soil Biol. Biochem. 19, 521–530. doi: 10.1016/0038-0717(87)90094-0
Ren M. E. (1986). Comprehensive investigation of the coastal zone and tidal land resources of jiangsu province (Beijing: Ocean Press).
Rentsch D., Schmidt S., Tegeder M. (2007). Transporters for uptake and allocation of organic nitrogen compounds in plants. FEBS Lett. 581, 2281–2289. doi: 10.1016/j.febslet.2007.04.013
Rovira P., Vallejo V. R. (2002). Labile and recalcitrant pools of carbon and nitrogen inorganic matter decomposing at different depths in soil: An acid hydrolysis approach. Geoderma 107, 109–141. doi: 10.1016/S0016-7061(01)00143-4
Sanders-DeMott R., Sorensen P. O., Reinmann A. B., Templer P. H. (2018). Growing season warming and winter freeze–thaw cycles reduce root nitrogen uptake capacity and increase soil solution nitrogen in a northern forest ecosystem. Biogeochemistry 137, 337–349. doi: 10.1007/s10533-018-0422-5
Schermelleh-Engel K., Moosbrugger H., Müller H. (2003). Evaluating the fit of structural equation models: tests of significance and descriptive goodness-of -fit measures. Methods Psychol. Res. Online 8, 23–74. doi: 10.13287/j.1001-9332.202201.010
Schimel J. P., Bennett J. (2004). Nitrogen mineralization: Challenges of a changing paradigm. Ecology 85, 591–602. doi: 10.1890/03-8002
Schütt M., Borken W., Spott O., Stange C. F., Matzner E. (2014). Temperature sensitivity of c and n mineralization in temperate forest soils at low temperatures. Soil Biol. Biochem. 69, 320–327. doi: 10.1016/j.soilbio.2013.11.014
Shi F. C., Bao F. (2007). Effects of salt and temperature stress on ecophysiological characteristics of exotic cordgrass, Spartina alterniflora. Acta Ecol. Sin. 27, 2733–2741. doi: 10.1016/S1872-2032(07)60061-4
Slavich P. G., Petterson G. H. (1990). Estimating average rootzone salinity from electromagnetic induction (EM-38) measurements. Aust. J. Soil Res. 28, 453–463. doi: 10.1071/SR9900453
Socci A. M., Templer P. H. (2011). Temporal patterns of inorganic nitrogen uptake by mature sugar maple (Acer saccharum marsh.) and red spruce (Picea rubens sarg.) trees using two common approaches. Plant Ecol. Divers. 4, 141–152. doi: 10.1080/17550874.2011.624557
Ueda M. U., Muller O., Nakamura M., Nakaji T., Hiura T. (2013). Soil warming decreases inorganic and dissolved organic nitrogen pools by preventing the soil from freezing in a cool temperate forest. Soil Biol. Biochem. 61, 105–108. doi: 10.1016/j.soilbio.2013.02.016
Urakawa R., Shibata H., Kuroiwa M., Inagaki Y., Tateno R., Hishi T., et al. (2014). Effects of freeze–thaw cycles resulting from winter climate change on soil nitrogen cycling in ten temperate forest ecosystems throughout the Japanese archipelago. Soil Biol. Biochem. 74, 82–94. doi: 10.1016/j.soilbio.2014.02.022
van de Broek M., Baert L., Temmerman S., Govers G. (2019). Soil organic carbon stocks in a tidal marsh landscape are dominated by human marsh embankment and subsequent marsh progradation. Eur. J. Soil Sci. 70, 338–349. doi: 10.1111/ejss.12739
Vitousek P. M., Aber J. D., Howarth R. W., Likens G. E., Matson P. A., Schindler D. W., et al. (1997). Human alteration of the global nitrogen cycle: Sources and consequences. Ecol. Appl. 7, 737–750. doi: 10.1890/1051-0761(1997)007[0737:HAOTGN]2.0.CO;2
Wang Z. X., Chen Q. M., Huang Y. Y., Deng H. N., Shen X., Tang S. Y., et al. (2019). Response of soil respiration and microbial biomass carbon and nitrogen to nitrogen application in subalpine forests of western sichuan. Acta Ecol. Sin. 39, 7197–7207. doi: 10.5846/stxb201805271164
Wang T., Wang K., Jiang X. (2018). Transportation of nitrogen between water column and surficial sediments in simulate wetting season. Fresen. Environ. Bull. 27, 6460–6468.
Wan S. W., Qin P., Liu J. N., Zhou H. X. (2009). The positive and negative effects of exotic Spartina alterniflora in China. Ecol. Eng. 35, 444–452. doi: 10.1016/j.ecoleng.2008.05.020
Wuest S. B. (2015). Seasonal variation in soil bulk density, organic nitrogen, available phosphorus, and pH. Soil Sci. Soc Am. J. 79, 1188–1197. doi: 10.2136/sssaj2015.02.0066
Xing S. H., Chen C. R., Zhou B. Q., Zhang H., Nang Z. M., Xu Z. H. (2010). Soil soluble organic nitrogen and active microbial characteristics under adjacent coniferous and broadleaf plantation forests. J. Soil Sediment 10, 748–757. doi: 10.1007/s11368-009-0159-9
Xi D., Weng H. D., Hu Y. L., Wu J. P. (2021). Effects of canopy nitrogen addition and understory removal on soil organic carbon fractions in a Chinese fir plantation. Acta Ecol. Sin. 41, 8525–8534. doi: 10.5846/stxb202008162130
Xi D., Yu Z. P., Xiong Y., Liu X. Y., Liu J. (2020). Altitudinal changes of soil organic carbon fractions of evergreen broadleaved forests in guanshan mountain, Jiangxi, China. Chin. J. Appl. Ecol. 31, 3349–3356. doi: 10.13287/j.1001-9332.202010.009
Xu G., Zhuo R. (1985). Preliminary studies of introduced Spartina alterniflora loisel in China. J. Nanjing Univ. 1, 212–225.
Yang W., Jeelani N., Cai A. D., Cheng X. L., An S. Q. (2021). Coastal reclamation alters soil microbial communities following different land use patterns in the Eastern coastal zone of China. Sci. Rep. 11, 7265. doi: 10.1038/s41598-021-86758-2
Yang W., Li N., Leng X., Qiao Y. J., Cheng X. L., An S. Q. (2016). The impact of sea embankment reclamation on soil organic carbon and nitrogen pools in invasive Spartina alterniflora and native Suaeda salsa salt marshes in eastern China. Ecol. Eng. 97, 582–592. doi: 10.1016/j.ecoleng.2016.10.064
Zeng A., Hu W. F., Zeng C. S., Sun Z. G., Gao D. Z. (2020). Litter decomposition and nutrient dynamics of native species (Cyperus malaccensis) and alien invasive species (Spartina alterniflora) in a typical subtropical estuary (Min river) in China. Estuar. Coast. 43, 1873–1883. doi: 10.1007/s12237-020-00744-x
Zhang X. Q., Wang G. X., Wang Y. (2006). The changes of erosion or progradation of tidal flat and retreat or extension of wetland vegetation of the yancheng coast, jiangsu. Mar. Sci. 30, 35–39. doi: 10.3969/j.issn.1000-3096.2006.06.007
Zhang X., Yan C., Xu P., Dai Y., Yan W., Ding X., et al. (2013). Historical evolution of tidal flat reclamation in the jiangsu coastal areas. Acta Geogra. Sinca 68, 1549–1558. doi: 10.11821/dlxb201311010
Zhang Y. H., Zhang F. C., Li Y. X., Xie X. J., Zhou X. D., Li Q., et al. (2010). Influence of exogenous n import on growth and leaf character of Spartina alterniflora. Ecol. Environ. Sci. 19, 2297–2301. doi: 10.1016/S1872-5813(11)60001-7
Zhao Q. Q., Bai J. H., Liu Q., Lu Q. Q., Gao Z. Q., Wang J. J. (2016). Spatial and seasonal variations of soil carbon and nitrogen content and stock in a tidal salt marsh with Tamarix chinensis, China. Wetlands 36, 145–152. doi: 10.1007/s13157-015-0647-1
Zhao G. Q., Zhang L. Q., Liang X. (2005). A comparison of photosynthetic characteristics between an invasive plant spartina alterniflora and an indigenous plant Phragmites australis. Acta Ecol. Sin. 25, 1604–1611. doi: 10.3321/j.issn:1000-0933.2005.07.011
Zhou S. W., Bi X. L. (2020). Seawall effects in a coastal wetland landscape: spatial changes in soil carbon and nitrogen pools. J. Coast. Conserv. 24, 1–11. doi: 10.1007/s11852-019-00718-7
Keywords: coastal reclamation, soil nitrogen subpool, nitrogen cycling, plant nitrogen subpool, soil microbial biomass
Citation: Qin G, Feng H, Zhao H, Xia L, Yang W, Zhao Y, Jeelani N and An S (2022) Seasonal soil-plant nitrogen dynamics of a cordgrass salt marsh in response to coastal embankments in Eastern China. Front. Mar. Sci. 9:959144. doi: 10.3389/fmars.2022.959144
Received: 01 June 2022; Accepted: 04 July 2022;
Published: 25 July 2022.
Edited by:
Xianbiao Lin, Ocean University of China, ChinaReviewed by:
Weifang Hu, Guangdong Academy of Agricultural Sciences, ChinaGenmei Lin, School of Marine Sciences, Sun Yat-sen University, China
Copyright © 2022 Qin, Feng, Zhao, Xia, Yang, Zhao, Jeelani and An. This is an open-access article distributed under the terms of the Creative Commons Attribution License (CC BY). The use, distribution or reproduction in other forums is permitted, provided the original author(s) and the copyright owner(s) are credited and that the original publication in this journal is cited, in accordance with accepted academic practice. No use, distribution or reproduction is permitted which does not comply with these terms.
*Correspondence: Lu Xia, bHVsdTg2NjhAeWVhaC5uZXQ=;Shuqing An, YW5zaHFAbmp1LmVkdS5jbg==
†ORCID: Hongyu Feng, orcid.org/0000-0001-9658-3498
Lu Xia, orcid.org/0000-0001-6041-3008
Shuqing An, orcid.org/0000-0001-7948-6450
‡These authors have contributed equally to this work