- 1Laboratory of Molecular Cytogenetic and Mutagenesis, Department of Ecological and Biological Sciences, University of Tuscia, Viterbo, Italy
- 2Laboratory of Veterinary Anatomy and Pathology, Department of Comparative Biomedicine and Food Sciences, University of Padua, Padua, Italy
- 3Laboratory of Molecular Ecology, Ichthyogenic Experimental Marine Centre (CISMAR), Department of Ecological and Biological Sciences, University of Tuscia, Viterbo, Italy
- 4Inter-University Center for Research on Cetaceans (CIRCE), Department of Earth, Environment and Life Sciences, University of Genoa, Genoa, Italy
Marine plastic pollution is one of the most concerning worldwide environmental issues, and research is day by day demonstrating its adverse effects on marine ecosystems. Nevertheless, little is still known about the toxic potential on marine fauna of chemical additives released by plastic debris. Here we investigated the cyto- and genotoxicity of the most used plasticizer in plastic production, di(2-ethylhexyl)phthalate (DEHP), on a skin cell line (TT) derived from the bottlenose dolphin (Tursiops truncatus), a species particularly exposed to the accumulation of this lipophilic pollutant, being a coastal top predator rich in fatty subcutaneous tissues. Dolphin cell cultures were exposed to increasing DEHP doses (0.01–5 mM) to evaluate effects on cell viability, cell death, and induction of DNA damage. On the hypothesis that bottlenose dolphin cells show greater resistance to DEHP toxicity than terrestrial mammals, as already shown for other pollutants, the same parameters were analyzed on exposed Chinese hamster ovary (CHO) cell lines. Both MTT and Trypan Blue assays showed no significant decrease in dolphin’s cell viability after 24-h DEHP exposure. No induction of primary DNA damage was detected by the comet assay, whereas the cytokinesis-block micronucleus assay revealed significant micronuclei induction and inhibition of cell proliferation starting from the lowest DEHP doses. DEHP had similar but sharper and significant effects on cell viability in CHO cells, also causing a much greater induction of necrosis than that recorded on dolphin cells. For both cell lines, the lack of induction of primary DNA damage (i.e., strand breaks) together with the increase of micronuclei yield after DEHP treatment suggests an aneugenic effect of the phthalate, that is, the loss of entire chromosomes during cell division. Overall, the potential chromosome loss detected could constitute a threat for species of marine mammals constantly exposed to plastic marine litter.
1 Introduction
Marine debris contaminates the world’s oceans from polar regions to the equator (Zarfl and Matthies, 2010; Jambeck and Johnsen, 2015; Rangel-Buitrago et al., 2020). This debris can be found floating on the sea surface (Campana et al., 2018), on the seafloor (Hardesty et al., 2021), and on shorelines (Galgani et al., 2015). Plastic has been produced and used in large quantities by humans in the last decades, and, to date, it represents the main source of anthropogenic debris in the oceans (Law et al., 2010), contributing for 70%–90% to the marine litter (Andrady, 2011).
A wide range of marine taxa, including birds, sea turtles, and marine mammals, are affected by entanglement and ingestion of macroplastic debris, with consequences including impaired movement, decreased feeding ability, reduced reproductive fitness, gastrointestinal lesions, ulcerations, and, in the most severe cases, also death (Moore, 2008; Gregory, 2009; Parker et al., 2021). Due to physical and chemical degradation, work of atmospheric agents, and seawater, plastic is fragmented in micro (<5 mm) and nano debris (<20 µm) (Koelmans et al., 2015). Impacts caused by microplastic debris in the oceans derive from both fragmentation (i.e., secondary microplastic) and microplastics produced as such (i.e., primary microplastic). Fragments of small size are facilitated to enter the trophic chains, becoming a serious threat to aquatic organisms inhabiting both continental and marine ecosystems (Eriksen et al., 2013; Castañeda et al., 2014; do Sul and Costa, 2014; Gambardella et al., 2018; Costa et al., 2020). Indeed, laboratory studies have shown that invertebrates such as crustaceans, barnacles, polychaetes worms, mussels, and amphipods can ingest microplastic fragments (Browne et al., 2008; Graham and Thompson, 2009; Gambardella et al., 2017), and there is increasing evidence documenting the ingestion of plastic fragments by invertebrates also in the natural environment (Boerger et al., 2010; Murray and Cowie, 2011; Macali et al., 2018).
Although plastic polymers are considered to be chemically inert, in the last years, the scientific community has focused attention on the smallest plastic debris, since they can be vectors of lipophilic pollutants collected in the sea and then absorbed by feeding organisms (Koelmans et al., 2021). Moreover, plastic can contain plasticizer additives whose release is facilitated by plastic degradation in seawater (Paluselli et al., 2019). Phthalate esters (PAEs) are widely used as plasticizers in polyvinyl chloride (PVC) and other plastic polymers formulation, such as in the manufacture of construction products, medical devices, pharmaceuticals, and personal care products (Heudorf et al., 2007). PAEs are easily leached into the aquatic environment, as they are not chemically bound to polymers (Fromme et al., 2002). Moreover, wastewaters often contain relevant concentrations of PAEs (Tran et al., 2022), which are partly transported to the sea by river runoff. Among PAEs, di(2-ethylhexyl)phthalate (DEHP) is the most widely produced and used, as well as the most persistent phthalate found in seawaters (Chaler et al., 2004; Bergé et al., 2013). Indeed, DEHP was reported to reach a high concentration in the marine environment, up to 11,500 µg/kg dw in marine sediments, 4.35 µg/L in marine water, and 1,573 µg/kg ww in marine fishes (Stewart et al., 2014; Hermabessiere et al., 2017; Zhang et al., 2018; Malem et al., 2019; Hu et al., 2020). DEHP-related adverse effects on biota have been demonstrated for many organisms. DEHP has been reported to impair development and reproductive function through activation of the estrogen receptor (ER) and peroxisome proliferator-activated receptor (PPAR) in mammals (Lyche et al., 2009; Magdouli et al., 2013). The European Scientific Committee on Toxicity, Ecotoxicity and Environment (CSTEA) indicated DEHP as an endocrine disruptor, dangerous for reproduction in both mammals and aquatic species. Other studies focused on aquatic organisms, such as shellfish, crustaceans, annelids, and fishes (Oehlmann et al., 2009; Zhang et al., 2021). DEHP showed antiestrogenic activity in female medaka (Oryzias latipes), causing retardation of oocyte development (Kim et al., 2002), and it deeply impaired fecundity, oogenesis, and embryo production in female zebrafish (Danio rerio) by affecting signals involved in oocyte maturation (Carnevali et al., 2010). Furthermore, DEHP disrupted spermatogenesis by interfering with signaling pathways in the testis and the liver of adult male zebrafish (Uren-Webster et al., 2010). Additionally, DEHP caused endocrine-disrupting effects, altering sex hormone levels in some freshwater fish species, such as the Chinese rare minnow (Gobiocypris rarus) (Wang et al., 2013), carp (Cyprinus carpio) (Thibaut and Porte, 2004), and fathead minnows (Pimephales promelas) (Crago and Klaper, 2012). Finally, recent in vitro studies demonstrated DEHP hazard, emphasizing its cytotoxic, genotoxic, and aneugenic effects on marine organisms (Hermabessiere et al., 2017; Molino et al., 2019; Zhang et al., 2021). Although marine environments are heavily prone to phthalate pollution, studies on DEHP-related adverse effects on marine organisms are still relatively scarce. In particular, marine mammals can be subjected to such an accumulation of phthalates, which some authors consider concentrations of both DEHP and its metabolite MEHP in their tissues as a measure for assessing the levels of plastic pollution in the sea (Fossi et al., 2014). Indeed, marine mammals, such as cetaceans, are top predators of large dimensions, rich in subcutaneous adipose tissues in which pollutants tend to concentrate, due to the great quantities of contaminated food they ingest and to the processes of bioaccumulation and biomagnification of lipophilic xenobiotics (Zantis et al., 2021). However, marine mammals may exhibit higher resistance to the toxic effects of xenobiotics as compared to terrestrial mammals. For example, as reported by Chen and coworkers (2012), the exposure to chromium induced lower cytotoxicity and clastogenic effect in sperm whales’ skin fibroblast with respect to human fibroblast. Similarly, Taddei and coworkers (2001) detected a greater resistance of bottlenose dolphin leukocytes to the genotoxic effect of methyl mercury chloride when compared to human cells, demonstrating a higher DNA repair efficiency of dolphin cells. These results suggest the possible development of defense strategies to contrast both dietary and environmental exposure to pollutants in marine mammals (Taddei et al., 2001; Chen et al., 2012).
Among cetaceans, the bottlenose dolphin (Tursiops truncatus) is particularly exposed to pollution by plastic and its additives, inhabiting highly contaminated coastal areas, often in the proximity of the river mouths, where primary and secondary productivity is high (Cafaro et al., 2015). Despite this, to the best of our knowledge, there are no studies evaluating the toxic potential of phthalates on cetaceans.
This study aims to test the possible cytotoxic and genotoxic effects of DEHP in a bottlenose dolphin’s skin cell line. Cells were exposed to increasing doses of DEHP for 24 h, and effects were evaluated in terms of cell viability, cell death, primary DNA damage induction, and micronucleus formation. As a term of comparison, the same parameters were analyzed on Chinese hamster ovary (CHO) cells, an established cell line widely used in genotoxicity testing. This had the dual purpose of verifying whether DEHP produced comparable effects on different cell lines and whether bottlenose dolphin cells were more resistant also to the toxic effects of phthalates than those of a terrestrial mammal.
2 Materials and methods
2.1 Chemicals
Dulbecco’s modified Eagle’s medium (DMEM), Ham’s F10 Nutrient mix (F-10), phosphate-buffered saline (PBS) without Ca2+ and Mg+, and L-glutamine were purchased from Lonza (Rome, Italy). Penicillin/streptomycin and trypsin-EDTA were purchased from EuroClone (Pero, Italy). Bis(2-ethylhexyl)phthalate (DEHP, ≥98.0% purity, CAS: 117-81-7), 3-(4,5-dimethylthiazol-2-yl)-2,5-diphenyltetrazolium bromide (MTT), cytochalasin B, Trypan Blue solution (0.4%), dimethyl sulfoxide (DMSO), and sodium dodecyl sulfate (SDS) were purchased from Sigma-Aldrich (Milan, Italy). Fetal bovine serum (FBS) was purchased from Invitrogen (Milan, Italy).
2.2 Cell lines and culture conditions
Primary cell culture of T. truncatus (TT) derived from fresh skin tissue samples was collected during the post-mortem examination of a freshly dead stranded adult bottlenose dolphin. Primary cell culture was performed following an established laboratory protocol (Peruffo et al., 2004). To immortalize the cells, the primary cell culture was transfected with pSV3neo plasmid (LGC Promochem, Teddington, UK) by using the cationic lipid Lipofectamine 2000 (Invitrogen, Carlsbad, CA, USA), following the protocol by Suman et al. (2012). Resistant cells were selected with the antibiotic G418 (400 µg/ml; Gibco, Life Technologies BRL, Gaithersburg, MD, USA).
TT cells were grown in DMEM supplemented with 10% FBS, 1% L-glutamine, and 1% penicillin/streptomycin.
The CHO cell line was grown in Ham’s F10 supplemented with 10% FBS, 1% L-glutamine, and 1% penicillin/streptomycin. This epithelial-like cell line is routinely used in mutagenicity testing as recommended by the guidelines of European Economic Community (EEC) Council 79/831 and Organisation for Economic Co-operation and Development (OECD) for the test of chemical No. 471.
Both cell lines were maintained in an incubator at 37°C, 5% CO2, and 95% humidity.
2.3 Cell culture treatments
DEHP, purchased at a concentration of 100 mM, was diluted at 5 mM with DMSO. Further dilutions were freshly made before DEHP treatments in order to have a DMSO concentration not exceeding 1% in the culture medium. DEHP treatments were performed for 24 h in TT and CHO cell lines, and DEHP was used in a range between 0.01 and 5 mM in both cell lines.
The solvent sample was treated with 1% DMSO for 24 h, while the positive control was treated with 100 µM of H2O2 for 1 h. Each experiment was repeated at least two times, and the results are displayed as the mean of two independent experiments, showing good reproducibility and comparable outcomes.
2.4 Cytotoxicity
2.4.1 Cell viability assay
To study cell viability after DEHP treatment, the MTT assay was performed in accordance with Botta and collaborators (2019), with minor modifications. Briefly, for each experimental point, cells were seeded onto 96-well microplates at a density of 5,000 cells/100 µl for TT and 2,000 cells/100 µl for CHO and incubated for 24 h to allow cell adherence. The growth medium was then replaced by a fresh medium containing DEHP and incubated for a further 24 h. At the end of treatments, MTT was added to each well (0.5 mg/ml), and cells were incubated for an additional 3 h at 37°C. After incubation, the supernatant was replaced with 100 µl of lysis solution (10% SDS and 0.6% acetic acid in DMSO) to dissolve the formazan crystals and produce a purple solution. Optical density measurements were obtained using a scanning spectrophotometer DTX 880 Multimode Detector (Beckman Coulter, Brea, CA, USA). Readings were made using 630-nm (background) and 570-nm filters.
Cell viability, presented as the relative optical density (OD) at 570, was calculated using the following formula:
2.4.2 Trypan Blue exclusion assay
For each experimental point, cells were seeded onto 35-mm Petri dishes with 2 ml of the medium at a density of 1.5 × 105 cells/dish for TT and 1 × 105 cells/dish for CHO and incubated for 24 h. Cell lines were then treated with DEHP and incubated for a further 24 h. At the end of treatments, cells were harvested, and 10 µl of cell suspension was mixed with 10 µl of Trypan Blue Solution (1:1; w:w) for 5 min to allow cell staining; cells were then seeded on a slide and counted under an optical microscope (Molino et al., 2019). For each experimental point, 250 cells were counted by two different operators. The percentage of cell viability was calculated using the following formula:
2.4.3 Detection of DEHP-induced cell death by fluorescence staining
To evaluate DEHP-induced cell death, cells were seeded onto 35-mm Petri dishes at the same density of Trypan Blue exclusion (TBE) assay, incubated for 24 h, and then treated with DEHP for a further 24 h. At the end of treatments, cells were harvested, and to distinguish apoptotic and necrotic cells from viable cells, a combination of fluorescein di-acetate (FDA, 0.75 mg/ml), propidium iodide (PI, 0.25 mg/ml), and Hoechst (HO, 0.1 mg/ml) dyes were used (Proietti De Santis et al., 2001; Filippi et al., 2022). FDA and HO are vital dyes that stain, respectively, the cytoplasm and the nucleus of viable cells; PI staining identifies the necrotic and late stage of apoptotic cells; cells in the early phase (viable HO stained) and late phase (dead PI stained) of apoptosis displayed the characteristic pattern of chromatin fragmentation. For each experimental point, 500 randomly selected cells were counted by two different operators.
2.5 Genotoxicity
2.5.1 Single-cell gel electrophoresis analysis
To quantify primary DNA damage in terms of single-strand breaks, the alkaline version of the single-cell gel electrophoresis (Comet assay) was performed. Cells were seeded onto 35-mm Petri dishes with 2 ml of the medium at the same density of TBE assay, incubated for 24 h to allow cell adherence, and then treated for 24 h with DEHP. The test procedure was performed according to previous works (Egidi et al., 2018; Molino et al., 2019). After slide preparation and cell lysis, electrophoresis was conducted for 20 min at 25 V and 300 mA at 4°C preceded by a 15-min incubation in electrophoresis buffer to allow DNA unwinding. Slides were neutralized and stained with ethidium bromide (20 µg/ml, 50 µl), and nucleoids were analyzed at ×400 magnification with an automatic image analyzer (Comet Assay III, Perceptive Instruments, St Edmunds, UK) connected to a fluorescence microscope (Axioskop 2, Zeiss, Oberkochen, Germany). To evaluate the amount of DNA damage, computer-generated % DNA in the tail (tail intensity (TI)) values were used. For each experimental point, a total of 200 randomly selected cells were scored by two different operators.
2.5.2 Cytokinesis-block micronucleus assay
The cytokinesis-block micronucleus (CBMN) assay was carried out with the standard technique proposed by Fenech (1993), with minor adjustments (Meschini et al., 2018). For each experimental point, cells were seeded onto 60-mm Petri dishes with 3 ml of the medium at a density respectively of 2.5 × 105 cells/dish for TT and 3 × 105 cells/dish for CHO, incubated for 24 h, and then treated with DEHP for 24 h. At the end of DEHP treatment, cells were washed with PBS, and a fresh medium containing 6 µg/ml of cytochalasin B was added for a further 24 h in order to arrest cytokinesis. For each experimental point, 1,000 binucleated cells with intact cytoplasm were scored by three different operators for the presence of micronuclei (MN), a biomarker of chromosome breakage or loss. For the analysis of cell cycle progression, 1,000 cells for each sample were scored for the presence of one, two, or more than two nuclei.
Cytokinesis-block proliferation index (CBPI) was calculated using the following formula: (1N means cells with 1 nucleus; 2N, cells with 2 nuclei; and >2N, cells with more than 2 nuclei).
The percentage of cytostasis was calculated with the following formula: ,where t and c are treated and control samples, respectively (Lorge et al., 2008).
2.6 Statistical analysis
For viability tests (MTT, TBE, and cell death assays), statistical significance of raw data between treated samples and their relative solvent in each cell line was evaluated using one-way ANOVA followed by Sidak’s or Tukey’s multiple comparisons post-test. Data belonging to different experiments were represented and averaged in the same graph. The GraphPad Prism software package (GraphPad Software, San Diego, CA, USA) was used. Results were expressed as means ± SD. For the cytostatic effect (CBMN assay), the chi-squared test (χ2) was used to compare treated samples and their relative solvent for each cell line. For the Comet assay and the yield of micronuclei per cell, Student’s t-test was applied. For the comparison of cytotoxicity between the two cell lines, the data were analyzed with the Kruskal–Wallis test (KW). For pairwise comparisons (apoptosis, necrosis, and CBPI), the data were analyzed with the Wilcoxon test (WT). For comparisons, data were normalized with respect to the relative solvent, and the results of medium, positive, and solvent control were excluded. All analyses for the comparison of the two cell lines were performed with R. The levels for statistical significance were set at p ≤ 0.05 and p ≤ 0.01.
3 Results
3.1 Cytotoxicity
3.1.1 MTT assay
Figure 1A shows the results of cell viability measured by MTT assay in TT and CHO cell lines after 24 h of treatment with DEHP. The analysis revealed no significant effects of the solvent, whereas H2O2 (100 µM) caused a significant decrease in cell viability of about 70% in both cell lines (p ≤ 0.01). After DEHP treatments, a reduction in cell viability was observed in both cell lines. Only for the CHO cells the reduction was statistically significant (p ≤ 0.05; p ≤ 0.01), with respect to the solvent, starting at 0.02 mM. Conversely, in the TT cell line, no significant effect on the decrease of cell viability was detected. In both cell lines, the reduction reached a plateau at the three highest doses.
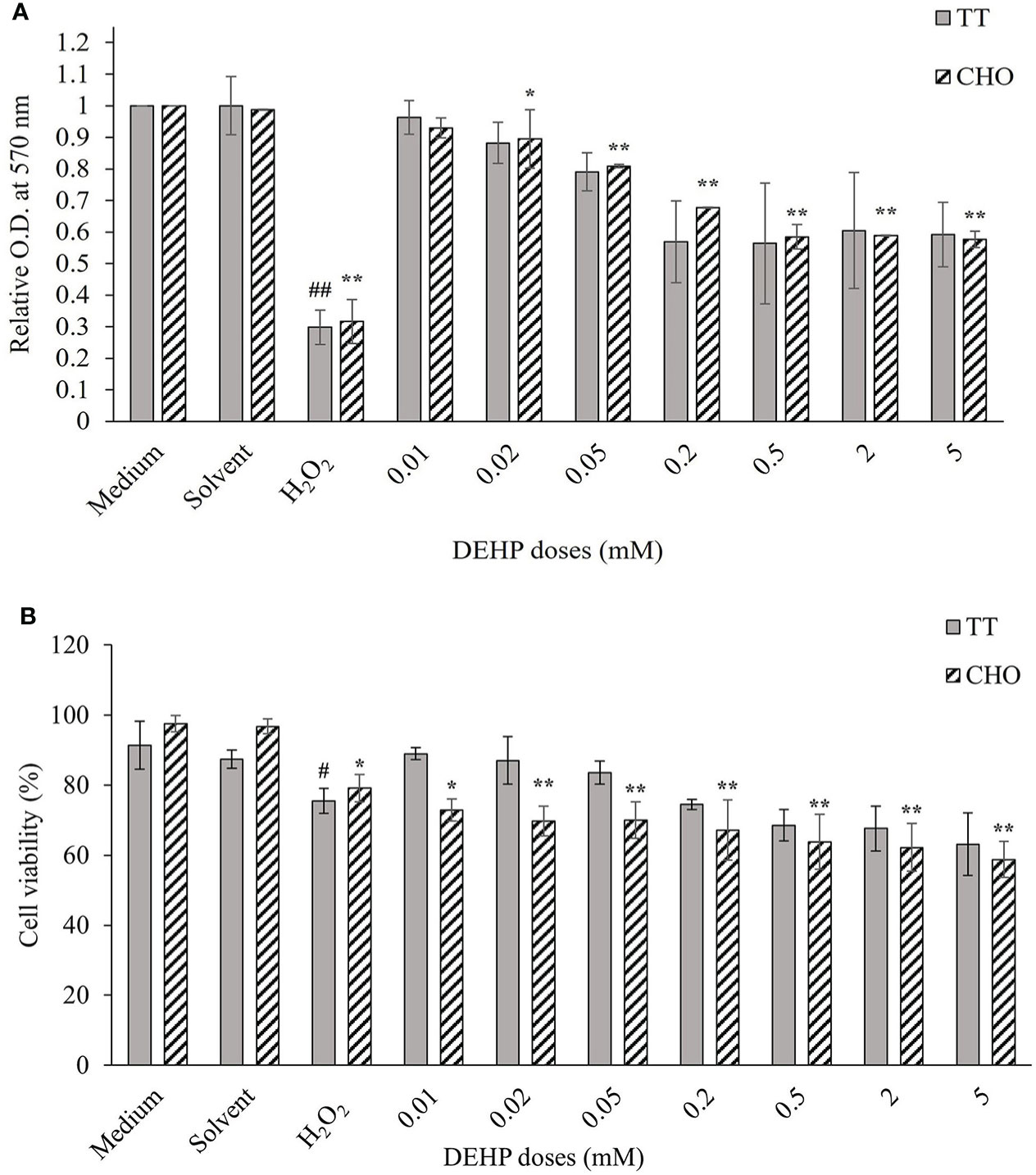
Figure 1 Cellular viability in DEHP-exposed TT and CHO cell lines. (A) MTT assay results are displayed as a mean of the optical density (OD) at 570 nm, at each treatment level normalized to the relative solvent (DMSO) in each cell line. Data are expressed as mean ± SD of two independent experiments. (B) Trypan Blue exclusion assay results are expressed as the percentage of viable cells out of the total cells at each treatment level. Data are expressed as mean ± SD of two independent experiments. One-way ANOVA significance: #p ≤ 0.05 H2O2 vs medium in TT cell line; ##p ≤ 0.01 H2O2 vs medium in TT cell line; *p ≤ 0.05 H2O2 vs medium and treated vs solvent in CHO cell line; **p ≤ 0.01 H2O2 vs medium and treated vs solvent in CHO cell line. DEHP, di(2-ethylhexyl)phthalate; TT, Tursiops truncatus; CHO, Chinese hamster ovary; DMSO, dimethyl sulfoxide.
3.1.2 Trypan Blue exclusion assay
Figure 1B shows the results of cell viability measured by TBE assay in TT and CHO cell lines, after 24 h of treatment with increasing DEHP concentrations. The solvent did not affect cell viability in both cell lines, whereas the treatment with H2O2 (100 µM) caused a decrease in cell viability, which was 24.5% and 20.9% in TT and CHO, respectively. DEHP treatments caused a dose-dependent reduction of cell viability in both cell lines but of a greater and statistically significant (p ≤ 0.05; p ≤ 0.01) magnitude only for the CHO cell line.
3.1.3 Detection of DEHP-induced cell death by fluorescence staining
Results obtained by fluorescence staining are shown in Figures 2A, B for TT and CHO cell lines, respectively. TT cells showed about 10% of dead cells in both control and solvent samples, mostly due to necrosis (8.7% and 7.9%, respectively), whereas total cell mortality of CHO cells resulted in approximately 1% and 2% for the two aforementioned samples. In both cell lines, the positive control (H2O2, 100 µM) showed a significant increase in necrotic cells, with respect to the control (p ≤ 0.05 for both TT and CHO cells). After 24 h of DEHP treatments, the TT cell line displayed no significant increases in either apoptosis or necrosis in treated cells with respect to the solvent. Conversely, the CHO cell line showed a significant increase of necrotic cells at all DEHP concentrations (p ≤ 0.05; p ≤ 0.01), which appears to be dose-dependent at the four higher concentrations. No increases in apoptosis occurred at any DEHP concentration.
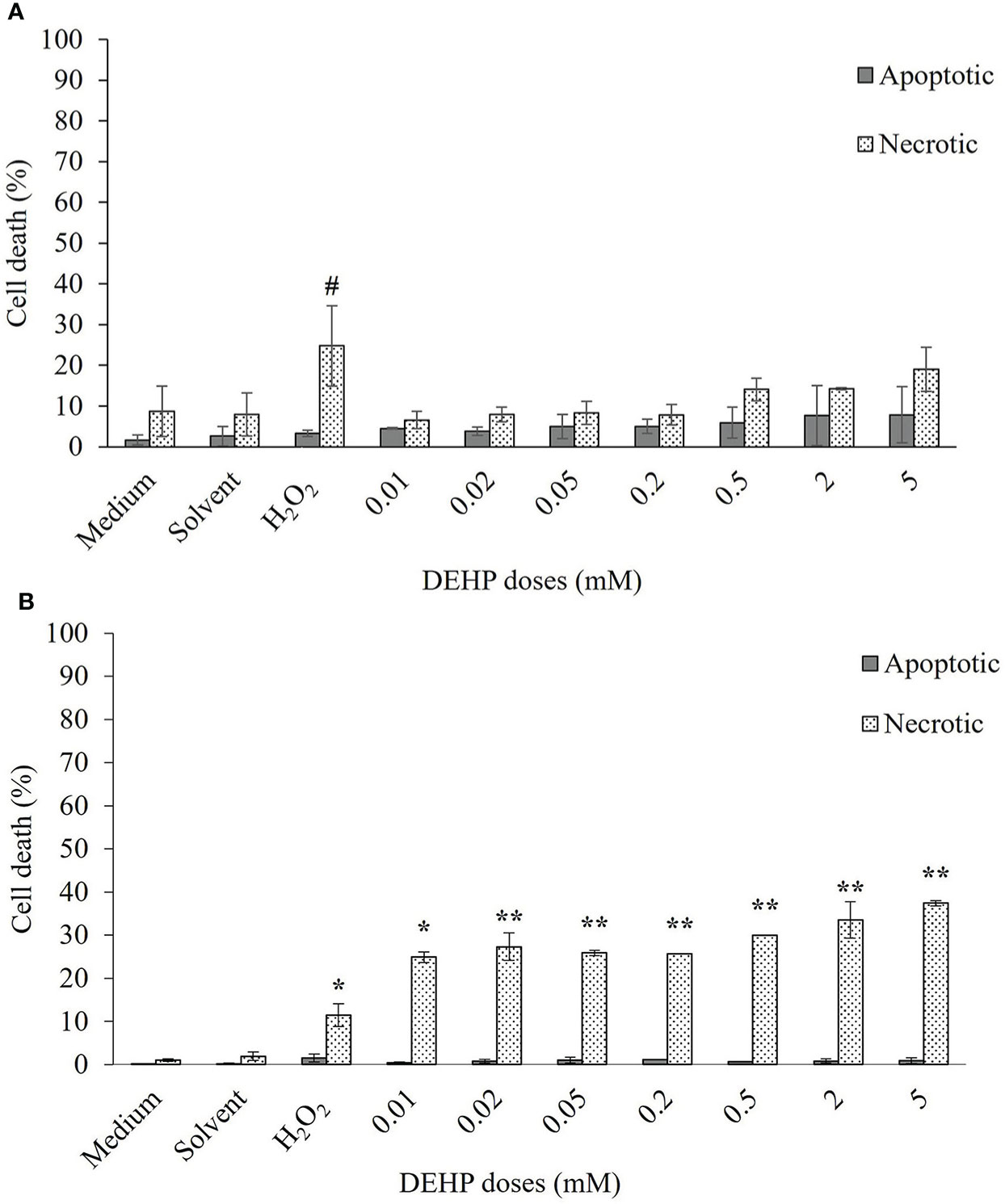
Figure 2 Induction of apoptosis and necrosis in TT (A) and CHO (B) cell lines after 24 h of exposure at increasing doses of DEHP. Data are expressed as means ± SD of two independent experiments. One-way ANOVA significance: # p ≤ 0.05 H2O2 vs medium in TT cell line; * p ≤ 0.05 H2O2 vs medium and treated vs solvent in CHO cell line; ** p ≤ 0.01 treated vs solvent in CHO cell line. TT, Tursiops truncatus; CHO, Chinese hamster ovary; DEHP, di(2-ethylhexyl)phthalate.
3.2 Genotoxicity
3.2.1 Comet assay
Comet assay results are shown in Table 1. In both TT and CHO cell lines, control cells showed a mean TI of 6.70 and 5.76, respectively. In both cell lines, treatment with solvent did not increase TI with respect to the control, while treatment with H2O2 (100 µM) caused a significant increase in both cell lines (p ≤ 0.01), reaching 12.27 and 11.95 in TT and CHO cell lines, respectively. Conversely, DEHP treatments did not exert any increase of primary DNA damage with respect to the solvent, neither in the TT nor in the CHO cell line.

Table 1 Tail intensity (%) values obtained through the Comet assay in TT and CHO cell lines treated for 24 h with DEHP.
3.2.2 Cytokinesis-block micronucleus assay
Table 2 shows the results obtained performing the CBMN assay in TT and CHO cell lines after 24 h of DEHP treatments. In both cell lines, no differences in either MN frequencies or CBPI values emerged in the solvent samples, when compared to controls. Treatment with H2O2 (100 µM) caused significant increases in MN frequencies (p ≤ 0.01) and significant decreases in CBPI values (p ≤ 0.01) in both cell lines, coupled with an increase in the percentage of cytostasis.
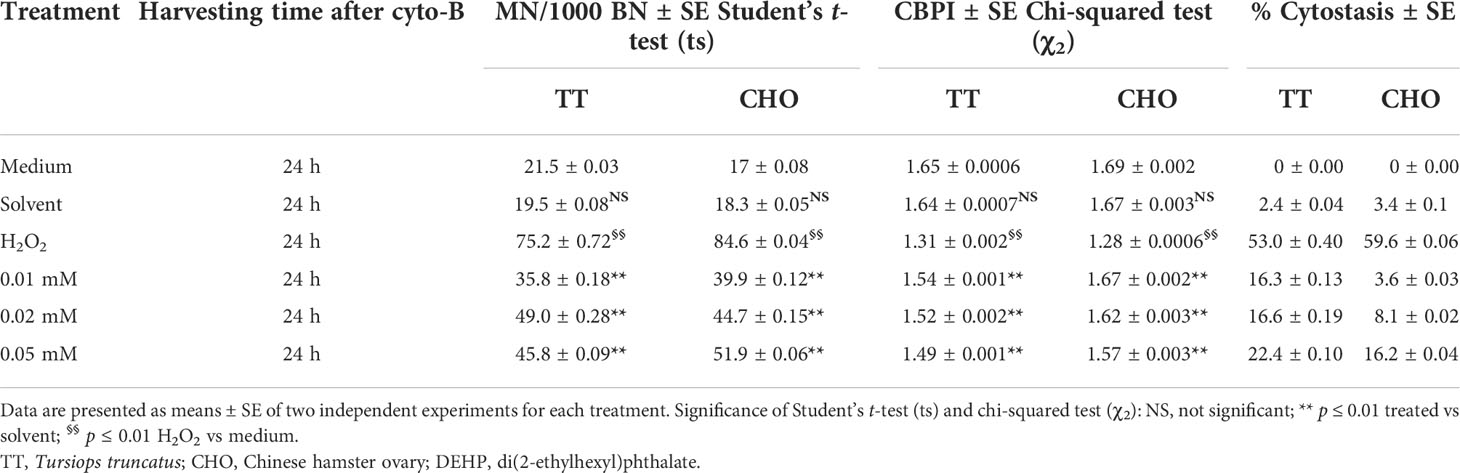
Table 2 Induction of micronuclei (MN), cytokinesis-block proliferation index (CBPI), and % of cytostasis in TT and CHO cell lines treated for 24 h with DEHP.
After DEHP treatments, in the TT cell line, the CBMN assay revealed a statistically significant increase in the frequencies of MN at all DEHP concentrations (p ≤ 0.01), when compared to the solvent. Moreover, a plateau trending increase in the yield of MN was observed, as well as a dose-dependent decrease of CBPI values and a statistically significant (p ≤ 0.01) increase in the percentage of cytostasis with respect to the solvent.
In the CHO cell line, DEHP treatments induced a statistically significant (p ≤ 0.01) and dose-dependent increase in the frequency of MN when compared with the solvent. A dose-dependent decrease of CBPI values and a statistically significant increase in the percentage of cytostasis at all DEHP concentrations (p ≤ 0.01) with respect to the solvent were observed.
3.2.3 Statistical comparison of cytotoxic effects in the two cell lines
The comparison of the cumulative cytotoxic effect (MTT + TBE) detected in the two cell lines highlighted the greater effect exerted by DEHP on the CHO cell line in terms of cell viability reduction and cell death induction, which resulted in statistical significance (KW p ≤ 0.01) when compared to TT cells. Nevertheless, the higher CHO mortality was due to a significantly higher necrosis induction (WT p ≤ 0.01), whereas the TT cell line still overwhelmed the CHO cell line in terms of induced apoptosis (WT p ≤ 0.01). Lastly, the comparison of the inhibition of cell proliferation (CBPI) showed a higher effect exerted by DEHP on the TT cell line (WT p ≤ 0.05).
4 Discussion
In the last few years, plastic pollution has become a global problem. DEHP, a plasticizer used in various plastic products, is one of the most common PAEs detected in the environment and especially in the marine environment, where it derives mainly from rivers’ input and chemical and physical degradation of plastic litter (Selvaraj et al., 2015; Paluselli et al., 2018; Zhang et al., 2021). DEHP causes immunotoxicity, metabolic toxicity, neurotoxicity, and endocrine toxicity in both terrestrial mammals (Chang et al., 2017; Radke et al., 2020; Weaver et al., 2020) and aquatic organisms (Molino et al., 2019; Yu et al., 2019; Xu et al., 2021; Zhang et al., 2021). In the current study, results of in vitro exposure to DEHP on TT and CHO cell lines highlighted cytotoxic and genotoxic effects, confirming that this phthalate constitutes an important threat to both marine and terrestrial mammals.
Both cytotoxicity assays revealed an effect of DEHP treatments in both cell lines, which resulted in statistical significance only in CHO cells. Therefore, both tests highlighted a higher sensitivity in terms of DEHP cytotoxicity on the CHO cell line; results were also supported by the analysis of cell death. It is worth noting that the differences that occurred between the two cell lines were likely due to a different sensitivity to DEHP, given that treatments with H2O2 caused nearly the same detrimental effect in terms of both cell viability and cell death in TT and CHO lines. Indeed, hydrogen peroxide can have immediate cellular effects when diffusing through cells and tissues (Sies, 2017). Similarly, previous studies have shown that DEHP has direct cytotoxic and genotoxic effects on several eukaryotic cell lines (Caldwell, 2012; Peropadre et al., 2013; Erkekoglu and Kocer-Gumusel, 2014; Pournejati et al., 2021). DEHP is rather stable in the aqueous phase, degrading under the specific condition of UV radiation intensity, pH, and temperature (Chen, 2010; Huang et al., 2017). Therefore, considering the purity of the DEHP tested (≥98%) and that the experiments were carried out in stable environmental conditions, our results could be explained by a direct effect of DEHP on both TT and CHO cell lines with a greater sensitivity of the latter. Cytotoxicity results are in agreement with previous studies, which demonstrated the cytotoxic effect of DEHP on both fishes (Zheng et al., 2013; Molino et al., 2019; Wang et al., 2020) and mammals, including humans (Eljezi et al., 2017; Eljezi et al., 2019; Weaver et al., 2020; Radke et al., 2020; Zhang et al., 2021). Furthermore, it has been observed a greater resistance of marine mammal cells to the cytotoxic effect of persistent pollutants, which might be due to adaptations and the development of more efficient detoxification mechanisms (Chen et al., 2009; Chen et al., 2012; Gui et al., 2014). In this context, the results obtained in vitro in this study also suggest a higher resistance to the effect of DEHP treatment on the fibroblasts of the bottlenose dolphin cell line compared to the CHO epithelial-like cells.
As for genotoxicity, in both TT and CHO cells, a clear genotoxic effect of DEHP was observed, as revealed by micronuclei induction. The Comet assay, carried out to verify the potential clastogenic effect of DEHP, detected no effect in either cell line, whereas the positive control with H2O2 always induced a statistically significant increase in DNA strand breaks. Conversely, in both cell lines, the CBMN assay showed an effect of DEHP with an increase in the frequency of micronuclei coupled with an increment of cytostasis and a dose-dependent decrease in cell proliferation. Moreover, when compared with CHO cells, the TT cell line displayed a higher cytostatic effect of DEHP. This affects the expression of chromosomal damage in terms of micronuclei that are dose-dependent in the CHO cell line while reaching a plateau in TT cells. Thus, the higher cytostasis detected in TT probably did not allow to fully detect cytogenetic damage induced by DEHP on the TT cell line. Performing both the Comet assay and the CBMN assay allows to detect with high sensitivity clastogenic and aneugenic substances (Araldi et al., 2015); therefore, their comparison suggests that micronuclei formation after DEHP exposure was not due to chromosome breakage but potentially by the loss of the entire chromosomes, due to an aneugenic effect of DEHP. This result indicates that DEHP may represent a greater risk for the bottlenose dolphin.
Previous genotoxic investigation of DEHP effects in mammals (Caldwell, 2012; Erkekoglu and Kocer-Gumusel, 2014) and fishes (Khalil et al., 2016; Ma et al., 2018; Molino et al., 2019) demonstrated both an increase in DNA strand breaks and an induction of micronuclei. The comparison with other data on aquatic organisms, like the European seabass (Molino et al., 2019) and the zebrafish (Chen et al., 2014), emphasizes a higher sensitivity of teleosts when compared to the bottlenose dolphin. Higher resistance of marine mammals, compared to other aquatic organisms, has already been suggested for other xenobiotics (e.g., PCB and heavy metals), which has been ascribed as an adaptation in response to high levels of contamination to which marine mammals are subjected (Chen et al., 2009; Desforges et al., 2016). With regard to PAEs, it has been shown that their concentration in the body of high-trophic-level organisms is often lower than that of organisms at the lower trophic levels (Sun et al., 2016; Zhang et al., 2021). This suggests a higher metabolic capacity of high-trophic-level organisms to produce PAE metabolites, which usually results in less toxicity than their parent compounds (Ye et al., 2014; Zhang et al., 2021).
The concentration of anthropogenic xenobiotics in marine mammals’ blubber can be used as an indicator of the sea’s contamination levels since marine mammals play an important role at the top of the food webs (Bossart, 2011; Desforges et al., 2016). However, due to obvious ethical and conservation reasons, there is severe legislation aimed at protecting marine mammals (Habitat Directive, 1992; ACCOBAMS, 2001; Marine Strategy Framework Directive, 2008), which prevents in vivo studies on these organisms. Thus, in vitro experiments afford the opportunity to evaluate marine mammals’ cellular response to xenobiotics and to make hypotheses regarding ecotoxicology hazards to wild organisms. The present work pays attention to one of the main and, to date, still poorly investigated threats of marine plastic litter: the ecotoxicological risk of plastic additives and its potential threat to marine mammals. Our approach permitted the detection of the cytotoxic and genotoxic effects caused by DEHP exposure, showing its effects on both cellular viability and DNA integrity in T. truncatus. Therefore, DEHP might be considered an additional stressor to the multiple threats that act synergistically and undermine marine mammals’ conservation. It is also relevant to pay attention to the potential chromosome loss detected since it is considered a mutation, which could be particularly insidious for the species and the conservation of genetic patterns (Fenech, 2008; Fan et al., 2019). Indeed, genetic damage can potentially extend from the individual to the population (Fan et al., 2019). Moreover, a link between marine mammal death and chemical pollution in the sea cannot be excluded, as in the past epizootic events (e.g., Cetacean morbillivirus) were connected to immunosuppression caused by high levels of contamination in the organism (Beineke et al., 2005; Mori et al., 2008; Beineke et al., 2010).
In conclusion, this study showed relevant cytotoxic and genotoxic effects of DEHP on both TT and CHO cell lines, occurring mainly as cell death, inhibition of cell proliferation, and induction of micronuclei. Moreover, data indicated also a different effect of DEHP treatment on the two cell lines such as a higher cytostasis on TT cells and stronger cytotoxicity on the CHO cell line as well as a greater resistance of the former to the toxic effects of phthalates. Although relatively high, the DEHP concentrations applied in the present study are similar to those recently found in T. truncatus blubber samples of 26,068 ng/g (about 0.07 mM), which is in the lower range of our treatments (Baini et al., 2017). Therefore, the current study underlines the importance of learning more about DEHP’s potential threat to the bottlenose dolphin and possibly other marine mammals, which are constantly exposed to plastic marine litter. In this respect, ex vivo studies could represent an additional approach to further assess the effects of DEHP.
Data availability statement
The datasets presented in this study can be found in online repositories. The names of the repository/repositories and accession number(s) can be found below: http://hdl.handle.net/2067/43634 - University of Tuscia Archive of research doctorate thesis.
Author contributions
GG contributed by conducting both cytotoxicity and genotoxicity assays, drawing figures and tables, performing data analysis, statistical analysis with the R software, and writing the first draft of the manuscript. SF contributed to designing the experimental work, collaborating in both cytotoxicity and genotoxicity assays, performing data analysis, and revising the final draft of the manuscript. CM contributed by conducting both cytotoxicity and genotoxicity assays, drawing figures and tables, performing data analysis, and revising the first and the final draft of the manuscript. AP and CC contributed by kindly providing the T. truncatus cell line and revising the final draft of the manuscript. RM contributed to the conception and design of the work, interpretation of the data, and revising the final draft of the manuscript. DA contributed to the conception and design of the work, interpretation of the data, and writing the first draft of the manuscript. All the authors approved the final version of the manuscript to be published.
Acknowledgments
During the time that this manuscript was being prepared for publication, our colleague DA unexpectedly passed away. The scientific community lost an excellent scientist, but mostly we lost a very good friend and a wise and talented colleague. DA was very fond of this work and was looking forward to its publication; therefore, we wish to dedicate this paper to his memory. We would like to thank the Mediterranean Marine Mammal Tissue Bank (Department of Comparative Biomedicine and Food Science, University of Padua, Viale dell’Università 16, 35020 Legnaro - Agripolis PD, Italy) for providing us with the T. truncatus cell line. We express our thanks to our colleague Prof. Claudio Carere for kindly revising the manuscript. Lastly, we wish to thank Profs. Daniele Canestrelli and Claudio Carere without whom the publication of this paper would not have been possible.
Conflict of interest
The authors declare that the research was conducted in the absence of any commercial or financial relationships that could be construed as a potential conflict of interest.
Publisher’s note
All claims expressed in this article are solely those of the authors and do not necessarily represent those of their affiliated organizations, or those of the publisher, the editors and the reviewers. Any product that may be evaluated in this article, or claim that may be made by its manufacturer, is not guaranteed or endorsed by the publisher.
References
Peropadre A., Freire P. F., Herrero O., Martín J. P., Hazen M. J. (2013). Cytotoxic effects of di (2-ethylhexyl) phthalate on cultured mammalian cells. Toxicology 9, 35–42.
Peruffo A, Massimino M.L., Ballarin C., Carmignoto G., Rota A., Cozzi B. (2004). Primary cultures from fetal bovine brain. Neuroreport 15 (11), 1719–1722. doi: 10.1097/01.wnr.0000136036.15977.3a
ACCOBAMS (2001). The agreement on the conservation of cetaceans of the black Sea, Mediterranean Sea and contiguous Atlantic area. Available at: http://www.accobams.org.
Andrady A. L. (2011). Microplastics in the marine environment. Mar. pollut. Bull. 62 (8), 1596–1605. doi: 10.1016/j.marpolbul.2011.05.030
Araldi R. P., de Melo T. C., Mendes T. B., de Sá Júnior P. L., Nozima B. H. N., Ito E. T., et al. (2015). Using the comet and micronucleus assays for genotoxicity studies: A review. BioMed. Pharmacother. 72, 74–82. doi: 10.1016/j.biopha.2015.04.004
Baini M., Martellini T., Cincinelli A., Campani T., Minutoli R., Panti C., et al. (2017). First detection of seven phthalate esters (PAEs) as plastic tracers in superficial neustonic/planktonic samples and cetacean blubber. Anal. Methods 9 (9), 1512–1520. doi: 10.1039/C6AY02674E
Beineke A., Siebert U., McLachlan M., Bruhn R., Thron K., Failing K., et al. (2005). Investigations of the potential influence of environmental contaminants on the thymus and spleen of harbor porpoises (Phocoena phocoena). Environ. Sci. Technol. 39 (11), 3933–3938. doi: 10.1021/es048709j
Beineke A., Siebert U., Wohlsein P., Baumgärtner W. (2010). Immunology of whales and dolphins. Vet. Immunol. Immunop. 133 (2-4), 81–94. doi: 10.1016/j.vetimm.2009.06.019
Bergé A., Cladière M., Gasperi J., Coursimault A., Tassin B., Moilleron R. (2013). Meta-analysis of environmental contamination by phthalates. Environ. Sci. pollut. R. 20 (11), 8057–8076. doi: 10.1007/s11356-013-1982-5
Boerger C. M., Lattin G. L., Moore S. L., Moore C. J. (2010). Plastic ingestion by planktivorous fishes in the north pacific central gyre. Mar. pollut. Bull. 60 (12), 2275–2278. doi: 10.1016/j.marpolbul.2010.08.007
Bossart G. D. (2011). Marine mammals as sentinel species for oceans and human health. Vet. Pathol. 48 (3), 676–690. doi: 10.1177/0300985810388525
Botta L., Filippi S., Bizzarri B. M., Meschini R., Caputo M., Proietti-De-Santis L., et al. (2019). Oxidative nucleophilic substitution selectively produces cambinol derivatives with antiproliferative activity on bladder cancer cell lines. Bioorg. Med. Chem. Lett. 29 (1), 78–82. doi: 10.1016/j.bmcl.2018.11.006
Browne M. A., Dissanayake A., Galloway T. S., Lowe D. M., Thompson R. C. (2008). Ingested microscopic plastic translocates to the circulatory system of the mussel, Mytilus edulis (L.). Environ. Sci. Technol. 42 (13), 5026–5031. doi: 10.1021/es800249a
Cafaro V., Angeletti D., Bellisario B., Macali A., Carere C., Alessi J. (2015). Habitat overlap between bottlenose dolphins and seabirds: a pilot study to identify high-presence coastal areas in the tyrrhenian Sea. J. Mar. Biol. Assoc. UK. 96 (4), 891–901. doi: 10.1017/S0025315415001447
Caldwell J. C. (2012). DEHP: Genotoxicity and potential carcinogenic mechanisms - a review. Mut. Res-Rev. Mutat. 751 (2), 82–157. doi: 10.1016/j.mrrev.2012.03.001
Campana I., Angeletti D., Crosti R., Di Miccoli V., Arcangeli A. (2018). Seasonal patterns of floating macro-litter across the Western Mediterranean Sea: a potential threat for cetacean species. Rend. Lincei-Sci. Fis. 29 (2), 453–467. doi: 10.1007/s12210-018-0680-0
Carnevali O., Tosti L., Speciale C., Peng C., Zhu Y., Maradonna F. (2010). DEHP impairs zebrafish reproduction by affecting critical factors in oogenesis. PloS One 5 (4), e10201. doi: 10.1371/journal.pone.0010201
Castañeda R. A., Avlijas S., Simard M. A., Ricciardi A. (2014). Microplastic pollution in st. Lawrence river sediments. Can. J. Fish. Aquat. Sci. 71 (12), 1767–1771. doi: 10.1139/cjfas-2014-0281
Chaler R., Cantón L., Vaquero M., Grimalt J. O. (2004). Identification and quantification of n-octyl esters of alkanoic and hexanedioic acids and phthalates as urban wastewater markers in biota and sediments from estuarine areas. J. Chromatogr. A. 1046 (1-2), 203–210. doi: 10.1016/j.chroma.2004.06.098
Chang Y. J., Tseng C. Y., Lin P. Y., Chuang Y. C., Chao M. W. (2017). Acute exposure to DEHP metabolite, MEHP cause genotoxicity, mutagenesis and carcinogenicity in mammalian Chinese hamster ovary cells. Carcinogenesis 38 (3), 336–345. doi: 10.1093/carcin/bgx009
Chen C. Y. (2010). The oxidation of di-(2-Ethylhexyl) phthalate (DEHP) in aqueous solution by UV/H2O2 photolysis. Water Air. Soil pollut. 209, 411–417. doi: 10.1007/s11270-009-0209-3
Chen T. L., LaCerte C., Wise S. S., Holmes A., Martino J., Wise J. P. Jr., et al. (2012). Comparative cytotoxicity and genotoxicity of particulate and soluble hexavalent chromium in human and sperm whale (Physeter macrocephalus) skin cells. Comp. Biochem. Phys. C. 155 (1), 143–150. doi: 10.1016/j.cbpc.2011.03.011
Chen T. L., Wise S. S., Kraus S., Shaffiey F., Levine K. M., Thompson W. D., et al. (2009). Particulate hexavalent chromium is cytotoxic and genotoxic to the north Atlantic right whale (Eubalaena glacialis) lung and skin fibroblasts. Environ. Mol. Mutagen. 50 (5), 387–393. doi: 10.1002/em.20471
Chen X., Xu S., Tan T., Lee S. T., Cheng S. H., Lee F. W. F., et al. (2014). Toxicity and estrogenic endocrine disrupting activity of phthalates and their mixtures. Int. J. Env. Res. Pub. He. 11 (3), 3156–3168. doi: 10.3390/ijerph110303156
Costa E., Piazza V., Lavorano S., Faimali M., Garaventa F., Gambardella C. (2020). Trophic transfer of microplastics from copepods to jellyfish in the marine environment. Front. Environ. Sci. 158. doi: 10.3389/fenvs.2020.571732
Council Directive 92/43/EEC (1992). Conservation of natural habitats and of wild fauna and flora. Available at: http://data.europa.eu/eli/dir/1992/43/oj.
Crago J., Klaper R. (2012). A mixture of an environmentally realistic concentration of a phthalate and herbicide reduces testosterone in male fathead minnow (Pimephales promelas) through a novel mechanism of action. Aquat. Toxicol. 110, 74–83. doi: 10.1016/j.aquatox.2011.12.021
De Santis L. P., Garcia C. L., Balajee A. S., Calvo G. T. B., Bassi L., Palitti F. (2001). Transcription coupled repair deficiency results in increased chromosomal aberrations and apoptotic death in the UV61 cell line, the Chinese hamster homologue of cockayne’s syndrome b. Mutat. Res-DNA. Repair 485 (2), 121–132. doi: 10.1016/S0921-8777(00)00065-3
Desforges J. P. W., Sonne C., Levin M., Siebert U., De Guise S., Dietz R. (2016). Immunotoxic effects of environmental pollutants in marine mammals. Environ. Int. 86, 126–139. doi: 10.1016/j.envint.2015.10.007
Directive 2008/56/EC (2008). The European parliament and the council establishing a framework for community action in the field of marine environmental policy (Marine Strategy Framework Directive). Available at: http://data.europa.eu/eli/dir/2008/56/oj.
do Sul J. A. I., Costa M. F. (2014). The present and future of microplastic pollution in the marine environment. Environ. pollut. 185, 352–364. doi: 10.1016/j.envpol.2013.10.036
Egidi A., Filippi S., Manganello F., Lopez-Martinez W., Meschini R. (2018). Modulation of chromatin conformation by the histone deacetylase inhibitor trichostatin. a promotes the removal of radiation-induced lesions in ataxia telangiectasia cell lines. Mutat. Res-Gen. Tox. En. Mutagen. 836, 109–116. doi: 10.1016/j.mrgentox.2018.06.016
Eljezi T., Pinta P., Nativel F., Richard D., Pinguet J., Roy O., et al. (2019). In vitro cytotoxic effects of secondary metabolites of DEHP and its alternative plasticizers DINCH and DINP on a L929 cell line. Int. J. Hyg. Envir. Heal. 222 (3), 583–589. doi: 10.1016/j.ijheh.2019.03.005
Eljezi T., Pinta P., Richard D., Pinguet J., Chezal J. M., Chagnon M. C., et al. (2017). In vitro cytotoxic effects of DEHP-alternative plasticizers and their primary metabolites on a L929 cell line. Chemosphere 173, 452–459. doi: 10.1016/j.chemosphere.2017.01.026
Eriksen M., Mason S., Wilson S., Box C., Zellers A., Edwards W., et al. (2013). Microplastic pollution in the surface waters of the laurentian great lakes. Mar. pollut. Bull. 77 (1-2), 177–182. doi: 10.1016/j.marpolbul.2013.10.007
Erkekoglu P., Kocer-Gumusel B. (2014). Genotoxicity of phthalates. Toxicol. Mech. Method. 24 (9), 616–626. doi: 10.3109/15376516.2014.960987
Fan G., Zhang Y., Liu X., Wang J., Sun Z., Sun S., et al. (2019). The first chromosome-level genome for a marine mammal as a resource to study ecology and evolution. Mol. Ecol. Res. 19 (4), 944–956. doi: 10.1111/1755-0998.13003
Fenech M. (1993). The cytokinesis-block micronucleus technique: a detailed description of the method and its application to genotoxicity studies in human populations. Mutat. Res-Fund. Mol. M. 285, 35–44. doi: 10.1016/0027-5107(93)90049-L
Fenech M. (2008). The micronucleus assay determination of chromosomal level DNA damage. Methods Mol. Biol. 410, 185–216. doi: 10.1007/978-1-59745-548-0_12
Filippi S., Paccosi E., Balzerano A., Ferretti M., Poli G., Taborri J., et al. (2022). CSA Antisense targeting enhances anticancer drug sensitivity in breast cancer cells, including the triple-negative subtype. Cancers 14 (7), 1687. doi: 10.3390/cancers14071687
Fossi M. C., Coppola D., Baini M., Giannetti M., Guerranti C., Marsili L., et al. (2014). Large Filter feeding marine organisms as indicators of microplastic in the pelagic environment: The case studies of the Mediterranean basking shark (Cetorhinus maximus) and fin whale (Balaenoptera physalus). Mar. Environ. Res. 100, 17–24. doi: 10.1016/j.marenvres.2014.02.002
Fromme H., Küchler T., Otto T., Pilz K., Müller J., Wenzel A. (2002). Occurrence of phthalates and bisphenol a and f in the environment. Water Res. 36 (6), 1429–1438. doi: 10.1016/S0043-1354(01)00367-0
Galgani F., Hanke G., Maes T. (2015). “Global distribution, composition and abundance of marine litter,” in Marine anthropogenic litter. Eds. Bergmann M., Gutow L., Klages M. (Cham: Springer), 29–56. doi: 10.1007/978-3-319-16510-3_2
Gambardella C., Morgana S., Bramini M., Rotini A., Manfra L., Migliore L., et al. (2018). Ecotoxicological effects of polystyrene microbeads in a battery of marine organisms belonging to different trophic levels. Mar. Environ. Res. 141, 313–321. doi: 10.1016/j.marenvres.2018.09.023
Gambardella C., Morgana S., Ferrando S., Bramini M., Piazza V., Costa E., et al. (2017). Effects of polystyrene microbeads in marine planktonic crustaceans. Eco. Environ. Safe. 145, 250–257. doi: 10.1016/j.ecoenv.2017.07.036
Graham E. R., Thompson J. T. (2009). Deposit-and suspension-feeding sea cucumbers (Echinodermata) ingest plastic fragments. J. Exp. Mar. Biol. Ecol. 368 (1), 22–29. doi: 10.1016/j.jembe.2008.09.007
Gregory M. R. (2009). Environmental implications of plastic debris in marine settings–entanglement, ingestion, smothering, hangers-on, hitch-hiking and alien invasions. Philos. T. Roy. Soc. B. 364 (1526), 2013–2025. doi: 10.1098/rstb.2008.0265
Gui D., Yu R., He X., Tu Q., Chen L., Wu Y. (2014). Bioaccumulation and biomagnification of persistent organic pollutants in indo-pacific humpback dolphins (Sousa chinensis) from the pearl river estuary, China. Chemosphere 114, 106–113. doi: 10.1016/j.chemosphere.2014.04.028
Hardesty B. D., Roman L., Leonard G. H., Mallos N., Pragnell-Raasch H., Campbell I., et al. (2021). Socioeconomics effects on global hotspots of common debris items on land and the seafloor. Global Environ. Chang. 71, 102360. doi: 10.1016/j.gloenvcha.2021.102360
Hermabessiere L., Dehaut A., Paul-Pont I., Lacroix C., Jezequel R., Soudant P., et al. (2017). Occurrence and effects of plastic additives on marine environments and organisms: a review. Chemosphere 182, 781–793. doi: 10.1016/j.chemosphere.2017.05.096
Heudorf U., Mersch-Sundermann V., Angerer J. (2007). Phthalates: toxicology and exposure. Int. J. Hyg. Envir. Heal. 210 (5), 623–634. doi: 10.1016/j.ijheh.2007.07.011
Huang J., Li X., Ma M., Li D. (2017). Removal of di-(2-ethylhexyl) phthalate from aqueous solution by UV/peroxymonosulfate: Influencing factors and reaction pathways. Chem. Eng. J. 314, 182–191. doi: 10.1016/j.cej.2016.12.095
Hu H., Mao L., Fang S., Xie J., Zhao M., Jin H. (2020). Occurrence of phthalic acid esters in marine organisms from hangzhou bay, China: implications for human exposure. Sci. Tot. Environ. 721, 137605. doi: 10.1016/j.scitotenv.2020.137605
Jambeck J. R., Johnsen K. (2015). Citizen-based litter and marine debris data collection and mapping. Comput. Sci. Eng. 17 (4), 20–26. doi: 10.1109/MCSE.2015.67
Khalil S. R., Elhakim Y. A., EL-Murr A. E. (2016). Sublethal concentrations of di-n-butyl phthalate promote biochemical changes and DNA damage in juvenile Nile tilapia (Oreochromis niloticus). Jpn. J. Vet. Res. 64 (1), 67–80. doi: 10.14943/jjvr.64.1.67
Kim E. J., Kim J. W., Lee S. K. (2002). Inhibition of oocyte development in Japanese medaka (Oryzias latipes) exposed to di-2-ethylhexyl phthalate. Environ. Int. 28 (5), 359–365. doi: 10.1016/S0160-4120(02)00058-2
Koelmans A. A., Besseling E., Shim W. J. (2015). ““Nanoplastics in the aquatic environment. critical review”,” in Marine anthropogenic litter. Eds. Bergmann M., Gutow L., Klages M. (Cham: Springer), 325–340. doi: 10.1007/978-3-319-16510-3_12
Koelmans A. A., Diepens N. J., Mohamed Nor N. H. (2021). ““Weight of evidence for the microplastic vector effect in the context of chemical risk assessment”,” in Microplastic in the environment: pattern and process. environmental contamination remediation and management. Ed. Bank M. S. (Cham: Springer), 155–197. doi: 10.1007/978-3-030-78627-4_6
Law K. L., Morét-Ferguson S., Maximenko N. A., Proskurowski G., Peacock E. E., Hafner J., et al. (2010). Plastic accumulation in the north Atlantic subtropical gyre. Science 329 (5996), 1185–1188. doi: 10.1126/science.1192321
Lorge E., Hayashi M., Albertini S., Kirkland D. (2008). Comparison of different methods for an accurate assessment of cytotoxicity in the in vitro micronucleus test. I. Theoretical aspects. Mutat. Res. - Genet. Toxicol. Environ Mutagen 655 , 1–3. doi: 10.1016/j.mrgentox.2008.06.003
Lyche J. L., Gutleb A. C., Bergman Å., Eriksen G. S., Murk A. J., Ropstad E., et al. (2009). Reproductive and developmental toxicity of phthalates. J. Toxicol. Env. Heal. B. 12 (4), 225–249. doi: 10.1080/10937400903094091
Macali A., Semenov A., Venuti V., Crupi V., D’Amico F., Rossi B., et al. (2018). Episodic records of jellyfish ingestion of plastic items reveal a novel pathway for trophic transference of marine litter. Sci. Rep. 8 (1), 1–5. doi: 10.1038/s41598-018-24427-7
Magdouli S., Daghrir R., Brar S. K., Drogui P., Tyagi R. D. (2013). Di 2-ethylhexylphtalate in the aquatic and terrestrial environment: A critical review. J. Environ. Manag. 127, 36–49. doi: 10.1016/j.jenvman.2013.04.013
Ma Y. B., Jia P. P., Junaid M., Yang L., Lu C. J., Pei D. S. (2018). Reproductive effects linked to DNA methylation in male zebrafish chronically exposed to environmentally relevant concentrations of di-(2-ethylhexyl) phthalate. Environ. pollut. 237, 1050–1061. doi: 10.1016/j.envpol.2017.11.025
Malem F., Soonthondecha P., Khawmodjod P., Chunhakorn V., Whitlow H. J., Chienthavorn O. (2019). Occurrence of phthalate esters in the eastern coast of Thailand. Environ. Monit. Assess. 191 (10), 1–13. doi: 10.1007/s10661-019-7785-5
Meschini R., D’Eliseo D., Filippi S., Bertini L., Bizzarri B. M., Botta L., et al. (2018). Tyrosinase-treated hydroxytyrosol-enriched olive vegetation waste with increased antioxidant activity promotes autophagy and inhibits the inflammatory response in human THP-1 monocytes. J. Agr. Food Chem. 66 (46), 12274–12284. doi: 10.1021/acs.jafc.8b03630
Molino C., Filippi S., Stoppiello G. A., Meschini R., Angeletti D. (2019). In vitro evaluation of cytotoxic and genotoxic effects of di (2-ethylhexyl)-phthalate (DEHP) on European sea bass (Dicentrarchus labrax) embryonic cell line. Toxicol. in Vitro 56, 118–125. doi: 10.1016/j.tiv.2019.01.017
Moore C. J. (2008). Synthetic polymers in the marine environment: a rapidly increasing, long-term threat. Environ. Res. 108 (2), 131–139. doi: 10.1016/j.envres.2008.07.025
Mori C., Morsey B., Levin M., Gorton T. S., De Guise S. (2008). Effects of organochlorines, individually and in mixtures, on b-cell proliferation in marine mammals and mice. J. Toxicol. Env. Healt. A. 71 (4), 266–275. doi: 10.1080/15287390701612860
Murray F., Cowie P. R. (2011). Plastic contamination in the decapod crustacean Nephrops norvegicus (Linnaeus 1758). Mar. pollut. Bull. 62 (6), 1207–1217. doi: 10.1016/j.marpolbul.2011.03.032
Oehlmann J., Schulte-Oehlmann U., Kloas W., Jagnytsch O., Lutz I., Kusk K. O., et al. (2009). A critical analysis of the biological impacts of plasticizers on wildlife. Philos. T. Roy. Soc. B. 364 (1526), 2047–2062. doi: 10.1098/rstb.2008.0242
Paluselli A., Aminot Y., Galgani F., Net S., Sempere R. (2018). Occurrence of phthalate acid esters (PAEs) in the northwestern Mediterranean Sea and the Rhone river. Prog. Oceanogr. 163, 221–231. doi: 10.1016/j.pocean.2017.06.002
Paluselli A., Fauvelle V., Galgani F., Sempéré R. (2019). Phthalates release and biodegradation from plastic fragments in seawater. Env. Sci. Technol. 53 (1), 166–175. doi: 10.1021/acs.est.8b05083
Parker B., Andreou D., Green I. D., Britton J. R. (2021). Microplastics in freshwater fishes: Occurrence, impacts and future perspectives. Fish. 22 (3), 467–488. doi: 10.1111/faf.12528
Pournejati R., Gust R., Sagasser J., Kircher B., Jöhrer K., Ghanbari M. M., et al. (2021). In vitro evaluation of cytotoxic effects of di (2-ethylhexyl) phthalate (DEHP) produced by bacillus velezensis strain RP137 isolated from Persian gulf. Toxicol. In. Vitro 73, 105148. doi: 10.1016/j.tiv.2021.105148
Proietti De Santis L., Lorenti Garcia C., Balajee A. S., Brea Calvo G. T., Bassi L., Palitti F. (2001). Transcription coupled repair deficiency results in increased chromosomal aberrations and apoptotic death in the UV61 cell line, the Chinese hamster homologue of cockayne’s syndrome. B. Mutat. Res. 485, 121–132. doi: 10.1016/S0921-8777(00)00065-3
Radke E. G., Braun J. M., Nachman R. M., Cooper G. S. (2020). Phthalate exposure and neurodevelopment: A systematic review and meta-analysis of human epidemiological evidence. Environ. Int. 137, 105408. doi: 10.1016/j.envint.2019.105408
Rangel-Buitrago N., Williams A., Costa M. F., de Jonge V. (2020). Curbing the inexorable rising in marine litter: An overview. Ocean. Coast. Manag. 188, 105133. doi: 10.1016/j.ocecoaman.2020.105133
Selvaraj K. K., Sundaramoorthy G., Ravichandran P. K., Girijan G. K., Sampath S., Ramaswamy B. R. (2015). Phthalate esters in water and sediments of the kaveri river, India: environmental levels and ecotoxicological evaluations. Environ. Geochem. Hlth. 37 (1), 83–96. doi: 10.1007/s10653-014-9632-5
Sies H. (2017). Hydrogen peroxide as a central redox signaling molecule in physiological oxidative stress: Oxidative eustress. Redox Biol. 11, 613–619. doi: 10.1016/j.redox.2016.12.035
Stewart M., Olsen G., Hickey C. W., Ferreira B., Jelić A., Petrović M., et al. (2014). A survey of emerging contaminants in the estuarine receiving environment around Auckland, new Zealand. Sci. Tot. Environ. 468, 202–210. doi: 10.1016/j.scitotenv.2013.08.039
Suman M., Giacomello M., Corain L., Ballarin C., Montelli S., Cozzi B., et al. (2012). Estradiol effects on intracellular Ca2+ homeostasis in bovine brain-derived endothelial cells. Cell Tissue Res. 350, 109–118. doi: 10.1007/s00441-012-1460-2
Sun J., Pan L., Zhan Y., Lu H., Tsang D. C., Liu W., et al. (2016). Contamination of phthalate esters, organochlorine pesticides and polybrominated diphenyl ethers in agricultural soils from the Yangtze river delta of China. Sci. Tot. Environ. 544, 670–676. doi: 10.1016/j.scitotenv.2015.12.012
Taddei F., Scarcelli V., Frenzilli G., Nigro M. (2001). Genotoxic hazard of pollutants in cetaceans: DNA damage and repair evaluated in the bottlenose dolphin (Tursiops truncatus) by the comet assay. Mar. pollut. Bull. 42 (4), 324–328. doi: 10.1016/S0025-326X(00)00159-4
Thibaut R., Porte C. (2004). Effects of endocrine disrupters on sex steroid synthesis and metabolism pathways in fish. J. Steroid Biochem. Mol. Biol. 92 (5), 485–494. doi: 10.1016/j.jsbmb.2004.10.008
Tran H. T., Lin C., Bui X. T., Nguyen M. K., Cao N. D. T., Mukhtar H., et al. (2022). Phthalates in the environment: characteristics, fate and transport, and advanced wastewater treatment technologies. Biores. Technol. 344, 126249. doi: 10.1016/j.biortech.2021.126249
Uren-Webster T. M., Lewis C., Filby A. L., Paull G. C., Santos E. M. (2010). Mechanisms of toxicity of di (2-ethylhexyl) phthalate on the reproductive health of male zebrafish. Aquat. Toxicol. 99 (3), 360–369. doi: 10.1016/j.aquatox.2010.05.015
Wang S., Cao Y., Wang S., Cai J., Zhang Z. (2020). DEHP induces immunosuppression through disturbing inflammatory factors and CYPs system homeostasis in common carp neutrophils. Fish. Shellfish Immun. 96, 26–31. doi: 10.1016/j.fsi.2019.11.073
Wang X., Yang Y., Zhang L., Ma Y., Han J., Yang L., et al. (2013). Endocrine disruption by di-(2-ethylhexyl)-phthalate in Chinese rare minnow (Gobiocypris rarus). Environ. Toxicol. Chem. 32 (8), 1846–1854 doi: 10.1002/etc.2261
Wang Y. Q., Li Y. W., Chen Q. L., Liu Z. H. (2013). Long-term exposure of xenoestrogens with environmental relevant concentrations disrupted spermatogenesis of zebrafish through altering sex hormone balance, stimulating germ cell proliferation, meiosis and enhancing apoptosis. Environ. pollut. 244, 486–494. doi: 10.1016/j.envpol.2018.10.079
Weaver J. A., Beverly B. E., Keshava N., Mudipalli A., Arzuaga X., Cai C., et al. (2020). Hazards of diethyl phthalate (DEP) exposure: A systematic review of animal toxicology studies. Environ. Internat. 145, 105848. doi: 10.1016/j.envint.2020.105848
Xu H. E., Cao W., Sun H., Zhang S., Li P., Jiang S., et al. (2021). Dose-dependent effects of di-(2-Ethylhexyl) phthalate (DEHP) in mussel Mytilus galloprovincialis. Front. Mar. Sci. 8. doi: 10.3389/fmars.2021.658361
Ye T., Kang M., Huang Q., Fang C., Chen Y., Shen H., et al. (2014). Exposure to DEHP and MEHP from hatching to adulthood causes reproductive dysfunction and endocrine disruption in marine medaka (Oryzias melastigma). Aquat. Toxicol. 146, 115–126. doi: 10.1016/j.aquatox.2013.10.025
Yu H., Caldwell D. J., Suri R. P. (2019). In vitro estrogenic activity of representative endocrine disrupting chemicals mixtures at environmentally relevant concentrations. Chemosphere 215, 396–403. doi: 10.1016/j.chemosphere.2018.10.067
Zantis L. J., Carroll E. L., Nelms S. E., Bosker T. (2021). Marine mammals and microplastics: A systematic review and call for standardisation. Environ. pollut. 269, 116142. doi: 10.1016/j.envpol.2020.116142
Zarfl C., Matthies M. (2010). Are marine plastic particles transport vectors for organic pollutants to the Arctic? Mar. pollut. Bull. 60 (10), 1810–1814. doi: 10.1016/j.marpolbul.2010.05.026
Zhang Y., Jiao Y., Li Z., Tao Y., Yang Y. (2021). Hazards of phthalates (PAEs) exposure: A review of aquatic animal toxicology studies. Sci. Tot. Environ. 771, 145418. doi: 10.1016/j.scitotenv.2021.145418
Zhang Z. M., Zhang H. H., Zou Y. W., Yang G. P. (2018). Distribution and ecotoxicological state of phthalate esters in the sea-surface microlayer, seawater and sediment of the bohai Sea and the yellow Sea. Environ. pollut. 240, 235–247. doi: 10.1016/j.scitotenv.2021.145418
Keywords: plastic additives, DEHP, DNA damage, cytotoxicity, genotoxicity, bottlenose dolphin cell line
Citation: Giovani G, Filippi S, Molino C, Peruffo A, Centelleghe C, Meschini R and Angeletti D (2022) Plastic additive di(2-ethylhexyl)phthalate (DEHP) causes cell death and micronucleus induction on a bottlenose dolphin’s (Tursiops truncatus) in vitro-exposed skin cell line. Front. Mar. Sci. 9:958197. doi: 10.3389/fmars.2022.958197
Received: 31 May 2022; Accepted: 20 July 2022;
Published: 11 August 2022.
Edited by:
Morgana Vighi, Consultant, Barcelona, SpainReviewed by:
Angela Cuttitta, Institute for Studies on the Mediterranean, National Research Council (CNR), ItalyThales Renato Ochotorena De Freitas, Federal University of Rio Grande do Sul, Brazil
Copyright © 2022 Giovani, Filippi, Molino, Peruffo, Centelleghe, Meschini and Angeletti. This is an open-access article distributed under the terms of the Creative Commons Attribution License (CC BY). The use, distribution or reproduction in other forums is permitted, provided the original author(s) and the copyright owner(s) are credited and that the original publication in this journal is cited, in accordance with accepted academic practice. No use, distribution or reproduction is permitted which does not comply with these terms.
*Correspondence: Chiara Molino, Yy5tb2xpbm9AdW5pdHVzLml0; Roberta Meschini, bWVzY2hpbmlAdW5pdHVzLml0
†Deceased. The other authors wish to dedicate this work to his memory
‡These authors have contributed equally to this work