- 1School of Life and Environmental Sciences, Centre for Integrative Ecology, Warrnambool Campus, Deakin University, Warrnambool, VIC, Australia
- 2Institute for Marine and Antarctic Studies, University of Tasmania, Hobart, TAS, Australia
- 3School of Life and Environmental Sciences, Melbourne Burwood Campus, Deakin University, Burwood, VIC, Australia
- 4School of Life and Environmental Sciences, Queenscliff Marine Science Centre, Deakin University, Queenscliff, VIC, Australia
- 5Spring Bay Seafoods Pty. Ltd., Triabunna TAS, Australia
- 6Tassal Group Pty. Ltd., Hobart, TAS, Australia
Seaweed cultivation is gaining interest world-wide for both food and non-food applications. Global seaweed aquaculture production currently exceeds 32 Mt WW per annum but is dominated (86% of total) by Asian countries. To meet future demand for seaweed products, regions beyond Asia with aquaculture production potential are being explored. The goal of this study was to assess the suitability of the native kelp Macrocystis pyrifera (Phaeophyceae, Laminariales), for aquaculture in Tasmania, south-eastern Australia. M. pyrifera was cultivated on seeded twine on loops (1 – 5 m depth) along 100-m longlines at two sites (Okehampton Bay and Great Taylor Bay) from April-November 2020. Temporal and spatial variability in (1) yield (kg m-1, WW), (2) biofouling (% coverage), and (3) biochemical composition (including proximate composition, fatty acids, dietary minerals, heavy metal profiling, C, N, H, S concentrations and C:N ratio, antioxidants (phenolic compounds), and pigments (Chl-a, Chl-c, fucoxanthin)) was compared amongst the two cultivation sites, at two depths (1 and 5 m) from harvests between July – November 2020. Yield (kg m-1, WW) did not significantly change across harvest times, but was greater at a depth of 1 m compared to 5 m. Biofouling on the kelp blades increased significantly in early spring (September). The biochemical composition of the cultured biomass varied over time, between sites and with depth for most of the compounds analysed. Higher lipid, protein and ash content was reported for cultures cultivated at Okehampton Bay compared to Great Taylor Bay and at 5 m compared to 1 m depth, and levels of these macronutrients decreased during the harvest period. The iodine content was slightly above the tolerable content for dried seaweed products in Australia and New Zealand. The combined results of yield, biofouling, and biochemical composition suggest that, for an April deployment at the sites investigated, M. pyrifera should be harvested in July-August (mid to late winter) to optimise yield and quality of the cultured kelp biomass. These findings provide a better understanding of the variation in growth and quality of cultivated M. pyrifera biomass in the region, and inform future management and development of kelp aquaculture in south-eastern Australia and in a global context.
1 Introduction
Global seaweed production has more than tripled in the past 20 years to an annual production of > 32 Mt WW in 2018, but is dominated (86% of total) by Asian countries, particularly China (58%) (FAO, 2020). Seaweeds are now the fastest-growing aquaculture sector (Chopin and Tacon, 2020) and a number of seaweeds are increasingly being viewed as an exciting new primary industry worldwide, including in countries such as Australia (Kelly, 2020) where the sector is still in its infancy. This has led to the exploration of novel culture species, native to areas outside of Asia, with unique downstream applications such as sources of bioactive compounds (Holdt and Kraan, 2011). The seaweed aquaculture sector has the potential to facilitate more sustainable marine production systems (beyond what is attainable for the fed fin-fish sector in isolation) and to develop tailored and efficient social license processes (e.g. spatial planning), particularly in Western countries (Duarte et al., 2022). To meet future food demands, seaweed aquaculture has to expand beyond Asia (Alexandratos and Bruinsma, 2012; Searchinger et al., 2019), into other regions with high production potential, such as temperate southeastern Australia. These regions have the potential to diversify the seaweed farming sector by focusing on novel native and/or endemic seaweeds with significant cultivation and commercial potential.
Profitability and success of aquaculture ventures worldwide can be significantly hampered by biofouling (Dürr and Watson, 2010). This includes the production of seaweeds (Lüning and Mortensen, 2015; Stévant et al., 2017; Visch et al., 2020), in which fouling can affect both hatchery cultivation (Su et al., 2017) as well as at-sea grow out (Førde et al., 2015; Rolin et al., 2017). When seeded lines are fouled in the hatchery, it may affect subsequent cultivation at sea. However, both marine infrastructure and developing thalli can also become fouled by a suite of invertebrates and algae irrespective of initial hatchery conditions, and negatively affect the quality of the harvestable biomass (Park and Hwang, 2012). Effects of biofouling in seaweed aquaculture include reduced commercial value and quality of the final product (Park and Hwang, 2012), loss of productivity due to competition for light (Cancino et al., 1987) and nutrients (Hurd, 2000), breakage of fronds (Dixon et al., 1981; Krumhansl et al., 2011), and interference with the farm infrastructure (Bannister et al., 2019). Furthermore, there are considerable costs associated with the management/treatment of fouled biomass and infrastructure (Marroig and Reis, 2011; Marroig and Reis, 2016; Bannister et al., 2019). Hence, preventing and controlling biofouling with site selection, exploring suitable substrata, good husbandry practices and optimized harvest strategies is paramount to ensure efficient production of high-quality biomass.
High-quality biomass, with favourable biochemical composition, can be a valuable resource for the food, feed, nutraceutical, and pharmaceutical sectors. The biochemical composition of numerous species of seaweeds have been studied, showing a range of interesting and nutritional compounds, including protein, fatty acids, minerals, vitamins, and antioxidant compounds (Wells et al., 2017). However, studies have also demonstrated that the biochemical composition of seaweeds can vary by species, location, season, and between wild and cultured biomass (Lourenço et al., 2002; Holdt and Kraan, 2011; Tibbetts et al., 2016; Wells et al., 2017). Furthermore, seaweeds can accumulate potentially toxic heavy metal ions from the environment (Cheney, 2016). Hence, optimal seaweed cultivation is dependent on an understanding of the effects of spatial and temporal variation in varying environmental conditions (e.g., light, nutrients, temperature, and pollutants) on the biochemical profile of the cultivated species for further commercial applications (e.g., as food, feed, nutraceutical, or fertilizer), particularly when culturing a novel species in a previously unexploited area (e.g., Gutierrez et al., 2006).
Currently, knowledge gaps in the optimization of the hatchery production and at-sea cultivation of commercially interesting species in Australia are hindering the industry growth. The Seaweed Solutions for Sustainable Aquaculture project (www.seaweedsolutions-crc.com/) is attempting to address these knowledge gaps for Australian kelps cultivated alongside salmonid and mussel farms in Tasmanian coastal waters. Giant kelp (Macrocystis pyrifera) was selected as target species due to its large size, fast growth rate, availability of culture manuals, being native to Australia, and existing commercial applications (Purcell-Meyerink et al., 2021). This paper reports on the first stage of this project, which aimed to determine the optimal at-sea culture and harvest conditions for cultivated M. pyrifera by assessing the variation in yield, biofouling, and biochemical composition of harvestable M. pyrifera throughout the cultivation period at two sites and two depths.
2 Material and methods
2.1 Hatchery cultivation and preparation of culture lines
To incorporate sufficient genetic diversity to promote successful sporophyte recruitment, given low genetic diversity of Macrocystis pyrifera in the southern hemisphere (Macaya and Zuccarello, 2010; Durrant et al., 2015), five fertile individuals of Macrocystis pyrifera were haphazardly selected from Blackmans Bay (43.0163°C S, 147.3315°C E) and used as seedstock for cultivation at both experimental sites. Spores were released following the methods described in Forbord et al. (2018). Briefly, fertile sorus tissue was dissected out of the thallus, thoroughly wiped clean with paper towel, then washed three times for 10 s in a betadine® solution (5 mL L-1) and rinsed with sterile seawater. Cleaned sori were kept overnight in the dark in damp paper towel at 12°C, and subsequent spore-release was induced by submerging the treated sori in filtered seawater (0.2 μm) with F/2-medium (Guillard and Ryther, 1962). The spore solution was kept in aerated glass flasks (3 L) under long-day photoperiod (16 h red light: 8 h dark), red-light conditions (~15 μmol photons m-2 s-1) provided by LEDs (Fluval aquasky ®) at 12 °C. The spores were allowed to develop into gametophytes that were grown vegetatively under the aforementioned red-light culture conditions for >1 year (Bartsch, 2018). The medium was changed approximately every 3-4 weeks and the gametophytes were fragmented using a sterilized handheld blender for approximately 10 seconds. After the vegetative growth period, the gametophyte solution used to seed the spools was then concentrated using a 10-μm filter and evenly sprayed using a sterilised conical sprayer (500 mL) onto ~1-mm diameter rough texturized seeding twine specifically developed for kelp aquaculture (AtSeaNova, AlgaeTex®) wrapped on spools made of PVC pipe (75-mm diameter and 50-cm length). Gametophytes were then cultured on the spools in 600-L plastic tubs (internal dimensions 1230-mm length × 930-mm width × 525-mm height; 20 spools per tub) under controlled laboratory conditions until young sporophytes developed, using white light at approximately 80 μmol photos m-2 s-1, neutral photoperiod (LEDs, Ledcanse Osram©, 6500k, 150-Watt, 12 h light: 12 h dark), in filtered seawater (to 0.2 μm) with F/2-medium at 12 °C. The total hatchery period was 51 and 54 days for the spools deployed at Great Taylor Bay and Okehampton Bay, respectively. To ensure sufficient nutrients, complete filtered seawater changes occurred weekly during laboratory culture, at which time F/2-medium was also replaced. Once sporophytes reached 1-2 cm in length, the seeded twine was transported to the cultivation sites on the spools in insulated containers without submersion in water. To ensure they did not dry out, the spools were covered with cloths soaked in filtered seawater and doused periodically while being transported. The seeded twine was then wound around cultivation long lines (Quality Equipment ©, pre-brushed, lead-core UV-treated PP mussel spat rope) in situ by spinning the spools around the rope as the rope was fed through the middle of the spool, aided by a modified drill (Supplementary Figure S1). The seeded long lines were deployed at the culture sites as detailed below.
2.2 At-sea cultivation and site description
Macrocystis pyrifera was cultivated at two sites with existing aquaculture leases (Okehampton Bay and Great Taylor Bay) on the south-east coast of Tasmania, Australia (Figure 1A) as part of a larger industry partnership (https://www.seaweedsolutions-crc.com/). Both Okehampton Bay and Great Taylor Bay have Atlantic salmon (Salmo salar) aquaculture cages approximately 450-m and 600-m from the seaweed culture lines, respectively, and at Okehampton Bay there were also mussels (Mytilus edulis) cultured within 80-m of the seaweed culture lines. Both salmon and mussel production overlapped with the period of M. pyrifera cultivation during this study. Furthermore, both sites have similar bathymetry, with average depths of 35 m and 27 m at Great Taylor Bay and Okehampton Bay, respectively.
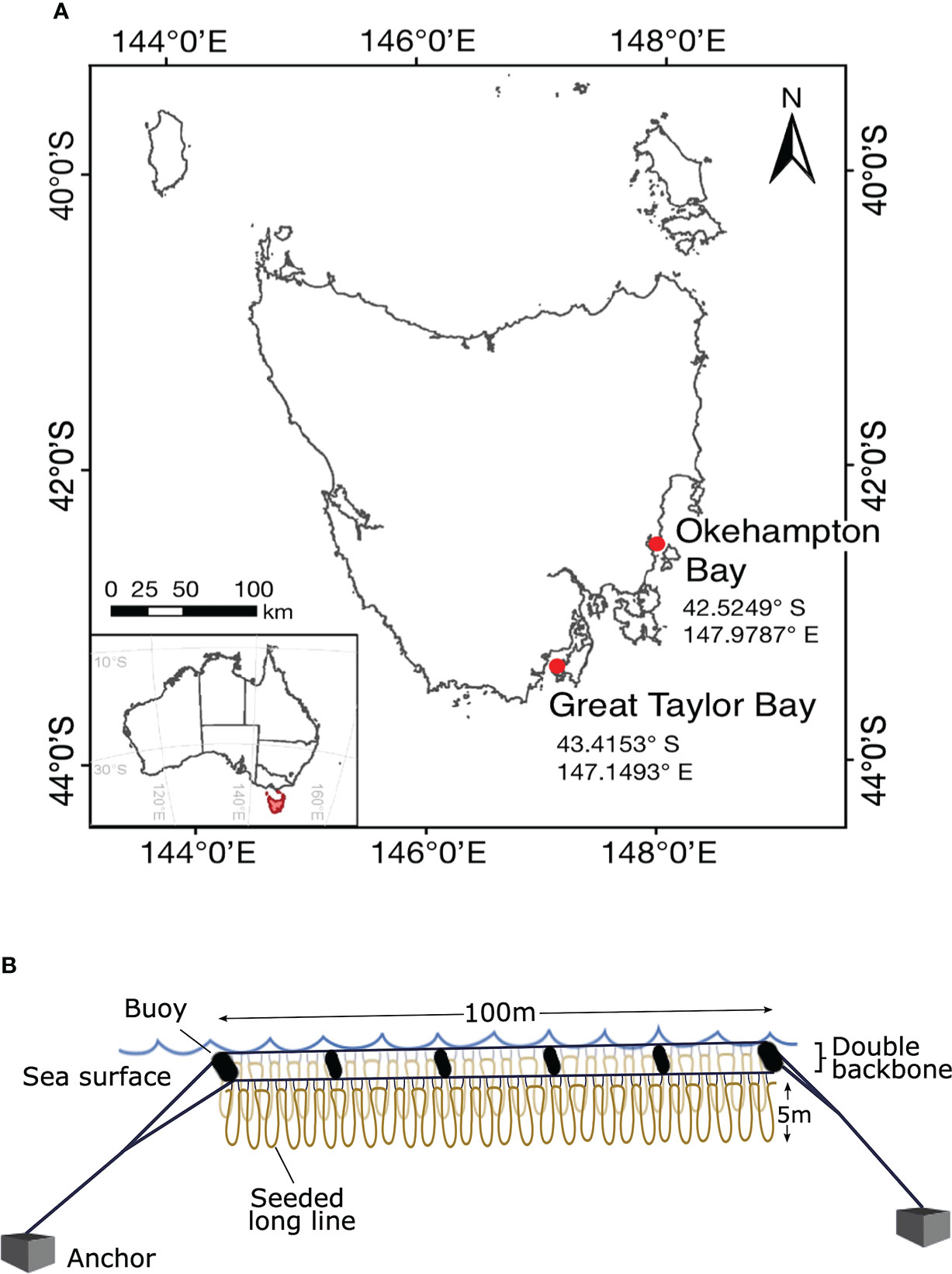
Figure 1 Geographic location and study design. Map of Tasmania, Australia, showing (A) the study area of the two farm sites in south-east Tasmania, and (B) the long-line cultivation system replicated (N = 3) at each site where the loops represent the seeded line wrapped around the mussel spat rope attached to the headline with twine. The double backbones are separated by 1.3-m wide buoys.
At each site, seeded long lines were deployed in a looped arrangement (1 to 5-m depth) onto three 100-m double-backbone long lines (32-mm polypropylene rope) (Figure 1B). This set-up was chosen to maximise the space available in the marine lease. The three double-backbones were aligned parallel and separated by ~25-m to allow for the passage of service vessels between. The seeded long lines were looped parallel along each backbone and secured at ~1-m intervals with 5-mm nylon rope to float submerged from 1-m depth. This allowed for a total of approximately 2000-m seeded rope on each 100-m double-backbone. Each 100-m double-backbone was kept afloat and separated by 1.3-m with buoys (300-L) on either end and four 110-L buoys spaced along the backbone (Figure 1B), and secured at the sites by 350-kg concrete anchors. In the southern hemisphere, kelps are typically cultured from autumn (March, April, May) throughout winter (June, July, August) and harvested in spring (September, October, November). Therefore, the experimental period was from 23/04/2020 until 16/11/2020 at Okehampton Bay and from 20/04/2020 until 24/11/2020 at Great Taylor Bay. In October one of three lines was lost at Okehampton Bay due to bad weather affecting replication at that site thereafter.
Sea-surface temperature (°C) was measured throughout the experimental period at 30-minute intervals using Onset HOBO Pendant MX2201 loggers, with two loggers deployed at 1-m and 5-m depths at each site. As no differences were observed between the two depths, logger data were pooled. Dissolved inorganic nutrients at each site were haphazardly sampled monthly by subsampling 10-mL seawater (N = 3) from seawater samples taken at 1-m and 5-m depths using a 2.5-L Niskin bottle. Seawater samples were filtered immediately after collection using glass microfibre filter paper (0.7 μM, Whatman, GF/F), stored in 12-mL polyethylene nutrient vials (LabServ®) and transported on ice (< 4 h) to the laboratory where they were stored at −20°C for < 3 months, until analysis. Nutrient concentrations of nitrate + nitrite and phosphate in seawater were analysed using a QuickChem® 8500 Automated Ion Analyser (LaChat Instruments) following methods outlined in Diamond (2008) and Liao (2008). Data on Secchi depths were recorded and provided by Tassal Ltd from their routine sampling, but the numbers of replicate measurements available varied across the duration of our study. At Great Taylor Bay N = 1 in November, N = 6 in July, N = 9 in May, N = 11 in August, N = 12 in December and N = 13 in June; whilst at Okehampton Bay, N = 6 in December, N = 11 in August/September/November, N = 13 in May, N = 15 in July, and N = 18 in June.
2.3 Assessment of yield and biofouling of cultivated Macrocystis pyrifera
The yield (kg m-1 WW) of cultivated M. pyrifera and amount of biofouling on the blades were assessed monthly during the harvest period by randomly selecting 1-m sections from the top and bottom of three haphazardly selected loops at approximately 1- and 5-m depths on each long line (N = 3 for each site), and then stripping all seaweed biomass from these sections. The stripped biomass was placed into labelled plastic bags for each replicate, returned to the laboratory on ice and then stored at ~4°C until analysis. Yield was assessed within 24-h of collection by blotting the stripped biomass with paper towel and then measuring the wet weight for each 1-m length of cultivated rope (average of the 3 haphazardly selected loops per long line, 3 replicate long lines per site) on an analytical balance (Ohaus Pioneer PA2102).
The percentage cover of biofouling organisms on the surfaces of the cultivated M. pyrifera was then assessed by haphazardly selecting 10 individual sporophytes from each of the 1-m sections of seeded rope (i.e. each replicate sample) and photographing the largest lamina on each individual. Photographs were then imported into ImageJ image analysis software (https://imagej.nih.gov/ij/) and the area (in mm2) of biofouling organisms on each blade was calculated by selecting (though image adjustment) the outer margin of the fouled area. The total area of each blade was also calculated by selecting the outer blade margin in photographs. The percentage cover of biofouling for each replicate sample was calculated by averaging the proportion of the surface area of biofouling (BA) relative to the whole blade area (WBA) for each of the 10 lamina in each replicate sample and multiplying by 100 (Eqn. 1):
2.4 Nutritional profiles of seaweeds
2.4.1 Sample preparation
To compare the variability in the biochemical composition of M. pyrifera according to harvesting time, site, and depth, tissue samples (up to 600-g wet weight of blades, pneumatocysts, and stipes) were harvested from the culture lines (N = 3) at 1-m and 5-m depths from each site at monthly intervals from July to November 2020. Samples were placed in labelled zip-lock plastic bags without water and transported on ice from the field to the laboratory where they were stored at ~4°C for < 24 h. Prior to subsampling and analysis of proximate composition, fatty acids, dietary minerals, heavy metal contaminants and antioxidant, seaweed samples were cleaned, washed with seawater first and then freshwater, blotted dry, and stored at -20°C for < 6 months until freeze dried by Forager Food Co., after which they were ground to powder (1 – 3 mm) using a Vitamix (Vitamix®, Total Nutrition Centre, 5200 Food Blender), and analysed. Analyses of C, N, H and S were performed on oven-dried (60°C) biomass and pigment analyses on fresh biomass, as detailed below. Samples analysed for pigment content were collected by removing a piece (0.1 ± 0.01 g wet weight) ~7 cm above the stipe from 10 individual thalli (distinguishable by an intact holdfast/stipe; or from all intact, distinguishable thalli per metre of long-line when there were fewer than 10 available) before further analysis (as detailed below).
2.4.2 Biochemical analyses
The dried biomass was cleaned of biofouling and invertebrates as thoroughly as possible prior to the analyses. Analysis of the nutritional profiles included proximate composition, fatty acids (FA), dietary minerals, heavy metal contaminants antioxidant, C (%), N (%), H (%), S (%) and C:N ratio, and pigments (Chl-a, Chl-c, Fucoxanthin). Protein content was estimated according to the AOAC (2006) and determined using an automated KjelFlex K-360 (BUCHI, Switzerland) with N × 5 as conversion factor for seaweeds (Angell et al., 2016). Total lipid content was determined with cold extraction using dichloromethane: methanol (2:1) according to (Folch et al., 1957), modified by (Ways and Hanahan, 1964). Residual moisture in samples was determined by oven drying at 105°C for at least 18 h until weight stabilised. Total ash was determined by incineration of samples in a muffle furnace at 550°C for 18 h (Atkinson et al., 1984). Carbohydrate content was included in the Nitrogen-free extract (NFE), and calculated by subtraction (Skrzypczyk et al., 2019; Eqn. 2):
Fatty acids in each sample were analysed following Mock et al. (2020) and as described in Biancacci et al. (2022).
Dietary minerals and metal ion content were extracted and analysed with an inductively coupled plasma-mass spectrometer (ICP-MS, NexION 350X, PerkinElmer, USA) as described in Biancacci et al. (2022). High purity standard solutions were purchased from PerkinElmer (Springvale, Melbourne). A certified reference material (Standard Reference Material® 1573a, Tomato Leaves, National Institute of Standards and Technology, USA) was run simultaneously with the seaweed samples (see Supplementary Table S1 for % recovery). Calibration standards between 0.05 - 1000 µg L-1 were prepared using multi-element stock 2 and 3. For the elements of Na, K, P separate calibration solutions of 500 - 5000 µg L-1 were prepared. For mercury, calibration solutions from 0.05, 0.1, 0.5, 1 and 5 µg L-1 was prepared. An internal standard solution containing Rh (100 ppb) and Ir (50 ppb) in 1% aqua regia were used for correction added via a T-piece prior to entering the spray chamber. This internal standard was mixed prior to the source using a T-piece in a 1:1 ratio. Quantitative data was collected on the elements present in multi-elemental calibration standards for elements without a standard the raw signal was compared against the blank to determine if the element was detected. The plasma settings were set according to Biancacci et al. (2022).
For the extraction of phenolic compounds, 15 mg of freeze-dried material were extracted with 1 mL of 70% ethanol (ethanol:MilliQ water 70:30, v:v), first sonicated for 15 min and then placed on an agitating plate in a heat block (≤ 40°C) for 2 h. The extraction was repeated twice on the same material. The pooled supernatant was dried using a heat block (≤ 40°C) coupled with nitrogen evaporation and the dried pellet resuspended to get a 2 g L-1 concentration. Analyses for phenolic compounds were performed following a modified Folin-Ciocalteu colorimetric assay (Dang et al., 2017; Marine Environmental Sciences Laboratory, IUEM, Brest, France).
Samples analysed for carbon, nitrogen, C:N-ratio, sulphur, and hydrogen were collected by removing a 5 cm2 piece at the centre of the blade ~5 cm above the stipe from 10 individual thalli (distinguishable by an intact holdfast/stipe; or from all intact, distinguishable thalli per metre of long-line when there were fewer than 10 available). Pieces were dried at 60 °C for 48 h and pooled before ground to a fine powder (<0.1 mm grain size) using mortar and pestle. Analyses were performed using an elemental analyser (NA1500) coupled to a Thermo Scientific Delta V Plus via a Conflo IV.
Pigments were extracted and the concentration in each replicate determined following Seely et al. (1972) and expressed as mg g-1 WW. Briefly, a section of defrosted tissue (0.1 ± 0.01 g WW) was placed in a test tube, and 4 mL dimethyl sulfoxide (DMSO) was added for 10 min. The absorbance of the supernatants (i.e., DMSO extract and acetone extract) was measured using a scanning spectrophotometer (Halo RB-10, Dynamica Scientific Ltd) at 480, 582, 631 and 665 nm for the DMSO extract and 470, 581, 631 and 664 for the acetone extract.
2.5 Statistical analysis
COVID-19 restrictions and associated logistics, inclement weather and the amount of harvestable biomass affected sampling such that our planned fully orthogonal, multi-factor sampling regime was not achieved. Consequently, harvest time, site and depth were analysed separately in single factor analyses pooling across other factors. We acknowledge that this precludes the examination of interactions between factors, and suggest future studies attempt to untangle such complexities. Furthermore, because one of the three replicate lines was lost at Okehampton Bay in October due to bad weather, our analyses involving comparisons between sites were slightly unbalanced (N = 2 or 3 for Okehampton and Great Taylor Bay, respectively) and non-significant results for all analyses involving samples from Okehampton after October should be interpreted cautiously. This is because there may have been insufficient statistical power to detect significant effects if they did occur. Subsequent studies could thus provide more robust tests of any non-significant results reported herein by repeating the comparison of interest with higher numbers of replicates and statistical power.
Biofouling coverage was analysed using a one-way ANOVA with harvest time as the factor (pooling among the other factors due to the reduced size of the available dataset). Effects of the factors harvesting time, site, and depth on the nutritional composition and yield of cultured M. pyrifera were analysed using one-way ANOVAs followed by Tukey’s HSD post-hoc test on proximate composition and yield for each factor (pooling among the other factors due to the reduced size of the available dataset) and one-way PERMANOVAs for each of FA (mg g-1 DW sample), dietary minerals and heavy metal ions (mg kg-1 DW). Univariate ANOVAs accompanied by a Tukey’s HSD test were used to determine the difference in the score of the attributes identified by SIMPER among the factors of interest. Multivariate analyses on minerals, heavy metals contaminants and fatty acids included non-metric multidimensional scaling (NMDS) (Kruskal, 1964) based on Euclidean distances (with accepted stress values < 0.20 and ideally < 0.10 as recommended by Clarke (1993), PERMANOVA+ (v. 1.0.5) test with 999 permutations, combined with a test for homogeneity of dispersions within factor groups performed using PERMDISP (Anderson et al., 2008) with distance to centroids. After significant interaction in PERMANOVA, an additional PERMANOVA was run on the interaction term comparing treatments individually by the factor of interest. In these cases, the P-value based on Monte Carlo random draws [P(MC)] was selected as it is more robust with the reduced number of possible permutations in pairwise tests (Anderson et al., 2008). Similarity percentages (SIMPER) test was used to assess the contribution of individual attributes to the dissimilarities according to the group factors investigated. Univariate analyses were performed using Minitab 19 software and multivariate analyses on PRIMER v6.1.1 (PRIMER-E Ltd. 2013). Univariate data were tested for normality with Kolmogorov–Smirnov test, and for the homogeneity of variance using a Levene’s test and transformed accordingly when necessary. All statistical tests were tested at α = 0.05. Summary results of all ANOVAs and PERMANOVAs are listed in Supplementary Tables S2, S3, respectively.
3 Results
3.1 Environmental parameters (temperature, irradiance, and dissolved inorganic nutrients)
Environmental data differed between the two sites, with Great Taylor Bay generally being cooler, but with poorer light penetration, and nutrient concentrations higher in July but lower thereafter, compared to Okehampton Bay (Figure 2). During the cultivation period the water temperature (mean ± SEM, N = 2) was 11.4 ± 0.5°C and 12.5 ± 0.6°C at Great Taylor Bay and Okehampton Bay, respectively, with respective maxima of 13.5°C and 16.1°C (Figure 2A). The Secchi depth (mean ± SEM, N = 1 – 18) was 6.2 ± 0.3 m and 10.5 ± 0.9 m at Great Taylor Bay and Okehampton Bay, respectively (Figure 2). Dissolved inorganic nutrients (mean ± SEM, N = 6) changed during the cultivation period (Figure 2). Nitrate + nitrite (Figure 2) peaked in July (winter) at 4.6 ± 0.6 µmol L-1 and 3.8 ± 0.6 µmol L-1 at Great Taylor Bay and Okehampton Bay, respectively, while minima were in October (spring) at 1.4 ± 0.1 µmol L-1 and 1.2 ± 0.1 µmol L-1 at Great Taylor Bay and Okehampton Bay, respectively. Dissolved phosphate (Figure 2) peaked in Great Taylor Bay in July (winter) at 0.7 ± 0.09 µmol L-1 and at Okehampton Bay in August at 0.6 ± 0.01 µmol L-1. Minimum phosphate concentrations (0.4 ± 0.01 µmol L-1 and 0.4 ± 0.01 µmol L-1 at Great Taylor Bay and Okehampton Bay, respectively) were observed in November (spring).
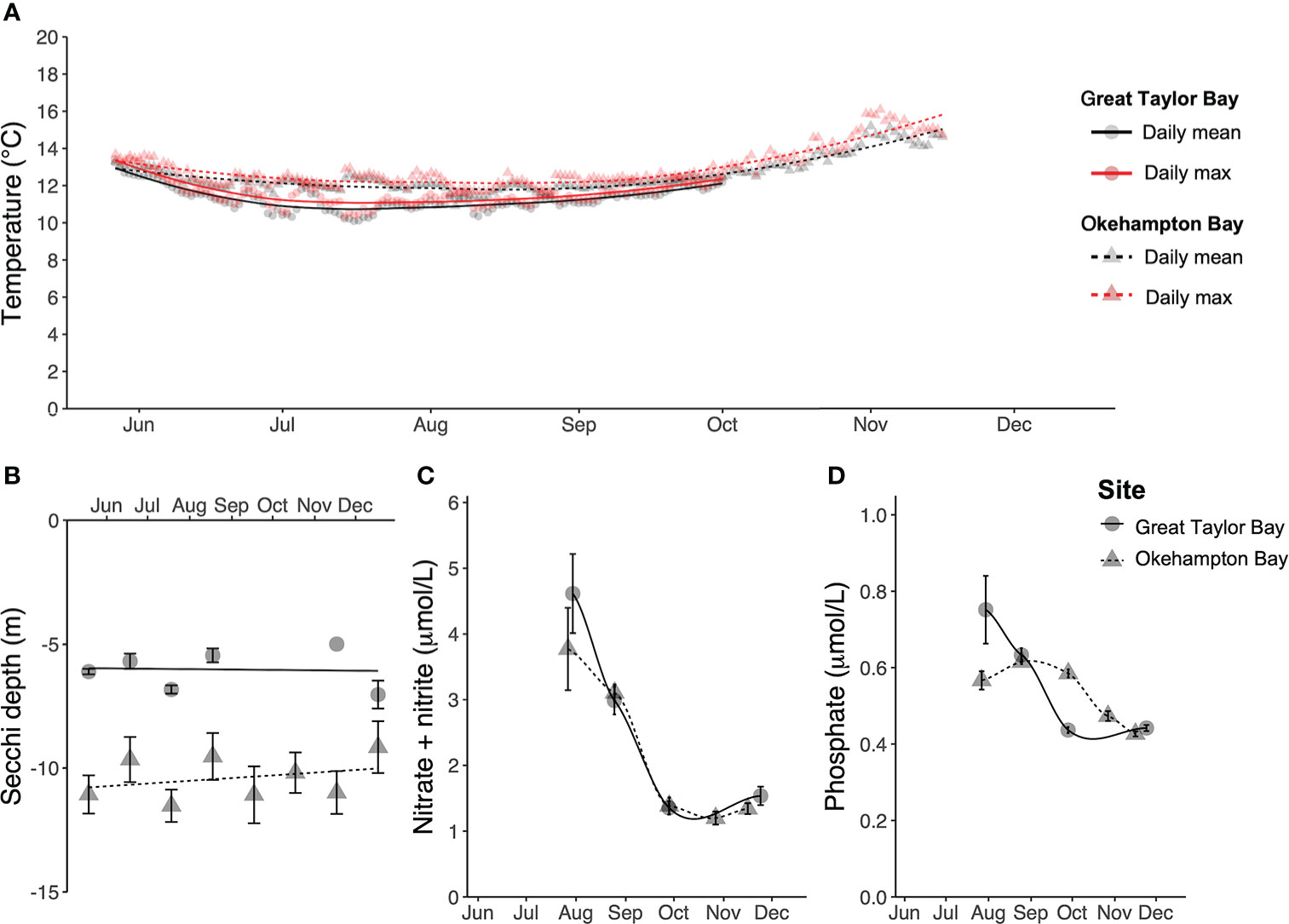
Figure 2 Environmental conditions at both farm sites in Tasmania (A) Daily mean and maximum temperature (°C), (B) Secchi depth (m), (C) dissolved nitrate + nitrite (µmol L-1), and (D) dissolved phosphate (µmol L-1). Error bars show SEM, with N = 1 – 18 for Secchi depth, and N = 6 for nitrate + nitrite and phosphate. For temperature, N = 2 for each site, but SEM (ranging 9.7 – 16.1°C) bars ommitted for visual clarity.
3.2 Yield
Overall, the yield (kg m-1, WW) of cultivated M. pyrifera averaged 1.20 ± 0.1 kg m-1 (mean ± SEM). There was no significant difference in yield over time (Supplementary Table S2) although there was a non-significant trend of increasing biomass from July to November, reaching a maximum in October of 1.50 kg m-1 (Figure 3A). Similarly, there was no significant difference in yield between sites (Supplementary Table S2, Figure 3B), but yield was significantly higher at 1-m compared to 5-m depth (Supplementary Table S2, Figure 3C).
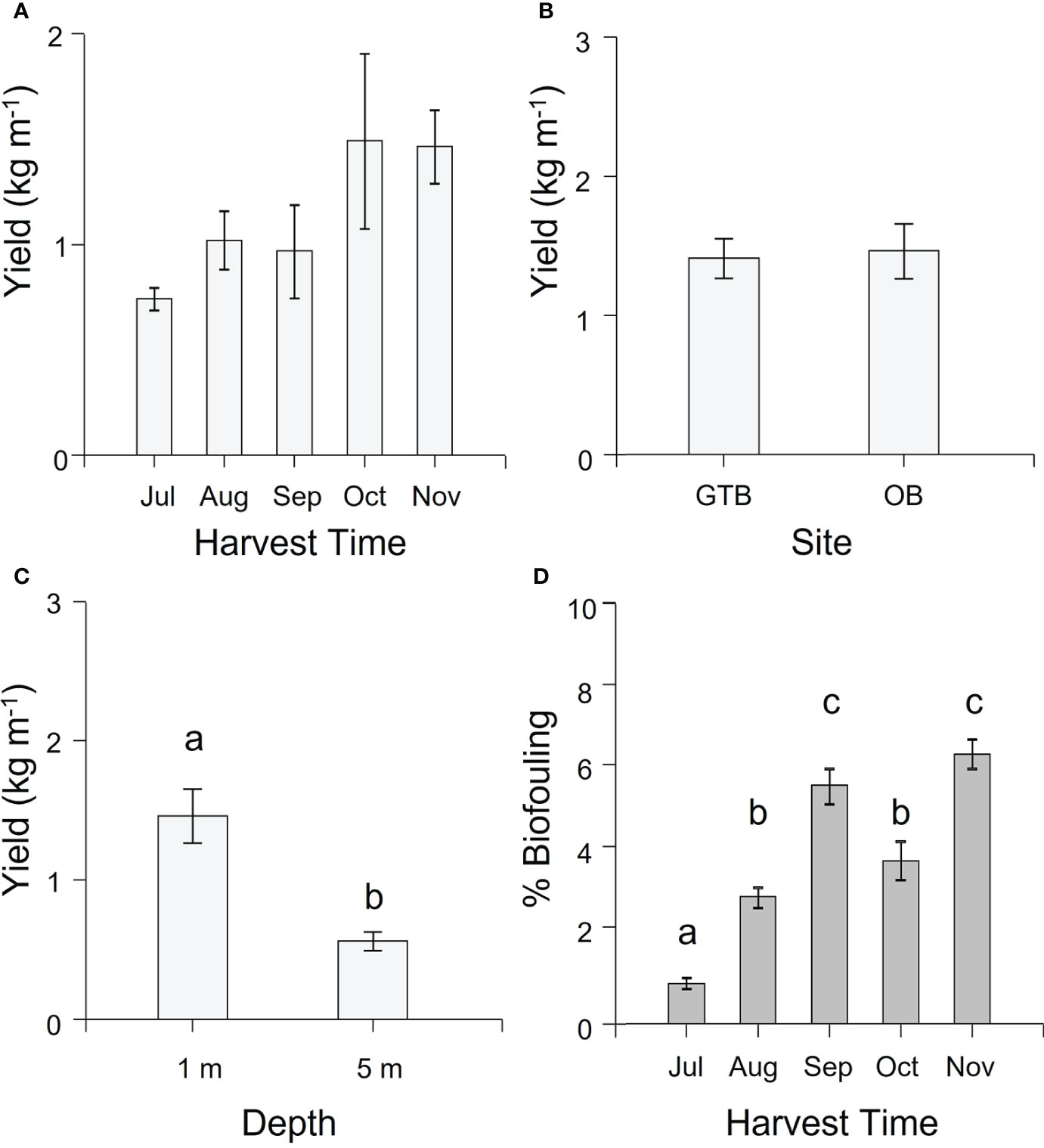
Figure 3 Mean (± SEM) yield (kg m-1 in wet weight) and biofouling coverage (%). (A) Yield per harvesting time. Values are pooled across site and depth. (B) Yield per Site. Values pooled across season for 1-m depth. (C) Yield per Depth. Values for Okehampton Bay pooled across season. Letters indicate groups that differ significantly between depths (Tukey’s test, P < 0.05). (D) Average biofouling on the largest lamina per individual for 10 individual sporophytes from each of the 1-m sections of seeded rope per each factor (site and depth) across the cultivation season. Values are pooled across site and depth. Different letters above bars represent significant differences amongst harvest times (P < 0.05) from Tukey’s HSD test.
3.3 Biofouling
There was significantly more biofouling on the cultivated biomass in all months after July (Supplementary Table S2, Tukey’s HSD test P < 0.05 for all comparisons; Figure 3D; Supplementary Figure S2), going from just below 1% to almost 7% by the end of the cultivation period. Fouling organisms could be found on the seaweed blades, especially on the terminal part of the blades, and included species of red, green and brown seaweeds and various invertebrates; however, these were not identified for the purpose of the study.
3.4. Biochemical composition
3.4.1 Proximate composition and total phenolic content
The proximate composition varied temporally for all the components analysed (Supplementary Table 2) with lipid, protein, and ash decreasing from July to November, whilst NFE and moisture increased in the same interval (Figures 4A–E). There were no significant temporal differences in total phenolic content (TPC) (Supplementary Table S2, Figure 4F).
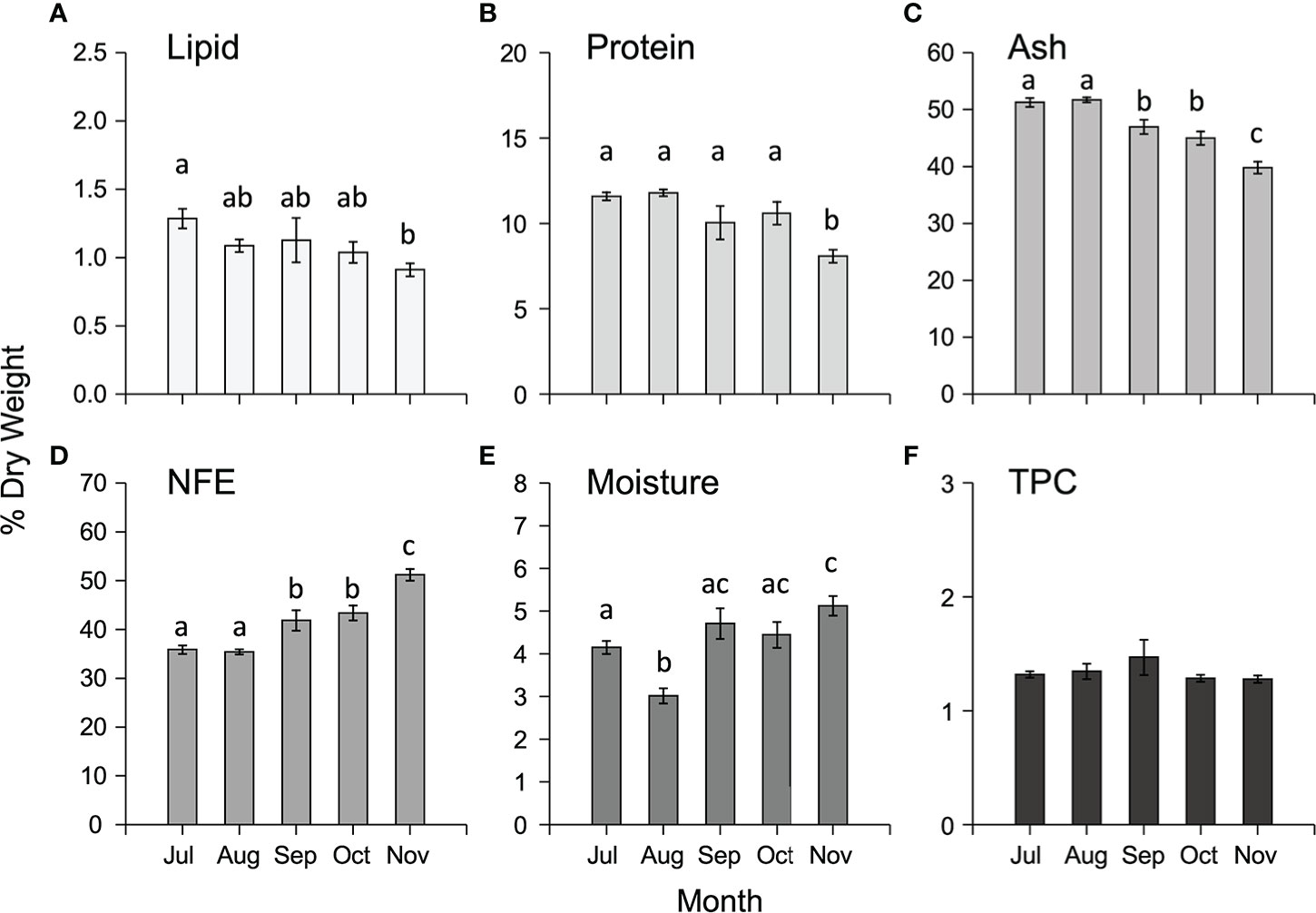
Figure 4 Mean (± SEM) proximate composition (expressed as % DW) including (A) lipid, (B) protein, (C) ash, (D) NFE, (E) moisture and (F) total phenolic content (TPC, expressed as mg g -1 DW) of cultivated M. pyrifera in south-east Tasmania along the cultivation period. Different letters above bars represent significant differences (P < 0.05) amongst harvest times from Tukey’s HSD tests.
There was significant spatial variation amongst both sites and depths (1-m and 5-m) in the proximate composition of cultivated M. pyrifera biomass (Supplementary Table S2). Overall, concentrations of total lipid were low (<1.5% DW), protein low to moderate (7.5-14%), and ash and NFE high (37-53%, 34-53% respectively), with lipid, protein and ash significantly higher in samples from Okehampton Bay compared to samples from Great Taylor Bay, while NFE and moisture content were higher in Great Taylor Bay samples (Supplementary Tables S2, S4). Samples from 1- and 5-m depths for Okehampton Bay were compared, with statistically higher content reported for all proximate components at 5-m, except NFE, which was higher in samples cultivated at 1-m (Supplementary Table S2). In contrast, there were no significant differences in total phenolic contents amongst either sites or depths (Supplementary Tables S2, S4).
3.4.2 C, N, S, H and C:N ratio
The concentration of C, N, S, H (as percentages) and C:N ratio varied temporally and spatially among sites and depths (Supplementary Table S2). While N and S decreased from July to November, C, H, and C:N ratio increased in the same interval (Figure 5A, Supplementary Table S4). The C:N ratio and the percentage of N and S varied significantly among sites (Supplementary Table S2), with higher C:N ratio in samples from Great Taylor Bay (Figure 5B) and higher N and S concentrations in samples from Okehampton Bay (Figure 5B; Supplementary Table S4). In contrast, there were no significant differences in the concentrations of C and H among sites (Supplementary Tables S2, S4, and Figure 5B). With respect to depth, there were significantly higher concentrations of C and H in samples at 1-m compared to 5-m depth, and S at 5-m compared to 1-m depth (Supplementary Table S2, Tukey’s HSD test P < 0.05, Figure 5C). However, there was no significant effect of depth on either N or C:N ratio (Supplementary Table S2, Figure 5C).
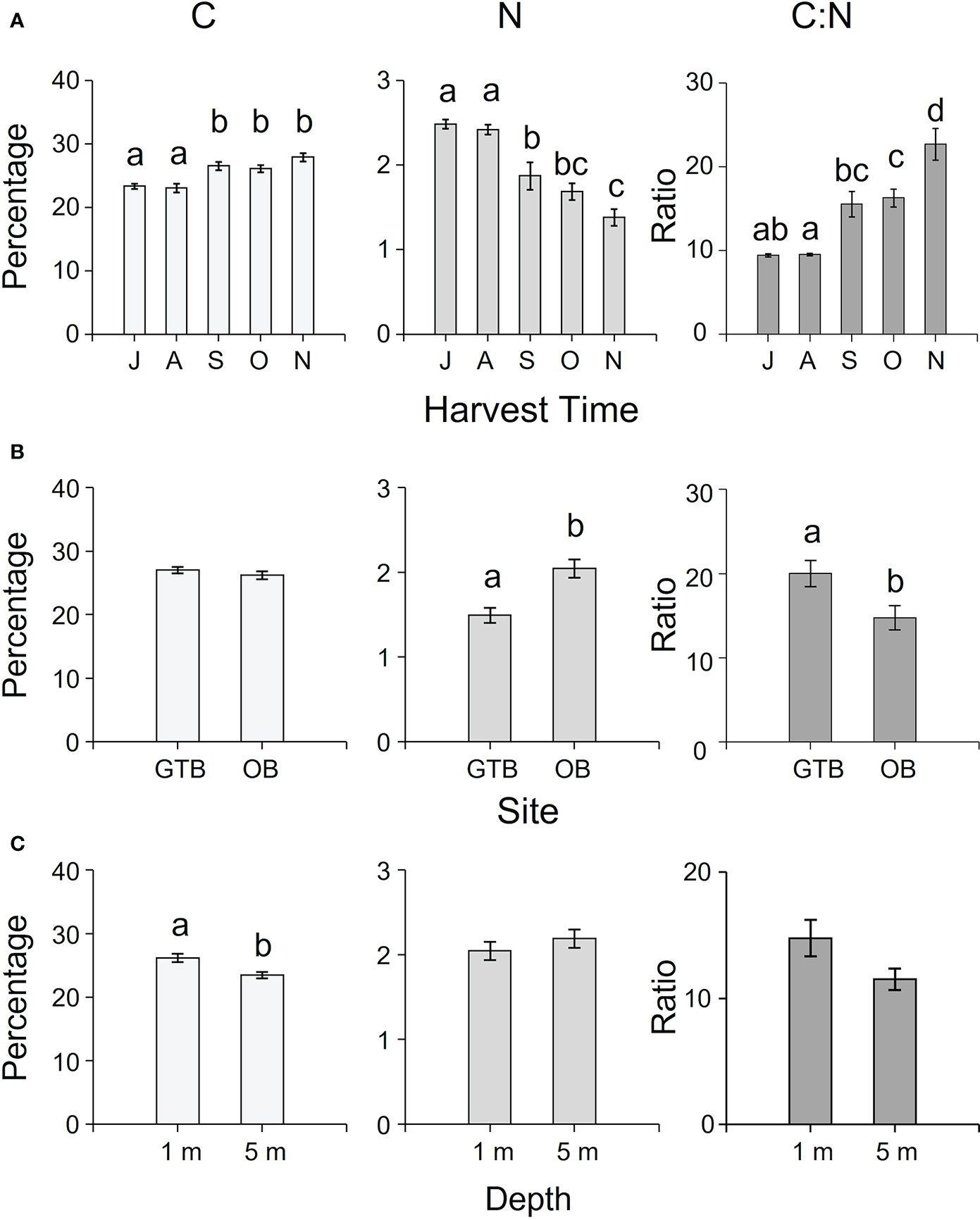
Figure 5 Mean (± SEM) percentages of C, N and C:N ratio of cultivated M. pyrifera according to (A) harvesting time (J = July, A = August, S = September, O = October, N = November), (B) site (GTB = Great Taylor Bay, OB = Okehampton Bay), and (C) depth (1-m and 5-m for Okehampton). Different letters above bars represent significant differences (P < 0.05) amongst the relevant factors from Tukey’s HSD test.
3.4.3 Pigments
There was significant temporal variation in the concentrations of Chl-a and fucoxanthin both decreasing from July through November but not Chl-c (Supplementary Table S2). Chl-a varied spatially with significantly higher concentrations in samples from Okehampton Bay compared to Great Taylor Bay, but there were no significant differences in the concentration of Chl-c and fucoxanthin amongst sites or any of the pigments amongst depths (Supplementary Tables S2, S4).
3.4.4 Elements and metal ions
3.4.4.1 Dietary minerals
There was significant temporal variation in the dietary mineral composition of cultivated M. pyrifera biomass, both for individual elements (see below) and when all elements were considered in combination (Supplementary Table S3), but the latter were not due to differences in dispersion among groups (PERMDISP, P > 0.05). The overall dietary mineral composition did not differ between July and August (pair-wise test on interaction term: P(MC) = 0.054) but there was a clear separation among winter and spring months (Figure 6A; pair-wise test on interaction term: P < 0.05 for all pair-wise tests on interaction term between winter and spring months) that was most strongly influenced by differences in concentrations of K, Na, P, and Fe (SIMPER Cum % August and November, K and Na 70.17%; July and November K and Na 74.05%; August and October K, Na, and Fe 74.87%; July and October K, Na, Ca 74.17%; July and September K and Na 70.36%). For individual elements, there were significant temporal differences in the concentrations of I, Na, K, Ca, Fe (Supplementary Table S2). K and Na decreased from July through to November, Ca and I generally increased from July onwards, whilst Fe and P varied temporally but not in a consistent pattern (Supplementary Tables S2, S4).
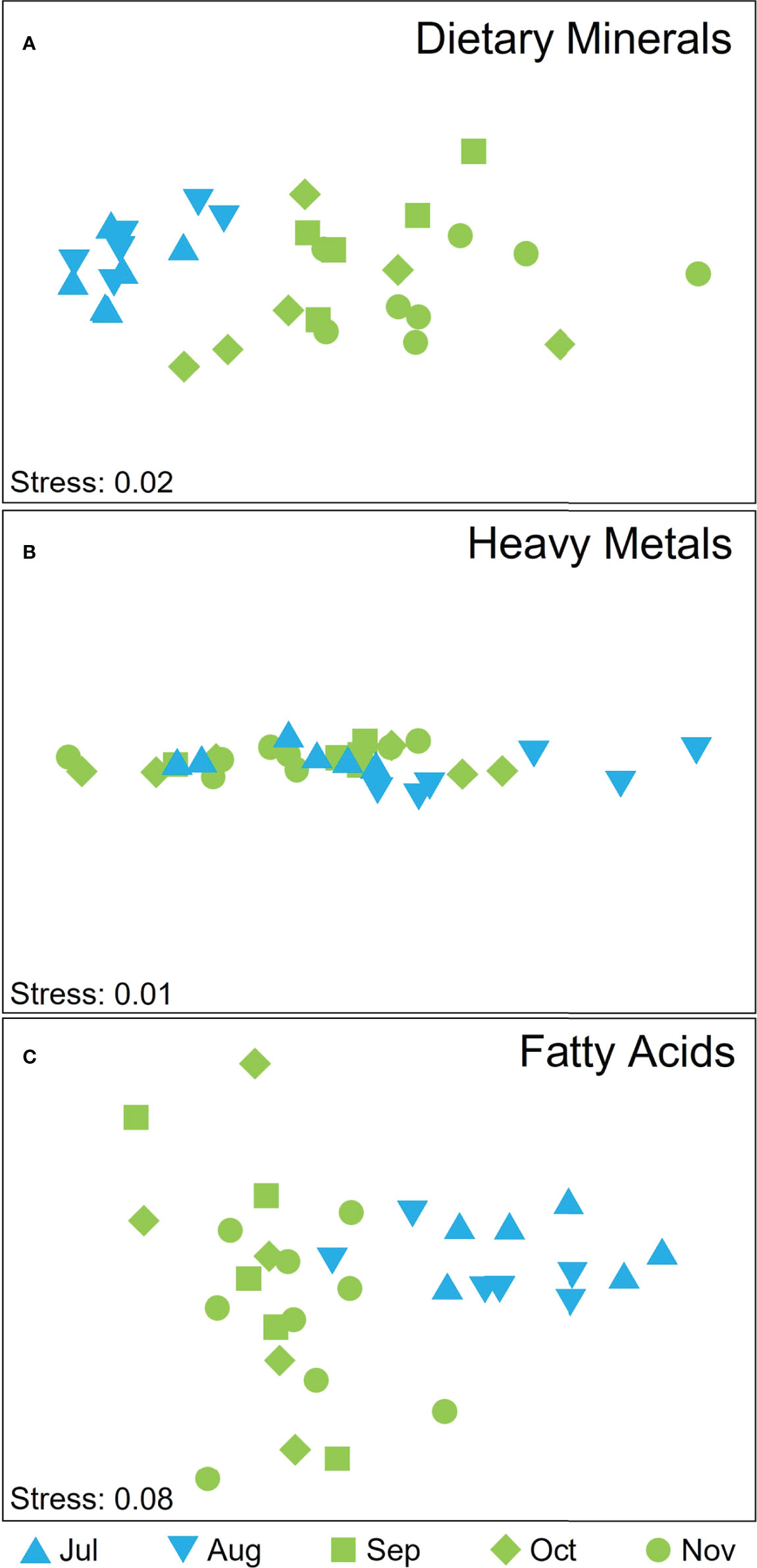
Figure 6 nMDS displaying attributes scores for (A) dietary minerals, (B) heavy metal ions, and (C) FA according to harvesting time, where blue symbols depict winter months and green spring months and shapes depict the month of harvest.
In contrast, neither site nor depth influenced the overall composition of dietary minerals (Supplementary Table S3), although there were significant differences in dispersion among sites (PERMDISP, P(perm) = 0.016). For individual elements, there were significant differences in concentrations among sites for I, Mo, Na and Sr (Supplementary Table S2), with higher concentrations of I and Sr at Great Taylor Bay and Mo and Na in samples from Okehampton Bay (Supplementary Table S4). The elements Sr, Mo, Ni, Na, Fe, P and Ge varied significantly among depths (Supplementary Table S2), all of which were higher in samples from 5-m than 1-m depth (Supplementary Table S4).
3.4.4.2 Heavy metal ion content
Overall, the concentrations of the toxic heavy metal ions Al, As, Cd, Hg and Pb were low in all samples, within the limits for human consumption (Supplementary Tables S4, S5). There was, however, significant temporal and spatial variation (related to site but not depth) in the overall composition of metals, unaffected by differences in dispersion (Supplementary Table S3; PERMDISP, P(perm) > 0.05 for all comparisons). Whilst there was overlap in metal ion composition among harvest times (Figure 6B), composition varied significantly among some months: July and August, August and September and August and November (pair-wise test on interaction term; t=0.003, P(MC)=0.009; t=0.006, P(MC)=0.021; t=0.002, P(MC)=0.004, respectively). Differences among both harvest times and sites were most strongly influenced by Al (SIMPER % Cum ranged between 75 and 86%). Individually, all metals varied significantly over time (Supplementary Table S2), with an increase from July to October of Hg, a decrease of Pb content in the same interval, and the highest value for Cd, As and Al reported in August (Supplementary Table S4). Pb, Hg and Al differed significantly among sites (Supplementary Table S2) where Hg was higher in samples from Great Taylor Bay compared to Okehampton Bay while Al and Pb higher in samples from Okehampton Bay. However, concentrations of Al, Cd and Pb were statistically higher at 5-m depth compared to 1-m depth (one-way ANOVA, Tukey’s test P < 0.05).
3.4.5 Fatty acids
There was significant temporal, but not spatial (site nor depth), variation in the overall FA composition of cultivated M. pyrifera (Supplementary Table S3, PERMDISP, P(perm) > 0.05; Figure 6C). The spring and winter months clearly separated, with no separation within the two seasons groups (P(MC) > 0.05 for all pairwise comparisons within seasons). Differences between the most dissimilar months were influenced by 16:0, SFA, MUFA (July and September, 70.77% Cum), 18:4 n-3,16:0, SFA (July and October, 73.5% Cum), MUFA, 18:4 n-3, 18:0, 16:0 (July and November, 72.19% Cum). Individually, temporal patterns differed among FA classes with some FA increasing over the harvest season from July to November (e.g. SFA), some decreasing over the harvest season (e.g. n-6:n-3 ratio) or increasing in October (e.g. n-3 PUFA, n-3 LC PUFA), while others did not significantly vary over time (e.g. MUFA, n-6 PUFA, n-6 LC PUFA; Supplementary Tables S2, S4). Although the overall FA composition did not differ spatially, the n-6:n-3 ratio was significantly higher in Okehampton Bay and at 1-m depth, whilst MUFA and SFA were significantly higher in Great Taylor Bay (Supplementary Tables S2, S4). Similarly, n-6 LC PUFA, n-6 PUFA, 20:4 n-6, 18:2 n-6 were significantly higher at 1-m compared to 5-m depth (Supplementary Tables S2, S4).
4 Discussion
The results of this study suggest that a winter harvest, between July and August, for the two sites investigated, should maximise the quality of Macrocystis pyrifera cultivated in Tasmanian coastal waters with respect to both biofouling and biochemical composition, without compromising the yield. Additionally, cultivation at 1-m may lead to greater production than at 5-m depth. Moreover, the biochemical composition of the cultured biomass appeared suitable for further commercial applications (e.g. food and feed). However, complex interactions in growth and composition between season, site and depth may warrant further investigation in future studies, alongside further optimisation of culture conditions to reduce the potential for biofouling and maximise high-quality yields.
4.1 Yield and biofouling of cultivated Macrocystis pyrifera
Yield and quality of cultured M. pyrifera biomass can be affected by environmental conditions such as light, temperature, nitrogen and water motion (e.g., Camus et al., 2018). In the present study, site variation was not statistically significant, and the yield did not significantly increase throughout the harvest period. This could be due to an increase in biofouling that negatively impacted the cultured biomass, especially later in the season. Moreover, the available nutrients decreased during the culture period, which may have limited algal growth. Interestingly, the yield was higher at the shallower depth (1 m) compared to a deeper depth (5 m), which contrasts with the optimal yields in deeper water (3 m) previously reported in Chile (Buschmann et al., 2007). Furthermore, the mean yield (± SEM) reported in this study (1.20 ± 0.1 kg m-1) was lower compared to previous studies (Gutierrez et al., 2006; Westermeier et al., 2006; Buschmann et al., 2007). This difference in yield is likely linked to spatial and temporal variability in light and nutrient availability between Tasmanian and Chilean coastal waters, or suboptimal hatchery conditions (e.g., fouling contaminants). Moreover, the Great Taylor Bay site experiences the influx of tannin-rich river run-off water from the nearby Huon River which temporarily reduces light penetration at the site (see Figure 2B). Our results indicate that a relatively early harvest (between July and August) would ensure high-quality biomass without a loss of productivity for M. pyrifera cultivated in the areas investigated.
To optimise the commercial production of seaweed it is essential to minimise or manage the biofouling on the cultivated biomass. The effect and presence of biofouling has been shown to vary with season, seawater temperature, wave exposure, irradiance, and nutrient availability (e.g., Bannister et al., 2019; Visch et al., 2020). We observed an increase in biofouling on cultivated biomass of M. pyrifera correlated with increasing seawater temperatures independent of site or depth variation; consistent with previous studies that have recommended harvesting before rising seawater temperatures promote increased biofouling (Park and Hwang, 2012; Ateweberhan et al., 2015; Førde et al., 2015; Marinho et al., 2015; Keesing et al., 2016). We suggest an early harvest of M. pyrifera during the cooler winter months (July and August), coupled with both high-standard practices in the hatchery cultivation process to avoid contamination in the earlier stages and prior to deployment, whilst an earlier deployment (between February and March) may maximise the window of production for optimal biomass before the warmer spring/summer waters promote increased biofouling.
4.2 Nutritional profile
Existing commercialisation of M. pyrifera in feed, nutraceuticals, pharmaceuticals, food and functional foods in various countries (Purcell-Meyerink et al., 2021) suggests that this species represents a good candidate for aquaculture and further biochemical investigations to address potentially interesting compounds for commercial application in Australia. Overall, the results of this study suggest that the nutritional profiles of M. pyrifera cultivated in Tasmania are suitable for potential use as food and feed. However, consistent with previous studies for this region (Biancacci et al., 2022), the relatively high iodine content of M. pyrifera might represent a barrier to applications in these sectors, unless post-harvest processing can reduce iodine concentrations in the final products.
4.2.1 Proximate composition, elemental, and total phenolic content
As C and N are the building blocks of carbohydrates and proteins, respectively, their availability in the environment and tissues can affect the proximate composition of cultivated biomass. For example, the protein content in seaweeds can be positively correlated with seawater nitrogen concentration, and assimilated nitrogen can be stored in internal reserves, which is usually depleted or diluted during growth (Gorham and Lewey, 1984). In this study, the carbohydrate content (as NFE) increased from spring to summer and (between 34 - 53% DW as NFE) was similar to values previously reported for M. pyrifera (between 30 - 70% w/w DW, Holdt and Kraan, 2011). Carbon content also increased from winter to spring, reflecting the increased production of carbohydrates (Gordillo et al., 2002). Protein content was within the ranges previously reported for M. pyrifera (e.g.: between 5 - 13%, Ortiz et al., 2009; Biancacci et al., 2022), and both protein and N decreased from July to November. Thus, C:N ratios increased from winter to spring and ranged from 9 to 26.40. A C:N ratio of < 20 indicates nitrogen sufficiency, whereas, C:N > 25 indicates nitrogen-limited growth in M. pyrifera (Hurd et al., 1996). In southern New Zealand and British Columbia, Canada, tissue nitrogen of M. pyrifera is 3 - 4% in winter, compared to 1 - 1.5% in winter in Tasmanian M. pyrifera (Hurd et al., 1996; Brown et al., 1997; Smart et al., 2022). The C:N ratios of M. pyrifera in Tasmania suggest they are mildly N-limited in winter compared to the same species in New Zealand and Canada, and they become progressively more nitrogen limited as the seasons progressed from spring to summer.
In addition to temporal changes in proximate composition, we also reported spatial variation relative to depth and site. These spatial differences in proximate composition might be related to differences in water motion, nutrients, and light availability, particularly if we look at the carbohydrate content. This was higher at 1-m depth, where light intensity was higher, presumably enhancing photosynthesis and carbohydrate production (Chapman and Craigie, 1978). Similarly, C:N ratios were higher in samples collected at 1-m depth compared to 5-m and higher in Great Taylor Bay compared to Okehampton Bay, possibly due to the nutrient distribution in the water column, seawater temperature, and site variability, as previously reported in other studies (e.g., Hurd, 2017). Spatial differences highlighted in this study might be important to consider when scaling up investment in seaweed aquaculture in this region.
4.2.1.1 Lipid and fatty acids
Lipid content in seaweeds vary according to species, maturity, and/or seasonality (Castro-González et al., 1994; Nelson et al., 2002; Hernández-Carmona et al., 2009). Higher levels of lipids are usually common in cold habitats and/or seasons (Gómez and Westermeier, 1995), as reported in this study where lipids were statistically higher in winter samples compared to spring ones. However, overall, lipid levels were low, as already reported for other brown algae (between 1.5 and 3.6% DW; Rohani-Ghadikolaei et al., 2012) and preliminary cultivation trials of M. pyrifera in this region (between 0.5 and 2% DW, Biancacci et al., 2022).
Characterisation of the fatty acid profiles of seaweeds can aid the development of new sources of healthful diets for human consumption as well as aquaculture feed (e.g. for abalone and finfish, Durazo-Beltrán et al., 2003). Generally, seaweeds have a low-fat content but a unique profile of fatty acids including long-chain polyunsaturated essential fatty acids from the n-3 family (n-3 LC-PUFA), such as eicosapentaenoic acid (EPA, 20:5n-3) (Johns et al., 1979; Mishra et al., 1993; Khotimchenko et al., 2002). These make them ideal candidates for further applications (such as food and feed) (Ortiz et al., 2009). The main fatty acids reported for M. pyrifera in this study included, in decreasing order of abundance: SFA, MUFA, n-3 PUFA and LC PUFA, n-6 PUFA and EPA. The n-6:n-3 ratio was between 0.20 and 0.83, considerably lower than that reported for some other seaweeds and terrestrial plants, and well below the recommended upper limit of 10 (Dubois et al., 2007; Kumari et al., 2013; Schmid et al., 2018), suggesting potential application in sustainable dietary supplements to reduce the n-6:n-3 ratio (Mahan and Escott-Stump, 2003) compared to land crops and fish oil (Pauly et al., 2005; Dubois et al., 2007). However, overall low concentrations of lipids may be a barrier to such application, such that future studies that focus on breeding and post-processing methods to increase total lipid content in M. pyrifera are recommended.
Variation in fatty acid contents can depend on seasonal and population differences (Nelson et al., 2002). The main drivers reported previously in other brown seaweeds include nutrient availability (Gómez and Wiencke, 1998; Gordillo et al., 2001), water temperature (Al-Hasan et al., 1991; Floreto et al., 1993), variation in light level (Hotimchenko, 2002) and/or salinity (Kumar et al., 2010). In this study, variations in FA contents were mainly due to temporal variation, with overall higher average values reported in spring and lower in winter, contrary to what was observed in the total lipid content and to what was previously reported for FA content in other brown algae (e.g., Undaria pinnatifida, Gerasimenko et al., 2010), while n-6:n-3 ratios were higher in winter samples compared to those collected in spring. In this case, other factors, besides light and temperature, might be at play, such as age and growth stage.
4.2.1.2 Ash and dietary minerals
Seaweeds can accumulate minerals from the environment, in different quantities and composition according to variation in species, season, site and growth stage (Hou and Yan, 1998; Brown et al., 1999; Van Netten et al., 2000). Due to this capacity, ash content is generally high in seaweeds (between 8 – 40% DW). The values reported in this study were between 37 and 53% DW, which were slightly higher (between 25 - 40% DW, Leyton et al., 2016) or similar (between 36 and 50% DW, Westermeier et al., 2012) than the values reported by previous studies.
In this study, dietary mineral composition in cultivated M. pyrifera varied temporally. Minerals more concentrated in decreasing order were K> Na> Mg> Ca> P>I> Sr>Fe. Levels of minerals were similar to values reported previously for M. pyrifera (Biancacci et al., 2022) and for other brown algae (Smith et al., 2010); however higher levels of Na were reported in this study and lower values for Ca and I. The high level of Na could be explained by insufficient rinsing of the processed material prior to drying. Essential dietary minerals in trace amounts included in decreasing order were Zn>Mn>Se>Cu>Ni>Cr>Co, some of them particularly important for various biological and metabolomic functions such as the formation of protein (e.g., Se), enzyme function (e.g., Zn), regulation of insulin response (e.g., Cr) and metabolism of lipids, amino acid and carbohydrates (e.g., Mn) (Smith et al., 2010). Overall, minerals levels fell withing the reference daily intake (RDI) levels recommended by WHO for human consumption, including Ca, Na, Fe, and K. However, average levels of I reported here for cultivated M. pyrifera (1,100 ± 120 SD mg kg-¹) exceeded the RDI and were, on average, above the maximum tolerable level set for algal food in Australia (1000 mg kg-¹ DW, FSANZ, 2012), ranging from 690 - 1365 mg kg-1 (Supplementary Table S4). Harvested biomass could be further processed (e.g. boiled for a certain time, (Nielsen et al., 2020)) in order to reduce the content of iodine. However, whilst high, I values in this study were lower than previous results reported for this species and for other kelps (<4000 mg kg-1, Smith et al., 2010; < 4700 mg kg-1, Biancacci et al., 2022).
4.2.1.3 Total phenolic content
Phenolics are important antioxidants and prevent physiological stress derived from exposure to high light, UV, and desiccation stresses (Ragan and Glombitza, 1986; Schoenwaelder, 2002; Connan et al., 2007; Cruces et al., 2012). TPCs in M. pyrifera samples were overall low, ranging from 1.20 - 1.70 mg g-1 DW, but within the values reported previously for this species (between 1.20 - 1.40 mg g-1 DW, Umanzor et al., 2020). Slightly higher values were reported for September compared to the other months. However, this growing trend did not continue in the following spring months, possibly due to the increasing biofouling on the cultivated biomass.
4.2.2. Pigments
Fucoxanthin along with chlorophylls and β-carotene, play an important role in light-harvesting, photoprotection, and upregulation of photosynthesis in brown seaweeds (D’Orazio et al., 2012; McKew et al., 2013). Due to the large gradient occupied in the water column, some seaweeds, such as M. pyrifera, can photo-acclimate and respond according to the position in the water column (Colombo‐Pallotta et al., 2006), and to the decreased light intensity with depth (Kirk, 1992). Surprisingly, the pigment concentrations in this study were not affected by depth. Overall, concentrations of pigments in the cultivated M. pyrifera biomass were within the range reported for the species, with Chl-c more abundant than Chl-a or fucoxanthin (Supplementary Table S4). Chl-a contents were lower or similar to those reported previously (between 0.1 - 0.5 mg g -1, Mabin et al., 2019), while fucoxanthin and Chl-c contents were higher for the samples analysed in this study (between 0.3 - 0.9 and 2 - 2.4 mg g -1, respectively, Koch et al., 2016). Temporal variations were reported in this study for fucoxanthin and Chl-a that decreased from winter to spring harvests, probably due to the increased biofouling on the blades and a concomitant reduction in light availability. Indeed, previous studies have found that seasonality in fucoxanthin contents is mainly due to light exposure and temperature fluctuations (Nomura et al., 2013).
4.2.3. Heavy metal ion content
Concentrations of heavy metals in seaweed-based foods are regulated by intake limits in various countries (e.g., Europe, Australia and New Zealand, Almela et al., 2002; FSANZ, 2019), particularly for As, an abundant element in seaweeds, often bound into organic molecules (e.g., arsenosugars), which are less toxic compared to the inorganic forms (Andrewes et al., 2004). FSANZ (2013) recommends a maximum level (ML) of 1 mg kg-1 DW of seaweed and seaweed-containing foods for inorganic arsenic. Whilst we did not measure the concentration of inorganic arsenic in this study, the total arsenic content ranged from 62 to 100 mg kg-1, which is similar to previous data reported for this species and other laminarian seaweeds, but lower than Sargassum fusiforme (a fucoid seaweed known for high As levels) (< 117 mg kg -1, Almela et al., 2006; < 97 mg kg -1, Smith et al., 2010; < 105 mg kg -1, Biancacci et al., 2022).
Marine organisms can also have relatively high levels of mercury compared to terrestrial foods with toxicity highest for inorganic and methyl forms of mercury (Neff, 2002). Provisional Tolerable Weekly Intake (PTWI) levels have been set for inorganic Hg and methylmercury at 4 µg kg-1 BW per week and 1.6 µg kg-1 BW per week, respectively (FSANZ, 2019). Again, we did not measure speciation in mercury but the values reported in this study for total Hg ranged from 0.68 - 1.60 mg kg-1 DW of seaweed and do not pose a significant risk to human health based on conservative estimates of toxicity of inorganic Hg and methylmercury (Supplementary Table S5).
Overall, heavy metal ion concentrations in the cultivated M. pyrifera in this study showed temporal and spatial variability, but all were below toxicity limits based on a suggested weekly consumption between 15 – 24-g DW M. pyrifera, with arsenic as the limiting factor (Supplementary Table S5). A weekly consumption limit of 15 – 24 g DW is significantly lower than the previously suggested daily intake of 10-g DW seaweeds for omnivores and 20 – 30 g DW for vegans based on protein and FA contents for optimal health (Skrzypczyk et al., 2019). However, our weekly consumption estimate is conservatively calculated against the tolerable limits for the toxic inorganic forms of As (rather than total As as measured in our study), such that future studies that examine arsenic speciation to quantify the concentration of inorganic As in cultivated M. pyrifera may allow this recommended maximum weekly consumption level to be revised to optimise the health benefits of consuming this kelp, whilst minimising toxicity risks. In addition, post-harvest processing and cooking methods may also reduce the metal concentrations (including both total and inorganic As) in seaweeds and seaweed-containing foods (Noriega-Fernández et al., 2021), influencing the recommended weekly intake limits for human consumption, and thus warrant further investigation.
5 Conclusion
In this study, we present the result of investigations on the temporal and spatial variability in yield, biofouling and biochemical composition (including proximate composition, fatty acids, dietary minerals, heavy metal profiling, C, N, H, S concentrations and C:N ratio, antioxidants (phenolic compounds), and pigments (Chl-a, Chl-c, fucoxanthin)) of M. pyrifera cultivated in Tasmania, southeastern Australia, between July – November 2020. All results converge to suggest an optimal harvest period in winter months (July – August) to limit biofouling and ensure good quality biomass without reducing the overall biomass productivity. The biochemical composition varied temporally and spatially for many of the components analysed. Overall, the lipid content of M. pyrifera was low, consistent with other kelp species, but the FA profiles were favourable for consumption by humans for associated health benefits (particularly for n-3 LC PUFA); although concentrations were below recommended daily intake levels. Results reported for fucoxanthin content are in line with previous studies and with current applications of M. pyrifera as a nutraceutical. Minerals were within the RDI levels, except for iodine, which was slightly above the tolerable content for dried seaweed products (FSANZ, 2012), but still low compared to other brown algae, commercialised and not. Metals did not represent a health concern, based on a suggested weekly consumption for humans between 15 – 24-g DW M. pyrifera cultivated in Tasmania. Opportunities exist for the hatchery and at-sea farming to be further optimized (with attention to tailoring incubation conditions and control/mitigate contaminants) to ensure increased production of high-quality biomass. Future studies repeating these analyses across multiple years, will allow us to assess the generality of harvesting in winter for optimal quality and production of cultivated M. pyrifera. Overall, these results show that cultivation of M. pyrifera in Tasmania is possible and that this species may represent a viable candidate for further commercial applications. However, given the demonstrated vulnerability of M. pyrifera populations to increasing ocean temperatures in eastern Tasmania, and the correlations between declining quality of biomass with increasing temperature reported in this study, future research efforts should also seek to optimise aquaculture production, potentially focusing on heat-resistant/tolerant strains, of M. pyrifera in a warming climate to ensure viability of investment.
Data availability statement
The raw data supporting the conclusions of this article will be made available by the authors, without undue reservation.
Author contributions
CB, WV, CH, JCS, GF, BE, PL, CM, and AB contributed to conception and design of the study. BE, CM, PL, CH and AB lead the funding acquisition and project management. GF, JCS, WV, CB, JS, AN, AM, DC and DF were involved in the field and/or laboratory data acquisition. CH, AB, DC and DF provided resources for the biochemical analysis. PL and CH provided resources for hatchery culture. CB, WV, and AB performed the statistical analysis. CB and WV are co-lead authors and contributed equally to the preparation of the manuscript from the first draft through to completion, under the guidance of senior author AB. All other authors contributed to writing of the manuscript through providing feedback on drafts, and all read and approved the final submitted version.
Funding
This research was funded by the “Seaweed solutions for sustainable aquaculture CRC Project” (CRCPSIX000144) funded by the Australian government, Tassal P/L, Spring Bay Seafoods, Institute for Marine and Antarctic Sciences/University of Tasmania and Deakin University.
Conflict of interest
The authors declare the following financial interests/personal relationships which may be perceived as potential conflicts interests: The Seaweed Solutions for Sustainable Aquaculture CRC project (CRCPSIX000144) that funded this research included financial contributions from both Tassal Group Ltd and Spring Bay Seafoods. Pty Ltd. Authors JCS and BE report relationships with Tassal Group Ltd. Author PL reports a relationship with Spring Bay Seafoods Pty Ltd that include employment at the time of the study. All were involved in the study design and writing of the manuscript and JCS was also involved in data acquisition. The following authors were supported financially through the Seaweed Solutions for Sustainable Aquaculture CRC project, via employment (CB, WV and GF) or PhD scholarships (JS and AN) during the time of this study.
The remaining authors declare that the research was conducted in the absence of any commercial or financial relationships that could be construed as a potential conflict of interest.
Publisher’s note
All claims expressed in this article are solely those of the authors and do not necessarily represent those of their affiliated organizations, or those of the publisher, the editors and the reviewers. Any product that may be evaluated in this article, or claim that may be made by its manufacturer, is not guaranteed or endorsed by the publisher.
Supplementary material
The Supplementary Material for this article can be found online at: https://www.frontiersin.org/articles/10.3389/fmars.2022.951538/full#supplementary-material
References
Alexandratos N., Bruinsma J. (2012). World agriculture towards 2030/2050: the 2012 revision. ESA working paper no. 12-03 (Rome: FAO).
Al-Hasan R. H., Hantash F. M., Radwan S. S. (1991). Enriching marine macroalgae with eicosatetraenoic (arachidonic) and eicosapentaenoic acids by chilling. Appl. Microbiol. Biotechnol. 35, 530–535. doi: 10.1007/BF00169763
Almela C., Algora S., Benito V., Clemente M., Devesa V., Suner M., et al. (2002). Heavy metal, total arsenic, and inorganic arsenic contents of algae food products. J. Agric. Food Chem. 50, 918–923. doi: 10.1021/jf0110250
Anderson M. J., Gorley R. N., Clarke K. R. (2008). PERMANOVA+ for PRIMER: guide to software and statistical methods. Primer-e. Plymouth. UK. 32, 87–91.
Andrewes P., Demarini D. M., Funasaka K., Wallace K., Lai V. W., Sun H., et al. (2004). Do arsenosugars pose a risk to human health? the comparative toxicities of a trivalent and pentavalent arsenosugar. Environ. Sci. Technol. 38, 4140–4148. doi: 10.1021/es035440f
Angell A. R., Mata L., De Nys R., Paul N. A. (2016). The protein content of seaweeds: a universal nitrogen-to-protein conversion factor of five. J. Appl. Phycol. 28, 511–524. doi: 10.1007/s10811-015-0650-1
AOAC (2006). Official methods of analysis, 18th edition (Gaithersburgs, MD: Association of Official Analytical Chemists).
Ateweberhan M., Rougier A., Rakotomahazo C. (2015). Influence of environmental factors and farming technique on growth and health of farmed Kappaphycus alvarezii (cottonii) in south-west Madagascar. J. Appl. Phycol. 27, 923–934. doi: 10.1007/s10811-014-0378-3
Atkinson J. L., Hilton J. W., Slinger S. J. (1984). Evaluation of acid-insoluble ash as an indicator of feed digestibility in rainbow trout (Salmo gairdneri). Can. J. Fish. Aquat. Sci. 41, 1384–1386. doi: 10.1139/f84-170
Bannister J., Sievers M., Bush F., Bloecher N. (2019). Biofouling in marine aquaculture: a review of recent research and developments. Biofouling 35, 631–648. doi: 10.1080/08927014.2019.1640214
Bartsch I. (2018). ““Derivation of clonal stock cultures and hybridization of kelps”,” in Protocols for macroalgae research (New York, NY, USA: CRC Press), 61–78.
Biancacci C., Sanderson J., Evans B., Callahan D., Francis D., Skrzypczyk V., et al. (2022). Nutritional composition and heavy metal profiling of Australian kelps cultured in proximity to salmon and mussel farms. Algal. Res. 64, 102672. doi: 10.1016/j.algal.2022.102672
Brown M., Hodgkinson W., Hurd C. (1999). Spatial and temporal variations in the copper and zinc concentrations of two green seaweeds from otago harbour, new Zealand. Mar. Environ. Res. 47, 175–184. doi: 10.1016/S0141-1136(98)00113-5
Brown M. T., Nyman M. A., Keogh J. A., Chin N. K. M. (1997). Seasonal growth of the giant kelp Macrocystis pyrifera in new Zealand. Mar. Biol. 129, 417–424. doi: 10.1007/s002270050182
Buschmann A., Riquelme V., Hernández-González M., Henríquez L. (2007). “Additional perspectives for ecosystem approaches for aquaculture,” in Aquaculture and ecosystems: An integrated coastal and ocean management approach. (BatonRouge, LA: The World Aquaculture Society) 168–176.
Camus C., Infante J., Buschmann A. H. (2018). Overview of 3 year precommercial seafarming of Macrocystis pyrifera along the Chilean coast. Rev. Aquacult. 10, 543–559. doi: 10.1111/raq.12185
Cancino J., Mufioz J., Mufioz M., Orellana M. (1987). Effects of the bryozoan Membranipora tuberculata (Bosc.) on the photosynthesis and growth of Gelidium rex santelices et Abbott. J. Exp. Mar. Biol. Ecol. 113, 105–112. doi: 10.1016/0022-0981(87)90158-4
Castro-González M. I., Carrillo-Domínguez S., Pérez-Gil F. (1994). Chemical composition of Macrocystis pyrifera (Giant sargazo) collected in summer and winter and its possible use in animal feeding. Cienc. Mar. 20, 33–40. doi: 10.7773/cm.v20i1.955
Chapman A. R. O., Craigie J. S. (1978). Seasonal growth in Laminaria longicuris: Relations with reserve carbohydrate storage and production. Mar. Biol. 46, 209–213. doi: 10.1007/BF00390682
Cheney D. (2016). ““Toxic and harmful seaweeds. seaweed in health and disease prevention,”,” in Seaweed in health and disease prevention (San Diego: Academic Press), 407–421.
Chopin T., Tacon A. G. J. (2020). Importance of seaweeds and extractive species in global aquaculture production. Rev. Fish. Sci. Aquacult. 29, 1–10. doi: 10.1080/23308249.2020.1810626
Clarke K. R. (1993). Non-parametric multivariate analyses of changes in community structure. Aust. J. Ecol. 18, 117–143. doi: 10.1111/j.1442-9993.1993.tb00438.x
Colombo-Pallotta M. F., García-Mendoza E., Ladah L. B. (2006). Photosynthetic performance, light absorption, and pigment composition of Macrocystis pyrifera (Laminariales, phaeophyceae) blades from different depths. J. Phycol. 42, 1225–1234. doi: 10.1111/j.1529-8817.2006.00287.x
Connan S., Deslandes E., Gall E. A. (2007). Influence of day–night and tidal cycles on phenol content and antioxidant capacity in three temperate intertidal brown seaweeds. J. Exp. Mar. Biol. Ecol. 349, 359–369. doi: 10.1016/j.jembe.2007.05.028
Cruces E., Huovinen P., Gomez I. (2012). Phlorotannin and antioxidant responses upon short-term exposure to UV radiation and elevated temperature in three south pacific kelps. Photochem. Photobiol. 88, 58–66. doi: 10.1111/j.1751-1097.2011.01013.x
Dang T. T., Van Vuong Q., Schreider M. J., Bowyer M. C., Van Altena I. A., Scarlett C. J. (2017). Optimisation of ultrasound-assisted extraction conditions for phenolic content and antioxidant activities of the alga Hormosira banksii using response surface methodology. J. Appl. Phycol. 29, 3161–3173. doi: 10.1007/s10811-017-1162-y
Diamond D. (2008). “Determination of nitrate/nitrite in brackish or seawater by flow injection analysis,”. QuickChem method (Loveland, USA: LaChat Instruments).
Dixon J., Schroeter S. C., Kastendiek J. (1981). Effects of the encrusting bryozoan, Membranipora membranacea, on the loss of blades and fronds by the giant kelp, Macrocystis pyrifera (Laminariales). J. Phycol. 17, 341–345. doi: 10.1111/j.1529-8817.1981.tb00860.x
D’orazio N., Gemello E., Gammone M. A., De Girolamo M., Ficoneri C., Riccioni G. (2012). Fucoxantin: A treasure from the sea. Mar. Drugs 10, 604–616. doi: 10.3390/md10030604
Duarte C. M., Bruhn A., Krause-Jensen D. (2022). A seaweed aquaculture imperative to meet global sustainability targets. Nat. Sust. 5, 185–193. doi: 10.1038/s41893-021-00773-9
Dubois V., Breton S., Linder M., Fanni J., Parmentier M. (2007). Fatty acid profiles of 80 vegetable oils with regard to their nutritional potential. Eur. J. Lipid Sci. Technol. 109, 710–732. doi: 10.1002/ejlt.200700040
Durazo-Beltrán E., D’abramo L. R., Toro-Vazquez J. F., Vasquez-Peláez C., Viana M. (2003). Effect of triacylglycerols in formulated diets on growth and fatty acid composition in tissue of green abalone (Haliotis fulgens). Aquaculture 224, 257–270. doi: 10.1016/S0044-8486(03)00223-0
Durrant H. M., Barrett N. S., Edgar G. J., Coleman M. A., Burridge C. P. (2015). Shallow phylogeographic histories of key species in a biodiversity hotspot. Phycologia 54 (6), 556–565. doi: 10.2216/15-24.1
Dürr S., Watson D. I. (2010). Biofouling and antifouling in aquaculture. Biofouling 12, 267–287. doi: 10.1002/9781444315462.ch19
Førde H., Forbord S., Handå A., Fossberg J., Arff J., Johnsen G., et al. (2015). Development of bryozoan fouling on cultivated kelp (Saccharina latissima) in Norway. J. Appl. Phycol. 28, 1225–1234. doi: 10.1007/s10811-015-0606-5
FAO (2020). ““The state of world fisheries and aquaculture”,” in State of world fisheries and aquaculture - SOFIA 2020 (Rome: Fisheries and Aquaculture Department).
Floreto E., Hirata H., Ando S., Yamasaki S. (1993). Effects of temperature, light intensity, salinity and source of nitrogen on the growth, total lipid and fatty acid composition of Ulva pertusa kjellman (Chlorophyta). Botanica Mar. 36, 149–158. doi: 10.1515/botm.1993.36.2.149
Folch J., Lees M., Sloane Stanley G. H. (1957). A simple method for the isolation and purification of total lipids from animal tissues. J. Biol. Chem. 226, 497–509. doi: 10.1016/S0021-9258(18)64849-5
Forbord S., Steinhovden K. B., Rød K. K., Handå A., Skjermo J. (2018). “Cultivation protocol for saccharina latissima” in Protocols for macroalgae research (New York, NY, USA CRC Press), 37–59.
FSANZ, Food Standard Australia New Zealand (2012) “Survey of iodine levels in seaweed and seaweed containing products in australia”. In: . Available at: https://www.foodstandards.govt.nz/science/surveillance/documents/Iodine%20in%20Seaweed.pdf.
FSANZ, Food Standard Australia New Zealand (2013)"Survey of inorganic arsenic in seaweed and seaweed-containing products available in australia". In: . Available at: https://www.foodstandards.gov.au/science/surveillance/documents/Survey%20of%20inorganic%20arsenic%20in%20seaweed%20and%20seaweed%20.pdf.
FSANZ, Food Standard Australia New Zealand (2019)"25th Australian total diet study". In: . Available at: https://www.foodstandards.gov.au/publications/Documents/25thAustralianTotalDietStudy.pdf.
Gerasimenko N., Busarova N., Moiseenko O. (2010). Age-dependent changes in the content of lipids, fatty acids, and pigments in brown alga Costaria costata. Russian J. Plant Physiol. 57, 62–68. doi: 10.1134/S1021443710010085
Gómez I., Westermeier R. (1995). Energy contents and organic constituents in Antarctic and south Chilean marine brown algae. Pol. Biol. 15, 597–602. doi: 10.1007/BF00239653
Gómez I., Wiencke C. (1998). Seasonal changes in c, n and major organic compounds and their significance to morpho-functional processes in the endemic Antarctic brown alga Ascoseira mirabilis. Pol. Biol. 19, 115–124. doi: 10.1007/s003000050222
Gordillo F. J. L., Dring M. J., Savidge G. (2002). Nitrate and phosphate uptake characteristics of three species of brown algae cultured at low salinity. Mar. Ecol. Prog. Ser. 234, 111–118. doi: 10.3354/meps234111
Gordillo F. J., Jiménez C., Goutx M., Niell X. (2001). Effects of CO2 and nitrogen supply on the biochemical composition of Ulva rigida with especial emphasis on lipid class analysis. J. Plant Physiol. 158, 367–373. doi: 10.1078/0176-1617-00209
Gorham J., Lewey S. (1984). Seasonal changes in the chemical composition of Sargassum muticum. Mar. Biol. 80, 103–107. doi: 10.1007/BF00393133
Guillard R. R., Ryther J. H. (1962). Studies of marine planktonic diatoms: I. Cyclotella nana hustedt, and Detonula confervacea (Cleve) gran. Can. J. Microbiol. 8, 229–239. doi: 10.1139/m62-029
Gutierrez A., Correa T., Muñoz V., Santibañez A., Marcos R., Cáceres C., et al. (2006). Farming of the giant kelp Macrocystis pyrifera in southern Chile for development of novel food products. J. Appl. Phycol. 18, 259–267. doi: 10.1007/s10811-006-9025-y
Hernández-Carmona G., Carrillo-Domínguez S., Arvizu-Higuera D. L., Rodríguez-Montesinos Y. E., Murillo-Álvarez J. I., Muñoz-Ochoa M., et al. (2009). Monthly variation in the chemical composition of Eisenia arborea JE areschoug. J. Appl. Phycol. 21, 607–616. doi: 10.1007/s10811-009-9454-5
Holdt S., Kraan S. (2011). Bioactive compounds in seaweed: functional food applications and legislation. J. Appl. Phycol. 23, 543–597. doi: 10.1007/s10811-010-9632-5
Hotimchenko S. (2002). Fatty acid composition of algae from habitats with varying amounts of illumination. Russian J. Mar. Biol. 28, 218–220. doi: 10.1023/A:1016861807103
Hou X., Yan X. (1998). Study on the concentration and seasonal variation of inorganic elements in 35 species of marine algae. Sci. Tot. Environ. 222, 141–156. doi: 10.1016/S0048-9697(98)00299-X
Hurd C. L. (2000). Water motion, marine macroalgal physiology, and production. J. Phycol. 36, 453–472. doi: 10.1046/j.1529-8817.2000.99139.x
Hurd C. L. (2017). Shaken and stirred: the fundamental role of water motion in resource acquisition and seaweed productivity. Perspect. Phycol. 4, 73–81. doi: 10.1127/pip/2017/0072
Hurd C., Harrison P., Druehl L. (1996). Effect of seawater velocity on inorganic nitrogen uptake by morphologically distinct forms of Macrocystis integrifolia from wave-sheltered and exposed sites. Mar. Biol. 126, 205–214. doi: 10.1007/BF00347445
Johns R. B., Nichols P. D., Perry G. J. (1979). Fatty acid composition of ten marine algae from australian waters. Phytochemistry 18, 799–802. doi: 10.1016/0031-9422(79)80018-7
Keesing J. K., Liu D., Shi Y., Wang Y. (2016). Abiotic factors influencing biomass accumulation of green tide causing Ulva spp. on Pyropia culture rafts in the yellow Sea, China. Mar. pollut. Bull. 105, 88–97. doi: 10.1016/j.marpolbul.2016.02.051
Kelly J. (2020) Australian Seaweed industry blueprint – a blueprint for growth, AgriFutures australia. publication no. 20-072. Available at: https://www.agrifutures.com.au/wp-content/uploads/2020/09/20-072.pdf.
Khotimchenko S. V., Vaskovsky V. E., Titlyanova T. V. (2002). Fatty acids of marine algae from the pacific coast of north California. Botanica Mar. 45, 17–22. doi: 10.1515/BOT.2002.003
Kirk J. T. O. (1992). ““The nature and measurement of the light environment in the ocean,”,” in Primary productivity and biogeochemical cycles in the Sea (Boston, MA, USA: Springer), 9–29.
Koch K., Thiel M., Hagen W., Graeve M., Gómez I., Jofre D., et al. (2016). Short-and long-term acclimation patterns of the giant kelp Macrocystis pyrifera (Laminariales, phaeophyceae) along a depth gradient. J. Phycol. 52, 260–273. doi: 10.1111/jpy.12394
Krumhansl K. A., Lee J. M., Scheibling R. E. (2011). Grazing damage and encrustation by an invasive bryozoan reduce the ability of kelps to withstand breakage by waves. J. Exp. Mar. Biol. Ecol. 407, 12–18. doi: 10.1016/j.jembe.2011.06.033
Kruskal J. B. (1964). Nonmetric multidimensional scaling: a numerical method. Psychometrika 29, 115–129. doi: 10.1007/BF02289694
Kumari P., Bijo A., Mantri V. A., Reddy C., Jha B. (2013). Fatty acid profiling of tropical marine macroalgae: an analysis from chemotaxonomic and nutritional perspectives. Phytochemistry 86, 44–56. doi: 10.1016/j.phytochem.2012.10.015
Kumar M., Kumari P., Gupta V., Reddy C., Jha B. (2010). Biochemical responses of red alga Gracilaria corticata (Gracilariales, rhodophyta) to salinity induced oxidative stress. J. Exp. Mar. Biol. Ecol. 391, 27–34. doi: 10.1016/j.jembe.2010.06.001
Leyton A., Pezoa-Conte R., Barriga A., Buschmann A., Mäki-Arvela P., Mikkola J.-P., et al. (2016). Identification and efficient extraction method of phlorotannins from the brown seaweed Macrocystis pyrifera using an orthogonal experimental design. Algal. Res. 16, 201–208. doi: 10.1016/j.algal.2016.03.019
Liao N. (2008). “Determination of ammonia in brackish or seawater by flow injection analysis”. QuickChem method (Loveland, USA: LaChat Instruments).
Lourenço S. O., Barbarino E., De-Paula J. C., Pereira L. O. D. S., Marquez U. M. L. (2002). Amino acid composition, protein content and calculation of nitrogen-to-protein conversion factors for 19 tropical seaweeds. Phycol. Res. 50, 233–241. doi: 10.1111/j.1440-1835.2002.tb00156.x
Lüning K., Mortensen L. (2015). European Aquaculture of sugar kelp (Saccharina latissima) for food industries: iodine content and epiphytic animals as major problems. Botanica Mar. 58, 449–455. doi: 10.1515/bot-2015-0036
Mabin C. J., Johnson C. R., Wright J. T. (2019). Physiological response to temperature, light, and nitrates in the giant kelp Macrocystis pyrifera from Tasmania, Australia. Mar. Ecol. Prog. Ser. 614, 1–19. doi: 10.3354/meps12900
Macaya E. C., Zuccarello G. C. (2010). Genetic structure of the giant kelp Macrocystis pyrifera along the southeastern pacific. Mar. Ecol. Prog. Ser. 420, 103–112. doi: 10.3354/meps08893
Mahan K. L., Escott-Stump S. (2003). ““Krause’s food, nutrition and diet therapy.”,” (Philadelphia, PA, USA: Saunders), 1115.
Marinho G. S., Holdt S. L., Birkeland M. J., Angelidaki I. (2015). Commercial cultivation and bioremediation potential of sugar kelp, Saccharina latissima, in Danish waters. J. Appl. Phycol. 27, 1963–1973. doi: 10.1007/s10811-014-0519-8
Marroig R. G., Reis R. P. (2011). Does biofouling influence Kappaphycus alvarezii (Doty) doty ex Silva farming production in Brazil? J. Appl. Phycol. 23, 925–931. doi: 10.1007/s10811-010-9602-y
Marroig R. G., Reis R. P. (2016). Biofouling in Brazilian commercial cultivation of Kappaphycus alvarezii (Doty) doty ex p. c. Silva. J. Appl. Phycol. 28, 1803–1813. doi: 10.1007/s10811-015-0713-3
Mckew B. A., Lefebvre S. C., Achterberg E. P., Metodieva G., Raines C. A., Metodiev M. V., et al. (2013). Plasticity in the proteome of Emiliania huxleyi CCMP 1516 to extremes of light is highly targeted. New Phytol. 200, 61–73. doi: 10.1111/nph.12352
Mishra V. K., Temelli F., Ooraikul B., Shacklock P. F., Craigie J. S. (1993). Lipids of the red alga, Palmaria palmata. Botanic. Mar. 36, 169–174. doi: 10.1515/botm.1993.36.2.169
Neff J. (2002). ““Mercury in the ocean,”,” in Bioaccumulation in marine organisms: Effect of contaminants from oil well produced water (Oxford, UK: Elsevier), 103–130.
Nelson M., Phleger C., Nichols P. (2002). Seasonal lipid composition in macroalgae of the northeastern pacific ocean. Botanica Mar. 45, 58–65. doi: 10.1515/BOT.2002.007
Nielsen C. W., Holdt S. L., Sloth J. J., Marinho G. S., Sæther M., Funderud J., et al. (2020). Reducing the high iodine content of Saccharina latissima and improving the profile of other valuable compounds by water blanching. Foods 9, 569. doi: 10.3390/foods9050569
Nomura M., Kamogawa H., Susanto E., Kawagoe C., Yasui H., Saga N., et al. (2013). Seasonal variations of total lipids, fatty acid composition, and fucoxanthin contents of Sargassum horneri (Turner) and Cystoseira hakodatensis (Yendo) from the northern seashore of Japan. J. Appl. Phycol. 25, 1159–1169. doi: 10.1007/s10811-012-9934-x
Noriega-Fernández E., Sone I., Astráin-Redín L., Prabhu L., Sivertsvik M., Álvarez I., et al. (2021). Innovative ultrasound-assisted approaches towards reduction of heavy metals and iodine in macroalgal biomass. Foods 10, 649. doi: 10.3390/foods10030649
Ortiz J., Uquiche E., Robert P., Romero N., Quitral V., Llantén C. (2009). Functional and nutritional value of the Chilean seaweeds Codium fragile, Gracilaria chilensis and Macrocystis pyrifera. Eur. J. Lipid Sci. Technol. 111, 320–327. doi: 10.1002/ejlt.200800140
Park C. S., Hwang E. K. (2012). Seasonality of epiphytic development of the hydroid Obelia geniculata on cultivated Saccharina japonica (Laminariaceae, phaeophyta) in Korea. J. Appl. Phycol. 24, 433–439. doi: 10.1007/s10811-011-9755-3
Pauly D., Watson R., Alder J. (2005). Global trends in world fisheries: impacts on marine ecosystems and food security. Philos. Trans. R. Soc.: Biol. Sci. 360, 5–12. doi: 10.1098/rstb.2004.1574
Purcell-Meyerink D., Packer M. A., Wheeler T. T., Hayes M. (2021). Aquaculture production of the brown seaweeds Laminaria digitata and Macrocystis pyrifera: Applications in food and pharmaceuticals. Molecules 26, 1306. doi: 10.3390/molecules26051306
Ragan M. A., Glombitza K. W. (1986). Phlorotannins, brown algal polyphenols. Prog. Phycol. Res. 4, 177–241.
Rohani-Ghadikolaei K., Abdulalian E., Ng W.-K. (2012). Evaluation of the proximate, fatty acid and mineral composition of representative green, brown and red seaweeds from the Persian gulf of Iran as potential food and feed resources. J. Food Sci. Technol. 49, 774–780. doi: 10.1007/s13197-010-0220-0
Rolin C., Inkster R., Laing J., Mcevoy L. (2017). Regrowth and biofouling in two species of cultivated kelp in the Shetland islands, UK. J. Appl. Phycol. 29, 2351–2361. doi: 10.1007/s10811-017-1092-8
Schmid M., Kraft L. G., van der Loos L. M., Kraft G. T., Virtue P., Nichols P. D., et al. (2018). Southern Australian seaweeds: a promising resource for omega-3 fatty acids. Food Chem. 265, 70–77. doi: 10.1016/j.foodchem.2018.05.060
Schoenwaelder M. E. (2002). Physode distribution and the effect of thallus sunburn in Hormosira banksii (Fucales, phaeophyceae). Botanica Mar. 45, 262–266. doi: 10.1515/BOT.2002.025
Searchinger T., Waite R., Hanson C., Ranganathan J., Dumas P., Matthews E., et al. (2019). ““Creating a sustainable food future: A menu of solutions to feed nearly 10 billion people by 2050. final report”,” (Washington, DC, USA: World Resources Report).
Seely G. R., Duncan M. J., Vidaver W. E. (1972). Preparative and analytical extraction of pigments from brown algae with dimethyl sulfoxide. Mar. Biol. 12, 184–188. doi: 10.1007/BF00350754
Skrzypczyk V. M., Hermon K. M., Norambuena F., Turchini G. M., Keast R., Bellgrove A. (2019). Is Australian seaweed worth eating? nutritional and sensorial properties of wild-harvested Australian versus commercially available seaweeds. J. Appl. Phycol. 31, 709–724. doi: 10.1007/s10811-018-1530-2
Smart J. N., Schmid M., Paine E. R., Britton D., Revill A., Hurd C. L. (2022). Seasonal ammonium uptake kinetics of four brown macroalgae: Implications for use in integrated multi-trophic aquaculture. J. Appl. Phycol. 34, 1693–1708. doi: 10.1007/s10811-022-02743-w
Smith J., Summers G., Wong R. (2010). Nutrient and heavy metal content of edible seaweeds in new Zealand. New Z. J. Crop Hortic. Sci. 38, 19–28. doi: 10.1080/01140671003619290
Stévant P., Rebours C., Chapman A. (2017). Seaweed aquaculture in Norway: recent industrial developments and future perspectives. Aquacult. Int. 25, 1373–1390. doi: 10.1007/s10499-017-0120-7
Su L., Pang S. J., Shan T. F., Li X. (2017). Large-Scale hatchery of the kelp Saccharina japonica: a case study experience at lvshun in northern China. J. Appl. Phycol. 29, 3003–3013. doi: 10.1007/s10811-017-1154-y
Tibbetts S. M., Milley J. E., Lall S. P. (2016). Nutritional quality of some wild and cultivated seaweeds: Nutrient composition, total phenolic content and in vitro digestibility. J. Appl. Phycol. 28, 3575–3585. doi: 10.1007/s10811-016-0863-y
Umanzor S., Ramírez-García M. M., Sandoval-Gil J. M., Zertuche-González J. A., Yarish C. (2020). Photoacclimation and photoprotection of juvenile sporophytes of Macrocystis pyrifera (Laminariales, phaeophyceae) under high-light conditions during short-term shallow-water cultivation. J. Phycol. 56, 380–392. doi: 10.1111/jpy.12951
Van Netten C., Cann S. H., Morley D., Van Netten J. (2000). Elemental and radioactive analysis of commercially available seaweed. Sci. Tot. Environ. 255, 169–175. doi: 10.1016/S0048-9697(00)00467-8
Visch W., Nylund G. M., Pavia H. (2020). Growth and biofouling in kelp aquaculture (Saccharina latissima): the effect of location and wave exposure. J. Appl. Phycol. 32, 3199–3209. doi: 10.1007/s10811-020-02201-5
Ways P., Hanahan D. J. (1964). Characterization and quantification of red cell lipids in normal man. J. Lipid Res. 5, 318–328. doi: 10.1016/S0022-2275(20)40200-7
Wells M. L., Potin P., Craigie J. S., Raven J. A., Merchant S. S., Helliwell K. E., et al. (2017). Algae as nutritional and functional food sources: revisiting our understanding. J. Appl. Phycol. 29, 949–982. doi: 10.1007/s10811-016-0974-5
Westermeier R., Murúa P., Patiño D. J., Muñoz L., Ruiz A., Müller D. G. (2012). Variations of chemical composition and energy content in natural and genetically defined cultivars of Macrocystis from Chile. J. Appl. Phycol. 24, 1191–1201. doi: 10.1007/s10811-011-9752-6
Westermeier R., Patiño D., Piel M., Maier I., Mueller D. G. (2006). A new approach to kelp mariculture in Chile: production of free-floating sporophyte seedlings from gametophyte cultures of Lessonia trabeculata and Macrocystis pyrifera. Aquacult. Res. 37, 164–171. doi: 10.1111/j.1365-2109.2005.01414.x
Keywords: Australia, giant kelp, macroalgae, mariculture, nutritional composition, protein, omega-3, sustainability
Citation: Biancacci C, Visch W, Callahan DL, Farrington G, Francis DS, Lamb P, McVilly A, Nardelli A, Sanderson JC, Schwoerbel J, Hurd CL, Evans B, Macleod C and Bellgrove A (2022) Optimisation of at-sea culture and harvest conditions for cultivated Macrocystis pyrifera: yield, biofouling and biochemical composition of cultured biomass. Front. Mar. Sci. 9:951538. doi: 10.3389/fmars.2022.951538
Received: 24 May 2022; Accepted: 11 July 2022;
Published: 29 July 2022.
Edited by:
Amalia Pérez-Jiménez, University of Granada, SpainReviewed by:
Alan T. Critchley, Verschuren Centre for Sustainability in Energy and Environment, CanadaLeonel Pereira, University of Coimbra, Portugal
Sophie Steinhagen, University of Gothenburg, Sweden
Copyright © 2022 Biancacci, Visch, Callahan, Farrington, Francis, Lamb, McVilly, Nardelli, Sanderson, Schwoerbel, Hurd, Evans, Macleod and Bellgrove. This is an open-access article distributed under the terms of the Creative Commons Attribution License (CC BY). The use, distribution or reproduction in other forums is permitted, provided the original author(s) and the copyright owner(s) are credited and that the original publication in this journal is cited, in accordance with accepted academic practice. No use, distribution or reproduction is permitted which does not comply with these terms.
*Correspondence: C. Biancacci, Yy5iaWFuY2FjY2lAZGVha2luLmVkdS5hdQ==; W. Visch, d291dGVyLnZpc2NoQHV0YXMuZWR1LmF1
†These authors have contributed equally to this work