- 1State Key Laboratory of Tropical Oceanography, South China Sea Institute of Oceanology, Chinese Academy of Sciences, Guangzhou, China
- 2Southern Marine Science and Engineering Guangdong Laboratory (Guangzhou), Guangzhou, China
- 3Innovation Academy of South China Sea Ecology and Environmental Engineering, Chinese Academy of Sciences, Guangzhou, China
- 4Daya Bay Marine Biology Research Station, Chinese Academy of Sciences, Shenzhen, China
- 5College of Earth and Planetary Science, University of Chinese Academy of Sciences, Beijing, China
- 6Guangzhou Chemical Reagent Factory, Guangzhou, China
- 7Guangdong Zhihuan Innovative Environmental Technology Co. Ltd, Guangzhou, China
Phytoplankton closely connects with the hydrodynamics and biogeochemical environment of the ocean. While research focusing on both the physiochemical factors and hydrodynamics regulating phytoplankton has already been conducted, the coupling mechanism between mesoscale eddies and the vertical distribution of phyto plankton in the South China Sea (SCS) is still not well understood. Here, phytoplankton was studied under one weak-cold and two warm eddies along the 18°N transect in the SCS. The results show that the vertical distribution of chlorophyll (chl-a) presented a similar pattern at all four sampling stations. The mixed layer is less than 50 m. It is the same as the meridional salinity gradients that may be distinguished above a depth of 60 m. The subsurface chlorophyll maximum at the edge of a warm (WI) and cold eddy (CI) at E413 and E411 was shallower than that at the edge of a warm eddy (WII) at E407 and E409, indicating that temperature and salinity may be the important driving factors. On the whole, mesoscale eddies had a significant influence on the vertical pattern of chl-a in the SCS during the study period.
Introduction
Phytoplankton is considered the most important marine primary producer at the bottom of the food web. They produce about half of the oxygen in the atmosphere, by photosynthesis, which is equal to the amount of oxygen produced by all land plants per year. During the period 1998–2018, global marine primary production ranged from 38.8 to 42.1 Gt C/year (Kulk et al., 2020). It is estimated that diatoms account for 45% of marine primary productivity (Mann, 1999). Phytoplankton has an important influence on marine biogeochemical cycles. Generally, phytoplankton is affected by the environment of the water column, including its physical dynamics, chemical elements, and biological activities. In coastal ecosystems, phytoplankton succession is governed largely by upwelling events, indicating the importance of mesoscale variability for the functioning of the ecosystem in the southern Benguela upwelling system (Burger et al., 2020). In the southeast Gulf of Mexico, upwelling can enhance phytoplankton growth and change its composition, resulting in phytoplankton blooms (Medina-Gomez et al., 2019).
In the open sea, short-term changes in phytoplankton communities are affected by hydrodynamics such as mesoscale eddies. Biochemical elements in cold eddies are brought to shallower waters, while in warm eddies, they are sent to deeper waters. For example, chlorophyll concentrations in cold eddies are higher than in warm eddies (depth > 70 m) in the western part of the North Pacific (Chang et al., 2017). The maximum chlorophyll a (chl-a) layer in an eddy center rises to form a dome structure, although the total chl-a biomass does not increase significantly (Wang et al., 2016). Cold and warm eddies have different influences on phytoplankton and nutrients. The cyclonic eddy characterized by enhanced nutrients and chlorophyll is mostly restricted to the subsurface waters (Jyothibabu et al., 2015). In the western South China Sea (SCS), total chl-a increases significantly in warm eddies but does not change much at cold eddies (Zhong and Huang, 2013; Xu et al., 2022). Nonetheless, in the southwestern Canada Basin, the warm core eddy with high ammonium shelf water can provide ammonium for the euphotic zone of the Basin and maintain a 30% higher biomass of picophytoplankton (<2 μm) than the surrounding waters (Nishino et al., 2011). In the SCS, both upwelling and cold eddies are characterized by high nutrient levels and chlorophyll levels, and low levels of dissolved oxygen, while warm eddies are just the opposite (Ning et al., 2004). High dissolved oxygen (DO) content is moved from the upper layer to the lower layer by warm eddies in the SCS (Liu et al., 2012). However, very few studies attempt to examine the influence of cold and warm eddies on the vertical distribution of phytoplankton maxima.
A cold core eddy is formed intermittently throughout the year due to the Kuroshio intrusion, which may have an important impact on the biogeochemical cycle in the SCS (Chen et al., 2007; Sun et al., 2022). The cold eddy significantly increases the primary productivity at the 25-m layer, which is mainly affected by the nutrients in the western part of the SCS (Leng et al., 2016). The phytoplankton features influenced by the different eddies are significantly different (Huang et al., 2010). Lin et al. (2014) used satellite data to discover that in the SCS, phytoplankton size classes change from picoplankton to micro- and nanoplankton driven by cold eddies, but the contribution of picoplankton to chlorophyll remained almost unchanged. Mesoscale eddies did not significantly influence the dominance of the dominant groups in the western SCS (Zhong and Huang, 2013).
The SCS is a large, semienclosed marginal sea with several straits that are connected to the Pacific and Indian oceans, and is characterized by complex hydrodynamics and biogeochemistry. In the northern SCS, phytoplankton in the summer is mainly driven by upwelling and river plume processes (Xu et al., 2018). Similarly, phytoplankton relatively responds to the hydrodynamics of the SCS. In the present study, the vertical distribution of phytoplankton caused by the combined action between the warm and cold eddies is explained. In particular, we explored whether the physical environment, including a thermocline, is advantageous to the formation and maintenance of chl-a at its maximum in the euphotic layer.
Materials and methods
Sampling and sample analysis
The research cruise was conducted from 15 September to 04 October 2004. During the cruise, temperatures and salinities were obtained with a SeaBird model SBE9/11. Discrete water samples (surface, 25, 50, 75, 100, 150, and 200 m) were collected with GO-FLO bottles mounted on a rosette sampling assembly (Whatman Company: General Oceanic). Seawater was filtered through a GF/F filter (Whatman, 25 mm), and the filter paper was wrapped in aluminum foil and stored at −20°C pending analysis. Chl-a content was measured by fluorescence with a Turner Design 10 fluorometer. The sampling stations of this study, located at 18°N, were collected during the cruise from 23 to 25 September 2004 (Figure 1).
Data from remote sensing
Sea-level anomaly data (SLA) obtained from the TOPEX/Poseidon and JASON altimeters (http://www.aviso.oceanobs.com/) were used to estimate surface circulations. The SLA data were used to estimate thermocline displacement. In an ideal two-layer system, the depth of the upper layer (A) is D, with density ρ1 and temperature T1 , while a motionless lower layer (B) has density ρ2=ρ1+ Δρ and temperature T2 . Δρ and Δh are the density differences between A and B and the SLA, respectively (Rebert et al., 1985). The thermocline displacement (ΔD) was calculated with Eq. (1).
Mixed layer and depth of the thermocline top
Mixed layer depth (MLD) is the depth characterized by the density gradient, and this gradient was σθ = 0.1 unit m−1 (Tseng et al., 2005). The top thermocline depth (TTD) was defined as the depth above which the temperature is greater than the surface temperature (the latter measured at 10 m depth) minus 0.2°C.
Results
Physical background
Temperature and salinity
Both the salinity and temperature profiles of the transect at 18°N, from the surface to 200 m depth, are shown in Figure 2. The surface salinity indicated that there was a high temperature (~29.5°C) and low salinity (~33.4) at the transect stations (E405 and E407), which was in good agreement with the positive SLA field. Above 50 m, there was low salinity and a high-temperature tongue from about 114.5°E towards the east. The TTD along the transect from westward to eastward (from E413 to E407) deepened. The characteristic of a warm core eddy is that there is a deep thermocline in the center and the depth of the thermocline around the eddy fringe is very shallow.
The isotherms show how the warm surface seawater (above 28°C) moved eastward, generating a meridional temperature gradient. Meridional salinity gradients may be distinguished above a depth of 60 m (Figure 2). The maximum salinity (s = 34.6) in tropical waters reached ~120 m. It is the intermediate water circulation from the west to the east in SCS.
Mixed layer and euphotic layer depth
MLD is less than 50 m in the upper layer in E411 and E413 (Figure 3). That is to say, MLD is the same water layer in E411 and E413, and the water layer at less than 50 m depth has the same temperature and salinity. On the other hand, chemical and biological parameters may show a small difference.
Sea surface height anomaly
The SLA showed that there were two warm eddies and a weak cold eddy in the sampling transect before and during the study period. The sampling stations located at the warm eddy edge or inside it (E413 located in c; and E407 and E409 located in WII) have high values of SLA, while E11 located in the cold eddy (CI) has low values of SLA (Figure 4). The mesoscale eddy changed over time. Interestingly, the WI was distinct from 28 August to 22 September and then disappeared. In contrast, the cold eddy (CI) became gradually stronger before and during the sampling period.
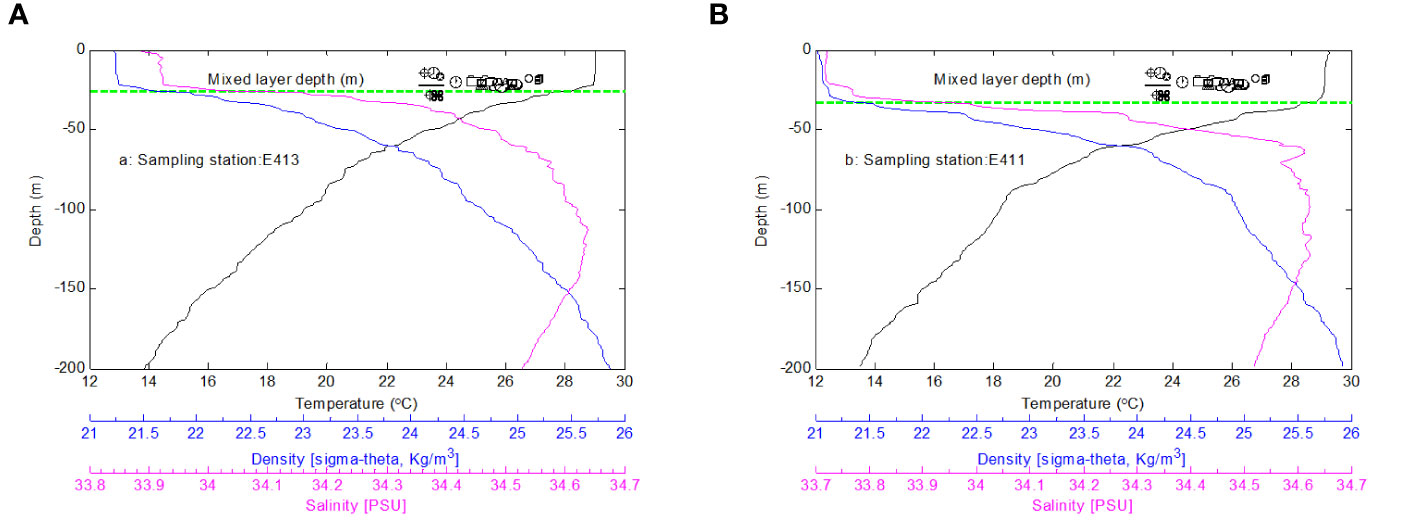
Figure 4 The 8-day mean SLA from AVISO. (A) Before the sampling period, from 28/08/2004 to 29/09/2004; (B) during the sampling period, 23/09/2004 to 25/09/2004.
Biological activities
The vertical distribution of chl-a at the sampling stations is shown in Figure 5. The subsurface chlorophyll maximum, with values between 0.1 and 0.3 mg m−3, varied from 50 to 150 m (Figure 5). In addition, the chl-a maximum (0.3 mg m−3) at E407 was 50% higher than in the water of the cold eddy at E413 (0.2 mg m−3) above the euphotic zone (about 80 m).
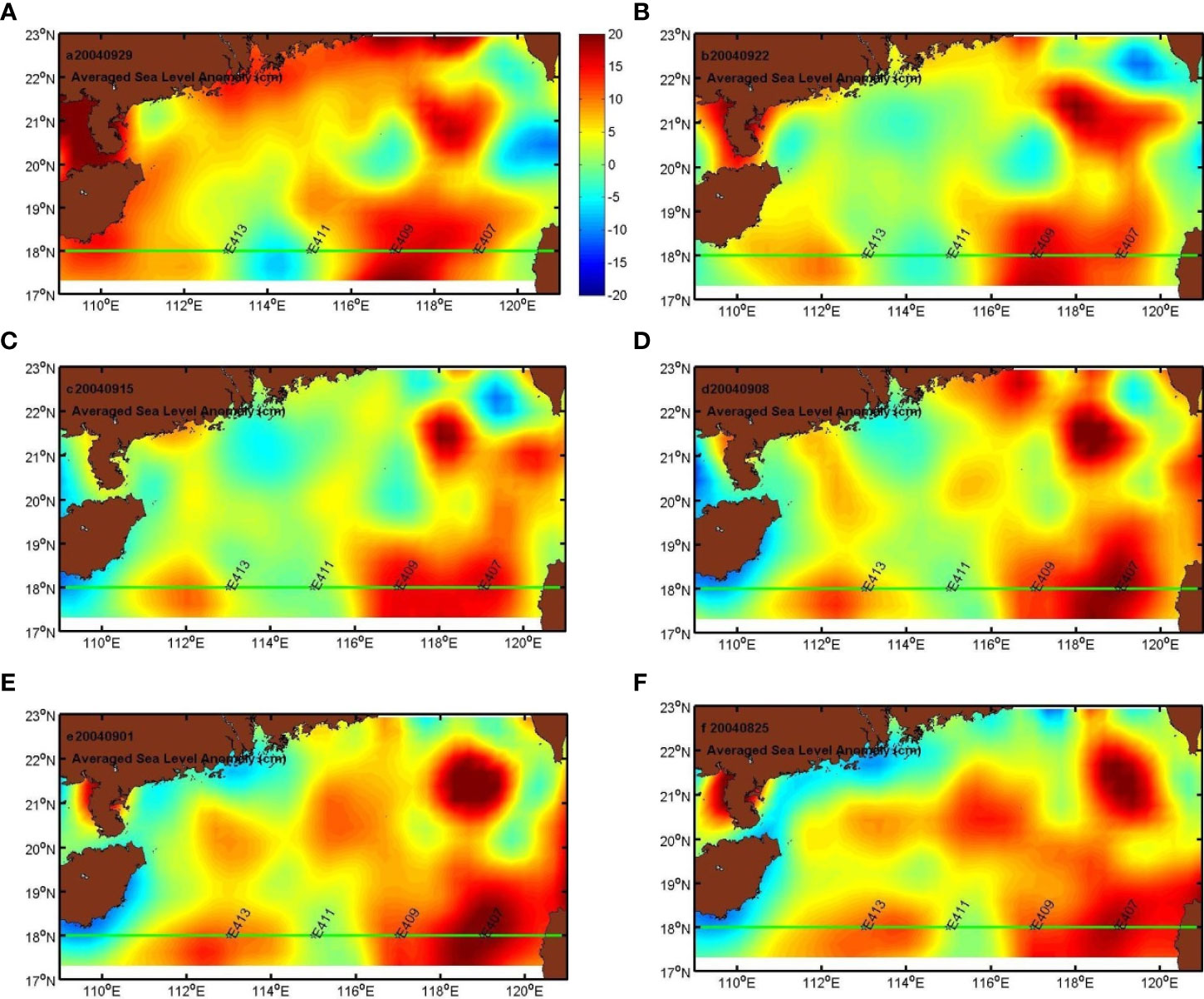
Figure 5 8-day mean SLA from AVISO (A–F) Before and after the sampling time: from 28/8/2004 to 29/9/2004; (B) the sampling time: 23-25/9/2004.
Discussion
Chl-a content ranged from 0.10 to 0.30 mg m−3 in the SCS. The chlorophyll maximum was 0.30 mg m−3 in the top 75 m of E407 and 0.20 mg m−3 in the top 25 m of E413. Chl-a content varied from 0.01 to 1.95 mg m−3 in the SCS (Ning et al., 2004). The subsurface chlorophyll maximum, with values between 0.10 and 0.60 mg m−3, ranged from 50 to 100 m in the SCS. Integrated phytoplankton biomass (chl-a) at four stations was similar in the euphotic layer. These observations are similar to those in a previous study (Ning et al., 2004). However, there are differences between the edges of cold and warm eddies. The chl-a maximum (~0.20 mg m−3) at the base of the surface mixed layer (25 m) was found at the edge of the warm (WI) and cold eddy (CI) at E413, while chl-a maxima (~0.30 mg m−3) at the warm eddy edge were observed at E407 and E409, and deepened to 75 m depth in that area (Figure 5). The chl-a maximum (0.24 ± 0.60 mg m−3) at 75 m was found in the water of a warm eddy in the western SCS (Zhong and Huang, 2013). The averaged water column-integrated concentration of total chlorophyll inside the eddy was similar to that outside the eddy, and an enhancement of phytoplankton biomass and an increase in the range of the depth of the chl-a maximum (DCM) layer were detected within the eddy (Dai et al., 2020). The subsurface chlorophyll maxima layers contained approximately 50% of the chl-a standing stock in the water column, and the contribution increased to >70% for high-biomass areas, suggesting the biological importance of the summer subsurface chlorophyll maxima in the shelf ecosystem (Zhuang et al., 2020).
What made the difference? The explanation may be physical processes. From the SLA figures, the cold eddy began to form for several days at the stations (E411 and E413). The isotherm at the depth range of 60 to 200 m at E411 was shallower than at E413 and other stations (Figure 5), by about 10 m, and a warm eddy may have played a role in determining this scenario. The research stations (E407 and E409) were located at the edge of the warm eddy shown in Figure 3. Thus, the alternating patches of cold and warm eddies along 18°N can be further verified. The altimetry data were used to estimate thermocline displacement. Figure 3 indicates that the surface depression (Δh = −3 cm) occurred before the period of sampling (23/09/2004). If necessary, Δρ = 2.3 kg m−3 was used in Eq. (1); ΔD was 20.5 m. Therefore, the thermocline displacement is similar to the observed displacement at E407. Compared with E413, the displacement inferred that the subsurface chlorophyll maximum at E407 may deepen by about 20 m in the upper layer. According to the above chl-a profile, the downward movement caused by WII resulted in phytoplankton moving from the mixed layer to the deep layer (Figure 5).
This interesting phenomenon was found in the East Sea (Kim et al., 2012), the SCS, and the Indian Ocean where the subsurface chlorophyll maximum was below the euphotic zone (Thompson et al., 2007; Huang et al., 2010). Because there was a significant difference between the warm (WII) and cold (CI) eddies above the euphotic zone, phytoplankton might grow under different physical conditions. Nutrients (nitrate and phosphate) are depleted within the euphotic zone in the SCS (Wong et al., 2007). Under this scenario, nutrients may be the limiting factor for phytoplankton in the mixed layer. Warm eddies could determine the distribution of nutrients in the euphotic layer and regulate phytoplankton patterns in the coastal, shelf, and open ocean ecosystem (Kim et al., 2012).
The uplift or downward movement of environmental factors was accompanied by the cold or warm eddies, respectively. From the SLA figures, there was about a 1-month time lag from 22 August to 23 September. There were two warm eddies (E413, E407, and E409) and embryonic forms of a cold eddy (E411) at the sampling stations before the sampling period. As time went by, the E413 site was situated at the edge of the warm and cold eddies (WI and CI), while E411 was sited at the edge of the cold and warm eddies (CI and WII) during the sampling period. Interestingly, the observed chl-a maximum layer at E413 (50 m) was less than that at E407 and E409 (75 m), while it was also found at E411 (75 m), where the cold eddy (CI) gradually strengthened before and during the sampling period (Figure 5).
Conclusion
In this study, we provide field evidence for the vertical distribution of chl-a being regulated by mesoscale eddies, including the cold and warm eddies in the euphotic layer of the SCS. Inside a warm eddy, the maximum depth of chl-a is deeper than it is on the edge of such an eddy—the point of interaction between the gradually vanishing warm eddy and slowly boosting cold eddy. The occurrence of mesoscale eddies, confirmed by the SLA satellite data and in situ measured temperature and salinity, induced the vertical pattern of chl-a.
Interpretation of these findings concludes that the mesoscale eddies have an important influence on the chemical parameters and biological activities of the open sea. The water convergency induced by a warm eddy causes downwards transport while the water convergency induced by a cold eddy induces upwards transport; therefore, the presence of phytoplankton and nutrients in cold eddies is accomplished by the motion associated with eddy-induced divergence (convergence). It is the complicated interaction among different mesoscale eddies in the open sea that creates the resulting biogeochemistry.
Data availability statement
The original contributions presented in the study are included in the article/supplementary material. Further inquiries can be directed to the corresponding author/s.
Author contributions
All authors contributed to the article and approved the submitted version.
Funding
The research was supported by the National Natural Science Foundation of China (No. 31971480), the Key Special Project for Introduced Talents Team of Southern Marine Science and Engineering Guangdong Laboratory (Guangzhou) (Nos. GML2019ZD0303 and GML2019ZD0404), the Strategic Priority Research Program of the Chinese Academy of Sciences (No. XDA19060204), Hainan Province Science and Technology Special Fund (ZDYF2021XDNY131), and members of the scientific research team of the RV Shiyan 3 for help at sea.
Acknowledgments
The research was supported by the National Natural Science Foundation of China (No. 31971480), Key Special Project for Introduced Talents Team of Southern Marine Science and Engineering Guangdong Laboratory (Guangzhou) (Nos. GML2019ZD0303 and GML2019ZD0404), the Strategic Priority Research Program of the Chinese Academy of Sciences (No. XDA19060204), Hainan Province Science and Technology Special Fund (ZDYF2021XDNY131) and members of the scientific research team of the RV Shiyan 3 for help at sea.
Conflict of interest
Author J-CX was employed by Guangdong Zhihuan Innovative Environmental Technology Co. Ltd.
The remaining authors declare that the research was conducted in the absence of any commercial or financial relationships that could be construed as a potential conflict of interest.
Publisher’s note
All claims expressed in this article are solely those of the authors and do not necessarily represent those of their affiliated organizations, or those of the publisher, the editors and the reviewers. Any product that may be evaluated in this article, or claim that may be made by its manufacturer, is not guaranteed or endorsed by the publisher.
References
Burger J. M., Moloney C. L., Walker D. R., Parrott R. G., Fawcett S. E. (2020). Drivers of short-term variability in phytoplankton production in an embayment of the southern benguela upwelling system. J. Mar. Syst. 208, 1–16. doi: 10.1016/j.jmarsys.2020.103341
Chang Y. L., Miyazawa Y., Oey L. Y., Kodaira T., Huang S. (2017). The formation processes of phytoplankton growth and decline in mesoscale eddies in the western north pacific ocean. J. Geophysical Rese-Oceans 122 (5), 4444–4455. doi: 10.1002/2017JC012722
Chen Y. L. L., Chen H. Y., Lin I. I., Lee M. A., Chang J. (2007). Effects of cold eddy on phytoplankton production and assemblages in Luzon strait bordering the south China Sea. J. Oceanography 63 (4), 671–683. doi: 10.1007/s10872-007-0059-9
Dai S., Zhao Y. F., Liu H. J., Hu Z. Y., Zheng S., Zhu M. L., et al. (2020). The effects of a warm-core eddy on chlorophyll a distribution and phytoplankton community structure in the northern south China Sea in spring 2017. J. Mar. Syst. 210, 1–12. doi: 10.1016/j.jmarsys.2020.103396
Huang B. Q., Hu J., Xu H. Z., Cao Z. R., Wang D. X. (2010). Phytoplankton community at warm eddies in the northern south China Sea in winter 2003/2004. Deep-Sea Res. Part Ii-Topical Stud. Oceanography 57 (19-20), 1792–1798. doi: 10.1016/j.dsr2.2010.04.005
Jyothibabu R., Vinayachandran P. N., Madhu N. V., Robin R. S., Karnan C., Jagadeesan L., et al. (2015). Phytoplankton size structure in the southern bay of Bengal modified by the summer monsoon current and associated eddies: Implications on the vertical biogenic flux. J. Mar. Syst. 143, 98–119. doi: 10.1016/j.jmarsys.2014.10.018
Kim D., Yang E. J., Kim K. H., Shin C. W., Park J., Yoo S., et al. (2012). Impact of an anticyclonic eddy on the summer nutrient and chlorophyll a distributions in the ulleung basin, East Sea (Japan Sea). ICES J. Mar. Sci. 69 (1), 23–29. doi: 10.1093/icesjms/fsr178
Kulk G., Platt T., Dingle J., Jackson T., Jonsson B. F., Bouman H. A., et al. (2020). Primary production, an index of climate change in the ocean: Satellite-based estimates over two decades. Remote Sens. 12 (5), 1–26. doi: 10.3390/rs12050826
Leng X., Yang Y., Sun J., Zhang G., Xu W., Feng Y., et al. (2016). Mesoscale physical processes on the effects of distribution of nutrients and chlorophyll ain the western south China Sea in summer. Acta Oceanol Sin. 38 (4), 66–75. doi: 10.3969/j.issn.0253-4193.2016.04.006
Lin J., Cao W., Wang G., Hu S. (2014). Satellite-observed variability of phytoplankton size classes associated with a cold eddy in the south China Sea. Mar. pollut. Bull. 83 (1), 190–197. doi: 10.1016/j.marpolbul.2014.03.052
Liu C., Zhuang W., Xia H., Du Y. (2012). Mesoscale observation in the northeast south China Sea during winter 2009-2010. Acta Oceanol Sin. 34 (1), 8–16. doi: 10.1007/s11783-011-0280-z
Mann D. G. (1999). The species concept in diatoms. Phycologia 38 (6), 437–495. doi: 10.2216/i0031-8884-38-6-437.1
Medina-Gomez I., Trujillo A. A., Marino-Tapia I., Cruz G., Herrera-Silveira J., Enriquez C. (2019). Phytoplankton responses under a joint upwelling event and an algal bloom scenario in the southeast gulf of Mexico. Continental Shelf Res. 184, 30–43. doi: 10.1016/j.csr.2019.07.006
Ning X., Chai F., Xue H., Cai Y., Liu C., Shi J. (2004). Physical-biological oceanographic coupling influencing phytoplankton and primary production in the south China Sea. J. Geophys. Res. 109, C10005. doi: 10.1029/2004JC002365
Nishino S., Itoh M., Kawaguchi Y., Kikuchi T., Aoyama M. (2011). Impact of an unusually large warm-core eddy on distributions of nutrients and phytoplankton in the southwestern Canada basin during late summer/early fall 2010. Geophysical Res. Lett. 38, 1–6. doi: 10.1029/2011GL047885
Rebert J. P., Donguy J.-R., Eldin G., Wyrtki K. (1985). Relations between sea level, thermocline depth, heat content, and dynamic height in the tropical pacific ocean. J. Geophysical Res: Oceans (1978–2012) 90 (C6), 11719–11725. doi: 10.1029/JC090iC06p11719
Sun F., Xia X., Simon M., Wang Y., Zhao H., Sun C., et al. (2022). Anticyclonic eddy driving significant changes in prokaryotic and eukaryotic communities in the south China Sea. Front. Mar. Sci. 9. doi: 10.3389/fmars.2022.773548
Thompson P. A., Pesant S., Waite A. M. (2007). Contrasting the vertical differences in the phytoplankton biology of a dipole pair of eddies in the south-eastern Indian ocean. Deep Sea Res. Part II: Topical Stud. Oceanography 54 (8), 1003–1028. doi: 10.1016/j.dsr2.2006.12.009
Tseng C. -M., Wong G. T., Lin I. -I., Wu C. -R., Liu K. -K. (2005). A unique seasonal pattern in phytoplankton biomass in low-latitude waters in the South China Sea. Geophys. Res. Lett. 32 (8), L08608. doi: 10.1029/2004GL022111
Wang L., Huang B., Chiang K. P., Liu X., Chen B., Xie Y., et al. (2016). Physical-biological coupling in the Western south China Sea: The response of phytoplankton community to a mesoscale cyclonic eddy. PloS One 11 (4), 1–18. doi: 10.1371/journal.pone.0153735
Wong G. T., Tseng C. M., Wen L. S., Chung S. W. (2007). Nutrient dynamics and n-anomaly at the SEATS station. Deep Sea Res. Part II: Topical Stud. Oceanography 54 (14), 1528–1545. doi: 10.1016/j.dsr2.2007.05.011
Xu Z., Li S., Hu J., Wang S., Wang B., Guo M., et al. (2018). Summer phytoplankton responses to upwelling and river plume in northern south China Sea. J. Trop. Oceanography 37 (6), 92–103. doi: 10.11978/2018001
Xu W., Wang G., Cheng X., Jiang L., Zhou W., Cao W. (2022). Characteristics of subsurface chlorophyll maxima during the boreal summer in the south China Sea with respect to environmental properties. Sci. Total Environ. 820, 1–11. doi: 10.1016/j.scitotenv.2022.153243
Zhong C. X., Xiao W. P., Huang B. Q. (2013). The response of phytoplankton to mesoscale eddies in Western south China Sea. Advance Mar. Sci. 31 (2), 213–220.
Keywords: South China Sea, phytoplankton, subsurface chlorophyll maximum, cold eddy, warm eddy
Citation: Wu M-L, Wang Y-S, Wang Y-T, Sun F-L, Li X, Gu F-F and Xiang J-C (2022) Vertical patterns of chlorophyll a in the euphotic layer are related to mesoscale eddies in the South China Sea. Front. Mar. Sci. 9:948665. doi: 10.3389/fmars.2022.948665
Received: 20 May 2022; Accepted: 04 July 2022;
Published: 28 July 2022.
Edited by:
Guangzhe Jin, Guangdong Ocean University, ChinaReviewed by:
Yiguo Hong, Guangzhou University, ChinaPeng Wu, South China Sea Fisheries Research Institute, Chinese Academy of Fishery Sciences (CAFS), China
Copyright © 2022 Wu, Wang, Wang, Sun, Li, Gu and Xiang. This is an open-access article distributed under the terms of the Creative Commons Attribution License (CC BY). The use, distribution or reproduction in other forums is permitted, provided the original author(s) and the copyright owner(s) are credited and that the original publication in this journal is cited, in accordance with accepted academic practice. No use, distribution or reproduction is permitted which does not comply with these terms.
*Correspondence: Mei-Lin Wu, bWx3dUBzY3Npby5hYy5jbg==; You-Shao Wang, eXN3YW5nQHNjc2lvLmFjLmNu; Yu-Tu Wang, eXR3YW5nQHNjc2lvLmFjLmNu; Fu-Lin Sun, ZmxzdW5Ac2NzaW8uYWMuY24=