- Department of Biological Sciences, University of Wisconsin-Milwaukee, WI, United States
Given the impacts of climate change and other anthropogenic stressors on marine systems, there is a need to accurately predict how species respond to changing environments and disturbance regimes. The use of genetic tools to monitor temporal trends in populations gives ecologists the ability to estimate changes in genetic diversity and effective population size that may be undetectable by traditional census methods. Although multiple studies have used temporal genetic analysis, they usually involve commercially important species, and rarely sample before and after disturbance. In this study, we run a temporal analysis of giant kelp, Macrocystis pyrifera, genetic diversity over the scope of 10 years (2008-2018) using the same microsatellite marker panel to assess the genetic consequences of disturbance in several populations of giant kelp (Macrocystis pyrifera) in the Southern California Bight. The study is a rare pre- and post-disturbance microsatellite analysis that included declines to giant kelp caused by the 2015/16 El Nino Southern Oscillation event. We used canopy biomass estimated by remote sensing (Landsat) to quantify the extent of disturbance to kelp beds, and sea surface temperature data to understand how kelp was pushed towards its temperature limits during this period. Despite prolonged periods with decreased canopy at several sites, no changes in genetic structure and allelic richness were observed. We argue that giant kelp in the region is best described as a “patchy population” system where true extinctions are rare. We discuss how deep refugia of subsurface sporophytes and cryptic microscopic life stages could have kept genetic diversity through disturbance. Given the increasing effects of climate change and uncertainty in modeling impacts of species with cryptic life history stages, we suggest further investigation to reveal the role such stages play in species resilience. Genetic monitoring studies of sites selected by remote census demographic and climate surveys should be continued in the future given the predicted impacts of climate change.
Introduction
The habitats of the world’s oceans are altering vastly due to anthropogenic stressors, such as pollution, habitat loss, invasive species, and climate change (Rahel and Olden, 2008; Johnston and Roberts, 2009; Claudet and Fraschetti, 2010; Davidson et al., 2015; McCauley et al., 2015; García Molinos et al., 2016). Therefore, monitoring and predicting how species react to changing regimes is of concern for species and biodiversity conservation levels (Bellard et al., 2012; Tilman et al., 2017). Resilience, the capacity of a system to absorb disturbance and reorganize to a previous state (Walker et al., 2004), is often studied by census and area cover changes, informed by current and historical distributions and population demography (Timpane-Padgham et al., 2017). However, the change in genetic diversity, an essential biodiversity component, is rarely studied when populations recover from disturbance to previous census sizes. Moreover, we note that in marine systems census sizes might be several orders of magnitude lower than effective population sizes (Hare et al., 2011).
It is generally accepted that more genetically diverse populations have greater adaptive potential and functioning (Reusch et al., 2005; Johnson et al., 2006; Hughes et al., 2008). Intraspecies diversity arises from heterogeneity in ecological and evolutionary forces that act on spatially structured populations through time. Fluctuations in patterns of recruitment, census size, and selection events, often resulting from disturbance, shape the contemporary genetic structure of populations. A strong disturbance event, outside of the physiological tolerance of a species, can reduce within-population genetic diversity by selecting tolerant alleles or, if census sizes are drastically reduced, by genetic drift alone. Following disturbance, changes in recruitment patterns during demographic recovery can also alter the between-population genetic diversity depending on whether recovery occurs through the propagation of fewer local (Pannell and Charlesworth, 2000; Evanno et al., 2009) or many foreign individuals (Spear et al., 2012), resulting in increasing or decreasing genetic differentiation compared to previous levels (Banks et al., 2013). Although many genetic studies have explored how ecological and evolutionary forces shape populations through space, little is known about how patterns of diversity change through time because most studies have a single time point. When measured across a species distribution and in conjunction with environmental variables associated with disturbance, conservation biologists can quantify population sensitivity to disturbance, rates of change in genetic diversity, and identify critical regions for conservation (Funk et al., 2012; Flanagan et al., 2018).
Genetic monitoring, the collection of temporal genetic data for the same sites, is a powerful tool that provides complementary information for census studies, especially when pre-disturbance genetic data is available (Schwartz et al., 2007). Single point genetic studies rely heavily on the assumption of stability in genetic diversity and rarely incorporate temporal factors like population demographic history and dynamics and how these relate to biotic and abiotic change. Consequently, they have limited capacity to link environmental change and genetic erosion, which is of particular interest for species with wide spatial distribution encompassing a range of environmental change and variable extinction probabilities. When used in conjunction with census and environmental data, genetic monitoring can reveal hidden mechanisms of population persistence, such as cryptic life history stages or behaviors (Arnaud and Laval, 2004; Orsini et al., 2016). Currently, genetic monitoring studies are typically conducted on terrestrial vertebrates (i.e., mammals and birds) or commercially important aquatic species (e.g., fish) (Bellinger et al., 2003; Schwartz et al., 2007), with only a few studies focused on other aquatic taxa (Reynolds et al., 2017; Gurgel et al., 2020; Manent et al., 2020). Until recently, only a few studies had applied genetic monitoring techniques using a before-after disturbance approach (Gurgel et al., 2020; Holt et al., 2020; Manent et al., 2020). In both Holt et al. (2020) and Manent et al. (2020), clonal aquatic plants showed a reduction in demographic and genetic diversity. In contrast, in Gurgel et al. (2020), the reduction in genetic diversity was cryptic after demographic recovery in two forest-forming seaweeds.
Kelps are large, brown seaweeds that grow in nutrient-rich saltwater in rocky reefs of coastal and seamount habitats worldwide. Kelp forests are among some of the most productive systems on earth (Mann, 1973; Vilalta-Navas et al., 2018) and are essential in supporting other coastal and open ocean pelagic systems through detrital export (Duggins et al., 1989; Hobday, 2000a; Hobday, 2000b; Krumhansl and Scheibling, 2012). Their ecological (Bertocci et al., 2015; Bennett et al., 2016; Blamey and Bolton, 2018) and economic (Chung et al., 2011; Bennett et al., 2016; FAO, 2018) importance make them a key target group for conservation. As coastal ecosystems, kelp forests are exposed to a variety of anthropogenic and natural stressors that are both regional (pollution, invasive species, sedimentation, harvesting, fishing, storm surges, disease, herbivory and gene pollution from aquaculture) and global (climate change, El Niño Southern Oscillation) (Steneck et al., 2002; Schiel and Foster, 2015; Bennett et al., 2016). Kelp recruitment success is particularly susceptible to increased temperature (Muth et al., 2019), but it is important to consider regional intraspecific differences (Hollarsmith et al., 2020). Consequently, numerous kelp studies reported (Steneck et al., 2002; Valero et al., 2011; Mineur et al., 2015; Filbee-Dexter et al., 2016; Krumhansl et al., 2016; Vergés et al., 2016; Wernberg et al., 2016; Rogers-Bennett and Catton, 2019; Butler et al., 2020) or predicted regional declines (Araújo et al., 2016; Assis et al., 2018a; de la Hoz et al., 2019; Sudo et al., 2020).
Kelp species from around the world have been the focus of population genetics studies (Billot et al., 2003; Fraser et al., 2009; Tellier et al., 2009; Macaya, 2010; Johansson et al., 2015; Assis et al., 2016; Assis et al., 2018b; Neiva et al., 2018; Mao et al., 2020, among others). Despite our considerable current knowledge of kelp population genetics and conservation status, only two studies have incorporated a temporal genetic analysis (Valero et al., 2011; Wootton and Pfister, 2013), and only Gurgel et al. (2020) on other forest-forming seaweeds was conducted following a major disturbance event. This is unfortunate because the cryptic, microscopic, gametophyte life stage of kelps may offer different strategies for post-disturbance demographic recovery, similar to the seed banks of some higher plants (Barradas et al., 2011; Carney et al., 2013). After disturbance, the effective population size of kelp might be much larger than perceived from persisting reduced census sizes measured on the sporophytes (macroalga). This will depend on the number of sporophytes that could release their spores before population decline, how long the demographic decline in the sporophyte generation persisted, and how long the gametophyte stage can survive.
One species of particular interest for conservation is the habitat-forming kelp, Macrocystis pyrifera (giant kelp). In southern California, there has been much effort to understand the population dynamics of giant kelp (Deysher and Dean, 1986; Reed, 1990; Reed et al., 2006; Dayton et al., 1992; Reed et al., 1997; Pakker and Flores-Moya, 2000; Gaylord et al., 2002; Reed et al., 2004; Gaylord et al., 2006; Cavanaugh et al., 2010; Cavanaugh et al., 2011; Cavanaugh et al., 2013; Castorani et al., 2015; Castorani et al., 2017), including genetics studies (Alberto et al., 2010; Alberto et al., 2011; Johansson et al., 2013; Johansson et al., 2015; Hargarten et al., 2019). The surface breaching nature of giant kelp has enabled remote sensing techniques (Landsat imagery) to build long-term time series in canopy area coverage and biomass estimates (Cavanaugh et al., 2010; Bell et al., 2020). Analysis from both remote sensing and fieldwork has revealed several environmental factors (herbivory, wave height, light, nutrients, temperature, sedimentation, and substrate availability) that influence the distribution and range of giant kelp in the northeast pacific (Cavanaugh et al., 2011; Bell et al., 2015; Johansson et al., 2015; Young et al., 2016). Events such as El Niño Southern Oscillation (ENSO) that bring warm nutrient-depleted waters can have substantial adverse effects on canopy biomass and are increasing in frequency and intensity (Solomon, 2007; Oliver et al., 2018). Despite these events, giant kelp in southern California has the highest level of genetic diversity across the species range (Macaya, 2010; Johansson et al., 2015) and exhibits resilience of kelp area coverage (Reed et al., 2016; Cavanaugh et al., 2019) and fast recovery following disturbance (Dayton et al., 1992; Ladah et al., 1999). However, kelp area resilience is uninformative about possible losses of genetic diversity.
This study analyzes the temporal variation in genetic differentiation and the allelic richness of giant kelp. After ten years since previous genetic analysis, we looked at change for five sites in the Southern California Bight (SCB). We tested the hypothesis that demographic bottlenecks produced by disturbances from 2014-to 2016 have depressed the levels of genetic diversity in giant kelp. We choose sites that differ in genetic coancestry, oceanographic conditions, and disturbance severity to assess how disturbance impacts may vary across the study region.
Materials and methods
Giant kelp is the largest and most widely distributed benthic species in the world (Graham et al., 2007). In the Northeast Pacific, it forms large surface breaching forests along the coastline from Alaska to Bahia Tortugas, Mexico. As with other kelps, M. pyrifera has a biphasic haplodiplontic life cycle in which a large sporophyte (2n) produces spores (1n) via meiosis that settle on hard, rocky substrate, which then differentiates into microscopic male and female gametophytes (1n). Male gametophytes release motile sperm that fertilize a non-motile egg, retained on the female thallus. Following fertilization, the zygote develops into a blade, producing the next generation of sporophytes. Dispersal occurs mainly through the spore stage, in which planktonic individuals can survive for at least one week (Reed et al., 1992) and may settle several kilometers from the origin. However, most spores settle within a few meters (Reed et al., 1992; Gaylord et al., 2006). There is some evidence for longer dispersal distance by kelp rafts (Hobday, 2000a; Hernández-Carmona et al., 2006). However, this dispersal mechanism is negligible in maintaining connectivity between local populations when compared to spores (Reed et al., 2004; Hargarten et al., 2019).
Study sites and sampling
To conduct a temporal genetic analysis, we sampled five sites between 2018 and 2019 located in three regions differing in genetic coancestry in the Southern California Bight (SCB), hereafter referred to as 2018 samples. These regions had also been sampled before, in 2007 and 2008, and genotyped by Johansson et al. (2015) using microsatellite marker analysis, hereafter referred to as 2008 samples. Two of our locations are continental, Leo Carrillo, and Camp Pendleton, with one site sampled in each. Our third location was Catalina Island, where we sampled three sites (Figure 1). We sampled more sites on Catalina Island because this was the location where disturbance to kelp forests during the 2008 to 2018 period was higher. Johansson et al. (2015) found that Catalina Island belongs to a genetic coancestry group comprised of both the northern and southern channel island archipelagos while the northernmost continental site, Leo Carrillo, showed admixture between three genetic coancestry groups in SCB. The southernmost sampling location, Camp Pendleton, belonged to the Southern California/Baja California genetic group. We note that the 2018 site from this location is located 12 km south of the 2008 site (San Mateo) from Johansson et al. (2015), due to logistic constraints that precluded sampling the same site. Genetic differentiation at this scale is weak, Johansson et al. (2015) reported that kelp forests from a large swath of the coast, south of Los Angeles to Baja California, belonged to the same genetic group, and all sites had similar allelic richness.
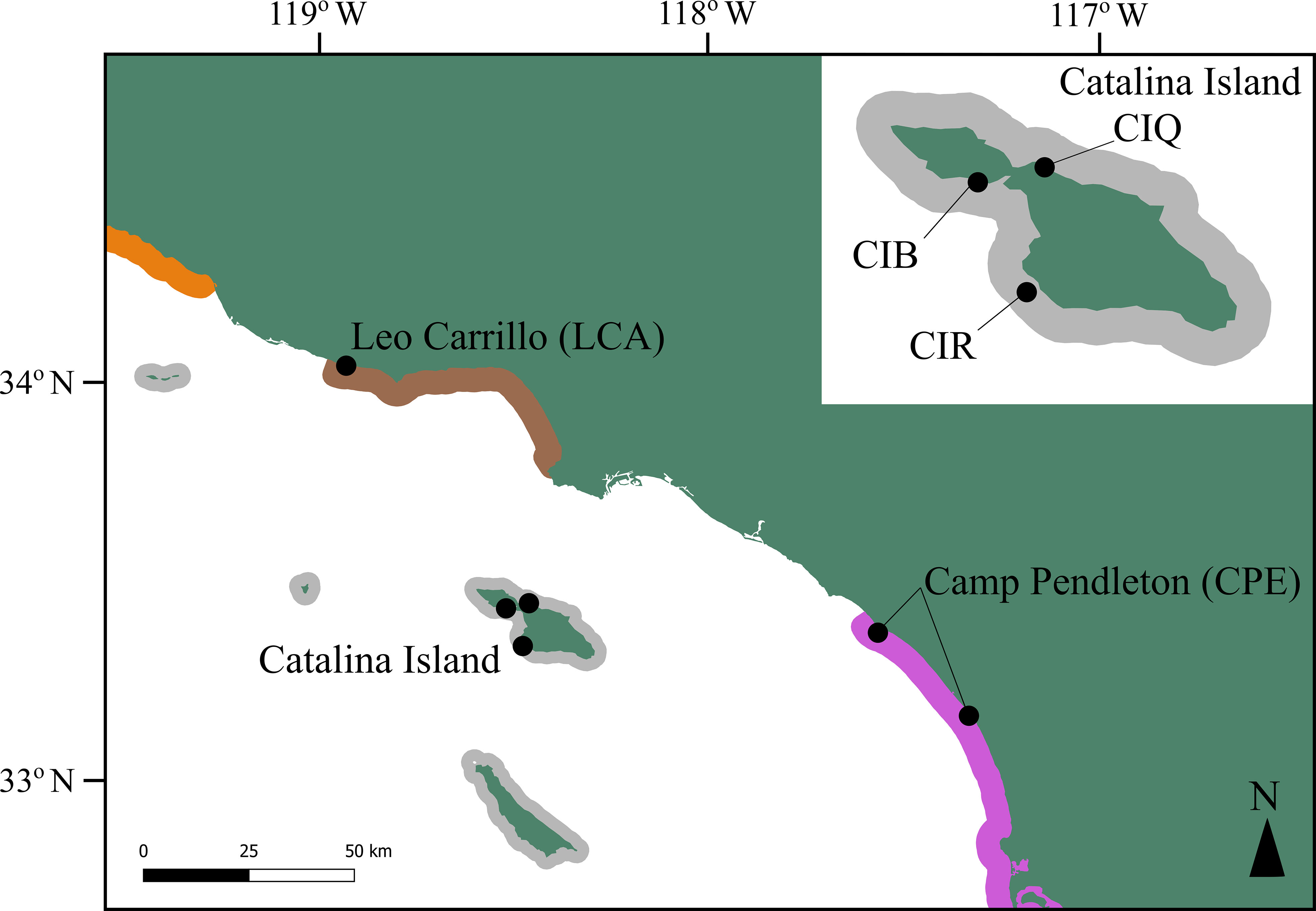
Figure 1 Map showing Macrocystis pyrifera resampled locations in the Southern California Bight. Colors represent three genetic coancestry groups described by Johansson et al. (2015), including one region of admixture (brown): Continental Santa Barbara (orange), Northern and Southern Channel Islands (gray) and Southern California/Baja California (purple) groups. The 2018 sample location for Camp Pendleton is located 30 km SE of the 2008 location. Note that giant kelp beds do not occur continuously along the colored regions.
New sample collections (Figure 1) occurred between January 2018 and June 2019. The sampling protocol involved haphazardly collecting (~n=30 per site) sporophyte blade tissue by snorkeling and SCUBA. We collected tissue from individual holdfasts to ensure non-repetitive sampling. Blades were either dried in silica or immediately frozen and desiccated using an Eppendorf Vacufuge Plus (Hamburg, Germany) for subsequent DNA extraction.
Disturbance quantification
There are several reports of disturbance to kelp forests in Southern California from 2008 to 2018. During this period, a series of large marine heatwaves (MHW) occurred, starting in the summer of 2014, followed by the 2015/16 El Nino-Southern Oscillation (ENSO) event. On Catalina, two large storm surges hit the Island in the summer and winter of 2014. Additionally, the invasive algal species, Sargassum horneri, continued to spread on the Island likely facilitated by the ENSO event (Marks et al., 2017).
To quantify the scale of disturbance to kelp forests in our study, we estimated proportional changes in surface canopy biomass from 2008 to 2018 for Leo Carrillo, Catalina Island, and Camp Pendleton using satellite data. We used surface canopy biomass derived from Landsat 5 and 7 Thematic mapper multispectral 30-meter resolution data taken quarterly (Bell et al., 2017). To compare across locations and determine the spatial scale of surface canopy changes, we summed for each quarter the biomass within an area buffer surrounding the sampling coordinates and divided by the maximum biomass observed from 2008 to 2018. We used 5, 15, and 30 km buffers to compare the severity of local changes in biomass while controlling for neighboring kelp beds that could disperse individuals into studied sites (had they experienced different dynamics). We chose a maximum buffer of 30 km, as it well exceeds the mean dispersal capabilities of giant kelp (Reed et al., 2004; Gaylord et al., 2006; Alberto et al., 2010). Spatial buffers around the location coordinates were estimated using the GEOSPHERE v.10 R Package (Hijmans et al., 2012). We averaged the proportions for 5 and 15 km buffers for each of the three sites on Catalina to obtain a single time-series for the Island. Given the size of the island and site spacing, the same 30 km buffer encompassed all possible habitat surrounding the three sites on the Island. For Camp Pendleton, we averaged the 5, 15, and 30 km proportions between the 2008 and 2018 sample sites. Differences in distances for biomass changes between the three spatial buffers were compared with Pearson’s correlation.
MHW is calculated as a deviation from historical temperatures at a particular location. Specifically, a marine heatwave was defined as five or more consecutive days with a mean temperature warmer than the 90th percentile value over the last 30 years for the same time of the year (Hobday et al., 2016). MHW impact on a species will depend on how each population’s environment is close to the species’ temperature tolerance limit, assuming no localized adaptation. Therefore, an MHW can have very different outcomes for the population depending on the location of the population and the presence or not of localized adaptation. Replacing the 90th percentile with an absolute temperature threshold generates an indicator statistic for temperature effects on all populations, regardless of location, if there is no localized adaptation. For example, Reed et al. (2016) found no impact on kelp forests in the Santa Barbara channel experiencing a prolonged heatwave (3 years). These kelp forests are located in the northern part of the Southern California Bight, which is still further north of those in Baja California, Mexico, where higher absolute temperatures have been associated with kelp loss (Ladah et al., 1999). Additionally, Cavanaugh et al. (2019) found that giant kelp resistance throughout SCB was most strongly correlated with the mean temperature of the warmest month, indicating that the loss of canopy was more sensitive to exceeding an absolute temperature threshold than to the magnitude of relative changes in temperature. Qualitatively, MHW and temperature tolerance threshold statistics differ in that the former uses the 90th percentile as a threshold to count consecutive days while the latter uses an absolute threshold value.
We quantified the number of marine heatwaves and their duration from January 2008 to December 2018. We also calculated the time in consecutive days each site experienced above a conservative species temperature tolerance threshold of 22°C. We used daily temperature from January 1984 to December 2019, obtained from the National Climatic Data Center Optimal Interpolation Sea Surface Temperature (OISST) dataset (Banzon et al., 2016), to produce a climatology baseline for determining temperature statistics. We estimated the quantity and duration of marine heatwaves as in Hobday et al. (2016), and the number of events lasting five consecutive days above a species threshold of 22 °C from 2008 to 2018 using the heatwaveR v. 0.4.2 R package (Schlegel and Smit, 2018). All analysis were done in R (R Core Team, 2019).
Genotyping
We extracted DNA using the NucleoSpin 96 Plant Kit II (Macherey-Nagel, Duren, Germany) with standard protocols and genotyped them for six microsatellite loci used in Johansson et al. (2015) (Mpy-8, Mpy-14, BC-4, BC-18, BC-19, BC-25), following modified PCR protocols in Alberto (2009). PCR product fragment sizes were separated and scored on an ABI 3730 FVNPL (Applied Biosystems) using GeneScan-500 LIZ as a size standard (at the UW-Wisconsin Biotechnology Center). We scored alleles using STRAND v. 2.4.110 and binned them using the MsatAllele v. 1.0.4 R package (Alberto et al., 2009). While binning the new data set, we genotyped a few standard samples from Johansson et al. (2015) to help match the new and old MsatAllele databases, ensuring that all alleles were binned using the same system.
Population genetics analysis
To compare changes in allelic richness between sample periods, we standardized sample size at n=15 samples for both 2008 and 2018 samples, using the StandArich2 R package v. 1.00 (https://github.com/UWMAlberto-Lab/StandArich2). We tested for significant temporal changes in richness across all sites using a non-parametric Wilcoxon signed-rank test (Arnaud and Laval, 2004). We calculated general population genetics statistics such as expected and observed heterozygosity, as well as FIS (inbreeding coefficient) for 2008 and 2018 data using GENETIX v 4.05.2 (Belkhir et al., 2001). We calculated FIS using a permutation test consisting of 1,000 permutations. We estimated Fisher’s probability of deviations from Hardy-Weinberg equilibrium using a Markov chain Monte Carlo method (Ayres and Balding, 1998) with 10,000 dememorizations steps, 1,000 batches, and 5,000 iterations in the GENEPOP v. 4.7.5 (Rousset and Raymond, 1995; Rousset, 2008). We applied a Bonferroni correction to all Fisher’s probabilities to control for multiple testing.
Genetic differentiation and spatial structure
We calculated pairwise FST (fixation index) between all paired 2008 and 2018 sites, and associated p-values, using an exact G-test with default settings in GENEPOP v.4.7.5. To visualize changes in genetic differentiation between 2008 and 2018 data, we used a factorial correspondence analysis (FCA) performed in GENETIX v. 4.05.2. We included all Southern California sites analyzed in Johansson et al. (2015) in the FCA analysis to visualize how the 2018 sites were ordered compared to 2008 ones in the context of a larger number of sites. We estimated individual genetic coancestry using STRUCTURE v. 2.3.4 for all specimen in 2018 and 2008 samples (Pritchard et al., 2000). Given that the 2008 samples in our study belonged to two of the genetic coancestry clusters identified in Johansson et al. (2015), we ran the current analysis for K=2-3 with 2008 and 2018 samples run separately. The run at K=3 revealed no additional structure, so K=2 was used in this analysis. We used parameters that included an admixture model with allele frequencies correlated across populations and an initial burn-in period of 250,000 and 750,000 reps post burn-in.
Results
Disturbance quantification with landsat and temperature analysis
Canopy changes were synchronous at spatial scales of at least 30 km, as suggested by high correlation (R > 0.94) between 5, 15, and 30 km buffers for all locations (Table 1). The results reported hereafter are for the 30km buffer analysis. Giant kelp canopy coverage varied on both intra- and interannual time scales at all locations from 2008 to 2018. With marine heatwave events from 2014 to 2016, all locations showed seasonal reductions in canopy coverage, especially Catalina Island and Camp Pendleton (Figure 2). Overall, kelp canopy biomass (measured for each quarter as the proportion of maximum biomass observed during the study period, 2008-2018) was much higher before rather than after 2014, when large MHW started (Figure 2). The before and after 2014 differences in median proportions of max biomass where very large for Camp Pendleton (before: 29.7%, after: 3.8%; Mann-Whitney p-value < 0.0001) and Catalina Island (before: 33.4%, after: 1.8%; Mann-Whitney p-value < 0.0001) and less so for Leo Carillo (before: 26.2%, after: 12.7%; Mann-Whitney p-value< 0.01), although all were highly significant.
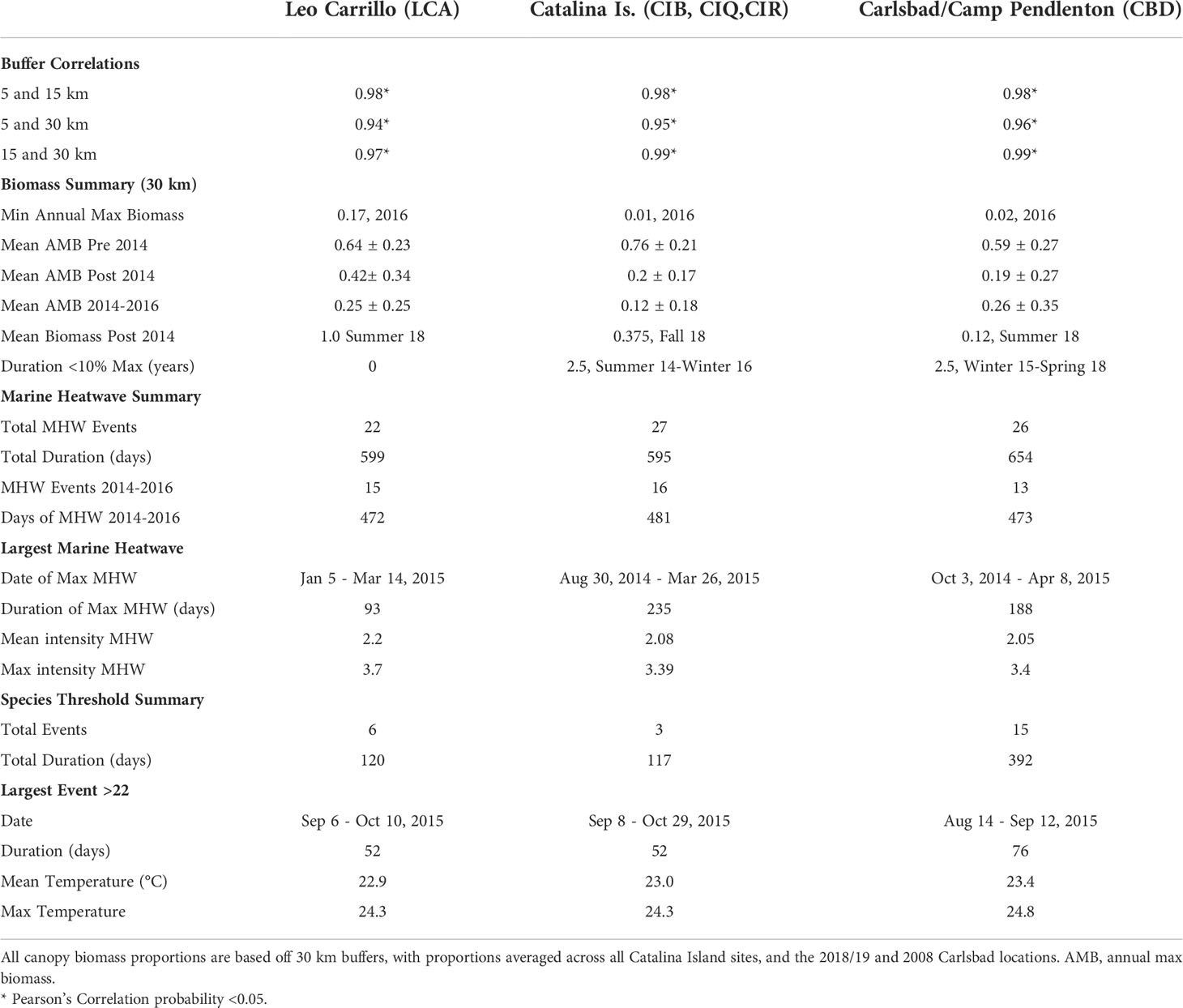
Table 1 Summary statistics for Macrocystis pyrifera canopy biomass (Landsat) and marine heatwave (MHW) analysis.
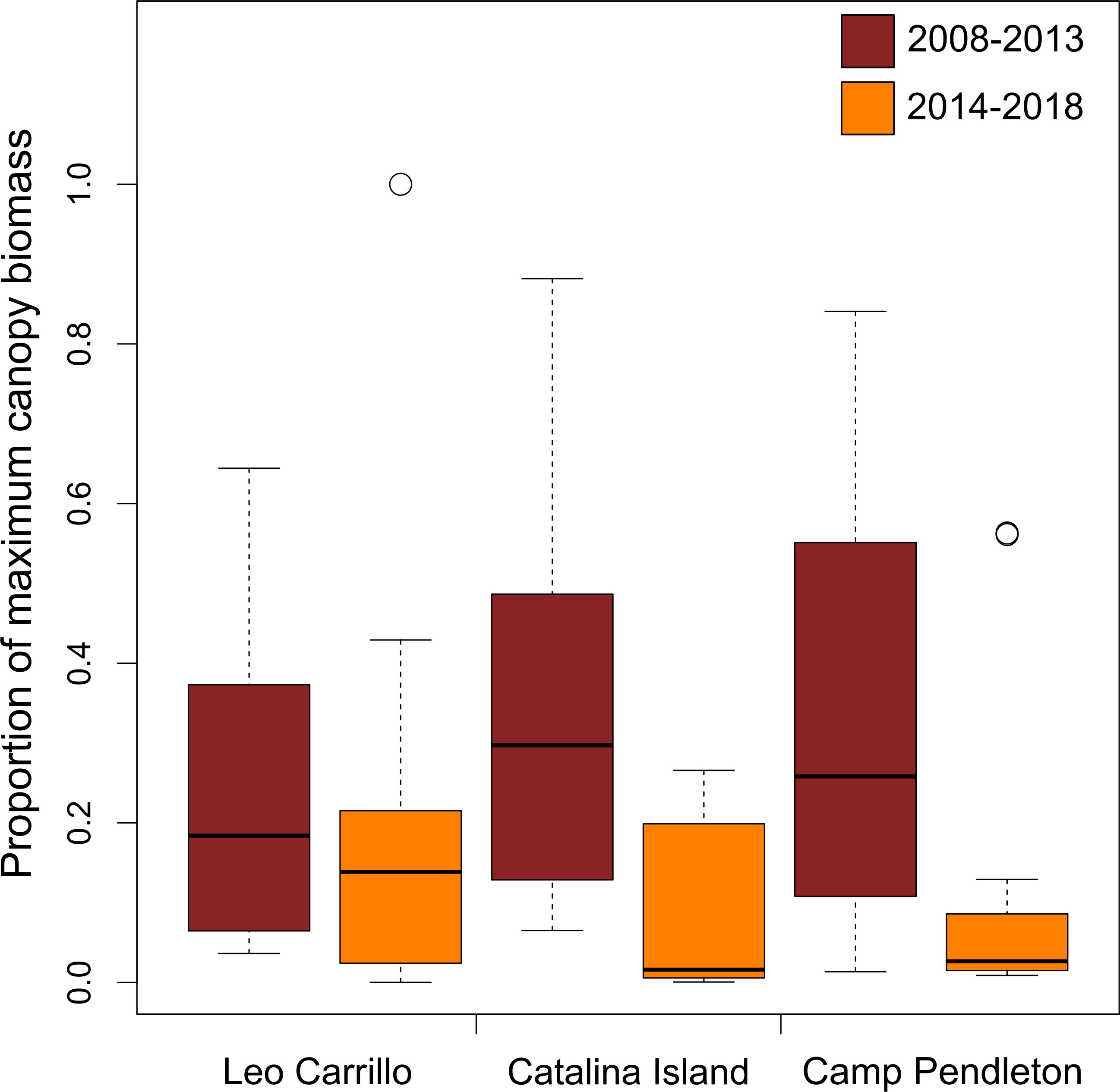
Figure 2 Distribution of Macrocystis pyrifera maximum proportion of biomass observed per quarter. Two boxplots shown for each population showing pre- (brick color) or post-disturbance periods (orange color).
Reduction and subsequent recovery in canopy biomass were variable among locations in both magnitude and duration. Leo Carrillo had the lowest peak biomass values in the summers of 2015 and 2016, but recovered quickly in the summer of 2017, with annual peaks never declining below 17% of maximum biomass. Following the ENSO event, Leo Carrillo reached maximum biomass in the summer of 2018 (Figure 3). Catalina Island and Camp Pendleton, however, showed substantial reductions in biomass for extended periods (Figure 3; Table 1); Catalina experienced two and a half years with biomass peaks below 10% of the maximum, from summer 2014 to the end of 2016 (winter) (Figure 3; Table 1). Some canopy recovery occurred on Catalina in the spring of 2017 but remained below 32% of the maximum biomass observed in the period studied (Figure 3). Camp Pendleton had reduced biomass in the fall of 2014 with minimal recovery until the end of the time-series. Peak biomass during this period occurred in the fall of 2015 and summer of 2018 (11% of max, 30 km buffer), but remained well below pre-disturbance canopy coverage (Figure 3; Table 1).
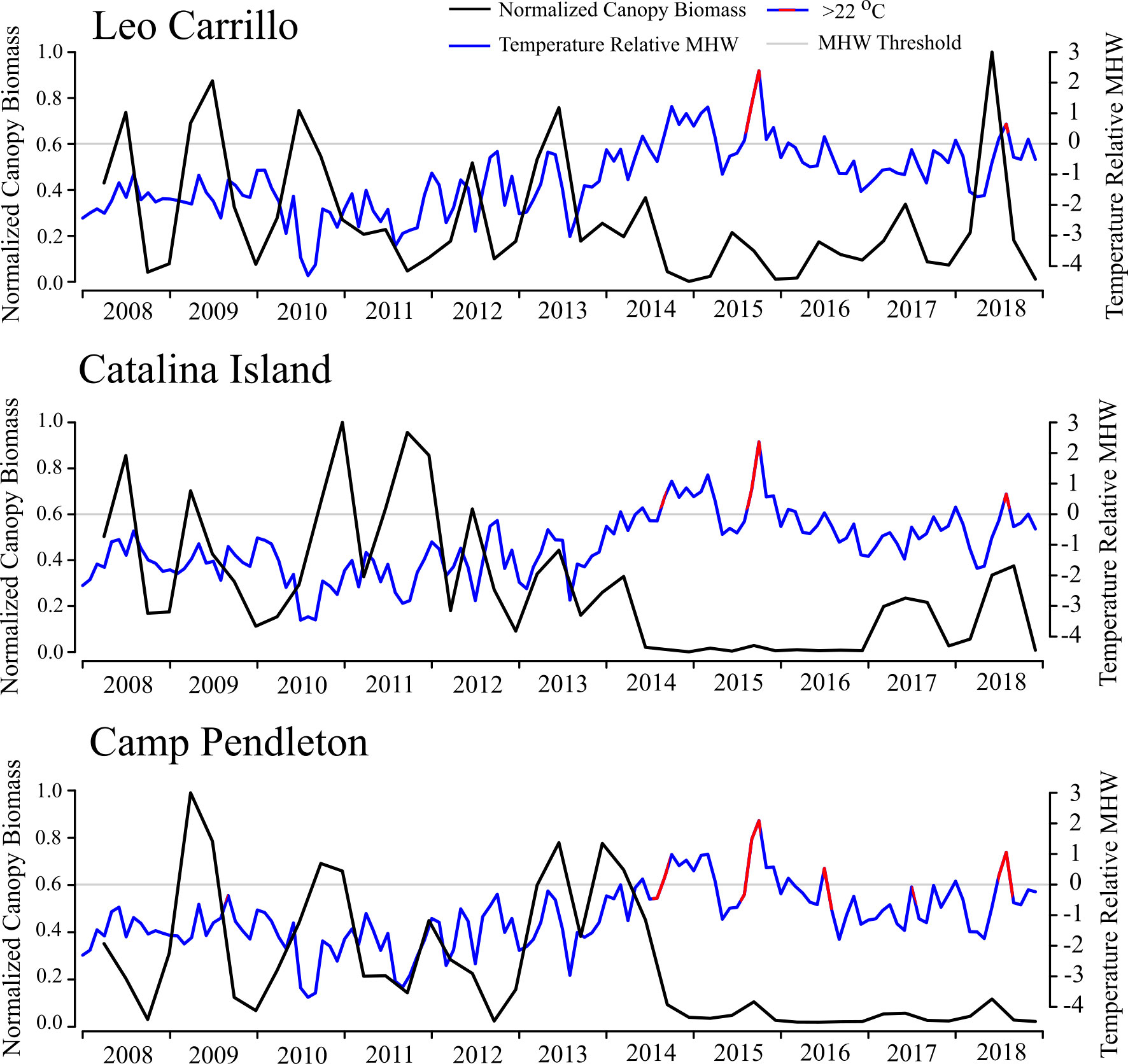
Figure 3 Normalized Macrocystis pyrifera biomass (30 km radius buffer) and Marine Heatwave (MHW) events from 2008 to 2018/19 for Leo Carrillo, Catalina Island (averaged over different locations), and Carlsbad (averaged over 2008 and 2018/19 locations). Kelp biomass is shown quarterly and normalized by the quarter of maximum biomass from 2008 to 2018/19 (black). Temperatures (Blue) are shown as monthly means relative to the MHW threshold (gray). Shown in red are periods when the mean monthly temperature exceeded a conservative species tolerance threshold of 22 °C.
Between sampling periods from 2008 to 2018, all three locations experienced multiple heatwaves (as defined by Hobday et al., 2016) and multiple day periods above the conservative temperature tolerance for giant kelp of 22°C (Table 1). However, locations varied in the quantity and duration of both MHWs and events ≥ 22°C. Between 2014 and 2016, Leo Carrillo had a total of 15 MHW events, in which 43% of days met the criteria for marine heatwaves (Hobday et al., 2016). The longest event in Leo Carrillo was 93 days long, from January to March of 2015, but was half as long as Catalina and Camp Pendleton’s longest events (Figure 3; Table 2). From 2008 to 2018, Leo Carrillo had several events that exceeded 22°C, the longest of which lasted 52 days from mid of August to late-October 2015. Catalina experienced similar trends in the total number of MHW events and heatwave days but had a long MHW event that well exceeded that of Leo Carrillo and Camp Pendleton. This MHW event lasted for 235 days from the summer of 2014 to the spring of 2015 (Table 1). Catalina also had multiple events that exceeded 22°C; the longest was of the same duration and dates as Leo Carrillo (Table 1). Camp Pendleton also showed similar trends in the total number of MHW events, but experienced longer marine heatwaves, with the longest lasting 188 days from the fall of 2014 to the spring of 2015. The number of days exceeding 22°C well exceeded Catalina and Leo Carrillo, with the longest-lasting for 76 days from August to October of 2015 (Table 1).
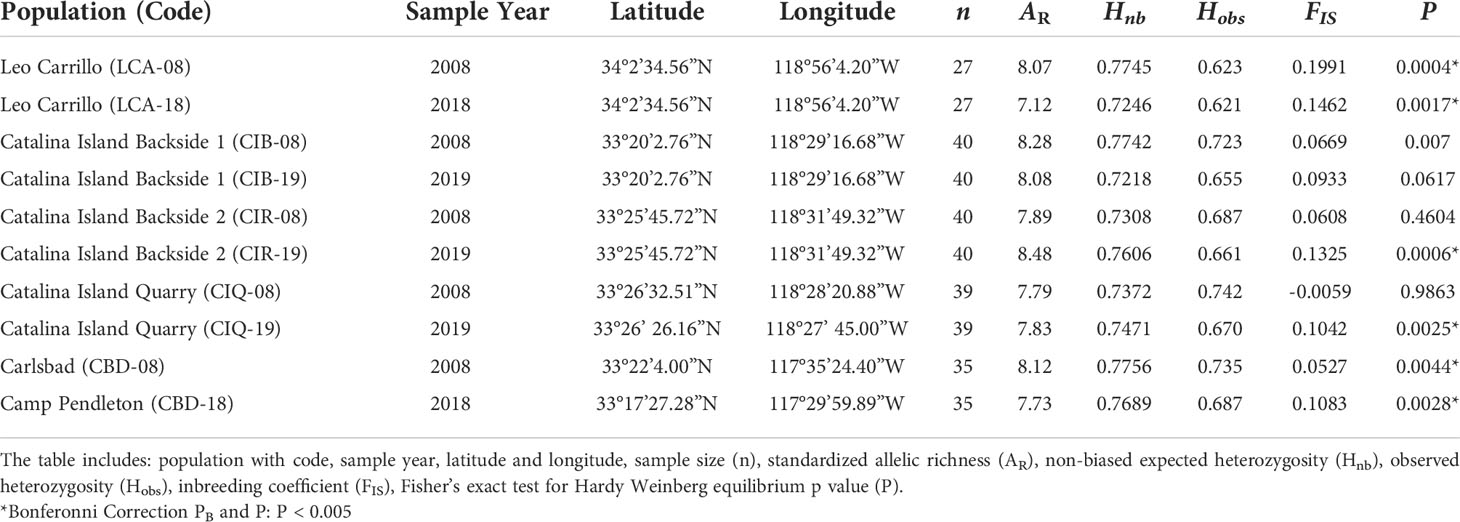
Table 2 Population genetics summary statistics for Macrocystis pyrifera temporal genetic diversity for five sites in Southern California.
Temporal analysis of genetic diversity
Despite canopy coverage loss at all sites and substantial reductions on Catalina and Camp Pendleton, all five sites showed little change in within and among-population genetic diversity following disturbance. Allelic richness in 2018 was ~10% lower in Leo Carillo but ~6% higher in Catalina Island backside; for all other sites differences between dates were small ranging from 1% increase to 3% decrease. The mean difference in standardized allelic richness between 2008-2018 paired sites was 0.43 ± 0.35 (mean alleles per locus ± sd, for n=15, Table 2; Figure 4). There was no significant change in allelic richness between paired sites across sample periods (Wilcoxon sign test: p= 0.1875). Sites retained a high level of allelic richness (mean= 7.84 alleles per locus with n standardized to 15, sd= 0.45), maintaining a pattern of high genetic diversity towards the southern distribution of the species (Johansson et al., 2015).
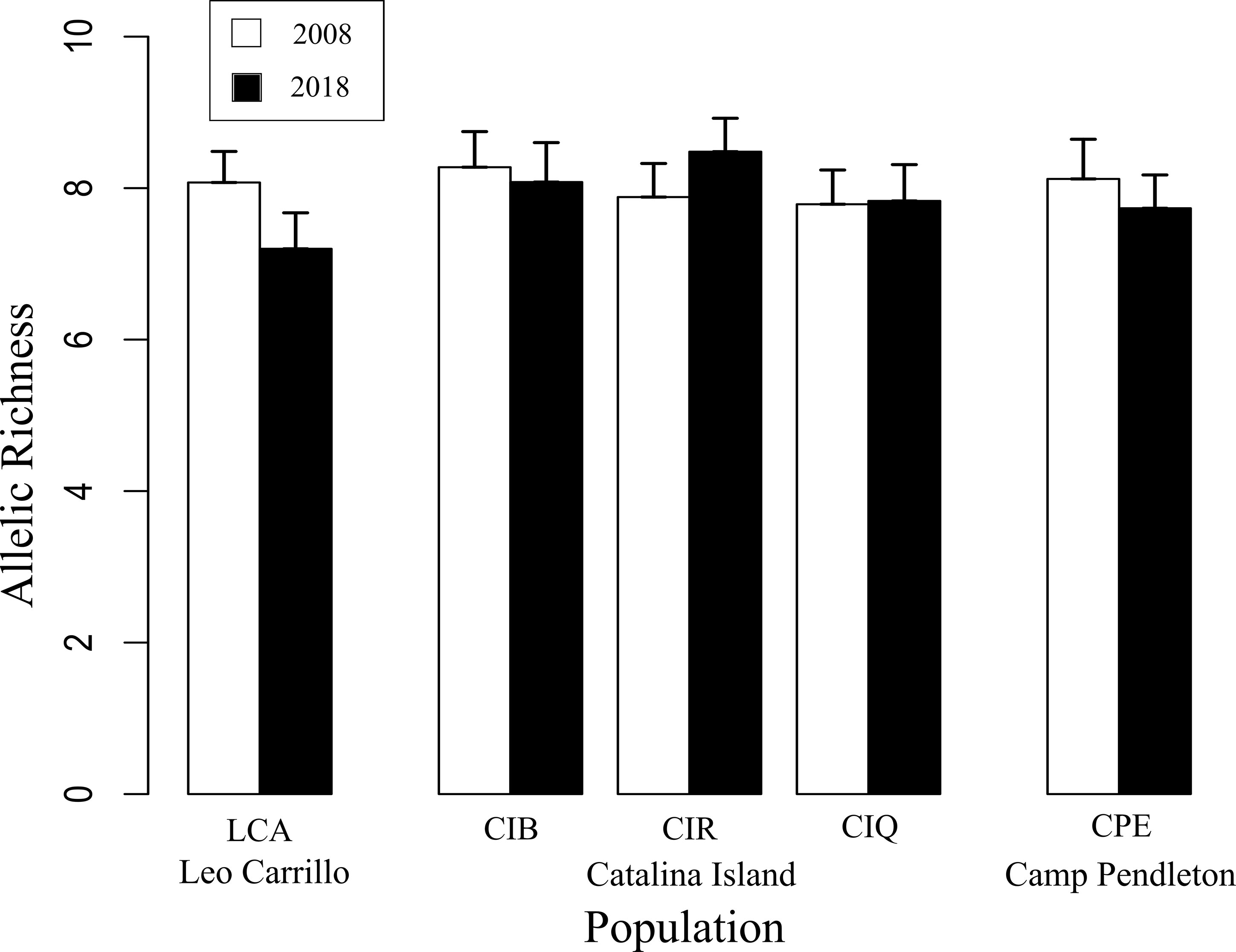
Figure 4 Pairwise comparisons of standardized microsatellite allelic richness (n=15) between 2008 and 2018/19 Macrocystis pyrifera samples at each of the three locations sampled in Southern California. No significant change in allelic richness was found between sampling periods (Wilcoxon: p= 0.1875).
Pairwise comparisons of genetic differentiation between sampling periods showed that there was little change in genetic structure following the ENSO event. Pairwise FST values were significant, except for Camp Pendleton (Table 3). The lowest FST values occurred between the two sampling dates for the same site (mean ± sd = 0.01 ± 0.006), except for the 2018 samples for both Catalina backside sites (CIB and CIR) which were genetically closer than either was to their 2008 samples (Table 3). This stability of genetic differentiation between sites was also apparent in the ordination of microsatellite alleles (FCA); 2008-2018 sampling dates paired by site in ordination space (Figure 5). Catalina remained in a genetic group containing the northern and southern Channel Islands, while Leo Carrillo and Camp Pendleton clustered with the Southern California/Baja California group (Figure 5). The comparison of STRUCTURE results between sample periods revealed that individual genetic assignments remained similar over time (Figure 6). Specimen sampled in Leo Carrillo and Camp Pendleton had their individual proportions of genetic co-ancestry assigned dominantly to one cluster while specimens from all Catalina sites were assigned to a different cluster.
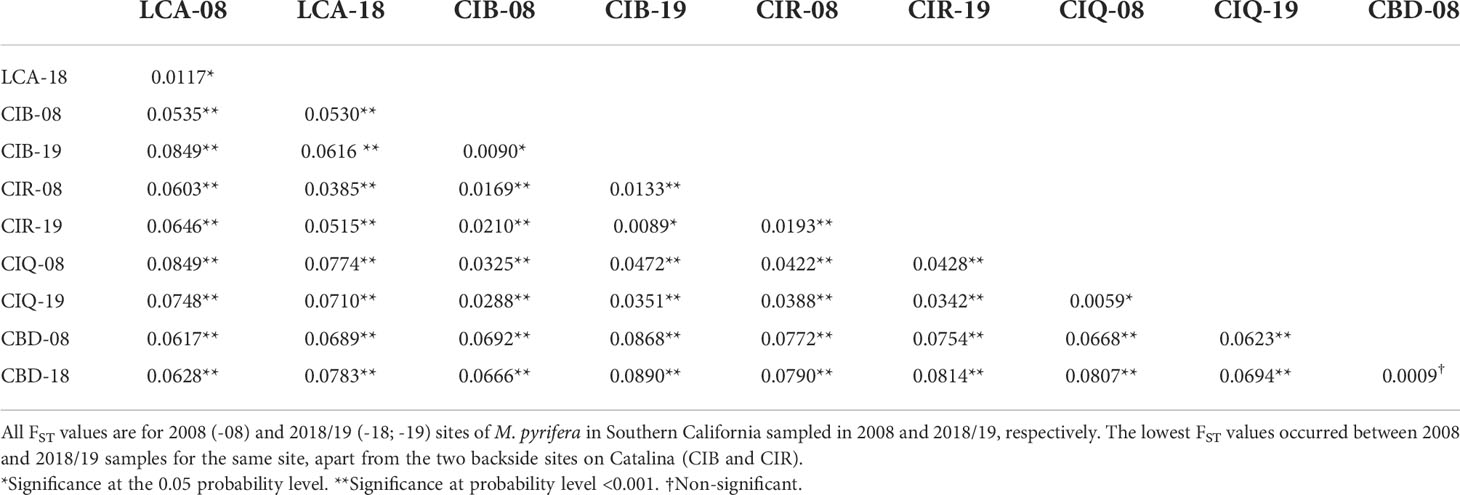
Table 3 Pairwise FST between Macrocystis pyrifera samples with G-test of significance computed in GENEPOP v. 4.7.5. .
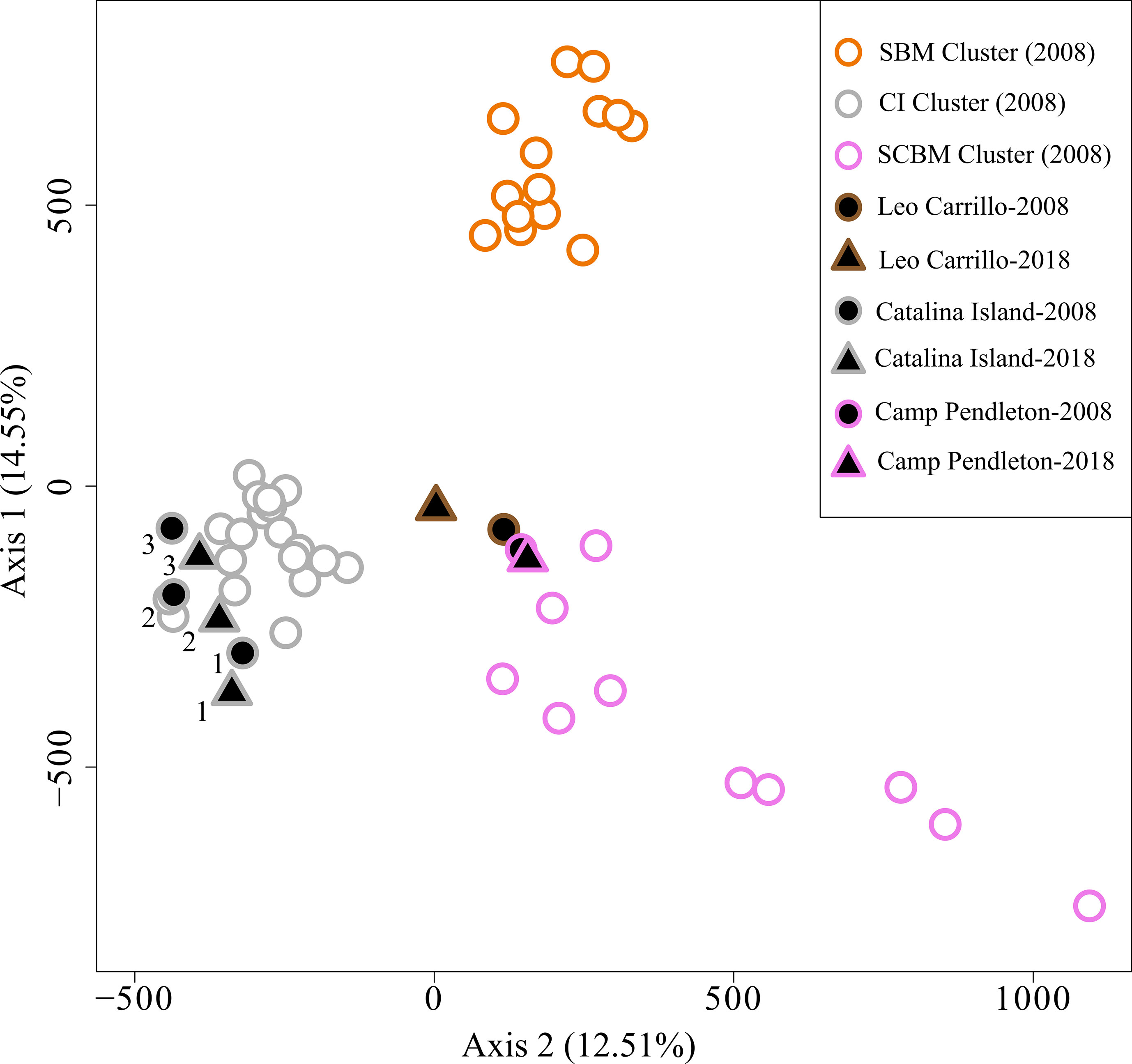
Figure 5 Factorial Correspondence Analysis (FCA) of Macrocystis pyrifera microsatellite alleles. Samples from 2008 and 2018/19 are represented with circles and triangles, respectively. Black filled symbols are for 2008-2018/19 specific comparisons made in this study while open symbols are for additional 2008 samples from Johansson et al. (2015). Samples with data only from 2008 are used here to represent the spatial genetic structure of the before and after samples studied here in the overall regional context. The outline color in symbols represents the genetic coancestry clusters identified in Johansson et al. (2015) using STRUCTURE. Samples collected at both dates, 2008 and 2018/19, clustered together reflecting minor changes in genetic structure.
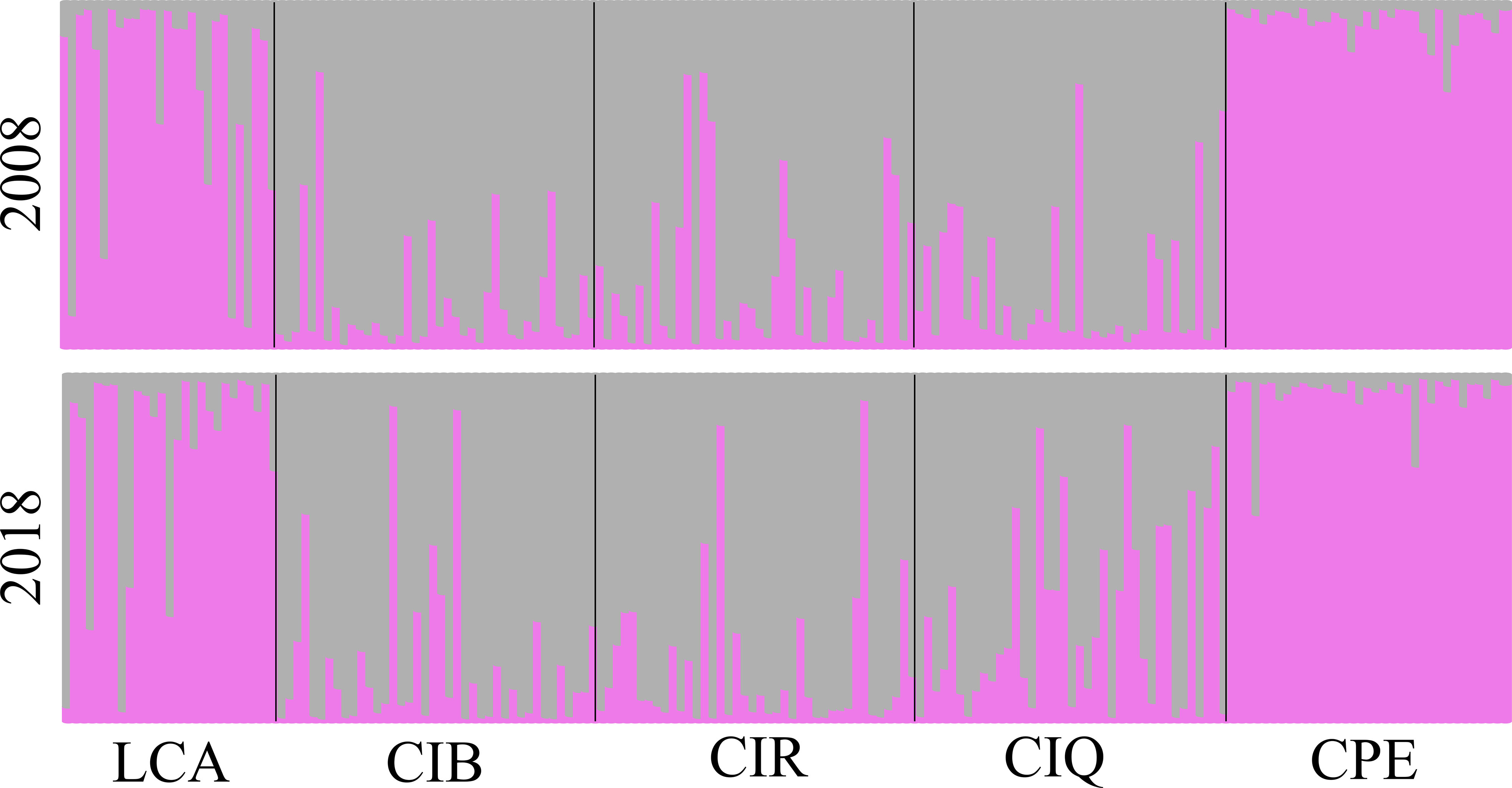
Figure 6 Structure analysis (STRUCTURE v. 2.3.4) of Macrocystis pyrifera individual assignments to two genetic co-ancestry groups. Upper pannel 2008 samples and lower pannel 2018/19 samples. Bars represent proportions of individual genetic assignment. Individual genetic assignments for all sites did not change temporally during the period studied.
In the original study describing the 2008 data, several sites showed significant homozygosity excess, including two sites used here (LCA and CBD) potentially caused by null alleles (Johansson et al., 2015). In that study, corrected measures of genetic differentiation (FST) for the presence of null alleles (FREENA method) and uncorrected ones were highly correlated and did not affect the patterns observed. In the present study, two additional sites showed homozygosity excess (CIQ and CIR). We believe that additional null alleles caused this because different PCR machines were used across studies which required PCR condition optimization. The fact that genetic differentiation between 2008 and 2018 was minimal indicates that the presence of these null alleles had negligible effects in the present study.
Discussion
In a before and after disturbance analysis of giant kelp in the Southern California Bight, we observed stable allelic richness and unchanged genetic differentiation among kelp beds. Giant kelp in the Southern California Bight can be considered a case study where genetic diversity did not follow the sizable demographic decline observed in the macroscopic sporophyte stage. These results are extremely positive regarding the conservation of giant kelp in a region where the species finds its global hotspot for genetic diversity. Although high fluctuations characterize the “macroscopic kelp” population dynamics, the canopy area in the study area has yet to recover to levels observed before the recent disturbance, and heatwaves have been increasingly common. Therefore, although we take solace that these impacts on giant kelp forests have yet to affect their rich evolutionary potential, we note that a tipping point for its decline might be closer. Consequently, our findings raise important questions regarding the mechanisms behind the maintenance of genetic diversity and structure of giant kelp in southern California.
The stability of allelic richness and genetic structure in M. pyrifera between 2008 and 2018 implies that populations in the region maintained drift-gene flow equilibrium and kept a large effective population size, despite significant canopy cover reductions at Catalina and Camp Pendleton. Genetic differentiation between sample dates (within site) was minimal, indicating that recruitment before and after disturbance occurred through a similar balance between self-replenishment and dispersal. One expectation from disturbance is decreased space competition for immigrants. Because disturbance did not affect kelp forests in SCB the same way, homogenizing gene flow from less disturbed sites could have decreased genetic differentiation. However, this was not observed, except for a stable temporal pattern of genetic diversity found between the two western sites on Catalina (CIR and CIB), where geographic and genetic distance (lower FST) between sites was minimal. Samples collected in the same year had comparably lower FST values than temporally spaced samples within the same CIR and CIB sites. Because these sites are relatively close (~10km) and thus genetically similar, we likely had insufficient power to detect minor differences in genetic differentiation. An overall increase in genetic differentiation between all sites could also be expected from increased genetic drift (Frankham, 1995) if all sites experienced demographic declines. Genetic drift is most profound in isolated populations with small numbers of individuals and could have been easily detected as a reduction in allelic richness. Nevertheless, allelic richness was remarkably stable between sampled dates, not supporting increased genetic drift. Alberto et al. (2010) estimated the effective population size (Ne) for a nearby system of giant kelp populations connected by migration under isolation by distance model. The authors estimated Ne to be between 400 to 2,400 and the ratio of effective population size to adult population size (Ne/N) to be between 0.2% to 9.6%. The small Ne/N is typical of marine systems (Hedgecock, 1994; Hare et al., 2011). However, given the size of many interconnected giant kelp patches (Cavanaugh et al., 2014), the effective population size can be still considered very large.
The maintenance of genetic diversity due to a stable gene flow and genetic drift equilibrium is informative of the role of gene flow. Kelp’s main dispersal propagules are the haploid spores produced by the sporophyte (macroalga). Thus, the observed kelp cover declines had to result in a decline in the spore source size (Reed et al., 2004). Castorani et al. (2017) found that patch connectivity in giant kelp was best explained by kelp fecundity (i.e., the density of spore-bearing sorus tissue, which scales with Landsat estimates of kelp biomass) than by spatial and temporally explicit oceanographic transport distances. Migration rates between populations are a function of the dispersal probability of individual spores and the spore source size (Gaylord et al., 2006). The fact that a reduction in source size did not alter the pattern of population genetic differentiation suggests that in the SCB population self-replenishment plays an important role despite fluctuations in spore source size. After the disturbance, either source size reduction was not limiting to normal levels of gene flow or populations retained large effective sizes in the form of non-canopy-forming sporophytes or microscopic gametophytes, or both. Understanding the relative contribution of each is challenging for populations of large effective size and weak genetic differentiation within the spatial scale of main genetic co-ancestry groups in SCB (Waples and Gaggiotti, 2006; Johansson et al., 2015).
Our Landsat kelp cover and SST analyses showed that Leo Carrillo experienced less disturbance than Camp Pendleton and Catalina. Leo Carrillo retained some canopy during disturbance regimes, while Camp Pendleton and Catalina showed significant reductions for more than two and a half years. Additionally, two large storm surges in 2014 and an increase in the abundance of the invasive alga, Sargassum horneri (Marks et al., 2015), suggested Catalina could have received greater disturbance than other locations. However, we observed that kelp canopy cover recovered more at Catalina than in Camp Pendleton following disturbance regimes from 2014 to 2016. Recruitment and maintenance of a large effective population size at Leo Carrillo can conceivably be explained by remaining adult sporophytes that survived temperature anomalies and recruited spores locally. However, the maintenance of a high effective population size for Catalina and Camp Pendleton is more intriguing given the prolonged absence of canopy at these locations. Two non-mutually exclusive mechanisms, elusive to Landsat census, could explain the maintenance of large effective population size. Firstly, given remote sensing challenges in an aquatic environment, canopy-cover-based population size estimation is biased as Landsat does not detect sub-surface juvenile sporophytes (Bell et al., 2020; Hamilton et al., 2020). In locations near Camp Pendleton, M. pyrifera annual kelp monitoring conducted by divers since the year 2000 shows a decline in the total number of fronds per individual since 2015 but small changes in density (pers. comm. Dan Reed). Lower frond number following disturbance can explain canopy decline, younger population age structure, and spore production decline. However, sufficient spore production from a large population could explain genetic stability because density was relatively stable. In Catalina Island, high density kept after disturbance is less likely to have occurred because kelp was reported absent in many locations. Secondly, local populations might survive extended periods of disturbance through more tolerant microscopic life stages, such as embryonic sporophytes or gametophytes. In comparison to adult sporophytes, the microscopic life-history stages of giant kelp are more tolerant of extreme environmental conditions (Tom Dieck, 1993; Schiel and Foster, 2015) and may be critical in population persistence during unfavorable conditions for adult sporophytes. These stages may delay growth (juvenile and embryonic sporophytes) or reproduction (gametophytes) until conditions return favorable for canopy-forming sporophytes (Ladah et al., 1999; Kinlan et al., 2003; Ladah and Zertuche-González, 2007).
A unique aspect of the 2015/16 ENSO event is that it was not associated with the onset of large storm surges throughout the region (Reed et al., 2016), which is a major factor controlling the presence of giant kelp. (Cavanaugh et al., 2011; Young et al., 2016). However, evidence of storm surge impacts on Catalina in 2014 and the delay of recovery despite favorable growth conditions in the spring of 2015 hints toward the absence of at least shallow subsurface individuals. Storms would have likely removed shallow sub-surface individuals, increased sedimentation, and limited recruitment around Catalina. In shallow water, recruitment on the Island could also have been limited by competition with the introduced and more temperature tolerant Sargassum horneri (Sullaway, 2017). Together these mechanisms imply that surviving giant kelp on the Island would be limited to deeper habitat. Catalina and other island giant kelp populations can occur much deeper than their continental counterparts, as increased light penetration enables deeper colonization. It is possible that individuals survived deeper, escaping storm effects and competition, and persisted with a nutrient influx from internal waves below the thermocline (Zimmerman and Kremer, 1984). Such observations of deep refugia have been previously noted in a Baja California population during 1997/1998 ENSO (Ladah and Zertuche-González, 2004) and in other kelp species. Following the 2015/16 ENSO, our observations of the first giant kelp recruitment in deep waters of several locations in Catalina support this hypothesis. Nevertheless, the canopy typically reforms within six months of removal (Schiel and Foster, 2015), making it less likely that subsurface adult sporophytes were solely responsible for maintaining a large effective population size when Catalina and Camp Pendleton had minimal canopy for two and a half years.
A bank of microscopic gametophyte or embryonic sporophyte stages, or both, may also contribute to the maintenance of large effective population size and consequent preservation of genetic diversity over ten years. After a period of delayed development, evidence of recruitment from these stages comes from both laboratory and field-based studies and has good support in other brown algae (Edwards, 2000; Santelices et al., 2002; Barradas et al., 2011; Edwards, 2022). However, this remains a topic of controversy for giant kelp. Laboratory studies have shown that gametophyte and juvenile sporophytes can be induced into a state of dormancy, either by limited nutrients or altered light conditions and resume reproduction and growth when suitable conditions are reestablished (Kinlan et al., 2003). Carney (2011) showed that laboratory-reared giant kelp gametophytes could resume sexual reproduction following a dormancy state for seven months. Several field studies have demonstrated the role of dormant microscopic stages in sporophyte recruitment during population recovery (Barradas et al., 2011; Carney et al., 2013). It is unknown how long such stages can survive in the wild and how much they contribute to standing population recruitment. Carney et al. (2013) employed a paternity analysis study using plots where kelp was experimentally removed, and recruits and neighboring adults were sampled for genetic analysis. By assigning the paternity of recruits to adults that had since died, the authors found that some recruits went through a delayed recruitment period of at least seven months. Following the 1997-1998 El Nino event, Ladah et al. (1999) reported giant kelp sporophyte recruitment in Baja California, after a 7-month absence of adult sporophytes, with the nearest source population of adult sporophytes over 100 km away (further than spore dispersal capability). Given their observations, Ladah et al. (1999) proposed that recruitment occurred via local gametophyte or embryonic seedbanks. Other studies have found no evidence for giant kelp recruitment via delayed microscopic stages (Deysher and Dean, 1986; Reed et al., 1997). Reed et al. (1997) suggest that most sporophyte recruitment occurs from recently released spores and not dormant stages. Additionally, other field studies conclude that gametophyte stages can survive only for short periods in the field (Deysher and Dean, 1986; Reed et al., 1994) However, these studies did not look at the contribution of dormant stages to recruitment following extreme environmental conditions, such as ENSO, or the potential for variation in dormancy capacity between different populations.
The metapopulation paradigm has been used to interpret giant kelp population dynamics (Reed et al., 2006; Cavanaugh et al., 2013; Cavanaugh et al., 2014; Castorani et al., 2015; Castorani et al., 2017). These studies were informed by kelp canopy cover dynamics (aerial photography and Landsat) and modeled connectivity. It is timely now to revise the current giant kelp metapopulation perspective with the stability and levels of genetic diversity found here and in previous studies (Alberto et al., 2010; Alberto et al., 2011; Johansson et al., 2015). Previous patch connectivity studies consider single generation dispersal distances (Cavanaugh et al., 2014; Castorani et al., 2015; Castorani et al., 2017), where most spores disperse a maximum distance of ~2 km. This scale exceeds the scale of patch demographic synchrony (~500m) in SCB (Cavanaugh et al., 2013; Cavanaugh et al., 2014). It is, therefore, appropriate to understand how connectivity mediates the interdependence of patches, e.g., their extinction and colonization dynamics at an ecological relevant time scale. However, genetic differentiation, which results from multiple generation processes including stepping-stone dispersal (Alberto et al., 2010) is weak at this spatial scale. Multiple neighboring kelp patches, asynchronous in their canopy cover extinction-recolonization dynamics (Cavanaugh et al., 2013), are essentially a single genetic population (Johansson et al., 2015). Therefore, we argue that giant kelp forests in SCB should be described as “patchy populations,” defined by Harrison (1991) as a set of habitat patches linked by high levels of dispersal or escaping in time through dormancy, or both, preventing local extinctions and strong genetic structure. Patchy population dynamics arise when dispersal takes place on a spatial scale larger than that of local events causing population fluctuations. This includes dormancy effects that extend in time the genetic background of the patch and results from (among other processes) multiple generation dispersal. Giant kelp patchy populations differ from true classical metapopulations, sensu Hanski et al. (1995), because these require true patch extinction, which for kelp need to include all microscopic life stages and not only remotely-sensed sporophyte canopy. Until the “black box” of microscopic kelp stages is truly open it will be impossible to quantify true kelp patch extinction directly. The present study suggests that in SCB true giant kelp patch population extinctions might be a rare event. Undoubtedly, sporophyte patch extinction is critical to understanding the many ecological services provided by kelp forests, but only true extinction will inform kelp forest evolutionary trajectories.
Conclusion
The stability of genetic diversity despite extensive demographic decline is a positive finding for the health of this vital habitat. However, we still know very little about the tipping points (demographic or environmental) that would impact genetic diversity. The direction of environmental change in the region is concerning; for example, the observed long series of successive days above a threshold temperature of 22 °C are recent phenomena. Cavanaugh et al. (2019) showed, for the same region and disturbance period, that giant kelp canopy cover resistance is best explained by an absolute thermal threshold (mean SST of the warmest month) than by relative increases in temperature as measured by SST anomalies and heatwave days. We showed that these canopy cover effects have yet to impact the large effective population size typical of SCB giant kelp forest, but elsewhere we are already seeing the damaging effects of climate change in giant kelp (Wernberg et al., 2016; Arafeh-Dalmau et al., 2019; Cavanaugh et al., 2019), and other kelp taxa (Rogers-Bennett and Catton, 2019; Smale, 2020).
Remote census time series of demographic and environmental variation can be used to identify specific sites for additional genetic monitoring. Any future declines in genetic diversity associated with specific demographic and environmental variation, and their contrast to the levels reported in this study, should reveal the thresholds for genetic diversity loss in M. pyrifera. The extensive sampling in Johansson et al. (2015) provides ample geographic cover for future comparisons of genetic diversity change. At the same time, the Santa Barbara Coastal LTER curates a continuous time series of giant kelp canopy biomass for Central and Southern California (LTER, S. B. C. et al., 2017), and many remotely-sensed environmental variables are freely available from NOAA. These resources can be easily combined to design a genetic monitoring program that could be part of a regional kelp management plan.
More studies are needed to understand if, where, and how gametophytes play a role in population persistence, e.g., how long they can survive in the wild without a large adult population to replenish them. In addition, recent work shows the potentially damaging effects of climate change on microscopic stages (Hollarsmith et al., 2020). With such uncertainty about the impact of climate change on kelp systems, gametophyte banking is a valuable tool that may aid in restoring future populations and further understanding of cryptic life-history stages (Wade et al., 2020). The ex-situ maintenance of genetically diverse collections of gametophytes is also vital to reseed threatened populations. This approach has already been implemented in terrestrial seed collections held by many botanical institutions (Van Dooren, 2009) but is still in its infancy in their marine analogs. Our study highlights the need to establish such collections while genetic diversity remains. These collections can be used in the laboratory and field-based studies to understand variability in the niches of microscopic stages (Hollarsmith et al., 2020) and can help target specific traits that may promote resilience for future populations (Coleman et al., 2020; Edwards, 2022).
Data availability statement
The original contributions presented in the study are publicly available. This data can be found here: doi: 10.5061/dryad.nzs7h44v9. The data link: https://datadryad.org/stash/share/fHKFVylYh5L0t1M0RLyPBvqyTXfRs5wMlzN5OaXu0lw.
Author contributions
This work was the core thesis of WK, Master of Sciences dissertation (https://dc.uwm.edu/etd/2539/); he designed the study, conducted the sampling, performed genetic analysis, and temporal analysis of environmental and kelp cover data, and finally he took the lead writing. FA, designed the study, obtained funding, supervised all aspects of the research, and edited the manuscript to the final stage. GM, contributed with DNA extraction, genotyping, and molecular lab supervision. All authors contributed to the article and approved the submitted version.
Funding
Funding was provided by the US Dept. of Energy, ARPAe MARINER project contract number DE-FOA-0001726 (AF, PI) (https://arpa-e.energy.gov/?q=arpa-e-programs/mariner), CA Sea Grant: Kelp Recovery Research Program, award C0874002. The funders had no role in study design, data collection and analysis, decision to publish, or preparation of the manuscript. The funders had no role in study design, data collection and analysis, decision to publish, or preparation of the manuscript.
Acknowledgments
We thank UW-Milwaukee for support to Klingbeil during is Master of Science (https://dc.uwm.edu/etd/2539/). We thank Dan C. Reed for sharing data on diver kelp monitoring data from the Camp Pendleton area. We thank Karly Kushway for help with the microsatellite genotyping.
Conflict of interest
The authors declare that the research was conducted in the absence of any commercial or financial relationships that could be construed as a potential conflict of interest.
Publisher’s note
All claims expressed in this article are solely those of the authors and do not necessarily represent those of their affiliated organizations, or those of the publisher, the editors and the reviewers. Any product that may be evaluated in this article, or claim that may be made by its manufacturer, is not guaranteed or endorsed by the publisher.
Supplementary material
The Supplementary Material for this article can be found online at: https://www.frontiersin.org/articles/10.3389/fmars.2022.947393/full#supplementary-material
References
Alberto F. (2009a). MsatAllele_1.0: An r package to visualize the binning of microsatellite alleles. J. Hered. 100, 394–397. doi: 10.1093/jhered/esn110
Alberto F., Raimondi P., Reed D., Coelho N., Leblois R., Whitmer A., et al. (2010). Habitat continuity and geographic distance predict population genetic differentiation in giant kelp. Ecology 91, 49–56. doi: 10.1890/09-0050.1
Alberto F., Raimondi P. T., Reed D. C., Watson J. R., Siegel D. A., Mitarai S., et al. (2011). Isolation by oceanographic distance explains genetic structure for Macrocystis pyrifera in the Santa Barbara channel. Mol. Ecol. 20, 2543–2554. doi: 10.1111/j.1365-294X.2011.05117.x
Alberto F., Whitmer C., Coelho N. C., Zippay M., Varela-Alvarez E., Raimondi P. T., et al. (2009b). Microsatellite markers for the giant kelp Macrocystis pyrifera. Conserv. Genet. 10, 1915–1917. doi: 10.1007/s10592-009-9853-9
Arafeh-Dalmau N., Montaño-Moctezuma G., Martínez J. A., Beas-Luna R., Schoeman D. S., Torres-Moye G. (2019). Extreme marine heatwaves alter kelp forest community near its equatorward distribution limit. Front. Mar. Sci. 6. doi: 10.3389/fmars.2019.00499
Araújo R. M., Assis J., Aguillar R., Airoldi L., Bárbara I., Bartsch I., et al. (2016). Status, trends and drivers of kelp forests in Europe: An expert assessment. Biodivers. Conserv. 25, 1319–1348. doi: 10.1007/s10531-016-1141-7
Arnaud J.-F., Laval G. (2004). Stability of genetic structure and effective population size inferred from temporal changes of microsatellite DNA polymorphisms in the land snail Helix aspersa (Gastropoda: Helicidae). Biol. J. Linn. Soc 82, 89–102. doi: 10.1111/j.1095-8312.2004.00320.x
Assis J., Araújo M. B., Serrão E. A. (2018a). Projected climate changes threaten ancient refugia of kelp forests in the north Atlantic. Glob. Change Biol. 24, e55–e66. doi: 10.1111/gcb.13818
Assis J., Coelho N. C., Lamy T., Valero M., Alberto F., Serrão E. A. (2016). Deep reefs are climatic refugia for genetic diversity of marine forests. J. Biogeogr. 43, 833–844. doi: 10.1111/jbi.12677
Assis J., Serrão E.Á., Coelho N. C., Tempera F., Valero M., Alberto F. (2018b). Past climate changes and strong oceanographic barriers structured low-latitude genetic relics for the golden kelp Laminaria ochroleuca. J. Biogeogr. 45, 2326–2336. doi: 10.1111/jbi.13425
Ayres K. L., Balding D. J. (1998). Measuring departures from hardy-Weinberg: A Markov chain Monte Carlo method for estimating the inbreeding coefficient. Heredity (Edinb). 80, 769–777. doi: 10.1046/j.1365-2540.1998.00360.x
Banks S. C., Cary G. J., Smith A. L., Davies I. D., Driscoll D. A., Gill A. M., et al. (2013). How does ecological disturbance influence genetic diversity? Trends Ecol. Evol. 28, 670–679. doi: 10.1016/j.tree.2013.08.005
Banzon V., Smith T. M., Chin T. M., Liu C., Hankins W. (2016). A long-term record of blended satellite and in situ sea-surface temperature for climate monitoring, modeling and environmental studies. Earth Syst. Sci. Data 8, 165–176. doi: 10.5194/essd-8-165-2016
Barradas A., Alberto F., Engelen A. H., Serrao E. A. (2011). Fast sporophyte replacement after removal suggests banks of latent microscopic stages of Laminaria ochroleuca (Phaeophyceae) in tide pools in northern Portugal. CBM-Cahiers Biol. Mar. 52, 435–439. doi: 10.21411/CBM.A.B987DCC2
Belkhir K., Borsa P., Chikhi L., Raufaste N., Bonhomme F. (2001). GENETIX 4.02, logiciel sous windows TM pour la génétique des populations. Available at: https://kimura.univ-montp2.fr/genetix/
Bell T. W., Cavanaugh K. C., Siegel D. A. (2017). SBC LTER: Time series of quarterly NetCDF files of kelp biomass in the canopy from Landsat 5, 7 and 8, 1984–2016 (ongoing). Santa Barbara Coastal LTER.
Bell T. W., Allen J. G., Cavanaugh K. C., Siegel D. A. (2020). Three decades of variability in california’s giant kelp forests from the landsat satellites. Remote Sens. Environ. 238, 110811. doi: 10.1016/j.rse.2018.06.03
Bellard C., Bertelsmeier C., Leadley P., Thuiller W., Courchamp F. (2012). Impacts of climate change on the future of biodiversity. Ecol. Lett. 15, 365–377. doi: 10.1111/j.1461-0248.2011.01736.x
Bell T. W., Cavanaugh K. C., Reed D. C., Siegel D. A. (2015). Geographical variability in the controls of giant kelp biomass dynamics. J. Biogeogr. 42, 2010–2021. doi: 10.1111/jbi.12550
Bellinger M. R., Johnson J. A., Toepfer J., Dunn P. (2003). Loss of genetic variation in greater prairie chickens following a population bottleneck in Wisconsin, USA. Conserv. Biol. 17, 717–724. doi: 10.1046/j.1523-1739.2003.01581.x
Bennett S., Wernberg T., Connell S. D., Hobday A. J., Johnson C. R., Poloczanska E. S. (2016). The `Great southern reef’: Social, ecological and economic value of australia’s neglected kelp forests. Mar. Freshw. Res. 67, 47–56. doi: 10.1071/MF15232
Bertocci I., Araújo R., Oliveira P., Sousa-Pinto I. (2015). Potential effects of kelp species on local fisheries. J. Appl. Ecol. 52, 1216–1226. doi: 10.1111/1365-2664.12483
Billot C., Engel C. R., Rousvoal S., Kloareg B., Valero M. (2003). Current patterns, habitat discontinuities and population genetic structure: The case of the kelp Laminaria digitata in the English channel. Mar. Ecol. Prog. Ser. 253, 111–121. doi: 10.3354/meps253111
Blamey L. K., Bolton J. (2018). The economic value of south African kelp forests and temperate reefs: Past, present and future. J. Mar. Syst. 188, 172–181. doi: 10.1016/j.jmarsys.2017.06.003
Butler C. L., Lucieer V. L., Wotherspoon S. J., Johnson C. R. (2020). Multi-decadal decline in cover of giant kelp Macrocystis pyrifera at the southern limit of its Australian range. Mar. Ecol. Prog. Ser. 653, 1–18. doi: 10.3354/meps13510
Carney L. T. (2011). A multispecies laboratory assessment of rapid sporophyte recruitment from delayed kelp gametophytes. J. Phycol. 47, 244–251. doi: 10.1111/j.1529-8817.2011.00957.x
Carney L. T., Bohonak A. J., Edwards M. S., Alberto F. (2013). Genetic and experimental evidence for a mixed-age, mixed-origin bank of kelp microscopic stages in southern California. Ecology 94, 1955–1965. doi: 10.1890/13-0250.1
Castorani M. C. N., Reed D. C., Alberto F., Bell T. W., Simons R. D., Cavanaugh K. C., et al. (2015). Connectivity structures local population dynamics: A long-term empirical test in a large metapopulation system. Ecology 96, 3141–3152. doi: 10.1890/15-0283.1
Castorani M. C. N., Reed D. C., Raimondi P. T., Alberto F., Bell T. W., Cavanaugh K. C., et al. (2017). Fluctuations in population fecundity drive variation in demographic connectivity and metapopulation dynamics. P. R. Soc B-Biol. Sci. 284, 20162086. doi: 10.1098/rspb.2016.2086
Cavanaugh K. C., Kendall B. E., Siegel D. A., Reed D. C., Alberto F., Assis J. (2013). Synchrony in dynamics of giant kelp forests is driven by both local recruitment and regional environmental controls. Ecology 94, 499–509. doi: 10.1890/12-0268.1
Cavanaugh K. C., Reed D. C., Bell T. W., Castorani M. N., Beas-Luna R. (2019). Spatial variability in the resistance and resilience of giant kelp in southern and Baja California to a multiyear heatwave. Front. Mar. Sci. 6. doi: 10.3389/fmars.2019.00413
Cavanaugh K., Siegel D., Kinlan B., Reed D. (2010). Scaling giant kelp field measurements to regional scales using satellite observations. Mar. Ecol. Prog. Ser. 403, 13–27. doi: 10.3354/meps08467
Cavanaugh K., Siegel D., Raimondi P., Alberto F. (2014). Patch definition in metapopulation analysis: A graph theory approach to solve the mega-patch problem. Ecology 95 (2), 316–328. doi: 10.1890/13-0221.1
Cavanaugh K., Siegel D., Reed D., Dennison P. (2011). Environmental controls of giant kelp biomass in the Santa Barbara channel, California. Mar. Ecol. Prog. Ser. 429, 1–17. doi: 10.3354/meps09141
Chung I. K., Beardall J., Mehta S., Sahoo D., Stojkovic S. (2011). Using marine macroalgae for carbon sequestration: A critical appraisal. J. Appl. Phycol. 23, 877–886. doi: 10.1007/s10811-010-9604-9
Claudet J., Fraschetti S. (2010). Human-driven impacts on marine habitats: A regional meta-analysis in the Mediterranean Sea. Biol. Conserv. 143, 2195–2206. doi: 10.1016/j.biocon.2010.06.004
Coleman M. A., Wood G., Filbee-Dexter K., Minne A. J. P., Goold H. D., Vergés A., et al. (2020). Restore or redefine: Future trajectories for restoration. Front. Mar. Sci. 7, 237. doi: 10.3389/fmars.2020.00237
Davidson A. D., Campbell M. L., Hewitt C. L., Schaffelke B. (2015). Assessing the impacts of nonindigenous marine macroalgae: An update of current knowledge. Bot. Mar. 58, 55–79. doi: 10.1515/bot-2014-0079
Dayton P. K., Tegner M. J., Parnell P. E., Edwards P. B. (1992). Temporal and spatial patterns of disturbance and recovery in a kelp forest community. Ecol. Monogr. 62, 421–445. doi: 10.2307/2937118
de la Hoz C. F., Ramos E., Puente A., Juanes J. A. (2019). Climate change induced range shifts in seaweeds distributions in Europe. Mar. Environ. Res. 148, 1–11. doi: 10.1016/j.marenvres.2019.04.012
Deysher L. E., Dean T. A. (1986). In situ recruitment of sporophytes of the giant kelp, Macrocystis pyrifera (L.) CA agardh: Effects of physical factors. J. Exp. Mar. Bio. Ecol. 103, 41–63. doi: 10.1016/0022-0981(86)90131-0
Duggins D. O., Simenstad C. A., Estes J. A. (1989). Magnification of secondary production by kelp detritus in coastal marine ecosystems. Science 245, 170–173. doi: 10.1126/science.245.4914.170
Edwards M. S. (2000). The role of alternate life-history stages of a marine macroalga: A seed bank analogue? Ecology 81, 2404–2415. doi: 10.1890/0012-9658(2000)081[2404:TROALH]2.0.CO;2
Edwards M. S. (2022). It’s the little things: The role of microscopic life stages in maintaining kelp populations. Front. Mar. Sci 9. doi: 10.3389/fmars.2022.871204
Evanno G., Castella E., Antoine C., Paillat G., Goudet J. (2009). Parallel changes in genetic diversity and species diversity following a natural disturbance. Mol. Ecol. 18, 1137–1144. doi: 10.1111/j.1365-294X.2009.04102.x
FAO (2018). The state of the world fisheries and aquaculture 2018 - meeting the sustainable development goals (Rome). Available at: https://www.fao.org/documents/card/en/c/I9540EN/
Filbee-Dexter K., Feehan C. J., Scheibling R. E. (2016). Large-Scale degradation of a kelp ecosystem in an ocean warming hotspot. Mar. Ecol. Prog. Ser. 543, 141–152. doi: 10.3354/meps11554
Flanagan S. P., Forester B. R., Latch E. K., Aitken S. N., Hoban S. (2018). Guidelines for planning genomic assessment and monitoring of locally adaptive variation to inform species conservation. Evol. Appl. 11, 1035–1052. doi: 10.1111/eva.12569
Frankham R. (1995). Effective population-size adult-population size ratios in wildlife - a review. Genet. Res. 66, 95–107. doi: 10.1017/S0016672300034455
Fraser C. I., Nikula R., Spencer H. G., Waters J. M. (2009). Kelp genes reveal effects of subantarctic sea ice during the last glacial maximum. DNA Seq. 2008, 1–5. doi: 10.1073/pnas.0810635106
Funk W. C., McKay J. K., Hohenlohe P. A., Allendorf F. W. (2012). Harnessing genomics for delineating conservation units. Trends Ecol. Evol. 27, 489–496. doi: 10.1016/j.tree.2012.05.012
García Molinos J., Halpern B. S., Schoeman D. S., Brown C. J., Kiessling W., Moore P. J., et al. (2016). Climate velocity and the future global redistribution of marine biodiversity. Nat. Clim. Change 6, 83–88. doi: 10.1038/nclimate2769
Gaylord B., Reed D. C., Raimondi P. T., Washburn L. (2006). Macroalgal spore dispersal in coastal environments: Mechanistic insights revealed by theory and experiment. Ecol. Monogr. 76, 481–502. doi: 10.1890/0012-9615(2006)076[0481:MSDICE]2.0.CO;2
Gaylord B., Reed D. C., Raimondi P. T., Washburn L., McLean S. R. (2002). A physically based model of macroalgal spore dispersal in the wave and current-dominated nearshore. Ecology 83, 1239–1251. doi: 10.1890/0012-9658(2002)083[1239:APBMOM]2.0.CO;2
Graham M. H., Vasquez J. A., Buschmann A. H. (2007). Global ecology of the giant kelp Macrocystis: From ecotypes to ecosystems. Oceanogr. Mar. Biol.: Annu. Rev. 45, 39–88. doi: 10.1201/9781420050943.ch2
Gurgel C. F. D., Camacho O., Minne A. J. P., Wernberg T., Coleman M. A. (2020). Marine heatwave drives cryptic loss of genetic diversity in underwater forests. Curr. Biol. 30, 1199–1206. doi: 10.1016/j.cub.2020.01.051
Hamilton S. L., Bell T. W., Watson J. R., Grorud-Colvert K. A., Menge B. A. (2020). Remote sensing: Generation of long-term kelp bed data sets for evaluation of impacts of climatic variation. Ecology 101 (7), e03031. doi: 10.1002/ecy.3031
Hanski I. A., Pakkala T., Kuussaari M., Lei G. C. (1995). Metapopulation persistence of an endangered butterfly in a fragmented landscape. Oikos 72, 21–28. doi: 10.2307/3546033
Hare M. P., Nunney L., Schwartz M. K., Ruzzante D. E., Burford M., Waples R. S., et al. (2011). Understanding and estimating effective population size for practical application in marine species management. Conser. Biol. 25, 438–449. doi: 10.1111/j.1523-1739.2010.01637.x
Hargarten H. L., Johansson M. L., Reed D. C., Coelho N. C., Siegel D. A., Alberto F. (2019). Seascape genetics of the stalked kelp Pterygophora californica and comparative population genetics in the Santa Barbara channel. J. Phycol. 120, 110–120. doi: 10.1111/jpy.12918
Harrison S. (1991). Local extinction in a metapopulation context- an empirical evaluation. Biol. J. Linn. Soc 42, 73–88. doi: 10.1111/j.1095-8312.1991.tb00552.x
Hedgecock D. (1994). “Does variance in reproductive successlimit effective population sizes of marine organisms,” in Genetics and evolution of aquatic organisms. Ed. Beaumont A. R. (London, UK: Chapman and Hall), 122–134.
Hernández-Carmona G., Hughes B., Graham M. H. (2006). Reproductive longevity of drifting kelp Macrocystis pyrifera (Phaeophyceae) in Monterey bay, USA 1. J. Phycol. 42, 1199–1207. doi: 10.1111/j.1529-8817.2006.00290.x
Hijmans R. J., Williams E., Vennes C. (2012) Geosphere: Spherical trigonometry. R package version 1.2–28. Available at: https://CRAN.R-project.org/package=geosphere.
Hobday A. J. (2000a). Abundance and dispersal of drifting kelp Macrocystis pyrifera rafts in the southern California bight. Mar. Ecol. Prog. Ser. 195, 101–116. doi: 10.3354/meps195101
Hobday A. J. (2000b). Age of drifting Macrocystis pyrifera (L.) c. agardh rafts in the southern California bight. J. Exp. Mar. Bio. Ecol. 253, 97–114. doi: 10.1016/S0022-0981(00)00255-0
Hobday A. J., Alexander L. V., Perkins S. E., Smale D. A., Straub S. C., Oliver E. C. J., et al. (2016). A hierarchical approach to defining marine heatwaves. Prog. Oceanogr. 141, 227–238. doi: 10.1016/j.pocean.2015.12.014
Hollarsmith J. A., Buschmann A. H., Camus C., Grosholz E. D. (2020). Varying reproductive success under ocean warming and acidification across giant kelp (Macrocystis pyrifera) populations. J. Exp. Mar. Bio. Ecol. 522, 151247. doi: 10.1016/j.jembe.2019.151247
Holt R., Kwok A., Dorken M. E. (2020). Increased spatial-genetic structure in a population of the clonal aquatic plant Sagittaria latifolia (Alismataceae) following disturbance. Heredity (Edinb). 124, 514–523. doi: 10.1038/s41437-019-0286-z
Hughes A. R., Inouye B. D., Johnson M. T. J., Underwood N., Vellend M. (2008). Ecological consequences of genetic diversity. Ecol. Lett. 11, 609–623. doi: 10.1111/j.1461-0248.2008.01179.x
Johansson M. L., Alberto F., Reed D. C., Raimondi P. T., Coelho N. C., Young M. A., et al. (2015). Seascape drivers of Macrocystis pyrifera population genetic structure in the northeast pacific. Mol. Ecol. 24, 4866–4885. doi: 10.1111/mec.13371
Johansson M. L., Raimondi P. T., Reed D. C., Coelho N. C., Serrão E. A., Alberto F. A. (2013). Looking into the black box: Simulating the role of self-fertilization and mortality in the genetic structure of Macrocystis pyrifera. Mol. Ecol. 22 (19), 4842–4854. doi: 10.1111/mec.12444
Johnson M. T. J., Lajeunesse M. J., Agrawal A. A. (2006). Additive and interactive effects of plant genotypic diversity on arthropod communities and plant fitness. Ecol. Lett. 9, 24–34. doi: 10.1111/j.1461-0248.2005.00833.x
Johnston E. L., Roberts D. A. (2009). Contaminants reduce the richness and evenness of marine communities: A review and meta-analysis. Environ. Pollut. 157, 1745–1752. doi: 10.1016/j.envpol.2009.02.017
Kinlan B. P., Graham M. H., Sala E., Dayton P. K. (2003). Arrested development of giant kelp (Macrocystis pyrifera, phaeophyceae) embryonic sporophytes: A mechanism for delayed recruitment in perennial kelps? J. Phycol. 39, 47–57. doi: 10.1046/j.1529-8817.2003.02087.x
Klingbeil W. H. (2020). Giant kelp genetic monitoring before and after disturbance reveals stable genetic diversity in southern California (Milwaukee, USA: Master of Science dissertation, University of Wisconsin Milwaukee).
Krumhansl K. A., Okamoto D. K., Rassweiler A., Novak M., Bolton J. J., Cavanaugh K. C., et al. (2016). Global patterns of kelp forest change over the past half-century. Proc. Natl. Acad. Sci. 113, 13785–13790. doi: 10.1073/pnas.1606102113
Krumhansl K. A., Scheibling R. E. (2012). Production and fate of kelp detritus. Mar. Ecol. Prog. Ser. 467, 281–302. doi: 10.3354/meps09940
Ladah L. B., Zertuche-González J. A. (2004). Giant kelp (Macrocystis pyrifera) survival in deep water (25–40 m) during El niño of 1997–1998 in Baja California, Mexico. Bot. Mar. 47 (5), 367–372. doi: 10.1046/j.1529-8817.1999.3561106.x
Ladah L. B., Zertuche-González J. A. (2007). Survival of microscopic stages of a perennial kelp (Macrocystis pyrifera) from the center and the southern extreme of its range in the northern hemisphere after exposure to simulated El niño stress. Mar. Biol. 152, 677–686. doi: 10.1007/s00227-007-0723-z
Ladah L. B., Zertuche-Gonzalez J. A., Hernandez-Carmona G. (1999). Giant kelp (Macrocystis pyrifera, phaeophyceae) recruitment near its southern limit in Baja California after mass disappearance during ENSO 1997-1998. J. Phycol. 35, 1106–1112. doi: 10.1046/j.1529-8817.1999.3561106.x
Macaya E. C. (2010). Phylogeny, connectivity and dispersal patterns of the giant kelp macrocystis (Phaeophyceae) (Wellington, New Zealand: Doctoral dissertation, Victoria University of Wellington).
Manent P., Bañolas G., Alberto F., Curbelo L., Espino F., Tuya F. (2020). Long-term seagrass degradation: Integrating landscape, demographic, and genetic responses. Aquat. Conserv. Mar. Freshw. Ecosyst. 30, 1111–1120. doi: 10.1002/aqc.3325
Mann K. H. (1973). Seaweeds: Their productivity and strategy for growth: The role of large marine algae in coastal productivity is far more important than has been suspected. Science 182, 975–981. doi: 10.1126/science.182.4116.975
Mao X., Augyte S., Huang M., Hare M. P., Bailey D., Umanzor S., et al. (2020). Population genetics of sugar kelp throughout the northeastern united states using genome-wide markers. Front. Mar. Sci. 7, 694. doi: 10.3389/fmars.2020.00694
Marks L. M., Reed D. C., Obaza A. K. (2017). Assessment of control methods for the invasive seaweed Sargassum horneri in California, USA. Manage. Biol. Invasions 8, 205–213. doi: 10.3391/mbi.2017.8.2.08
Marks L. M., Salinas-Ruiz P., Reed D. C., Holbrook S. J., Culver C. S., Engle J. M., et al. (2015). Range expansion of a non-native, invasive macroalga Sargassum horneri (Turner) c. agardh 1820 in the eastern pacific. BioInvasions Rec 4, 243–248. doi: 10.3391/bir.2015.4.4.02
McCauley D. J., Pinsky M. L., Palumbi S. R., Estes J. A., Joyce F. H., Warner R. R. (2015). Marine defaunation: Animal loss in the global ocean. Science 347, 1255641. doi: 10.1126/science.1255641
Mineur F., Arenas F., Assis J., Davies A. J., Engelen A. H., Fernandes F., et al. (2015). European Seaweeds under pressure: Consequences for communities and ecosystem functioning. J. Sea. Res. 98, 91–108. doi: 10.1016/j.seares.2014.11.004
Muth A. F., Graham M. H., Lane C. E., Harley C. D. G. (2019). “Recruitment tolerance to increased temperature present across multiple kelp clades.” e02594. doi: 10.1002/ecy.2594
Neiva J., Paulino C., Nielsen M. M., Krause-Jensen D., Saunders G. W., Assis J., et al. (2018). Glacial vicariance drives phylogeographic diversification in the amphi-boreal kelp Saccharina latissima. Sci. Rep. 8, 1–12. doi: 10.1038/s41598-018-19620-7
Oliver E. C. J., Donat M. G., Burrows M. T., Moore P. J., Smale D. A., Alexander L. V., et al. (2018). Longer and more frequent marine heatwaves over the past century. Nat. Commun. 9, 1–12. doi: 10.1038/s41467-018-03732-9
Orsini L., Marshall H., Cuenca Cambronero M., Chaturvedi A., Thomas K. W., Pfrender M. E., et al. (2016). Temporal genetic stability in natural populations of the waterflea Daphnia magna in response to strong selection pressure. Mol. Ecol. 25, 6024–6038. doi: 10.1111/mec.13907
Pakker H., Flores-Moya A. (2000). Impact of UV-radiation on viability , photosynthetic characteristics and DNA of brown algal zoospores : Implications for depth zonation. Mar. Ecol. Prog. Ser. 197, 217–229. doi: 10.3354/meps197217
Pannell J. R., Charlesworth B. (2000). Effects of metapopulation processes on measures of genetic diversity. Philos. Trans. R. Soc London. Ser. B Biol. Sci. 355, 1851–1864. doi: 10.1098/rstb.2000.0740
Pritchard J. K., Stephens M., Donnelly P. (2000). Inference of population structure using multilocus genotype data. Genetics 155, 945–959. doi: 10.1093/genetics/155.2.945
Rahel F. J., Olden J. D. (2008). Assessing the effects of climate change on aquatic invasive species. Conserv. Biol. 22, 521–533. doi: 10.1111/j.1523-1739.2008.00950.x
R Core Team (2019). “R: A language and environment for statistical computing,” in R foundation for statistical computing.(Vienna, Austria). Available at: https://www.R-project.org/.
Reed D. C. (1990). The effects of variable settlement and early competition on patterns of kelp recruitment. Ecology 71, 776–787. doi: 10.2307/1940329
Reed D. C., Amsler C. D., Ebeling A. W. (1992). Dispersal in kelps - factors affecting spore swimming and competence. Ecology 73, 1577–1585. doi: 10.2307/1940011
Reed D. C., Anderson T. W., Ebeling A. W., Anghera M. (1997). The role of reproductive synchrony in the colonization potential of kelp. Ecology 78, 2443–2457. doi: 10.1890/0012-9658(1997)078[2443:TRORSI]2.0.CO;2
Reed D. C., Kinlan B. P., Raimondi P. T., Washburn L., Gaylord B., Drake P. T. (2006). “A metapopulation perspective on the patch dynamics of giant kelp in southern california,” in Marine metapopulations. Eds. Kritzer J. P., Sale P. F. (Academic Press: San Diego, California, USA), 353–386.
Reed D. C., Lewis R. J., Anghera M. (1994). Effects of an open-coast oil-production outfall on patterns of giant kelp (Macrocystis pyrifera) recruitment. Mar. Biol. 120, 25–31. doi: 10.1007/BF00381938
Reed D. C., Schroeter S. C., Raimondi P. T. (2004). Spore supply and habitat availability as sources of recruitment limitation in the giant kelp Macrocystis pyrifera (Phaeophyceae). J. Phycol. 40, 275–284. doi: 10.1046/j.1529-8817.2004.03119.x
Reed D., Washburn L., Rassweiler A., Miller R., Bell T., Harrer S. (2016). Extreme warming challenges sentinel status of kelp forests as indicators of climate change. Nat. Commun. 7 (1), 1–7. doi: 10.1038/ncomms13757
Reusch T. B. H., Ehlers A., Hammerli A., Worm B. (2005). Ecosystem recovery after climatic extremes enhanced by genotypic diversity. P. Natl. Acad. Sci-Biol. 102, 2826–2831. doi: 10.1073/pnas.0500008102
Reynolds L. K., Stachowicz J. J., Hughes A. R., Kamel S. J., Ort B. S., Grosberg R. K. (2017). Temporal stability in patterns of genetic diversity and structure of a marine foundation species (Zostera marina). Heredity 118, 404–412. doi: 10.1038/hdy.2016.114
Rogers-Bennett L., Catton C. A. (2019). Marine heat wave and multiple stressors tip bull kelp forest to sea urchin barrens. Sci. Rep. 9, 1–9. doi: 10.1038/s41598-019-51114-y
Rousset F. (2008). GENEPOP ‘ 007: A complete re-implementation of the GENEPOP software for windows and Linux. Mol. Ecol. Resour. 8, 103–106. doi: 10.1111/j.1471-8286.2007.01931.x
Rousset F., Raymond M. (1995). Testing heterozygote excess and deficiency. Genetics 140, 1413–1419. doi: 10.1093/genetics/140.4.1413
Santelices B., Aedo D., Hoffmann A. (2002). Banks of microscopic forms and survival to darkness of propagules and microscopic stages of macroalgae. Rev. Chil. Hist. Natural 75, 547–555. doi: 10.4067/S0716-078X2002000300006
Schiel D. R., Foster M. S. (2015). The biology and ecology of giant kelp forests (Oakland: University of California Press).
Schlegel R. W., Smit A. J. (2018). heatwaveR: A central algorithm for the detection of heatwaves and cold-spells. J. Open Source Softw. 3, 821. doi: 10.21105/joss.00821
Schwartz M. K., Luikart G., Waples R. S. (2007). Genetic monitoring as a promising tool for conservation and management. Trends Ecol. Evol. 22, 25–33. doi: 10.1016/j.tree.2006.08.009
Smale D. A. (2020). Impacts of ocean warming on kelp forest ecosystems. New Phytol. 225, 1447–1454. doi: 10.1111/nph.16107
Solomon S. (2007). The physical science basis: Contribution of working group I to the fourth assessment report of the intergovernmental panel on climate change. Intergov. Panel Clim. Change (IPCC) Clim. Change 2007, 996. Available at: https://archive.ipcc.ch/publications_and_data/ar4/wg1/en/contents.html
Spear S. F., Crisafulli C. M., Storfer A. (2012). Genetic structure among coastal tailed frog populations at mount st. helens is moderated by post-disturbance management. Ecol. Appl. 22, 856–869. doi: 10.1890/11-0627.1
Steneck R. S., Graham M. H., Bourque B. J., Corbett D., Erlandson J. M., Estes J. A., et al. (2002). Kelp forest ecosystems: Biodiversity, stability, resilience and future. Environ. Conserv. 29, 436–459. doi: 10.1017/S0376892902000322
Sudo K., Watanabe K., Yotsukura N., Nakaoka M. (2020). Predictions of kelp distribution shifts along the northern coast of Japan. Ecol. Res. 35, 47–60. doi: 10.1111/1440-1703.12053
Sullaway G. H. (2017). Assessing the impact of the invasive species, sargassum horneri, on kelp forest net community production (San Diego, USA: Doctoral dissertation, San Diego State University).
Tellier F., Meynard A. P., Correa J. A., Faugeron S., Valero M. (2009). Phylogeographic analyses of the 30° s south-east pacific biogeographic transition zone establish the occurrence of a sharp genetic discontinuity in the kelp Lessonia nigrescens: Vicariance or parapatry? Mol. Phylogenet. Evol. 53, 679–693. doi: 10.1016/j.ympev.2009.07.030
Tilman D., Clark M., Williams D. R., Kimmel K., Polasky S., Packer C. (2017). Future threats to biodiversity and pathways to their prevention. Nature 546, 73–81. doi: 10.1038/nature22900
Timpane-Padgham B. L., Beechie T., Klinger T. (2017). A systematic review of ecological attributes that confer resilience to climate change in environmental restoration. PloS One 12, e0173812. doi: 10.1371/journal.pone.0173812
Tom Dieck I. (1993). Temperature tolerance and survival in darkness of kelp gametophytes (Laminariales, phaeophyta): Ecological and biogeographical implications. Mar. Ecol. Ser. 100, 253–253. doi: 10.3354/meps100253
Valero M., Destombe C., Mauger S., Ribout C., Engel C. R., Daguin-Thiébaut C., et al. (2011). Using genetic tools for sustainable management of kelps: A literature review and the example of Laminaria digitata. CBM-Cahiers Biol. Mar. 52, 467–483. doi: 10.21411/CBM.A.A57922DD
Van Dooren T. (2009). Banking seed: Use and value in the conservation of agricultural diversity. Sci. Cult. (Lond) 18, 373–395. doi: 10.1080/09505430902873975
Vergés A., Doropoulos C., Malcolm H. A., Skye M., Garcia-Pizá M., Marzinelli E. M., et al. (2016). Long-term empirical evidence of ocean warming leading to tropicalization of fish communities, increased herbivory, and loss of kelp. Proc. Natl. Acad. Sci. 113, 13791–13796. doi: 10.1073/pnas.1610725113
Vilalta-Navas A., Beas-Luna R., Calderon-Aguilera L. E., Ladah L., Micheli F., Christensen V., et al. (2018). A mass-balanced food web model for a kelp forest ecosystem near its southern distributional limit in the northern hemisphere. Food Webs 17, e00091. doi: 10.1016/j.fooweb.2018.e00091
Wade R., Augyte S., Harden M., Nuzhdin S., Yarish C., Alberto F. (2020). Macroalgal germplasm banking for conservation, food security, and industry. PloS Biol. 18, e3000641. doi: 10.1371/journal.pbio.3000641
Walker B., Holling C. S., Carpenter S. R., Kinzig A. (2004). Resilience, adaptability and transformability in social–ecological systems. Ecol. Soc 9 (2). doi: 10.5751/ES-00650-090205
Waples R. S., Gaggiotti O. (2006). What is a population? an empirical evaluation of some genetic methods for identifying the number of gene pools and their degree of connectivity. Mol. Ecol. 15, 1419–1439. doi: 10.1111/j.1365-294X.2006.02890.x
Wernberg T., Bennett S., Babcock R. C., De Bettignies T., Cure K., Depczynski M., et al. (2016). Climate-driven regime shift of a temperate marine ecosystem. Science 353, 169–172. doi: 10.1126/science.aad8745
Wootton J. T., Pfister C. A. (2013). Experimental separation of genetic and demographic factors on extinction risk in wild populations. Ecology 94, 2117–2123. doi: 10.1890/12-1828.1
Young M., Cavanaugh K., Bell T., Raimondi P., Edwards C. A., Drake P. T., et al. (2016). Environmental controls on spatial patterns in the long-term persistence of giant kelp in central California. Ecol. Monogr. 86, 45–60. doi: 10.1890/15-0267.1
Keywords: genetic monitoring, giant kelp, disturbance, microsatellite, metapopulation, patchy population, climate-change
Citation: Klingbeil WH III, Montecinos GJ and Alberto F (2022) Giant kelp genetic monitoring before and after disturbance reveals stable genetic diversity in Southern California. Front. Mar. Sci. 9:947393. doi: 10.3389/fmars.2022.947393
Received: 18 May 2022; Accepted: 17 August 2022;
Published: 08 September 2022.
Edited by:
Anne Chenuil, Centre National de la Recherche Scientifique (CNRS), FranceReviewed by:
Alejandro H. Buschmann, University of Los Lagos, ChileJean-baptiste Ledoux, University of Porto, Portugal
Copyright © 2022 Klingbeil, Montecinos and Alberto. This is an open-access article distributed under the terms of the Creative Commons Attribution License (CC BY). The use, distribution or reproduction in other forums is permitted, provided the original author(s) and the copyright owner(s) are credited and that the original publication in this journal is cited, in accordance with accepted academic practice. No use, distribution or reproduction is permitted which does not comply with these terms.
*Correspondence: Filipe Alberto, YWxiZXJ0b2ZAdXdtLmVkdQ==