- School of Biological Sciences, Georgia Institute of Technology, Atlanta, GA, United States
Predators control prey abundance and behavior, both of which strongly influence community dynamics. However, the relative importance of these predator effects may shift with climate change stressors, suggesting understanding the potential effects on these different processes is critical to predicting effects of climate change on community function. We investigated the effects of global warming and ocean acidification on the transmission and detection of chemical cues from blue crab predators (Callinectes sapidus) by mud crab prey (Panopeus herbstii). We measured mud crab feeding rates in the presence of blue crab predator cues, using either predator cues stressed in acidified conditions or mud crabs stressed in warmed and acidified conditions. Mud crabs consumed less food in the presence of predator cues, but acidifying the cues or subjecting mud crabs receiving the cues to acidified environment did not affect this antipredator response. Mud crabs in warmed conditions consumed significantly less food regardless of predator cue, but this effect was reversed in ambient conditions. Therefore, climate change may produce shifts in community regulation as warming potentially compromises consumptive effects of predators by reducing motor function, whereas non-consumptive effects mediated by sensory transmission and detection remain unaffected by acidification. Overall, warming may have stronger effects than acidification on community dynamics in oyster reefs as global temperatures continue to rise.
1 Introduction
Predator-prey interactions strongly influence ecological communities. Predators affect community structure by changing prey abundance (consumptive effects - CEs) or when predator presence alters prey properties such as morphology, development, and behavior (non-consumptive effects - NCEs) (Werner and Peacor, 2003). NCEs can outweigh CEs in situations where conditions allow for effective detection of predators because many more individuals will respond simultaneously, which can be the case in some aquatic systems (Werner and Peacor, 2003; Preisser et al., 2005; Weissburg et al., 2014).
The environmental context for predator and prey strongly influences their interactions, which is especially important to consider as anthropogenic climate change is drastically altering coastal ecosystems worldwide (Hoegh-Guldberg and Bruno, 2010; IPCC, 2014). Increased carbon dioxide (CO2) emissions are increasing global surface temperatures by recapturing heat from the sun (i.e. global warming), while excess CO2 is simultaneously being absorbed into the oceans and altering seawater chemistry to make marine and coastal environments more acidic (i.e. ocean acidification) (Doney et al., 2009; IPCC, 2014). By the end of the century, global mean surface temperatures are likely to exceed an additional 2°C, and global ocean pH is expected to decrease by 0.3-0.4 units under the business-as-usual scenario (IPCC, 2014).
Warming and acidification are negatively impacting many species interactions, including those between predator and prey (Nagelkerken and Munday, 2016). Warming affects basal metabolic processes and stresses organisms beyond their thermal limits (Pörtner and Farrell, 2008; Pörtner et al., 2017). Additionally, increased temperatures can increase predator foraging activity to meet higher metabolic demands (as seen in fish and crustaceans; Wu et al., 2017; Goldenberg et al., 2018), while prey activity that provides for escape behaviors may decrease (Kidawa et al., 2010). Acidification not only contributes to stress on physiological processes for predator and prey (especially calcification rates – Ries et al., 2009), but also may alter sensory capabilities underlying predator-prey interactions (reviewed by Draper and Weissburg, 2019).
Most studies that examine climate change effects on predator-prey interactions focus on the behavioral endpoint such as escape time or survival rate, which primarily affect CEs (Draper and Weissburg, 2019). However, the relative strengths of CEs and NCEs may shift with climate change depending on the mechanism of predator control affected, the magnitude of the effect, and in response to which stressor (e.g. warming or acidification). Warming may primarily change CEs by affecting movement of predator and prey that determines encounter rate, while acidification potentially affects NCEs via disruption of the sensory transduction pathway where cue production, transmission, and perception of predation risk determine behavioral outcomes (Draper and Weissburg, 2019). Recent climate change studies have focused on individual components of the sensory pathway between predator and prey, but more realistic predictions should include multiple steps of sensory information exchange. Current literature is biased toward effects on tropical reef fish (Draper and Weissburg, 2019), and the effects of climate change have been particularly understudied in temperate coastal ecosystems such as oyster reef communities.
Oyster reefs are ecologically and economically important ecosystems along the Atlantic and Gulf coasts of the United States (Beck et al., 2011) and are connected to other important marine processes within estuarine environments (McCormick-Ray, 2005). Oyster reefs are ecologically important by providing ecosystem services such as habitat stabilization, creation of refugia from predation, water filtration, and protection from shoreline erosion (Grabowski et al., 2012). Economically, blue crabs and oysters historically have provided important fisheries, but overharvesting has caused most of oyster reef communities to collapse (Jackson et al., 2001; Kirby, 2004). Currently, more than 85% of oyster reefs worldwide have degraded due to local and global stressors caused by anthropogenic activities (Beck et al., 2011).
To provide insight into how warming and acidification will influence different mechanisms of predator control in oyster reefs, we used the model system of blue crab predators (Callinectes sapidus) and mud crab prey (Panopeus herbstii). Blue crabs are important predators in estuaries by feeding on bivalves and crustaceans such as mud crabs (Micheli, 1997; Hill and Weissburg, 2013). Mud crabs are small, cryptic xanthid crabs that live within oyster beds (Meyer, 1994) and are important consumers of oysters (Bisker and Castagna, 1987). As a result, blue crab predation on mud crabs (CEs) and foraging suppression in mud crabs when they sense blue crab predators (NCEs) both increase oyster survival (Grabowski et al., 2008; Hill and Weissburg, 2013; Pruett and Weissburg, 2021). Limited studies have examined predator-prey interactions with these species in response to climate change stressors. Dodd and colleagues (2015) tested mud crab (Panopeus herbstii) foraging behavior on eastern oysters (Crassostrea virginica) under acidified conditions. Although both species experienced reduced calcification rates, acidification reduced handling time and prey consumption by mud crabs. Another study examined foraging behavior of blue crabs (Callinectes sapidus) on soft-shell clams (Mya arenaria) in acidified waters (Glaspie et al., 2017). Acidification reduced both calcification rates and antipredator behavior in clams, but it also reduced effective foraging ability by blue crabs, resulting in no change in predator-related clam mortality. However, these studies on blue crabs and mud crabs fail to consider how both mechanisms of predator control (i.e. CEs and NCEs) will change with warming and acidification.
In this study, we tested the effects of warming and acidification on chemosensory transmission and detection by stressing (i.e. exposing to elevated temperature and/or reduced pH) mud crabs and blue crab predator chemical cues and measuring mud crab feeding rates in the presence of these predator cues. As it has been suggested that mud crabs suppress aerobic metabolism in temperatures above 30°C (Dame and Vernberg, 1978), we hypothesized that warming in this study would increase physical stress that consequently reduces food consumption rates. Acidification can differentially affect cue transmission (via changes to cue itself) and detection (via changes to cue receptor or neural processing) (Draper and Weissburg, 2019), so predictions for acidification effects on NCEs were less clear. Since the bioactive molecules present in blue crab predator cue (Poulin et al., 2018) contain functional groups that are predicted to be robust to CO2-induced acidification (see Roggatz et al., 2016), we hypothesized that acidification in this study would not affect cue transmission and mud crab feeding responses would be unaffected. However, it is unknown whether mud crab perception of predators is weakened by acidification that would consequently reduce mud crab behavioral responses (i.e. foraging suppression) to predator cues.
2 Methods
2.1 Animal collection and maintenance
Adult blue crabs (10-14 cm carapace width; CW) and mud crabs (15-30 mm CW) were obtained from Wassaw Sound (Savannah, GA, USA) and associated tributaries in 2019 and 2021, under a scientific collection permit (29-WJH-16-222) issued by the Georgia Department of Natural Resources and renewed annually. Adult blue crabs were caught using baited crab traps, and mud crabs were collected by hand from oyster reefs during low tide. Animals were transported to Georgia Institute of Technology (GT) in Atlanta, GA and each species was separately housed in 37 L aquarium tanks.
Tanks were filled with artificial seawater (ASW; Instant Ocean™) and equipped with basic filtration, heaters, and aerators to maintain conditions similar to the collection site (25 psu salinity, 26-28°C water temperature, 7.8-8.0 pH). Partial water changes (25-50%) were conducted weekly to prevent waste buildup. Animals were individually isolated in labeled holding chambers to prevent antagonistic interactions, fed raw shrimp ad libitum twice per week, and acclimated to laboratory conditions (including a 12:12 light:dark cycle) for at least one week before experiments began. All crabs used in experiments were sexed, weighed, measured (carapace width), and number of injuries recorded. Only intermolt animals with claws intact and less than three missing pereopods were selected for experimentation.
2.2 Experiment design – stressed mud crabs
We tested for climate change effects on crab behavioral responses using 16 independent 37 L tanks to create a 2 x 2 factorial design with warming (two temperature levels: 28.6°C and 35.0°C) and acidification (two pH levels: 7.8 and 7.2) as treatments, with 4 replicate tanks for each treatment combination. Target baseline (control) conditions were determined using mean summer (May – August) temperature and pH values from 2007 – 2016 from Sapelo Island NERR monitoring station (Lower Duplin River shallow subtidal; 2-hour continuous monitoring). For climate change projections, we used the high intermediate emissions scenario (RCP 8.5), which predicts an average 3.7°C increase of temperature and 0.3 units decrease of pH by 2100 (IPCC, 2014). However, estuary environments fluctuate strongly so that current organisms may be exposed to daily extreme conditions that are similar to average predicted conditions in IPCC business-as-usual climate change models (Hofmann et al., 2011; IPCC, 2014). For example, standard deviations from the Sapelo Island dataset for mean temperature and pH are 2.7°C and 0.3 units respectively, and a maximum water temperature of 39°C at ~3 m depth. (However, we note that mean temperatures are often unreliable indicators of body temperature and thermal stress in topographically complex habitats where body position, orientation, and substrate variation have considerable effects (Helmuth and Hofmann, 2001; Jost and Helmuth, 2007). Additionally, coastal environments are projected to experience even more extreme conditions than predicted for the global average (i.e. open ocean) (Helmuth et al., 2002; Wootton et al., 2008; Waldbusser and Salisbury, 2014). This includes increased magnitude of extreme temperatures (Helmuth et al., 2002), as well as extreme pH values (Kwiatkowski and Or, 2018; Pacella et al., 2018) due to variations in salinity (i.e. buffer capacity) and DIC inputs from benthic organic matter (Cai and Wang, 1998; Ringwood and Keppler, 2002). Therefore, we determined projected future conditions for our system by combining IPCC predictions with one standard deviation from the mean baseline temperature and pH values provided by the NERR monitoring station (mean + IPCC predictions + 1 standard deviation). Target stress treatments were calculated as follows: 28.6 + 3.7 + 2.7 = 35.0°C for temperature, and 7.8 - 0.3 - 0.3 = 7.2 units for pH.
2.2.1 Seawater carbonate chemistry
All treatments were maintained using a microprocessor-based system (AquaMedic) where temperature and pH were continuously monitored and adjusted by automatic heaters and CO2 input (via solenoid valves and pH probes) in response to conditions in each individual tank. Heaters and pH probe/valve systems had 1°C and 0.1 unit differentials, respectively. All pH probes (Cole-Parmer Oakton) for the CO2 system were calibrated weekly, and water parameters (pH, temperature, salinity) were measured daily using a handheld Orion Star A325 meter with temperature-compensated pH and conductivity electrodes. Water samples were collected weekly from each tank, filtered using 0.45 μm filtration, and stored in polypropylene vials at 4°C for up to 6 months for later analysis of alkalinity (Mos et al., 2021). Total alkalinity was determined using a Hanna 901c precision automatic titrator and certified seawater standards (Dickson standard, Scripps Institution of Oceanography). The pCO2 and saturation states of calcite and aragonite were calculated using the CO2SYS program (Pierrot et al., 2006), using the first and second dissociation constants of Mehrbach et al. (1973) as refit by Dickson and Millero (1987). These methods provided highly consistent temperature and pH offset conditions (Table 1).
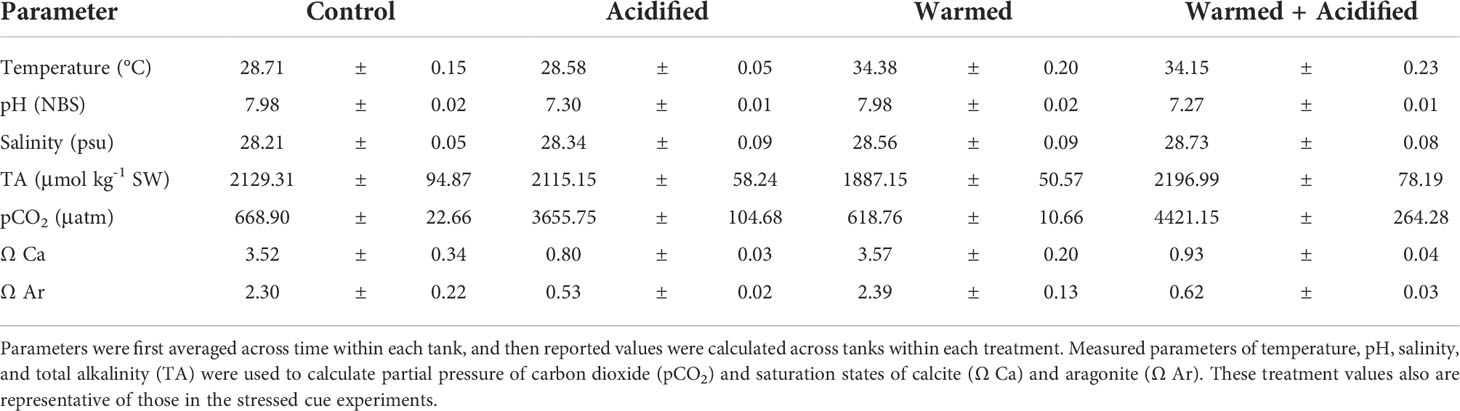
Table 1 Mean (± SE) seawater carbonate chemistry parameters during the 5-week exposure period of the stressed crab experiment for each treatment (n = 4 tanks).
Our treatments were designed to examine the effects of projected pH values rather than CO2, which may be overestimated here due to higher alkalinity in artificial seawater. While higher alkalinity leads to higher calculated pCO2 values on a pH-based system, these pCO2 values are still reasonable for predicted future conditions of coastal environments. For example, Chesapeake Bay can experience pCO2 values as high as 10,000 μatm (Glandon et al., 2019). Therefore, these methods align with those used in other acidification studies with crustaceans (reviewed by Dissanayake, 2014).
2.2.2 Exposure period
The exposure period began by gradually increasing the temperature and decreasing the pH (via increased CO2 input) in each tank over four days until target treatment conditions were achieved. Mud crabs were then exposed to stress treatments for 5 weeks, which is sufficient time for these stressors to produce physiological effects in this species that may influence behavior (Draper, unpublished data). The experiment began with 15 mud crabs per tank and total biomass per tank was balanced to minimize variation in waste buildup. Survivorship and molting events were monitored daily, and molts/dead individuals were promptly removed from tanks. The size and mass of individuals who had molted were remeasured approximately 3 days after the event, and all individuals were remeasured after the exposure period.
2.3 Predator cue collection and preparation
One week prior to any feeding assay, 7 adult blue crabs (10-14 cm CW) were fed every other day ~10-15 g crushed mud crabs. This diet was chosen to maximize prey behavioral response as shown in other studies that indicate metabolites released by blue crabs and injured prey affect mud crab risk responses (Hill and Weissburg, 2014; Poulin et al., 2018). Forty-eight hours before each feeding assay, blue crabs were placed in a shared pool of 53 L of freshly prepared artificial seawater (ASW) that was aerated at control conditions (temperature: ~29°C, pH: ~7.8), and crabs were fed twice in this pool (i.e. every 24 hours). This minimized any variation in cue based on individual differences of the predators (hunger level, amount of cue produced, etc.). This method also produced cues of both the predator (i.e. metabolic waste products) and crushed conspecifics to maximize behavioral response. Mud crab carcasses were then removed and water was collected from this pool to be used as predator cue.
2.3.1 Cue preparation – stressed predator cue
Exposing the predator risk cue to acidified conditions (termed here as “stressed predator cue”) tests whether the cue retains its efficacy in future expected environments. Predator cue and ASW treatments were prepared in 34 L tanks at ambient temperature (28°C) and either ambient or low pH (i.e. acidified). Ambient treatment water was maintained at pH of ~8.0 using air bubblers. The pH of acidified treatment water was reduced by slowly bubbling in CO2 for 1 hr until pH reached ~7.2. To test stressed predator cue in acidified conditions, acidified treatment water pH was maintained at 7.2 immediately prior to the feeding assay. Stressed predator cue was also tested in ambient conditions by turning off CO2 of treatment water and oxygenating with air bubblers for ~30 minutes until pH reached ambient levels (~7.9).
2.4 Feeding assays – stressed mud crabs
To determine the effects of future conditions on mud crab prey behavior, feeding assays were conducted in August 2019 to observe if stressed mud crabs still suppress foraging in the presence of a predator (blue crab) cue (Figure 1). Behavior of stressed animals was examined in small (30 x 20 x 20 cm) test chambers housed in 34 L aquarium tanks with a water bath at ambient conditions (temperature: ~29°C, pH: ~7.9), with one chamber containing 2 L freshly made ASW and another chamber containing 2 L predator cue. The level of the water bath was lower than the test chambers, ensuring the two water types remained independent of each other. Ambient conditions were chosen to isolate any effects of climate change to the cue receiver only and avoid confounding changes to the cue itself. In each chamber, 4 oyster shells were clustered to a randomly selected side to serve as a refuge. After allowing the chamber water to equalize in temperature with the water bath, 3 mud crabs were placed in each chamber and allowed to acclimate for 2 hours. Mud crabs were chosen to ensure no more than 2 of each sex was placed in each group, and missing limbs and size ranges were kept to a minimum.
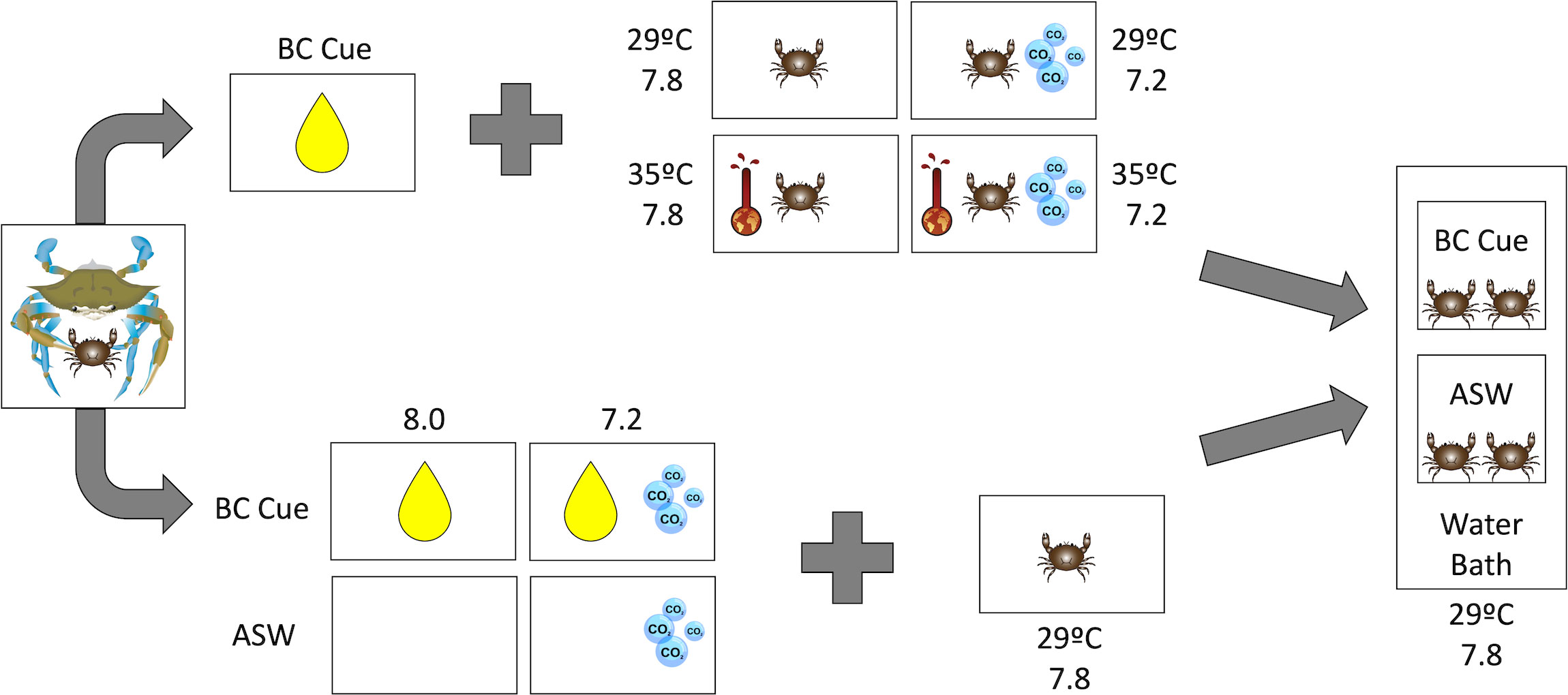
Figure 1 A schematic of experimental design for the stressed mud crab (top portion) and stressed cue (bottom portion) experiments. Adult blue crabs were fed mud crabs and the tank water was collected to be used as predator cue (“BC Cue”). In the stressed mud crab experiments, mud crabs were exposed to increased temperature (35°C) and/or decreased pH (7.2). In the stressed cue experiments, predator cue and artificial seawater (“ASW”) were exposed to decreased pH (7.2). Feeding assays for both experiments were conducted with small groups of mud crabs in test chambers with BC cue or ASW, housed in a water bath at ambient conditions (temperature: 29°C, pH: 7.8). Mud crab image provided by J. Pruett, all other images provided by the Integration & Application Network, University of Maryland Center for Environmental Science (https://ian.umces.edu/symbols/).
After the acclimation period, ~3 g raw shrimp (2.9-3.2 g) cut into 4-6 pieces was added to each chamber on the opposite side of the oyster cluster. This created a choice between refuge use and food consumption for the mud crabs. Chambers with shrimp but no mud crabs were included to control for ambient mass loss/gain, with two replicates for each cue treatment. Mud crabs were allowed to feed undisturbed in the dark for 6 hours, at which time the remaining shrimp was collected from each chamber and weighed to determine difference in mass and calculate proportion consumed. Four replicates of each treatment were performed simultaneously in one run of the feeding assay.
To test if climate change effects can be reversed, previously stressed mud crabs were returned to ambient water conditions (temperature: ~29°C, pH: ~7.8) for 7 days before the feeding assay was repeated, compared to mud crabs that were never stressed. Due to several deaths across treatments during the 7-day period, a subsample of individuals was used for the second feeding assay. For each chamber, two mud crabs were chosen, of opposite sexes when possible, with similar sizes and minimal injuries. The deaths of some of the animals in during the 7 day reversal period necessitated using 2 rather than 3 animals as before. To maintain ratio of food mass to crab density, ~2 g raw shrimp (1.9-2.2 g) cut into 2-4 pieces were added to each chamber after the acclimation period, but the feeding assay was otherwise conducted as previously described.
2.4.1 Feeding assays – stressed predator cue
Feeding assays with acidified predator cue were conducted in September 2021 – January 2022 with methods as above (Figure 1), but with two different test conditions. Mud crabs that were pre-acclimated to acidified conditions 3 days prior were tested in acidified water with acidified predator cue. This ensured that any changes to the cue were maintained in acidified conditions, despite the small risk of stressed mud crabs driving any changes in behavioral response. In addition, as previously described (Section 2.3.1), mud crabs from ambient conditions were tested with historically acidified predator cue in ambient water to minimize stress on the animals that could influence their behavioral response. Each test condition manages the tradeoff in different ways, and together they can provide insight into effects of stressing the animals vs. the cue.
Test chambers were filled with 1.5 L treatment water and 4 oyster shells and placed in a water bath held at ambient conditions (temperature: ~28°C, pH: ~7.9). Two mud crabs were placed in each chamber and allowed to acclimate for 2 hrs, then pre-weighed food (~2 g) was added to each chamber. Feeding assays were conducted as previously described in section 2.4, with 8 replicates of each treatment performed in each run. Each feeding assay type (stressed predator cue and historically stressed predator cue) was repeated with at least two weeks in between each run, for a total of 2 runs per feeding assay type.
2.5 Statistical analysis
All analyses were conducted in R version 4.1.1 (R Core Team, 2021) using the car 3.0 (Fox and Weisberg, 2019), lme4 1.1-27 (Bates et al., 2015), and emmeans 1.7 packages (Lenth, 2022). Data from the stressed and historically stressed mud crab feeding assays were each analyzed using three-way ANOVAs with temperature, pH, and cue treatment as fully crossed fixed effects. Model assumptions of normality and homogeneity of variances were confirmed using Shapiro-Wilk test and Levene’s test, respectively. Planned contrast t-tests between ASW and predator cue were conducted within each stress treatment for the stressed mud crab feeding assay.
Data from the stressed cue feeding assays were analyzed using linear mixed models with date as a random effect and fully crossed fixed effects of stress treatment and cue treatment. Including tank as a random effect did not significantly improve the fit of the models based on log-likelihood tests and was therefore excluded from analysis. Shrimp mass loss/gain for no-crab controls in all experiments was small (<3%) but included in the analysis by correcting proportion consumed for each replicate by subtracting the treatment controls’ average proportion in lost mass. Replicates with crab mortality or crabs displaying an unusually weakened state were excluded from the analysis. Observations with studentized residuals greater than 3 and Cook’s distance greater than 4 times the mean were considered outliers and therefore removed from analysis. Model assumptions of normality and homogeneity of variances were confirmed visually using diagnostic plots. Planned contrast t-tests between ASW and predator cue were conducted within each historical stress treatment for the feeding assay tested in ambient conditions.
3 Results
3.1 Stressed mud crabs
The effects of stress on mud crab responses showed a significant interaction between temperature and cue treatment (F1,23 = 5.364, p = 0.0298; Table 2), so that the effects of cue depended on temperature (Figure 2A). Blue crab predator cue reduced consumption at ambient temperature, but this effect was not present at high temperature, which depressed feeding rates regardless of cue presence. Predator cue remained efficacious for crabs exposed to ambient, but not warmed conditions (Table 3). There was no effect of pH treatment on consumption, and model results did not change with removal of the three-way interaction of temperature, pH, and cue (Table 2).
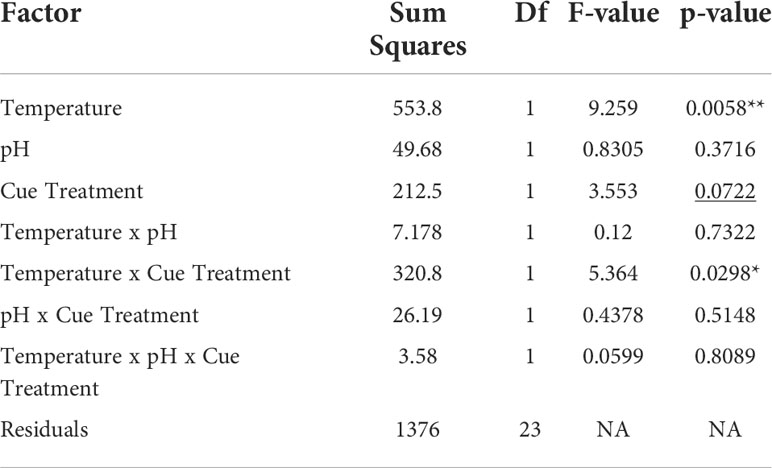
Table 2 Analysis of variance of the effects of cue treatment and temperature and pH stress treatments on mud crab consumption rates (underline denotes significant p < 0.1, *denotes significant p < 0.05, **denotes significant p < 0.01).
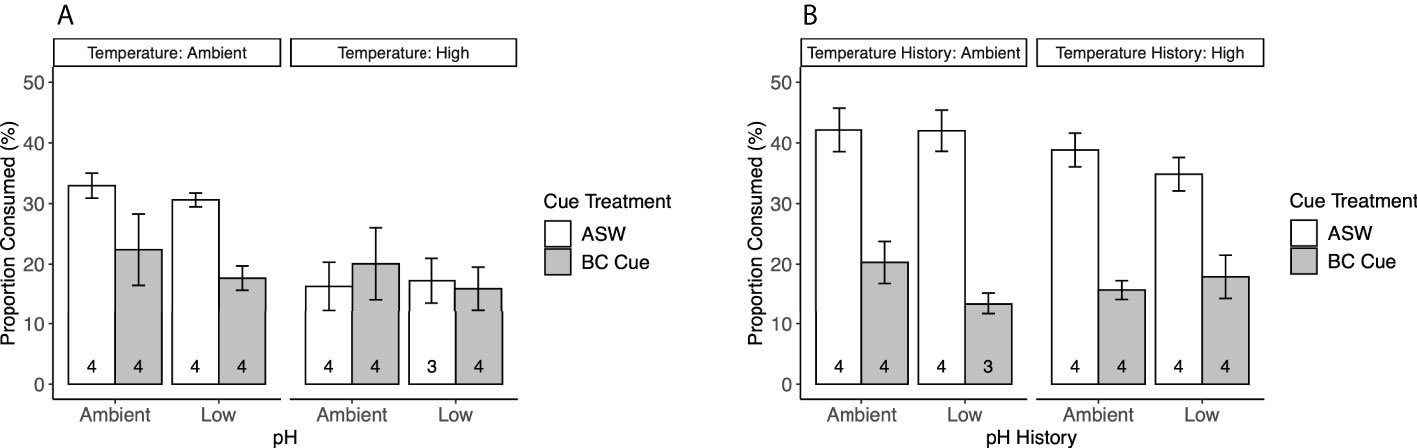
Figure 2 Mud crab consumption rates (mean ± SE) in response to blue crab predator cue treatments, (A) after 5 weeks of exposure to stress treatments of warmed and/or acidified conditions, and (B) after 1 week of return to ambient conditions. Numbers at the bottom of each bar denote sample size, and cue treatments denote blue crab (BC) predator cue vs. artificial seawater (ASW).

Table 3 Planned contrast t-tests between artificial seawater (ASW) and blue crab (BC) cue for the effects of temperature and pH stress treatments on mud crab consumption rates (underline denotes significant p < 0.1, *denotes significant p < 0.05).
Repeating the feeding experiment on mud crabs that had been returned to ambient conditions for 1 week showed the effects of stress can be reversed. Stress history of temperature and pH exposure did not affect consumption rates, while cue treatment significantly affected consumption (F1,23 = 109.7, p < 0.0001; Table 4; Figure 2B); animals exposed to blue crab predator cues reduced food consumption regardless of whether they had been previously held in ambient, or acidified and/or warmed conditions, and responded similarly to animals that had not been previously stressed.
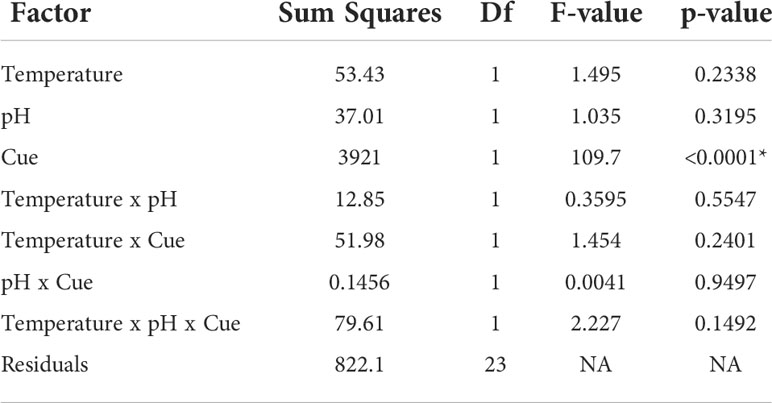
Table 4 Analysis of variance of the effects of cue treatment and historical stress treatments of temperature and pH on mud crab consumption rates (*denotes significant p < 0.05).
3.2 Stressed predator cue
Using low pH to “stress” the predator cue had no impact on whether mud crabs responded to blue crab predator cues. Mud crabs showed reduced feeding in the presence of predator cues when “stressed” cues were presented in stressed conditions (F1,39.134 = 51.3975, p < 0.0001), and there was no significant interaction between cue treatment and cue treatment pH (Table 5, Figure 3A). The effects were similar when the stressed cues were presented in ambient conditions; cue treatment and not pH had a significant effect, but there was a significant cue*pH interaction when tested in ambient conditions (F1, 52.045 = 5.6068, p = 0.0216; Table 6, Figure 3B). Despite the interaction, planned contrast t-tests showed that the predator cue continued to suppress feeding relative to the control regardless of whether it was prepared in stressed vs. ambient conditions (Table 7).
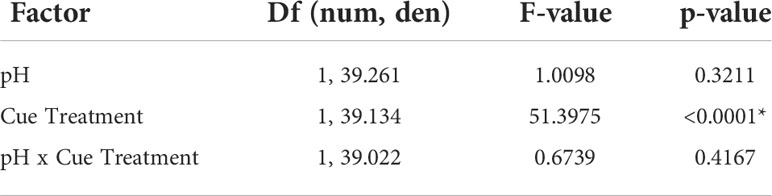
Table 5 Linear mixed model analysis of the effects of acidified predator cue on mud crab consumption rate in acidified conditions, with degrees of freedom calculated using Satterthwaite’s method (*denotes significant p < 0.05).
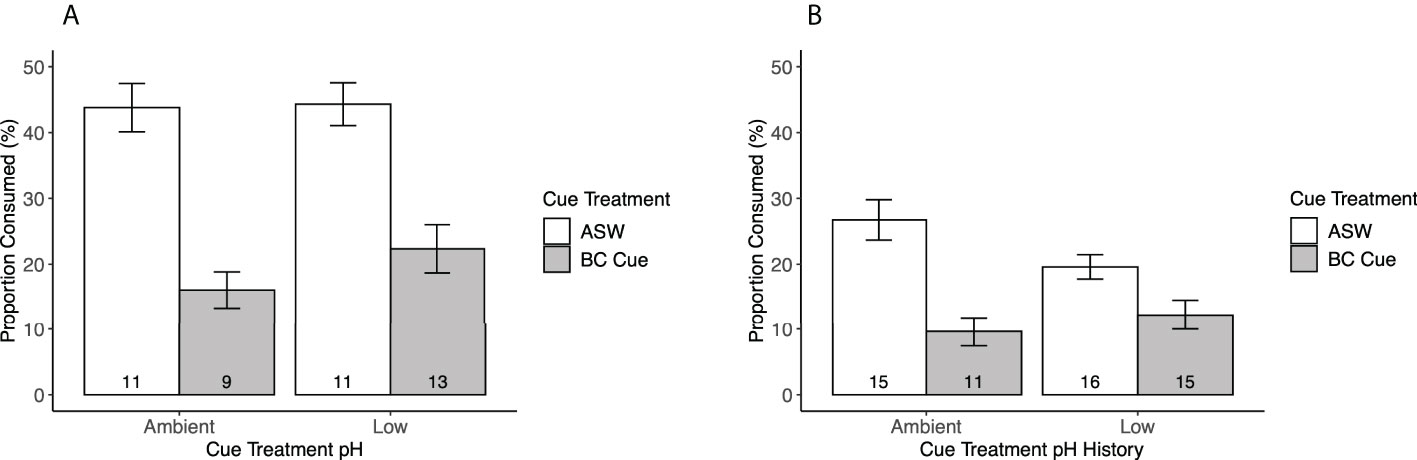
Figure 3 Mud crab consumption rates (mean ± SE) in response to blue crab predator cue treatments. Numbers at the bottom of each bar denote sample size. Cue treatments denote blue crab (BC) predator cue vs. artificial seawater (ASW), and cue treatment pH indicate the conditions in which cue treatments were prepared. (A) Cue treatments were tested in same pH conditions that they were prepared. (B) All cue treatments were returned to ambient pH conditions before the experiment.
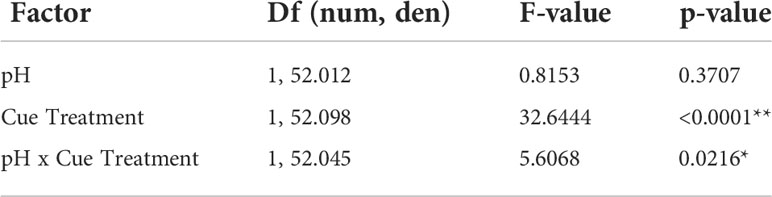
Table 6 Linear mixed model analysis of the effects of acidified predator cue on mud crab consumption rate in ambient conditions, with degrees of freedom calculated using Satterthwaite’s method (*denotes significant p < 0.05, **denotes significant p < 0.01).
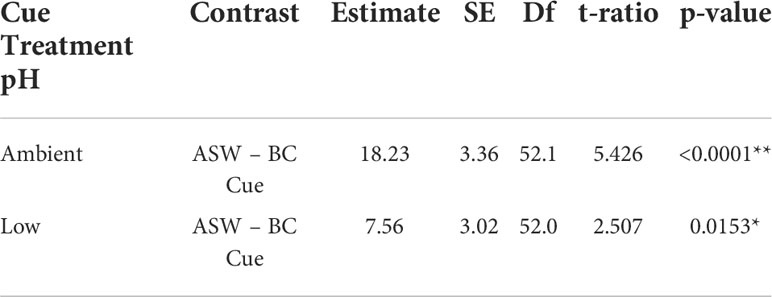
Table 7 Planned contrast t-tests between artificial seawater (ASW) and blue crab (BC) cue for ambient or historically acidified predator cue tested in ambient conditions (*denotes significant p < 0.05, **denotes significant p < 0.01).
4 Discussion
Global warming and ocean acidification can influence predator effects on lower trophic levels through physical and sensory stressors. Our results suggest that warming inflicts physical stress on predator-prey interactions in oyster reefs, while acidification does not affect the sensory pathway in this system. We found that warming significantly reduced feeding behavior regardless of predator cue presence, but these effects are reversed in ambient conditions (Figure 2). Acidification had no effect on the mud crabs’ ability to detect and respond to predator cue by reducing their feeding as shown by the consistent effect of predator cue across the different experiments. These results are similar to a study on blue mussels (Mytilus edulis × trossulus), where warming increased the startle response time (i.e. time to reopen their valves) after a tactile predator cue, but acidification had no effects on mussel behavior (Clements et al., 2020). Additionally, acidification did not affect predator cue effectiveness in suppressing feeding when tested in either acidified or ambient conditions (Figure 3), which also indicates that the crabs were not affected by short-term exposure to acidification that might influence these results. Thus, warming may be the more critical driver than acidification for coastal organisms, with the potential to strengthen the relative importance of non-consumptive effects in estuarine ecosystems.
It is widely known that elevated temperatures predicted for global warming increase metabolic stress in many organisms (Pörtner et al., 2017). As temperature approaches upper thermal limits for an organism, the capacity to deliver enough oxygen to meet tissue demands decreases, causing an overall decline in metabolic performance (Pörtner et al., 2017). Mud crab respiration rates are not affected by warming relevant to this habitat (Draper, unpublished data), although it has been suggested that mud crabs suppress aerobic metabolism in temperatures above 30°C (Dame and Vernberg, 1978). Metabolic suppression may lead to reductions in motor function and overall activity that lowers encounter rate with food, which is suggested from this study (Figure 2A). However, if the temperature stress is temporary, activity levels and foraging rates of mud crabs can quickly recover (Figure 2B). Given their intertidal environment that experiences large fluctuations in temperature daily, this adaptation is useful on the short-term. However, the ability to recover from these extremes may decrease as the mean and extreme temperatures continue to rise and organisms are pushed closer to their thermal limits.
The lack of an effect of acidification on the ability of mud crabs to respond to predation risk (Figure 2) contrasts with some studies on chemosensory behavior in crustaceans. For example, acidification decreased antennular flicking rates in hermit crabs, which consequently increased the time for crabs to detect and find prey (de la Haye et al., 2012; Kim et al., 2016). However, our results are similar to Richardson et al. (2021), which found that shore crabs in ambient and acidified conditions took the same amount of time to respond to predator cue. One possible explanation is that mud crabs live in the intertidal zone where diel tide cycles and freshwater input create large fluctuations in pH of 0.4 – 0.6 units daily (Sapelo Island NERR Lower Duplin monitoring station). Although the effects of acidification on crab chemoreceptors are unknown (but see Bednaršek et al., 2020 for acidification effects on mechanoreceptors), mud crab olfactory perception likely is pre-adapted to naturally extreme environmental conditions. However, a caveat from our study is the predator cue was well-mixed into the experimental arena occupied by the mud crabs during the experiments. Natural chemical signals often (but not always) are contained in complex turbulent plumes in flowing water (Weissburg, 2000). Preliminary results suggest that mud crabs in acidified conditions have trouble tracking food plumes in flowing water (Draper, unpublished data), so detection and processing of predator cue may have been easier in our experimental set up and sufficient even if lowered pH produced some olfactory disruption. Future studies should test the tracking ability of stressed mud crabs to prey cues and avoidance of predator cues to better address this issue.
Cue efficacy was also not affected by acidification in this study (Figure 3). As acidification increases the concentration of protons (i.e. hydrogen ions), changes to bioactive signaling molecules induced by acidification is dependent on the presence of chemical functional groups susceptible to protonation, such as amine or carboxyl groups (Porteus et al., 2021). The sensitivity of a functional group to protonation is expressed as the pKa value, which is the pH value at which there is a 1:1 ratio of protonated and deprotonated molecules of the target functional group in solution. For example, Roggatz et al. (2016) identified three peptide signaling molecules used in shore crab ventilation behavior for their eggs that are protonated under ocean acidification. These bioactive molecules contain amine groups with pKa values close to 8, so reduced pH (~7.7) caused these groups to become protonated. The altered cue structure reduced crab receptor sensitivity to the cue, so acidification reduced the frequency of ventilation behavior (Roggatz et al., 2016).
The bioactive molecules in blue crab metabolic waste that suppress mud crab foraging have been identified as homarine and trigonelline (Poulin et al., 2018). These small molecules contain carboxyl groups with predicted pKa values much lower than 7.2 (homarine: 2.63, trigonelline: 3.38; https://chemicalize.com/), so these molecules were not susceptible to protonation caused by acidification in our study. Another possible consequence of acidification is that chemical cues could be susceptible to decomposition (i.e. chemical reactions facilitated under acidic conditions). We were unable to find evidence that homarine and trigonelline are labile in mildly acidic conditions, but our results indicated these cues remained effective and therefore likely remained intact.
Homarine and trigonelline may not be the only compounds responsible for foraging suppression in mud crab prey, as we combined both blue crab metabolites and crushed conspecific cues for the predation risk cue. Although crushed conspecific cues often are indicators of predator presence in aquatic systems (reviewed by Ferrari et al., 2010), cues from crushed conspecifics alone produce less intense antipredator responses from mud crabs than blue crab predator metabolites (Hill and Weissburg, 2014). It is unknown what the bioactive molecules are in crushed mud crab cue, so while it is possible that acidification altered the crushed conspecific cue, our study demonstrated that any chemical changes that occurred were minimal and did not reduce the behavioral response of mud crab prey. Small changes to the cue might become important at lower concentrations (e.g. in the field and in flow), but it is unknown what these specific cue concentrations of predation risk (e.g. blue crab metabolites, crushed conspecific) are like in the field. However, we predict the concentrations used in this study were within range for mud crabs in naturally occurring oyster reefs.
Warming effects on the chemical cue, as well as effects of warming and acidification on chemical cue production (i.e. stressed blue crabs), were not tested due to logistical constraints. Warming effects on the chemical cue was not tested due to insufficient number of mud crabs collected during the timeline of experiments, but this remains an important question. Further work should explore how warming might alter the chemical structure of predation risk cues via other mechanisms such as natural or microbially-mediated degradation. Limited studies suggest cues indicative of predation risk have half-lives that commonly exceed 10 hours (Van Buskirk et al., 2014), which is far longer than residence times in our system where currents effectively dissipate cues (Weissburg and Beauvais, 2015). Thus, in our system and similar environments, degradation would have to increase considerably in order to have effects.
Testing effects of acidification and warming on cue production was similarly impossible given the large size of experimental tanks required to house multiple cue-producing adult blue crabs. Future studies should examine how warming and acidification affect chemical cue production, for potential changes in food consumption (i.e. feeding rates) combined with metabolic changes may alter the quantity or chemical composition of cues produced. Notably, however, the chemicals produced by blue crabs and detected by mud crab prey are primary metabolites (Poulin et al., 2018), suggesting that large disruptions may be necessary to alter cue characteristics. Testing all components of the sensory transduction pathway should allow for more realistic predictions regarding predator-prey interactions in a changing environment.
We propose a conceptual model for how differential effects of warming and acidification may change the relative strengths of predator CEs and NCEs in future expected environments (Figure 4). As a physical stressor, warming can decrease foraging activity to promote NCEs (e.g. sensory behavior) of predator control (Figure 4A), which is suggested for oyster reefs based on the results from this study. If warming weakened only NCEs of blue crab predators on mud crab prey (i.e. mud crabs did not respond to blue crab predator cues), we would expect to see similar food consumption at ambient conditions when cue is absent. We did not see this effect, and instead found reduced food consumption regardless of cue (Figure 2A). This suggests that warming reduced overall mud crab movement that may affect success rate of predator attacks, therefore NCEs of predators on mud crab prey would become more important. In the absence of other effects, this will increase the indirect survival benefit to the basal resource. While this study suggests that acidification does not act as a sensory stressor in oyster reefs, acidification can theoretically decrease predator and prey perception in other systems, making CEs more important in the system of interest as foraging becomes dependent on movement and encounter rate (Figure 4B). This also increases basal prey survival although perhaps at a smaller level given NCEs often have greater effects than CEs (Preisser et al., 2005). Warming and acidification have the potential to interact and simultaneously influence predator control, so the ecological outcome will depend on initial relative importance of CEs and NCEs in the system of interest (Figure 4C) and the magnitude of the response. For example, changes to CEs and NCEs in oyster reefs have been studied with other environmental stressors such as turbulence and flow (Pruett and Weissburg, 2021). In low sensory stress environments (i.e. turbulence) with low physical stress (i.e. flow speed), blue crab predators control mud crab consumption of oysters primarily through NCEs. As sensory stress increases, blue crabs indirectly promote oyster survival through CEs on mud crabs. In high physical stress environments, mud crab foraging is constrained thereby increasing oyster survival. Therefore, warming and acidification may weaken the strengths of predator CEs and NCEs that indirectly benefit basal resources, with the mechanism depending on the stressor and pathway. Regardless, the different consequences of changes in CEs and NCEs argue that it is necessary to understand how warming and acidification affect these different processes.

Figure 4 A conceptual model of warming and acidification effects on CEs and NCEs of predator control. (A) If warming more strongly affects predator and prey, then CEs will become less important and NCEs will dominate the system. (B) If acidification more strongly affects predator-prey interactions, then NCEs will decrease and CEs will be more important. (C) If both stressors affect predator and prey, then the initial relative strengths of CEs and NCEs in the system will determine the overall change in predator control. Mud crab image provided by J. Pruett, all other images provided by the Integration & Application Network, University of Maryland Center for Environmental Science (https://ian.umces.edu/symbols/).
In summary, this study suggests that warming may increase physical stress, while acidification does not affect sensory transmission and detection. Weakened consumptive effects may strengthen the relative importance of non-consumptive effects in this system, especially behavioral cascades to basal prey (Pruett and Weissburg, 2018; Pruett and Weissburg, 2021). If warming becomes too extreme, both predator and prey may be physically stressed in ways that could reduce distribution and survival of populations and consequently affect other members of the community. Thus, future studies should focus on warming effects in combination with other significant stressors (e.g. pollution, sea level rise) to better understand how climate change will affect community dynamics in this threatened ecosystem.
Data availability statement
The raw data supporting the conclusions of this article will be made available by the authors, without undue reservation.
Author contributions
AD and MW conceived and designed the experiments. AD collected and analysed the data and wrote the first manuscript draft with MW. Both authors contributed to the final manuscript.
Funding
This research was funded by Georgia Sea Grant Graduate Research Traineeship awarded to AMD, and NSF grant Bio-OCE #1234449 awarded to MJW.
Acknowledgments
We thank Philippe Lamarche, Tanner Lee, Tyler Vernon, and Karolina Zabinski for field and laboratory assistance. We also appreciate the help and support of the staff at the Skidaway Institute of Oceanography and UGA Marine Extension and Georgia Sea Grant. We thank Jessica Pruett for the drawing of Panopeus herbstii.
Conflict of interest
The authors declare that the research was conducted in the absence of any commercial or financial relationships that could be construed as a potential conflict of interest.
Publisher’s note
All claims expressed in this article are solely those of the authors and do not necessarily represent those of their affiliated organizations, or those of the publisher, the editors and the reviewers. Any product that may be evaluated in this article, or claim that may be made by its manufacturer, is not guaranteed or endorsed by the publisher.
References
Bates D., Mächler M., Bolker B., Walker S. (2015). Fitting linear mixed-effects models using lme4. J. Stat. Software 67, 1–48. doi: 10.18637/jss.v067.i01
Beck M. W., Brumbaugh R. D., Airoldi L., Carranza A., Coen L. D., Crawford C., et al. (2011). Oyster reefs at risk and recommendations for conservation, restoration, and management. BioScience 61, 107–116. doi: 10.1525/bio.2011.61.2.5
Bednaršek N., Feely R. A., Beck M. W., Alin S. R., Siedlecki S. A., Calosi P., et al. (2020). Exoskeleton dissolution with mechanoreceptor damage in larval dungeness crab related to severity of present-day ocean acidification vertical gradients. Sci. Total Environ. 716, 136610. doi: 10.1016/j.scitotenv.2020.136610
Bisker R., Castagna M. (1987). Predation on single spat oysters crassostrea virginica by blue crabs callinectes sapidus and mud crabs, panopeus herbstii. J. Shellfish Res. 6, 37–40. Available at: https://scholarworks.wm.edu/vimsarticles/1290
Cai W.-J., Wang Y. (1998). The chemistry, fluxes, and sources of carbon dioxide in the estuarine waters of the satilla and altamaha rivers, Georgia. Limnol Oceanog 43, 657–668. doi: 10.4319/lo.1998.43.4.0657
Clements J., Ramesh K., Nysveen J., Dupont S., Jutfelt F. (2020). Animal size and seawater temperature, but not pH, influence a repeatable startle response behaviour in a wide-ranging marine mollusc. EcoEvoRxiv 173, 191–205. doi: 10.32942/osf.io/5t478
Dame R. F., Vernberg F. J. (1978). The influence of constant and cyclic acclimation temperatures on the metabolic rates of panopeus herbstii and uca pugilator. Biol. Bull. 154, 188–197. doi: 10.2307/1541121
de la Haye K. L., Spicer J. I., Widdicombe S., Briffa M. (2012). Reduced pH sea water disrupts chemo-responsive behaviour in an intertidal crustacean. J. Exp. Mar. Biol. Ecol. 412, 134–140. doi: 10.1016/j.jembe.2011.11.013
Dickson A. G., Millero F. J. (1987). A comparison of the equilibrium constants for the dissociation of carbonic acid in seawater media. Deep Sea Res. Part A. Oceanog Res. Papers 34, 1733–1743. doi: 10.1016/0198-0149(87)90021-5
Dissanayake A. (2014). ““Ocean acidification and warming effects on Crustacea: Possible future scenarios,”,” in The Mediterranean Sea. Eds. Goffredo S., Dubinsky Z. (Dordrecht: Springer Netherlands) 363–372. doi: 10.1007/978-94-007-6704-1_20
Dodd L. F., Grabowski J. H., Piehler M. F., Westfield I., Ries J. B. (2015). Ocean acidification impairs crab foraging behaviour. Proc. Biol. Sci. 282, 20150333. doi: 10.1098/rspb.2015.0333
Doney S. C., Fabry V. J., Feely R. A., Kleypas J. A. (2009). Ocean acidification: The other CO2 problem. Annu. Rev. Mar. Sci. 1, 169–192. doi: 10.1146/annurev.marine.010908.163834
Draper A. M., Weissburg M. J. (2019). Impacts of global warming and elevated CO2 on sensory behavior in predator-prey interactions: A review and synthesis. Front. Ecol. Evol. 7. doi: 10.3389/fevo.2019.00072
Ferrari M. C. O., Wisenden B. D., Chivers D. P. (2010). Chemical ecology of predator–prey interactions in aquatic ecosystems: a review and prospectus. Can. J. Zool. 88, 698–724. doi: 10.1139/Z10-029
Fox J., Weisberg S. (2019). An r companion to applied regression (Third. Thousand Oaks CA: Sage). Available at: https://socialsciences.mcmaster.ca/jfox/Books/Companion/.
Glandon H. L., Paynter K. T., Rowe C. L., Miller T. J. (2019). Resilience of oxygen consumption rates in the juvenile blue crab callinectes sapidus to future predicted increases in environmental temperature and pCo2 in the mesohaline Chesapeake bay. J. Shellfish Res. 38, 711. doi: 10.2983/035.038.0323
Glaspie C. N., Longmire K., Seitz R. D. (2017). Acidification alters predator-prey interactions of blue crab callinectes sapidus and soft-shell clam mya arenaria. J. Exp. Mar. Biol. Ecol. 489, 58–65. doi: 10.1016/j.jembe.2016.11.010
Goldenberg S. U., Nagelkerken I., Marangon E., Bonnet A., Ferreira C. M., Connell S. D. (2018). Ecological complexity buffers the impacts of future climate on marine consumers. Nat. Climate Change 8, 229–233. doi: 10.1038/s41558-018-0086-0
Grabowski J. H., Brumbaugh R. D., Conrad R. F., Keeler A. G., Opaluch J. J., Peterson C. H., et al. (2012). Economic valuation of ecosystem services provided by oyster reefs. BioScience 62, 900–909. doi: 10.1525/bio.2012.62.10.10
Grabowski J. H., Hughes A. R., Kimbro D. L. (2008). Habitat complexity influences cascading effects of multiple predators. Ecology 89, 3413–3422. doi: 10.1890/07-1057.1
Helmuth B., Harley C. D. G., Halpin P. M., O’Donnell M., Hofmann G. E., Blanchette C. A. (2002). Climate change and latitudinal patterns of intertidal thermal stress. Science 298, 1015–1017. doi: 10.1126/science.1076814
Helmuth B. S. T., Hofmann G. E. (2001). Microhabitats, thermal heterogeneity, and patterns of physiological stress in the rocky intertidal zone. Biol. Bull. 201, 374–384. doi: 10.2307/1543615
Hill J. M., Weissburg M. J. (2013). Predator biomass determines the magnitude of non-consumptive effects (NCEs) in both laboratory and field environments. Oecologia 172, 79–91. doi: 10.1007/s00442-012-2488-4
Hill J. M., Weissburg M. J. (2014). Crabs interpret the threat of predator body size and biomass via cue concentration and diet. Anim. Behav. 92, 117–123. doi: 10.1016/j.anbehav.2014.03.025
Hoegh-Guldberg O., Bruno J. F. (2010). The impact of climate change on the world’s marine ecosystems. Science 328, 1523–1528. doi: 10.1126/science.1189930
Hofmann G. E., Smith J. E., Johnson K. S., Send U., Levin L. A., Micheli F., et al. (2011). High-frequency dynamics of ocean pH: A multi-ecosystem comparison. PloS One 6, e28983. doi: 10.1371/journal.pone.0028983
IPCC (2014). Climate change 2014: Synthesis report. contribution of working groups I, II and III to the fifth assessment report of the intergovernmental panel on climate change. Eds. Core Writing Team, Pachauri R. K., Mayer L. (Geneva, Switzerland: Intergovernmental Panel on Climate Change).
Jackson J. B. C., Kirby M. X., Berger W. H., Bjorndal K. A., Botsford L. W., Bourque B. J., et al. (2001). Historical overfishing and the recent collapse of coastal ecosystems. Science 293, 629–637. doi: 10.1126/science.1059199
Jost J., Helmuth B. (2007). Morphological and ecological determinants of body temperature of Geukensia demissa, the Atlantic ribbed mussel, and their effects on mussel mortality. Biol. Bull. 213, 141–151. doi: 10.2307/25066630
Kidawa A., Potocka M., Janecki T. (2010). The effects of temperature on the behaviour of the Antarctic sea star odontaster validus. Polish Polar Res. 31 (3), 273–284. doi: 10.2478/v10183-010-0003-3
Kim T. W., Taylor J., Lovera C., Barry J. P. (2016). CO 2 -driven decrease in pH disrupts olfactory behaviour and increases individual variation in deep-sea hermit crabs. ICES J. Mar. Science: J. du Conseil 73, 613–619. doi: 10.1093/icesjms/fsv019
Kirby M. X. (2004). Fishing down the coast: Historical expansion and collapse of oyster fisheries along continental margins. Proc. Natl. Acad. Sci. U.S.A. 101, 13096–13099. doi: 10.1073/pnas.0405150101
Kwiatkowski L., Or J. C. (2018). Diverging seasonal extremes for ocean acidification during the twenty-first century. Nat. Clim Change 8, 141–145. doi: 10.1038/s41558-017-0054-0
Lenth R. V. (2022) Emmeans: Estimated marginal means, aka least-squares means. Available at: https://CRAN.R-project.org/package=emmeans.
McCormick-Ray J. (2005). Historical oyster reef connections to Chesapeake bay – a framework for consideration. Estuarine Coast. Shelf Sci. 64, 119–134. doi: 10.1016/j.ecss.2005.02.011
Mehrbach C., Culberson C. H., Hawley J. E., Pytkowicx R. M. (1973). Measurement of the apparent dissociation constants of carbonic acid in seawater at atmospheric pressure. Limnol. Oceanogr. 18, 897–907. doi: 10.4319/lo.1973.18.6.0897
Meyer D. L. (1994). Habitat partitioning between the xanthid crabs panopeus herbstii and eurypanopeus depressus on intertidal oyster reefs (Crassostrea virginica) in southeastern north Carolina. Estuaries 17, 674. doi: 10.2307/1352415
Micheli F. (1997). Effects of predator foraging behavior on patterns of prey mortality in marine soft bottoms. Ecol. Monogr. 67, 203–224. doi: 10.1890/0012-9615(1997)067[0203:EOPFBO]2.0.CO;2
Mos B., Holloway C., Kelaher B. P., Santos I. R., Dworjanyn S. A. (2021). Alkalinity of diverse water samples can be altered by mercury preservation and borosilicate vial storage. Sci. Rep. 11, 9961. doi: 10.1038/s41598-021-89110-w
Nagelkerken I., Munday P. L. (2016). Animal behaviour shapes the ecological effects of ocean acidification and warming: moving from individual to community-level responses. Global Change Biol. 22, 974–989. doi: 10.1111/gcb.13167
Pacella S. R., Brown C. A., Waldbusser G. G., Labiosa R. G., Hales B. (2018). Seagrass habitat metabolism increases short-term extremes and long-term offset of CO 2 under future ocean acidification. Proc. Natl. Acad. Sci. U.S.A. 115, 3870–3875. doi: 10.1073/pnas.1703445115
Pierrot D., Lewis E., Wallace D “MS Excel Program developed for CO2 system calculations” in ORNL/CDIAC-105a, Carbon Dioxide Information Analysis Center, Oak Ridge National Laboratory, US Department of Energy, Oak Ridge, Tennessee (Oak Ridge:Tennesee, USA).
Porteus C. S., Roggatz C. C., Velez Z. (2021). Acidification can directly affect olfaction in marine organisms. J. Exp. Biol. 224 (8), jeb237941. doi: 10.1242/jeb.237941
Pörtner H.-O., Bock C., Mark F. C. (2017). Oxygen- and capacity-limited thermal tolerance: bridging ecology and physiology. J. Exp. Biol. 220, 2685–2696. doi: 10.1242/jeb.134585
Pörtner H. O., Farrell A. P. (2008). Physiology and climate change. Science 322, 690–692. doi: 10.1126/science.1163156
Poulin R. X., Lavoie S., Siegel K., Gaul D. A., Weissburg M. J., Kubanek J. (2018). Chemical encoding of risk perception and predator detection among estuarine invertebrates. PNAS 115, 662–667. doi: 10.1073/pnas.1713901115
Preisser E. L., Bolnick D. I., Benard M. F. (2005). Scared to death? the effects of intimidation and consumption in predator-prey interactions. Ecology 86, 501–509. doi: 10.1890/04-0719
Pruett J. L., Weissburg M. J. (2018). Hydrodynamics affect predator controls through physical and sensory stressors. Oecologia 186, 1079–1089. doi: 10.1007/s00442-018-4092-8
Pruett J. L., Weissburg M. J. (2021). Environmental stress gradients regulate the relative importance of predator density- and trait-mediated indirect effects in oyster reef communities. Ecol. Evol. 11, 796–805. doi: 10.1002/ece3.7082
R Core Team (2021). R: A language and environment for statistical computing (Vienna, Austria: R Foundation for Statistical Computing). Available at: https://www.R-project.org/.
Richardson B., Martin H., Bartels-Hardege H., Fletcher N., Hardege J. D. (2021). The role of changing pH on olfactory success of predator–prey interactions in green shore crabs, carcinus maenas. Aquat Ecol 56, 409–418. doi: 10.1007/s10452-021-09913-x
Ries J. B., Cohen A. L., McCorkle D. C. (2009). Marine calcifiers exhibit mixed responses to CO2-induced ocean acidification. Geology 37, 1131–1134. doi: 10.1130/G30210A.1
Ringwood A. H., Keppler C. J. (2002). Water quality variation and clam growth: Is pH really a non-issue in estuaries? Estuaries 25, 901–907. doi: 10.1007/BF02691338
Roggatz C. C., Lorch M., Hardege J. D., Benoit D. M. (2016). Ocean acidification affects marine chemical communication by changing structure and function of peptide signalling molecules. Global Change Biol. 22, 3914–3926. doi: 10.1111/gcb.13354
Van Buskirk J., Krügel A., Kunz J., Miss F., Stamm A. (2014). The rate of degradation of chemical cues indicating predation risk: An experiment and review. Ethology 120, 942–949. doi: 10.1111/eth.12266
Waldbusser G. G., Salisbury J. E. (2014). ““Ocean acidification in the coastal zone from an organism’s perspective: Multiple system parameters, frequency domains, and habitats,”,” in Annual review of marine science, vol 6 annual review of marine science. Eds. Carlson C. A., Giovannoni S. J. (Palo Alto: Annual Reviews), 221–247. Available at: http://www.annualreviews.org/doi/abs/10.1146/annurev-marine-121211-172238.
Weissburg M. J. (2000). The fluid dynamical context of chemosensory behavior. Biol. Bull. 198, 188–202. doi: 10.2307/1542523
Weissburg M., Beauvais J. (2015). The smell of success: the amount of prey consumed by predators determines the strength and range of cascading non-consumptive effects. PeerJ 3, e1426. doi: 10.7717/peerj.1426
Weissburg M., Smee D. L., Ferner M. C. (2014). The sensory ecology of nonconsumptive predator effects. Am. Nat. 184, 141–157. doi: 10.1086/676644
Werner E. E., Peacor S. D. (2003). A review of trait-mediated indirect interactions in ecological communities. Ecology 84, 1083–1100. doi: 10.1890/0012-9658(2003)084[1083:Arotii]2.0.Co;2
Wootton J. T., Pfister C. A., Forester J. D. (2008). Dynamic patterns and ecological impacts of declining ocean pH in a high-resolution multi-year dataset. Proc. Natl. Acad. Sci. 105, 18848–18853. doi: 10.1073/pnas.0810079105
Keywords: predator-prey, sensory ecology, animal behavior, climate change, crustacean
Citation: Draper AM and Weissburg MJ (2022) Differential effects of warming and acidification on chemosensory transmission and detection may strengthen non-consumptive effects of blue crab predators (Callinectes sapidus) on mud crab prey (Panopeus herbstii). Front. Mar. Sci. 9:944237. doi: 10.3389/fmars.2022.944237
Received: 14 May 2022; Accepted: 25 July 2022;
Published: 11 August 2022.
Edited by:
Cosima Porteus, University of Toronto Scarborough, CanadaReviewed by:
Michiya Kamio, Tokyo University of Marine Science and Technology, JapanRebecca Kulp, Stony Brook University, United States
John Carroll, Georgia Southern University, United States
Copyright © 2022 Draper and Weissburg. This is an open-access article distributed under the terms of the Creative Commons Attribution License (CC BY). The use, distribution or reproduction in other forums is permitted, provided the original author(s) and the copyright owner(s) are credited and that the original publication in this journal is cited, in accordance with accepted academic practice. No use, distribution or reproduction is permitted which does not comply with these terms.
*Correspondence: Alex M. Draper, YWRyYXBlcjNAZ2F0ZWNoLmVkdQ==