- 1Laboratory of Trophic and Isotopes Ecology, Department of Biology, Ecology and Evolution, University of Liège, Liège, Belgium
- 2Centre for Marine Ecosystems Research, School of Science, Edith Cowan University, Joondalup, WA, Australia
Seagrass, systems export significant amounts of their primary production as large detritus (i.e. macrophytodetritus). Accumulations of exported macrophytodetritus (AEM) are found in many areas in coastal environment. Dead seagrass leaves are often a dominant component of these accumulations, offering shelter and/or food to numerous organisms. AEM are particular habitats, different from donor habitats (i.e. seagrass meadow, kelp or macroalgae habitats) and with their own characteristics and dynamics. They have received less attention than donor habitats despite the fact they often connect different coastal habitats, are the place of intense remineralization processes and shelter associated detritus food web. As for seagrass meadows themselves, AEM are potentially affected by global change and by tropicalization processes. Here, we review briefly general characteristic of AEM with a focus on Mediterranean Sea and Western Australia and we provide some hypotheses concerning their tropicalization in a near future. We conclude that AEM functioning could change either through: (1) declines in biomass or loss of seagrass directly due to increased ocean temperatures or increased herbivory from tropicalized herbivores; (2) increased degradation and processing of seagrass detritus within seagrass meadows leading to reduced export; (3) replacement of large temperate seagrass species with smaller tropical seagrass species; and/or (4) loss or changes to macroalgae species in neighboring habitats that export detritus. These processes will alter the amount, composition, quality, timing and frequency of inputs of detritus into ecosystems that rely on AEM as trophic subsidies, which will alter the suitability of AEM as habitat and food for invertebrates.
Introduction
Seagrasses form highly productive ecosystems that provide a range of important ecosystem functions in coastal environments, including carbon sequestration, habitat provision, and a direct and indirect food source for a range of consumers (e.g. Orth et al., 2006). They are net autotrophic ecosystems (e.g. Champenois and Borges, 2012; Champenois and Borges, 2021), capable of exporting massive detrital primary production to other coastal and offshore habitats (Mann, 1988; Cebrian, 2002; Heck et al., 2008). While herbivory can remove high proportions of primary production, forming the green food web, the extent of this process depends on seagrass system type, latitude or local variability (Valentine and Heck, 2021; Heck et al., 2021). Nevertheless, whatever the importance of herbivory, a significant part of the primary production is not consumed and forms detritus, fueling the brown food web (Cebrian, 2002; Mateo et al., 2006). However, both the green and brown food webs linked to seagrass ecosystems are almost certainly being impacted by the loss of seagrass in many parts of the world (Waycott et al., 2009), while the relative importance of each will likely be affected by ocean warming, shifting distributions of seagrass species, and functional change in seagrass meadows (Hyndes et al., 2016).
Production of detritus in seagrass meadows is enhanced by the fact that seagrass leaves senesce, die and are released from the plant. This is a process controlled by the plant physiology, allowing nutrient reclamation by seagrass shoots (Stapel and Hemminga, 1997; Lepoint et al., 2002) and elimination of epiphytic biomass (Orth and Van Montfrans, 1984; Borowitzka et al., 2006). Leaf fall can be a continuous phenomenon, particularly in tropical and subtropical areas, or a more seasonal one, particularly in temperate areas. This detritus material (or necromass) can form seagrass litter that lays and decomposes among seagrass shoots (Walker et al., 2001), and in which fauna contribute to decomposition via mechanical fragmentation, production of feces and assimilation of seagrass carbon (Harrison, 1989). Residence time of seagrass litter in the meadow itself is highly variable (i.e. from hours to months), depending on meadow depth, microbial and mechanical degradation rates, fauna processing and local hydrodynamics (i.e. swell, current, wind gust or storm) or local seascape morphology (Hyndes et al., 2014; Ricart et al., 2015; Ricart et al., 2017; Costa et al., 2019). In many cases, a significant part of this detritus can be exported outside the meadow as large fragments (i.e. macrophytodetritus) (e.g. Boudouresque et al., 2016). It is estimated that, as a global average, between 15 and 25% of carbon fixed by seagrass is exported to adjacent system (Cebrian, 2002; Mateo et al., 2006) but this can vary between few percent and 100% depending of the seagrass system and local environmental condition (seascape, hydrodynamics for example) (reviewed in Heck et al., 2008 and Mateo et al., 2006). Long term study by Champenois and Borges (2021) in Calvi (i.e. 12 year mooring survey) estimate to 80% the total NPP produced in Posidonia oceanica bed exported to adjacent systems, matching the calculation of Pergent et al., 1997 for this system (40 – 70% of leaf production). Thus, seagrass detritus can fuel the brown food web both within the seagrass meadow and in connected ecosystems, depending on seagrass and seascape characteristics and hydrodynamics (Hyndes et al., 2014). For examples, massive release of Posidonia oceanica (L.) Delile leaves starts in the Mediterranean Sea in late summer, but export is often delayed to mid to late autumn, depending of autumnal storms (Pergent et al., 1997; Gobert et al., 2006). This means that exported material is already modified by decomposition (i.e. impoverished in N and P) and colonized by microbial decomposers (Mateo and Romero, 1997). In temperate Western Australia, detritus production in meadows of Posidonia spp. occurs throughout the year, but peaks in late summer while export occurs in autumn (Cambridge and Hocking, 1997). Compared to temperate systems, decomposition rates in the tropics are generally faster than for temperate seagrass systems. For example, in Indonesia, Vonk and Stapel (2008) measured decomposition rates (k) for leaf biomass of Thalassia hemprichii; Cymodocea rotundata or Halodule uninervis ranging from 0.023 to 0.070 d–1. In comparison, in similar litter bag experiences, measured decomposition rate of P. oceanica dead leaves ranged from 0.003 (Costa et al., 2019) to a maximum of 0.022 d-1 (Mateo and Romero, 1997). Faster decomposition rate can lead to efficient nutrient recycling inside the meadows (Vonk and Stapel, 2008) and, therefore, to less NPP export (Hyndes et al., 2014). In their data compilation, Hyndes et al. (2014) calculate that, in tropical seagrass meadow, a greater proportion of seagrass net primary production (NPP) is being processed through decomposition and herbivory than in temperate seagrass meadow (global average 85.2 vs. 34.1% of annual NPP (calculated form Table 1 in Hyndes et al., 2014) indicating as a general observation that NPP export is proportionally lower in tropical seagrass meadow than in temperate seagrass meadows.
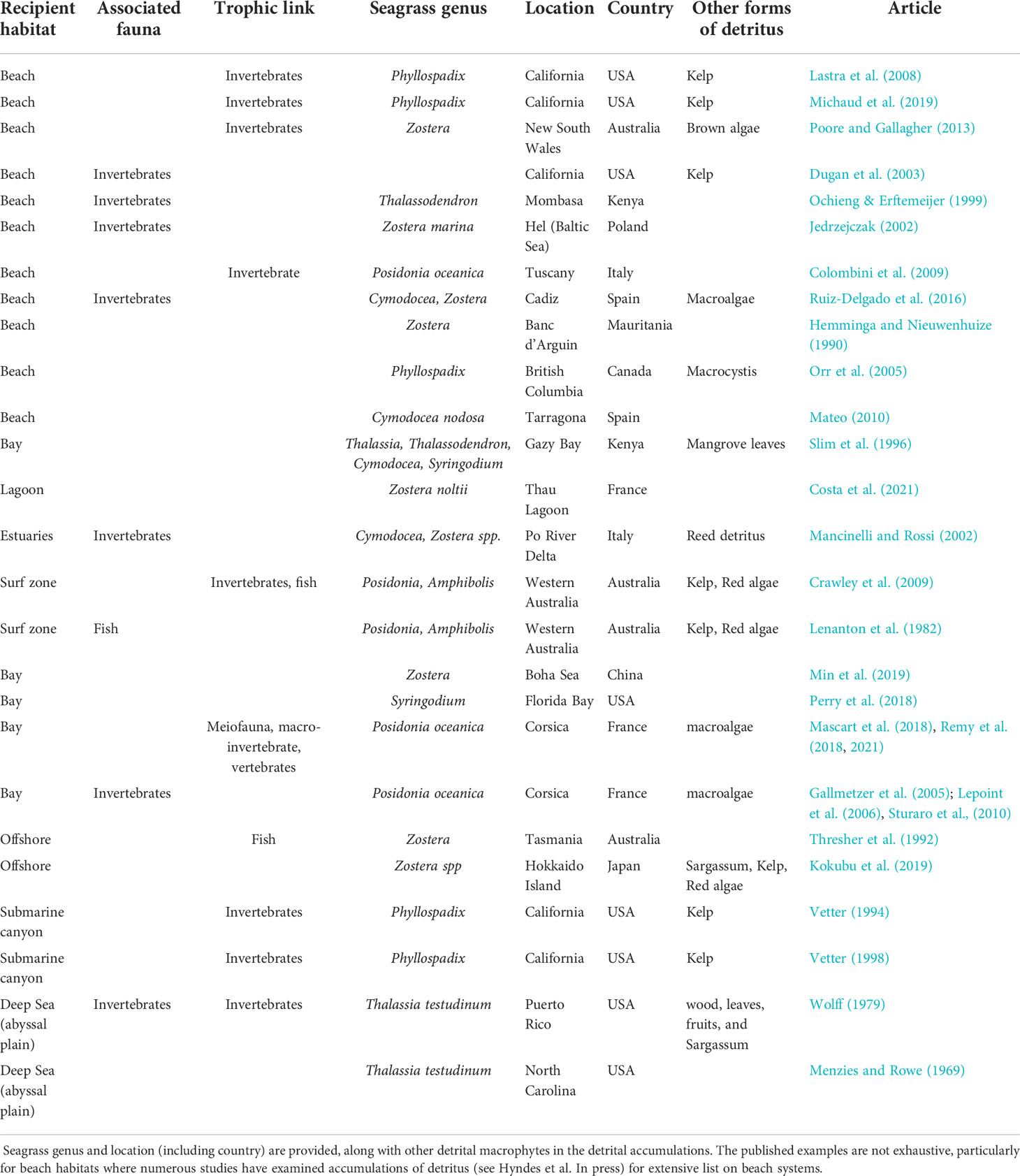
Table 1 Published examples of habitats and locations in which seagrass detritus has been linked to habitat or trophic associations with fauna in coastal and offshore systems.
Accumulations of exported macrophytodetritus (AEM) (also known as dead leaf mat, wrack, beach cast or exported litter in the literature) can be found in a wide range of ecosystems including the ocean floor of the deep sea and continental shelf, to coastal systems including coral reefs, saltmarshes, mangroves, beaches and dunes (Table 1). Seagrass detritus forms part of beach-cast wrack across the globe (Hyndes et al., in press), often constituting high proportions in regions where large seagrass meadows occur, particularly in the Mediterranean Sea and southern Australia, but also in tropical areas (Figure 1). Accumulations of dead seagrass leaves are also found in subtidal areas, close or distant to meadow sources (Figure 2). This is particularly the case in the initial phase of detritus export before mechanical fragmentation. Fossil evidence for extinct (Jagt et al., 2019) and extant (Moissette et al., 2007) seagrass species indicates that this process has been occurring for millions of years.
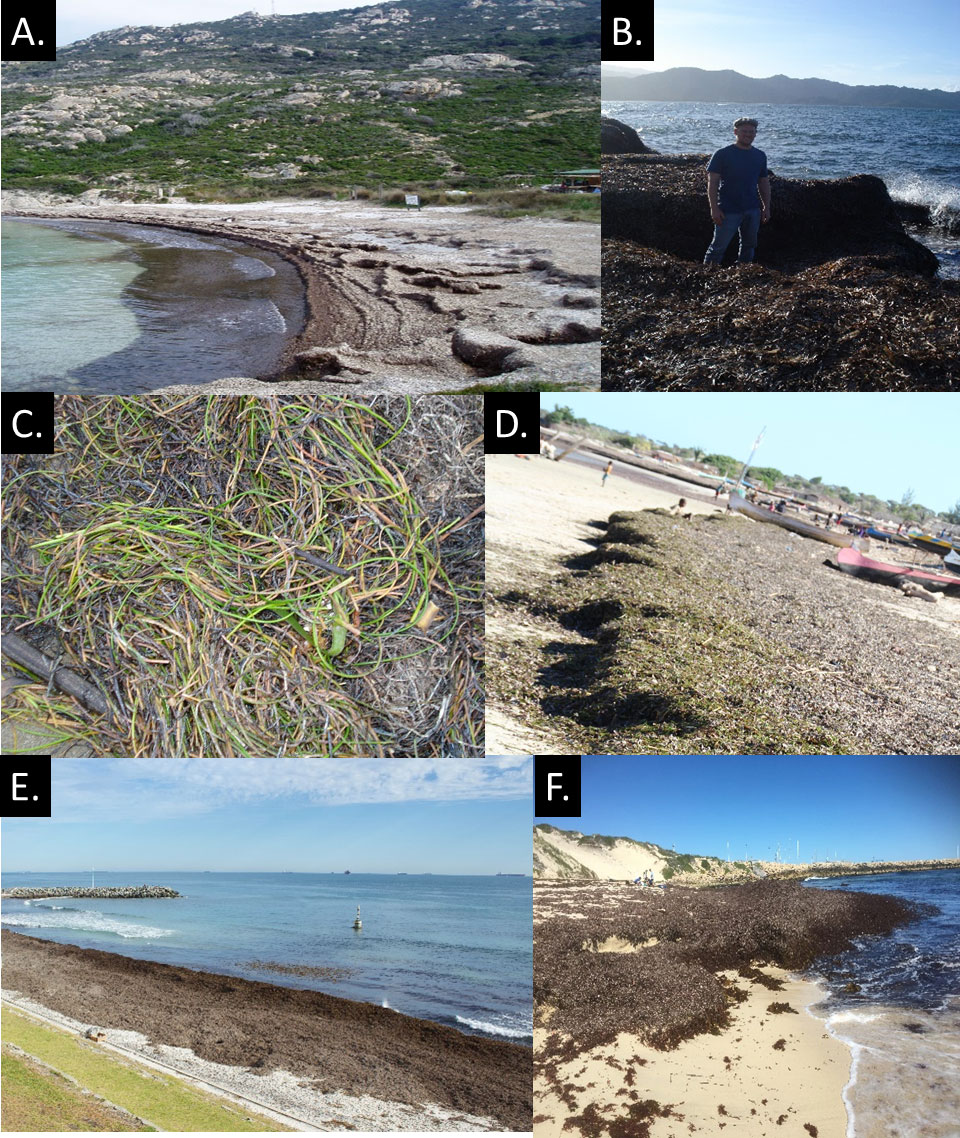
Figure 1 Some example of seagrass beach cast. (A) beach accumulation of Posidonia oceanica dead leaves, also known locally as “banquette”, on Alga Bay Beach, Calvi, Corsica, March 2007; (B) 2 m high banquette on a beach in St Florent Gulf, St Florent, Corsica; (C) beach cast of Syringodium filiforme and other tropical species on a Guadeloupe beach (Caribbean Sea); (D) brown and green leaves of diverse tropical species (mainly Cymodocea serrulata, Syringodium isoetifolium, Thalassia emprichii and Halodule uninervis) on Andravo beach (Toliara, Madagascar), May 2018; (E) and (F) beach cast in the Perth region of southwestern Western Australia. Typically, it comprises Posidonia spp., Amphibolis spp., the kelp Ecklonia radiata and other brown and red macroalgae.
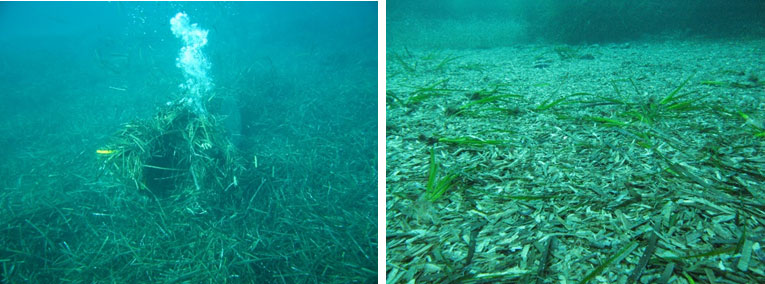
Figure 2 Subtidal accumulation of Posidonia oceanica macro detritus. Left: November 2010, recently exported dead leaves (80 cm thick), 10 m depth, Revellata Bay, Calvi, Mediterranean Sea. Right: June 2012, fragmented macrophytodetritus with uprooted living P. oceanica shoots, 10 m depth, Revellata Bay, Calvi Corsica.
Here we discuss the potential flow-on effects of tropicalization of temperate seagrass meadows to the functioning of other inter-connected habitats in the coastal seascape, focusing on shifts in the quantity and quality of detritus exported from seagrass meadows and imported into AEM habitats (Figure 3). We use the term tropicalization to refer both to the expansion of tropical species to temperate areas (Arntz and Tarazona, 1990) and to the functional change occurring in temperate ecosystems due to this species shift (Vergés et al., 2014a). We will focus in particular on seagrass ecosystems and AEM in temperate Western Australia and Mediterranean Sea, regions which are characterized by large and expansive seagrass meadows comprising the genus Posidonia and have been shown to be already affected by tropicalization (Vergés et al., 2014a) and where structure and function of subtidal or intertidal AEM are relatively well documented. We will also refer to tropical seagrass meadows functioning as a possible functional scenario for temperate areas under tropicalization threats. Prior to this discussion, we provide a synopsis of what is currently known about the ecosystem functioning of AEM habitats.
Macrophytodetritus accumulation as habitats
Unlike seagrass meadows that support both green and brown food webs, habitats formed by AEM generally support brown food webs and display their own characteristics compared to seagrass meadows or the surrounding environment (Boudouresque et al., 2016). For example, AEM may experience very fast change in detrital composition and quantity (height, cover) or in oxygenation (Mascart et al., 2015; Ricart et al., 2015), and be highly variable across seasons in temperate regions particularly (Romero et al., 1992). In Calvi bay, studied AEM accumulation height varies seasonally from 5cm (i.e. less than 400 gDM.m-2) of very compact and degraded material in spring to more than 50cm (1800 gDM.m-2) of less compacted material just after the dead leaf export in November (Mascart et al., 2015). Depending on hydrodynamics and seascape morphology, subtidal AEM habitats can be highly mobile, displaced or disrupted by tidal currents, waves and storms (Ricart et al., 2015, Slim et al., 1996). Oxygen is often depleted inside the AEM due to decomposition and remineralization processes and intrusion of reduced compounds from sediment (e.g. sulfides, ammonium) (Vetter and Dayton, 1998; Gallmetzer et al., 2005) and hypoxia is often experienced by fauna (Gallmetzer et al., 2005; Mascart et al., 2015). Furthermore, composition of these AEM is highly variable. In temperate regions, they are often mixed with various macroalgae detached from their substrate (e.g. Lepoint et al., 2006; Crawley et al., 2009), or with litter from terrestrial or salt marsh origin, notably in areas close to estuaries or transitional waters (Mancinelli and Rossi, 2002; Mascart et al., 2015) (Table 1). In the tropics, mangrove leaves as well as detached macroalgae from rocks and coral reefs can contribute to the AEM (Slim et al., 1996). In addition, anthropogenic material such as microfiber can be present and enter the food web (Remy et al., 2015). While AEM is generally considered heterotrophic with high respiration and remineralization rates, detached macroalgae can still be living and photosynthetically active in subtidal habitats (Frontier et al., 2021), providing an autotrophic source of production for a period prior to the algae senescing. Furthermore, leaf litter can be colonized by macro and micro epiphytes (e.g. microalgae including diatoms) (Lepoint et al., 2006), paradoxically, providing a source of primary production in a heterotrophic system. Despite this, AEM is typically driven by decomposition through the colonization and expansion of microbial decomposers (bacteria, fungi) (Cuomo et al., 1987; Lepoint et al., 2006; Singh et al., 2021). Little is known about the microbial communities in AEM, but microbial decomposers can contribute to a large part of nitrogen and other nutrients found within the dead material of salt marshes (Newell et al., 1989) and it could also be the case in seagrass AEM.
AEM can be viewed as a pulsed system (Yang et al., 2008), where litter pulses provide both habitat and food for resident fauna (Mancinelli and Rossi, 2002; Remy et al., 2017; Costa et al., 2021). Beach AEM is generally colonized by specialized semi-aquatic fauna. Crustaceans, particularly talitrid amphipods and isopods, are by far the dominant taxa in beach-cast systems (see Hyndes et al., In press). In subtidal AEM, species are more related to adjacent donor ecosystems (i.e. macroalgae or seagrass habitats), but are often inhabited by fewer species than vegetated habitats (Borg et al., 2006; Como et al., 2008; Calizza et al., 2013). It is likely that some of the small vagile species found in AEM are exported from donor habitats via their substrate (dead leaf or ripped macroalgae). Fauna transport via rafting (floating or not) is known to be an important colonizing process in coastal areas (Thiel and Gutow, 2005). Regardless of the composition of the AEM (seagrass, reed, macroalgae or kelp), these less diverse macrofauna communities are dominated by crustaceans (particularly amphipods and isopods), and to a lesser extent, annelids, mollusks and echinoderms (Vetter, 1995; Vetter, 1998; Gallmetzer et al., 2005; Mancinelli et al., 2007; Duggins et al., 2016; Costa et al., 2019; de Bettignies et al., 2020). Copepods generally dominate the meiofauna of subtidal AEM (Mascart et al., 2015), but the hypoxic sediment under AEM or beach cast are often dominated by nematodes (Vetter and Dayton, 1998). Meiofauna and macrofaunal densities in AEM, whatever their composition, are generally huge (densities from 1×105 to 1×107 individuals m-1 are often observed), often far above densities measured in seagrass meadows (Vetter, 1998; Norkko et al., 2000; Gallmetzer et al., 2005; Como et al., 2008; Mascart et al., 2015). These high invertebrate densities are likely to provide an accessible foraging resource for the many fishes that are observed around intertidal and subtidal AEM (Crawley et al., 2006; Boudouresque et al., 2016; Baring et al., 2019). In Mediterranean surf zones, these fishes, mostly represented by invertivorous (e.g., labrids) and omnivorous species, are observed feeding in P. oceanica AEM and using the resuspended dark-brown vegetal fragments to hide; higher species richness and fish density are observed on AEM than on sand. (Bussotti et al., 2022).
The food web in subtidal P. oceanica AEM in the Mediterranean Sea is simplified when compared to P. oceanica meadows due to a lower species diversity and the absence of trophic guilds such as suspension feeders (Remy et al., 2018). Herbivorous fishes such as Sarpa salpa that are dominant in P. oceanica meadows (Havelange et al., 1997) can be observed around AEM but not feeding in them (Jadot et al., 2006). Consumers of AEM are dominated by detritivores (i.e. consumers of dead material), but herbivores (consumers of living macrophytes or microepiphytes) or consumers switching between the two modes are also present and sometimes dominant (Remy et al., 2018). While there is no evidence of direct consumption of P. oceanica detritus by talitrid amphipods in beach cast (Colombini et al., 2009), gut contents provide evidence of the ingestion of dead seagrass material by dominant taxa, in particular by amphipods in subtidal AEM (Lepoint et al., 2006). Furthermore, stable isotopes show that these dominant species also assimilate carbon from ingested seagrass (Vizzini and Mazzola, 2008; Remy et al., 2018). This assimilation occurs directly (i.e. digestive ability of the species itself) or indirectly (i.e. assimilation of microbial biomass degrading seagrass detritus). The first enhances mechanical degradation of macro-detritus (Costa et al., 2019), while the second way is potentially important for most species of meiofauna (Mascart et al., 2018). Additionally, it is demonstrated that the food web responds to seasonal pulses of litter, with an increase of litter contribution to the diets of dominant invertebrates, including meiofauna, in autumn (Mascart et al., 2018; Remy et al., 2021). Compared to the Mediterranean, there is limited evidence of direct consumption of seagrass detritus in Western Australia. Similar to seagrass meadows (Smit et al., 2005; Smit et al., 2006), macroalgae typically fuel the food web (Hyndes and Lavery, 2005), although seagrass detritus may contribute as a food source for a limited number of species on beaches (Ince et al., 2007). Indeed, the dominant detritivore in surf-zone AEM in the region, the amphipod Allorchestes compressa, feeds preferentially and almost exclusively on macroalgae, particularly kelp and other brown algae (Crawley and Hyndes, 2007; Crawley et al., 2009), and these detritivores form the bulk of the diet of fish in the AEM (71-99%; Crawley et al., 2006). Thus, this semi-aquatic amphipod, like many other species in beach ecosystems elsewhere in the world (Hyndes et al., In press), displays high feeding rates and preferences for kelp.
Tropicalization
The poleward expansion of tropical species resulting from ocean warming has occurred throughout the world, including seagrasses (Kim et al., 2009; Virnstein and Hall, 2009; Gorman et al., 2016). The pole-ward range extensions of tropical herbivorous fishes have had profound effects on ecosystem structure and functioning of kelp forests in Japan, Australia and the Mediterranean Sea (Vergés et al., 2014a). Spread of tropical species into the Mediterranean Sea has occurred since the opening of the Suez Canal in 1869, resulting in the introduction of Red Sea species in east Mediterranean Sea (known as Lessepsian species) (Bianchi and Morri, 2000). Additionally, tropical species of macroalgae, seagrasses, invertebrates and fishes have been introduced via tropical aquarium release and maritime transport (Katsanevakis et al., 2014; Galil et al., 2018; Zenetos and Galanidi, 2020). Until recently, many introduced species were only settled in the southern basin of the Mediterranean Sea and often considered as native by present day fishermen, but recent sea temperature increases have now allowed Lessepsian species to expand their range northward (all Aegean Sea, and Adriatic and northern Mediterranean basin) (Katsanevakis et al., 2014; Zenetos and Galanidi, 2020; Thibaut et al., 2022). This has also allowed species that have recently been introduced via ballast water, yachting or aquarium trading to acclimate themselves in Adriatic and north-western Mediterranean waters (Katsanevakis et al., 2014).
In Western Australia, a warm pole-ward flowing current, the Leeuwin Current, strongly influences the coastal waters along the entire west coast. While this current has been a feature of the coast for millennia, ocean temperatures have been increasing ~0.1°C per decade for the last 100 years, increasing to ~0.3°C per decade since the mid-1980s (Pearce and Feng, 2007; Lough, 2008). On top of this, a marine heatwave event in the summer of 2010/11 increased sea surface temperatures by up to 5°C above average, leading to the transport and maintenance of tropical species in temperate waters, and major seagrass loss in the sub-tropics (Strydom et al., 2020) and functional changes to temperate reefs due to declining kelp biomass and increasing abundances of herbivorous fishes (Wernberg et al., 2013). Since the 2010/11 marine heatwave, several tropical fish species have been observed in temperate coastal waters (Lenanton et al., 2017; Zarco-Perello et al., 2020). The impact on temperate seagrasses by these tropical invaders is currently unknown, but it is predicted that they will substantially increase grazing rates on seagrass leaves over the next century (Hyndes et al., 2016). This will be enhanced by the pole-ward shift in the distribution of the seagrass-grazing megafauna dugongs and green turtles, and ultimately, there will be a shift in seagrass species to those from the tropics (Hyndes et al., 2016). Combined, this tropicalization will lead to fundamental changes in the ecosystem functioning of seagrasses in the region.
Shifts in animal community living in AEM
Most AEM macrofauna are dominated in diversity and abundance by small crustacea (mainly amphipods and copepods, sometimes isopods, decapods and leptostracans), small mollusks and errant annelids. Most of them are also found in the litter layer of the meadow itself (Como et al., 2008). Moreover, they are generally not specific to one type of detritus accumulation: species colonizing seagrass AEM are also found in macroalgae or reed detritus (Péres and Picard, 1964, Mancinelli and Rossi, 2002). Physico-chemical and biological conditions act as a strong environmental filter: most AEM macrofauna are opportunist species tolerant to short-term environmental change (oxygen, salinity, temperature) and able to cope with habitat and food source variability and quality. Beside diversity or abundance changes linked to change of their composition (Haram et al., 2020) (see below), AEM are likely to be also colonized by non-native species and therefore to be subject to biodiversity tropicalization. AEM have presently received little attention from an invading species point of view and, globally, small invertebrate changes are clearly less known than changes in macrophytes, large invertebrates or fishes. Nevertheless, in Mediterranean Sea, Lessepsian amphipod invaders are recorded in Levantine waters, Aegean Sea and South Western Mediterranean and are likely to spread north like other taxa (Sezgin et al., 2007; Christodoulou et al., 2013; Khammassi et al., 2019). We can predict that tropical species invading AEM will possess opportunistic behavior, plastic trophic ecology and high tolerance to short-term environment parameter changes (e.g. oxygen), like native AEM macrofauna. It is difficult to predict if their tropical origin will give them competitive advantage over native fauna (such as higher metabolism, higher growth and reproductive rate) and it is possible that current AEM communities resist these new invaders. Nevertheless, if current AEM macrofauna is outcompeted and replaced by other species, it is likely to have consequences on coastal fish that use AEM as nursery or as foraging habitats.
Shifts in the export of seagrass detritus
Tropicalization of seagrass ecosystems is predicted to influence the supply of seagrass detritus to other ecosystems through a number of processes: (1) increases in tropical grazers will lead to greater consumption of seagrass biomass and reduced release of leaves for internal processing or export; (2) increases in microbial and invertebrate processing with increasing temperatures and invasive invertebrates will lead to less export due to increased internal cycling and a change in the quality of litter being exported; (3) shifts in seagrass species to tropical species will lead to less export of detritus; and/or (4) seagrass meadows exhibiting a shift from seagrass to macroalgae will shift the quality of the material in AEM. Most of these potential changes will affect AEM as habitat for associated fauna and AEM trophic functioning. It must be noted that these processes are not mutually exclusive, and are likely to have a compounding influence on the amount of seagrass detritus being exported from seagrass systems.
Seagrass beds in tropical areas show higher herbivore diversity than temperate areas and, potentially, higher herbivory rate in relation with absence of biomass seasonality (i.e. continuous grazing) (Heck and Valentine, 2021). Tropical herbivore fishes, mainly rabbitfishes and parrotfishes, are successfully expanding from tropical to more temperate waters where they have been shown to increase grazing on macroalgae (Vergés et al., 2014a; Vergés et al. 2014b). Far less is known about the effects of these and other grazers on seagrass meadows, but we do know that seagrass grazing fishes are far more abundant and diverse in the tropics compared temperate seagrass beds (Valentine and Duffy 2006; Heck et al., 2021). In Western Australia, Hyndes et al. (2016) predicted that these tropical fishes will be the first to increase their ranges to temperate regions, with a Siganus species already establishing a viable population in southwest Western Australia (Lenanton et al., 2017) and occurring in seagrass meadows (Zarco-Perello et al., 2020). Little is known of their impact on the temperate seagrasses, but very little herbivory occurs in these temperate systems normally. In the Mediterranean Sea, the endemic sparid S. salpa can graze on significant amounts of the dominant seagrass, P. oceanica, in the region (Havelange et al., 1997, Tomas et al., 2005). However, tropical species have moved into the Mediterranean. In eastern Mediterranean Sea, occurrences of rabbitfish have been reported since the beginning of 20th century (Shakman and Kinzelbach, 2007). Furthermore, Siganus spp. and parrotfishes have invaded P. oceanica meadows, sometimes replacing S. salpa in the region. This invasion has significantly increased the diversity and abundance of herbivore fishes in Mediterranean (Stergiou and Karpouzi, 2002), where they have been shown to consume P. oceanica (Stergiou, 1988). Both in Western Australia and in Mediterranean Sea, this has increased the functional diversity as the co-existing species have different trophic niches (Azzurro et al., 2007; Zarco-Perello et al., 2020). High herbivory rates are often recorded in tropical seagrass meadows (Heck et al., 2021), and with tropicalization of herbivores, it is the likely fate of temperate seagrass meadows. Increases in abundance, species diversity and functional diversity of herbivores will likely lead to an increase of the proportion of primary production removed by herbivores (Ozvarol et al., 2011; Hyndes et al., 2016; Zarco-Perello et al., 2020) and, therefore, reduce macrophytodetritus production, disrupt the detritus cycle and reduce its export (Hyndes et al., 2016). This would likely result in a decrease of AEM occurrence and abundance in recipient ecosystems and, therefore, reduce the subsidies this material provides in those ecosystems.
Increases in herbivory will also shift the production of macrophytodetritus to feces (Zarco-Perello et al., 2019), drastically changing the nature and the size of exported detritus to dissolved and fine particulate matter. This would lead to a decrease in the value of AEM as both habitat and food for fauna since seagrass-based feces do not have the same nutritive characteristics or composition than senesced detritus (Velimirov, 1984; Velimirov, 1987), and would not provide the habitat structure that senescing leaves provide. Furthermore, while larger sections of seagrass leaves are likely to be released from the foraging activities of herbivores, the release and export of these green leaves are likely to occur at different rates, times and amounts compared to the seasonal release of senescing leaves under normal temperate conditions. Furthermore, these exported green leaves would be at a lower level of decomposition compared to senesced leaves and potentially less palatable. In P. oceanica AEM, green leaves are generally not found in gut content of AEM macrofauna, except the crab Lissocarcinus navigator (Remy et al., 2018). Thus, the functional tropicalization of seagrass meadows would likely result in modified amounts and quality of macrophytodetritus exported to other systems, deeply affecting AEM occurrence, location, abundance, quality as habitat, and food webs in recipient systems.
Seagrass leaf litter is initially processed in the seagrass meadow itself, when released leaves fall and lay between standing shoots. Decomposition by microbes, and consumption by animals, start in the meadow during the senescing process (Harrison, 1989). Increasing temperature is likely to increase microbial metabolism leading to a greater generation and export of dissolved versus particulate organic material in macrophyte habitats (Säwström et al., 2016). For example, a 5°C temperature increase has been shown to boost the short term decomposition rates of Zostera muelleri from 0.04 to 0.06 d-1 and this effect increases with decomposition progress (Trevathan-Tackett et al., 2020).
Detritivores are also present in the litter laying in donor meadow (e.g. Gambi et al., 1992; Como et al., 2008 for P. oceanica system). Like microbes, increasing temperatures are likely to increase the metabolism of temperate detritivores in seagrass meadows, thereby increasing the consumption rate of litter, and facilitating its decomposition. Presence of detritivore is shown to boost seagrass detritus mechanical degradation (e.g. Costa et al., 2019). A shift to more tropical conditions will also likely affect the processing of detritus by macrofauna. It appears that many invertebrate species in tropical seagrass meadows depend on seagrass detritus (Vonk et al., 2008a). Additionally, tropical seagrass meadows are massively colonized by different burrowing crustaceans (e.g. Thalassanidae shrimp) (Vonk et al., 2008b; Kneer et al., 2008). Many of those crustaceans either use detritus as a food source which is found in the sediment or they actively collect plant material from the area surrounding their burrow openings (Kneer et al., 2008). The burrowing shrimps Neaxius acanthus and Alpheus macellarius have been shown to collect in their burrows an amount of seagrass leaf material corresponding to more than 50% of the leaf production in a tropical meadow (Vonk et al., 2008b). Native burrowing shrimp are also present in the Mediterranean Sea where they also exhibit such behavior regarding macrophyte detritus (Papaspyrou et al., 2004), but their impact on litter processing is probably lower than in tropical regions considering their lower abundance and size. Presently, to our best knowledge, tropical burrowing shrimp have not been recorded in the Mediterranean Sea, but they could occur in the near future through either their range extensions via tropicalization or aquarium introductions. This could lead to an increase of litter processing in the meadow itself, mimicking the functioning of tropical seagrass meadow and therefore reduce the amount of exported detritus.
Increased in situ degradation rates and detritus fragmentation by fauna will decrease the amount of macrophytodetritus exported from seagrass meadows and, therefore, alter the occurrence and abundance of AEM in other systems. It will also affect the quality of the exported material as the seagrass material will be more degraded and fragmented and will contain less epiphytes, leading to material more impoverished in nutrients. More degraded and fragmented material devoid of epiphytes are potentially less valuable as habitat or food sources for associated fauna in those recipient systems.
Ultimately, tropicalization could lead to a shift in meadow composition from temperate to tropical seagrass species (Hyndes et al., 2016). The tropical seagrass Halophila stipulacea, a Lessepsian species, has been observed in the southern part of Mediterranean Sea since the opening of the Suez Canal. It has progressively settled northward and has been frequently observed in Ionan Sea since the 1990s, detected in Tyrrhenian Sea in 2006) (Di Genio et al., 2021), and is presently reaching northwestern Mediterranean (Winters et al., 2020; Di Genio et al., 2021; Thibaut et al., 2022). In Cannes harbor (NW Mediterranean Sea), H. stipulacea was probably introduced via big yachting coming by Suez Canal and are establishing stable population which could indicate that conditions are now more favorable for long-term population establishment (Thibaut et al., 2022). H. stipulacea is presently the only non-native seagrass in the Mediterranean Sea (García-Escudero et al., 2022). It generally colonizes habitats devoid of native seagrass, forms mixed meadows with C. nodosa (Winters et al., 2020), or colonizes dead matte of P. oceanica (Thibaut et al., 2022). Presently, H stipulacea is not replacing any native seagrass species, but this may occur when temperatures rise beyond the thermal tolerances of the native species (Wesselmann et al., 2021). In comparison, this invasive seagrass has already spread rapidly and displaced several native macrophytes in eastern Caribbean islands (Winters et al., 2020). H. stipulacea is a small seagrass species, compared to P. oceanica and C. nodosa, the two main native Mediterranean species. The lower biomass and a higher leaf renewal rate (Duarte 1991), which reduce epiphytic load (Borowitzka et al., 2006) will alter the amount and timing of detritus export, and the morphological difference in the leaves of H. stipulacea will reduce the 3D-structure of the AEM habitat. Also, the higher levels of decomposition in tropical seagrasses (Hyndes et al., 2014), at least partly reflecting the more efficient recycling of detritus in the meadow itself (Vonk and Stapel, 2008; Hyndes et al., 2014), will reduce the export of seagrass detritus. While there is no evidence of this shift to tropical species in southern Western Australia yet, Hyndes et al. (2016) predicted that several tropical species, including Cymodocea rotundata, Enhalus acoroides, and Thalassodendron ciliatum, will extend their southern limit by over 500 km, and temperate species such as Amphibolis antarctica or Posidonia spp. will reduce their distribution ranges by 200 km by 2100. This is predicted to result in the tropical system replacing the current temperate seagrass ecosystem, and altering the processing and export of detritus as stated above.
From the above, shifts to tropical species, along with increased processing of litter within the meadow via microbial degradation, burrowing organisms or other detritivores is likely to decrease macrophytodetritus export, and, therefore occurrence and quantity of AEM. Nevertheless, global change has very complex consequences – not only tropicalization - and for example, the predicted increase of storm and wind gusts frequency could increase this export and counteract the accelerated in situ processing (i.e. decomposition or consumption) of litter. The influence of these two opposite trends should be determined by the match (or the mismatch) between leaf fall period (or cycle) and occurrence of export.
Shifts in other forms of detritus across the seascape
The composition of AEM is often very heterogeneous and, depending on the proximity of the recipient system to different forms of donor material (i.e. macroalgae, salt marshes or mangroves) or local conditions that contribute to its composition and retention (Mancinelli and Rossi, 2002; Wernberg et al., 2006; Mascart et al., 2015). The AEM composition and diversity influences the diversity of the communities inhabiting them (Mancinelli and Rossi, 2002; Bishop and Kelaher, 2013; Haram et al., 2020) and the food web functioning (Vonk et al., 2016). For example, the addition of detritus from the invasive Caulerpa taxifolia drastically changed the macroinvertebrates in soft sediments of estuaries of eastern Australia (Taylor et al., 2010). Furthermore, considering the role of drift macroalgae as raft for benthic invertebrates (Thiel and Gutow, 2005), the nature of drift macroalgae can influence the composition of AEM macrofauna (Baring et al., 2014). Absence of drift macroalgae in AEM could lead to fauna impoverishment by the absence of new colonizers. This could happen where overgrazing by new tropical grazers leads to declines of loss of macroalgae standing stock in donor systems (Vergés et al., 2004b). Knowledge of vegetated benthic communities beyond seagrass meadows at the seascape level (e.g. macroalgae habitats, saltmarsh or mangroves) is therefore essential to understand the values and functioning of AEM (Ricart et al., 2015).
In the Mediterranean Sea, macroalgae assemblages on rocks are deeply affected by invasive species, which are often of tropical origin (Piazzi and Balata, 2009). Changes of macroalgae in hard substrate habitats have a direct effect on the material found in AEM. Multi causal and long-term trends in the loss of native forests of the macroalgae Cystoseira spp. in the Mediterranean Sea (Piazzi and Ceccherelli, 2017) have resulted in these algae no longer being present in the AEM (G. Lepoint, personal observation in Revellata Bay, Corsica), and being replaced as drift algae in AEM by other native brown algae or invasive species. Similar to seagrasses, another threat on macroalgae biota is the increase in herbivory due to invading herbivorous fishes. Both in Western Australia and Mediterranean Sea, they have already had drastic impacts on macroalgae habitats leading to an increase of standing stock consumption and sometimes creating barrens dominated by incrusting corallinaceae (Vergés et al., 2014b; Zarco-Perello et al., 2017). Replacement of macroalgae habitats by barrens will affect AEM composition by decreasing the contribution of drift macroalgae to detritus accumulations. Absence of macroalgae in AEM may affect the quality of habitat and food for AEM macrofauna. For example, in Western Australia, the kelp Ecklonia radiata provides a critical food source for the food web in AEM of surf zones comprising both seagrass and kelp (Crawley et al., 2009), yet this kelp is being lost from reefs in the region due to marine heat waves (Wernberg et al., 2016). While Sargassum spp. may replace kelp on reefs and can contribute to the surf-zone food web (Crawley and Hyndes, 2007), it is unclear how much of this material will contribute to the AEM in the surf zone.
Mediterranean seagrass meadows (i.e. P. oceanica and Cymodocea nodosa) are also invaded by the green macroalga Caulerpa cylindracea, which was introduced via ballast water or aquarium trading (Piazzi et al., 2016 for a review). This is an endemic species from southwestern Australia that showed high development capacity throughout the central and western Mediterranean Sea at the end of the past century (Piazzi et al., 2016). Presently, C. cylindracea is often restricted to the meadow periphery in healthy meadows, but dead matte of P. oceanica meadow and anthropized areas are often heavily colonized (Piazzi et al., 2016). Where C. cylindracea occurs, Caulerpa fragments are found in Posidonia AEM (G. Lepoint, Pers. Obs. in Calvi Bay).
Changes in contributions, but also specific diversity and quantity of dead seagrass leaves vs drifted macroalgae will probably change AEM food web topology and energy fluxes. Influence of AEM composition on food web structure is not well known but, as stated before, living algae material (i.e. micro and macro epiphytes, decaying drifted macroalgae) is often preferred over dead seagrass material (Hyndes and Lavery, 2005), even if dominant fauna component are able to use dead seagrass and respond positively to dead material pulse (Remy et al., 2017; 2021). A relative increase of macroalgae contribution (or a relative decrease of dead seagrass contribution) would lead to green AEM food web and to select more herbivorous species. Nevertheless, many species found in subtidal AEM, particularly gammarid amphipods, are relatively plastic regarding their diet and are found in AEM whatever their composition. These more plastic and opportunist species may adapt their diet to new AEM composition. Nevertheless, changes in the source habitat may also affect AEM food web in two other ways. Firstly, many tropical algae are often better defended against herbivory (e.g. Demko et al., 2017) and, therefore, could be less integrated in the AEM food web. Change in macroalgae sourcing via the replacement of native species by tropical invaders is therefore likely to affect potential food sources available for AEM inhabitants, food web structure and detritus processing. Secondly, herbivory increase in tropicalized seagrass meadows would lead to an increase of feces export relative to macrophytodetritus export. This could have consequence for AEM as habitat but also on AEM food web as fecal material do not have the same dietary quality than macrophytodetritus (see above).
Conclusions and perspectives
There is considerable evidence that seagrass meadows are already shifting to another state under the pressure of global change (Strydom et al., 2020), and this rate of change is likely to increase in the future (Hyndes et al., 2016). In temperate areas, tropicalization of seagrass communities and/or functioning is one potential scenario of these changes. Temperate seagrass beds are recognized as a net autotrophic ecosystem, exporting variable parts of its primary production to other marine and coastal terrestrial systems. Here, we show that shifts in the quantity and quality of exported detritus is likely to impact the functioning of AEM in recipient ecosystems across the coastal seascape. AEM could change either through: (1) declines in biomass or loss of seagrass directly due to increased ocean temperatures (e.g. via marine heat waves) or increased herbivory from tropicalized herbivores shifting seagrass export to dissolved or finer particulate material; (2) increased degradation and processing of seagrass detritus within seagrass meadows leading to reduced export; (3) replacement of large temperate seagrass species with smaller tropical seagrass species with different leaf cycling, or macroalgae species; and/or (4) loss or changes to macroalgae species in neighboring habitats that export detritus (Figure 3B). These processes will alter the amount, composition, quality, timing and frequency of inputs of detritus into ecosystems that rely on AEM as trophic subsidies, which will alter the suitability of AEM as habitat and food for invertebrates. In addition, changes in exported material associated with those processes could decrease “rafting” of invertebrates into recipient habitats, thereby altering recruitment of invertebrates, while the invasion of tropical opportunistic invertebrates could alter the invertebrate community structure of AEM. It is possible that present dominant species resist these invasions considering these dominant species are able to cope with a very large range of environmental conditions and are able to adapt to diverse AEM type and composition.
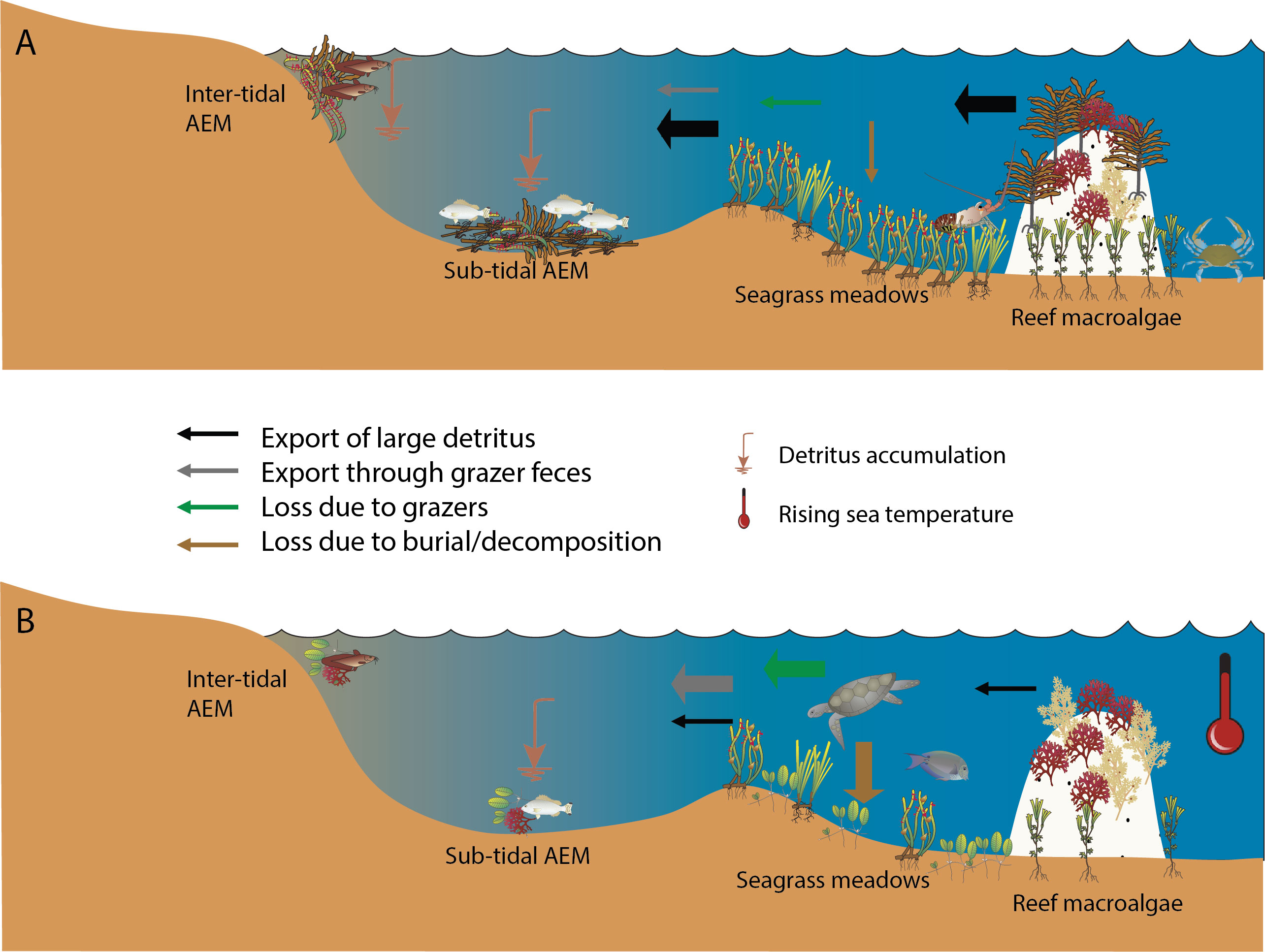
Figure 3 Conceptual representation of the relative magnitude of the export of particulate and dissolved organic matter from seagrass meadows and other subtidal macrophyte systems: (A) under current temperate conditions; and (B) and under tropicalized conditions with increasing sea temperatures. Changes in the forms of seagrass, macroalgae and consumers are represented by the different symbols. The color and thickness of each arrows indicates the type and the relative importance of the vector, respectively.
Ultimately, tropicalization of temperate seagrasses will alter the export of seagrass detritus, and as a result, the AEM food web will change too as food web topology and energy flow are largely linked to AEM composition. Notably, this will be largely influenced by the relative proportion of macroalgae vs seagrass detritus (Crawley et al., 2009; Haram et al., 2020; Remy et al., 2021). Increases of macroalgae contributions could shift this brown food web greener than presently when AEM has been dominated by dead seagrass leaves. However, beyond beaches and to a lesser extent surf zones, we know very little about the biodiversity and functioning of AEM in marine systems. Yet, AEM is known to accumulate in a range of habitats across the coastal seascapes and offshore systems (Table 1). Furthermore, there has been very limited advances in our knowledge on export rates of seagrass detritus since the review of Heck et al. (2008). Accumulations of kelp detritus in sub-tidal areas have recently received much more attention, showing their importance as habitats, delineating complex associated food webs and underlining their role as trophic subsidies for coastal areas (Duggins et al., 2016, Feehan et al., 2018; Filbee-Dexter et al., 2018; De Bettignies et al., 2020; Frontier et al., 2021). We therefore recommend far greater research effort into determining export rates of seagrass detritus from temperate seagrass meadows, and the role of AEM in a range of sub-tidal coastal and offshore systems. This will allow for a greater understanding of the flow-on effects of tropicalized seagrass ecosystems beyond the systems themselves.
Author Contributions
All authors listed have equally contributed to the work and approved it for publication.
Funding
GL is a senior permanent researcher appointed by the Belgian Fund for Scientific Research (FRS-FNRS). This study was conducted within the scope of FRS-FNRS research project entitle “dynamics of Posidonia oceanica macrophytodetritus accumultaion” contract number: FRFC 2.4511.09).
Conflict of Interest
The authors declare that the research was conducted in the absence of any commercial or financial relationships that could be construed as a potential conflict of interest.
Publisher’s Note
All claims expressed in this article are solely those of the authors and do not necessarily represent those of their affiliated organizations, or those of the publisher, the editors and the reviewers. Any product that may be evaluated in this article, or claim that may be made by its manufacturer, is not guaranteed or endorsed by the publisher.
References
Arntz W. E., Tarazona J. (1990). “Effects of el niño 1982-83 on benthos, fish and fisheries off the south american pacific coast,” in Elsevier oceanography series 52 (Amsterdam: Elsevier), 323–360.
Azzurro E., Fanelli E., Mostarda E., Catra M., Andaloro F. (2007). Resource partitioning among early colonizing Siganus luridus and native herbivorous fish in the Mediterranean: An integrated study based on gut-content analysis and stable isotope signatures. J. Mar. Biol. Assoc. United. Kingdom. 87, 991–998. doi: 10.1017/S0025315407056342
Baring R. J., Lester R. E., Fairweather P. G. (2019). Short-term accumulation of fauna colonising natural versus artificial seagrass floating near to shore. Mar. Biol. 166, 5–11. doi: 10.1007/s00227-019-3505-5
Baring R.J., Fairweather P.G., Lester R.E. (2014). Storm versus calm: Variation in fauna associated with drifting macrophytes in sandy beach surf zones. Journal of Experimental Marine Biology and Ecology 461, 397–406. doi: 10.1016/j.jembe.2014.09.011
Bianchi C. N., Morri C. (2000). Marine biodiversity of the Mediterranean Sea: Situation, problems and prospects for future research. Mar. pollut. Bull. 40, 367–376. doi: 10.1016/S0025-326X(00)00027-8
Bishop M. J., Kelaher B. P. (2013). Context-specific effects of the identity of detrital mixtures on invertebrate communities. Ecol. Evol. 3, 3986–3999. doi: 10.1002/ece3.775
Borg J. A., Rowden A. A., Attrill M. J., Schembri P. J., Jones M. B. (2006). Wanted dead or alive: High diversity of macroinvertebrates associated with living and 'dead' Posidonia oceanica matte. Mar. Biol. 149, 667–677. doi: 10.1007/s00227-006-0250-3
Borowitzka M. A., Lavery P. S., Van Keulen M. (2006). “Epiphytes of seagrasses,” in Seagrasses: Biology, ecology and conservation (Netherlands: Springer), 441–461.
Boudouresque C. F., Pergent G., Pergent-Martini C., Ruitton S., Thibaut T., Verlaque M. (2016). The necromass of the Posidonia oceanica seagrass meadow: fate, role, ecosystem services and vulnerability. Hydrobiologia 781, 25–42. doi: 10.1007/s10750-015-2333-y
Bussotti S., Guidetti P., Rossi F. (2022). Posidonia oceanica wrack beds as a fish habitat in the surf zone. Estuarine. Coast. Shelf. Sci. 272. doi: 10.1016/j.ecss.2022.107882
Calizza E., Costantini M. L., Carlino P., Bentivoglio F., Orlandi L., Rossi L. (2013). Posidonia oceanica habitat loss and changes in litter-associated biodiversity organization: A stable isotope-based preliminary study. Estuarine. Coast. Shelf. Sci. 135, 137–145. doi: 10.1016/j.ecss.2013.07.019
Cambridge M. L., Hocking P. J. (1997). Annual primary production and nutrient dynamics of the seagrasses Posidonia sinuosa and Posidonia australis in south-western Australia. Aquat. Bot. 59, 277–295. doi: 10.1016/S0304-3770(97)00062-4
Cebrian J. (2002). Variability and control of carbon consumption, export, and accumulation in marine communities. Limnol. Oceanogr. 47, 11–22. doi: 10.4319/lo.2002.47.1.0011
Champenois W., Borges A. V. (2012). Seasonal and interannual variations of community metabolism rates of a Posidonia oceanica seagrass meadow. Limnol. Oceanogr. 57, 347–361. doi: 10.4319/lo.2012.57.1.0347
Champenois W., Borges A. V. (2021). Net community metabolism of a Posidonia oceanica meadow. Limnol. Oceanogr. 66, 2126–2140. doi: 10.1002/lno.11724
Christodoulou M., Paraskevopoulou S., Syranidou E., Koukouras A. (2013). The amphipod (Crustacea: Peracarida) fauna of the Aegean Sea, and comparison with those of the neighbouring seas. J. Mar. Biol. Assoc. United. Kingdom. 93, 1303–1327. doi: 10.1017/S002531541200183X
Colombini I., Mateo M.A., Serrano O., Fallaci M., Gagnarli E., Serrano L., et al (2009). On the role of Posidonia oceanica beach wrack for macroinvertebrates of a Tyrrhenian sandy shore. Acta Oecologica-International Journal of Ecology 35, 32–44. doi: 10.1016/j.actao.2008.07.005
Como S., Magni P., Baroli M., Casu D., De Falco G., Floris A. (2008). Comparative analysis of macrofaunal species richness and composition in Posidonia oceanica, Cymodocea nodosa and leaf litter beds. Mar. Biol. 153, 1087–1101. doi: 10.1007/s00227-007-0881-z
Costa V., Chemello R., Iaciofano D., Lo Brutto S., Rossi F. (2021). Small-scale patches of detritus as habitat for invertebrates within a Zostera noltei meadow. Mar. Environ. Res. 171, 105474. doi: 10.1016/j.marenvres.2021.105474
Costa V., Mazzola A., Rossi F., Vizzini S. (2019). Decomposition rate and invertebrate colonization of seagrass detritus along a hydrodynamic gradient in a Mediterranean coastal basin: The stagnone di marsala (Italy) case study. Mar. Ecol. 40 (6). doi: 10.1111/maec.12570
Crawley K. R., Hyndes G. A. (2007). The role of different types of detached macrophytes in the food and habitat choice of a surf-zone inhabiting amphipod. Mar. Biol. 151, 1433–1443. doi: 10.1007/s00227-006-0581-0
Crawley K. R., Hyndes G. A., Ayvazian S. G. (2006). The influence of different volumes and types of detached macrophytes on fish community structure in surf zones of sandy beaches. Mar. Ecol. Prog. Ser. 307, 233–246. doi: 10.3354/meps307233
Crawley K. R., Hyndes G. A., Vanderklift M. A., Revill A. T., Nichols P. D. (2009). Allochthonous brown algae are the primary food source for consumers in a temperate, coastal environment. Mar. Ecol. Prog. Ser. 376, 22–44. doi: 10.3354/meps07810
Cuomo V., Pagano S., Pecorella M. A., Parascandola P. (1987). Evidence of the active role of ligno-cellulosic enzymes of marine fungi in degradation of Posidonia oceanica leaves. Biochem. System. Ecol. 15, 635–637. doi: 10.1016/0305-1978(87)90035-4
De Bettignies F., Dauby P., Lepoint G., Riera P., Bocher E., Bohner O., et al. (2020). Temporal succession of a macrofaunal community associated with kelp fragment accumulations in an in situ experiment. Mar. Ecol. Prog. Ser. 656, 109–121. doi: 10.3354/meps13391
Di Genio S., Gaglioti M., Meneghesso C., Barbieri F., Cerrano C., Gambi M. C. (2021). Phenology and ecology of the alien seagrass Halophila stipulacea in its northern range limit in the Mediterranean Sea. Aquat. Bot. 168, 103304. doi: 10.1016/j.aquabot.2020.103304
Duarte C.M. (1991). Allometric scaling of seagrass form and productivity. Marine Ecology Progress Series 77, 289–300. doi: 10.3354/meps077289
Dugan J.E., Hubbard D.M., Mccrary M.D., Pierson M.O. (2003). The response of macrofauna communities and shorebirds to macrophyte wrack subsidies on exposed sandy beaches of southern California. Estuarine, Coastal and Shelf Science 58, 25–40. doi: 10.1016/S0272-7714(03)00045-3
Duggins D. O., Gómez-Buckley M. C., Buckley R. M., Lowe A. T., Galloway A. W. E., Dethier M. N. (2016). Islands in the stream: kelp detritus as faunal magnets. Mar. Biol. 163, 1–10. doi: 10.1007/s00227-015-2781-y
Feehan C. J., Grauman-Boss B. C., Strathmann R. R., Dethier M. N., Duggins D. O. (2018). Kelp detritus provides high-quality food for sea urchin larvae. Limnology and Oceanography 63, S299–S306. doi: 10.1002/lno.10740
Filbee-Dexter K., Wernberg T., Norderhaug K. M., Ramirez-Llodra E., Pedersen M. F. (2018). Movement of pulsed resource subsidies from kelp forests to deep fjords. Oecologia 187, 291–304. doi: 10.1007/s00442-018-4121-7
Frontier N., De Bettignies F., Foggo A., Davoult D. (2021). Sustained productivity and respiration of degrading kelp detritus in the shallow benthos: Detached or broken, but not dead. Mar. Environ. Res. 166, 105277. doi: 10.1016/j.marenvres.2021.105277
Galil B. S., Marchini A., Occhipinti-Ambrogi A. (2018). East Is east and West is west? management of marine bioinvasions in the Mediterranean Sea. Estuarine. Coast. Shelf. Sci. 201, 7–16. doi: 10.1016/j.ecss.2015.12.021
Gallmetzer I., Pflugfelder B., Zekely J., Ott J. A. (2005). Macrofauna diversity in Posidonia oceanica detritus: Distribution and diversity of mobile macrofauna in shallow sublittoral accumulations of Posidonia oceanica detritus. Mar. Biol. 147, 517–523. doi: 10.1007/s00227-005-1594-9
Gambi M. C., Lorenti M., Russo G. F., Scipione M. B., Zupo V. (1992). Depth and seasonal distribution of some groups of the vagile fauna of the posidonia-oceanica leaf stratum - structural and trophic analyses. Marine Ecology-Pubblicazioni Della Stazione Zoologica Di Napoli I 13, 17–39. doi: 10.1111/j.1439-0485.1992.tb00337.x
García-Escudero C. A., Tsigenopoulos C. S., Gerakaris V., Tsakogiannis A., Apostolaki E. T. (2022). ITS DNA barcoding reveals that Halophila stipulacea still remains the only non-indigenous seagrass of the Mediterranean Sea. Diversity 14, 76. doi: 10.3390/d14020076
Gobert S., Cambridge M. L., Velimirov B., Pergent G., Lepoint G., Bouquegneau J.-M., et al. (2006). Biology of posidonia in: Seagrasses: Biology, ecology and conservation (Amsterdam: Springer Netherlands), 387–408.
Gorman D., Turra A., Bergstrom E.R., Horta P.A. (2016). Population expansion of a tropical seagrass (Halophila decipiens) in the southwest Atlantic (Brazil). Aquatic Botany 132, 30–36. doi: 10.1016/j.aquabot.2016.04.002
Haram L. E., Sotka E. E., Byers J. E. (2020). Effects of novel, non-native detritus on decomposition and invertebrate community assemblage. Mar. Ecol. Prog. Ser. 643, 49–61. doi: 10.3354/meps13335
Harrison P. G. (1989). Detrital processing in seagrass systems: A review of factors affecting decay rates, remineralization and detritivory. Aquat. Bot. 35, 263–288. doi: 10.1016/0304-3770(89)90002-8
Havelange S., Lepoint G., Dauby P., Bouquegneau J.M. (1997). Feeding of the sparid fish Sarpa salpa in a seagrass ecosystem: Diet and carbon flux. Marine Ecology-Pubblicazioni Della Stazione Zoologica Di Napoli I 18, 289–297. doi: 10.1111/j.1439-0485.1997.tb00443.x
Heck K. L. Jr., Carruthers T. J. B., Duarte C. M., Hughes A. R., Kendrick G., Orth R. J., et al. (2008). Trophic transfers from seagrass meadows subsidize diverse marine and terrestrial consumers. Ecosystems 11, 1198–1210. doi: 10.1007/s10021-008-9155-y
Heck K. L. Jr., Samsonova M., Poore A. G. B., Hyndes G. A. (2021). Global patterns in seagrass herbivory: why, despite existing evidence, there are solid arguments in favor of latitudinal gradients in seagrass herbivory. Estuaries. Coasts. 44, 481–490. doi: 10.1007/s12237-020-00833-x
Hemminga M. A., Nieuwenhuize J. (1991). Transport, deposition and in situ decay of seagrasses in a tropical mudflat area (Banc D'Arguin, Mauritania). Netherlands Journal of Sea Research 27, 183–190. doi: 10.1016/0077-7579(91)90011-O
Hyndes G. A., Berdan E. L., Duarte C., Dugan J. F., Emery K. A., Hambäck P. A., et al. (In press). The role of inputs of marine wrack and carrion in sandy-beach ecosystems: a global review. Biol. Rev 97. doi: 10.1111/brv.12886
Hyndes G. A., Heck K. L. Jr., Vergés A., Harvey E. S., Kendrick G. A., Lavery P. S., et al. (2016). Accelerating tropicalization and the transformation of temperate seagrass meadows. BioScience 66, 938–945. doi: 10.1093/biosci/biw111
Hyndes G. A., Lavery P. S. (2005). Does transported seagrass provide an important trophic link in unvegetated, nearshore areas? Estuarine. Coast. Shelf. Sci. 63, 633–643. doi: 10.1016/j.ecss.2005.01.008
Hyndes G. A., Nagelkerken I., Mcleod R. J., Connolly R. M., Lavery P. S., Vanderklift M. A. (2014). Mechanisms and ecological role of carbon transfer within coastal seascapes. Biol. Rev. 89, 232–254. doi: 10.1111/brv.12055
Ince R., Hyndes G. A., Lavery P. S., Vanderklift M. A. (2007). Marine macrophytes directly enhance abundances of sandy beach fauna through provision of food and habitat. Estuar. Coast. Shelf. Sci. 74, 77–86. doi: 10.1016/j.ecss.2007.03.029
Jadot C., Donnay A., Acolas M. L., Cornet Y., Bégout Anras M. L. (2006). Activity patterns, home-range size, and habitat utilization of Sarpa salpa (Teleostei: Sparidae) in the Mediterranean Sea. ICES Journal of Marine Science 63, 128–139. doi: 10.1016/j.icesjms.2005.06.010
Jagt J. W. M., Deckers M., Donovan S. K., Fraaije R., Goolaerts S., van der Ham R., et al. (2019). Latest Cretaceous storm-generated sea grass accumulations in the maastrichtian type area, the Netherlands – preliminary observations. Proc. Geolog. Assoc. 130 (5), 590–598. doi: 10.1016/j.pgeola.2019.05.003
Jedrzejczak M. F. (2002). Stranded Zostera marina L. vs wrack fauna community interactions on a Baltic sandy beach (Hel, Poland): a short-term pilot study. Part I. Driftline effects of fragmented detritivory, leaching and decay rates. Oceanologia 44, 273–286.
Katsanevakis S., Coll M., Piroddi C., Steenbeek J., Lasram F. B. R., Zenetos A., et al. (2014). Invading the Mediterranean Sea: Biodiversity patterns shaped by human activities. Front. Mar. Sci. 1. doi: 10.3389/fmars.2014.00032
Khammassi M., Jourde J., Zaabar W., Laabidi S., Sauriau P. G., Achouri M. S. (2019). Inventory and new records of benthic amphipods from macrophytes and fine sand communities of the bizerte lagoon (Tunisia, SW Mediterranean Sea). Mar. Biodiv. Rec. 12, 24. doi: 10.1186/s41200-019-0182-5
Kim J. B., Park J. I., Jung C. S., Lee P. Y., Lee K. S. (2009). Distributional range extension of the seagrass Halophila nipponica into coastal waters off the Korean peninsula. Aquat. Bot. 90, 269–272. doi: 10.1016/j.aquabot.2008.10.007
Kneer D., Asmus H., Vonk J. A. (2008). Seagrass as the main food source of Neaxius acanthus (Thalassinidea: Strahlaxiidae), its burrow associates, and of Corallianassa coutierei (Thalassinidea: Callianassidae). Estuarine. Coast. Shelf. Sci. 79, 620–630. doi: 10.1016/j.ecss.2008.05.013
Kokubu Y., Rothäusler E., Filippi J. B., Durieux E. D.H., Komatsu T. (2019). Revealing the deposition of macrophytes transported offshore: Evidence of their long-distance dispersal and seasonal aggregation to the deep sea. Scientific Reports 9. doi: 10.1038/s41598-019-39982-w
Lastra M., Page H. M., Dugan J. E., Hubbard D. M., Rodil I. F. (2008). Processing of allochthonous macrophyte subsidies by sandy beach consumers: estimates of feeding rates and impacts on food resources. Marine Biology 154, 163–174. doi: 10.1007/s00227-008-0913-3
Lenanton R. C.J., Roberson A. I., Hansen J. A. (1982). Nearshore accumulations of detached macrophytes as nursery areas for fish. Marine Ecology Progress Series 9, 51–57. doi: 10.3354/meps009051
Lenanton R. C.J., Dowling C. E., Smith K. A., Fairclough D. V., Jackson G. (2017). Potential influence of a marine heatwave on range extensions of tropical fishes in the eastern Indian Ocean—Invaluable contributions from amateur observers. Regional Studies in Marine Science 13, 19–31. doi: 10.1016/j.rsma.2017.03.005
Lepoint G., Cox A. S., Dauby P., Poulicek M., Gobert S. (2006). Food sources of two detritivore amphipods associated with the seagrass Posidonia oceanica leaf litter. Mar. Biol. Res. 2, 355–365. doi: 10.1080/17451000600962797
Lepoint G., Defawe O., Gobert S., Dauby P., Bouquegneau J. M. (2002). Experimental evidence for n recycling in the leaves of the seagrass Posidonia oceanica. J. Sea. Res. 48, 173–179. doi: 10.1016/S1385-1101(02)00164-8
Lough J. M. (2008). Shifting climate zones for Australia's tropical marine ecosystems. Geophysical Research Letters 35. doi: 10.1029/2008GL034634
Mancinelli G., Rossi L. (2002). The influence of allochthonous leaf detritus on the occurrence of crustacean detritivores in the soft-bottom macrobenthos of the po river delta area (northwestern Adriatic Sea). Estuarine. Coast. Shelf. Sci. 54, 849–861. doi: 10.1006/ecss.2001.0861
Mancinelli G., Sabetta L., Basset A. (2007). Colonization of ephemeral detrital patches by vagile macroinvertebrates in a brackish lake: a body size-related process? Oecologia 151, 292–302. doi: 10.1007/s00442-006-0586-x
Mann K. H. (1988). Production and use of detritus in various freshwater, estuarine, and coastal marine ecosystems. Limnol. Oceanogr. 33, 910–930.
Mascart T., De Troch M., Remy F., Loïc N M., Lepoint G. (2018). Seasonal dependence on seagrass detritus and trophic niche partitioning in four copepod eco-morphotypes. Food Webs. 16, 73–79. doi: 10.1016/j.fooweb.2018.e00086
Mascart T., Lepoint G., Deschoemaeker S., Binard M., Remy F., De Troch M. (2015). Seasonal variability of meiofauna, especially harpacticoid copepods, in posidonia oceanica macrophytodetritus accumulations. J. Sea. Res. 95, 149–160. doi: 10.1016/j.seares.2014.07.009
Mateo M. A. (2010). Beach-Cast Cymodocea nodosa Along the Shore of a Semienclosed Bay: Sampling and Elements to Assess Its Ecological Implications. Journal of Coastal Research 26, 283–291. doi: 10.2112/08-1100.1
Mateo M. A., Cebrián J., Dunton K., Mutchler T. (2006). “Carbon flux in seagrass ecosystems,” in Seagrasses: Biology, ecology and conservation (Amsterdam: Springer Netherlands), 159–192.
Mateo M. A., Romero J. (1997). Detritus dynamics in the seagrass Posidonia oceanica: elements for an ecosystem carbon and nutrient budget. Mar. Ecol-Prog. Ser. 151, 43–53. doi: 10.3354/meps151043
Menzies R.J., Rowe G.T. (1969). The Distribution and Significance of Detrital Turtle Grass, Thallassia testudinata, on the Deep‐Sea Floor off North Carolina. Internationale Revue der gesamten Hydrobiologie und Hydrographie 54, 217–222. doi: 10.1002/iroh.19690540206
Michaud K. M., Emery K. A., Dugan J. E., Hubbard D. M., Miller R. J. (2019). Wrack resource use by intertidal consumers on sandy beaches. Estuarine, Coastal and Shelf Science 221, 66–71. doi: 10.1016/j.ecss.2019.03.014
Min X., Yi Z., Xiao-Jing S., Yun-Ling Z., Hai-Peng Z. (2019). The distribution of large floating seagrass (Zostera marina) aggregations in northern temperate zones of bohai bay in the bohai sea, China. PLoS ONE 14. doi: 10.1371/journal.pone.0201574
Moissette P., Koskeridou E., Cornee J.-J., Guillocheau F., Lecuyer C. (2007). Spectacular preservation of seagrasses and seagrass-associated communities from the pliocene of rhodes, Greece. Palaios 22, 200–211. doi: 10.2110/palo.2005.p05-141r
Newell S. Y., Fallon R. D., Miller J. D. (1989). Decomposition and microbial dynamics for standing, naturally positioned leaves of salt-marsh grass Spartina alterniflora. Mar. Biol. 101, 471–481. doi: 10.1007/BF00541649
Norkko J., Bonsdorff E., Norkko A. (2000). Drifting algal mats as an alternative habitat for benthic invertebrates: Species specific response to a transient resource. J. Exp. Mar. Biol. Ecol. 248, 79–104. doi: 10.1016/S0022-0981(00)00155-6
Ochieng C. A., Erftemeijer P. L.A. (1999). Accumulation of seagrass beach cast along the Kenyan coast: A quantitative assessment. Aquatic Botany 65, 221–238. doi: 10.1016/S0304-3770(99)00042-X
Orr M., Zimmer M., Jelinski D. E., Mews M. (2005). Wrack deposition on different beach types: Spatial and temporal variation in the pattern of subsidy. Ecology 86, 1496–1507. doi: 10.1890/04-1486
Orth R. J., Carruthers T. J. B., Dennison W. C., Duarte C. M., Fourqurean J. W., Heck K. L. Jr., et al. (2006). A global crisis for seagrass ecosystems. BioScience 56, 987–996. doi: 10.1641/0006-3568(2006)56[987:AGCFSE]2.0.CO;2
Orth R. J., Van Montfrans J. (1984). Epiphyte-seagrass relationships with an emphasis on the role of micrograzing: A review. Aquat. Bot. 18, 43–69. doi: 10.1016/0304-3770(84)90080-9
Ozvarol Y., Osman Ertan O., Ismail Turna I. (2011). The grazing effect of Siganus luridus rüppell, 1828 on Posidonia oceanica (L.) Delile, 1813 meadows in Turkish Mediterranean coast (Gazipaşa/Antalya). J. Food. Agric. Environ. 9, 531–533.
Papaspyrou S., Thessalou-Legaki M., Kristensen E. (2004). Impact of Pestarella tyrrhena on benthic metabolism in sediment microcosms enriched with seagrass and macroalgal detritus. Mar. Ecol. Prog. Ser. 281, 165–179. doi: 10.3354/meps281165
Pearce A., Feng M. (2007). Observations of warming on the Western Australian continental shelf. Marine and Freshwater Research 58, 914–920. doi: 10.1071/MF07082
Peres J.-M., Picard J. (1964). Nouveau manuel de bionomie benthique de la mer Méditerranée. Edition revue et augmentée. Marseille: Station Marine d'Endoume.
Pergent G., Rico-Raimondino V., Pergent-Martini C. (1997). Fate of primary production in Posidonia oceanica meadows of the Mediterranean. Aquat. Bot. 59, 307–321. doi: 10.1016/S0304-3770(97)00052-1
Perry R. A., Vaudrey J. M.P., Dierssen H. M. (2018). Long range transport and carbon and nitrogen dynamics of floating seagrass wracks in Greater Florida Bay. Estuarine, Coastal and Shelf Science 209, 7–17. doi: 10.1016/j.ecss.2018.05.006
Piazzi L., Balata D. (2009). Invasion of alien macroalgae in different Mediterranean habitats. Biological Invasions 11, 193–204. doi: 10.1007/s10530-008-9224-3
Piazzi L., Ceccherelli G. (2017). Concomitance of oligotrophy and low grazing pressure is essential for the resilience of Mediterranean subtidal forests. Mar. pollut. Bull. 123, 197–204. doi: 10.1016/j.marpolbul.2017.08.061
Poore A. G.B., Gallagher K. M. (2013). Strong consequences of diet choice in a talitrid amphipod consuming seagrass and algal wrack. Hydrobiologia 701, 117–127. doi: 10.1007/s10750-012-1263-1
Remy F., Collard F., Gilbert B., Compère P., Eppe G., Lepoint G. (2015). When microplastic is not plastic: the ingestion of artificial cellulose fibers by macrofauna living in seagrass macrophytodetritus. Environ. Sci. Technol. 49, 11158–11166. doi: 10.1021/acs.est.5b02005
Remy F., Gobert S., Lepoint G. (2017). Effects of an experimental resource pulse on the macrofaunal assemblage inhabiting seagrass macrophytodetritus. Belgian. J. Zoology. 147, 1–15. doi: 10.26496/bjz.2017.1
Remy F., Mascart T., De Troch M., Michel L. N., Lepoint G. (2018). Seagrass organic matter transfer in Posidonia oceanica macrophytodetritus accumulations. Estuarine. Coast. Shelf. Sci. 212, 73–79. doi: 10.1016/j.ecss.2018.07.001
Remy F., Michel L. N., Mascart T., De Troch M., Lepoint G. (2021). Trophic ecology of macrofauna inhabiting seagrass litter accumulations is related to the pulses of dead leaves. Estuarine. Coast. Shelf. Sci. 252, 107300. doi: 10.1016/j.ecss.2021.107300
Ricart A. M., Dalmau A., Pérez M., Romero J. (2015). Effects of landscape configuration on the exchange of materials in seagrass ecosystems. Mar. Ecol. Prog. Ser. 532, 89–100. doi: 10.3354/meps11384
Ricart A. M., Pérez M., Romero J. (2017). Landscape configuration modulates carbon storage in seagrass sediments. Estuarine. Coast. Shelf. Sci. 185, 69–76. doi: 10.1016/j.ecss.2016.12.011
Romero J., Pergent G., Pergentmartini C., Mateo M. A., Regnier C. (1992). The detritic compartment in a Posidonia oceanica meadow – litter features, decomposition rates, and mineral stocks. Mar. Ecol-Pubb. Della. Stazione. Zoologica. Di. Napoli. I. 13, 69–83. doi: 10.1111/j.1439-0485.1992.tb00341.x
Ruiz-Delgado M. C., Vierheller Vieira J., Reyes-Martínez M. J., Borzone C. A., Sánchez-Moyano J. E., García-García F. J. (2016). Wrack removal as short-term disturbance for talitrus saltator density in the supratidal zone of sandy beaches: An experimental approach. Estuaries and Coasts 39, 1113–1121. doi: 10.1007/s12237-015-0060-2
Säwström C., Hyndes G. A., Eyre B. D., Huggett M. J., Fraser M. W., Lavery P. S., et al. (2016). Coastal connectivity and spatial subsidy from a microbial perspective. Ecology and Evolution 6, 6662–6671. doi: 10.1002/ece3.2408
Sezgin M., Bakir K., Katağan T. (2007). New record of a lessepsian amphipod from the levantine coast of Turkey: Elasmopus pectenicrus (Bat). Crustaceana 80, 247–251. doi: 10.1163/156854007780121465
Shakman E. A., Kinzelbach R. (2007). Distribution and characterization of lessepsian migrant fishes along the coast of Libya. Acta Ichthyologica. Piscatoria. 37, 7–15. doi: 10.3750/AIP2007.37.1.02
Singh C. L., Huggett M. J., Lavery P. S., Säwström C., Hyndes G. A. (2021). Kelp-associated microbes facilitate spatial subsidy in a detrital-based food web in a shoreline ecosystem. Front. Mar. Sci. 8. doi: 10.3389/fmars.2021.678222
Slim F. J., Hemminga M. A., Cocheret De La Morinière E., Van Der Velde G. (1996). Tidal exchange of macrolitter between a mangrove forest and adjacent seagrass beds (Gazi bay, Kenya). Aquatic Ecology 30, 119–128.
Smit A. J., Brearley A., Hyndes G. A., Lavery P. S., Walker D. I. (2005). Carbon and nitrogen stable isotope analysis of an Amphibolis griffithii seagrass bed. Estuarine. Coast. Shelf. Sci. 65, 545–556. doi: 10.1016/j.ecss.2005.07.002
Smit A. J., Brearley A., Hyndes G. A., Lavery P. S., Walker D. I. (2006). δ15N and δ13C analysis of a Posidonia sinuosa seagrass bed. Aquat. Bot. 84, 277–282. doi: 10.1016/j.aquabot.2005.11.005
Stapel J., Hemminga M. A. (1997). Nutrient resorption from seagrass leaves. Mar. Biol. 128, 197–206. doi: 10.1007/s002270050083
Stergiou K. I., Karpouzi V. S. (2002). Feeding habits and trophic levels of Mediterranean fish. Rev. Fish. Biol. Fish. 11, 217–254. doi: 10.1023/A:1020556722822
Strydom S., Murray K., Wilson S., Huntley B., Rule M., Heithaus M., et al. (2020). Too hot to handle: Unprecedented seagrass death driven by marine heatwave in a World Heritage Area. Global Change Biology 26, 3525–3538. doi: 10.1111/gcb.15065
Sturaro N., Caut S., Gobert S., Bouquegneau J. M., Lepoint G. (2010). Trophic diversity of idoteids (Crustacea, Isopoda) inhabiting the Posidonia oceanica litter. Marine Biology 157, 237–247. doi: 10.1007/s00227-009-1311-1
Taylor S. L., Bishop M. J., Kelaher B. P., Glasby T. M. (2010). Impacts of detritus from the invasive alga Caulerpa taxifolia on a soft sediment community. Mar. Ecol. Prog. Ser. 420, 73–81. doi: 10.3354/meps08903
Thibaut T., Blanfuné A., Boudouresque C. F., Holon F., Agel N., Descamps P., et al. (2022). Distribution of the seagrass Halophila stipulacea: A big jump to the northwestern Mediterranean Sea. Aquat. Bot. 176, 103465. doi: 10.1016/j.aquabot.2021.103465
Thiel M., Gutow L. (2005). The ecology of rafting in the marine environment. II. the rafting organisms and community. Oceanogr. Mar. Biol. 43, 279–418. doi: 10.1201/9781420037449.ch7
Thresher R. E., Nichols P. D., Gunn J. S., Bruce B. D., Furlani D. M. (1992). Seagrass detritus as the basis of a coastal planktonic food chain. Limnology and Oceanography 37, 1754–1758. doi: 10.4319/lo.1992.37.8.1754
Trevathan-Tackett S. M., Brodersen K. E., Macreadie P. I. (2020). Effects of elevated temperature on microbial breakdown of seagrass leaf and tea litter biomass. Biogeochemistry 151, 171–185. doi: 10.1007/s10533-020-00715-1
Valentine J. F., Heck K. L. Jr. (2021). Herbivory in seagrass meadows: an evolving paradigm. Estuaries. Coasts. 44, 491–505. doi: 10.1007/s12237-020-00849-3
Velimirov B. (1984). Grazing of Sarpa salpa l. @ on Posidonia oceanica and utilization of soluble compounds. Int. Workshop. Posidonia. Oceanica. Beds. 1, 381–387.
Velimirov B. (1987). Organic matter derived from a seagrass meadow: origin, properties, and quality of particles. Mar. Ecol. 8, 143–173. doi: 10.1111/j.1439-0485.1987.tb00180.x
Vergés A., Steinberg P. D., Hay M. E., Poore A. G. B., Campbell A. H., Ballesteros E., et al. (2014a). The tropicalization of temperate marine ecosystems: Climate-mediated changes in herbivory and community phase shifts. Proc. R. Soc. B.: Biol. Sci. 281, 20140846. doi: 10.1111/1365-2745.12324
Vergés A., Tomas F., Cebrian E., Ballesteros E., Kizilkaya Z., Dendrinos P., et al. (2014b). Tropical rabbitfish and the deforestation of a warming temperate sea. J. Ecol. 102, 1518–1527. doi: 10.1111/1365-2745.12324
Vetter E. W. (1995). Detritus-based patches of high secondary production in the nearshore benthos. Mar. Ecol. Prog. Ser. 120, 251–262. doi: 10.3354/meps120251
Vetter E. W. (1998). Population dynamics of a dense assemblage of marine detritivores. J. Exp. Mar. Biol. Ecol. 226, 131–161. doi: 10.1016/S0022-0981(97)00246-3
Vetter E. W., Dayton P. K. (1998). Macrofaunal communities within and adjacent to a detritus-rich submarine canyon system. Deep-Sea. Res. Part II.: Topical. Stud. Oceanogr. 45, 25–54.
Virnstein R. W., Hall L. M. (2009). Northern range extension of the seagrasses Halophila johnsonii and Halophila decipienis along the east coast of Florida, USA. Aquat. Bot. 90, 89–92. doi: 10.1016/j.aquabot.2008.05.007
Vizzini S., Mazzola A. (2008). The fate of organic matter sources in coastal environments: a comparison of three Mediterranean lagoons. hydrobiologia 611, 67–79. doi: 10.1007/s10750-008-9458-1
Vonk J. A., Christianen M. J. A., Stapel J. (2008a). Redefining the trophic importance of seagrasses for fauna in tropical indo-pacific meadows. Estuar. Coast. Shelf. Sci. 79, 653–660. doi: 10.1016/j.ecss.2008.06.002
Vonk J. A., Kneer D., Stapel J., Asmus H. (2008b). Shrimp burrow in tropical seagrass meadows: An important sink for litter. Estuar. Coast. Shelf. Sci. 79, 79–85. doi: 10.1016/j.ecss.2008.03.003
Vonk J. A., Stapel J. (2008). Regeneration of nitrogen (15N) from seagrass litter in tropical indo-pacific meadows. Mar. Ecol. Prog. Ser. 368, 165–175. doi: 10.3354/meps07619
Vonk J. A., Van Kuijk B. F., Van Beusekom M., Hunting E. R., Kraak M. H. S. (2016). The significance of linoleic acid in food sources for detritivorous benthic invertebrates. Sci. Rep. 6, 35785. doi: 10.1038/srep35785
Walker D. I., Pergent G., Fazi S. (2001). “Chapter 16: Seagrass decomposition,” in Global seagrass research methods. Eds. Short F. T., Coles R. G. (Amsterdam: Elsevier), 313–324.
Waycott M., Duarte C. M., Carruthers T. J.B., Orth R. J., Dennison W. C., Olyarnik S., et al. (2009). Accelerating loss of seagrasses across the globe threatens coastal ecosystems. Proceedings of the National Academy of Sciences of the United States of America 106, 12377–12381. doi: 10.1073/pnas.0905620106
Wernberg T., Bennett S., Babcock R. C., De Bettignies T., Cure K., Depczynski M., et al. (2016). Climate-driven regime shift of a temperate marine ecosystem. Science 353, 169–172. doi: 10.1126/science.aad8745
Wernberg T., Smale D. A., Tuya F., Thomsen M. S., Langlois T. J., De Bettignies T., et al. (2013). An extreme climatic event alters marine ecosystem structure in a global biodiversity hotspot. Nature Climate Change 3, 78–82. doi: 10.1038/nclimate1627
Wernberg T., Vanderklift M. A., How J., Lavery P. S. (2006). Export of detached macroalgae from reefs to adjacent seagrass beds. Oecologia 147, 692–701. doi: 10.1007/s00442-005-0318-7
Wesselmann M., Chefaoui R. M., Marbà N., Serrao E. A., Duarte C. M. (2021). Warming threatens to propel the expansion of the exotic seagrass Halophila stipulacea. Front. Mar. Sci. 8. doi: 10.3389/fmars.2021.759676
Winters G., Beer S., Willette D. A., Viana I. G., Chiquillo K. L., Beca-Carretero P., et al. (2020). The tropical seagrass Halophila stipulacea: Reviewing what we know from its native and invasive habitats, alongside identifying knowledge gaps. Front. Mar. Sci. 7, 1–28. doi: 10.3389/fmars.2020.00300
Wolff T. (1979). Macrofaunal utilization of plant remains in the deep sea. Sarsia 64, 117–136. doi: 10.1080/00364827.1979.10411373
Yang L. H., Bastow J. L., Spence K. O., Wright A. N. (2008). What can we learn from resource pulses. Ecology 89, 621–634. doi: 10.1890/07-0175.1
Zarco-Perello S., Carroll G., Vanderklift M., Holmes T., Langlois T. J., Wernberg T. (2020). Range-extending tropical herbivores increase diversity, intensity and extent of herbivory functions in temperate marine ecosystems. Funct. Ecol. 34, 2411–2421. doi: 10.1111/1365-2435.13662
Zarco-Perello S., Langlois T. J., Holmes T., Vanderklift M. A., Wernberg T. (2019). Overwintering tropical herbivores accelerate detritus production on temperate reefs. Proc. R. Soc. B.: Biol. Sci. 286, 20192046. doi: 10.1098/rspb.2019.2046
Zarco-Perello S., Wernberg T., Langlois T. J., Vanderklift M. A. (2017). Tropicalization strengthens consumer pressure on habitat-forming seaweeds. Sci. Rep. 7, 820. doi: 10.1038/s41598-017-00991-2
Keywords: seagrass, tropicalization, detritivore, food web, macroalgae, climate change, carbon cycle, detritus
Citation: Lepoint G and Hyndes GA (2022) Tropicalization of seagrass macrophytodetritus accumulations and associated food webs. Front. Mar. Sci. 9:943841. doi: 10.3389/fmars.2022.943841
Received: 14 May 2022; Accepted: 05 July 2022;
Published: 28 July 2022.
Edited by:
Zhijian Jiang, South China Sea Institute of Oceanology (CAS), ChinaReviewed by:
Kun-Seop Lee, Pusan National University, South KoreaDelian Huang, South China Sea Fisheries Research Institute (CAFS), China
Copyright © 2022 Lepoint and Hyndes. This is an open-access article distributed under the terms of the Creative Commons Attribution License (CC BY). The use, distribution or reproduction in other forums is permitted, provided the original author(s) and the copyright owner(s) are credited and that the original publication in this journal is cited, in accordance with accepted academic practice. No use, distribution or reproduction is permitted which does not comply with these terms.
*Correspondence: Gilles Lepoint, Ry5MZXBvaW50QHVsaWVnZS5iZQ==