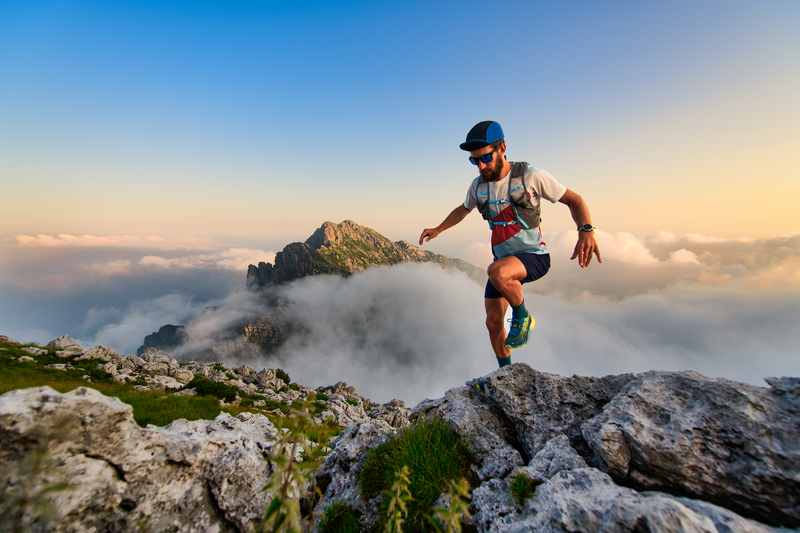
94% of researchers rate our articles as excellent or good
Learn more about the work of our research integrity team to safeguard the quality of each article we publish.
Find out more
PERSPECTIVE article
Front. Mar. Sci. , 25 July 2022
Sec. Marine Biogeochemistry
Volume 9 - 2022 | https://doi.org/10.3389/fmars.2022.936109
This article is part of the Research Topic Environmental Contaminants in Aquatic Systems and Chemical Safety for Environmental and Human Health, Volume II View all 11 articles
The effects of climate change (CC) on contaminants and their potential consequences to marine ecosystem services and human wellbeing are of paramount importance, as they pose overlapping risks. Here, we discuss how the interaction between CC and contaminants leads to poorly constrained impacts that affects the sensitivity of organisms to contamination leading to impaired ecosystem function, services and risk assessment evaluations. Climate drivers, such as ocean warming, ocean deoxygenation, changes in circulation, ocean acidification, and extreme events interact with trace metals, organic pollutants, excess nutrients, and radionuclides in a complex manner. Overall, the holistic consideration of the pollutants-climate change nexus has significant knowledge gaps, but will be important in understanding the fate, transport, speciation, bioavailability, toxicity, and inventories of contaminants. Greater focus on these uncertainties would facilitate improved predictions of future changes in the global biogeochemical cycling of contaminants and both human health and marine ecosystems.
The multiple environmental stressors associated with human activities are dramatically impacting ocean systems, particularly the functions and ecological services that they provide (Doney et al., 2012). The magnitude of the cumulative impacts of multiple concurrent environmental stressors has been higher in coastal ecosystems than offshore areas, with contamination being one of the most prominent pressures (Halpern et al., 2008). The input of contaminants to the environment is of global concern when these contaminants exhibit persistence, widespread distribution and accumulation in organisms and the environment, and threatens the resilience of the Earth System processes (Steffen et al., 2015).
While successful efforts have been made to reduce specific pollutants (e.g., Stockhom Convention and Minamata Convention) in the marine environment, increased contamination continues to cause degradation with negative impacts on food security, food safety, and marine biodiversity (UN, 2021). Moreover, high technology industries are increasing the amount and variety of chemicals in use. Major technologies for decarbonization are expected to increase the inputs of new contaminants into marine waters, including the technology-critical elements (TCEs), such as rare earth elements (REE), platinum group elements (PGE), substitutes for regulated organic compounds, and nanoparticles, with undefined toxicity and fate in the environment (Lodeiro et al., 2017; Hatje et al., 2018; Dang et al., 2021; Pell et al., 2021).
The ubiquitous presence of contaminants, such as trace metals, persistent organic pollutants, plastics, and excess nutrients, in the marine ecosystems raises challenges for achieving the Sustainable Development Goal target 14.1 (to prevent and significantly reduce marine pollution of all kinds) by 2025. The relevance of ocean pollution, relative to contaminant type and impacts in ecosystems, is rapidly changing not only as a function of the magnitude, transport, exposure pathways, and proximity to sources but also because of parallel climate change (CC). Contaminants, in particular plastics, and CC are connected in different ways. Plastic production, for instance, relies on fossil fuels and contributes to the emissions of global greenhouse gases (GHG) at each stage of their life cycle (Zheng and Suh, 2019). It is estimated that over 56 billion Mt of CO2e in GHG will be emitted between 2015 and 2050 due to plastic production (Hamilton et al., 2019).
Rising atmospheric CO2 is one of the most critical problems of CC (Figure 1) because its effects are globally pervasive, leading to increasing ocean temperatures which in turn alter ocean circulation, drive a reduction of oxygen concentrations, retreating sea ice, rising sea-level, and altered precipitation and runoff. The increase in atmospheric CO2, which results in a net transfer of CO2 to the ocean, has also already caused the seawater [H+] to increase (known as ‘ocean acidification’). Since the beginning of the industrial era, it is estimated that ocean acidification has caused a global mean reduction of ca. 0.1 pH units (i.e., an [H+] increase of ~1.6 nmol kg-1) in the surface ocean (Fassbender et al., 2021), and a further decrease in pH of 0.3 - 0.5 units by the year 2100 in the surface ocean is possible (IPCC, 2021), posing far reaching effects on marine life (Gehlen et al., 2014; Boyd et al., 2016).
Figure 1 Conceptual model of the main climate change drivers and contaminants interacting and potentially exacerbating negative impacts on coastal and ocean ecosystems.
Climate change drivers and pressures, specifically warming, stratification, acidification, deoxygenation, sea-level rise, extreme events (Figure 1) display interlinkages that lead to cumulative, antagonistic and synergic interactions, which can then alter the environmental fate, transport, chemical and physical speciation, availability, toxicity of contaminant, and pathways in marine food webs (Borga et al., 2012; Avendaño et al., 2016; Kibria et al., 2021). The interaction types differ among organisms from different climatic regions, and their variability is also dependent on the type and concentration of the contaminant (Jin et al., 2021). The interactions between CC and contaminants can exacerbate global pollution and must be considered in an integrated manner to properly assess the risk and vulnerability of ecosystem structure and functions, and also human well-being. Therefore, investigating the responses of individual contaminants (e.g., change in speciation, bioavailability, and transport) to single CC forcing factors, although essential, provides an incomplete story and highlights the need for comprehensive, multi-stressors analyses to predict the impacts of these changes on coastal and ocean ecosystems, therefore, important science, policy, and societal goals. These current knowledge gaps hamper the reliable analysis and modeling of risks, vulnerabilities and impacts, and the sound management of contaminants.
Ocean warming that has grown substantially since the 1970s (IPCC, 2021) impacts the ocean circulation and water column stratification, affecting nutrient and contaminant supply as well as the distribution, growth, and a range of physiological rates of many species, including phytoplankton. Net primary production by phytoplankton primes the biological carbon pump and plays a key role in supporting climate regulation services, besides provision services, such as fisheries. Ocean warming is expected to decrease the primary production and the negative impacts on animal biomass can be amplified at higher trophic levels (Lotze et al., 2019). One of the main reasons is the potential shift in the essential trace metals (e.g., manganese, iron, zinc, copper, and cobalt) distribution and bioavailability that has a significant biological role in marine primary production. Iron, for instance, is a micronutrient that supports many metabolic reactions necessary for phytoplankton and bacteria. Its availability controls species composition, trophic structure, and the sensitivity of net primary production to CC (Morel and Price, 2003; Hutchins and Boyd, 2016; Tagliabue et al., 2020). However, some essential trace metals such as Cu and Zn can also act as contaminants at high concentrations. Several studies have shown interactive effects of temperature increase and contaminants resulting, mostly, in enhanced bioaccumulation and toxicity of trace metals and organic contaminants (Baines et al., 2005; Mubiana and Blust, 2007; Delorenzo, 2015; Bates et al., 2021). The speciation of trace metals, which determines to a large extent the mobility, toxicity, and bioavailability (Tessier and Turner, 1996), is sensitive to CC drivers and responds to the environment physicochemical conditions and biotic interactions.
Additional CC drivers projected to worsen under ocean warming conditions and changes in upper-ocean stratification are associated with ocean oxygen loss and a subsequent expansion of the oxygen minimum zones (Stramma et al., 2008; Keeling et al., 2010). The increasing low oxygen conditions predicted by 2100 will drive substantial changes in water chemistry, as already can be seen in the Baltic Sea (Liblik and Lips, 2019; Limburg and Casini, 2019), Arabian Sea (Al Azhar et al., 2017), and other areas. In addition, changes are expected to ocean-climate feedbacks through the production of N2O (Schmidtko et al., 2017) and in the biological pump strength that has a critical role in the fate and transport of carbon and persistent organic pollutants (POPs) (Galbán-Malagón et al., 2012).
Changes in the carbonate chemistry and ocean acidification (OA) resulting from the increase in CO2 uptake by the ocean has a diverse effect on calcifying organisms, such as mollusks and pteropods, important prey groups for ecologically and economically important fish diets (Bednarsek et al., 2014). It also has negative impacts on foraminifera, corals, and phytoplankton (Doney et al., 2020), with the potential reduction in carbon export (Martin et al., 2020). Nitrogen fixation is expected to enhance by ~30%, whereas nitrification processes may be reduced by about the same factor (Wannicke et al., 2018) as a result of OA.
Thus, it affects nitrous oxide production, reducing the supply of oxidized nitrogen to the surface waters, creating an imbalance in the nitrogen cycle throughout the ocean (Beman et al., 2011). OA may also affect the production of other marine trace gases and result in further feedback to the atmosphere (Hopkins et al., 2020). Ocean acidification can have a significant impact on biogeochemical cycles and may alter the solubility, adsorption, bioavailability, toxicity, and rates of redox processes of metals in seawater (Millero et al., 2009; Gledhill et al., 2015; Stockdale et al., 2016). There is growing evidence that the combination of CC stressors and individual contaminants amplifies the negative effects produced in organisms (Nardi et al., 2017; Nardi et al., 2018; Freitas et al., 2019). On the other hand, an antagonistic effect of acidification, temperature increase, and Hg contamination has also been reported (Sampaio et al., 2018). However, well-designed studies assessing the combined impacts of a suite of contaminants and multiple CC drivers are rare and the effects of changing pH in the interactions of metals complexed to organic ligands (Avendaño et al., 2016; Zhu et al., 2021) with marine organisms (Leal et al., 2018; Romero-Freire et al., 2020) are still lacking, although extremely important for the ocean productivity.
CC drivers operate alongside with contaminants synergistically and thus contribute to environmental change at a global scale. Moreover, some regions, such as the Arctic, are known to be more vulnerable and are therefore changing more rapidly due to the multiple co-occurring changes in temperature, freshwater content, sea ice cover, nutrient concentrations, and pH (Wassmann et al., 2011; Stjern et al., 2019; Arrigo et al., 2020; AMAP, 2021b). In addition, to these CC drivers, the Arctic ecosystems also present a high vulnerability to radionuclides contamination due to nuclear testing in the 1950s with additional inputs from accidents, weapons tests and substantial amounts of radioactivity dumped at sea with the potential for corrosion/leakage of the containers (UNSCEAR, 2000; AMAP, 2015). Further, permafrost, ice sheet, sediments, and soils can potentially become new sources of plutonium and cesium-137 to the marine environment through remobilization of radioactivity (Macdonald et al., 2003). Persistent organic pollutants (POPs) and metals (Hg, Pb, and Cd) and microorganisms released from thawing permafrost are also overlapping problems in the Arctic, whose risks are underestimated (Miner et al., 2021). There is evidence that Hg and POPs removed from the atmosphere and deposited on snow have been released to the environment at snowmelt, rapidly dispersing hazardous compounds through the atmosphere, continental and aquatic system and becoming bioavailable to be incorporated into food webs (Ma et al., 2016; AMAP, 2021a). As the Arctic warms, CC drivers may exacerbate this process beyond the biological threshold, which amplifies the significance for the understanding of the emission rate, cycling, and trends of contaminants under global changes.
Coastal vegetated ecosystems such as seagrasses, mangroves, and tidal marshes, are important sinks for contaminants, but are also environments that are particularly sensitive to CC (Bindoff et al., 2019). In particular, these environments are exposed to extreme events, like hurricanes and heat waves, which will likely increase thermal stress in these systems, and storm surges that may modify the water cycle intensity and promote contaminants remobilization from soils. It is predicted a mean global sea-level rise by 2100 of up to 1 m under a very high, but not unrealistic greenhouse emissions scenario (IPCC, 2021), resulting in higher susceptibility of small island states and coastal ecosystems to erosion and flooding. Global riverine contaminant inputs are likely to increase due to more intense and frequent precipitation and storm surges. For instance, Hg concentrations can increase up to six-fold in coastal areas following scenarios projecting up to 30 percent increased terrestrial runoff (Jonsson et al., 2017).
The understanding of the combined contaminants’ response to CC drivers and how to forecast them will help policy makers to decide, for instance, whether the consumption of fish and shellfish is safe, or if areas where restoration and protection of coastal vegetated ecosystems must be prioritized to avoid the exposure of coastal communities to contaminants. For now, the scarcity of global pollution data, poor understanding of the effects and especially cumulative pressures of CC on multiple contaminants, as well as limited availability of global biogeochemical models are undermining projections and hampering sound pollution management. It will be necessary to increase our knowledge from laboratory, ecosystem-based field and process studies, as well as modelling, to have an overarching international action to facilitate and foster broad bidirectional science-policy interactions. The synergistic effects of various CC drivers are still mostly unexplored and demand urgent research studies (Cabral et al., 2019; Arrigo et al., 2020; Jin et al., 2021; Kibria et al., 2021).
We call on the international community to draw their immediate attention on these knowledge gaps and recommend to address appropriate research questions to ensure a systematic understanding of the effects of the complex interplay between contaminants and CC drivers on marine ecosystems. This knowledge will contribute to informed decision-making, following the Sustainable Development Goals (SGD) during the United Nations Decade of the Ocean Science.
There have been reviews of the impacts of CC on marine contaminants (Cabral et al., 2019; Kibria et al., 2021). Nevertheless, the available knowledge is limited to mostly laboratory studies that tested the effects of a single CC driver by one or more contaminants. The limited data on multiple concurrent CC drivers and their interaction with contaminants jeopardizes the construction of more generic patterns and models for predicting changes in biogeochemical cycles and their impacts on marine ecosystems. After reviewing the literature, we identified that the major knowledge gaps are:
1. The patchiness of the data on the spatial distribution of contaminants (nutrients, metals, radionuclides, and organic compounds), temporal trends, and associated uncertainties for the coastal and open ocean, especially in the southern hemisphere, has prevented investigators from reaching solid conclusions and assessments of exposure scenarios driving impacts to ecosystem integrity.
2. The complex mixture of contaminants in marine environments, coupled with the fact that even at low concentrations those contaminants can be toxic, poses the need to develop analytical capabilities on a global level. This has also to consider the additional challenges associated with the increasing number of emerging contaminants (e.g., REE, PGE, pharmaceuticals, personal care products) entering the environment (Pedreira et al., 2018; Pichler and Koopmann, 2019; Pell et al., 2021; Borgå et al., 2022).
3. The need to develop well-designed laboratory and field experiments to test the interactions and synergies between multiple contaminants (e.g., changes in chemical speciation, abiotic and biotic removal processes, pathways in food web) and combined CC drivers on various organizational levels (individual to ecosystem). Climate change-contaminants sensitivity and vulnerability analyses are needed.
4. State-of-the-art models that integrate both CC and contaminants are needed to predict changes in the distribution, fate, and transport of contaminants in response to the CC scenario and forecast interactions between contaminants and humans.
5. Innovative, cross-border solutions to prevent the input of contaminants to marine ecosystems and mitigate their combined impacts associated with CC.
To address the backdrop of the knowledge gaps, we call for a coordinated effort to assess the interaction between and impacts of CC on contaminants in marine environments. This endeavor should have an inclusive scope and promote field studies, including the definition of baseline levels in areas that haven’t yet been explored, monitoring studies to evaluate long term trends (gaps 1 and 2), and process studies in specific regions to understand and predict the consequences of interactive effects between contaminants and CC in the chemistry and ecology of coastal and ocean systems (gap 3). CC-induced contaminant sensitivity/vulnerability in terms of persistence, bioaccumulation potential, and toxicity of organic and inorganic contaminants must be evaluated and modeled to predict and minimize future risks for humans and ecosystems. Such an effort will require support and building of capacity to deliver the necessary geographic scope (gap 1). Although our objective here is not to identify all possible research questions that need to be addressed, some points deserve attention. Land-based sources and hotspot reservoirs of contaminants (e.g., mangroves in the tropics, and Arctic ecosystems) in combination with regionally specific hydroclimate projections will determine plausible trajectories of marine pollution over the coming decades and need to be investigated. Expected increases in river flows will make rivers priority sources of contaminants (chemicals and plastics). Enhanced river fluxes (and flash-flood events) and sea-level rise will also promote the remobilization of litter and contaminants accumulated over time. Subsistence communities across low-lying coastal areas and specifically Small Island Developing States (SIDS) are particularly vulnerable. The impact of multiple CC stressors and contaminants will affect biodiversity, ecosystem resilience, and shellfish/fish industries due to changes in the speciation, toxicity, and bioavailability of contaminants. We still don’t know which groups of contaminants are more likely to be most affected and become more toxic and deleterious for the marine food web and human health. Modeling exercises and combined model-data syntheses (gap 4) will help to address these critical issues providing a framework for quantifying the net CC and pollutant impacts on marine systems and to identify priority areas and strategies to minimize pollution impacts (gap 5) to maintain sustainable uses of the ocean. This approach could also help to prioritize contaminant classes that needs urgent attention, regional patterns, or effect trends (Persson et al., 2022).
Scientists are confident predicting that climate change is going to intensify and exacerbate extreme events (IPCC, 2021). Some of these changes are already happening, as seen in the unprecedented number and magnitude of extreme events of the last decade. Extreme events, such as floods, can promote the transport and translocation of chemical contaminants (Horowitz et al., 2014) and plastics (Ford et al., 2022) to large areas, exposing organisms to high concentrations of contaminants for an extended period (Barber et al., 2017; Izaditame et al., 2022), potentially causing more ecological adverse effects and health risks through various exposure routes, including bioaccumulation in the food web (Och et al., 2014; Crawford et al., 2022).
A general failure to achieve the integrated knowledge and management of human pressures on marine systems is increasing risks to the benefits that people draw from the ocean in terms of food security, material resources, human health and well-being, coastal safety, and the maintenance of key ecosystem functions. The scarce information on species and ecosystem-level threshold, tolerances, and tipping points for various CC drivers mean that predictions of risk, vulnerability, and responses are difficult to make, and confidence is low. This highlights the urgency for a better understanding of the synergies between contaminants and CC and the challenges to develop effective remediation and conservation of coastal and ocean ecosystems. This will only be achieved through fostering a different frame of interdisciplinary research including improved socio-ecological models and integrated ecosystem assessments, together with better integration of stakeholders (Holsman et al., 2017). A key next step will be to compile global databases of empirical measurements and modeling information on the effects of CC on contaminants for better informed predictions of future impacts, to support ecosystem-based planning decisions, to identify where pressing mitigation efforts are most needed, to plan proactive and more preventive management practices, and to monitor progress towards sustainable management actions. Aware of this, the Join Group of Experts on the Scientific Aspects of Marine Environmental Protection (GESAMP) Working Group 45 (http://www.gesamp.org/work/groups/wg-44-ghg-impacts-on-contaminants-in-the-ocean) in a joint effort is systematically reviewing existing literature on the effects of changes in ocean physics and chemistry on the speciation, cycling, fate, transport, and bioavailability of trace metals, organic pollutants, radionuclides, and nutrients to identify knowledge gaps, make recommendations, and planning for future research directions. The understanding of CC drivers and contaminants interactions depends on the collaboration of the scientific community and other stakeholders to produce sound information to subsidize the protection of human health, marine ecosystems services, and functions.
The original contributions presented in the study are included in the article/supplementary material. Further inquiries can be directed to the corresponding author.
The production of the manuscript was coordinated by VH, who also prepared the first draft. All authors contributed to the content, structure and framing of the article. All authors contributed to the article and approved the submitted version.
All authors are part of the Working Group of Experts on the Scientific Aspects of Marine Environmental Protection (GESAMP WG45 - Climate Change and Greenhouse Gas Related Impacts on Contaminants in the Ocean), supported by the International Atomic Energy Agency (IAEA), the United Nations Environmental Program (UNEP), International Oceanographic Commission (IOC-UNESCO), the World Meteorological Organization (WMO), and the International Maritime Organization (IMO).
The authors declare that the research was conducted in the absence of any commercial or financial relationships that could be construed as a potential conflict of interest.
All claims expressed in this article are solely those of the authors and do not necessarily represent those of their affiliated organizations, or those of the publisher, the editors and the reviewers. Any product that may be evaluated in this article, or claim that may be made by its manufacturer, is not guaranteed or endorsed by the publisher.
Al Azhar M., Lachkar Z., Lévy M., Smith S. (2017). Oxygen minimum zone contrasts between the Arabian Sea and the bay of Bengal implied by differences in remineraliz ation depth. Geophys. Res. Lett. 44, 106–114. doi: 10.1002/2017GL075157
AMAP (2015). Radioactivity in the arctic. Oslo, Norway: Arctic monitoring and assessment programme (AMAP) 89 pp. doi: 10.3402/ijch.v75.33949
AMAP (2021a). AMAP assessment 2021: Mercury in the arctic. Arctic monitoring and assessment programme (Tromsø: Norway). 324 pp. Available at: https://www.amap.no/documents/doc/amap-assessment-2021-mercury-in-the-arctic/3581
AMAP (2021b) POPs and chemicals of emerging Arctic concern: influence of climate change. Summary for policy-makers. Arctic monitoring and assessment programme (AMAP), (Tromsø, Norway) 16 pp Available at: https://www.amap.no/documents/doc/pops-and-chemicals-of-emerging-arctic-concern-influence-of-climate-change.-summary-for-policy-makers/3511.
Arrigo K. R., van Dijken G. L., Cameron M. A., van der Grient J., Wedding L. M., Hazen L., et al. (2020). Synergistic interactions among growing stressors increase risk to an Arctic ecosystem. Nat. Commun. 11, 1–8. doi: 10.1038/s41467-020-19899-z
Avendaño L., Gledhill M., Achterberg E. P., Rérolle V. M. C., Schlosser C. (2016). Influence of ocean acidification on the organic complexation of iron and copper in Northwest European shelf seas; a combined observational and model study. Front. Mar. Sci. 3. doi: 10.3389/fmars.2016.00058
Baines S. B., Fisher N. S., Kinney E. L. (2005). Influence of temperature on dietary metal uptake in Arctic and temperate mussels. Mar. Ecol. Prog. Ser. 289, 201–213. doi: 10.3354/meps289201
Barber L., Paschke S., Battaglin W., Douville C., Fitzgerald K., Keefe S., et al. (2017). Effects of an extreme flood on trace elements in river water from urban stream to major river basin. Environ. Sci. Technol. 51, 10344–10356. doi: 10.1021/acs.est.7b01767
Bates E. H., Alma L., Ugrai T., Gagnon A., Maher M., McElhany P., et al. (2021). Evaluation of the effect of local water chemistry on trace metal accumulation in puget sound shellfish shows that concentration varies with species, size, and location. Front. Mar. Sci. 8. doi: 10.3389/fmars.2021.636170
Bednarsek N., Feeley R., Reum J., Peterson B., Menkel J., Alin S., et al. (2014). Limacina helicina shell dissolution as an indicator of declining habitat suitability owing to ocean acidification in the California current ecosystem. Proc. R. Soc B Biol. Sci. 281, 20140123. doi: 10.1098/rspb.2014.0123
Beman J. M., Chow C. E., King A. L., Feng Y., Fuhrman J. A., Andersson A., et al. (2011). Global declines in oceanic nitrification rates as a consequence of ocean acidification. Proc. Natl. Acad. Sci. U. S. A. 108, 208–213. doi: 10.1073/pnas.1011053108
Bindoff N. L., Cheung W. W. L., Kairo J. G., Ariístegui J., Guinder V., Hallberg R., et al. (2019). “Changing ocean, marine ecosystems, and dependent communities,” in IPCC, Geneva special report on the ocean and cryosphere in a changing climate. Eds. Pörtner N. M. W. H.-O., Roberts D. C., Masson-Delmotte V., Zhai P., Tignor M., Poloczanska E., Mintenbeck K., Alegría A., Nicolai M., Okem A., Petzold J., Rama B..
Borga K., Kidd K. A., Muir D. C., Berglund O., Conder J. M., Gobas F. A., et al. (2012). Trophic magnification factors: considerations of ecology, ecosystems, and study design. Integr. Environ. Assess. Manage. 8, 64–84. doi: 10.1002/ieam.24
Borgå K., McKinney M. A., Routti H., Fernie K. J., Giebichenstein J., Hallanger I., et al. (2022). The influence of global climate change on accumulation and toxicity of persistent organic pollutants and chemicals of emerging concern in Arctic food webs. Environ. Sci. Process. Impacts. doi: 10.1039/d1em00469g
Boyd P. W., Cornwall C. E., Davison A., Doney S. C., Fourquez M., Hurd C. L., et al. (2016). Biological responses to environmental heterogeneity under future ocean conditions. Glob. Change Biol. 22, 2633–2650. doi: 10.1111/gcb.13287
Cabral H., Fonseca V., Sousa T., Leal M. C. (2019). Synergistic effects of climate change and marine pollution: An overlooked interaction in coastal and estuarine areas. Int. J. Environ. Res. Public Health 16, 1–17. doi: 10.3390/ijerph16152737
Crawford S. E., Brinkmann M., Ouellet J. D., Lehmkuhl F., Reicherter K., Schwarzbauer J., et al. (2022). Remobilization of pollutants during extreme flood events poses severe risks to human and environmental health. J. Hazard. Mater. 421, 126691. doi: 10.1016/j.jhazmat.2021.126691
Dang D. H., Filella M., Omanović D. (2021). Technology-critical elements: An emerging and vital resource that requires more in-depth investigation. Arch. Environ. Contam. Toxicol. 81, 517–520. doi: 10.1007/s00244-021-00892-6
Delorenzo M. E. (2015). Impacts of climate change on the ecotoxicology of chemical contaminants in estuarine organisms. Curr. Zool. 61, 641–652. doi: 10.1093/czoolo/61.4.641
Doney S. C., Busch D. S., Cooley S. R., Kroeker K. J. (2020). The impacts of ocean acidification on marine ecosystems and reliant human communities. Annu. Rev. Environ. Resour. 45, 83–112. doi: 10.1146/annurev-environ-012320-083019
Doney S. C., Ruckelshaus M., Emmett Duffy J., Barry J. P., Chan F., English C. A., et al. (2012). Climate change impacts on marine ecosystems. Ann. Rev. Mar. Sci. 4, 11–37. doi: 10.1146/annurev-marine-041911-111611
Fassbender A., Orr J., Dickson A. (2021). Technical note: Interpreting pH changes. Biogeosciences 18, 1407–1415. doi: 10.5194/bg-18-1407-2021
Ford H. V., Jones N. H., Davies A. J., Godley B. J., Jambeck J. R., Napper I. E., et al. (2022). The fundamental links between climate change and marine plastic pollution. Sci. Total Environ. 806, 150392. doi: 10.1016/j.scitotenv.2021.150392
Freitas R., Leite C., Pinto J., Costa M., Monteiro R., Henriques B., et al. (2019). The influence of temperature and salinity on the impacts of lead in mytilus galloprovincialis. Chemosphere 235, 403–412. doi: 10.1016/j.chemosphere.2019.05.221
Galbán-Malagón C., Berrojalbiz N., Ojeda M. J., Dachs J. (2012). The oceanic biological pump modulates the atmospheric transport of persistent organic pollutants to the Arctic. Nat. Commun. 3, 862. doi: 10.1038/ncomms1858
Gehlen M., Séférian R., Jones D. O. B., Roy T., Roth R., Barry J., et al. (2014). Projected pH reductions by 2100 might put deep North Atlantic biodiversity at risk. Biogeosciences 11, 6955–6967. doi: 10.5194/bg-11-6955-2014
Gledhill M., Achterberg E. P., Li K., Mohamed K. N., Rijkenberg M. J. A. (2015). Influence of ocean acidification on the complexation of iron and copper by organic ligands in estuarine waters. Mar. Chem. 177, 421–433. doi: 10.1016/j.marchem.2015.03.016
Halpern B., Wallbridge S., Selkoe K., Kappel C., Micheli F., D’Agrossa C., et al. (2008). A global map of human impact on marine ecosystems. Sci. (80-. ). 321, 948–952. doi: 10.1126/science.1157390
Hamilton L. A., Feit S., Muffett C., Kelso M. (2019). Plastic & climate: The hidden costs of a plastic planet. Center for International Environmental Law, Washington
Hatje V., Lamborg C. H., Boyle E. A. (2018). Trace-metal contaminants: Human footprint on the ocean. Elements 14, 403–408. doi: 10.2138/gselements.14.6.403
Holsman K., Samhouri J., Cook G., Hazen E., Olsen E., Dillard M., et al. (2017). An ecosystem-based approach to marine risk assessment. Ecosyst. Heal. Sustain. 3, e01256. doi: 10.1002/ehs2.1256
Hopkins F. E., Suntharalingam P., Gehlen M., Andrews O. D., Archer S., Bopp L., et al. (2020). The impacts of ocean acidification on marine trace gases and the implications for atmospheric chemistry and climate frances. Proc. R Soc. A 476, 20190769. doi; 10.1098/rspa.2019.0769
Horowitz A. J., Elrick K. A., Smith J. J., Stephens V. C. (2014). The effects of hurricane irene and tropical storm lee on the bed sediment geochemistry of U.S. Atlantic coastal rivers. Hydrol. Process. 28, 1250–1259. doi: 10.1002/hyp.9635
Hutchins D. A., Boyd P. W. (2016). Marine phytoplankton and the changing ocean iron cycle. Nat. Clim. Change 6, 1072–1079. doi: 10.1038/nclimate3147
IPCC (2021). Summary for policymakers. in Climate change 2021: the physical science basis. Contribution of working group I to the sixth assessment report of the intergovernmental panel on climate change Eds. Masson-Delmont V., Zhai P., Pirani A., Connors S., Péan C., Berger S., et al (Cambridge University Press). pp. 3–32. doi: 10.1017/9781009157896.001.
Izaditame F., Siebecker M. G., Sparks D. L. (2022). Sea-Level-rise-induced flooding drives arsenic release from coastal sediments. J. Hazard. Mater. 423, 127161. doi: 10.1016/j.jhazmat.2021.127161
Jin P., Zhang J., Wan J., Overmans S., Gao G., Ye M., et al. (2021). The combined effects of ocean acidification and heavy metals on marine organisms: A meta-analysis. Front. Mar. Sci. 8. doi: 10.3389/fmars.2021.801889
Jonsson S., Andersson A., Nilsson M. B., Skyllberg U., Lundberg E., Schaefer J. K., et al. (2017). Terrestrial discharges mediate trophic shifts and enhance methylmercury accumulation in estuarine biota. Sci. Adv. 3, 1–10. doi: 10.1126/sciadv.1601239
Keeling R. F., Körtzinger A., Gruber N. (2010). Ocean deoxygenation in a warming world. Ann. Rev. Mar. Sci. 2, 199–229. doi: 10.1146/annurev.marine.010908.163855
Kibria G., Nugegoda D., Rose G., Haroon A. K. Y. (2021). Climate change impacts on pollutants mobilization and interactive effects of climate change and pollutants on toxicity and bioaccumulation of pollutants in estuarine and marine biota and linkage to seafood security. Mar. Pollut. Bull. 167, 112364. doi: 10.1016/j.marpolbul.2021.112364
Leal P. P., Hurd C. L., Sander S. G., Armstrong E., Fernández P. A., Suhrhoff T. J., et al. (2018). Copper pollution exacerbates the effects of ocean acidification and warming on kelp microscopic early life stages. Sci. Rep. 8, 1–13. doi: 10.1038/s41598-018-32899-w
Liblik T., Lips U. (2019). Stratification has strengthened in the baltic sea – an analysis of 35 years of observational data. Front. Earth Sci. 7. doi: 10.3389/feart.2019.00174
Limburg K. E., Casini M. (2019). Otolith chemistry indicates recent worsened Baltic cod condition is linked to hypoxia exposure. Biol. Lett. 15, 20190352. doi: 10.1098/rsbl.2019.0352
Lodeiro P., Browning T. J., Achterberg E. P., Guillou A., El-Shahawi M. S. (2017). Mechanisms of silver nanoparticle toxicity to the coastal marine diatom chaetoceros curvisetus. Sci. Rep. 7, 1–10. doi: 10.1038/s41598-017-11402-x
Lotze H. K., Tittensor D. P., Bryndum-Buchholz A., Eddy T. D., Cheung W. W. L., Galbraith E. D., et al. (2019). Global ensemble projections reveal trophic amplification of ocean biomass declines with climate change. Proc. Natl. Acad. Sci. U. S. A. 116, 12907–12912. doi: 10.1073/pnas.1900194116
Macdonald R. W., Harner T., Fyfe J., Loeng H., Weingartner T. (2003). AMAP assessment 2002: The influence of global change on contaminant pathways to, within, and from the Arctic (Oslo: Arctic Monitoring and Assessment Programme (AMAP). Arctic.
Ma J., Hung H., Macdonald R. W. (2016). The influence of global climate change on the environmental fate of persistent organic pollutants: A review with emphasis on the northern hemisphere and the Arctic as a receptor. Glob. Planet. Change 146, 89–108. doi: 10.1016/j.gloplacha.2016.09.011
Martin A., Boyd P., Buesseler K., Cetinic I., Claustre H., Giering S., et al. (2020). Study the twilight zone before it is too late. Nature 580, 26–28. doi: 10.1038/d41586-020-00915-7
Millero F., Woosley R., DiTrolio B., Waters J. (2009). Effect of ocean acidification on the speciation of metals in seawater. Oceanography 22, 72–85. doi: 10.5670/oceanog.2009.98
Miner K. R., D’Andrilli J., Mackelprang R., Edwards A., Malaska M. J., Waldrop M. P., et al. (2021). Emergent biogeochemical risks from Arctic permafrost degradation. Nat. Clim. Change 11, 809–819. doi: 10.1038/s41558-021-01162-y
Morel F. M. M., Price N. M. (2003). The biogeochemical cycles of trace metals in the oceans. Science 300, 944–947. doi: 10.1126/science.1083545
Mubiana V. K., Blust R. (2007). Effects of temperature on scope for growth and accumulation of cd, Co, Cu and Pb by the marine bivalve mytilus edulis. Mar. Environ. Res. 63, 219–235. doi: 10.1016/j.marenvres.2006.08.005
Nardi A., Benedetti M., D’Errico G., Fattorini D., Regoli F. (2018). Effects of ocean warming and acidification on accumulation and cellular responsiveness to cadmium in mussels mytilus galloprovincialis: importance of the seasonal status. Aquat Toxicol. 204, 171–179. doi: 10.1016/j.aquatox.2018.09.009
Nardi A., Mincarelli L. F., Benedetti M., Fattorini D., d’Errico G., Regoli F. (2017). Indirect effects of climate changes on cadmium bioavailability and biological effects in the Mediterranean mussel mytilus galloprovincialis. Chemosphere 169, 493–502. doi: 10.1016/j.chemosphere.2016.11.093
Och L. M., Müller B., Wichser A., Ulrich A., Vologina E. G., Sturm M. (2014). Rare earth elements in the sediments of lake baikal. Chem. Geol. 376, 61–75. doi: 10.1016/j.chemgeo.2014.03.018
Pedreira R. M. A., Pahnke K., Böning P., Hatje V. (2018). Tracking hospital effluent-derived gadolinium in Atlantic coastal waters off Brazil. Water Res. 145, 62–72. doi: 10.1016/j.watres.2018.08.005
Pell R., Tijsseling L., Goodenough K., Wall F., Dehaine Q., Grant A., et al. (2021). Towards sustainable extraction of technology materials through integrated approaches. Nat. Rev. Earth Environ. 2, 665–679. doi: 10.1038/s43017-021-00211-6
Persson L., Almroth B., Collins C., Cornell S., de Wit C., Diamond M., et al. (2022). Outside the safe operating space of the planetary boundary for novel entities. Env. Sci. Technol 56 (3), 1510–21. doi: 10.1021/acs.est.1c04158
Pichler T., Koopmann S. (2019). Should monitoring of molybdenum (Mo) in groundwater, drinking water and well permitting made mandatory? Environ. Sci. Technol. 54 (1), 1–2. doi: 10.1021/acs.est.9b06869
Romero-Freire A., Lassoued J., Silva E., Calvo S., Pérez F. F., Bejaoui N., et al. (2020). Trace metal accumulation in the commercial mussel m. galloprovincialis under future climate change scenarios. Mar. Chem. 224, 103840. doi: 10.1016/j.marchem.2020.103840
Sampaio E., Lopes A. R., Francisco S., Paula J. R., Pimentel M., Maulvault A. L., et al. (2018). Ocean acidification dampens physiological stress response to warming and contamination in a commercially-important fish (Argyrosomus regius). Sci. Total Environ. 618, 388–398. doi: 10.1016/j.scitotenv.2017.11.059
Schmidtko S., Stramma L., Visbeck M. (2017). Decline in global oceanic oxygen content during the past five decades. Nature 542, 335–339. doi: 10.1038/nature21399
Steffen W., Richardson K., Rockström J., Cornell S. E., Fetzer I., Bennett E. M., et al. (2015). Planetary boundaries: Guiding human development on a changing planet. Sci. (80-. ). 347 , 1259855. doi: 10.1126/science.1259855
Stjern C., Lund M., Samset B., Myhre G., Forster P., Andrews T., et al. (2019). Arctic Amplification response to individual climate drivers. J. Geophysical Res. Atmos. 124, 6698–6717. doi: 10.1029/2018JD029726
Stockdale A., Tipping E., Lofts S., Mortimer R. J. G. (2016). The effect of ocean acidification on organic and inorganic speciation of trace metals Environ. Sci. Technol. 50 (4), 1906–13. doi: 10.1021/acs.est.5b05624
Stramma L., Johnson G., Sprintall J., Mohrholz V. (2008). Expanding oxygen-minimum zones in the tropical oceans. Sci. (80-. ) 320, 655–658. doi: 10.1126/science.1153847
Tagliabue A., Barrier N., Du Pontavice H., Kwiatkowski L., Aumont O., Bopp L., et al. (2020). An iron cycle cascade governs the response of equatorial pacific ecosystems to climate change. Glob. Change Biol. 26, 6168–6179. doi: 10.1111/gcb.15316
UNSCEAR (2000). “Sources and effects of ionizing radiation,” in The united nations scientific committee on the effects of atomic radiation (UNSCEAR) Report to the general assembly. (United Nations: New York). Available at: https://www.unscear.org/docs/publications/2000/UNSCEAR_2000_Report_Vol.I.pdf
Wannicke N., Frey C., Law C. S., Voss M. (2018). The response of the marine nitrogen cycle to ocean acidification. Glob. Change Biol. 24, 5031–5043. doi: 10.1111/gcb.14424
Wassmann P., Duarte C. M., Agustí S., Sejr M. K. (2011). Footprints of climate change in the Arctic marine ecosystem. Glob. Change Biol. 17, 1235–1249. doi: 10.1111/j.1365-2486.2010.02311.x
Zheng J., Suh S. (2019). Strategies to reduce the global carbon footprint of plastics. Nat. Clim. Change 9, 374–378. doi: 10.1038/s41558-019-0459-z
Keywords: pollutants, impacts, knowledge gaps, ecosystem impacts, health impacts, climate change, ocean change, contaminants
Citation: Hatje V, Sarin M, Sander SG, Omanović D, Ramachandran P, Völker C, Barra RO and Tagliabue A (2022) Emergent interactive effects of climate change and contaminants in coastal and ocean ecosystems. Front. Mar. Sci. 9:936109. doi: 10.3389/fmars.2022.936109
Received: 04 May 2022; Accepted: 27 June 2022;
Published: 25 July 2022.
Edited by:
Antonio Cobelo-Garcia, Spanish National Research Council (CSIC), SpainReviewed by:
Monica F. Costa, Federal University of Pernambuco, BrazilCopyright © 2022 Hatje, Sarin, Sander, Omanović, Ramachandran, Völker, Barra and Tagliabue. This is an open-access article distributed under the terms of the Creative Commons Attribution License (CC BY). The use, distribution or reproduction in other forums is permitted, provided the original author(s) and the copyright owner(s) are credited and that the original publication in this journal is cited, in accordance with accepted academic practice. No use, distribution or reproduction is permitted which does not comply with these terms.
*Correspondence: Vanessa Hatje, dmhhdGplQHVmYmEuYnI=
Disclaimer: All claims expressed in this article are solely those of the authors and do not necessarily represent those of their affiliated organizations, or those of the publisher, the editors and the reviewers. Any product that may be evaluated in this article or claim that may be made by its manufacturer is not guaranteed or endorsed by the publisher.
Research integrity at Frontiers
Learn more about the work of our research integrity team to safeguard the quality of each article we publish.