- 1Marine Ecology Research Division, GEOMAR Helmholtz Centre for Ocean Research Kiel, Kiel, Germany
- 2Ocean Circulation and Climate Dynamics Research Division, GEOMAR Helmholtz Centre for Ocean Research Kiel, Kiel, Germany
- 3School of Ocean and Earth Science, National Oceanography Centre Southampton, University of Southampton, Southampton, United Kingdom
Salinity is a major environmental factor shaping the distribution and abundance of marine organisms. Climate change is predicted to alter salinity in many coastal regions due to sea level rise, evaporation, and changes in freshwater input. This exerts significant physiological stress on coastal invertebrates whose body fluid osmolality follows that of seawater (‘osmoconformers’). In this study, we conducted a systematic review and meta-analysis of osmolytes (both organic and inorganic) utilized by osmoconforming marine invertebrates during a >14-day acclimation to reduced salinity. Of the 2,389 studies screened, a total of 56 fulfilled the search criteria. Thirty-eight studies reported tissue osmolyte. Following acclimation to reduced salinity, tissue concentrations of six organic compounds and sodium were consistently reduced across phyla. This suggests that intracellular inorganic ions are not only utilized as a rapid response system during acute exposure to low salinity stress but also, in concert with reductions in organic osmolyte concentrations, during longer-term acclimation. Our systematic review demonstrates that only a few studies (n = 13) have quantified salinity-induced long-term changes in intracellular ion concentrations. In addition, no study has compiled a complete intracellular osmolyte budget. Alanine, betaine, glycine, and taurine are the major organic osmolytes that are universally employed across five phyla. The characterization of organic osmolytes was heavily weighted towards free amino acids (FAAs) and derivatives—neglecting methylamines and methylsulfonium compounds, which can be as important as FAAs in modulating intracellular osmolality. As a consequence, we suggest best-practice guidelines to streamline experimental designs and protocols in osmoregulation research in order to better understand the conserved mechanisms that define the limits of salinity acclimation in marine invertebrates. To our best knowledge, this is the first systematic review and meta-analysis on osmolyte concentrations in osmoconformers acclimated to low salinity. It creates a valuable baseline for future research and reveals large research gaps. Our meta-analysis suggests that there are common osmolyte actors employed across phyla but no uniform concept since osmolyte pool composition and proportions were taxon-specific. In light of future salinity changes and their potential consequences, it becomes more important to understand salinity tolerance capacities and limits.
1 Introduction
Salinity is a major abiotic factor in the aquatic environment, with short-term fluctuations causing severe physiological stress in organisms (Braby and Somero, 2006). An increasing number of studies predict long-term changes in salinity caused by the ongoing climate change in coastal regions of the world, with potentially severe effects on ecosystems (Trenberth, 2011; IPCC, 2014). This includes increasing and decreasing trends. Generally, higher latitudes are predicted to receive an increased freshwater input via precipitation, riverine discharge, surface runoff, or meltwater (Bintanja and Selten, 2014; Moon et al., 2015; Vuorinen et al., 2015). On the other hand, subtropical or some estuarine regions (such as the Chesapeake Bay area) are estimated to experience an increase in salinity due to lower precipitation rates, or sea-level rise and a subsequent seawater influx into previously brackish areas (Hong and Shen, 2012; Du et al., 2019). Desalination can be as harmful to organisms as ocean warming or acidification, but has received little research attention up until now. In the Baltic Sea, widespread desalination by 1.5 is predicted by 2100. This would potentially render an area of >100.000 km2 inhabitable for many marine species and would shift the biome westward (Gräwe et al., 2013; Podbielski et al., 2016). Shallow, coastal reefs in Australia are more likely to experience prolonged low salinity events in the future (Haapkylä et al., 2011; Scott et al., 2013) and Antarctic shelf systems are predicted to receive an increased input of meltwater and atmospheric precipitation, causing salinity reductions by up to 0.5–2 (Meredith et al., 2008; Naughten et al., 2018). Therefore, the ability of resident species to acclimate or adapt to long-term decreases in salinity is crucial for their persistence and proliferation in the impacted areas.
Aquatic organisms employ different strategies to cope with changing salinity. Some species can avoid low salinity habitats by vertical or horizontal migration to higher saline regions. However, many benthic invertebrates have a lower degree of mobility and are thus more susceptible to local salinity changes. Some species then temporarily isolate themselves from the environment during hyposaline episodes through shell closure or encystment, a state that cannot be maintained permanently. Hence, only through physiological acclimation and or adaptation to changing salinities can fitness be maintained at a high level.
There are two physiological strategies adopted by aquatic invertebrates to adjust to salinity changes in their environment. Osmoregulators are species that can maintain the osmolality of their extracellular fluids at relatively stable levels when exposed to salinity stress, primarily by means of active ion transport (e.g., the crustacean Carcinus maenas or the annelid Marphysa gravelyi) (Krishnamoorthi and Krishnaswamy, 1965; Darling et al., 2008; Rivera-Ingraham and Lignot, 2017). The extracellular fluids of osmoconformers, on the other hand, remain isosmotic with respect to ambient salinity (Table 1). This does not, however, imply that acclimation to changing salinities is a passive process: osmoconformers achieve salinity acclimation through active cellular volume regulation (CVR). When cells are acutely exposed to dilute seawater, they tend to gain water and lose solutes (they swell). The opposite happens when external osmolality increases (Sardini et al., 2003; Torre et al., 2013; Veiga et al., 2016). Cells then respond by active CVR, thus resulting in a regulatory volume decrease in the case of cell swelling, or a regulatory volume increase in the case of shrinking to fully or partially restore the original cell volume. This is accomplished via adjustment of intracellular osmotically active compounds, so called osmolytes (Figure 1) (Häussinger, 1996; Sardini et al., 2003; Wehner et al., 2003; Hoffmann et al., 2009). Depending on the abruptness of the change in osmolality, CVR can keep pace with passive osmosis, whereas acute osmotic stress will cause volumetric changes (Häussinger, 1996). An increase in cell volume can also be observed when the capability for CVR is reached or CVR is incomplete (Lange, 1970). Osmolytes, used for CVR, are inorganic or organic solutes whose concentrations are regulated during periods of osmotic stress (sensu Somero and Yancey, 2011) (Table 1). While the osmolality of extracellular fluids is exclusively driven by inorganic osmolytes, cells partially substitute inorganic with organic osmolytes during intracellular osmolality adjustment (Figure 1). This is due to the fact that changing concentrations of inorganic salts strongly influence protein functioning (Hochachka and Somero, 2002; Somero and Yancey, 2011). Some organic osmolytes are termed compatible osmolytes as their high intracellular concentrations do not impair protein structure or function (Hochachka and Somero, 2002) (Table 1).
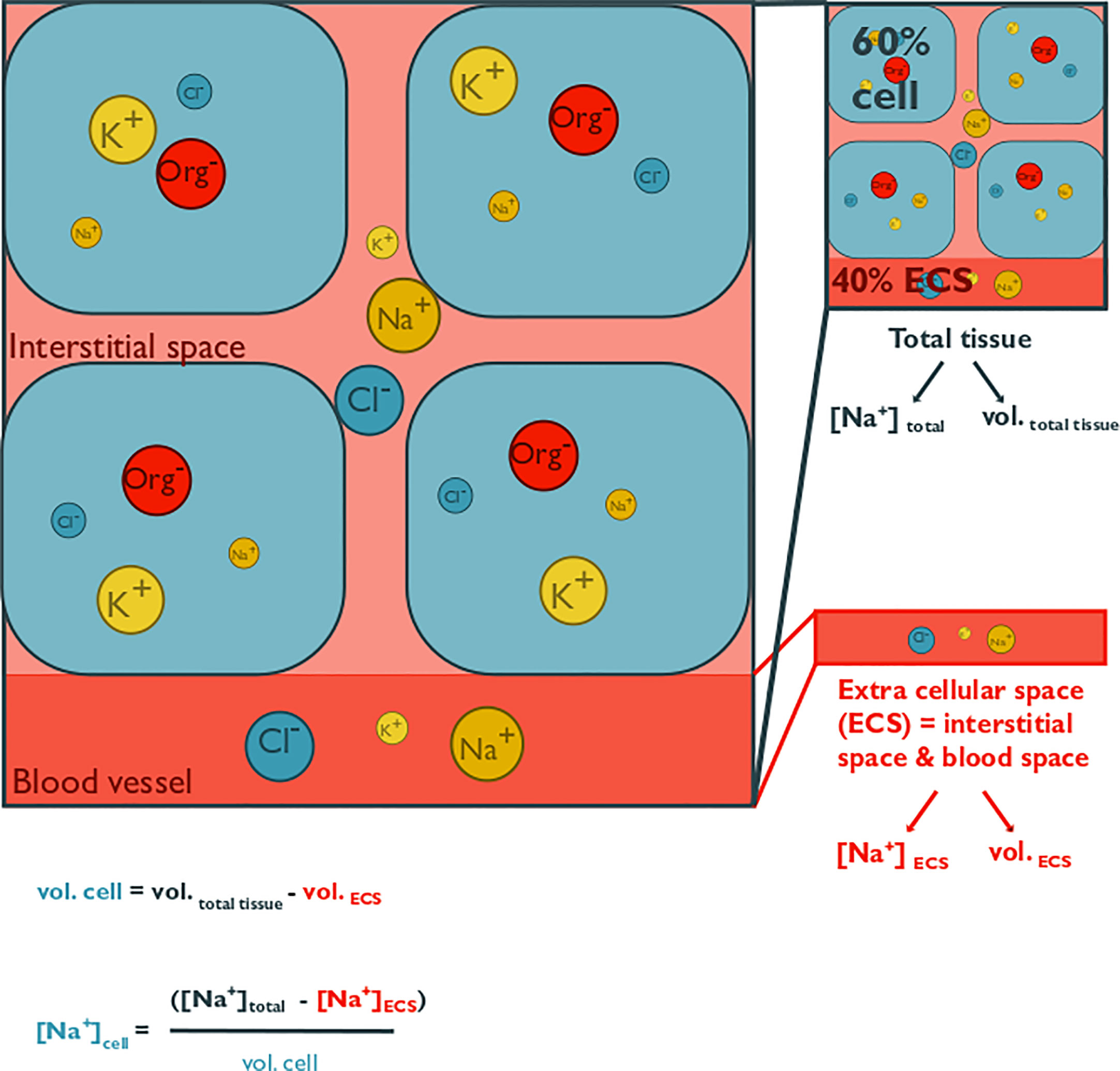
Figure 1 This conceptual figure shows the main components of cellular volume regulation in tissues and how intracellular solute concentrations can be estimated. It illustrates osmolyte concentrations in the extracellular space (ECS; consisting of interstitial space and blood, coelomic fluid, or hemolymph space) and within cells of a generic tissue. Extracellular fluid osmolality is modulated exclusively by inorganic osmolytes, whereas cells also utilize organic osmolytes. Only the major inorganic ions are depicted, namely chloride, potassium, and sodium. The abbreviation “Org” represents a variety of organic osmolytes, such as alanine, betaine, glycine, or taurine. The size of the circles represents the concentration of a specific osmolyte. A total tissue sample can contain a large proportion of the ECS, which is on average 40%, with a range from 10% to 80% of tissue mass. Since the ion concentration in the ECS roughly represents that of the surrounding seawater and intracellular osmolality is partially accounted for by organic osmolytes, intracellular inorganic ion concentrations are lower than those in the ECS. Measurements using tissue homogenates thus overestimate cellular inorganic osmolyte concentrations and underestimate organic concentrations, if they are not corrected for the ECS contribution. Extracellular ion concentration can be measured from blood, coelomic fluid, or hemolymph samples. The ECS can be estimated via the dilution of a known volume of a radioactive, or fluorescent tracer (such as 14C-inulin, or FITC-inulin) that is injected into extracellular fluid. Cell volume is estimated from the difference between tissue volume and ECS volume. Intracellular osmolyte concentration can then be calculated with the given formula, where vol. cell = cell volume, vol. ECS = ECS volume, vol. total = total tissue volume, [Na+] cell = intracellular sodium concentration, [Na+]ECS = ECS sodium concentration, [Na+]total = total tissue sodium concentration.
Time-dependent changes in intracellular osmolyte composition usually involve an immediate reduction or rise in inorganic ions such as sodium, magnesium, or potassium under hypo- or hyperosmotic stress, respectively (Berger et al., 1978; Natochin et al., 1979; Smith and Pierce, 1987; Stucchi-Zucchi and Salomão, 1998). Organic osmolyte concentrations are modified minutes later (Smith and Pierce, 1987; Villarreal and Kültz, 2015). Rapid release or uptake of ions through ion channels can rescue cells from mechanical damage (and death) that would otherwise be caused by excessive volume changes (Shumway, 1977). With ongoing salinity stress, inorganic ion concentrations have been suggested to be partially restored and instead organic osmolytes are reduced as osmotic replacements (Smith and Pierce, 1987). For the longer time of exposure or acclimation to hyperosmotic stress, species gradually modify the concentration of organic osmolytes and the composition of organic osmolyte budgets. Subsequently, these organic osmolytes are metabolized to organic osmolytes with higher osmoprotecting abilities or replaced replaced osmolytes accumulated via de novo synthesis (Reed et al., 1985; Somero and Yancey, 2011). This is a more time-intensive process that can last from hours to two to four weeks depending on the magnitude of hypo- or hyperosmotic stress (Baginski and Pierce, 1977).
To achieve long-term acclimation to reduced salinity, organic osmolyte concentrations must be reduced by discharging or catabolizing surplus osmolytes. The immediate responses of inorganic ions to osmotic stress have been examined in various studies in marine invertebrates. While the role of intracellular inorganic osmolytes in long-term acclimation to reduced salinity has been documented in a few individual mollusk studies (Potts, 1958; Berger et al., 1978; Stucchi-Zucchi and Salomão, 1998), the overall role of the inorganic osmolyte pool in long-term acclimation remains often underrated and more comprehensive coverage is needed. A commonly held assumption is that inorganic ion concentrations are replenished after an immediate reduction in response to low salinity stress, while organic osmolytes are reduced (Warren and Pierce, 1982; Smith and Pierce, 1987; Souza and Scemes, 2000). It has been stated that organic osmolytes are preferentially used intracellularly rather than inorganic ions for most long-term osmotic stresses (Yancey, 2004). The critical salinity concept even considers complete depletion of the organic osmolyte pool that marks the low salinity threshold of an organism, which was found to be applicable to the orange-striped sea anemone and the common starfish (Podbielski et al., 2016; Schmittmann, 2017). Characterization of intracellular inorganic vs. organic osmolyte concentration adjustments in relation to salinity changes is difficult, as there is a large and variable fraction of fluids in the extracellular space (ECS, interstitial fluid and blood, coelomic fluid or hemolymph) in tissue samples (Figure 1, Table 1). As a result, despite our knowledge of the general process of cellular volume regulation, it remains unclear which factors determine the capacity and limits for long-term salinity tolerance in osmoconformers.
Approximately >95% of all metazoan species are invertebrates (Zhang, 2011). Most invertebrates (Cnidaria, Porifera, Ctenophora, Echinodermata, Tunicata, most Mollusca, some Annelida, and Arthropoda) and a few vertebrates (Myxinidae, Euchselachii, and Coelacanthiformes) are osmoconformers (Oglesby, 1981; Gilles and Delpire, 2010; Khlebovich and Aladin, 2010; Yancey et al., 2014). Despite this vast number of osmoconforming species, it remains unclear how most osmoconformers acclimate to low salinity and whether similar osmolyte selection strategies are employed across taxa. Although it has previously been shown that there are differences in organic solute usage between plants, bacteria, invertebrates, and vertebrates (Kinne, 1993), it is unclear whether taxonomic differences in osmolyte usage exist within osmoconforming marine animals. It has been shown that different tissues can differ in their osmolyte usage, yet it is unclear whether these differences are systematic (Baginski and Pierce, 1977; George and Damodaran, 1999; Hiong et al., 2004).
In this review, we focus on a systematic comparison of inorganic and organic osmolytes in the tissues of benthic osmoconformers. We filtered the literature for studies that have acclimated marine osmoconformers to reduced salinity for a period of at least 14 days. Tissue organic osmolytes typically reach new steady state concentrations within such a time interval (Benson-Rodenbough and Ellington, 1982; de Vooys, 1991; Lucu and Devescovi, 1999). This screening procedure allows us to test whether decreased inorganic ion concentrations complement reductions in organic osmolyte concentrations during long-term acclimation to reduced salinity or whether inorganic ions are exclusively used for CVR during rapid salinity changes.
In our study, we assess the impacts of low salinity acclimation on the osmolyte concentration of osmoconformers to obtain information on osmolyte pool size and composition. We asked the following questions:
1. Which inorganic and organic solutes function as osmolytes in osmoconforming species?
2. Are both inorganic and organic osmolytes involved in salinity acclimation?
3. Are there taxonomic differences in osmolyte usage?
4. Are there differences in usage of osmolytes between the tissue types?
To answer these questions, we conducted an extensive systematic review of the available literature. The systematic approach follows a strict literature search and selection protocol that ensures an objective selection of suitable studies. We proceeded with a meta-analysis with the compiled data. The benefit of a meta-analysis over an ordinary literature review is the ability to statistically evaluate the individual effect sizes by computing a summary effect for multiple studies to estimate the mean of the distribution of the true effect sizes. To account for the expected biological variability, we conducted categorical subgroup meta-analyses for factors such as taxonomy and tissue.
2 Methods
2.1 Literature search and selection criteria
The literature was scanned for studies reporting inorganic and organic osmolyte concentrations of osmoconforming species in response to long-term salinity changes. To cover a wide literature base, we searched three scientific databases: ISI Web of Science, Google Scholar, and Scopus. The literature search was performed on studies collected until 30.01.2019. We used pre-determined, relevant keywords, their possible variations, and Boolean operators for the search strings (see Supplementary Material Data Sheet S1).
The search string was comprised as follows: marine benthic osmoconformers (free-living, non-parasitic metazoans >1 mm) as defined as our population of interest. Long-term low salinity stress (minimum 14-day exposure to the low salinity treatment) was chosen as the exposure variable in conjunction with marine salinity (or habitat conditions) as comparators (Pierce, 1971). To explore the effect of low salinity stress on osmotically active cellular substances, we selected studies that reported inorganic ion and/or organic osmolyte concentrations in tissues and extracellular space as outcome variables.
Following the initial literature search, three successional filtering steps followed: title scan, abstract scan, and full-text scan (Figure 2). During each step, the search results were assessed for relevance, and obviously irrelevant studies were excluded, whereas possibly relevant studies were included in the next assessment step. An abstract was conducted to validate a potential exclusion if the topic of the study was unclear during the title scan. Additionally, hand-picked studies were added, which were either already known or revealed following relevant literature trials during full-text analysis.
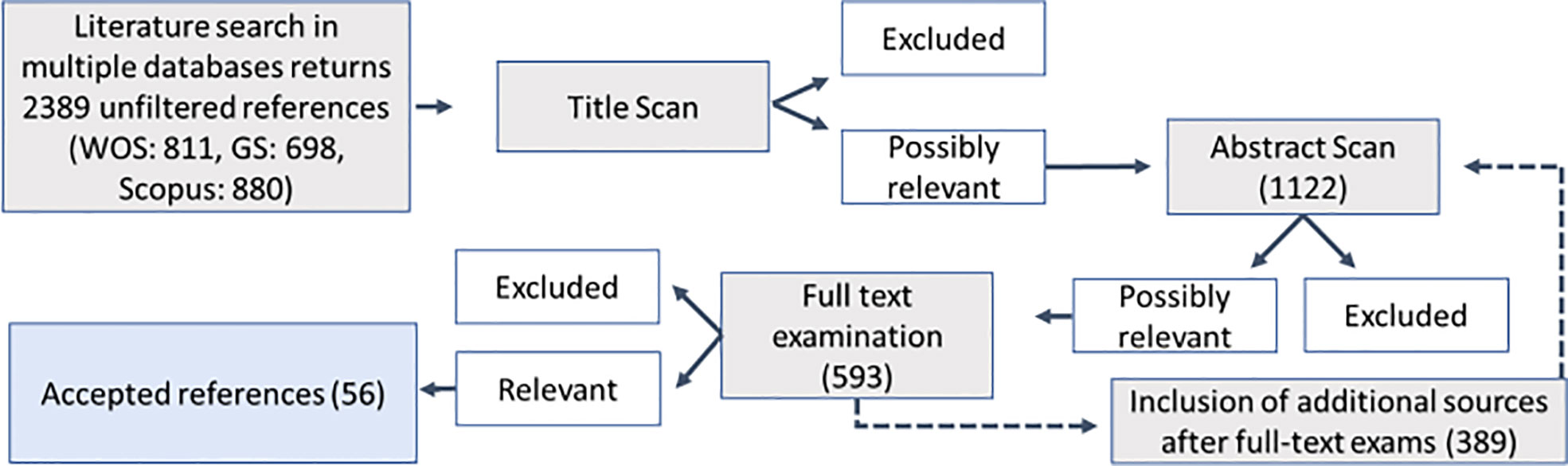
Figure 2 Flow chart for the systematic review process of literature retrieval and selection. Numbers indicate the number of papers per step.
The exclusion criteria were pre-defined and consistent throughout the filtering process. Included were studies with osmoconforming metazoan species from marine and brackish water habitats as study organisms. Partial osmoconformers were included in the study when they were reported to be isosmotic across >50% of their habitat salinity range but were found to regulate osmolality under severely hypoosmotic stress. Hypo- and hyperconforming species, whose osmolality is always slightly higher or lower (by <10 mOsm/kg in either direction) than that of the ambient medium but changes proportionally with salinity, were also included in our analysis. Studies were excluded when treatment salinity or acclimation time was unknown, when salinity fluctuated by more than 5, or when only a single salinity treatment was reported (absence of control treatment). To guarantee complete acclimation and exclude studies with incomplete acclimation or transient osmotic stages, we restricted our analyses to experiments with a salinity acclimation duration of at least 14 days. For multifactorial studies, only the response to altered salinity at the control condition of the co-variable was used for our analysis. Multifactorial studies where the salinity treatment could not be differentiated from the effect of other stressors were not included. When the classification of control conditions was unclear, the ambient or mid-range level of the additional factor was used. All articles reviewed at the full-text stage are listed in the Supporting Information (Table S2) with reasons for exclusion.
We assembled the results from 68 separate experiments from 56 studies (=published sources). Of these, 41 studies were revealed during the initial search, and 13 studies were identified as they were cited by studies collected via the database searches (List of included studies and study details: Table S2).
2.2 Data extraction
If a study met the general selection criteria, its data were extracted. Here, specific technical extraction rules were applied considering independent datasets, multiple outcome variables, or varying experimental designs. In cases with several independent experiments within a study (i.e., different species, different populations, or independent experiments using the same species), all experiments were treated as independent data if they met the overall criteria. When numerous experiments per study would have been added using this approach, experiments were averaged if possible to avoid bias (one study). Although incorporating multiple effect sizes from the same study decreases the independence of data points, this enables us to explore a wider range of species and to increase our sample size. When multiple effect sizes for the same outcome variable were reported from the same experiment (dependent effect sizes), dependent data were used if the outcome variable was listed for different tissue types or species, otherwise only one data point was chosen. When an experiment reported multiple outcome variables, all responses were used in separate analyses. When an experiment reported more than one parameter for a single response variable, the most generalizing response variable was included (e.g., Total Free Amino Acids < Ninhydrin-positive Substances < Total organic osmolytes). When the salinity response was recorded over a period with multiple sampling timepoints, the final time point was selected. For “before and after” study designs, the initial time point (T0) was used as a control if no control treatment was available.
When multiple treatment levels were reported, we used the lowest salinity treatment that the species survived at. For the high salinity group, we used the designated control treatment, or the salinity level representing marine or habitat conditions. The high salinity treatment was chosen to never exceed normal marine conditions to exclude hyperosmotic stress effects.
Studies were not considered if they did not meet specific statistical requirements. If response variables had an insufficient sample size (k <3) for meta-analysis, they were excluded (Table S1). We extracted the mean results, variance estimates, and sample size for low salinity and high salinity treatments from the selected studies. Regression results reporting values for R² and sample size were included and converted. When a regression study lacked statistical information but reported raw data, it was used instead. Graphical data was transferred from the primary literature using GetData Graph Digitizer (v 2.26.0.20; S. Fedorov).
2.3 Data analysis
The effect of low salinity stress was measured for each outcome variable for each experiment. by computing the effect size (here Hedge’s g (g)) and variance for each study and using weighed means to compute an overall effect (see Supplementary Material Data Sheet S2 for all relevant formulas).
The standardized mean difference is a wide-spread index for effect size used in meta-analysis. It has the advantage of being comparable even if studies use different scales (Hedges and Olkin, 1985). There is a large variety of units to express an osmolyte concentration in tissues. Although it would have been ideal to convert all measurements to the same scale before comparison, many authors have not reported the data necessary to enable these conversions. Thus, we used standardized means to include as many studies as possible. Hedge’s g corrects for a bias overestimating the standardized methods difference for small sample sizes (Hedges, 1981). A Hedge’s g of 0 is interpreted as the experimental treatment having no effect on the response variable, while a positive value indicates a positive effect and a negative value indicates a negative effect.
Meta-analyses weigh the individual effect sizes by the inverse of the effect size variance, thereby penalizing studies with high variance and low sample size (Borenstein et al., 2011). A random-effects model was used to calculate the overall mean effect for each response variable, thereby computing a weighed mean effect that accounts for sample size, within and between-study variance. An unweighted fixed effects meta-analysis was conducted to compare inorganic and organic pool sizes since most studies did not report variances. The effect size is considered significant (α = 0.05), when the 95% confidence interval excludes zero.
We accounted for dependent data clusters and potential underestimated variance by using robust variance estimates (Hedges et al., 2010). A robust test was used on the random or mixed-effects model, which estimates a variance–covariance matrix of the model coefficients. This test has the advantage of adjusting for small sample sizes, which improves the performance of the method when the number of clusters is small (Viechtbauer, 2010). This test yields more conservative results for confidence intervals and measures of significance. For comparability between response variables, the robust test was applied in all cases, even when dependent data clusters were absent.
When reporting the overall effect size, we also must consider the variation of effects between studies to estimate how much variance is due to the covariates (i.e., real differences in effect size) and what amount of variation is to be expected between studies. To examine the variation of effect sizes between studies, we calculated the Q-statistic. The QM value indicates how much heterogeneity is explained by the moderator and is used to test whether this proportion is significant. A significant QE statistic indicates that there is a significant underlying heterogeneity within the mean effect size and that other factors contribute to the effect size variation. The robust test does not report on heterogeneity measures, and thus results from the original model were used as indicators of residual underlying heterogeneity.
2.4 Subgroup analysis
Subgroup analysis was conducted to test moderators that could affect the overall effect size of low salinity on osmolyte concentration. We assumed that heterogeneity in effect sizes could be due to biological differences and compared different taxonomic groups at the phylum level (Annelida, Arthropoda, Chordata, Cnidaria, Echinodermata, Mollusca, Platyhelminthes, and Porifera) and down to class level where possible (Bivalvia and Gastropoda). Additionally, we compared the mean effect size among the various tissue types (i.e., whole body, gill tissue, intestinal tissue, muscle tissue, mantle tissue, heart tissue, and coelomocytes). To avoid repeated testing of the same data, which results in an increased probability of type 1 error, the analysis was limited to these a priori defined categories. Only subgroups containing sufficient data points (k ≥3) were considered.
To test for differences among subgroups, we performed separate mixed-effect models assuming random effects within-study variance and fixed-effects between-study variance for each category and response variable. A subgroup meta-analysis calculates a new summary mean effect size for all experiments included in the analysis and a mean effect size for each subgroup. The significance of the moderator variable is estimated by QM. The robust test was used for mixed-effects models. The number of studies included in the categorical analysis might differ in the number of datapoints from the initial overall mean effect size analysis. Thus, the new summary effect computed by subgroup analysis might differ from the overall meta-analysis result.
Lastly, the relationship between effect sizes and methodological factors was tested. Methodological factors can affect effect size and should be considered when designing experiments or evaluating the literature. The magnitude of salinity stress can impact the effect size value. The larger the salinity stress, the more the osmotic constituents are depleted. Thus, in stenohaline organisms with a smaller salinity range, this will have a smaller impact than in euryhaline organisms subjected to very low salinity conditions. We quantified the differences in effect size between studies for experimental duration and magnitude of salinity stress using a continuous meta-regression approach (Table S5).
Additionally, acute osmotic shock can overstrain the salinity tolerance of an organism, whereas a gradual acclimation would allow the species to acclimate successfully (Kapper et al., 1985; Pierce et al., 1992). We recorded the mode of salinity adjustment where possible (instantaneous vs. gradual; Table S2), but it was not defined a priori as a categorical moderator. Moreover, not all studies of the final data set described the time course of salinity transfer, thereby impairing statistical analysis. Despite this, an acclimation time of 14 days after salinity transfer should be sufficient for osmolyte concentrations to reach a new steady state (Pierce, 1970).
2.5 Sensitivity analysis
When conducting a meta-analysis, the diversity in study design across the literature can introduce potential biases. A rigorous sensitivity analysis was used to trim metadata, make the study diversity transparent and assess the quality of meta-analysis results and possible publication biases (Tables S2, S3).
First, the data were tested for its robustness and examined for statistical outliers and influential studies. This was done using multiple visual tools such as baujat and influence plots, as well as the influence function, which summarizes multiple statistics. When statistical outliers were detected and there was no suspected underlying heterogeneity, these data points were omitted from the analysis (Table S3).
To test for publication bias, we applied a series of indirect tests. To test for potential bias because of the lack of small studies with non-significant effects, we examined the symmetry of funnel plots, supported by Egger’s test and completed by the “trim and fill” procedure to adapt the model for potential asymmetry and check whether a significant effect remains valid after the inclusion of imputed studies (Egger et al., 1997; Duval and Tweedie, 2000).
However, recent research has shown that the assumptions of the small-effect study methods may be inaccurate in many cases (Peters et al., 2007; Koricheva et al., 2013; Simonsohn et al., 2014). Thus, Rosenberg’s Fail-Safe number was applied in addition since it does not rely on funnel plot asymmetry, giving an estimate of how many studies of the same weight (i.e., sample size) as the average of those already used studies are needed to reduce the significance of the effect size (Rosenberg, 2005).
P-curve analysis has been proposed as an alternative way to assess publication bias and estimate the true effect of the collected data (Simonsohn et al., 2014). Yet, p-value analysis is not robust against high heterogeneity. All results were considered concerning publication bias, but when high heterogeneity and small effects were present, more weight was put on the fail-safe number of results.
2.6 Statistics
All analyses were computed using R. Effect size calculation was performed using the “esc” package (Lüdecke, 2018). Meta-analyses were performed using the “metafor” package (Viechtbauer, 2010). Quality assessment was done using the latter as well as the “meta” and “dmetar” packages (Balduzzi et al., 2019; Harrer et al., 2021). The test and model results were considered significant with a p-value <0.05. Visualizations were conducted using R. The graphs show the mean effect sizes. Error bars depict 95% confidence intervals from robust tests. An effect can be considered statistically significant if the error bar does not overlap with null. Statistical significance is indicated by an asterisk above the effect size. A cross signifies a significant result that did not pass sensitivity analysis. In subgroup analysis, an asterisk above the overall effect symbolizes the significance of the moderator variable. For the overall effect size, Q denotes significant underlying heterogeneity from the original model results.
3 Results
3.1 Study and data characteristics
This database search yielded 2,389 results. From the initial unfiltered reference library, 1,122 studies passed the title scan. The following abstract scan reduced the number of studies to 593. This also included additional studies that emerged during full-text scans of the literature. After the full-text exam, the total number of accepted studies was 56, 38 of which assessed osmolyte concentrations in tissues, whereas the other studies sampled exclusively extracellular fluids (Figure 2). The resulting dataset covered five phyla, including 43 species. Most of those were Mollusca (n = 27), whereas fewer reports were obtained for Annelida (n = 4), Cnidaria (n = 4), Echinodermata (n = 1), and Porifera (n = 1) using our research criteria (Figure 3E). Reported ionic outcome variables in decreasing abundance were sodium, potassium, chloride, and magnesium. Sixty different organic solutes were identified. The minimum sample size for analysis (k ≥3) was available for 24 of those substances. These substances mainly belonged to the category of free amino acids (FAAs) and derivatives, methylamines, and methylsulfonium compounds. All organic solutes and inorganic ions whose concentrations were significantly affected by salinity in the following meta-analysis were osmolytes (sensu Somero and Yancey, 2011).
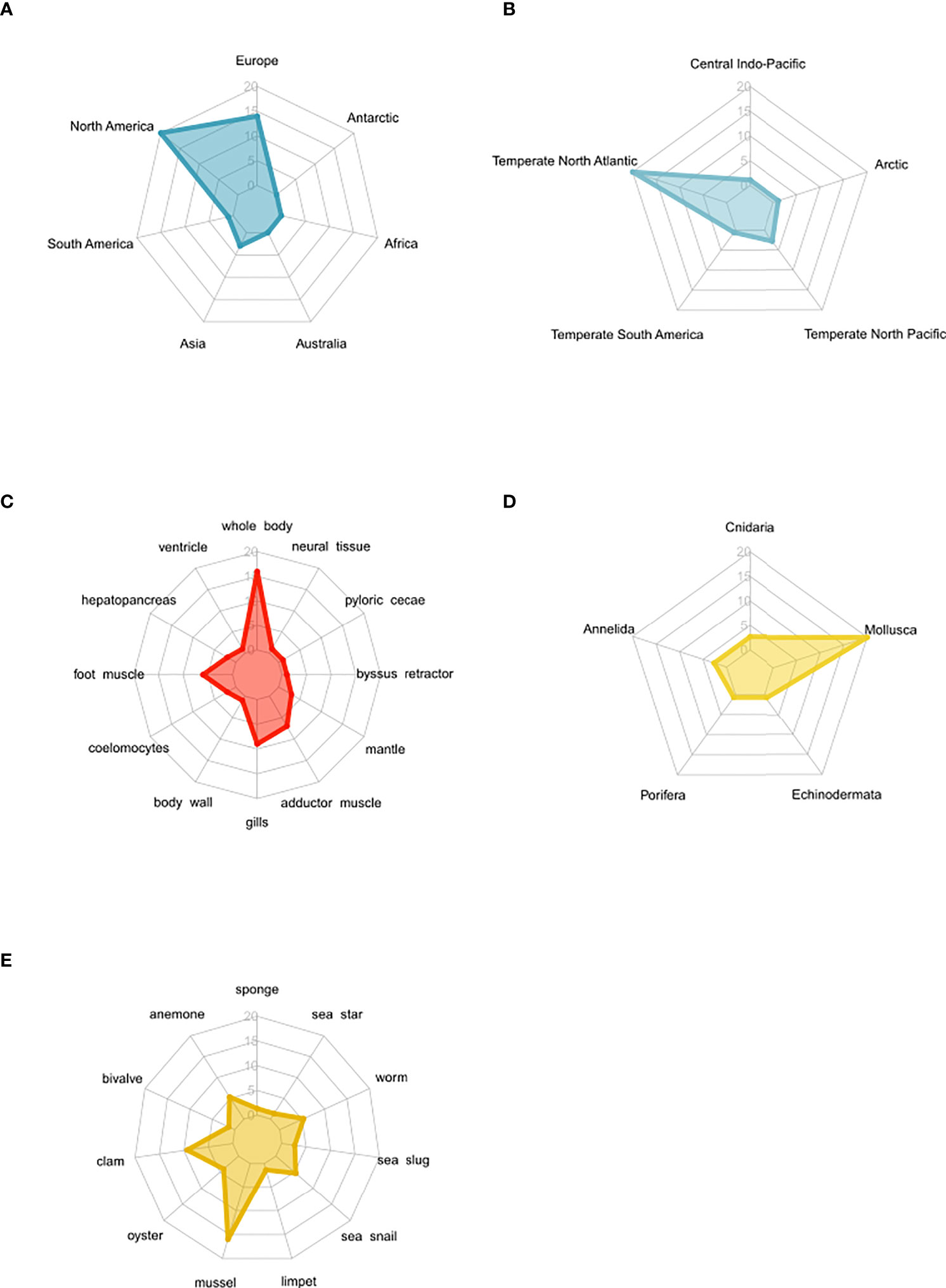
Figure 3 Spider plots highlighting the distribution of studies that measured osmolyte concentrations in osmoconformers following long-term acclimation to low salinity regimes. The number of studies increases from the center (n = 0) to the margin (n = 20). (A) Spread and geographic bias of osmolyte studies. (B) Spread and publication bias of osmolyte studies by biogeographic realms. (C) Spread and bias of osmolyte studies in different tissues. (D) Phylogenetic distribution of study organisms according to taxa. (E) Phylogenetic distribution of study organisms according to common name of phylum.
3.2 Effect of low salinity stress on osmolyte concentrations
We analyzed the overall effect of low salinity stress on various solutes across studies and phyla to identify osmolytes (Figure 4). Significant effect sizes indicate that the osmolyte concentration changes significantly at low salinity compared to high salinity conditions and is thus actively or passively modulated under salinity stress (see Table S4 for statistical outcomes of the meta-analysis). Of a total of 29 measured organic and inorganic substances, we identified six organic and one inorganic osmolytes. We observed a significant overall effect in 14 outcome variables, but only eight of these passed sensitivity analysis (Table S3). A significant salinity effect was observed for the following: the total organic osmolyte pool, alanine, betaine, glycine, proline, serine, sodium, and taurine. Significant heterogeneity of the true effect sizes between studies was observed for all these compounds.
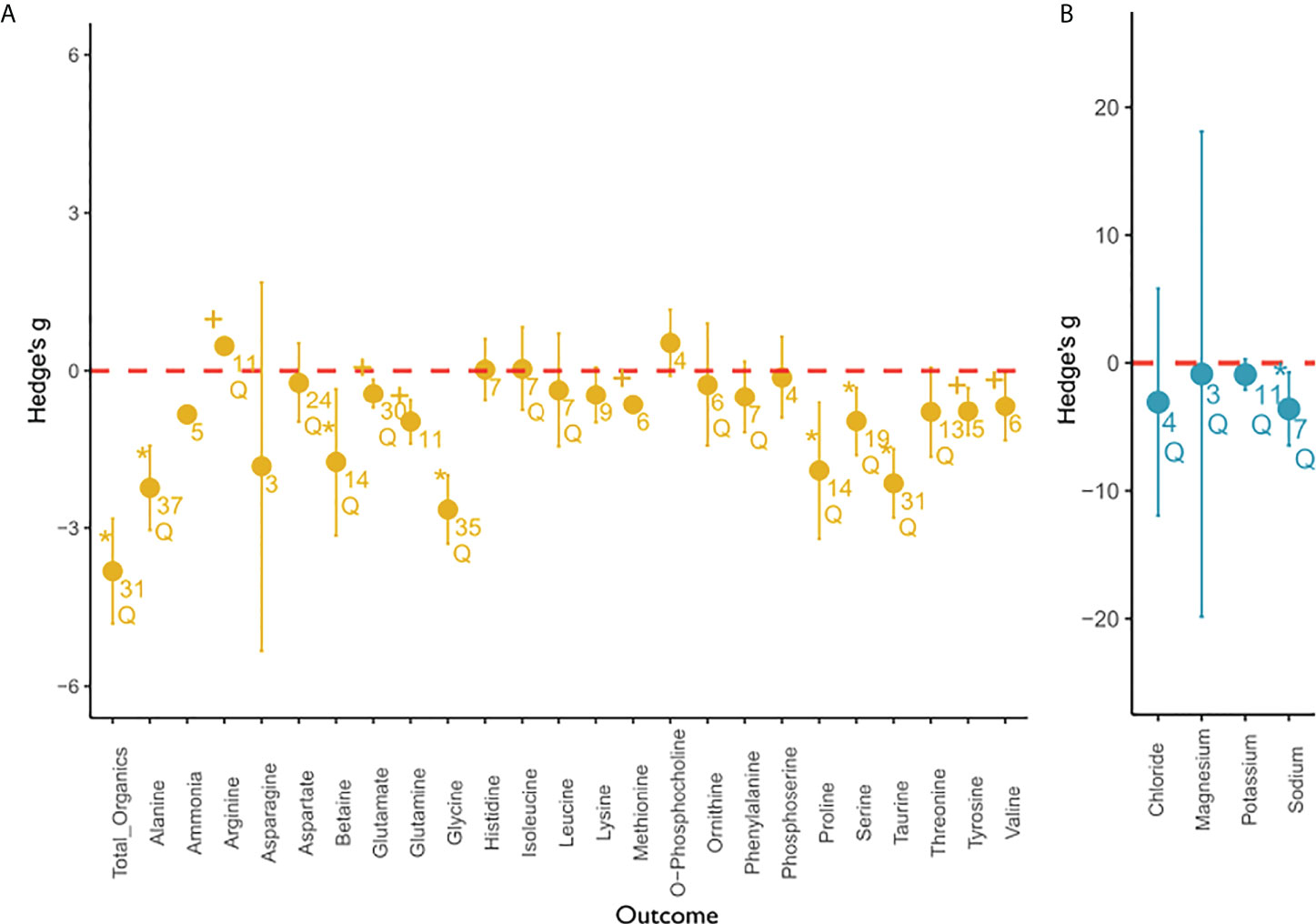
Figure 4 Overall meta-analysis of the salinity effect on (A) organic and (B) inorganic compounds. This figure depicts the overall effect of low salinity on solute concentration of organic compounds (yellow) and inorganic compounds (blue) from the random-effects meta-analysis. Individual outcome variables (compounds) are depicted on the x-axis and the effect sizes expressed as Hedge’s g are depicted on the y-axis. The mean effect size for each outcome variable is depicted as a circle with the error bars indicating robust confidence intervals (95%). If the confidence intervals do not overlap with zero (marked in red), an effect size is considered significant. If error bars are not visible, they are smaller than the data point. An asterisk indicates a significant, robust difference in effect size that passed sensitivity analysis. Results that were significant but were flagged during sensitivity analysis were marked with a plus. Q indicates significant residual heterogeneity as tested with the random effects model. Numbers below circles indicate k—the number of studies. All outcome variables can be considered intracellular, except for magnesium. Magnesium concentrations were reported for tissues only.
Few studies have measured intracellular (n = 11) or tissue (n = 5) inorganic ion responses to long-term salinity change. With respect to specific inorganic ions, the meta-analysis revealed that the intracellular potassium concentration was not significantly affected by salinity stress, but residual heterogeneity was observed. Concentrations of chloride and magnesium were not significantly affected by salinity. It must be noted that magnesium concentrations do not represent intracellular values but tissue values. Thus, these values include an unquantified extracellular ion component. Intracellular sodium was the only inorganic ion to decrease significantly with decreasing salinity. The mean effect sizes for sodium were similar to the effect sizes of total organics.
The effect sizes of individual organic osmolytes were smaller and usually less variable. More specifically, the random-effects model calculated an initial significant effect for ammonia, glutamate, glutamine, methionine, tyrosine, and valine, which we did not consider robust after sensitivity analysis due to low fail-safe numbers, the effect not being significant after the trim and fill procedure, or due to a significant p-curve test (see Table S3 for Sensitivity Analysis). No significant effect, but residual heterogeneity was observed for arginine, aspartate, isoleucine, leucine, ornithine, phenylalanine, and threonine. We could not find an effect of salinity on the concentration of asparagine, histidine, lysine, o-phosphocholine, and phosphoserine. Hence, we did not consider them to function as osmolytes. Additionally, there was no significant heterogeneity, which would indicate other involved moderator variables.
Significant heterogeneity of effect sizes (QE) was observed for the majority of solutes analysed (n = 19). All substances with underlying heterogeneity were investigated further using the a priori defined categories for subgroup analysis if the sample size allowed it.
3.2.1 Phyla
The categorical mixed-effect model analyzing the low salinity effect on tissue osmolyte concentrations in different phyla was conducted for 12 organic compounds that exhibited significant residual heterogeneity in the overall effect and provided a large enough sample size in multiple subgroups (Figure 5). There was not enough data to include any inorganic osmolyte in this analysis. The supported phyla were Annelida, Echinodermata, and Mollusca. For more taxonomic differentiation, we differentiated the salinity effects by class for Bivalvia and Gastropoda (Figure S1). We detected a significant phylogenetic difference for 10 organic compounds. Generally, the phylum was a significant moderator affecting total organic solute concentrations with respect to salinity stress.
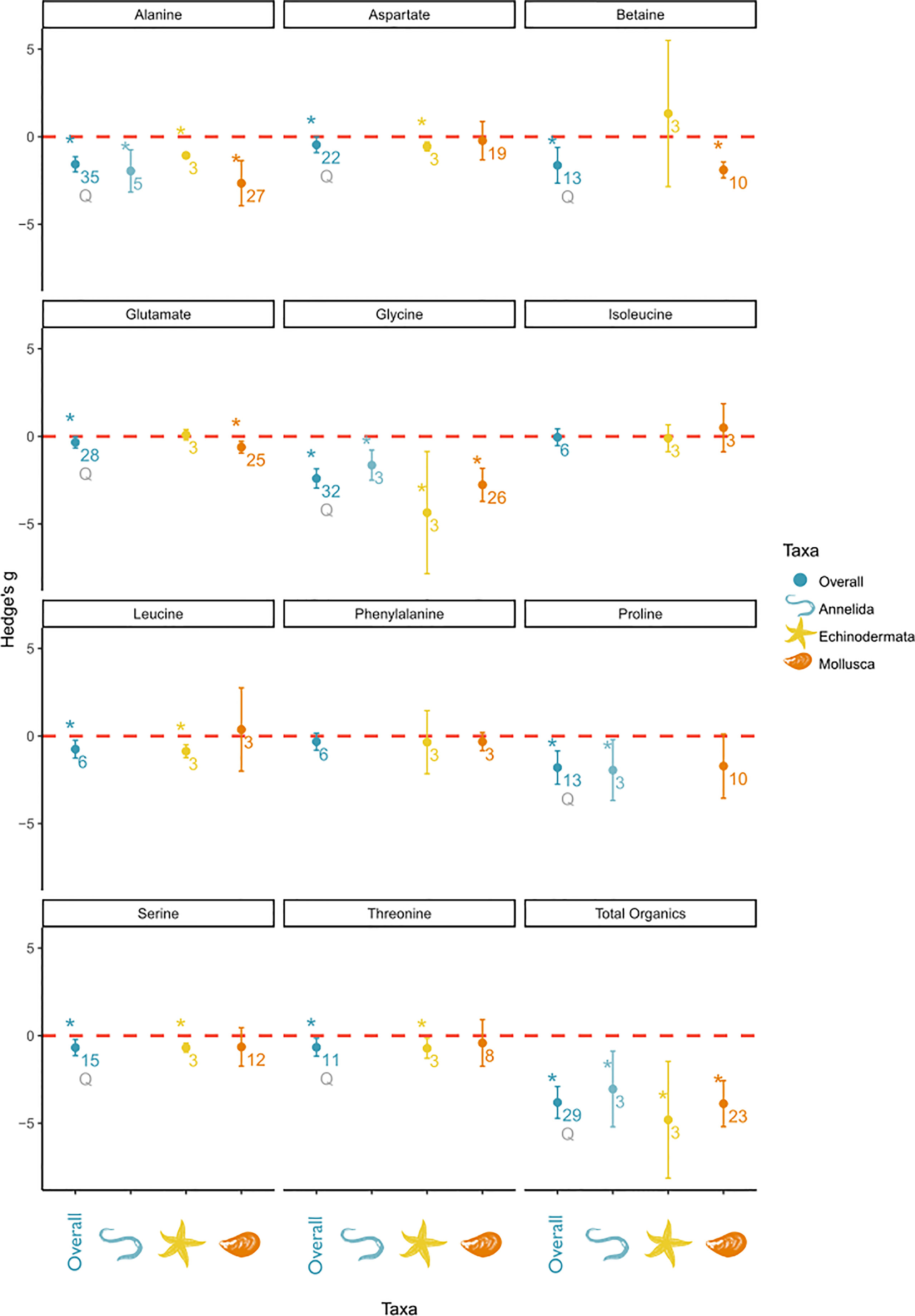
Figure 5 Subgroup analysis of salinity effects on organic osmolyte concentrations in tissue by taxa. The panel includes a separate plot, for each measured solute. In each plot the overall effect and the subgroups are depicted on the x-axis and the effect sizes as Hedge’s g on the y-axis. A positive value indicates a positive salinity effect (i.e., an increase in concentration at decreasing salinities), whereas a negative value indicates a negative salinity effect on solute concentration. The overall mean effect size might differ from Figure 4 due to changed sample size when subgroups could not be included due to small sample size. The mean effect size for each taxon is depicted as a circle with the error bars indicating robust confidence intervals (95%). An asterisk above the overall effect size indicates taxon affiliation as a significant moderator. Asterisks above the individual taxa indicate a significant salinity effect for that specific subgroup. Numbers below circles indicate k—the number of studies. Q indicates significant residual heterogeneity as tested with the mixed effects model.
Specifically, alanine, aspartate, betaine, glutamate, glycine, leucine, proline, serine, and threonine effect sizes were significantly affected by the phylum affiliation. However, all of these significant effects were accompanied by significant residual heterogeneity, except for leucine. Alanine, betaine, glycine, and proline were characterized by the largest effect sizes among the organic osmolytes. Alanine and glycine were identified as osmolytes in all taxonomic groups. Betaine and glutamate were identified as osmolytes in Mollusca but not in Echinodermata. The proline concentration was significantly affected by salinity in Annelida, but not in Mollusca. Because of a high variance in mollusk effect sizes, concentrations of aspartate, leucine, serine, and threonine were only significantly altered by salinity in Echinodermata despite similar effect sizes in Mollusca. However, the effect sizes of aspartate, leucine, serine, and threonine were smaller than those of other osmolytes.
To summarize, in Annelida, alanine, glycine, and proline were the main osmolytes, with an overall smaller effect size for alanine and glycine than in other taxonomic groups. In Echinodermata, glycine is the main organic osmolyte, accompanied by several minor organic osmolytes, e.g., alanine, aspartate, leucine, serine, and threonine. In Mollusca, the organic osmolyte pool was mostly composed of alanine, betaine, and glycine and was accompanied by glutamate as a minor osmolyte.
3.2.2 Tissue type
We further analyzed whether there were differences in osmolyte usage between the tissue types. In total, 14 outcome variables fulfilled the prerequisites for subgroup analysis. For those variables, meta-analysis outcomes had a significant residual heterogeneity in their salinity effects. We observed significant differences in effect sizes between tissue types in 10 compounds (Figure 6).
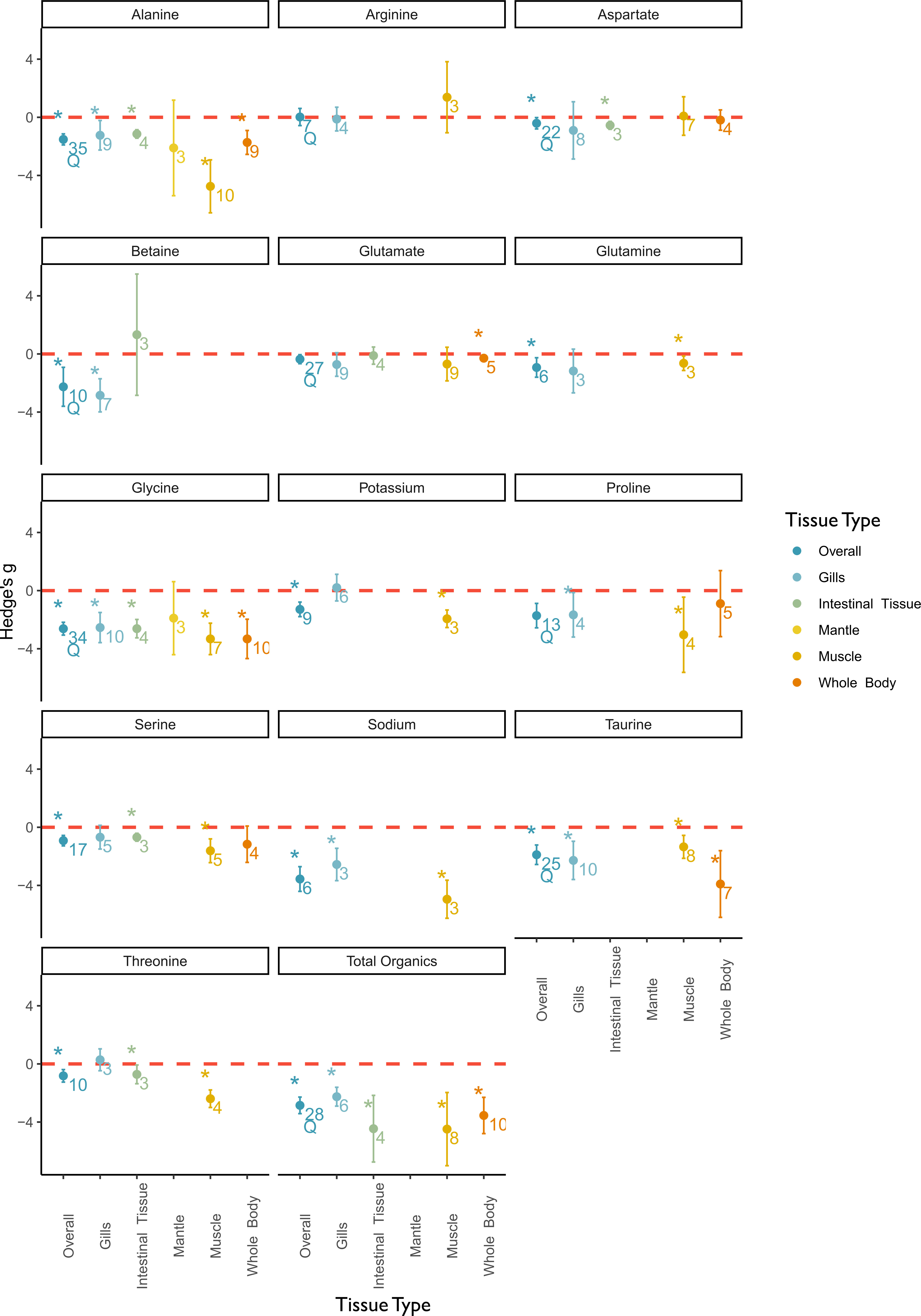
Figure 6 Meta-analysis results of the effect of low salinity stress on osmotic compounds by the categorical moderator variable tissue type. The panel includes a separate plot for each measured solute. In each plot, the overall effect and the subgroups are depicted on the x-axis and the effect sizes as Hedge’s g on the y-axis. A positive value indicates a positive salinity effect (i.e., an increase in concentration at decreasing salinities), whereas a negative value indicates a negative salinity effect on solute concentration. The overall mean effect size might differ from Figure 4 due to changed sample size when subgroups could not be included due to small sample size. The mean effect size for each tissue type is depicted as a circle with the error bars indicating robust confidence intervals (95%). An asterisk above the overall effect size indicates tissue type as a significant moderator. Asterisks above the individual tissue types indicate a significant salinity effect for that specific subgroup. Numbers below circles indicate k—the number of studies. Q indicates significant residual heterogeneity as tested with the mixed effects model.
Two inorganic compounds were included in this subgroup analysis. The intracellular sodium concentration was significantly more impacted by salinity stress in muscle tissues than in gills, and the variance was sufficiently explained by this moderator. The intracellular potassium concentration was not affected by salinity in gills, whereas a significant salinity effect was observed in muscle tissue. In the case of sodium and potassium, the data for muscle tissue originated from one study, which is why no additional robust tests were conducted.
The total organic osmolyte concentration was significantly impacted by salinity across all tissue types. The effect was most pronounced in intestinal and muscle tissue. Glycine and alanine concentrations were significantly affected in all tissue types except in mantle tissue and with the largest effect in muscle tissue. Betaine concentration was significantly affected in the gill tissue but not in the intestinal tissue. The main osmolytes in the gill tissue were betaine, glycine, and taurine. In intestinal tissues, the primary osmolyte used was glycine. Muscle tissue had a larger number of significantly affected osmolytes than other tissue types being studied and was the only tissue where sodium concentration was significantly affected by salinity. No osmolytes were identified in mantle tissue.
We identified tissue type as a significant moderator for the following minor osmolytes: aspartate, glutamine, serine, and threonine. Among these, the mean effect size of aspartate had a significant residual heterogeneity. Aspartate concentration was only affected by salt in intestinal tissues. Glutamine concentration was only affected by salinity in muscle tissue. Serine and threonine concentrations were significantly impacted by salinity in muscle tissues and less so in intestinal tissues. Tissue type was a non-significant moderator for arginine, glutamate, and proline concentrations and significant residual heterogeneity remained.
3.3 Methodological variation in response to low salinity stress
To test for the effect of methodological moderators on the outcomes of the meta-analyses, the magnitude of salinity stress and experiment duration were tested in separate meta-regression models (see Table S5 for statistical output of meta-regression testing of methodological moderators). A significant effect of the magnitude of stress (range of salinity reduction 3–33) on mean effect size was observed in five of the 29 outcome variables under study, namely alanine, leucine, potassium, proline, and valine. Alanine and proline had significant underlying heterogeneity. Experimental duration (range of duration: 14–70 days, and field studies) was significant for five of 29 outcome variables, namely, ammonia, glutamate, potassium, taurine, and valine. When excluding field studies, there was no significant effect of acclimation duration except for ammonia and potassium.
3.4 Impact of salinity stress on osmolyte budgets
Very few studies have examined the total inorganic ion as well as total organic osmolyte concentration budgets. In total, we identified only three studies that reported the necessary parameters (Figure 7). Two of the three studies reported tissue and intracellular values, but for comparative reasons, the meta-analysis of budgets was calculated for the tissue data (i.e., non-ECS-corrected) for all studies. Additionally, even though total osmolality was available for one study, we used the sum of the measured total organic and inorganic osmolytes to enable comparable normalization across studies. The unweighted fixed effects meta-analysis revealed no significant effect of salinity on the inorganic–organic osmolyte ratio, for either intracellular or tissue data. The inorganic portion of the total osmolyte pool in tissues and intracellular fluid changes significantly with salinity. However these outcomes were not robust. The same is true for the organic portion of the total pool (see Supplementary Material Data Sheet S3 for meta-analysis results of budgets).
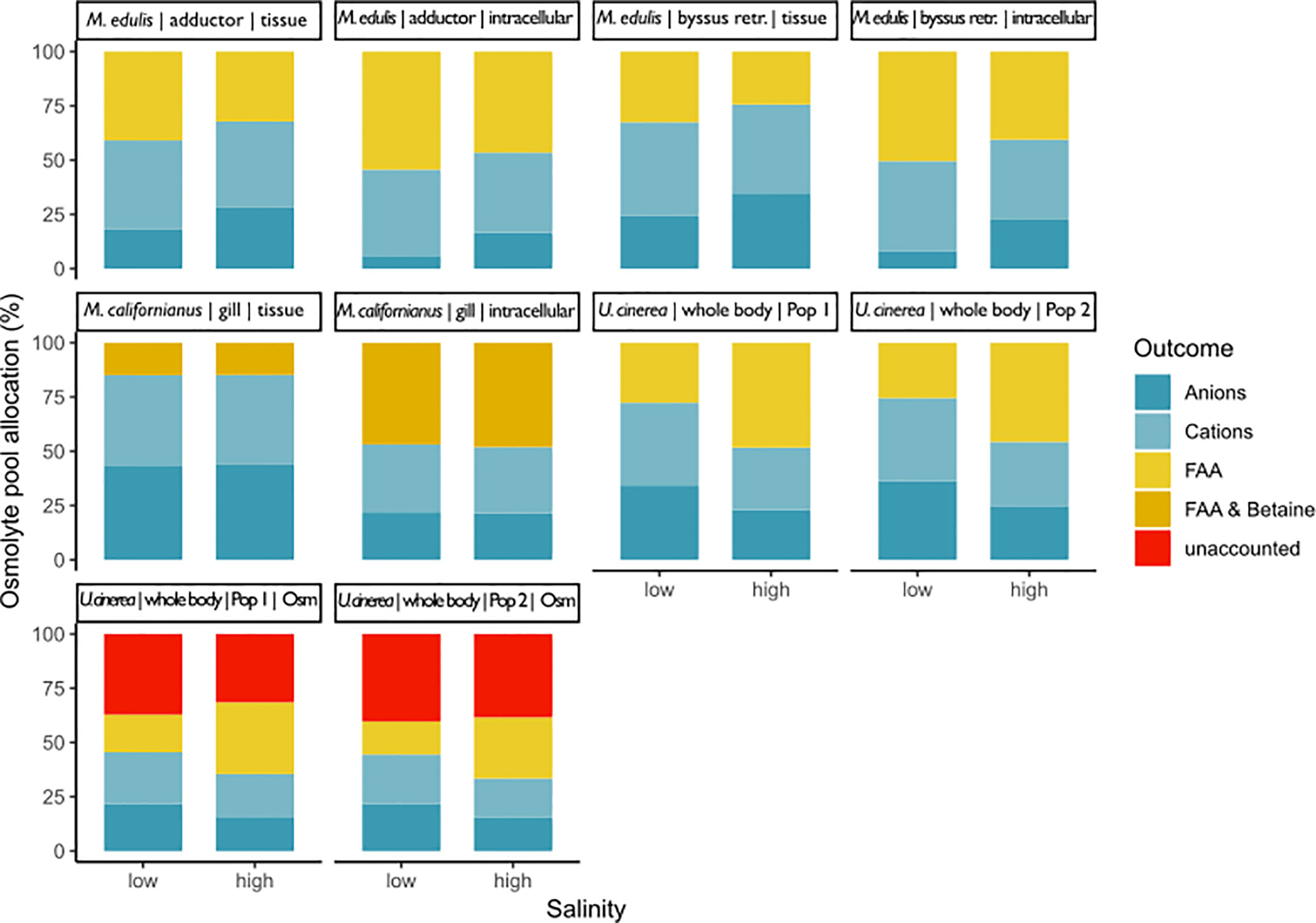
Figure 7 This graph shows the composition of the osmolyte pool at low and high salinity for three different bivalve and a gastropod mollusc species: Mytilus californianus gills (Silva, 1992), Mytilus edulis adductor muscle and byssus retractor (Potts, 1958), and Urosalpinx cinerea whole body samples from two different populations (Turgeon, 1973). The percentage of the total osmolyte pool is shown on the y-axis and salinity on the x-axis. Overall pool size was calculated as the sum of measured inorganic and organic pools and also total osmolality for Turgeon (1973). Potts (1958) and Silva (1992) did not measure total osmolality. Potts (1958) and Silva (1992) did account for extracellular space and thus have results for whole tissue and intracellular space. Potts (1958) and Turgeon (1973) did not account for methylamines. Silva (1992) did account for betaine but no other methylamines.
3.5 Sensitivity analysis
Within our data sets, some influential studies were removed (see Table S2 for a complete list of removed studies). Often, the same studies were outliers across multiple outcome variables, such as Kapper et al. (1985); Ran et al. (2017); Schmittmann (2017), and Silva (1992). The funnel plots together with Egger’s test demonstrated significant asymmetry for all major osmolytes (alanine, betaine, glycine, proline, sodium, and taurine) and glutamate. The minor osmolytes appeared to have no publication bias. When asymmetry was detected, the trim and fill procedure was used to test for the robustness of the effect size. The trim and fill procedure revealed that the adjusted effect sizes of alanine, betaine, glycine, proline, taurine, and total organics were robust. This was not true for sodium and glutamate. However, p-curve analysis indicates a true effect behind all of those data sets except for glutamate and glutamine. Outcome variables with significant salinity effects had Rosenberg fail-safe numbers from 5 to 1,974. Most effect sizes were robust. A few minor organic osmolytes had low fail-safe numbers, namely, valine, methionine, tyrosine, and ammonia. Here, low fail-safe numbers might be due to a small sample size along with a small effect size. In conclusion, there seems to be a moderate publication bias for all major osmolytes. Nevertheless, there was a true salinity effect for all major osmolytes, and fail-safe numbers were robust. The minor osmolytes ammonia, glutamate, glutamine, methionine, tyrosine, and valine results did not pass sensitivity analysis.
Further publication bias arises from the unbalanced distribution of studies across continents and biogeographic regions, with most studies being conducted in Europe and North America in the North Atlantic temperate region (Figures 3A, B). Also, there was a large bias in the sampled tissues toward whole-body and muscle tissues (Figure 3C). Considering the study organisms, mostly mollusks were studied with a specific focus on bivalves (Figures 3D, E).
4 Discussion
To the best of our knowledge, osmolyte data in osmoconformers has never been systematically and statistically analyzed using meta-analysis. This holistic approach revealed general patterns of cellular osmolyte composition and concentration changes in osmoconformers. Our review revealed large gaps in knowledge, especially with respect to the role of inorganic ions in osmoregulation. Furthermore, the lack of data on complete osmolyte budgets for intra- and extracellular compartments in marine invertebrate species was highlighted by this analysis.
4.1 Inorganic osmolyte pool
While the role of inorganic ions as an immediate response to salinity change has been well documented (Silva and Wright, 1994), studies on their use in long-term salinity acclimation are presently contradictory. Some studies clearly document an important role of the inorganic ion pool in long-term acclimation to salinity changes (Berger et al., 1978; Natochin and Berger, 1979; Prosser, 1991; Berger and Kharazova, 1997), whereas other studies report that intracellular inorganic ion pools, especially potassium (but also sodium), are highly conserved across taxa (Yancey, 2005; Burg and Ferraris, 2008; Kültz, 2012; Palmer, 2015; Somero et al., 2017). This meta-analysis indicates that at least some inorganic ions, here sodium, are not exclusively used intracellularly during short-term responses to changed salinity. Despite the limits in the study number, the subgroup meta-analysis also revealed significant differences between intracellular concentrations in muscle and gill tissues for sodium and potassium. We thus conclude that inorganic ions play a potentially important role during long-term salinity acclimation, which needs to be studied further.
One reason for the assumption of the long-term stability of inorganic ion concentrations is that significantly altered intracellular inorganic ion concentrations can have strong perturbing effects on cellular protein function (Bowlus and Somero, 1979; Burg et al., 2007). Perturbing effects of monovalent ions under low salinity conditions are more likely to be due to the ligand–enzyme interaction than to perturbing effects on protein structures (Yancey and Somero, 1978; Bowlus and Somero, 1979). This led to the commonly held assumption that all inorganic solutes are strongly conserved or replenished over time following acute osmotic disturbances (Schoffeniels, 1976; Yancey et al., 1982; Somero and Bowlus, 1983; Smith and Pierce, 1987). However, these studies are mainly based on hyperosmotic stress. Some studies found no effect on the enzyme activity at low salinity (Sarkissian and Gomolinski, 1976; Ballantyne and Moyes, 1987), whereas others reported that protein function and stability at sub-optimal ion concentrations still allow >60% enzyme activity within a normal osmolality range (Weber et al., 1977; Ballantyne and Berges, 1991). At the lower salinity experienced and tolerated by euryhaline marine invertebrates, monovalent ion concentrations could still be within the range that allows for sufficient enzyme activity. It was also found that enzymes from osmoconformers are not inhibited by salt, whereas enzymes from osmoregulators are inhibited (Sarkissian, 1974).
Furthermore, there are differences in the compatibility of individual inorganic ions with protein functions. Potassium seems to be preferred over sodium as an intracellular cation due to its higher solvent capacity of the cell water and its function as a weak protein stabilizer (e.g., Hofmeister Series) (Bowlus and Somero, 1979; Somero et al., 2017). Intracellular chloride concentrations are much lower than potassium concentrations, likely because high concentrations of the anion inhibit protein synthesis (Weber et al., 1977; Somero et al., 2017).
In this meta-analysis, no effect was detected for chloride, potassium, and magnesium. The lack of a significant decrease in the concentrations of chloride and magnesium is likely due to the small number of available studies and high variability. Out of the four available chloride studies, three found a significant salinity effect (Potts, 1958; Emerson, 1969; Silva, 1992). Intracellular potassium concentration analysis had a larger study number, but studies report contradicting results (Berger et al., 1978; Willmer, 1978; Stucchi-Zucchi and Salomão, 1998). The difference in these results could be due to a difference in salinity ranges since potassium concentrations typically become downregulated at extremely low salinities (<5–10) (Berger and Kharazova, 1997). However, the salinity range covered in the potassium and sodium studies was similar (Table S2), and moreover, the study with the lowest salinity treatments showed no effect of low salinity on potassium concentration. It could thus point to species-specific use of intracellular ions, which needs to be investigated further.
The second explanation is based on the different roles of specific inorganic ions. Intracellular potassium levels are generally better protected than those of sodium, possibly due to the importance of potassium gradients for maintaining membrane potential and the less perturbing effect of sodium variation on the membrane potential and the specific usage of the sodium gradient for paracellular transport (Yu, 2003; Palmer, 2015; Somero et al., 2017). An alternative hypothesis for the higher dependence of cells on potassium homeostasis (over sodium) refers to the evolution of cells in high potassium environments (Mulkidjanian et al., 2012). This theory of adaptation to high intracellular potassium concentrations is supported by optimal protein function and stability at high potassium levels (Bowlus and Somero, 1979; Kirschner, 1991). Only at extremely low salinity (<10) may CVR take priority over potassium homeostasis, decreasing intracellular potassium concentrations. Hence, this could be an explanation for why inorganic osmolytes are less interchangeable compared to organic osmolytes.
Generally, salinity had a larger effect on sodium than on individual organic osmolytes. This leads us to the assumption that intracellular inorganic ions, especially sodium, are more than a first response strategy to avoid cell damage under acute osmotic stress. Instead, inorganic ions seem to be as important as organic osmolytes in the salinity tolerance of osmoconformers during long-term low salinity acclimation. However, the other main discoveries of this meta-analysis regarding inorganic osmolyte research are the lack of data and the extreme bias toward mollusk studies.
4.2 Organic osmolyte pool
Organic osmolytes are key actors in cellular osmolyte regulation. Different substances are reported as organic osmolytes in the literature, yet reports differ on the role of specific substances or compound classes. Our analysis excluded isolated reports of osmolytes or listings of solutes whose concentrations do not change with salinity, thereby generating a more robust candidate list of intracellular osmolytes that are commonly used across taxa. The data for organic osmolyte concentrations discussed in this chapter originated from tissue samples only. However, only 20% of studies corrected tissue concentrations for extracellular volume. This resulted in an under-estimation of intracellular organic osmolyte concentrations, as extracellular volume, which does not contain organic osmolytes, constitutes 10%–80% of tissues in marine invertebrates (Figure 1) (Martin et al., 1958; Berger et al., 1978).
Over 80% of the identified organic osmolytes were FAAs, which are only one of many compound classes involved in CVR. Our analysis revealed that 85% of the studies did not aim to detect methylamines or methylsulfonium compounds. In this meta-analysis, betaine was the only methylamine identified as a major osmolyte. Hence, the lack of routine detection of methylamines or methylsulfonium compounds might have led to an underestimation of a potentially important part of the organic osmolyte pool. This methodological bias hampers the estimation of the contribution of methylamines (Somero and Bowlus, 1983; de Vooys, 1991). The contribution of methylamines to the organic osmolyte pool ranged from 3% to 76%. Quaternary ammonium compounds (QAC)s such as proline–betaine have been identified to constitute a large fraction of the organic osmolyte pool in studies that have measured both FAAs and QACs (Pierce et al., 1984). Known important methylamines and methylated sulfonium compounds include glycine–betaine, proline–betaine, dimethylsulfoniumproprionate (DSMP), homarine, sarcosine, or trimethylamine N-oxide (TMAO). Thus, it is necessary to include the measurement of methylamines when analyzing the organic osmolyte pool. Nevertheless, FAAs can be considered a major component of the organic osmolyte pool, and some species (the echinoderm Asterias rubens) completely rely on FAAs as their organic osmolytes (Schmittmann, 2017).
This systematic review revealed that the organic osmolyte pools of osmoconformers across taxa consist of a common set of a few organic compounds, which differ in relative concentrations. Over 70% of the study organisms only employed one or two major substances, which constituted 35%–70% of the osmolyte pool (Figure 8). The use of more than one primary osmolyte has been suggested as a strategy to guarantee osmoregulatory flexibility should the synthesis of one osmolyte be interrupted or limited (Greenwalt, 1981). The main osmolyte is often accompanied by one to three other minor osmolytes, whose contribution to the total pool is usually <20% (Figure 8). Minor osmotic compounds are often intermediate substances to the final major osmolytes (Greenwalt, 1981; Koyama et al., 2015). A constraint of our analysis is incomplete datasets or imprecise descriptions thereof in the reviewed literature. Therefore, organic osmolyte pool proportions displayed as a heat map (Figure 8) are therefore erely observational.
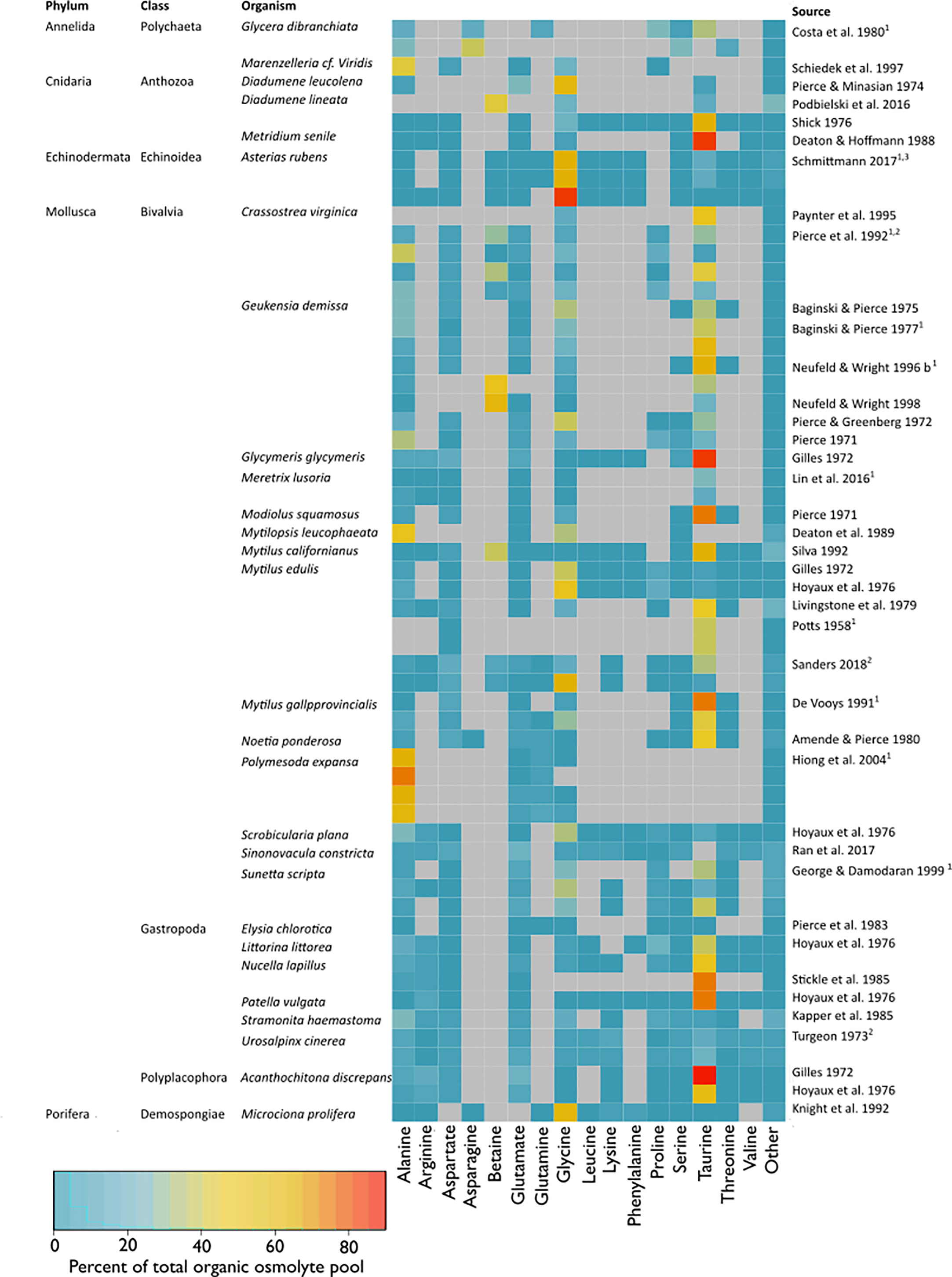
Figure 8 Composition of the organic osmolyte pool (% total organic osmolyte pool) at habitat salinity (11–42). The heatmap indicates percentages of single osmolytes in relation to the total organic pool, where red indicates a high percentage, blue a low percentage, and grey marks NAs. Only compounds that accounted for >5% of the total organic osmolyte pool in a study and were reported in more than 1 study were listed. Compounds <5% of the total organic osmolyte pool or with one entry were summed up under “Other”. Multiple entries per study are either different tissue types, separate populations, or independent experiments and are marked with superscripts 1–3, respectively. Studies that passed the full exam were included. Additionally, studies were included that had to be excluded from meta-analysis due to missing information for calculation of effect sizes but contained information that was sufficient to calculate the organic osmolyte pool composition.
We identified six organic osmolytes across the taxa: alanine, betaine, glycine, proline, serine, and taurine (Figure 5). Of these, alanine, betaine, glycine, and taurine were the main organic osmolytes in benthic marine osmoconformers (Figures 5, 8), in agreement with other comparative studies (Somero and Bowlus, 1983). When all substance classes are considered, betaine is often the main osmolyte, accompanied by taurine (Silva, 1992; Neufeld and Wright, 1996). Individual studies reported glutamate or proline to play more important roles than suggested by the compiled dataset in this study (Simpson et al., 1959; Somero and Bowlus, 1983). The random-effects model revealed significant heterogeneity of variances for most organic osmolytes, indicating further underlying differences in effect sizes, possibly due to taxonomic or tissue-specific differences.
4.3 Taxonomic differences in organic osmolyte concentrations
Our present study demonstrates that phylogeny is a significant underlying factor affecting organic osmolyte adjustment under salinity stress (Figures 5, 8). Mollusca were the most represented taxon (30 studies), with Echinodermata the least represented (one study, one species) (Figure 3D). All reviewed taxa exhibited a significant low-salinity effect on the organic osmolyte pool. The main osmolytes, alanine, betaine, and glycine, are used to different extents in different taxa. We observed that: 1) Glycine is widely used across taxa; 2) Echinodermata and Porifera rely heavily on glycine and do not use taurine. 3) Taurine is mainly used by Mollusca (also present in Cnidaria). Next to taurine, betaine and glycine are major osmolytes in Mollusca. 4) Annelida does not use a single main osmolyte in a large quantity, but uses various osmolytes in smaller amounts. 5) Echinodermata only employ FAAs, whereas methylamines play major roles in molluskan and cnidarian CVR. Additionally, some minor osmolytes seem to be phylum-specific, i.e., glutamate in Mollusca, or proline in Annelida.
Unfortunately, more specific conclusions from the meta-analysis were hindered by a lack of studies. We did not have a sufficient sample size available for analysis in other taxonomic groups to consider methylamines other than betaine. In mollusks, taurine has been reported to be ubiquitous (Awapara, 1962). This is in accordance with our findings (Figure 8), but could not be further explored due to a lack of data in other groups. Furthermore, we did not find a single study on organic osmolyte concentration in the tissues of osmoconforming Arthropoda under long-term salinity stress. Cnidaria, Chordata, and Porifera could not be included in our subgroup analysis due to low sample size. But single studies on Cnidaria, Chordata, and Porifera reveal that the main osmolytes are the same as in molluscs and annelids (Deaton and Hoffmann, 1988; Knight et al., 1992; Yancey et al., 2010; Podbielski et al., 2016).
4.4 Cellular strategies of volume regulation differ between tissue types
Salinity had a significantly different effect on inorganic and organic osmolyte concentrations between tissues (for discussion of inorganic ions see above chapter). Total organic compounds were significantly affected by salinity in all tissues. Yet, the organic osmolyte pool was less impacted in gill tissues. Also, we observed a more constrained composition of osmolytes in gills, whereas muscle tissues employed a wider range of osmolytes. Muscle tissue used more alanine and glycine, whereas taurine was used more heavily in gill tissue. Some minor compounds were more prevalent in certain tissues (such as aspartate in gills, and serine, threonine, and aspartate in intestinal tissues, and glutamine, serine, and threonine in muscle tissue). Our findings are in accordance with other comparative studies that have previously stated differences in osmolyte composition and CVR capacity between tissues (Florkin and Schoffeniels, 1965; Koyama et al., 2015). CVR is reported to be nearly perfect in gills and byssus retractor muscles and incomplete in nervous tissues and adductor muscles (Potts, 1958; Lange, 1968; Willmer, 1978; Gainey, 1994). Differences in osmolyte concentration or their transporters between tissues potentially reflect differences in metabolic pathways between tissues or the functional roles of specific osmolytes in different tissues (Hosoi et al., 2007; Inoue et al., 2008).
4.5 Characteristics of major osmolytes
Given the universal usage of certain organic osmolytes across the taxa under study, the question arises what makes these solutes appropriate osmolytes. Osmolyte usage is likely governed by compatibility and cytoprotective functions of compounds (Somero and Yancey, 2011). Osmolytes have physiochemical similarities with ions from the Hofmeister series. Compatible osmolytes are substances that are neither strongly denaturing nor extremely stabilizing (Somero et al., 2017). Yet, not all organic osmolytes are compatible. Some counteracting solutes occur in combination (i.e., TMAO and urea), canceling out the perturbing effect of each other. According to the Hofmeister series, destabilizing ions are, for example, chloride, sodium, calcium, and urea, whereas potassium, carboxylate, sulfate, phosphate, and methylammonium ions have stabilizing effects (ranked from weak to strong effects, respectively). Hence, organic osmolytes can be considered more compatible compared to inorganic ions. However, not all inorganic ions are protein destabilizers, and while they may be less preferred, weak protein destabilizers—such as chloride or sodium—can be tolerated in certain amounts.
The primary organic osmolytes identified in this study (alanine, glycine, and betaine) possess stabilizing effects on protein structures and presumably also membrane systems (Yancey, 1994; Somero and Yancey, 2011). The major FAA-osmolytes are mainly non-essential amino acids (i.e., glycine, alanine, or taurine) and were shown to not affect protein function at normal cellular concentrations. Glycine proved least inhibitory to enzyme-reaction compared to other FAAs (Bowlus and Somero, 1979). Taurine is known for its inert nature (Bishop et al., 1981) and along with betaine, counteracts negative impacts of salts on protein structure and function (Yancey, 1978; Buche et al., 1990), whereas other organic osmolytes seem inferior or even harmful in structure and function.
Another criterion for osmolyte selection is metabolic availability and energetic cost. The usage of end products of nitrogen metabolism is a cost-effective way to mobilize organic osmolytes (Bishop et al., 1981). Alanine is commonly present in osmolyte pools, likely because it is derived from components of key metabolic pathways such as glycolysis and the citric acid cycle. Thus it is thus fast and easy to produce via transamination in all cells. Alanine has also been reported as a first responder in CVR, which is later substituted by structurally more complex organic osmolytes such as taurine (Baginski and Pierce, 1977; Bishop et al., 1994). For taurine to be a metabolic end product, it must be produced via the degradation of methionine and cysteine (Allen and Garrett, 1972; Zhao et al., 2017). Betaine is derived from the catabolism of the common metabolic products, choline and glycine (Pierce et al., 1992). Glycine, a simple, low molecular mass amino acid, has been shown to be broadly used as an osmolyte across taxa and tissues. Despite its ubiquity, the metabolism of glycine and its derivatives (such as betaine) is generally poorly studied in invertebrates (Awapara, 1962; de Vooys, 1991).
At this point, it may be worth mentioning that organic osmolytes often have other beneficial characteristics. Their functional role may thus have multiple purposes in addition to cellular osmoregulation, such as resistance to desiccation, heat/freeze tolerance, scavenging of reactive oxygen species, or resistance to hydrostatic pressure (Yancey, 2005; Somero et al., 2017).
4.6 Estimation of complete intracellular osmolyte budgets
To estimate the degree of involvement of inorganic and organic osmolytes in cellular volume regulation (CVR), we examined the osmolyte pool sizes of tissues. The inorganic and organic pools changed similarly during salinity acclimation. Thus, the meta-analysis showed no salinity effect on the ratio of inorganic to organic substances. However, these conclusions have to be considered with caution because they are based on low sample sizes of exclusively mollusc species and were not normalized to the sum of the measured compounds.
There are a number of requirements to estimate a complete tissue osmolyte budget. 1) It is necessary to measure both inorganic and organic compounds. 2) The estimates of organic and inorganic pool sizes should comprise all relevant compounds including the main inorganic anions and cations, and a variety of organic osmolytes, namely methylamines and methylsulfonium compounds, FAAs, sugars, polyols, and urea (Hochachka and Somero, 2002). 3) The contribution of ECS to tissue solute and water content needs to be accounted for. 4) In addition, intracellular osmolality should be measured in order to determine whether important osmolytes might have been omitted.
Our systematic review revealed that no study fulfilled all of these criteria. Only three studies complied with the first requirement and quantified inorganic (anions and cations) as well as organic osmolytes in tissues following long-term acclimation to different salinity regimes (Figure 7). However, none of these studies constructed a complete budget. Potts (1958) disregarded all organic osmolytes other than FAAs, while Silva (1992) determined the concentration of inorganic osmolytes and FAAs plus betaine, but not that of other methylamines or methylsulfonium compounds. It should further be noted that these studies measured the total FAA pool, which also includes solutes that contribute to intracellular osmolality but do not change in concentration with salinity. Only one study reported total osmolality. Figure 7 shows that a large portion of the total solute pool can remain undetected if total osmolality is not used as a control. Since Turgeon (1973) only determined FAAs, the unknown portion of the osmolyte pool likely consisted of methylamines or methylsulfonium compounds. ECS was also not considered in the latter study. The research by Potts (1958) and Silva (1992) highlights the importance of considering the ECS in estimation of intracellular osmolyte concentrations. Inorganic ion concentrations were higher in tissue samples of Mytilus sp. compared to the corresponding intracellular values owing to the high contribution of ECS ions (Figure 7).
The intracellular organic osmolyte pool in these three mollusk studies accounted for approximately 30% of the overall osmolyte pool in tissue and 45% in the intracellular space. Previous studies estimated its proportion to account for ~50%–70% (Florkin and Schoffeniels, 1965; de Vooys, 1991; Yancey, 2005; Somero and Yancey, 2011). Yet, many estimates are based on data obtained from osmoregulators—often arthropods or teleosts. An experiment on the annelid Arenicola marina tissues revealed that inorganic ions constitute 60% and organic substances 40% of total osmolality (Weber and Spaargaren, 1979). In conclusion, the overall share of organic osmolytes in CVR has likely been overestimated, while the role of inorganic ions has been underrated. Nevertheless, CVR is a product of inorganic and organic osmolyte regulation combined, and more studies are needed to quantify the relative importance of organic vs. inorganic osmolytes in long-term salinity acclimation processes across taxa.
4.7 Best-practice guidelines
During this systematic review, we encountered numerous methodological problems in published salinity tolerance research, which led us to make recommendations for best-practices in future research of CVR in marine osmoconformers.
I. Measure intracellular inorganic osmolyte composition.
Inorganic osmolytes are estimated to account for half of the intracellular total osmolyte pool, but they are often disregarded. Results from our meta-analysis indicate the involvement of both pools in salinity acclimation. However, little is known about the role of intracellular inorganic ions during long-term salinity acclimation, while the use of an organic osmolyte pool is well established. We strongly advocate the need to measure both components of the osmolyte pool in tissue samples. To derive intracellular ion concentrations, tissue ion concentrations, extracellular ion concentrations, and ECS volume need to be measured (Figure 1). Tissue cation concentrations have been measured in the past via flame photometry (Neufeld and Wright, 1998; Sanders, 2018) and anions via numerous specific methods for each individual anion. Chloride was measured via titration methods (Turgeon, 1973; Silva, 1992) or a microdiffusion method (Potts, 1958). Sulfate can be estimated gravimetrically, and phosphate colorimetrically (Potts, 1958). Our group has recently developed a novel protocol using ion chromatography to determine anion concentrations in invertebrate tissues (Podbielski et al., 2022).
II. Analyze intracellular organic osmolytes using untargeted methods
Our literature analysis revealed that publication bias toward measuring FAAs likely resulted from the targeted metabolomic high performance liquid chromatography (HPLC) approaches common at the time these studies were published (1958–1992). This is a drawback since methylamines and methylsulfonium compounds remain undetected, although these substances account for a large portion (35%–75%) of the organic osmolyte pool (Pierce et al., 1984; Yancey et al., 2002; Podbielski et al., 2016). Recent developments in untargeted metabolomics using HPLC, gas chromatography–mass spectrometry (GC–MS), liquid chromatography–mass spectrometry (LC–MS), or proton nuclear magnetic resonance (1H-NMR) allow the capturing and quantification of a large spectrum of solutes, including novel or uncommon organic osmolytes. Untargeted methods also provide a solid basis for the exploratory analysis of poorly studied species. Another key shortcoming of past osmolyte work is that many studies did not distinguish whether substances were non-existent or undetectable with the chosen methodological approach. Thus, studies should report on the capabilities and constraints of their methods. Finally, compounds not detected (or detected at very low concentrations) should also be reported, even when not significantly impacted by treatments.
III. Measure total osmolality of tissues and extracellular fluids.
Intracellular osmolality along with total inorganic and organic pool size can help determine whether crucial portions of the osmolyte pool have remained undetected (Figure 7). Total osmolality of tissue homogenates is easily measured using an osmometer (Turgeon, 1973). To distinguish between extracellular and intracellular osmolality, the osmolality of extracellular fluid samples (blood, coelomic fluid, hemolymph) will have to be measured and the volume of the extracellular space in the tissue will have to be determined (Figure 1).
IV. Account for extracellular space
Osmolyte composition differs between cells and extracellular fluids, but in organic osmolyte studies, the ECS is often disregarded. The ECS can make up a large portion of the tissues (10%–80% of tissue mass). In the ECS, some inorganic ions (sodium, chloride, and calcium) are more highly concentrated (Figure 1), whereas organic osmolytes are negligible. Thus, the determination of the ECS is crucial for the reliable estimation of intracellular organic and inorganic osmolytes. Traditionally, the determination of the extracellular volume has been achieved by measuring the dilution of a known volume of radioactively labeled tracers, such as 3H-/14C-inulin, 3H-/14C-dextran, 13C-sucrose, I131-albumin, or 14C-polyethylene glycol injected into the ECS (Kruhøffer, 1946; Simon et al., 1964; Murphy and Dietz, 1976; Willmer, 1978; Thompson et al., 1980; Michaelidis et al., 2005). Recent advancements in labeling techniques permit non-radioactive assessment of ECS volume using fluorescent tracers such as FITC-inulin (Krol et al., 1999; Binder et al., 2004; Verkman, 2013).
V. Report major statistical parameters and outcomes and deposit data to public databases
Gaps in the documentation of experimental results led to the exclusion of many studies in this meta-analysis. This applied largely to older studies but also to recent publications. Absolute osmolyte concentrations and statistical parameters such as sample size, mean, and variance, or appropriate terms for other data types (such as risk ratios, risk difference, or odds ratio and sample size for binary data, or correlation coefficient and sample size for correlations) should be reported (see Borenstein et al., 2011 for a comprehensive introduction to different data types). A complete record of the statistical analysis is necessary. All data and metadata should ideally be deposited in publicly available databases (e.g., Pangaea) to facilitate future use.
VI. Use standard units
To enable direct comparison between studies, researchers should either use the same units or provide all the necessary information for the unit conversion. We recommend the use of mmol/L for extracellular fluids and intracellular water and mmol/kg dry weight for tissues and whole animal samples.
4.8 Future research
This meta-analysis revealed large gaps in knowledge and fragmented data in extracellular and cellular osmolyte dynamics. Our meta-analysis determined that taxonomy is an important factor influencing CVR and osmolyte adjustment in relation to salinity. In the analyzed literature, there is a large taxonomic bias toward Mollusca and more specifically Bivalvia (Figures 3D, E). When choosing study species, a wider taxonomic coverage should be considered. While it is impossible to study various species, we propose the use of model organisms as representatives for certain taxa. Here, it makes sense to focus efforts on models already used in other biological sub-disciplines, especially those with broad distribution (e.g., Placozoa: Trichoplax sp., Porifera: Amphimedon queenslandica, Cnidaria: Nematostella vectensis, Ctenophora: Mnemiopsis leidyi, Echinodermata: Strongylocentrotus purpuratus, Annelida: Platynereis dumerilii, Platyhelminthes: Macrostomum lignano, Xenacoelomorpha: Symsagittifera roscoffensis, Tunicata: Ciona intestinalis, Bivalvia: Mytilus edulis, Gastropoda: Aplysia sp., Cephalopoda: Loligo pealei, Chordata: Branchiostoma floridae, etc.).
It remains further unclear what defines the capacity for salinity tolerance in osmoconforming animals. Initially, we wanted to distinguish between different physiotypes (stenohaline, oligohaline, and euryhaline tolerance ranges). However, it was impossible to conduct a meta-analysis due to the lack of representation of stenohaline and oligohaline study organisms in the literature. We thus suggest choosing closely related study organisms that are characterized by different physiotypes to reveal what determines differences in salinity tolerance capacity.
Overall, this study only considered adult stages of osmoconforming species because data on larvae or juveniles is rudimentary. Nevertheless, future research should consider larval and juvenile ontogenetic stages. The limiting factor for successful acclimation to low salinity might also depend on the physiological capacities of the larval or juvenile stages (Casties et al., 2015). The processes involved in CVR, such as compound synthesis, membrane transport, membrane permeability regulation, and osmotic sensing, are poorly studied and are a promising field for future research (Huggins and Munday, 1968; Smith and Pierce, 1987; Hosoi et al., 2007; Lin et al., 2016).
5 Conclusion
In conclusion, this systematic review and meta-analysis has revealed that:
1. The osmolyte classes employed by marine osmoconforming invertebrates are monovalent inorganic ions, methylamines and methylated sulfonium compounds, free amino acids and derivatives.
2. Certain organic osmolytes are employed across taxa (i.e., alanine, betaine, glycine, and taurine) but in different magnitudes, whereas accompanying minor osmolytes differ between taxa and tissue type. Echinodermata and Mollusca differ in their main osmolyte, whereas Annelida does not use a single main osmolyte.
3. We further found an extreme mollusk bias in the osmolyte literature.
4. We hypothesize that methylamines play a similar or more important role than FAAs in the organic osmolyte pool, but data are insufficient for meta-analysis.
5. The tissue sodium ion pool is modulated during long-term salinity acclimation, but changes in the concentration of other inorganic ions have been poorly studied.
Data availability statement
The original contributions presented in the study are included in the article/Supplementary Material. Further inquiries can be directed to the corresponding author.
Author contributions
IP designed and conducted the systematic review. IP conducted the meta-analysis and wrote the paper. FM, LS, and TS edited the manuscript. All authors listed have made a substantial, direct, and intellectual contribution to the work and approved it for publication.
Acknowledgments
The authors would like to thank Dr. Tamar Guy-Haim for advice on the meta-analysis procedure. The authors also thank the GEOMAR library staff for help with retrieving references.
Conflict of interest
The authors declare that the research was conducted in the absence of any commercial or financial relationships that could be construed as a potential conflict of interest.
Publisher’s note
All claims expressed in this article are solely those of the authors and do not necessarily represent those of their affiliated organizations, or those of the publisher, the editors and the reviewers. Any product that may be evaluated in this article, or claim that may be made by its manufacturer, is not guaranteed or endorsed by the publisher.
Supplementary material
The Supplementary Material for this article can be found online at: https://www.frontiersin.org/articles/10.3389/fmars.2022.934378/full#supplementary-material
References
Allen J. A., Garrett M. R. (1972). Studies on taurine in the euryhaline bivalve mya arenaria. Comp. Biochem. Physiol. Part A: Physiol. 41 (2), 307–317. doi: 10.1016/0300-9629(72)90062-X
Awapara J. (1962). Free amino acids in invertebratres : A comparative study of their distribution. In: Amino Acid Pools : Distribution, Formation and Function of Free Amino Acids. , ed. by Holden J. T.. Amsterdam; Elsevier Publishing Company, pp. 158–175.
Baginski R. M., Pierce S. K. (1977). The time course of intracellular free amino acid accumulation in tissues of modiolus demissus during high salinity adaptations. Comp. Biochem. Physiol. Part A: Physiol. 57 (4), 407–412. doi: 10.1016/0300-9629(77)90137-2
Balduzzi S., Rücker G., Schwarzer G. (2019). How to perform a meta-analysis with r: A practical tutorial. Evidence-Based Ment. Health 22 (4), 153–160. doi: 10.1136/ebmental-2019-300117
Ballantyne J. S., Berges J. (1991). Enzyme-activities of gill, hepatopancreas, mantle, and adductor muscle. Can. J. Fisheries Aquat. Sci. 48 (6), 1117–1123. doi: 10.1139/f91-133
Ballantyne J. S., Moyes C. D. (1987). Osmotic effects on fatty acid, pyruvate, and ketone body oxidation in oyster gill mitochondria. Physiol. Zoology 60 (6), 713–721. doi: 10.1086/physzool.60.6.30159987
Benson-Rodenbough B., Ellington W. R. (1982). Responses of the euryhaline sea anemone bunodosoma cavernata (bosc) (anthozoa, actinaria, actiniidae) to osmotic stress. Comp. Biochem. Physiol. Part A: Physiol. 72 (4), 731–735. doi: 10.1016/0300-9629(82)90157-8
Berger V., Kharazova A. (1997). Mechanisms of salinity adaptations in marine molluscs. HYDROBIOLOGIA 355, 115–126. doi: 10.1023/A:1003023322263
Berger V. Y., Khlebovich V. V., Kovaleva N. M., Natochin Y. (1978). The changes of ionic composition and cell volume during adaptation of molluscs (Littorina) to lowered salinity. Comp. Biochem. Physiol. Part A: Physiol. 60 (4), 447–452. doi: 10.1016/0300-9629(78)90015-4
Binder D. K., Papadopoulos M. C., Haggie P. M., Verkman A. S. (2004). In vivo measurement of brain extracellular space diffusion by cortical surface photobleaching. J. Neurosci. 24 (37), 8049–8056. doi: 10.1523/JNEUROSCI.2294-04.2004
Bintanja R., Selten F. M. (2014). Future increases in Arctic precipitation linked to local evaporation and sea-ice retreat. Nature 509 (7501), 479–482. doi: 10.1038/nature13259
Bishop S. H., Greenwalt D. E., Burcham J. M. (1981). Amino acid cycling in ribbed mussel tissues subjected to hyperosmotic shock. J. Exp. Zoology Part A: Ecol. Genet. Physiol. 215 (3), 277–287. doi: 10.1002/jez.1402150306
Bishop C. D., Lee K. J., Watts S. A. (1994). A comparison of osmolality and specific ion concentrations in the fluid compartments of the regular sea urchin lytechinus variegatus lamarck (Echinodermata: Echinoidea) in varying salinities. Comp. Biochem. Physiol. Part A: Physiol. 108 (4), 497–502. doi: 10.1016/0300-9629(94)90333-6
Borenstein M., Hedges L. V., Higgins J. P. T., Rothstein H. R. (2011). Introduction to meta-analysis (The Atrium, Southern Gate, Chichester, West Sussex, PO19 8SQ, United Kingdom: John Wiley & Sons Ltd).
Bowlus R. D., Somero G. N. (1979). Solute compatibility with enzyme function and structure: Rationales for the selection of osmotic agents and end-products of anaerobic metabolism in marine invertebrates. J. Exp. Zoology 208 (2), 137–151. doi: 10.1002/jez.1402080202
Braby C. E., Somero G. N. (2006). Ecological gradients and relative abundance of native (<Emphasis Type=“Italic”>Mytilus trossulus</Emphasis>) and invasive (<Emphasis Type=“Italic”>Mytilus galloprovincialis</Emphasis>) blue mussels in the California hybrid zone. Mar. Biol. 148 (6), 1249–1262. doi: 10.1007/s00227-005-0177-0
Buche A., Colson P., Houssier C. (1990). Organic osmotic effectors and chromatin structure. J. Biomolecular Structure Dynamics 8 (3), 601–618. doi: 10.1080/07391102.1990.10507831
Burg M. B., Ferraris J. D. (2008). Intracellular organic osmolytes: function and regulation. J. Biol. Chem. 283 (12), 7309–7313. doi: 10.1074/jbc.R700042200
Burg M. B., Ferraris J. D., Dmitrieva N. I. (2007). Cellular response to hyperosmotic stresses. Physiol. Rev. 87 (4), 1441–1474. doi: 10.1152/physrev.00056.2006
Casties I., Clemmesen C., Melzner F., Thomsen J. (2015). Salinity dependence of recruitment success of the sea star asterias rubens in the brackish western Baltic Sea. Helgoland Mar. Res. 69 (2), 169–175. doi: 10.1007/s10152-015-0425-8
Darling J. A., Bagley M. J., Roman J. O. E., Tepolt C. K., Geller J. B. (2008). Genetic patterns across multiple introductions of the globally invasive crab genus carcinus. Mol. Ecol. 17 (23), 4992–5007. doi: 10.1111/j.1365-294X.2008.03978.x
Deaton L. E., Hoffmann R. J. (1988). Hypoosmotic volume regulation in the sea anemone metridium senile. Comp. Biochem. Physiol. Part C: Comp. Pharmacol. 91 (1), 187–191. doi: 10.1016/0742-8413(88)90185-5
de Vooys C. G. N. (1991). Anaerobic metabolism in sublittoral living mytilus galloprovincialis in the mediterranean–IV. role of amino acids in adaptation to low salinities during anaerobiosis and aerobiosis. Comp. Biochem. Physiol. Part A: Physiol. 100 (2), 423–431. doi: 10.1016/0300-9629(91)90494-W
Duval S., Tweedie R. (2000). Trim and fill: A simple funnel-plot–based method of testing and adjusting for publication bias in meta-analysis. Biometrics 56 (2), 455–463. doi: 10.1111/j.0006-341X.2000.00455.x
Du Y., Zhang Y., Shi J. (2019). Relationship between sea surface salinity and ocean circulation and climate change. Sci. China Earth Sci. 62 (5), 771–782. doi: 10.1007/s11430-018-9276-6
Egger M., Smith G. D., Schneider M., Minder C. (1997). Bias in meta-analysis detected by a simple, graphical test. Bmj 315 (7109), 629–634. doi: 10.1136/bmj.315.7109.629
Emerson D. N. (1969). Influence of salinity of ammonia excretion rates and tissue constituents of euryhaline invertebrates. Comp. Biochem. Physiol. 29 (3), 1115–1133. doi: 10.1016/0010-406X(69)91017-2
Florkin M., Schoffeniels E. (1965) Euryhalinity and the concept of physiological radiation, in Studies in Comparative Bio-chemistry (Munday K. A., ed), pp. 6–40. Pergamon, London.
Gainey L. (1994). Volume regulation in 3 species of marine mussels. J. Exp. Mar. Biol. Ecol. 181 (2), 201–211. doi: 10.1016/0022-0981(94)90128-7
George P., Damodaran R. (1999). The intracellular fluid isosmotic regulation in yellow clam sunetta scripta L.(Mollusca: Bivalvia) acclimated to different salnities. Indian J. Mar. Sci. 28, 83–86.
Gilles R., Delpire E. (1997). Variations in salinity, osmolarity, and water availability: vertebrates and invertebrates. In: Handbook of Physiology: Comparative Physiology, Bethesda, MD: Am. Physiol. Soc, sect. 13, vol. II, chapt. 22, p. 1523–1586.
Gräwe U., Friedland R., Burchard H. (2013). The future of the western Baltic Sea: Two possible scenarios. Ocean Dynamics 63 (8), 901–921. doi: 10.1007/s10236-013-0634-0
Greenwalt D. E. (1981). “Role of amino acids in cell volume control in the ribbed mussel: alanine and proline metabolism”. Retrospective Theses and Dissertations. 7170. #Available at: https://lib.dr.iastate.edu/rtd/7170
Haapkylä J., Unsworth R. K. F., Flavell M., Bourne D. G., Schaffelke B., Willis B. L. (2011). Seasonal rainfall and runoff promote coral disease on an inshore reef. PloS One 6 (2), e16893. doi: 10.1371/journal.pone.0016893
Harrer M., Cuijpers P., Furukawa T. A., Ebert D. D. (2021). Doing meta-analysis with r: A hands-on guide. Chapman and Hall/CRC: Boca Raton.
Häussinger D. (1996). The role of cellular hydration in the regulation of cell function. Biochem. J. 313 (3), 697–710. doi: 10.1042/bj3130697
Hedges L. V. (1981). Distribution theory for glass’s estimator of effect size and related estimators. J. Educ. Stat 6 (2), 107–128. doi: 10.3102/10769986006002107
Hedges L. V., Olkin I. (1985). Statistical methods for meta-analysis (Orlando, Florida, London, UK: Academic Press Inc.).
Hedges L. V., Tipton E., Johnson M. C. (2010). Robust variance estimation in meta-regression with dependent effect size estimates. Res. Synthesis Methods 1 (1), 39–65. doi: 10.1002/jrsm.5
Hiong K. C., Peh W. Y. X., Loong A. M., Wong W. P., Chew S. F., Ip Y. K. (2004). Exposure to air, but not seawater, increases the glutamine content and the glutamine synthetase activity in the marsh clam polymesoda expansa. J. Exp. Biol. 207 (26), 4605–4614. doi: 10.1242/jel.01334
Hochachka P. W., Somero G. N. (2002). Biochemical adaptation: Mechanism and process in physiological evolution (Oxford University Press: New York).
Hoffmann E. K., Lambert I. H., Pedersen S. F. (2009). Physiology of cell volume regulation in vertebrates. Physiol. Rev. 89 (1), 193–277. doi: 10.1152/physrev.00037.2007
Hong B., Shen J. (2012). Responses of estuarine salinity and transport processes to potential future sea-level rise in the Chesapeake bay. Estuarine Coast. Shelf Sci. 104–105, 33–45. doi: 10.1016/j.ecss.2012.03.014
Hosoi M., Shinzato C., Takagi M., Hosoi-Tanabe S., Sawada H., Terasawa E., et al. (2007). Taurine transporter from the giant pacific oyster crassostrea gigas: Function and expression in response to hyper- and hypo-osmotic stress. Fisheries Sci. 73 (2), 385–394. doi: 10.1111/j.1444-2906.2007.01346.x
Huggins A. K., Munday K. A. (1968). “Crustacean metabolism,” in Advances in comparative physiology and biochemistry#73, 271–378.
Inoue K., Tsukuda K., Koito T., Miyazaki Y., Hosoi M., Kado R., et al. (2008). Possible role of a taurine transporter in the deep-sea mussel bathymodiolus septemdierum in adaptation to hydrothermal vents. FEBS Lett. 582 (10), 1542–1546. doi: 10.1016/j.febslet.2008.03.052
IPCC (2014). Climate Change 2014: Synthesis Report. Contribution of Working Groups I, II and III to the Fifth Assessment Report of the Intergovernmental Panel on Climate Change [Core Writing Team, Pachauri R. K., Meyer L. A. (eds.)]. Geneva, Switzerland; IPCC, 151 pp. ISBN 978-92-9169-143-2
Kapper M., Stickle W., Blakeney E. (1985). Volume regulation and nitrogen metabolism in the muricid gastropod thais haemastoma. Biol. Bull. 169 (2), 458–475. doi: 10.2307/1541495
Khlebovich V. V., Aladin N. V. (2010). The salinity factor in animal life. Herald Russian Acad. Sci. 80 (3), 299–304. doi: 10.1134/S1019331610030172
Kinne R. K. H. (1993). The role of organic osmolytes in osmoregulation: From bacteria to mammals. J. Exp. Zoology 265 (4), 346–355. doi: 10.1002/jez.1402650403
Kirschner L. B. (1991). Water and ions In: Environmental and Metabolic Animal Physiology (John Wiley and Sons, Ltd.: New York), 13–107.
Knight P.-A., Loomis S. H., Fell P. E. (1992). The use of free amino acids for osmotic compensation by the euryhaline sponge microciona prolifera (Ellis and sollander). J. Exp. Mar. Biol. Ecol. 163 (1), 111–123. doi: 10.1016/0022-0981(92)90150-9
Koricheva J., Gurevitch J., Mengersen K. (2013). Handbook of meta-analysis in ecology and evolution (Princeton University Press: Princeton, Oxford).
Koyama H., Okamoto S., Watanabe N., Hoshino N., Jimbo M., Yasumoto K., et al. (2015). Dynamic changes in the accumulation of metabolites in brackish water clam corbicula japonica associated with alternation of salinity. Comp. Biochem. Physiol. B-Biochem Mol. Biol. 181, 59–70. doi: 10.1016/j.cbpb.2014.11.007
Krishnamoorthi B., Krishnaswamy S. (1965). Physiological studies on marphysa gravelyi southern v. regulation of chlorides, sodium, potassium and total free amino acids. Helgoländer Wissenschaftliche Meeresuntersuchungen 12 (3), 315. doi: 10.1007/BF01880937
Krol A., Maresca J., Dewhirst M. W., Yuan F. (1999). Available volume fraction of macromolecules in the extravascular space of a fibrosarcoma: Implications for drug delivery. Cancer Res. 59 (16), 4136–4141.
Kruhøffer P. (1946). Inulin as an indicator for the extracellular space. Acta Physiol Scand 11 (1), 16–36. doi: 10.1111/j.1748-1716.1946.tb00325.x
Kültz D. (2012). The combinatorial nature of osmosensing in fishes. Physiology 27 (4), 259–275. doi: 10.1152/physiol.00014.2012
Lange R. (1968). The relation between the oxygen consumption of isolated gill tissue of the common mussel, mytilus edvlis l and salinity. J. Exp. Mar. Biol. Ecol. 2 (1), 37–45. doi: 10.1016/0022-0981(68)90012-9
Lange R. (1970). Isosmotic intracellular regulation and euryhalinity in marine bivalves. J. Exp. Mar. Biol. Ecol. 5 (2), 170–179. doi: 10.1016/0022-0981(70)90015-8
Lin C.-H., Yeh P.-L., Lee T.-H. (2016). Ionic and amino acid regulation in hard clam (Meretrix lusoria) in response to salinity challenges. Front. Physiol. 7. doi: 10.3389/fphys.2016.00368
Lucu C., Devescovi M. (1999). Osmoregulation and branchial Na+,K+-ATPase in the lobster homarus gammarus acclimated to dilute seawater. J. Of Exp. Mar. Biol. And Ecol. 234 (2), 291–304. doi: 10.1016/S0022-0981(98)00152-X
Martin A. W., Harrison F. M., Huston M. J., Stewart D. M. (1958). The blood volumes of some representative molluscs. J. Exp. Biol. 35 (2), 260–279. doi: 10.1242/jeb.35.2.260
Meredith M. P., Brandon M. A., Wallace M. I., Clarke A., Leng M. J., Renfrew I. A., et al. (2008). Variability in the freshwater balance of northern Marguerite bay, Antarctic peninsula: Results from δ18O. Deep Sea Res. Part II: Topical Stud. Oceanogr 55 (3–4), 309–322. doi: 10.1016/j.dsr2.2007.11.005
Michaelidis B., Haas D., Grieshaber M. K. (2005). Extracellular and intracellular acid-base status with regard to the energy metabolism in the oyster crassostrea gigas during exposure to air. Physiol. Biochem. Zoology: Ecol. Evolutionary Approaches 78 (3), 373–383. doi: 10.1086/430223
Moon H.-W., Wan Hussin W. M. R., Kim H.-C., Ahn I.-Y. (2015). The impacts of climate change on Antarctic nearshore mega-epifaunal benthic assemblages in a glacial fjord on king George island: Responses and implications. Ecol. Indic. 57, 280–292. doi: 10.1016/j.ecolind.2015.04.031
Mulkidjanian A. Y., Bychkov A. Y., Dibrova D. V., Galperin M. Y., Koonin E. V. (2012). Origin of first cells at terrestrial, anoxic geothermal fields. Proc. Natl. Acad. Sci. 109 (14), E821–E830. doi: 10.1073/pnas.1117774109
Murphy W. A., Dietz T. H. (1976). The effects of salt depletion on blood and tissue ion concentrations in the freshwater mussel, ligumia subrostrata (Say). J. Comp. Physiol. B: Biochem Systemic Environ. Physiol. 108 (3), 233–242. doi: 10.1007/BF00691672
Natochin Y., Berger V. J. (1979). Ionic composition of mollusk cells–evolutionary and ecologic aspects. Zhurnal evoliutsionnoi biokhimii i fiziologii 15 (3), 295–302.
Natochin Y., Berger V. Y., Khlebovich V. V., Lavrova E. A., Michailova O. (1979). The participation of electrolytes in adaptation mechanisms of intertidal molluscs’ cells to altered salinity–ScienceDirect. Comp. Biochem. Physiol. 63A, 115–119. doi: 10.1016/0300-9629(79)90636-4
Naughten K. A., Meissner K. J., Galton-Fenzi B. K., England M. H., Timmermann R., Hellmer H. H. (2018). Future projections of Antarctic ice shelf melting based on CMIP5 scenarios. J. Climate 31 (13), 5243–5261. doi: 10.1175/JCLI-D-17-0854.1
Neufeld D., Wright S. (1996). Salinity change and cell volume: The response of tissues from the estuarine mussel geukensia demissa. J. Of Exp. Biol. 199 (7), 1619–1630. doi: 10.1242/jeb.199.7.1619
Neufeld D., Wright S. (1998). Effect of cyclical salinity changes on cell volume and function in geukensia demissa gills. J. Exp. Biol. 201 (9), 1421–1431. doi: 10.1242/jeb.201.9.1421
Oglesby L. C. (1981). Volume regulation in aquatic invertebrates. J. Exp. Zoology 215 (3), 289–301. doi: 10.1002/jez.1402150307
Palmer B. F. (2015). Regulation of potassium homeostasis. Clin. J. Am. Soc. Nephrol. 10 (6), 1050–1060. doi: 10.2215/CJN.08580813
Peters J. L., Sutton A. J., Jones D. R., Abrams K. R., Rushton L. (2007). Performance of the trim and fill method in the presence of publication bias and between-study heterogeneity. Stat Med. 26 (25), 4544–4562. doi: 10.1002/sim.2889
Pierce S. K. (1970). The water balance of modiolus (Mollusca: Bivalvia: Mytilidae): Osmotic concentrations in changing salinities. Comp. Biochem. Physiol. 36 (3), 521–533. doi: 10.1016/0010-406X(70)91028-5
Pierce J. S.K. (1971). A source of solute for volume regulation in marine mussels. Comp. Biochem. Physiol. Part A: Physiol. 38 (3), 619–635. doi: 10.1016/0300-9629(71)90129-0
Pierce S. K., Edwards S. C., Mazzocchi P. H., Klingler L. J., Warren M. K. (1984). Proline betaine: A unique osmolyte in an extremely euryhaline osmoconformer. Biol. Bull. 167 (2), 495–500. doi: 10.2307/1541294
Pierce S. K., Rowland-Faux L. M., O’Brien S. M. (1992). Different salinity tolerance mechanisms in atlantic and chesapeake bay conspecific oysters: glycine betaine and amino acid pool variations. Mar. Biol. 113 (1), 107–115. doi: 10.1007/BF00367644
Podbielski I., Bock C., Lenz M., Melzner F. (2016). Using the critical salinity (Scrit) concept to predict invasion potential of the anemone diadumene lineata in the Baltic Sea. Mar. Biol. 163 (11), 227. doi: 10.1007/s00227-016-2989-5
Podbielski I., Hajati M. C., Bleich M., Melzner F., Bock C., Hiebenthal C. (2022). Capacity for cellular osmoregulation defines critical salinity of marine invertebrates at low salinity. Front. Mar. Sci. 9. doi: 10.3389/fmars.2022.898364
Potts W. T. W. (1958). The inorganic and amino acid composition of some lamellibranch muscles. J. Exp. Biol. 35 (4), 749–764. doi: 10.1242/jeb.35.4.749
Prosser C. L. (1991). Comparative animal physiology, environmental and metabolic animal physiology (John Wiley and Sons: New York).
Ran Z., Li S., Zhang R., Xu J., Liao K., Yu X., et al. (2017). Proximate, amino acid and lipid compositions in sinonovacula constricta (Lamarck) reared at different salinities. J. Sci. Food Agric. 97 (13), 4476–4483. doi: 10.1002/jsfa.8311
Reed R. H., Warr S. R., Richardson D. L., Moore D. J., Stewart W. D. (1985). Multiphasic osmotic adjustment in a euryhaline cyanobacterium. FEMS Microbiol. Lett. 28 (3), 225–229. doi: 10.1111/j.1574-6968.1985.tb00796.x
Rivera-Ingraham G. A., Lignot J.-H. (2017). Osmoregulation, bioenergetics and oxidative stress in coastal marine invertebrates: Raising the questions for future research. J. Exp. Biol. 220 (10), 1749–1760. doi: 10.1242/jeb.135624
Rosenberg M. S. (2005). The file-drawer problem revisited: A general weighted method for calculating fail-safe numbers in meta-analysis. Evolution 59 (2), 464–468. doi: 10.1111/j.0014-3820.2005.tb01004.x
Sanders T. (2018). Bioenergetik der kalzifizierung in mytiliden muscheln entlang des salinitätsgradienten der ostsee [Dissertation] (Christian-Albrechts-Universität zu Kiel). Available at: https://macau.uni-kiel.de/receive/diss_mods_00023280
Sardini A., Amey J. S., Weylandt K.-H., Nobles M., Valverde M. A., Higgins C. F. (2003). Cell volume regulation and swelling-activated chloride channels. Biochim. Biophys. Acta (BBA)-Biomembranes 1618 (2), 153–162. doi: 10.1016/j.bbamem.2003.10.008
Sarkissian I. V. (1974). Regulation by salt of activity of citrate synthases from osmoregulators and osmoconformers. Trans. New York Acad. Sci. 36 (8 Series II), 775–782. doi: 10.1111/j.2164-0947.1974.tb01609.x
Sarkissian I. V., Gomolinski E. (1976). Regulation of malate dehydrogenase from an osmoconformer by salt. Comp. Biochem. Physiol. Part B: Comp. Biochem. 53 (2), 191–194. doi: 10.1016/0305-0491(76)90034-1
Schmittmann L. (2017). Local adaptation of the common sea star asterias rubens to different salinities [Master thesis] (Christian-Albrechts-Universität zu Kiel). Master Thesis. Available at: https://oceanrep.geomar.de/id/eprint/39163/1/LaraSchmittmann_thesis.pdf
Schoffeniels E. (1976). Biochemical approaches to osmoregulatory processes in Crustacea. In Zoology pp, 107–124). doi: 10.1016/B978-0-08-018767-9.50014-7
Scott A., Harrison P. L., Brooks L. O. (2013). Reduced salinity decreases the fertilization success and larval survival of two scleractinian coral species. Mar. Environ. Res. 92, 10–14. doi: 10.1016/j.marenvres.2013.08.001
Shumway S. E. (1977). The effect of fluctuating salinity on the tissue water content of eight species of bivalve molluscs. J. Comp. Physiol. B 116 (3), 269–285. doi: 10.1007/BF00689036
Silva A. L. (1992). Effect of salinity on integumental transport in marine bivalves. [Dissertation] (The University of Arizona). Available at: https://repository.arizona.edu/handle/10150/185863
Silva A. L., Wright S. H. (1994). Short-term cell volume regulation in mytilus californianus gill. J. Exp. Biol. 194 (1), 47–68. doi: 10.1242/jeb.194.1.47
Simon S. E., Muller J., Dewhurst D. J. (1964). Ionic partition in holothuroidean muscle. J. Cell. Comp. Physiol. 63 (1), 77–84. doi: 10.1002/jcp.1030630109
Simonsohn U., Nelson L. D., Simmons J. P. (2014). P-curve and effect size: Correcting for publication bias using only significant results. Perspect. psychol. Sci. 9 (6), 666–681. doi: 10.1177/1745691614553988
Simpson J. W., Allen K., Awapara J. (1959). Free amino acids in some aquatic invertebrates. Biol. Bull. 117 (2), 371–381. doi: 10.2307/1538916
Smith J. L.H., Pierce S. K. (1987). Cell volume regulation by molluscan erythrocytes during hypoosmotic stress: Ca2+ effects on ionic and organic osmolyte effluxes. Biol. Bull. 173 (2), 407–418. doi: 10.2307/1541553
Somero G. N., Bowlus R. D. (1983). “Osmolytes and metabolic end products of molluscs: The design of compatible solute systems,” in The Mollusca (Academic Press, Inc., New York, London) 2, 77–100.
Somero G. N., Lockwood B. L., Tomanek L. (2017). Biochemical adaptation: Response to environmental challenges, from life’s origins to the anthropocene (Sinauer Associates, Incorporated Publishers: Sunderland).
Somero G. N., Yancey P. H. (2011). “Osmolytes and cell-volume regulation: Physiological and evolutionary principles,” in Handbook of Physiology, Cell Physiology, Comprehensive Physiology (Oxford University Press: New York, NY, USA), 441–484. doi: 10.1002/cphy.cp140110
Souza M. M., Scemes E. (2000). Volume changes in cardiac ventricles from aplysia brasiliana upon exposure to hyposmotic shock. Comp. Biochem. Physiol. A-Molecular Integr. Physiol. 127 (1), 99–111. doi: 10.1016/S1095-6433(00)00243-9
Stucchi-Zucchi A., Salomão L.C. (1998). The ionic basis of membrane potentials and adaptation to hyposmotic stress in perna perna, an osmoconforming mollusc. Comp. Biochem. Physiol. Part A: Mol. Integr. Physiol. 121 (2), 143–148. doi: 10.1016/S1095-6433(98)10115-0
Thompson R. J., Livingstone D. R., de Zwaan A. (1980). Physiological and biochemical aspects of the valve snap and valve closure responses in the giant scallopPlacopecten magellanicus. J. Comp. Physiol. 137 (2), 97–104. doi: 10.1007/BF00689207
Torre A., Trischitta F., Corsaro C., Mallamace D., Faggio C. (2013). Digestive cells from mytilus galloprovincialis show a partial regulatory volume decrease following acute hypotonic stress through mechanisms involving inorganic ions. Cell Biochem. Funct. 31 (6), 489–495. doi: 10.1002/cbf.2925
Trenberth K. E. (2011). Changes in precipitation with climate change. Climate Res. 47 (1/2), 123–138. doi: 10.3354/cr00953
Turgeon K. W. (1973). Osmotic adjustment in two population of urosalpinx cinerea (Say) (Muricidae, prosobranchia, Gastropoda) [Dissertation] (University of New Hampshire).
Veiga M. P. T., Gutierre S. M. M., Castellano G. C., Freire C. A. (2016). Tolerance of high and low salinity in the intertidal gastropod stramonita brasiliensis (Muricidae): Behaviour and maintenance of tissue water content. J. Of Molluscan Stud. 82 (1), 154–160. doi: 10.1093/mollus/eyv044
Verkman A. S. (2013). Diffusion in the extracellular space in brain and tumors. Phys. Biol. 10 (4), 045003. doi: 10.1088/1478-3975/10/4/045003
Viechtbauer W. (2010). Conducting meta-analyses in r with the metafor package. J. Stat. Software 36 (1), 1–48. doi: 10.18637/jss.v036.i03
Villarreal F. D., Kültz D. (2015). Direct ionic regulation of the activity of myo-inositol biosynthesis enzymes in Mozambique tilapia. PloS One 10 (6), e0123212. doi: 10.1371/journal.pone.0123212
Vuorinen I., Hänninen J., Rajasilta M., Laine P., Eklund J., Montesino-Pouzols F., et al. (2015). Scenario simulations of future salinity and ecological consequences in the Baltic Sea and adjacent north Sea areas–implications for environmental monitoring. Ecol. Indic. 50, 196–205. doi: 10.1016/j.ecolind.2014.10.019
Warren M. K., Pierce S. K. (1982). Two cell volume regulatory systems in the limulus myocardium: An interaction of ions and quaternary ammonium compounds. Biol. Bull. 163 (3), 504–516. doi: 10.2307/1541460
Weber H., Maroney P. A., Baglioni C. (1977). Inhibition of protein synthesis by cl-. J. Biol. Chem. 252 (11), 4007–4010. doi: 10.1016/S0021-9258(17)40350-4
Weber R. E., Spaargaren D. H. (1979). Osmotic relations of the coelomic fluid and body wall tissues in arenicola marina subjected to salinity change. Netherlands J. Sea Res. 13 (3), 536–546. doi: 10.1016/0077-7579(79)90024-3
Wehner F., Olsen H., Tinel H., Kinne-Saffran E., Kinne R. K. H. (2003). “Cell volume regulation: Osmolytes, osmolyte transport, and signal transduction,” in Reviews of physiology, biochemistry and pharmacology (Springer: Berlin Heidelberg), 1–80. doi: 10.1007/s10254-003-0009-x
Willmer P. G. (1978). Volume regulation and solute balance in the nervous tissue of an osmoconforming bivalve (mytilus edulis). J. Exp. Biol. 77 (1), 157–179. doi: 10.1242/jeb.77.1.157
Yancey P. H. (1994). “Compatible and counteracting solutes,” in Cellular and molecular physiology of cell volume regulation (CRC press: Boca Raton).
Yancey P. H. (2004). Compatible and counteracting solutes: protecting cells from the dead sea to the deep sea. Sci. Prog. 87 (1), 1–24. doi: 10.3184/003685004783238599
Yancey P. H. (2005). Organic osmolytes as compatible, metabolic and counteracting cytoprotectants in high osmolarity and other stresses. J. Exp. Biol. 208 (15), 2819–2830. doi: 10.1242/jeb.01730
Yancey P. H., Blake W. R., Conley J. (2002). Unusual organic osmolytes in deep-sea animals: Adaptations to hydrostatic pressure and other perturbants. Comp. Biochem. Physiol. Part A: Mol. Integr. Physiol. 133 (3), 667–676. doi: 10.1016/S1095-6433(02)00182-4
Yancey P. H., Clark M. E., Hand S. C., Bowlus R. D., Somero G. N. (1982). Living with water stress: Evolution of osmolyte systems. Science 217 (4566), 1214–1222. doi: 10.1126/science.7112124
Yancey P. H., Gerringer M. E., Drazen J. C., Rowden A. A., Jamieson A. (2014). Marine fish may be biochemically constrained from inhabiting the deepest ocean depths. Proc. Natl. Acad. Sci. 111 (12), 4461–4465. doi: 10.1073/pnas.1322003111
Yancey P. H., Heppenstall M., Ly S., Andrell R. M., Gates R. D., Carter V. L., et al. (2010). Betaines and dimethylsulfoniopropionate as major osmolytes in cnidaria with endosymbiotic dinoflagellates. Physiol. Biochem. Zoology: Ecol. Evolutionary Approaches 83 (1), 167–173. doi: 10.1086/644625
Yancey P. H., Somero G. N. (1978). Urea-requiring lactate dehydrogenases of marine elasmobranch fishes. J. Comp. Physiol. 125 (2), 135–141. doi: 10.1007/BF00686749
Yu S. P. (2003). Regulation and critical role of potassium homeostasis in apoptosis. Prog. Neurobiol. 70 (4), 363–386. doi: 10.1016/S0301-0082(03)00090-X
Zhang Z.-Q. (2011). Animal biodiversity: An outline of higher-level classification and survey of taxonomic richness In Zootaxa, 3148 (1), 7–13 (Magnolia Press: Auckland)
Keywords: salinity stress, osmoconformer, cellular volume regulation, osmoregulation, osmolytes, marine invertebrates, systematic review, meta-analysis
Citation: Podbielski I, Schmittmann L, Sanders T and Melzner F (2022) Acclimation of marine invertebrate osmolyte systems to low salinity: A systematic review & meta-analysis. Front. Mar. Sci. 9:934378. doi: 10.3389/fmars.2022.934378
Received: 02 May 2022; Accepted: 20 July 2022;
Published: 02 September 2022.
Edited by:
Silvia Franzellitti, University of Bologna, ItalyCopyright © 2022 Podbielski, Schmittmann, Sanders and Melzner. This is an open-access article distributed under the terms of the Creative Commons Attribution License (CC BY). The use, distribution or reproduction in other forums is permitted, provided the original author(s) and the copyright owner(s) are credited and that the original publication in this journal is cited, in accordance with accepted academic practice. No use, distribution or reproduction is permitted which does not comply with these terms.
*Correspondence: Imke Podbielski, aXBvZGJpZWxza2lAZ2VvbWFyLmRl