- 1Department of Arctic Biology, The University Centre in Svalbard, Longyearbyen, Norway
- 2Oithona, Katarzyna Dmoch Company, Gdansk, Poland
- 3Department of Marine Ecology, Institute of Oceanology, Polish Academy of Sciences, Sopot, Poland
- 4Institute for Arctic and Marine Biology, Faculty for Biosciences, Fisheries and Economics, UiT- The Arctic University of Norway, Tromsø, Norway
Seasonal patterns in mesozooplankton composition, vertical distribution, and timing of reproduction are challenging to study in the open sea due to ocean currents and mix of populations of different origins. Sill fjords, on the other hand, with restricted water exchange, are ideal locations for studying taxa- and community-specific adaptations to the prevailing environment. Here, we present re-occurring patterns in the mesozooplankton community structure in Billefjorden, Svalbard, a high Arctic sill fjord with extensive seasonal ice cover, based on monthly sampling from 2011 to 2013. The zooplankton community composition confirmed the Arctic character of this fjord. Predominantly herbivorous taxa, such as Calanus glacialis and Pseudocalanus spp., showed strong seasonal variation in abundance and depth distribution, with population minima in spring being compensated by a rapid population recovery during summer. Omnivorous taxa, such as Microcalanus spp. and copepods of the family Aetideidae, largely remained at depth throughout the year and had an extended or year-round reproductive period. Deep-dwelling omnivorous/carnivorous species peaked in abundance in winter–spring when herbivorous populations were severely depleted. Taxa with seasonally limited occurrences, i.e., meroplankton, peaked in spring and summer at the surface, but were largely absent for the rest of the year. The different life histories, with contrasting feeding modes, depth preferences, and timing of reproduction lead to reduced interspecies competition and allow for a rather high and stable abundance of mesozooplankton year-round despite the short primary production window at high latitudes.
1 Introduction
The Arctic is characterized by extreme seasonality in incoming solar radiation with up to 4 months of continuous darkness and 4 months of continuous daylight (Cohen et al., 2020). For the marine life, the continuous darkness may extend far into the period of midnight sun, since sea ice with snow on top efficiently stops light to penetrate to the water column below (Leu et al., 2011). Light is the main limiting factor for primary production in winter and early spring. Depending on sea ice conditions, the spring phytoplankton bloom may start as early as April in open water or as late as August in regions with extensive sea ice at 75° latitude and above (Leu et al., 2015). Marine zooplanktons have to adapt to this pronounced seasonality in light and primary production. The onset of the spring bloom is a key biological event in Arctic marine ecosystems, and species life histories are directly or indirectly tuned to the timing of this event depending on their trophic mode (Hagen and Auel, 2001). Herbivorous zooplanktons have adapted to the short window of primary production by efficiently building up large lipid stores when algae food is plentiful. At the end of the productive season, they descend to greater depths, where they survive the long and food-poor winter by lowering their metabolism and benefiting from reduced predation risk. Omnivorous–predatory zooplankton, on the other hand, have limited lipid deposits and depend on feeding actively year-round (Hagen and Auel, 2001). The timing of reproduction differs among taxa with different trophic modes. Herbivorous zooplankton often have one major reproductive period per year, while omnivorous–predatory species are more flexible (Berge et al., 2020). Large body size is common in some Arctic zooplankton species, allowing for large lipid storages, which again make it possible for herbivorous zooplankton to reproduce prior to the spring bloom on internal resources alone (i.e., capital breeding; Varpe et al., 2007). A food-independent early reproduction is an adaptation to regions with consolidated sea ice and large inter-annual variability in the timing of the spring bloom (Sainmont et al., 2014; Ejsmond et al., 2018). In Arctic shelf seas and fjords, sea ice commonly disappears in summer, and the onset of the spring bloom varies rarely by more than 1 month (Leu et al., 2015). These regions are on average twice as productive as the deep Arctic Ocean (Sakshaug, 2004) and can sustain a more abundant zooplankton community with less Arctic specialized taxa (e.g., Gluchowska et al., 2016).
There is still a scarcity of seasonal zooplankton studies from the Arctic. Available data series are patchy on temporal and spatial scales and often not consistent in relation to methods used. Most zooplankton studies from the Arctic have been conducted during summer when the region is easily accessible, with other seasons being understudied, and restricted to epipelagic waters focusing on abundant herbivorous calanoids, hence omitting deeper waters inhabitants (Richter, 1994). Moreover, they have often concentrated on only one or few dominant species (Lischka and Hagen, 2005; Søreide et al., 2010; Ershova et al., 2021b) and rarely on the whole zooplankton community (Weydmann et al., 2013; Darnis and Fortier, 2014; Bandara et al., 2016).
In Svalbard, life histories of dominant copepod species and the pteropod Limacina helicina have been the subject of detailed year-round investigations in Kongsfjorden (Lischka and Hagen, 2005; Lischka and Hagen, 2016), Isfjorden (Arnkværn et al., 2005; Balazy et al., 2021; Boissonnot et al., 2021; Ershova et al., 2021b; Hatlebakk et al., 2022), and Rijpfjorden (Søreide et al., 2010). Seasonal research on the whole mesozooplankton community has been conducted in Kongsfjorden (Hop et al., 2002; Kwasniewski et al., 2003; Hop et al., 2006; Walkusz et al., 2009; Hop et al., 2019) and Rijpfjorden (Weydmann et al., 2013), but these studies are based on data collected with rather coarse resolution in time. Annual studies of meroplankton are available from Adventfjorden (Isfjorden system) (Kuklinski et al., 2013; Stübner et al., 2016), and the seasonal variability in the macrozooplankton community has been studied in Billefjorden (Bandara et al., 2016). In most of these studies, data from the polar night period are either lacking or restricted to very few data points, thus providing an important but temporarily limited description of the seasonal changes in the community structure.
In the face of ongoing global warming (Wassmann, 2011), there is an urgent need to describe the status quo ante climate change to enable us to recognize its impacts on ecosystems structure and functioning beyond the natural, seasonal variability. The increased influence of warm Atlantic water along West Spitsbergen is slowly but visibly imprinting on the biota in Svalbard fjords (Gluchowska et al., 2016), surrounding seas (Ingvaldsen et al., 2021), and the Arctic Ocean (Ershova et al., 2021a). In fjords of Svalbard, perturbations in the ice cover extent and thickness are notable together with an increase in the oceans heat content (Muckenhuber et al., 2016). The consequences anticipated with loss in sea ice include a decline in Arctic species (Wassmann, 2011), an earlier occurrence of phytoplankton blooms (Kahru et al., 2011; Ingvaldsen et al., 2021), a shift in protists dominance towards smaller picoplankton fraction (Hegseth et al., 2019), and a possible mismatch between timing of primary and secondary productions (e.g., Søreide et al., 2010). These alterations at the base of the food web may have cascading effects on higher trophic levels such as fish, marine mammals, and seabirds (e.g., Falk-Petersen et al., 2007; Vihtakari et al., 2018).
A good understanding of how zooplankton communities vary seasonally and interannually is essential to assess the impact of anthropogenic and climate change. However, it is not a trivial task to establish a baseline or reference for what is natural. Zooplankton population dynamics are notoriously difficult to follow due to their very nature of being passive drifters. Repeated sampling at one location does not guarantee that the same community is explored over time, and changes in population structure and species composition over time may be biased by the inflow or exchange of populations between sampling events.
In this study, we targeted a high Arctic fjord with very limited water exchange to overcome the many pitfalls that advection may introduce. The rather deep (196 m) and seasonally ice-covered inner basin of Billefjorden is largely isolated from the outer fjord system (Nilsen et al., 2008; Skogseth et al., 2020). We therefore assume that chronological changes in the community composition and abundance reflect internal processes (reproduction, growth, and survival in the local populations) rather than the in- and outflow of populations due to advection (e.g., Arnkværn et al., 2005). Billefjorden, therefore, functions as a valid non-advective model system for Arctic shelf seas to identify repeatable seasonal patterns in Arctic mesozooplankton communities that would not be clearly observable in open shelf seas. Here, we analyzed data on the mesozooplankton community structure collected at 21 sampling events (in total 88 samples) from 2011 to 2013 to identify consistent seasonal patterns in (1) mesozooplankton community composition, (2) vertical distribution and (3) timing of reproduction of key zooplankton taxa in this high-Arctic fjord environment. Our study provides a baseline that will be important to differentiate climate change and natural seasonal patterns in development of mesozooplankton communities in Arctic fjords and shelf seas.
2 Materials and methods
2.1 Study area
The study was conducted in Billefjorden (78°40′N, 16°40′E: Figure 1), a glacial fjord located in the distant inner part of Isfjorden, the largest fjord system on the western coast of Svalbard. Isfjorden regularly experiences intrusions of warm and saline Atlantic Water (AW) from the West Spitsbergen Current and of Arctic Water (ArW) originating from the Barents Sea with the East Spitsbergen Current and the Coastal Current (Skogseth et al., 2020). Hydrographical conditions vary depending on intensity of advection, water mass mixing, and exchange, and can alternate from typically Arctic to Atlantic conditions, with a dominance of Atlantic conditions due to increased advection of AW over the past decade (Skogseth et al., 2020). Billefjorden is about 30 km long and 5–8 km wide and is divided into an outer and an inner basin by two sills (the outer of 70 m and the inner of 50 m depth), which restrict exchange of fjord waters with Isfjorden outside (Nilsen et al., 2008). There is evidence that Billefjorden remains free of AW signatures even under extreme influxes of AW in Isfjorden (Cottier et al., 2005; Nilsen et al., 2008). The average depth of the inner basin is 160 m, with a maximum of 196 m. Our sampling site was located in Adolfbukta, the innermost basin, at 192 m depth (station BAB, 78°39.7 N, 16°43.9 E; Figure 1), which is dominated by locally formed winter cooled water (WCW) with temperatures <−0.5°C below 50 m year-round. While the inner basin is largely unaffected by influxes from outside (Arnkværn et al., 2005), it receives a discharge of fresh water and sediments from the adjacent glacier (Nordenskjöldbreen) during summer melting. An ice cover usually forms between December and February and lasts until June (https://cryo.met.no).
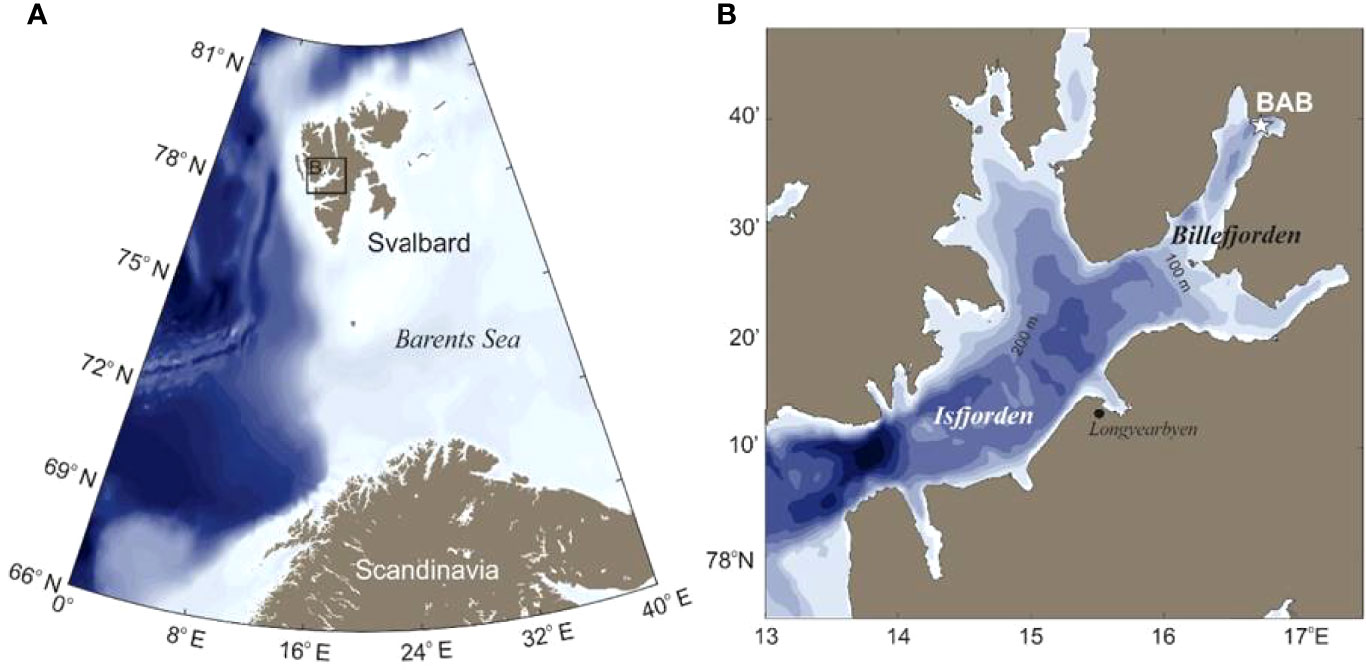
Figure 1 Study area. (A) Map of Svalbard. (B) Location of sampling site BAB (asterisk) in Billefjorden, Svalbard.
2.2 Data collection
Depending on sea-ice conditions, the sampling site was accessed either by sea using a research vessel (RV Helmer Hanssen, RV Lance) or small boats, or in winter–spring on the ice using snowmobiles with nets towed through a hole in the ice. Samples were collected regularly between May 2011 and September 2013 (21 times), generally in monthly intervals, with a break between September and November 2011 and from January to the beginning of June 2012 (Table 1). Sampling was generally conducted between noon and late afternoon. In February 2013, sampling was conducted in the outer basin (BAB*, 78° 34.3N 16° 27.4E; bottom depth, 154 m) due to challenging sea ice conditions.
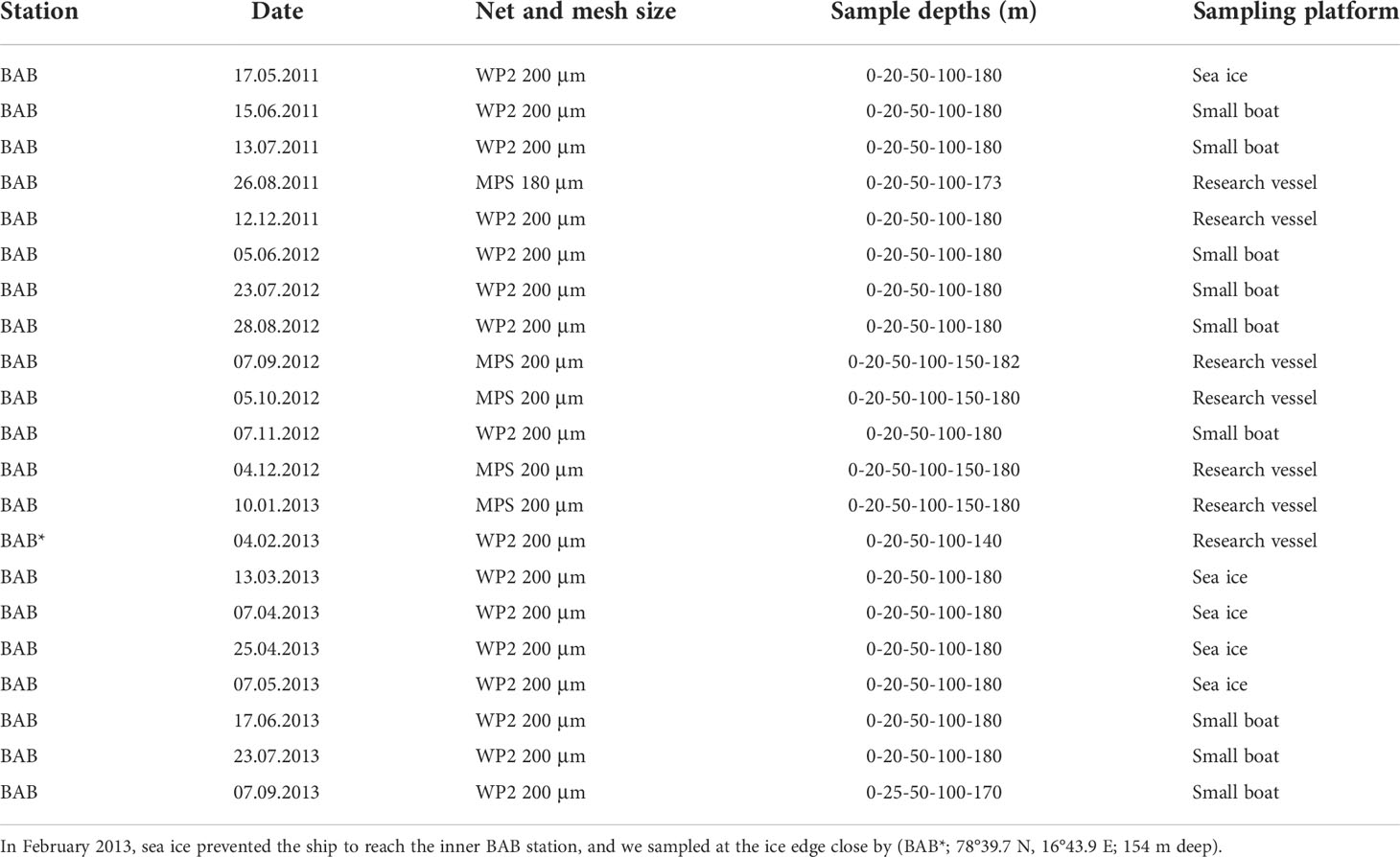
Table 1 Overview of sampling dates and nets used in Billefjorden at station BAB (78°40′N, 16°40′E; 192 m deep) from May 2011 to September 2013.
Vertical profiles of temperature, salinity, and fluorescence were obtained at each sampling date using a handheld CTD (SAIV model 204, Bergen, Norway) or a ship-board conductivity, temperature, and depth profiler (Sea-Bird Electronics, Bellevue, WA, USA) with a SeaPoint fluorometer attached. From October 2010 to September 2013, an instrumented mooring was deployed close to the sampling site. SeaBird Electronics MicroCats, recording temperature, conductivity, and pressure, were located at 16, 28, and 183 m. The moorings also included a SeaPoint fluorometer at 28 m. Vemco temperature miniloggers were fixed at intervals of 10–30 m at depths between 14 and 150 m. The mooring was serviced in August 2011 and September 2012. For that purpose, the mooring was recovered in its entirety, data were downloaded, instruments and batteries were replaced, and the mooring was redeployed within 2–3 weeks after the recovery.
Zooplankton was collected in vertical hauls using either a Multiple Plankton Sampler (MPS; Hydro-Bios, Kiel) from larger vessels (7 dates) or a closing WP2 net (Hydro-Bios, Kiel) when sampling from small boats and through the ice (14 dates) (Table 1). The two nets’ dimensions are comparable with an opening area of 0.25 m2 and a mesh size of 200 µm (except for 26 August 2011 when 180-µm mesh size was used). Tows were taken in a stratified manner from four standardized depth layers: 180–100 m, 100–50 m, 50–20 m, and 20–0 m. Three times the deepest layer was divided further: 180–150 and 150–100 m (Table 1), but these data were pooled before data analyses in this study, except for the calculation of weighted mean depth (WMD). Zooplankton samples were immediately preserved with 4% borax-buffered formaldehyde–seawater solution after collection.
2.3 Zooplankton identification and analysis
Prior to quantitative analysis larger (>5 mm) organisms were picked out and identified separately. Samples were diluted into 100–400-ml volume depending on the density, and 2-ml subsamples were taken using a pipette with enlarged (4 mm) opening. All organisms were enumerated and identified from three to five subsamples. Additional subsamples were analyzed (usually 1/10 of sample volume) to enumerate less abundant species and stages, and the entire sample was scanned to detect rare species and remaining macrozooplankton. All organisms were identified to the lowest possible taxonomic level. To distinguish between Calanus finmarchicus, Calanus glacialis, and younger stages of Calanus hyperboreus, we measured prosome length of all counted individuals and followed size classes obtained during previous studies from Billefjorden (Arnkværn et al., 2005; Gabrielsen et al., 2012). Only females of Pseudocalanus spp. were identified to species level (P. minutus and P. acuspes), but females of P. moultoni may also have been present (Ershova et al., 2021b), and erroneous been identified to P. acuspes. Copepods of the genus Microcalanus were attributed to Microcalanus pygmaeus, but Microcalanus pusillus could have occurred in low numbers as well. Copepods were divided into small and large copepods (total length as adults <2 mm or >2 mm, respectively). Species abundances were calculated from vertical net hauls, assuming 100% filtration efficiency, as number of individuals per cubic meter. Absolute data from November 2012 is a bit uncertain due to stormy weather and strong drift. Particularly, the upper net sample (0–20 m) was exposed to trawling with roughly five times more water (i.e., 25 m3) filtered than the theoretical 5 m3 if hauled vertically, and taxa abundance data were corrected accordingly. In August 2011 and February 2013, the deepest tow did not reach 180 m, but the abundances of this lowest stratum were recalculated to obtain comparable results of water column data.
2.4 Data analyses
Contour plots of sea water temperature and log x + 0.1 transformed zooplankton abundance in the water column from 2011 to 2013 (Figure 2) were performed in MatLab (version 2014b). All other analyses were done in R [version 3.6.1 (R Core Team, 2020)] using R Studio (version 1.2.5001) and PRIMER (version 7.021) (Clarke and Warwick, 2001) with the PERMANOVA+ add on (Anderson et al., 2008).
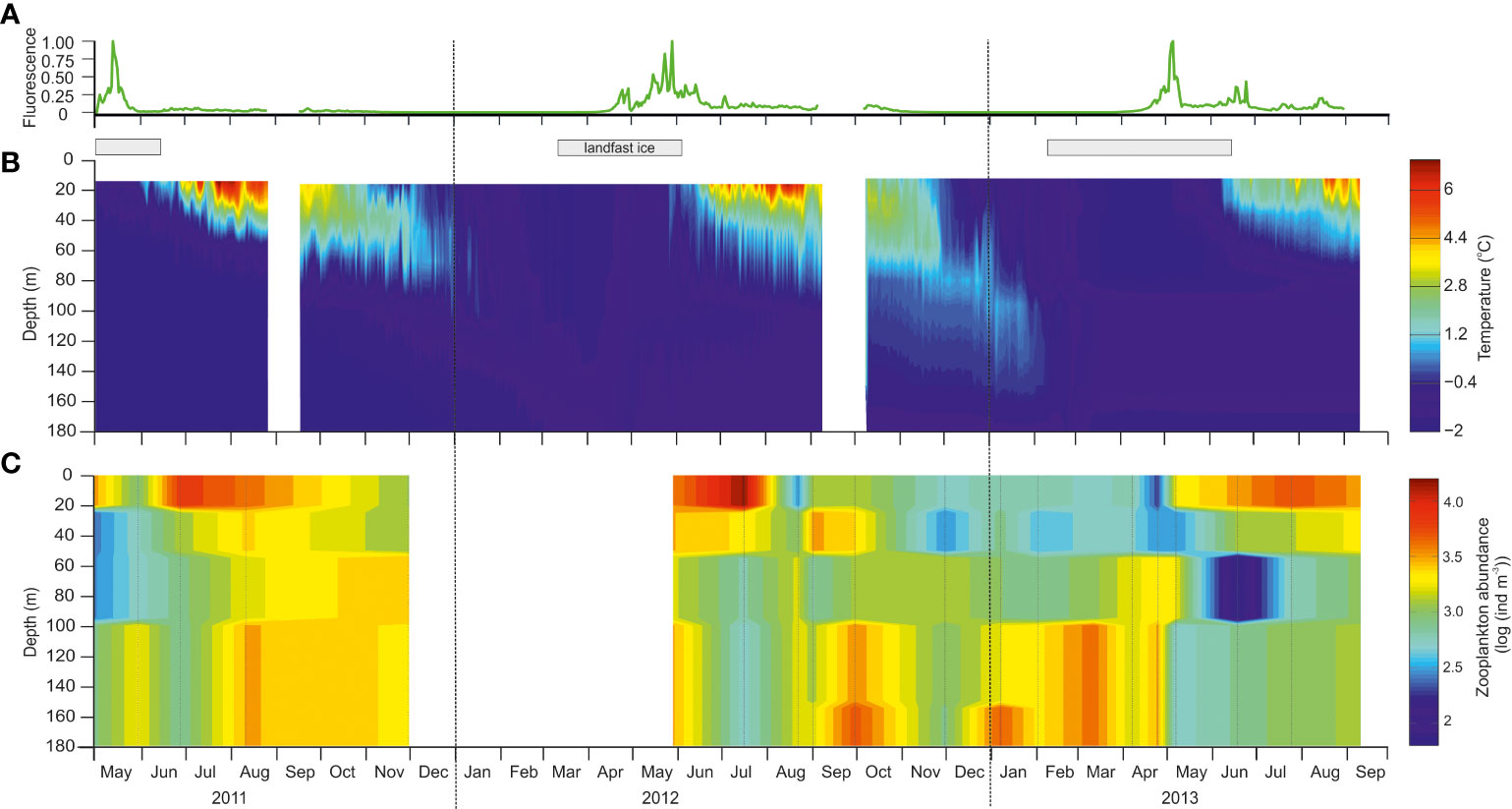
Figure 2 Seasonal development of environmental parameters and zooplankton abundance in the water column at station BAB in Billefjorden during the study period from May 2011 to September 2013. (A) Seasonal variability in fluorescence (normalized between 0 and 1) measured by a SeaPoint fluorometer placed at 28 m depth on the mooring line deployed close to station BAB in Billefjorden. (B) Seasonal variability in temperature (°C) based on several temperature loggers along the mooring line deployed close to the station. White field indicates gaps in measurement due to moorings being serviced. Gray bars on top indicate periods of ice cover. (C) Seasonal variability in total zooplankton abundance (log ind. m−3), there white field represent no data available.
2.4.1 Zooplankton community structures
Similarities in zooplankton composition were explored by hierarchical cluster analysis of integrated log x + 0.1 transformed species/taxa abundance using the R package cluster (Maechler et al., 2019). The results were visualized in a scatter plot using the fviz_cluster function in the factoextra package (Kassambara and Mundt, 2020). Fviz_cluster performs principal component analyses (PCAs) and plots the data points according to the first two principal components that explain the majority of the variance (dimensions 1 and 2). To estimate the similarities in taxa composition within the distinct clusters revealed in the cluster plot, we used SIMPER (similarity percentage) analyses in PRIMER (version 7.021) on log x + 0.1 log-transformed integrated zooplankton data and Bray–Curtis similarity.
To test whether differences in the zooplankton community composition (integrated log x + 0.1 transformed species/taxa abundance) was mainly due to season and/or year, we performed a permutational multivariate analysis of variance (PERMANOVA) test on Bray–Curtis similarity among sample units to obtain p-values using random permutations of residuals under a reduced model for both the dependency of singular factors (year; season) and their simultaneous acting (Anderson et al., 2008). PERMANOVA is an ANOVA-like statistical method designed for multivariate data clouds (a matrix of resemblances expressed either as distances, dissimilarities, or similarities).
2.4.2 Vertical Zooplankton distribution
We calculated the weighted mean depth (WMD) for selected species/taxa to determine seasonal changes in their vertical distribution. Similarly to Daase et al. (2016), we calculated the mean depth (Zm) and the standard deviation (Zs) of the frequency distribution along the depth axis using the method of Manly (1977):
where n is the number of depth intervals, dj = lower sample – upper sample depth (m) of sample interval j, zj is the midstrata (m) of sample interval j, fj is the density of individuals (per m3) observed in depth interval j, and wj is the relative abundance at depth stratum j taking into account the range of the depth interval. To account for the variability in bottom and sampling depth between our main station BAB (bottom depth, 192 m; sample depth, 170–182 m) and the station in the outer basin sampled in February 2013 (bottom depth, 154 m,; sample depth, 140 m) (see Table 1 for sampling details), we calculated the relative weighted mean depth as the ratio of WMD to the depth of the deepest sample at each sampling event to compare seasonal changes in depth distributions between species/taxa.
The WMD (Zm) indicates the mean depth at which the majority of population is located, while its standard deviation (Zs) is a proxy for the extent of the vertical distribution (small Zs indicates that organisms were concentrated at a certain depth, and large ones the opposite).
3 Results
3.1 Seasonal changes in environmental conditions and corresponding zooplankton patterns
3.1.1 Hydrography
The inner basin of Billefjorden was characterized by locally formed cold water (<−0.5°C) that prevailed year-round below 80 m and throughout the entire water column, while the fjord was ice covered (Figure 2B). Ice formed at the beginning of March in 2012 (https://cryo.met.no) and in early February in 2013 (JE Søreide pers. obs.). Maximum sea ice thickness varied between 30 cm (2013) and 50 cm (2011 and 2012), while snow depth varied from a maximum of 15 cm (2011 and 2012) to almost none in 2013 (max. 1 cm). Ice breakup occurred at similar times, at the beginning of June, in all 3 years (https://cryo.met.no).
As soon as the ice broke up, water temperatures increased rapidly in the upper 60 m (above the sill depths) resulting in a strong thermal stratification that persisted throughout the summer (Figure 2B). Maximum water temperatures were observed in August (up to +6°C), but below 100 m, water temperatures remained below −1°C (Figure 2B). Throughout autumn, surface waters cooled, and the stratification broke down. In January, the water column was well mixed and homogeneously cold (−1.7°C).
3.1.2 Algal food availability
Fluorescence measurements at 28 m (Figure 2A) and throughout the water column (CTD profiles; Supplementary Figure S1) indicated that phytoplankton increased in abundance under the ice in late April all 3 years with peak values observed in early to mid-May every year (~7 µg Chl a L−1; Freese et al., 2016). Fluorescence values were low in June 2011, while elevated values (~1 µg L−1) persisted into July in 2012 and 2013 (Figure 2A). Ice algae were present in April, but ice algal chlorophyll a concentrations were overall low in Billefjorden (<0.8 µg L−1, Leu et al., 2015; Søreide unpublished data).
3.1.3 Seasonal patterns in Zooplankton abundance
Zooplankton showed distinct seasonal patterns in vertical distribution (Figure 2C). In spring–summer, following the ice breakup (June–August 2011, June–July 2012, and July–August 2013), the highest total mesozooplankton abundance was found in surface waters. From August to September, zooplankton was mainly concentrated below 100-m depth where the majority remained until April, before descending again in August.
3.1.4 Zooplankton biodiversity
The zooplankton community consisted of 49 recognized zooplankton taxa, representing 9 phyla, 19 orders, 26 families, and 25 genera. Among these, 16 taxa belonged to Copepoda and 8 to other Crustacea, constituting 87% (69% Calanoidae) and 0.06% of the total zooplankton abundance, respectively. The taxonomical composition of the zooplankton community was relatively constant over time, with 11 taxa occurring at all sampling dates (Microcalanus spp., Pseudocalanus minutus, C. glacialis, Oithona similis, C. hyperboreus, C. finmarchicus, Metridia longa, Acartia longiremis, Aetideidae juveniles, Triconia borealis, and Parasagitta elegans) and 14 taxa occurring in more than 50% of the samples (Supplementary Table S1). Boreal species such as Limacina retroversa, Evadne nordmanni, and Oithona atlantica, and deeper living Metridia lucens and Tomopteris helgolandicus were occasionally found in low abundances in summer and autumn (data not shown). Meroplankton, larvae of Cirripedia, Bivalvia, Gastropoda, Polychaeta, and Echinodermata accounted for roughly half of the zooplankton abundance in May–July, but disappeared abruptly during autumn, being virtually absent in winter (Figure 3A).
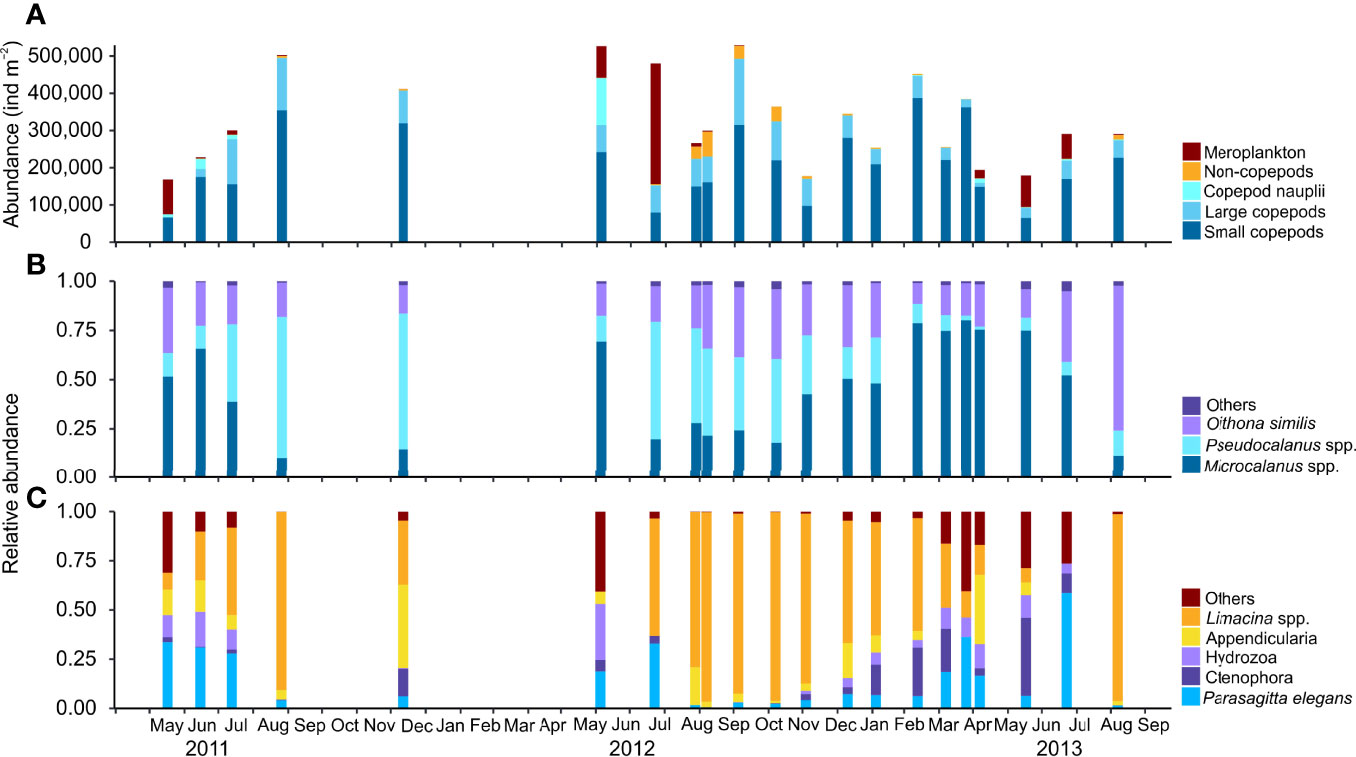
Figure 3 Monthly variability in zooplankton abundance (ind. m−2) and species composition of dominant zooplankton groups during the study period May 2011–September 2013 in Billefjorden, station BAB. (A) Total abundance of dominant zooplankton groups [large (total length > 2 mm) and small copepods (total length<2 mm)], copepod nauplii, non-copepod species, and meroplankton. (B) Relative composition of dominant small copepod taxa. (C) Relative composition of dominant non-copepod taxa.
Among copepods, small-sized taxa accounted for 45%–93% of the zooplankton abundance, except in May–July during peak abundance of meroplanktonic larvae. The two omnivorous copepods Microcalanus spp. and O. similis and the predominantly herbivorous Pseudocalanus spp. were major contributors to the overall zooplankton abundance (28%, 16%, and 18%, respectively), while the large copepod C. glacialis accounted for roughly 40% in late August–September but<1% in April (Figure 3B, Supplementary Table S1). Microcalanus spp. was observed in highest abundance in winter and spring, while Pseudocalanus spp. and O. similis were most numerous in autumn (Table 2, Figure 3B).
Among non-copepod holoplankton species, the pteropod L. helicina was the most abundant species comprising up to 25% of the non-copepods in autumn (64,025 ind. m−2) (Figure 3C). This mucus filtrator was almost always present (92% of sample events), mainly in the form of juvenile specimens with peak abundances observed between August and November (Table 2, Supplementary Figure S4). Other mucus filtrators such as the appendicularians Fritillaria borealis and Oikopleura spp. peaked also in abundance in autumn, but their abundance was one order of magnitude lower (<6,215 ind. m−2, Supplementary Figure S4).
Among the predatory zooplanktons, the chaetognath P. elegans was the most abundant (up to 82 ind. m-2) and the only consistently present carnivore in all samples (Figure 3C, Supplementary Table S1). The chaetognath Eukrohnia hamata (<6 ind. m−2), the pelagic amphipods Themisto abyssorum and T. libellula (<36 ind. m−2), and the pteropod Clione limacina (<66 ind. m−2) occurred less frequent and in very low numbers. Other predatory taxa that appeared regularly were juveniles and larvae of ctenophores (Beroë cucumis and Mertensia ovum) and hydromedusae (Aglantha digitale and Bougainvillia sp.) (Supplementary Table S1).
3.3 Seasonal community patterns
The cluster analyses on log x + 0.1 transformed integrated zooplankton abundances revealed that the seasonal variability in the community structure was higher than the inter-annual variability (Figure 4). The PERMANOVA analysis confirmed that season had a significant effect on community composition (p = 0.001), while neither year (p = 0.47) nor the interaction between year and season was statistically significant (p = 0.15). The zooplankton total abundances did not vary much between seasons, but the taxa composition did (Table 2, Figure 5).
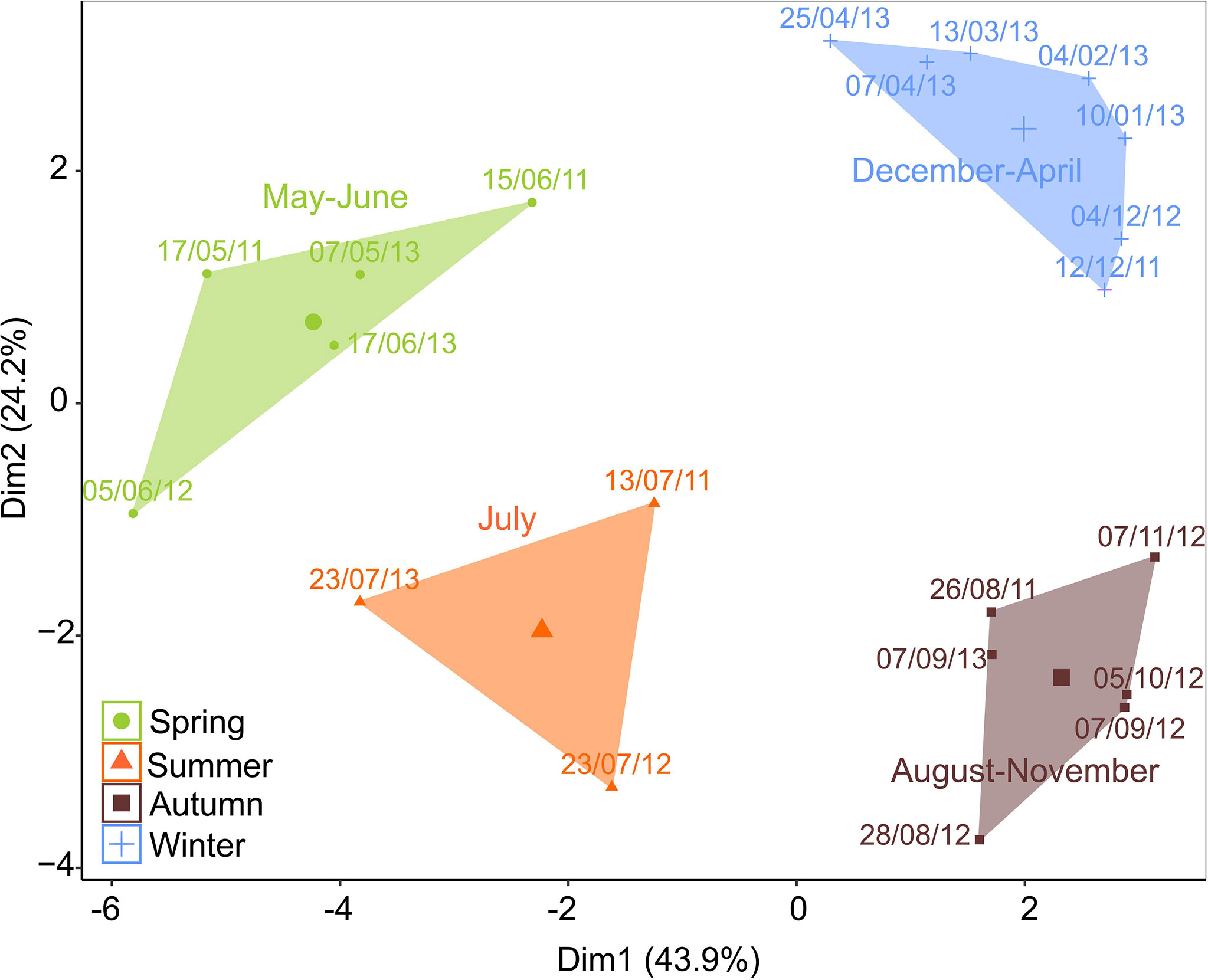
Figure 4 Multidimensional scaling (MDS) of cluster analyses performed on integrated (log x + 0.1 transformed) zooplankton species/taxa data (ind. m−3). The two dimensions display 68.1% of the total variability and show four distinct clusters of samples with high similarity (>69%; SIMPER): Spring (May–June), Summer (July), Autumn (August–November), and Winter (December–April).
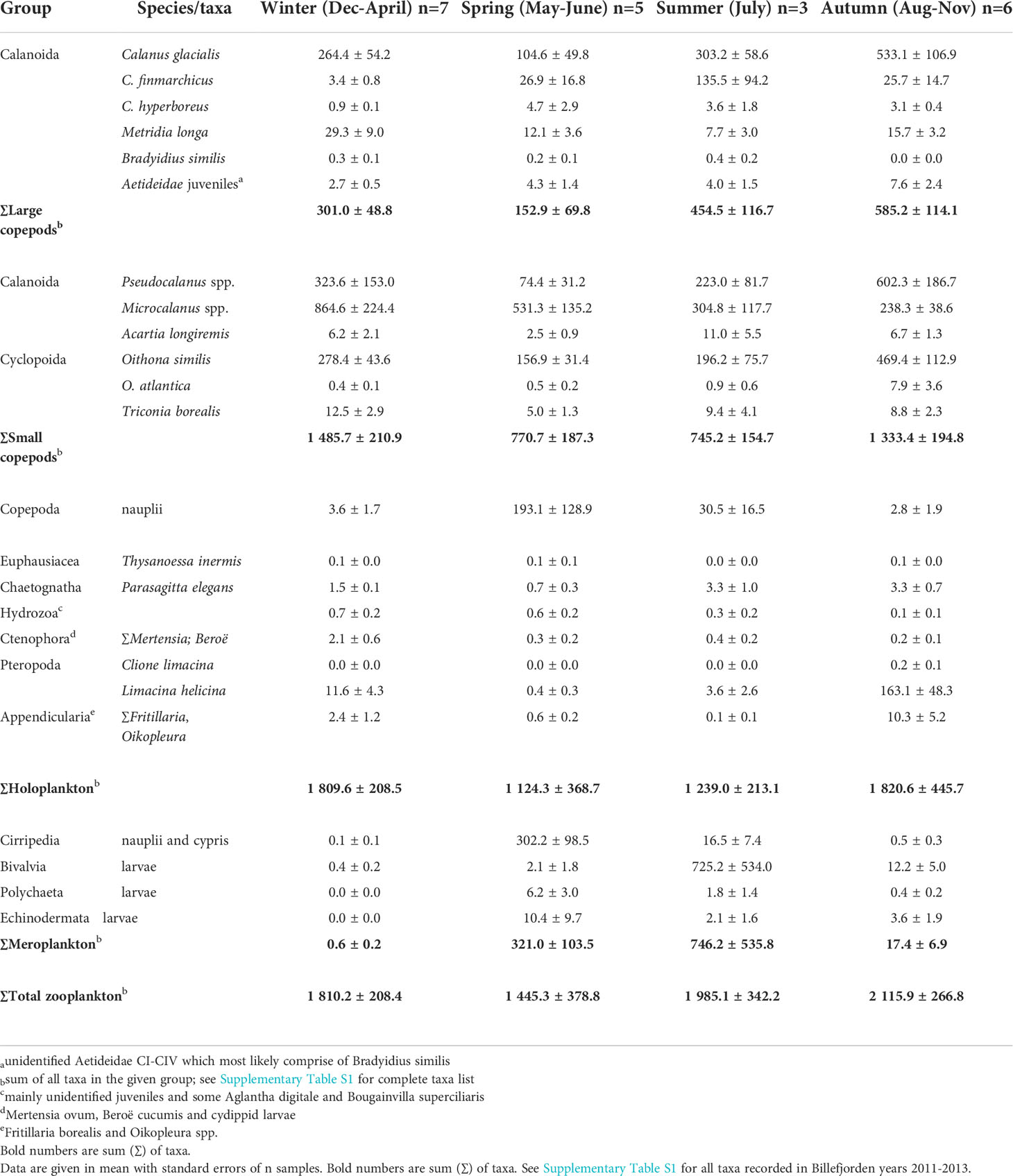
Table 2 Overview of main zooplankton species/ taxa (ind. m-3) in Billefjorden, Svalbard, divided into the four seasons: Winter, Spring, Summer and Autumn according to similarity (cluster) analyses on integrated zooplankton densities (see Figure 4).
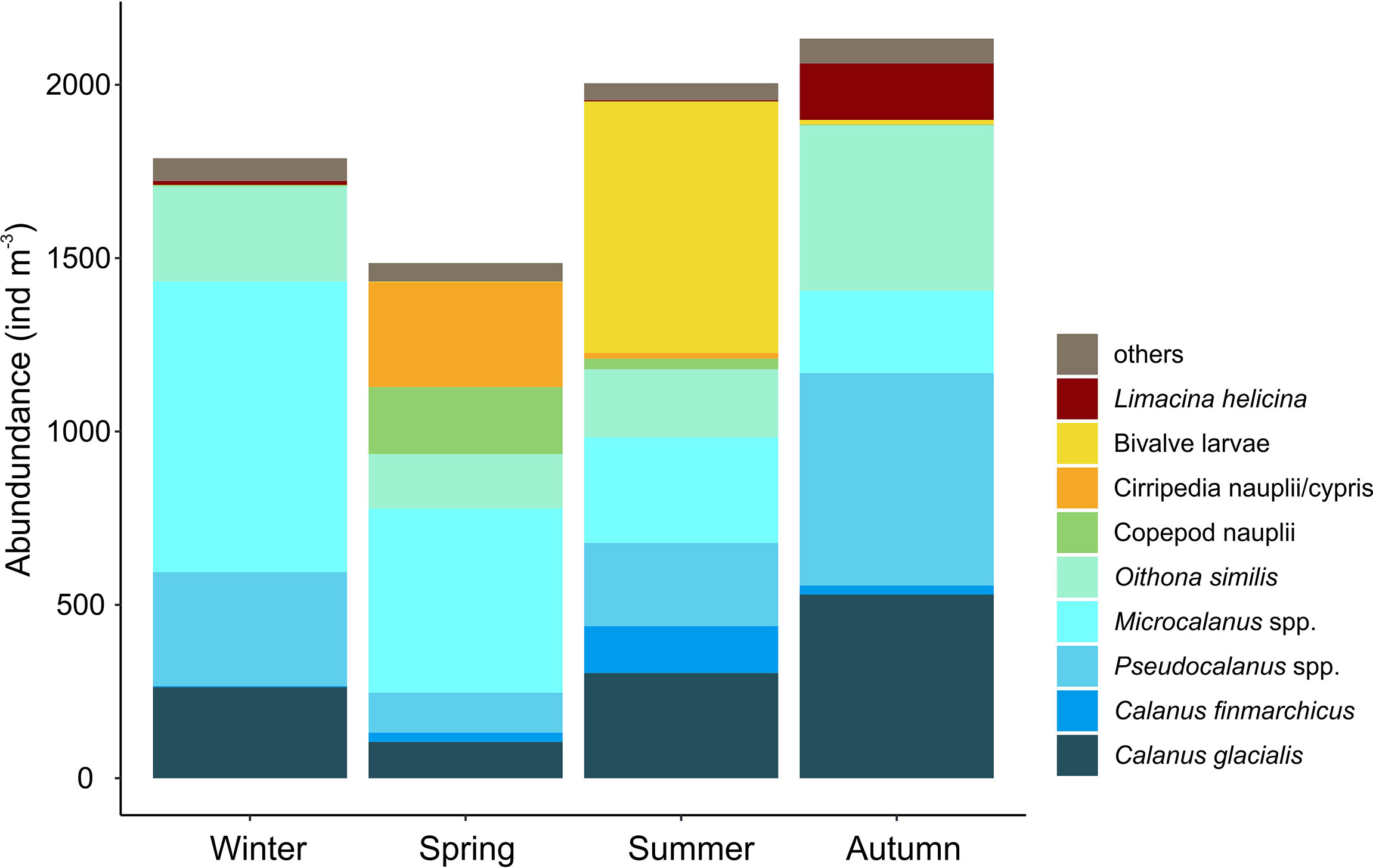
Figure 5 Average integrated abundance of the most dominant zooplankton species (ind. m−3) in Billefjorden (2011–2013) in the four seasons identified by multidimensional scaling (see Figure 4): Winter (December–April), Spring (May–June), Summer (July), and Autumn (August–November).
Four main zooplankton communities were apparent in the cluster analysis with a distinct winter community in December–April (81% similarity in community composition; SIMPER) followed by a spring community in May and June (69% similarity, SIMPER), developing into a summer community in July (75% similarity, SIMPER) and further to a distinct autumn community in August–November (76%, similarity SIMPER). Winter was characterized by a zooplankton community with high abundance of Microcalanus spp. combined with relative low abundance of C. glacialis. In spring, Cirripedia and copepod nauplii became abundant, and in summer, high numbers of bivalve larvae were observed. In autumn, Pseudocalanus spp. and O. similis peaked in abundance, together with C. glacialis and L. helicina (Table 2, Figure 5).
3.4 Seasonal vertical migration
The seasonal variability in taxa abundance and vertical distribution showed three main patterns: (1) taxa with high seasonal variability in their depth distribution, termed “seasonal migrators,” (2) taxa that remained at depth throughout the year, termed “deep-dwelling zooplankton,” and (3) taxa with highly seasonal occurrences termed “seasonal visitors” (e.g., meroplankton). Patterns in abundance and vertical distribution are described below following these three categories.
3.4.1 Seasonal migrators
Zooplankton taxa performing distinct seasonal migration were copepods of the genus Calanus spp. and Pseudocalanus spp. and, to a lesser extent, the cyclopoid copepod O. similis and the chaetognath P. elegans (Figures 6, 8A).
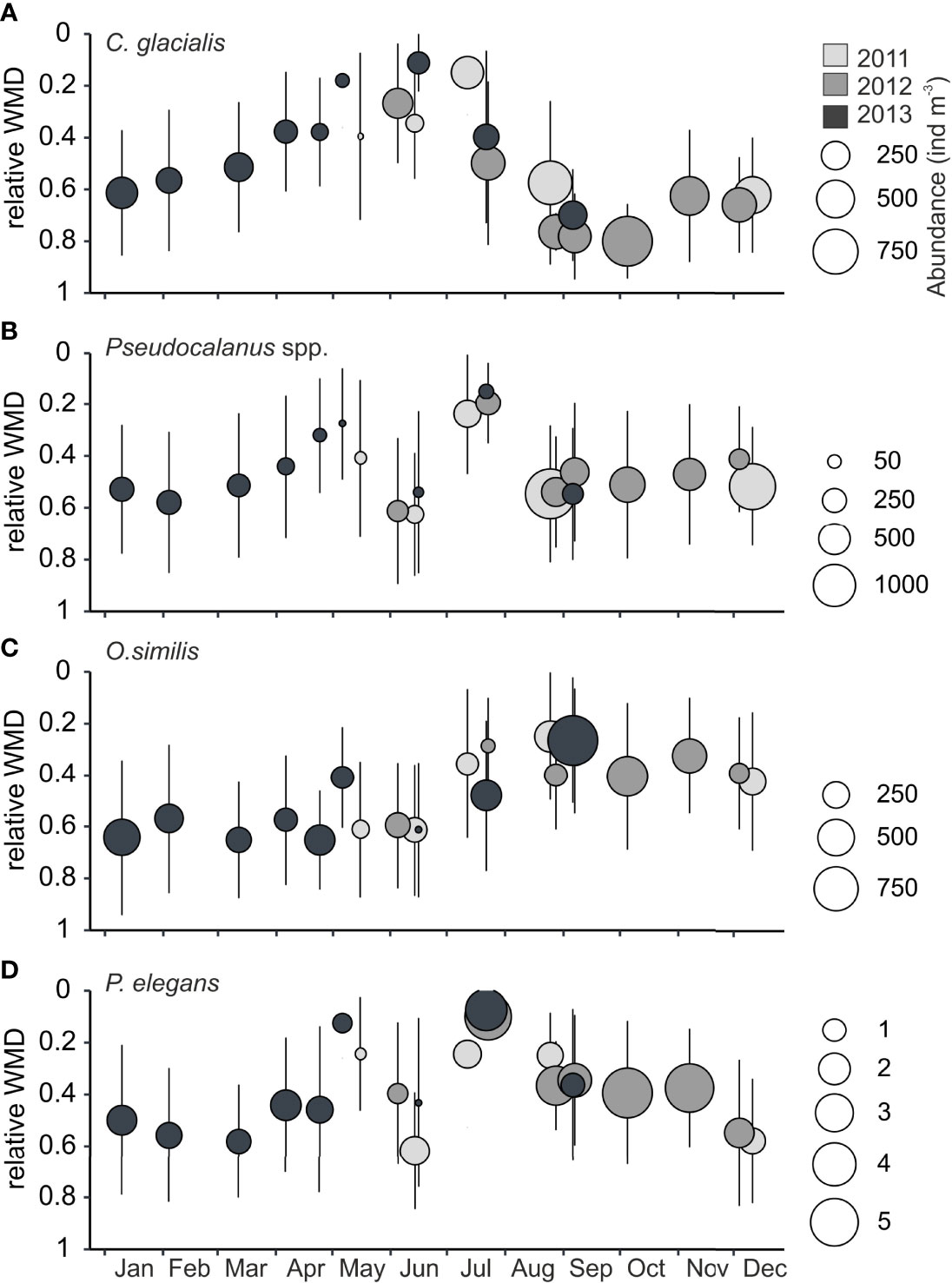
Figure 6 Relative weighted mean depths (WMDs) against day of year for species with high seasonal variability in WMD Billefjorden, years 2011-2013. Error bars indicate the spread (Zs) of individuals over the water column, bubble size reflects mean abundance in water column (ind m−3), and color indicates year. (A) Calanus glacialis, (B) Pseudocalanus spp., (C) Oithona similis, and (D) Parasagitta elegans. Note the differences in scale between species. The relative WMD is the ratio of WMD to the total sample depth, i.e., a WMD of<0.5 indicates that the taxa was centered in the upper half of the water column, a WMD closer to 1 indicates that the distribution was centered in the deeper part of the water column.
Calanus glacialis occurred in high abundances in autumn (up to 3,100 ind. m−3 at deepest depth; Supplementary Table S1) but decreased in numbers over winter with lowest abundances observed in April and May (<150 ind. m−3; Table 2). In winter and spring, the C. glacialis population primarily consisted of females and copepodite stages IV (CIV) with a WMD of 68–119 m in winter and 20–71 m in spring. In mid-July, it was still in the surface (WMD, 27 m) and descended deeper by the end of July (WMD, 72–90 m), and it primarily centered below 100 m throughout autumn and winter (WMD September–January, 100–144 m). From January onwards, a slow shallowing of the WMD was observed before C. glacialis entered the surface water again in spring (Figure 6A). The two other Calanus species occurred in much less numbers in Billefjorden (Supplementary Table S1) but showed similar seasonal migration patterns as C. glacialis, except that C. finmarchicus remained in the surface 50 m up to 1 month longer than the two others in summer–autumn.
Pseudocalanus spp. dominated the small-copepod fraction in summer and autumn and showed a consistent seasonal cycle in its total abundance (Figures 6B, 8A). Its abundance was generally low in winter and particularly low in spring (Table 2, Figure 5). During summer, it showed increasing numbers, reaching maximum abundances in August (Figures 5, 6B, Supplementary Table S1). In summer (July), the Pseudocalanus population was concentrated in the upper 50 m (WMD, 26–35 m) and comprised mainly of young developmental stages (CI–CII). Throughout autumn and winter, it was centered at intermediate depth (WMD, 58–95 m), and the overwintering stages CIV and CV prevailed. Females of P. minutes were always present with peak numbers in June, while females of P. acuspes only occurred from May to September. Males were present all months except November and December.
Oithona similis concentrated at subsurface and intermediate depths in summer (WMD, 51–86 m) and autumn (WMD, 43–73 m) to descend relatively deep during winter (WMD, 70–117 m) and spring (WMD, 74–110 m) (Figure 6C). The upward movement in July–August was followed by maximum abundance in autumn (Table 2, Figure 8A)
Parasagitta elegans showed also consistent seasonal changes in their vertical position (Figure 6D). It remained fairly deep in winter (WMD, 80–104 m) with an upward movement in May (WMD, 44 m), concentrating at the surface in July (WMD, 10–20 m), and relocating to subsurface and intermediate depth in autumn (WMD, 43–70 m). Parasagitta elegans was observed in highest abundance during summer and autumn (Figure 8A).
3.4.2 Deep-dwelling zooplankton
Microcalanus spp. was the most abundant deep-dwelling species, followed by the much less numerous Triconia borealis, M. longa, and Bradyidius similis (Figure 7). Microcalanus spp. had a predominantly deep distribution (WMD, 77–145 m) year-round (Figure 7A) with peak abundances in winter (Table 2, Figure 8B). The much less abundant M. longa (WMD, 71–139 m) and Triconia borealis (WMD, 70–140 m) showed similar deep preference as Microcalanus spp. with peak numbers in winter-spring (Table 2, Figure 7B, C, 8B).
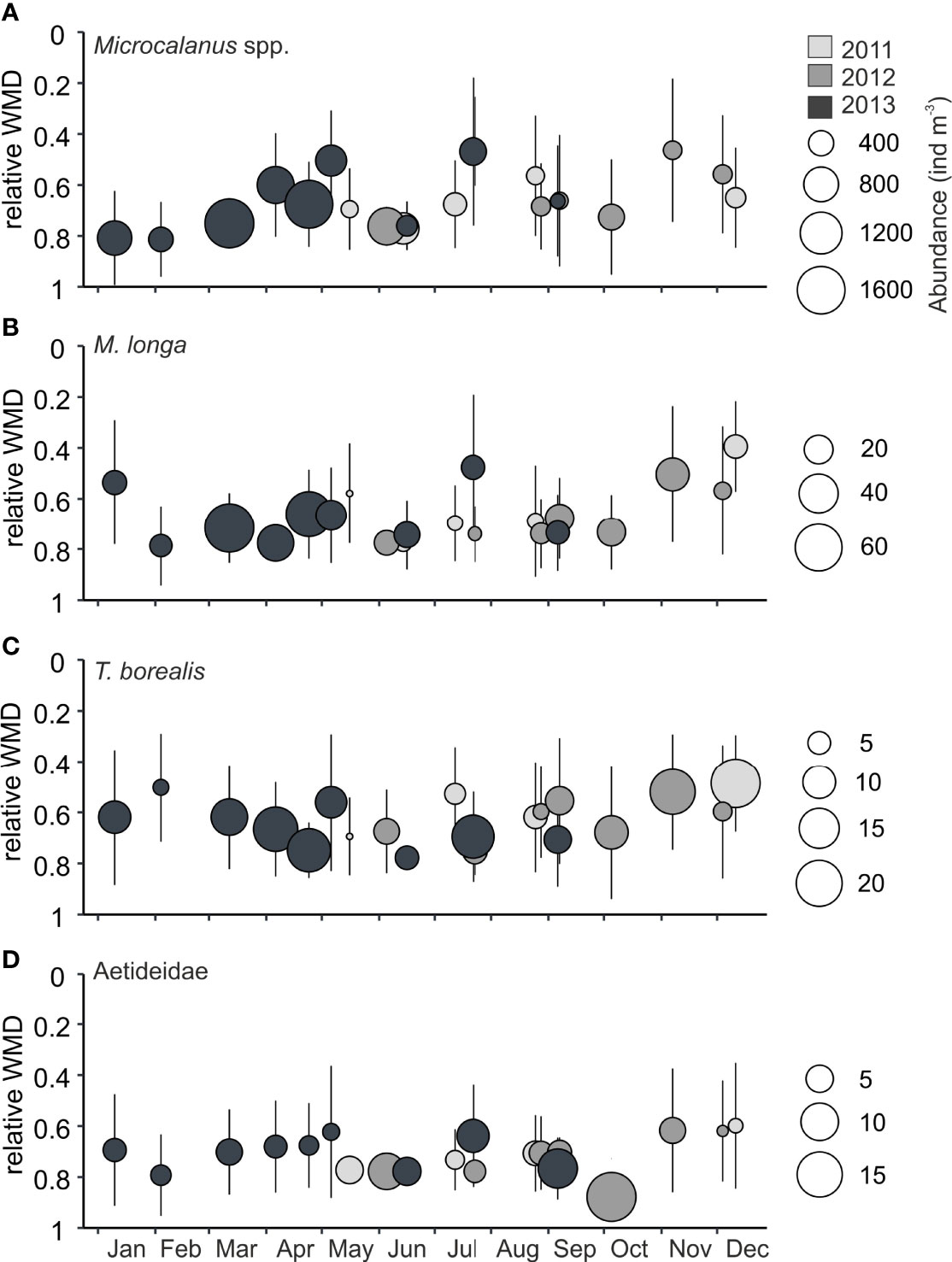
Figure 7 Relative weighted mean depths (WMDs) of taxa with deep distribution in Billefjorden, years 2011-2013. Error bars indicate the spread (Zs) of individuals over the water column, bubble size reflects mean abundance in water column (ind m−3), and color indicates year. (A) Microcalanus spp., (B) Metridia longa, (C) Triconia borealis, and (D) Aetideidae. Note the differences in scale between species.The relative WMD is the ratio of WMD to the total sample depth, i.e., a WMD of<0.5 indicates that the taxa was centered in the upper half of the water column, and a WMD closer to 1 indicates that the distribution was centered in the deeper part of the water column.
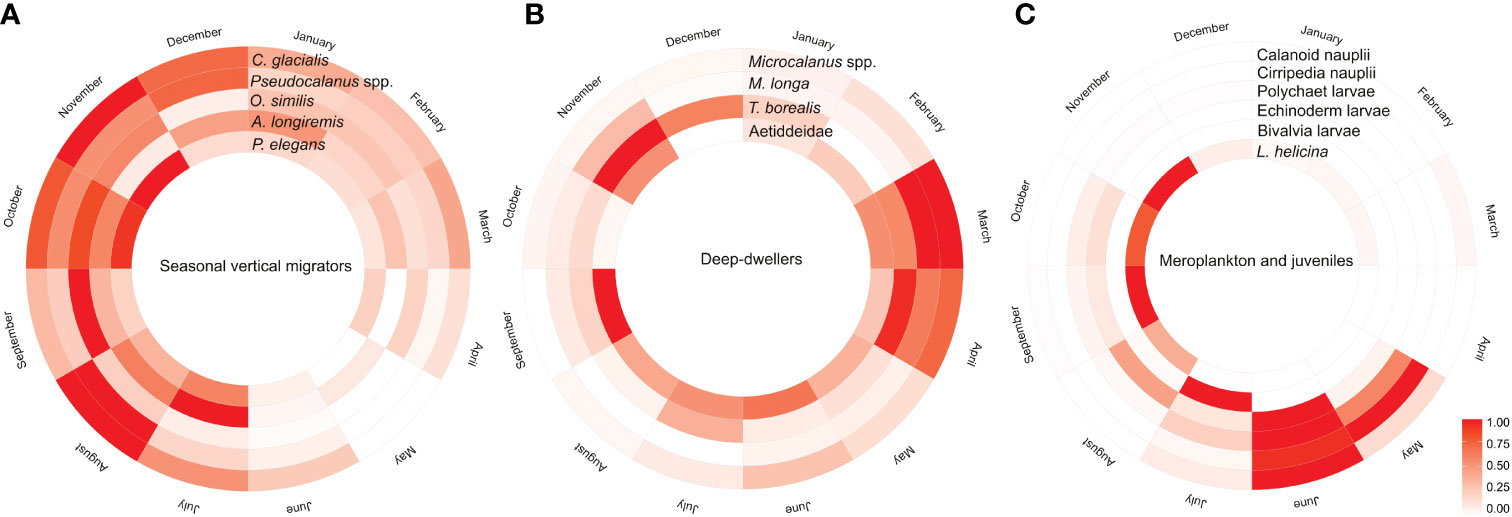
Figure 8 Circular heatmap summarizing the seasonal changes in relative abundance of (A) seasonal vertical migrators, (B) deep-dwellers, and (C) seasonal visitors occurring in high numbers during shorter periods such as larvae of benthic taxa (meroplankton) and holoplankton (copepod nauplii and juveniles of Limacina spp.).Color scale is a relative scale based on abundance (ind m−3), i.e., lowest abundance of each taxa = 0, the highest = 1, and all other values are linearly scaled accordingly so that dark color tones mark the month with highest abundance and lighter colors and white mark the month with lowest abundance.
Copepods of the family Aetideidae (mainly represented by B. similis) were constantly present and concentrated below 100 m year-round (WMD,<100 m) with highest abundances observed between May and September (Figures 7D, 8B).
3.4.3 Seasonal visitors
Larvae of benthic taxa (Meroplankton) and larvae/juveniles of holoplankton (copepod nauplii and L. helicina) occurred in seasonal pulses. Meroplankton and copepod nauplii were abundant in spring and summer, while juveniles of L. helicina were abundant in autumn (Table 2, Figure 8C). While the abundance of the different meroplanktonic larvae varied between years, the sequence of their appearance was similar (Supplementary Figure S3), and they were all primarily concentrated in the upper 50 m (data not shown). Cirripedia larvae (first nauplii, later cypris) appeared in May/June (up to 2,953 ind. m−3) together with Polychaeta larvae (up to 76 ind. m−3) and were largely concentrated in the upper 50 m (WMD, 10–48 m). Bivalvia larvae dominated the meroplankton community in July (up to 14,140 ind. m−3, Supplementary Table S1). They were concentrated in the surface layers during peak abundance (WMD, 13 m) but were found in low numbers at greater depth after their abundances decreased in August and September (WMD, 33–109 m). Pelagic larvae of macrobenthos (Hyas araneus and Eupagurus zoea) were found in surface layers in June/July but were of minor importance together with Bryozoa and Gastropod larvae, which peaked in abundance in September. Echinodermata larvae appeared for the first time in early May. They were present in low numbers throughout summer and early autumn occupying subsurface and intermediate water layers (WMD, 16–65 m).
Copepod nauplii (mostly Calanoid nauplii) were observed in low abundances (<10 ind. m−3) for the most parts of the year with high abundances observed between May and July (primarily C. glacialis and Pseudocalanus spp.), with a peak in June (up to 1,040 ind. m−3) (Figure 8C). From May to September, they were mainly found in the upper water column (WMD, 28–91 m), while the few ones discovered in winter was primarily found deep (WMD, 26–165 m) (Supplementary Figure S3). Peak abundances of copepodite stage CI of C. glacialis and Pseudocalanus spp. were found in June and July, respectively (Supplementary Figure S4). Copepodite stage 1 of the deep dwelling copepods of the family Aetideidae (primarily B. similis) were found all year-round concentrated below 100 m (WMD, 75–160) (Supplementary Figure S3)
Juveniles of L. helicina appeared in large numbers in surface and sub-surface waters in August–September (up to 1,973 ind. m−3) and persisted throughout autumn (Figure 8C) but descended to deeper water in October followed by a distinct drop in abundance (<10 ind. m−3) from December to July (Supplementary Figure S4).
4 Discussion
4.1 The arctic refuge
The true arctic fjord characteristics of Billefjorden was reflected in the species composition with cold-water species prevailing year-round (C. glacialis, P. minutes, and Microcalanus spp.) or representing important components of the community (M. longa, P. acuspes, L. helicina, and P. elegans) (Gluchowska et al., 2016). Species of more arcto-boreal provenance (C. finmarchicus, A. longiremis, T. borealis, and E. hamata) appeared in low numbers. The occasional occurrence of boreal species (Limacina retroversa, Evadne nordmanni, and Oithona atlantica, and deeper living Metridia lucens and Tomopteris helgolandicus) indicated that remnants of transformed Atlantic water (AW) can occasionally penetrate into Billefjorden. This was especially true during the ice-free summer and autumn when the upper 50 m (above the sill depth) receives occasional influx from Isfjorden (Skogseth et al., 2020). However, while extreme influxes of AW into the Isfjorden system were documented in autumn 2013 (Skogseth et al., 2020), Billefjorden remained relatively free of AW signatures and accompanied zooplankton taxa. We therefore conclude that advective processes are minor in Billefjorden and that the local in-fjord processes are the main ones. Interestingly, the total zooplankton abundance did not vary much across seasons in Billefjorden, and it was comparably higher to that found in ice-free Atlantic influenced fjords in Svalbard (Gluchowska et al., 2016; Hop et al., 2019). In terms of biomass, however, autumn was the season with the highest zooplankton biomass due to the high abundance of large, older copepodite stages of C. glacialis. Small-sized copepods (including young stages of Calanus spp.) and meroplankton (e.g., tiny Bivalvia larvae) dominated in numbers the other seasons, but these small taxa contribute little in terms of biomass (Blachowiak-Samolyk et al., 2008).
4.2 Seasonal patterns
The reoccurring seasonal patterns in the zooplankton community structure in Billefjorden are summarized in Figure 8. We identified three general patterns in the seasonal variation in abundance and vertical distribution of zooplankton: (1) seasonal migrators, taxa with distinct seasonal variability in their depth distribution, peaking in abundance in summer–autumn and showing lowest numbers in spring; (2) deep-dwelling zooplankton, taxa that remain at depth throughout the year being relatively more abundant in winter–spring compared to summer and autumn; and (3) seasonal visitors, taxa with temporarily limited occurrences in high numbers, i.e., meroplankton and other juveniles that peaked primarily in spring–summer at the surface but were largely absent during the rest of the year.
4.3 Seasonal migrators
Seasonal vertical migration (SVM) is a common feature among boreal and arctic zooplankton species (reviewed in Bandara et al., 2021). SVM is tightly connected to reproductive and overwintering strategies of species and is therefore usually an ontogenetical migration (e.g., Conover, 1988; Hirche, 1996; Madsen et al., 2001). SVM is an adaptation to deal with periodical resources limitation, which is typical for high latitudes, and is characterized by an upward migration in spring to utilize the high primary production in surface layers for reproduction, growth, and development, followed by a downward migration in autumn and a residence at greater depth during winter. The appearance of the spring bloom in early May every year in Billefjorden is similar to the timing of the spring bloom in ice-free fjords in Svalbard (Stübner et al., 2016; Hegseth et al., 2019). During the spring bloom and throughout summer, zooplankton abundance was highest in the surface 50 m, while the bulk of the zooplankton community moved to deeper waters during the non-productive autumn and winter. Seasonal migrators and seasonal visitors (meroplankton and copepod nauplii) were the cause for the dominant shallow distinct distribution pattern in bulk zooplankton in spring and summer.
In Billefjorden, SVM was displayed by the primarily herbivorous C. glacialis and Pseudocalanus spp., but also by the omnivore O. similis and the carnivore P. elegans. SVM is well documented for these species across boreal and Arctic seas (Kosobokova, 1999; Gislason and Astthorsson, 2004; Daase et al., 2013; Darnis and Fortier, 2014; Grigor et al., 2014; Lischka and Hagen, 2016). In C. glacialis and Pseudocalanus spp., the SVM was accompanied by ontogenetical changes in the population structure, with older copepodites descending during autumn to depths and ascending to surface the following spring while the young copepodites being abundant in surface waters during summer (Weydmann et al., 2013). Both species utilize the spring bloom for reproduction (Lischka and Hagen, 2005; Thor et al., 2005; Darnis and Fortier, 2014), and both displayed high seasonal variability in abundance with maxima in late summer and autumn, large decreases during winter, and very low numbers in spring. This indicates not only high mortality during winter (Daase and Søreide, 2021) but also successful recruitment during spring and summer, enabling them to build up large populations over a short period of time (e.g., Arnkværn et al., 2005; Leu et al., 2011; Hatlebakk et al., 2022).
The ascent of C. glacialis in spring seems to be a gradual process. Starting already in February, its WMD increased progressively. In a parallel study in 2012–2013, Freese et al. (2017) found that lipid catabolic activity increased and lipid content decreased in C. glacialis in Billefjorden from December to April. Gonad maturation and egg production (capital breeding) are energy demanding, and to start to prepare for reproduction so far in advance of the spring bloom increases the risk for resource limitation and non-consumptive mortality (Daase and Søreide, 2021). By early April, almost half of population was located at 50–100 m, and in the beginning of May, 87% was found above 100 m. The occurrence of young copepodites of C. glacialis in July 2011 and May–June 2012–2013 (Supplementary Figure S3) indicates similar timing of reproduction in Billefjorden as in Amundsen Gulf and other fjords in Svalbard (Daase et al., 2013; Hatlebakk et al., 2022), and Western Greenland (Madsen et al., 2001). The descent of overwintering CIV–V to depths was accomplished in a shorter period of time from late July to August (Freese et al., 2017).
Pseudocalanus spp. was distributed somewhat higher up in the water column than C. glacialis during winter and spring. In Amundsen Gulf, Pseudocalanus spp. was defined as the most epipelagic copepod with only weak SVM (Darnis and Fortier, 2014). Copepodite stages CIII–CV were present year-round, confirming that these are the main overwintering stages (Ussing, 1938; Lischka and Hagen, 2005; Lischka and Hagen, 2016; Ershova et al., 2021b). CI peaked in July (Supplementary Figure S3), indicating that reproduction occurred in May–June (Ershova et al., 2021b). This phenology is similar to that of Pseudocalanus populations in Kongsfjorden and in northern Norway (Norrbin, 1991; Halvorsen and Tande, 1999). CIs were present throughout autumn, indicating reproduction occurring throughout summer and not being restricted to the main phytoplankton bloom, which is most likely due to the presence of both P. minutes and P. acuspes that reproduce primarily in respective spring and summer–autumn (Ershova et al., 2021b). Pseudocalanus minutes is larger and more lipid-rich than P. acuspes (Boissonnot et al., 2016) and is also known to utilize the early ice algae food source to speed up reproduction (Conover et al., 1986). Pseudocalanus spp. may be able to utilize the post bloom much more efficiently than C. glacialis, being able to capture smaller algae cells and microplankton that become more important as food source throughout summer and autumn (Hegseth et al., 2019). Being more active during winter, they may invest in prolonged reproductive periods rather than in growth and lipid storage (Barth-Jensen et al., 2022). The boreal Pseudocalanus moultoni is morphologically very similar to P. acuspes, and this species was most likely also present in Billefjorden during this study (e.g., Ershova et al., 2021b). However, its strong decline in numbers over winter combined with no nauplii being identified to P. moultoni suggests that P. moultoni is not actively reproducing in Billefjorden (Ershova et al., 2021b).
The timing of decent and ascent was slightly shifted in O. smilis compared to C. glacialis and Pseudocalanus spp. Oithona similis became abundant in the upper 50 m first in August and peaked in abundance during autumn similarly to observations by Balazy et al. (2021) in the nearby Adventfjorden. At this time, C. glacialis and Pseudocalanus spp. had already descended to greater depths, leaving the surface waters to O. similis to exploit and reproduce with little competition (Balazy et al., 2021). Oithona similis is omnivorous, with predilection for motile prey and is feeding actively during summer, autumn, and winter (Lischka and Hagen, 2016), even though it is also able to accumulate energy stores in the form of lipids (Narcy et al., 2009). Oithona similis remained in the upper 100 m throughout autumn, descending to deeper layers as late as January. The relatively lower extent of SVM in O. similis is consistent with observations from the Beaufort Sea, where epipelagic O. similis exhibited only modest amplitude of SVM (Darnis and Fortier, 2014).
Parasagitta elegans is a common predator in the Arctic (Søreide et al., 2003; Blachowiak-Samolyk et al., 2008) and subarctic waters (Gislason and Astthorsson, 1998; Terazaki, 1998; Abramova and Tuschling, 2005) and was the most abundant carnivore with year-round presence in Billefjorden. We found the SVM pattern of P. elegans to mirror that of the copepods, indicating that this behavior is a response to the SVM conducted by their prey organisms, such as Calanus and smaller copepods (Terazaki, 1998; Grigor et al., 2014; Bandara et al., 2016). Parasagitta elegans also exhibited seasonal and interannual variability in abundance, which may be related to food abundance. Chaetognaths can have high predatory impact on plankton communities (Arashkevich et al., 2002; Baier and Terazaki, 2005). For instance, the strong decline in Pseudocalanus spp. abundance during winter 2013 coincided with a consistent presence of P. elegans and high abundance of ctenophores, suggesting predation pressure as the source for the high mortality in parallel with non-consumptive mortality due to resource shortage in the copepods when they molt and invest in gonad maturation (Daase & Søreide, 2021).
4.4 Year-round deep-dwellers
In contrast to the SVM behavior observed in herbivorous copepods and their most abundant predator, a number of taxa displayed only small changes in their vertical distribution, remaining below the euphotic zone year-round. At depths, microbial processes dominate (e.g., Bradford-Grieve et al., 1999), so dwelling deeper puts restrictions on a herbivorous feeding mode, and taxa with omnivorous, detrivorous, or carnivorous feeding strategies are mainly encountered (e.g., Yamaguchi et al., 2022).
The most spatially confined distribution was found in copepods of the family Aetideidae, which were found almost exclusive in the deepest layers. Little is known about the life cycles of these species (Bradford-Grieve, 2004), since they likely are regularly under-sampled in plankton tows that do not sample efficiently close to the sea floor, but the year-round presence of the youngest copepodite stages and nauplii of Aetideidae suggests continuous reproduction (Barth-Jensen et al., 2022).
Persistent year-round preference for deep waters was also observed in the abundant small-sized copepods Microcalanus spp. and T. borealis and the less abundant large M. longa. Vertical distribution patterns of M. longa should be taken with caution due to its relatively low abundance in our study and its tendency to conduct diel vertical migration (Daase et al., 2008). No clear SVM was observed in these three species in the Amundsen Gulf (Darnis and Fortier, 2014) nor during a drift study in the Beaufort Sea and the Chukchi Sea (Ashjian et al., 2003). However, signs of SVM were found in the permanently ice-covered central Arctic basins (Geinrikh et al., 1983).
Microcalanus spp. displayed small upward movements in July and a subsequent dispersion over intermediate layers during autumn. This is in accordance with observations from the Southern Ocean where M. pygmaeus spent late winter and early spring at greater depth, ascending to intermediate depths in summer and descending during autumn (Schnack-Schiel and Mizdalski, 1994).
4.5 Seasonal visitors
The third persistent pattern observed in the zooplankton community was the mass occurrence of larvae and juveniles of various taxa in the surface and subsurface strata in spring and summer. These taxa dominated the zooplankton community in terms of numbers during periods of mass occurrence. Increased temperatures and sufficient food availability shorten larval development time, thereby increasing larval survival and thus facilitating the outburst of juveniles and larvae during the phytoplankton bloom in surface layers (Fenaux et al., 1994). The overall low ice algae biomass in Billefjorden combined with the observation of high numbers of cirripedia in May–June suggests that it is the phytoplankton bloom that triggers the release of cirripedia nauplii (e.g. Stübner et al., 2016).
Due to the mesh size used (180–200 µm), our abundance estimates of larvae and juveniles are likely biased, as some larvae forms may be too small to be caught quantitatively. However, the different meroplankton taxa appeared in repeatable sequence each year, and the timing of peak abundances and succession of the different taxa in Billefjorden resembled the phenology in Adventfjorden (Kuklinski et al., 2013; Stübner et al., 2016) and Kongsfjorden (Lischka and Hagen, 2016): an early outburst of Cirripedia associated with an appearance of Polychaeta larvae in May-June was followed by a much larger peak in abundance of Bivalvia veliger in July, while Echinodermata larvae were present between May and October.
The sequence in emergence of different meroplankton taxa offers an additional insight into life history strategies of their benthic parents. Cirripedia, polychaets, and echinoderms appear to have adopted a capital breeding strategy and do not require external food intake to produce and to release their larvae but can release offspring simultaneously with the spring bloom, thus enabling their offspring to utilize the phytoplankton bloom to its full extent (Kuklinski et al., 2013). The exceptionally regular occurrence of Bivalvia larvae each year in July indicates an income breeding strategy, relying on pelagic bloom for maturation and reproduction and also highly synchronized spawning (Kuklinski et al., 2013). Furthermore, the occurrence of meroplankton in high abundances implies a rich benthic community and a strong pelagic–benthic coupling in Billefjorden. The importance of meroplankton is a common feature in coastal arctic and subarctic systems—high occurrence have been reported from, e.g., Icelandic waters where ophiuroid larvae accounted for 9% in total and 22% in July (Gislason and Astthorsson, 1998) and from the White Sea (Pertsova and Kosobokova, 2003), Greenland (Arendt et al., 2012), and the Beaufort Sea (Walkusz et al., 2013).
Another group that was characterized by highly seasonal peaks in abundance were mucus filtrators and, in particular, juveniles of the arctic pteropod L. helicina, which appeared in large numbers in August–September and persisted throughout autumn. Mucus filtrators have very high ingestion rates and play an important ecological role in the carbon transport from the euphotic layer to the sea floor by discarding mucous feeding webs, which, by flocculation with other particles, enhance aggregates formation and their downward vertical fluxes (Alldredge and Gotschalk, 1988; Hunt et al., 2008). Limacina helicina is also a major food source for a wide spectrum of predators (Gannefors et al., 2005).
Abundance of L. helicina is notoriously difficult to assess due to their highly patchy distribution, not only on annual or seasonal but also on daily and even hourly scale (Boissonnot et al., 2021). The presence of large numbers of juveniles in August–September indicates that juveniles are the main overwintering stage and not veligers as previously suggested (Gannefors et al., 2005; Lischka and Riebesell, 2012; Lischka and Hagen, 2016), which is in accordance with the year-round study by Boissonnot et al. (2021) in nearby Adventfjorden. In 2013, juvenile Limacina were absent between June and September, and high numbers of veligers occurred first in September, indicating that spawning was delayed that year.
The seasonal variation in the vertical distribution is poorly documented in pteropods (Hunt et al., 2008). Due to the highly patchy occurrence of L. helicina in Billefjorden, we can also only present a fragmented picture of the seasonal variability in their vertical distribution. The aggregation in surface/subsurface waters in August/September agrees with observations from coastal and oceanic waters of northern Norway (Halvorsen and Tande, 1999), the western Baffin Bay (Buchanan and Sekerak, 1982), and Rijpfjorden (Weydmann et al., 2013). In mid-winter, L. helicina was concentrated at greater depth; by early spring, they were gathered in the upper 50 m, but abundance was generally low in winter and spring. Winter data from southern hemisphere indicated that L. helicina antarctica can reduce their metabolic activity in response to low food availability (Seibel and Dierssen, 2003), but they will normally continue feeding throughout winter (Hunt et al., 2008). Thus, the variable vertical distribution in winter may be governed by both predator avoidance and food availability.
5 Concluding remark
The re-occurring mesozooplankton patterns identified in Billefjorden in years 2011–2013 provide a seasonal baseline that will serve as an important reference to differentiate between climate change impacts and natural mesozooplankton variability in Arctic shelf seas and fjords.
The variability in the zooplankton community structure and vertical distribution is best explained by the factor Season, which correlates to the seasonal changes in underwater light climate and algal food availability. Larvae and juveniles occur primarily when algal food is abundant. The timing of the spring bloom is therefore steering the phenology of most taxa, either directly (herbivores/seasonal migrators) or indirectly (omnivores/predators). Depending on zooplankton life history strategies, their occurrence in surface layers may be episodic (meroplankton), restricted to summer (herbivores), or prolonged until autumn (omnivores). Winter is characterized by high mortality in herbivorous and seasonal migrating species. Despite this high winter mortality, these taxa quickly rebuild their population over the summer. Deep-dwelling, omnivorous species are less seasonally restricted and often show peak abundances in winter and spring when herbivorous populations are severely depleted (Figure 8). These taxa often have an extended or year-round reproductive period.
The different life histories, depth preferences, and timing of reproduction among coexisting mesozooplankton taxa revealed in this study have a straightforward ecological meaning, as they reduce the inter-specific competition and allow for a relatively high and constant zooplankton abundance year-round despite the short window of primary production in high-Arctic fjords and shelf seas.
Data availability statement
The original contributions presented in the study are included in the article/Supplementary Material. Further inquiries can be directed to the corresponding authors.
Author contributions
Scientific idea and design JS, MD Sampling JS, MD Morphological identification KD Data analyses MD, KD, JS, ET Manuscript preparation KD, JS, MD, KB-S, ET. All authors contributed to the article and approved the submitted version.
Funding
This study was conducted in the frame of the project FACE-IT (The Future of Arctic Coastal Ecosystems – Identifying Transitions in Fjord Systems and Adjacent Coastal Areas). FACE-IT has received funding from the European Union’s Horizon 2020 research and innovation programme under grant agreement No 869154. Funding was also provided by the Research Council of Norway (RCN) through the projects CLEOPATRA II (216537/E10), IMOS (246747) and COPPY (227139), the Fram Centre, Norway (Flagship Arctic Ocean, project FADE 2019-2021) and the Polish Ministry of Science and Higher Education (project 3550/Norway/2016/2). Further funding was provided through the 2017-2018 Belmont Forum and BiodivERsA joint call for research proposals, under the BiodivScen ERA-Net COFUND programme, and the funding organisations RCN (#296836/31406) and National Science Center, Poland (NCN # 31382).In addition, M. Daase received extra funding through the NRC project Deep Impact (#300333).
Acknowledgments
We thank the captains and crew of RV Helmer Hanssen, RV Lance, RV Viking Explorer, KV Svalbard, MS Farm, and UNIS logistics for making it possible to conduct the year-round sampling for this study. We are grateful for the help provided by colleagues and UNIS students participating in some of the field campaigns, especially M.K. Hatlebakk, L. Boissonnot, and D. Freese. This study is part of Isfjorden Marine Observatory Svalbard (IMOS) and a contribution to the ARCTOS research network (arctos.uit.no).
Conflict of interest
The authors declare that the research was conducted in the absence of any commercial or financial relationships that could be construed as a potential conflict of interest.
Publisher’s note
All claims expressed in this article are solely those of the authors and do not necessarily represent those of their affiliated organizations, or those of the publisher, the editors and the reviewers. Any product that may be evaluated in this article, or claim that may be made by its manufacturer, is not guaranteed or endorsed by the publisher.
Supplementary material
The Supplementary Material for this article can be found online at: https://www.frontiersin.org/articles/10.3389/fmars.2022.933461/full#supplementary-material
References
Abramova E., Tuschling K. (2005). A 12-year study of the seasonal and interannual dynamics of mesozooplankton in the laptev Sea: Significance of salinity regime and life cycle patterns. Global Planetary Change 48, 141–164. doi: 10.1016/j.gloplacha.2004.12.010
Alldredge A. L., Gotschalk C. (1988). In situ settling behavior of marine snow. Limnol Oceanogr 33, 339–351. doi: 10.4319/lo.1988.33.3.0339
Anderson M., Gorley R., Clarke K. (2008). PERMANOVA for PRIMER: guide to software and statistical methods (Plymouth, UK: Primer-e), 32.
Arashkevich E., Wassmann P., Pasternak A., Riser C. W. (2002). Seasonal and spatial changes in biomass, structure, and development progress of the zooplankton community in the Barents Sea. J. Mar. Syst. 38, 125–145. doi: 10.1016/S0924-7963(02)00173-2
Arendt K. E., Juul-Pedersen T., Mortensen J., Blicher M. E., Rysgaard S. (2012). A 5-year study of seasonal patterns in mesozooplankton community structure in a sub-Arctic fjord reveals dominance of microsetella norvegica (Crustacea, copepoda). J. Plankt Res. 35, 105–120. doi: 10.1093/plankt/fbs087
Arnkværn G., Daase M., Eiane K. (2005). Dynamics of coexisting Calanus finmarchicus, Calanus glacialis and Calanus hyperboreus populations in a high-Arctic fjord. Polar Biol. 28, 528–538. doi: 10.1007/s00300-005-0715-8
Ashjian C. J., Campbell R. G., Welch H. E., Butler M., Van Keuren D. (2003). Annual cycle in abundance, distribution, and size in relation to hydrography of important copepod species in the western Arctic ocean. Deep-Sea Res. Part I 50, 1235–1261. doi: 10.1016/S0967-0637(03)00129-8
Baier C. T., Terazaki M. (2005). Interannual variability in a predator–prey interaction: climate, chaetognaths and copepods in the southeastern Bering Sea. J. Plankt Res. 27, 1113–1125. doi: 10.1093/plankt/fbi078
Balazy K., Boehnke R., Trudnowska E., Søreide J. E., Błachowiak-Samołyk K. (2021). Phenology of Oithona similis demonstrates that ecological flexibility may be a winning trait in the warming Arctic. Sci. Rep. 11, 18599. doi: 10.1038/s41598-021-98068-8
Bandara K., Varpe Ø, Søreide J. E., Wallenschus J., Berge J., Eiane K. (2016). Seasonal vertical strategies in a high-Arctic coastal zooplankton community. Mar. Ecol. Prog. Ser. 555, 49–64. doi: 10.3354/meps11831
Bandara K., Varpe Ø., Wijewardene L., Tverberg V., Eiane K. (2021). Two hundred years of zooplankton vertical migration research. Biol. Rev. doi: 10.1111/brv.12715
Barth-Jensen C., Daase M., Ormańczyk M. R., Varpe Ø, Kwaśniewski S., Svensen C. (2022). High abundances of small copepods early developmental stages and nauplii strengthen the perception of a non-dormant Arctic winter. Polar Biol 45, 675–90. doi: 10.1007/s00300-022-03025-4
Berge J., Daase M., Hobbs L., Falk-Petersen S., Darnis G., Søreide J.E. (2020). Zooplankton in the polar night. In Polar night marine ecology. advances in polar ecology Berge J., Johnsen G., Cohen J. Eds. (Cham: Springer) 4.
Blachowiak-Samolyk K., Kwasniewski S., Hop H., Falk-Petersen S. (2008). Magnitude of mesozooplankton variability: A case study from the marginal ice zone of the Barents Sea in spring. J. Plankt Res. 30, 311–323. doi: 10.1093/plankt/fbn002
Boissonnot L., Kohnert P., Ehrenfels B., Søreide J. E., Graeve M., Stübner E., et al. (2021). Year-round population dynamics of Limacina spp. early stages in a high-Arctic fjord (Adventfjorden, Svalbard). Polar Biol. 44, 1605–1618. doi: 10.1007/s00300-021-02904-6
Boissonnot L., Niehoff B., Hagen W., Søreide J. E., Graeve M. (2016). Lipid turnover reflects life-cycle strategies of small-sized Arctic copepods. J. Plankton Res. 38, 1420–1432. doi: 10.1093/plankt/fbw076
Bradford-Grieve J. M. (2004). Deep-sea benthopelagic calanoid copepods and their colonization of the near-bottom environment. Zoological Stud. 43, 276–291.
Bradford-Grieve J. M., Boyd P. W., Chang F. H., Chiswell S., Hadfield M., Hall J. A., et al. (1999). Pelagic ecosystem structure and functioning in the subtropical front region east of new Zealand in austral winter and spring 1993. J. Plankt Res. 21, 405–428. doi: 10.1093/plankt/21.3.405
Buchanan R. A., Sekerak A. D. (1982). Vertical distribution of zooplankton in Eastern Lancaster sound and Western Baffin-bay, July October 1978. Arctic 35, 41–55. doi: 10.14430/arctic2306
Clarke K. R., Warwick R. M. (2001). Change in marine communities: An approach to statistical analysis and interpretation. 2 (Plymouth: PRIMER-E).
Cohen J. H., Berge J., Moline M. A., Johnsen G., Zolich A. P. (2020). “Light in the polar night,” in Polar night marine ecology – life and light in the dead of the night, vol. 3 . Eds. Berge J., Johnsen G., Cohen J. H. (Cham: Springer, Springer Nature Switzerland), 38–62.
Conover R. J. (1988). Comparative life histories in the genera Calanus and Neocalanus in high latitudes of the northern hemisphere. Hydrobiologia 167, 127–142. doi: 10.1007/BF00026299
Conover R. J., Herman A. W., Prinsenberg S. J., Harris L. R. (1986). Distribution of and feeding by the copepod Pseudocalanus under fast ice during the arctic spring. Sci. (80) 232, 1245–1247. doi: 10.1126/science.232.4755.1245
Cottier F., Tverberg V., Inall M., Svendsen H., Nilsen F., Griffiths C. (2005). Water mass modification in an Arctic fjord through cross-shelf exchange: The seasonal hydrography of kongsfjorden, Svalbard. J. Geophys Res- Oceans 110, C12005. doi: 10.11029/12004JC002757
Daase M., Eiane K., Aksnes D. L., Vogedes D. (2008). Vertical distribution of Calanus spp. and Metridia longa at four Arctic locations. Mar. Biol. Res. 4, 193–207. doi: 10.1080/17451000801907948
Daase M., Falk-Petersen S., Varpe Ø, Darnis G., Søreide J. E., Wold A., et al. (2013). Timing of reproductive events in the marine copepod Calanus glacialis: a pan-Arctic perspective. Can. J. Fish Aquat Sci. 70, 871–884. doi: 10.1139/cjfas-2012-0401
Daase M., Hop H., Falk-Petersen S. (2016). Small-scale diel vertical migration of zooplankton in the high Arctic. Polar Biol. 39, 1213–1223. doi: 10.1007/s00300-015-1840-7
Daase M., Søreide J. E. (2021). Seasonal variability in non-consumptive mortality of Arctic zooplankton. J. Plankt Res. 43, 565–585. doi: 10.1093/plankt/fbab042
Darnis G., Fortier L. (2014). Temperature, food and the seasonal vertical migration of key arctic copepods in the thermally stratified Amundsen gulf (Beaufort Sea, Arctic ocean). J. Plankt Res. 36, 1092–1108. doi: 10.1093/plankt/fbu035
Ejsmond M. J., McNamara J. M., Søreide J., Varpe Ø (2018). Gradients of season length and mortality risk cause shifts in body size, reserves and reproductive strategies of determinate growers. Funct. Ecol. 32, 2395–2406. doi: 10.1111/1365-2435.13191
Ershova E. A., Kosobokova K. N., Banas N. S., Ellingsen I., Niehoff B., Hildebrandt N., et al. (2021a). Sea Ice decline drives biogeographical shifts of key Calanus species in the central Arctic ocean. Glob Change Biol 27, 2128–43. doi: 10.1111/gcb.15562
Ershova E. A., Nyeggen M. U., Yurikova D. A., Søreide J. E. (2021b). Seasonal dynamics and life histories of three sympatric species of Pseudocalanus in two Svalbard fjords. J. Plankt Res. 43, 209–223. doi: 10.1093/plankt/fbab007
Falk-Petersen S., Timofeev S., Pavlov V., Sargent J. R. (2007). “Climate variability and the effect on Arctic food chains. the role of calanus,” in Arctic-Alpine ecosystems and people in a changing environment. Eds. Ørbæk J. R., Tombre T., Kallenborn R., Hegseth E., Falk-Petersen S., Hoel A. H. (Berlin: Springer), 147–166.
Fenaux L., Strathmann M. F., Strathmann R. A. (1994). Five tests of food-limited growth of larvae in coastal waters by comparisons of rates of development and form of echinoplutei. Limnol Oceanogr 39, 84–98. doi: 10.4319/lo.1994.39.1.0084
Freese D., Søreide J. E., Graeve M., Niehoff B. (2017). A year-round study on metabolic enzymes and body composition of the Arctic copepod Calanus glacialis: implications for the timing and intensity of diapause. Mar. Biol. 164, 3. doi: 10.1007/s00227-016-3036-2
Freese D., Søreide J. E., Niehoff B. (2016). A year-round study on digestive enzymes in the Arctic copepod Calanus glacialis: Implications for its capability to adjust to changing environmental conditions. Polar Biol. 39, 2241–2252. doi: 10.1007/s00300-016-1891-4
Gabrielsen T. M., Merkel B., Søreide J. E., Johansson-Karlsson E., Bailey A., Vogedes D., et al. (2012). Potential misidentifications of two climate indicator species of the marine arctic ecosystem: Calanus glacialis and C. finmarchicus. Polar Biol 35, 1621–28. doi: 10.1007/s00300-012-1202-7
Gannefors C., Boer M., Kattner G., Graeve M., Eiane K., Gulliksen B., et al. (2005). The Arctic sea butterfly Limacina helicina: lipids and life strategy. Mar. Biol. 147, 169–177. doi: 10.1007/s00227-004-1544-y
Geinrikh A. K., Kosobokova K., Rudyakov Y. A. (1983). Seasonal variations in the vertical distribution of some prolific copepods of the Arctic basin. Can. Translation Fisheries Aquat. Sci. 4925, 1–22.
Gislason A., Astthorsson O. S. (1998). Seasonal variations in biomass, abundance and composition of zooplankton in the subarctic waters north of Iceland. Polar Biol. 20, 85–94. doi: 10.1007/s003000050280
Gislason A., Astthorsson O. S. (2004). Distribution patterns of zooplankton communities around Iceland in spring. Sarsia 89, 467–477. doi: 10.1080/00364820410009256
Gluchowska M., Kwasniewski S., Prominska A., Olszewska A., Goszczko I., Falk-Petersen S., et al. (2016). Zooplankton in Svalbard fjords on the Atlantic–Arctic boundary. Polar Biol. 39, 1785–1802. doi: 10.1007/s00300-016-1991-1
Grigor J. J., Søreide J. E., Varpe Ø (2014). Seasonal ecology and life-history strategy of the high-latitude predatory zooplankter Parasagitta elegans. Mar. Ecol. Prog. Ser. 499, 77–88. doi: 10.3354/meps10676
Hagen W., Auel H. (2001). Seasonal adaptations and the role of lipids in oceanic zooplankton. Zoology 104, 313–326. doi: 10.1078/0944-2006-00037
Halvorsen E., Tande K. S. (1999). Physical and biological factors influencing the seasonal variation in distribution of zooplankton across the shelf at Nordvestbanken, northern Norway. Sarsia 84, 279–292. doi: 10.1080/00364827.1999.10420432
Hatlebakk M., Kosobokova K., Daase M., Søreide J. E. (2022). Contrasting life traits of sympatric Calanus glacialis and C. finmarchicus in a warming Arctic revealed by a year-round study in Isfjorden, Svalbard. Front. Mar. Sci. 9. doi: 10.3389/fmars.2022.877910
Hegseth E. N., Assmy P., Wiktor J. M., Wiktor J., Kristiansen S., Leu E., et al. (2019). “Phytoplankton seasonal dynamics in kongsfjorden, Svalbard and the adjacent shelf,” in The ecosystem of kongsfjorden. Eds. Hop H., Wiencke C. (Svalbard: Springer International Publishing, Cham), 173–227. doi: 10.1007/978-3-319-46425-1_6
Hirche H. J. (1996). Diapause in the marine copepod, Calanus finmarchicus - a review. Ophelia 44, 129–143. doi: 10.1080/00785326.1995.10429843
Hop H., Falk-Petersen S., Svendsen H., Kwasniewski S., Pavlov V., Pavlova O., et al. (2006). Physical and biological characteristics of the pelagic system across fram strait to kongsfjorden. Prog. Oceanogr 71, 182–231. doi: 10.1016/j.pocean.2006.09.007
Hop H., Pearson T., Hegseth E. N., Kovacs K. M., Wiencke C., Kwasniewski S., et al. (2002). The marine ecosystem of kongsfjorden, Svalbard. Polar Res. 21, 167–208. doi: 10.1111/j.1751-8369.2002.tb00073.x
Hop H., Wold A., Vihtakari M., Daase M., Kwasniewski S., Gluchowska M., et al. (2019). “Zooplankton in kongsfjorde–2016) in relation to climate change,” in The ecosystem of kongsfjorden. Eds. Hop H., Wiencke C. (Svalbard: Springer International Publishing, Cham), 229–300. doi: 10.1007/978-3-319-46425-1_7
Hunt B. P. V., Pakhomov E. A., Hosie G. W., Siegel V., Ward P., Bernard K. (2008). Pteropods in southern ocean ecosystems. Prog. Oceanogr 78, 193–221. doi: 10.1016/j.pocean.2008.06.001
Ingvaldsen R. B., Assmann K. M., Primicerio R., Fossheim M., Polyakov I. V., Dolgov A. V. (2021). Physical manifestations and ecological implications of Arctic atlantification. Nat. Rev. Earth Environ. 2, 874–889. doi: 10.1038/s43017-021-00228-x
Kahru M., Brotas V., Manzano-Sarabia M., Mitchell B. G. (2011). Are phytoplankton blooms occurring earlier in the Arctic? Glob Change Biol. 17, 1733–1739. doi: 10.1111/j.1365-2486.2010.02312.x
Kassambara A., Mundt F. (2020). Factoextra: Extract and visualize the results of multivariate data analyses. r package version 1.0.7. https://CRAN.R-project.org/package=factoextra
Kosobokova K. N. (1999). The reproductive cycle and life history of the Arctic copepod Calanus glacialis in the white Sea. Polar Biol. 22, 254–263. doi: 10.1007/s003000050418
Kuklinski P., Berge J., McFadden L., Dmoch K., Zajaczkowski M., Nygård H., et al. (2013). Seasonality of occurrence and recruitment of Arctic marine benthic invertebrate larvae in relation to environmental variables. Polar Biol. 36, 549–60. doi: 10.1007/s00300-012-1283-3
Kwasniewski S., Hop H., Falk-Petersen S., Pedersen G. (2003). Distribution of Calanus species in kongsfjorden, a glacial fjord in Svalbard. J. Plankt Res. 25, 1–20. doi: 10.1093/plankt/25.1.1
Leu E., Mundy C. J., Assmy P., Campbell K., Gabrielsen T. M., Gosselin M., et al. (2015). Arctic Spring awakening – steering principles behind the phenology of vernal ice algal blooms. Prog. Oceanogr 139, 151–170. doi: 10.1016/j.pocean.2015.07.012
Leu E., Søreide J. E., Hessen D. O., Falk-Petersen S., Berge J. (2011). Consequences of changing sea-ice cover for primary and secondary producers in the European Arctic shelf seas: Timing, quantity, and quality. Prog. Oceanogr 90, 18–32. doi: 10.1016/j.pocean.2011.02.004
Lischka S., Hagen W. (2005). Life histories of the copepods Pseudocalanus minutus, p. acuspes (Calanoida) and Oithona similis (Cyclopoida) in the Arctic kongsfjorden (Svalbard). Polar Biol. 28, 910–921. doi: 10.1007/s00300-005-0017-1
Lischka S., Hagen W. (2016). Seasonal dynamics of mesozooplankton in the Arctic kongsfjord (Svalbard) during year-round observations from august 1998 to July 1999. Polar Biol. 39, 1859–78. doi: 10.1007/s00300-016-2005-z
Lischka S., Riebesell U. (2012). Synergistic effects of ocean acidification and warming on overwintering pteropods in the Arctic. Glob Change Biol. 18, 3517–3528. doi: 10.1111/gcb.12020
Madsen S. D., Nielsen T. G., Hansen B. W. (2001). Annual population development and production by Calanus finmarchicus, c. glacialis and C. hyperboreus in disko bay, western Greenland. Mar. Biol. 139, 75–93. doi: 10.1007/s002270100552
Maechler M., Rousseeuw P., Struyf A., Hubert M., Hornik K. (2019). Cluster: Cluster analysis basics and extensions. r package version 2.1.0.
Manly B. J. F. (1977). A further note on Kiritani and Nakasuji’s model for stage frequency data including comments on the use of Tukey’s jackknife technique for estimating variances. Res. Popul. Ecol. 18, 177–86.
Muckenhuber S., Nilsen F., Korosov A., Sandven S. (2016). Sea Ice cover in Isfjorden and hornsund, svalbar–2014) from remote sensing data. Cryosphere 10, 149–158. doi: 10.5194/tc-10-149-2016
Narcy F., Gasparini S., Falk-Petersen S., Mayzaud P. (2009). Seasonal and individual variability of lipid reserves in Oithona similis (Cyclopoida) in an Arctic fjord. Polar Biol. 32, 233–242. doi: 10.1007/s00300-008-0524-y
Nilsen F., Cottier F., Skogseth R., Mattson S. (2008). Fjord– shelf exchanges controlled by ice and brine production: The interannual variation of Atlantic water in Isfjorden, Svalbard. Cont Shelf Res. 28, 1838–1853. doi: 10.1016/j.csr.2008.04.015
Norrbin M. F. (1991). Gonad maturation as an indication of seasonal cycles for several species of small copepods in the Barents Sea. Polar Res. 10, 421–432. doi: 10.1111/j.1751-8369.1991.tb00663.x
Pertsova N. M., Kosobokova K. N. (2003). Zooplankton of the white Sea: Features of the composition and structure, seasonal dynamics, and the contribution to the formation of matter fluxes. Oceanology 43, S108–S122.
R Core Team (2020). R: A language and environment for statistical computing r foundation for statistical computing (Vienna, Austria).
Richter C. (1994). Regional and seasonal variability in the vertical distribution of mesozooplankton in the Greenland Sea. Berichte zur Polarforschung 154, 1–87. doi: 10.2312/BzP_0154_1994
Søreide J. E., Hop H., Falk-Petersen S., Gulliksen B., Hansen E. (2003). Macrozooplankton communities and environmental variables in the Barents Sea marginal ice zone in late winter and spring. Mar. Ecol. Prog. Ser. 263, 43–64. doi: 10.3354/meps263043
Søreide J. E., Leu E., Berge J., Graeve M., Falk-Petersen S. (2010). Timining in blooms, algal food quality and Calanus glacialis reproduction and growth in a changing Arctic. Glob Change Biol. 16, 3154–3163. doi: 10.1111/j.1365-2486.2010.02175
Sainmont J., Andersen K. H., Varpe Ø, Visser A. W. (2014). Capital versus income breeding in a seasonal environment. Am. Naturalist. 184, 466–476. doi: 10.1086/677926
Sakshaug E. (2004). “Primary and secondary production in the Arctic seas,” in The organic carbon cycle in the Arctic ocean. Eds. Stein R., Macdonald R. W. (Berlin: Springer Verlag), 57–82.
Schnack-Schiel S. B., Mizdalski E. (1994). Seasonal variations in distribution and population structure of Microcalanus pygmaeus and ctenocalanus citer (Copepoda: Calanoida) in the eastern Weddell Sea, Antarctica. Mar. Biol. 119, 357–366. doi: 10.1007/BF00347532
Seibel B. A., Dierssen H. M. (2003). Cascading trophic impacts of reduced biomass in the Ross Sea, Antarctica: Just the tip of the iceberg? Biol. Bull. 205, 93–97. doi: 10.2307/1543229
Skogseth R., Oliviera L. L. A., Nilsen F., Falck E., Fraser N., Tverberg V., et al. (2020). Variability and decadal trends in the Isfjorden (Svalbard) ocean climate and circulation – an indicator for climate change in the European Arctic. Prog. Oceanogr. 187, 102394. doi: 10.1016/j.pocean.2020.102394
Stübner E. I., Søreide J. E., Reigstad M., Marquardt M., Blachowiak-Samolyk K. (2016). Year-round meroplankton dynamics in high-Arctic Svalbard. J. Plankt Res. 38, 522–536. doi: 10.1093/plankt/fbv124
Terazaki M. (1998). Life history, distribution, seasonal variability and feeding of the pelagic chaetognath Sagitta elegans in the subarctic pacific: a review. Plankton Biol. Ecol. 45, 1–17.
Thor P., Nielsen T. G., Tiselius P., Juul-Pedersen T., Michel C., Moller E. F., et al. (2005). Post-spring bloom community structure of pelagic copepods in the Disko Bay, western Greenland. J. Plankt Res. 27, 341–356. doi: 10.1093/plankt/fbi010
Ussing H. H. (1938). The biology of some important plankton animals in the fjords of east Greenland. Medd Grønl 100, 1–108.
Varpe Ø, Jørgensen C., Tarling G. A., Fiksen Ø (2007). Early is better: seasonal egg fitness and timing of reproduction in a zooplankton life-history model. Oikos 116, 1331–1342. doi: 10.1111/j.0030-1299.2007.15893.x
Vihtakari M., Welcker J., Moe B., Chastel O., Tartu S., Jop H., et al. (2018). Black-legged kittiwakes as messengers of atlantification in the Arctic. Sci. Rep. 8, 1178. doi: 10.1038/s41598-017-19118-8
Walkusz W., Kwasniewski S., Falk-Petersen S., Hop H., Tverberg V., Wieczorek P., et al. (2009). Seasonal and spatial changes in the zooplankton community of Kongsfjorden, Svalbard. Polar Res. 28, 254–281. doi: 10.1111/j.1751-8369.2009.00107.x
Walkusz W., Williams W. J., Kwasniewski S. (2013). Vertical distribution of mesozooplankton in the coastal Canadian Beaufort Sea in summer. J. Mar. Syst. 127, 26–35. doi: 10.1016/j.jmarsys.2012.01.001
Wassmann P. (2011). Arctic Marine ecosystems in an era of rapid climate change. Prog. Oceanogr 90, 1–17. doi: 10.1016/j.pocean.2011.02.002
Weydmann A., Søreide J. E., Kwasniewski S., Leu E., Falk-Petersen S., Berge J. (2013). Ice-related seasonality in zooplankton community composition in a high Arctic fjord. J. Plankt Res. 35, 831–842. doi: 10.1093/plankt/fbt031
Yamaguchi A., Ashjian C. J., Campbell R. G. (2022). Comparison of population structure, vertical distribution and growth of sympatric, carnivorous, mesopelagic copepods, Paraeuchaeta glacialis and Heterorhabdus norvegicus, in the western Arctic ocean. J. Plankt Res. 44, 443–453. doi: 10.1093/plankt/fbac019
Keywords: Svalbard, seasonal migration, reproduction, Calanus glacialis, Pseudocalanus, Microcalanus, meroplankton
Citation: Søreide JE, Dmoch K, Blachowiak-Samolyk K, Trudnowska E and Daase M (2022) Seasonal mesozooplankton patterns and timing of life history events in high-arctic fjord environments. Front. Mar. Sci. 9:933461. doi: 10.3389/fmars.2022.933461
Received: 30 April 2022; Accepted: 29 June 2022;
Published: 11 August 2022.
Edited by:
Ulisses Miranda Azeiteiro, University of Aveiro, PortugalReviewed by:
Mie Hylstofte Sichlau Winding, Greenland Climate Research Centre, GreenlandDaria Martynova, Zoological Institute (RAS), Russia
Copyright © 2022 Søreide, Dmoch, Blachowiak-Samolyk, Trudnowska and Daase. This is an open-access article distributed under the terms of the Creative Commons Attribution License (CC BY). The use, distribution or reproduction in other forums is permitted, provided the original author(s) and the copyright owner(s) are credited and that the original publication in this journal is cited, in accordance with accepted academic practice. No use, distribution or reproduction is permitted which does not comply with these terms.
*Correspondence: Janne E. Søreide, amFubmVzQHVuaXMubm8=; Katarzyna Dmoch, a2FzaWFkbTFAZ21haWwuY29t