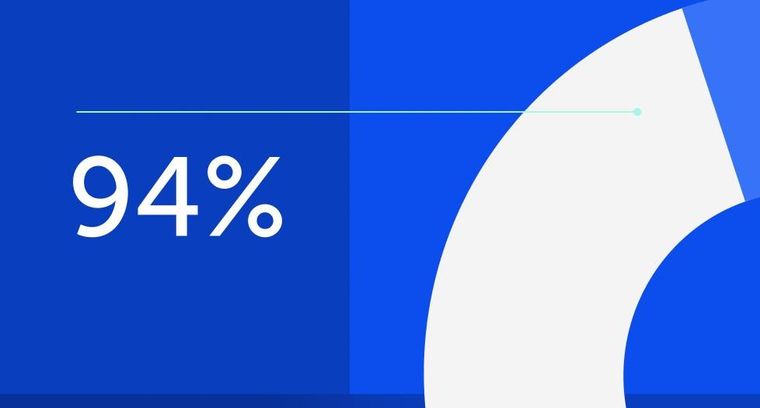
94% of researchers rate our articles as excellent or good
Learn more about the work of our research integrity team to safeguard the quality of each article we publish.
Find out more
ORIGINAL RESEARCH article
Front. Mar. Sci., 04 August 2022
Sec. Aquatic Physiology
Volume 9 - 2022 | https://doi.org/10.3389/fmars.2022.924018
This article is part of the Research TopicThe Relationship Between Nutrition and Stress on Intestinal Health and Reproduction in Aquatic AnimalsView all 8 articles
There is a trend towards using high-lipid diets in aquaculture, which can be optimized by using certain additives. This study investigated the effect of vitamin E (VE) supplementation of a high-lipid diet on the growth, serum and liver biochemical indexes, hepatic histology, and lipid metabolism of hybrid groupers (♀ Epinephelus fuscoguttatus × ♂ Epinephelus lanceolatus). Six groups of iso-protein (50.23%) and iso-lipidic high-lipid (15.36%) experimental diets were prepared by adding VE at concentrations of 49.6 (basic diet, controls), 100, 110, 163, 206, and 245 mg/kg. Each treatment consisted of three replicates and 30 fish (10.20 ± 0.02 g) in an 8-week feeding experiment. The results show that 1) compared with controls, growth performance was not affected by VE addition. However, the weight gain rate (WGR) and specific growth rate (SGR) were significantly lower in the high-VE group (245 mg/kg) than in the 163 and 206 mg/kg groups. 2) Compared with controls, VE significantly increased the contents of alpha-tocopherol in the liver and muscle. 3) Adding VE can reduce the fatty livers caused by high-lipid diets and significantly reduce total serum cholesterol, reduce the activity of lipid synthesis enzymes, and significantly increase the activity of lipolytic enzymes in the liver. 4) Compared with controls, appropriate amounts of VE significantly increased the expressions of the liver lipid-metabolism-related genes PPAR-α, PPAR-β, PPAR-γ, H-FABP , A-FABP, and L-FABP. Based on observations of lipid metabolism enzyme activity, histological sections, and lipid-metabolism-related gene expression, we conclude that the addition of 163–206 mg/kg VE to a high-lipid diet can promote lipid metabolism in groupers.
Lipids are essential nutrients for animal growth and play a vital role in the metabolism and immunity of organisms (Watanabe, 1982). Lipids also play an important role in aquafeeds by providing essential fatty acids and energy for the growth and health of aquatic animals (Lee et al., 2002). Providing the optimal level of lipids in an aquafeed can provide the necessary nutrients for optimal fish growth. When feed lipid levels are insufficient, the protein in the feed is consumed for energy supply, leading to a decrease in protein utilization. At the same time, a lack of lipid-soluble vitamins can lead to metabolic dysfunction, eventually reducing the growth and development of fish (Lin & Shiau, 2003). Higher lipid contents can serve to save protein and reduce production costs (Li et al., 2021). Therefore, high-lipid diets have become a trend in the aquafeed industry.
High-lipid diets have been widely used in many aquaculture animals to reduce feed protein being used as an energy source and to improve feed efficiency. However, high-lipid diets can also have a number of side effects, including fatty liver, inflammation, and dysbiosis of the intestinal flora (He et al., 2016; Zhou et al., 2018). High-lipid diets also produce lipid peroxidation in aquatic organisms and generate large amounts of reactive oxygen species. Therefore, the search for suitable additives that reduce the disadvantages of high-lipid diets is a focus of aquacultural research (Du et al., 2005; Li et al., 2012; Ni et al., 2016).
One lipid-soluble vitamin with strong antioxidant properties is VE, which can block free radical chain reactions and is an essential micronutrient for human and animal reproduction and growth. VE promotes growth, regulates metabolism, enhances immunity, reduces tissue lipid oxidation, and improves meat quality and flavor by inhibiting phospholipases contained in the muscles of freshwater prawns (Dan et al., 2018). Dietary VE can significantly reduce the degree of lipid peroxidation and improve the antioxidant capacity of juvenile turbot (Scophthalmus maximus; Wei et al., 2011). In addition, some studies have shown that VE can reduce lipid peroxidation reactions and protect biological membranes, lipoproteins, and lipids from the damage caused by oxygen-free radicals in turbot, grass carp (Ctenopharyngodon idella), halibut (Hippoglossus hippoglossus), and sea bream (Sparus aurata; Jia et al., 2017; Tocher et al., 2002). Furthermore, VE supplementation in fish diets can enhance immunity and adaptability to stress (Wang et al., 2019). In a recent study, the optimum amount of dietary VE for giant freshwater prawns ranged from 169.38 to 218.66 mg/kg (Dan et al., 2018). The dietary requirement for VE has been reported in many fishes, for example, 35 mg of α-tocopherol/kg diet and 60 mg/kg diet for Atlantic salmon (Lall et al., 1988; Hamre and Oyvind, 1995) and 104–115 mg/kg diet for groupers (Lin and Shiau, 2005).
Groupers are economically important farmed fish in coastal areas of China (Yan et al., 2020). The hybrid grouper (♀ Epinephelus fuscoguttatus × ♂ E. lanceolatus) is a carnivorous marine fish and also known as the gentian tiger spot or pearl spot. It has tender flesh, rapid growth, and strong disease resistance (Yan et al., 2022; Pan et al., 2022), with the appearance of tiger spot head and gentian tail, and can also be used as an ornamental fish; hence, it has broad market prospects (Liu et al., 2021; Lu et al., 2022). Many investigations have suggested that groupers’ protein and lipid requirements are in the ranges of 45%–50% and 8%–16%, respectively (Tuan and Williams, 2007). At present, there are many studies on the nutrition of juvenile groupers (Tang et al., 2018; Long et al., 2021); however, research on the relationship between high-lipid diets with added VE and lipid metabolism is limited. For these reasons, the present study aimed to investigate whether adding VE to high-lipid diets can mitigate the negative effects of such diets and enhance growth performance, improve body lipid metabolism, reduce liver lipid deposition, and affect the expression of genes related to lipid metabolism.
The gross and nutritional compositions of the experimental diets are shown in Table 1. Six iso-nitrogenous and iso-lipidic diets with different VE levels (49.6, 100, 110, 163, 206, and 245 mg/kg) were formulated, named V1 (control), V2, V3, V4, V5, and V6, respectively. All ingredients were crushed and passed through a 60-mesh sieve, then thoroughly mixed using the progressive enlargement method described by Long et al. (2022). After homogenization of the feed, it was mixed with distilled water until a wet dough was obtained that did not fall apart when held firmly in the hands. The diets were processed into 2.5-mm-diameter pellets by a twin-screw extruder (F-26, South China University of Technology, Guangdong Province, China), air dried at room temperature, then ground and sieved to an appropriate size and stored in bags at –20°C until use (Wei et al., 2021).
The experimental fish were purchased from Hong-Yun farm in Dongjian town (Zhanjiang, China) and transported to the Marine Biological Research Base of Guangdong Ocean University (Zhanjiang, China). The fish were temporarily reared in a cement pond of dimensions 5 m (length) × 4 m (width) × 1.8 m (depth) and fed a commercial diet (Haida Aquatic Diet Co. Ltd., Zhanjiang, China) for 2 weeks until they reached the size required for the experiment (10.20 ± 0.02 g). Some 540 fish were randomly allocated to 18 fiberglass tanks (500 L), each provided with one piece of polyvinylchloride (PVC) pipe of 20.0 cm (diameter) × 30.0 cm (length) as a shelter for the fish. Each feed was given to fish in three tanks twice a day (8:00 and 16:00) until apparent satiation was observed. The daily food intake was recorded for 8 weeks. About 70% of the water was changed every day to maintain water quality. The water conditions were temperature = 29–32°C, salinity = 28, dissolved oxygen >7 mg/L, and nitrates <0.05 mg/L, which were tested by a multi-parameter water quality detector (PTF-001B, WBD Biotechnology Co., Ltd., China).
At the end of the trial, the fish were fasted for 24 h. Fish were anesthetized with eugenol (1:10,000), after then all fish in each tank were weighed and counted to calculate weight gain rate (WGR), specific growth rate (SGR), feed intake (FI), and feed conversion ratio (FCR). Three fish from each tank were randomly selected to measure the corresponding body length, body weight, liver weight, and visceral mass weight to calculate condition factor (CF), hepatosomatic index (HSI), and visceral somatic index (VSI), and an additional three fish from each tank were stored at −20°C for routine composition analysis. Blood samples from four fish per tank were collected and centrifuged at 4°C (3,000 rpm for 10 min) to collect the serum, which was subsequently aliquoted and stored at −80°C for later determination of biochemical indexes and enzyme activity analysis. The same fish from which the blood samples were collected were dissected and their livers removed. Two of these livers were then used for enzyme activity analysis, and two were used for histological analysis. Liver samples used for histological examination were kept in 4% formalin solution, and the other samples were quickly placed into liquid nitrogen and later stored at −80°C for subsequent analysis.
The calculation formulas of growth parameters (NRC, 2011) and morphological indices were as follows:
Weight gain rate (WGR, %) = 100 × (final weight − initial weight)/(initial weight);
Specific growth rate (SGR, %/day) = 100 × [In (final weight) – In (initial weight)]/days of experiment;
Feed conversion ratio (FCR) = total dry feed intake/total weight gain;
Condition factors (CF, g/cm3) = 100 × (final body weight)/(body length)3;
Visceral somatic index (VSI, %) = 100 × (viscera weight)/(body weight); Hepatosomatic index (HSI, %) =100 × (liver weight)/(body weight).
The diets and whole fish were analyzed according to the official method of the Association of Official Analytical Chemists. The moisture content was measured by drying at 105°C to a constant weight, and ash content was determined by combustion at 550°C for 6 h. The crude protein content was determined by Kjeldahl method. The crude lipid content was determined by Soxhlet extraction method.
The level of triglycerides (TGs), high-density lipoprotein cholesterol (HDL), low-density lipoprotein cholesterol (LDL), free fatty acid (FFA), very low-density lipoprotein (VLDL), and total cholesterol (TC) in the serum were determined by ELISA kits (Shanghai Enzyme-Linked Biotechnology Co., Ltd., Shanghai, China), and the measurement wavelengths required for the above biochemical enzyme activity indexes were 505, 500, 546, 550, 450, and 510 nm, following a previously described method of Liu et al. (2020).
Total protein (TP), fatty acid synthase (FAS), hepatic lipase (HL), lipoprotein lipase (LPL), hormone-sensitive lipase (HSL), and triglyceride (TG) activities in the liver were analyzed by ELISA kits (Shanghai Enzyme-Linked Biotechnology Co., Ltd., Shanghai, China), and the measurement wavelengths required for the above biochemical enzyme activity indexes were 562, 340, 550, 550, 450, and 510 nm, following a previously described method of Yin et al. (2018).
The livers were set at 4°C of 4% paraformaldehyde (PFA) and then washed twice with 0.2 M phosphate buffer for 30 min. The tissues were prepared sequentially with 0.2 M phosphate buffer solutions of 10%, 20%, and 30% sucrose of each for 30 min and frozen sectioned. The sections were briefly washed in sterile water, dipped in 60% isopropanol for 1 min, and stained with Oil Red O solution for 15 min. Then, the sections were stained with hematoxylin. Finally, the sections were observed under a microscope camera (Long et al., 2021).
Transzol UP (TransGen Biotech, Beijing, China) of 1 ml was added to the samples, and the total RNA was extracted according to the manufacture’s protocol. The quantity and quality of isolated RNA were detected at 260 and 280 nm using a NanoDrop 2000 spectrophotometer (Gene Company Limited, Guangzhou, China) and by electrophoresis in 1% agarose gel, respectively. The first-strand cDNA was synthesized using PrimeScript™ RTreagent Kits with cDNA Eraser (Takara, Japan) according to the manufacturer’s introduction. The cDNA was stored at −20°C for real-time quantitative polymerase chain reaction (RT-qPCR). RT-qPCR was performed in a 384-well plate with a 10-µl reaction volume containing 5 µl of SYBR® Green Real-Time PCR Master Mix, 0.8 µl of each primer, 1 µl of cDNA sample, and 3.2 µl of RNase Free dH2O. The PCR conditions were set using a thermal programmer at 95°C for 30 s, 40 cycles of 95°C for 5 s and 60°C for 34 s. Each sample was tested in triplicate. Primers of the reference gene (β-actin) and target gene were designed according to published sequences of groupers (Table 2). Threshold cycle (Ct) values were collected from each sample after finishing the process. The relative expression levels were calculated using the 2−ΔΔ Ct method.
All data were first examined for homogeneity of variance using SPSS version 20.0 (SPSS Inc., USA). The results were subjected to one-way analysis of variance followed by Tukey to test significant differences among treatment groups, and probability values of p < 0.05 were deemed to be statistically significant. The results are presented as means ± standard error (SEM).
The growth and morphological results are shown in Table 3. Compared to the control group, the treatment diets had no significant effects on FI, FCR, HIS, or VSI (p > 0.05). The CF was significantly lower in groups V2 and V5 compared to controls (p < 0.05). Compared to controls, there were no significant differences in WGR or SGR (p > 0.05). However, group V3 had significantly higher WGR and SGR than group V6. (p < 0.05).
The whole-body compositions are presented in Table 4. It can be seen that dietary VE level had no significant effect on moisture, crude protein, or ash content (p > 0.05). Compared to controls, the crude lipid content was significantly higher in group V5 (p < 0.05).
According to Table 5, VE addition significantly affected VE deposition in the liver and muscle. In the liver, VE deposition was significantly higher in groups V3–V6 than in controls and group V2 (p < 0.05). In the muscle, VE deposition in groups V3–V5 was significantly higher than in other groups (p < 0.05). From the Oil Red O staining of the liver (Figure 1), we can see that lipid deposition decreased with increases in dietary VE. There were few lipid droplets in the livers of groups V4, V5, and V6 fish, while many lipid droplets appeared in the livers of fish in other groups. The number of lipid droplets had a positive relationship with dietary VE level.
The serum biochemical indices are shown in Table 6. The serum HDL content increased first and then decreased, and its content in VE-treated groups, except group V2, was significantly higher than in controls. The maximum HDL appeared in group V5 (p < 0.05). The serum LDL content decreased gradually and was significantly lower in groups V5 and V6 than in controls and group V2 (p < 0.05). The VLDL contents were significantly lower in groups V4, V5, and V6 than in controls and groups V2 and V3 (p < 0.05). Compared with controls, the FFA values of groups V3 and V6 were significantly higher (p < 0.05). The TC values of groups V1 and V2 were significantly higher than in other groups, with the maximum value appearing in group V1 (p < 0.05). However, the serum TG content was not significantly affected by VE (p > 0.05).
The lipid metabolism enzyme activities in the liver are shown in Table 7. It was observed that the hepatic TP content was highest in group V2, but there were no significant differences among groups (p > 0.05). The FAS contents were significantly lower in groups V3–V6 than in controls and V2, and there was a downward trend in FAS (p < 0.05). Hepatic HL showed a trend of increasing first and then decreasing. The HL contents of groups V3 and V4 were significantly higher than in controls and V2 (p < 0.05). Except for group V2, the hepatic LDL contents of VE-treated groups were significantly higher than in controls (p < 0.05). The trend in liver HSL content was similar to that of HL, and compared with controls, the hepatic HSL levels were significantly higher in VE-treated groups except V6 (p < 0.05), with the maximum obtained in V4. Compared with controls, low levels of VE (100–300 mg/kg) did not affect the liver TG content, while high levels (400–500 mg/kg) significantly reduced it (p < 0.05).
The expression of genes related to lipid metabolism is shown in Figure 2. The PPAR-α mRNA expression levels of groups V2 and V4 and the PPAR-β mRNA expression levels of group V4 were significantly upregulated compared with other groups (p < 0.05). Compared with controls, the high level of VE (300–500 mg/kg) supplement significantly upregulated PPAR-γ mRNA expression levels (p < 0.05). The relative expression level of L-FABP mRNA basically increased first and then decreased. Compared with the control group, the relative expression level of L-FABP mRNA in groups V2 and V5 was significantly lower (p < 0.05). On the contrary, the L-FABP mRNA expression level was significantly upregulated in group V4 (p < 0.05). Compared with other groups, the H-FABP mRNA expression level was significantly upregulated in group V4 (p < 0.05). The A-FABP mRNA expression level of group V3 was significantly upregulated compared with controls and group V2 (p < 0.05) and was not significantly different to that of other groups (p > 0.05).
Figure 2 Hepatic relative expression of lipid-metabolism-related genes of hybrid grouper. Values are means ± SEM (n = 3). Different letters assigned to the bars represent significant differences (p < 0.05). PPAR-α, peroxisome-proliferator-activated receptorα; PPAR-β, peroxisome proliferator-activated receptorβ; PPAR-γ, peroxisome proliferator-activated receptorγ; L-FABP, liver-type fatty acid-binding protein; H-FABP, heart-type fatty acid-binding protein; A-FABP, adipocyte-type fatty acid-binding protein.
As an essential nutrient, lipids provide energy and essential fatty acids for fish growth and development. An appropriate dietary lipid level can promote fish growth and reduce FCR (Xiao et al., 2013). Appropriately increasing the lipid level of feed can save feed protein to a certain extent, but fish growth will be inhibited if it is too high (Wang et al., 2005). Fortunately, these negative effects can be alleviated by nutritional regulation. Vitamin E is a lipid-soluble vitamin involved in antioxidation and in enhancing immunity and stress resistance. It is an essential nutrient for the growth of aquatic animals and has a catalytic effect on fish growth at an appropriate level (Montero et al., 2001). However, fish cannot synthesize VE and can only get it from the diet (Yi et al., 2018). In the present study, fish growth performance was improved by supplying the proper amount of VE in a high-lipid diet, but there was no significant difference between VE-treated groups and controls. This is a little different from the results of previous studies. It has been reported that VE supplementation had no significant effect on the growth of red drum (Sciaenops ocellatus) or turbot (Scophthalmus maximus) (Peng and Gatlin, 2009; Wei et al., 2011). In addition, compared with groups V3 and V4, WGR and SGR were significantly lower in group V6, which suggests that higher dietary VE levels may inhibit growth in groupers. It has been reported that a high VE level has the tendency to reduce the growth performance of grass shrimp (Penaeus monodon) and turbot (Lee and Shiau, 2004; Niu et al., 2014a). Thus, the effects of VE differ according to species, which may be due to species specificity, different stages of development, different forms of VE addition, and the amount and type of dietary lipids used in the feed. Meanwhile, FCR and FI did not differ between groups, confirming many previous studies (Wei et al., 2011; Wang et al., 2019), nor did VSI or HIS differ between treatments, which is consistent with many previous studies (Zhong et al., 2008; Zhang et al., 2016). This demonstrates that the addition of VE to a high-lipid diet does not affect the morphological indexes of groupers.
Body composition, especially lipid content, was significantly affected by dietary VE level (p < 0.05) and had no significant effect on crude protein or moisture (p > 0.05), which is consistent with previous studies (Gao et al., 2012; Zhang et al., 2016). In the present study, the crude lipid content differed according to VE dosage, which might be related to the preferential metabolism of certain nutrients by groupers. Fish in different states do selectively consume sugars, lipids, and proteins, and when they preferentially utilize sugar or protein as their primary function, it leads to lipid accumulation (Dong et al., 2015).
Alpha-tocopherol is the most bioactive form of vitamin E. Different tissues do not have the same capacity to store VE, with the liver and muscle being the main tissues of VE deposition. It has also been shown that VE intake and tissue concentrations of alpha-tocopherol are highly correlated (Stephan et al., 1995) . In the present study, compared with controls, dietary VE supplement significantly increased the content of α-tocopherol in the muscle and liver of groups V3–V6, which is consistent with other studies (Zhang et al., 2012; Liu et al., 2018). The alpha-tocopherol content in the liver was higher than that in the muscle, suggesting that the liver is an important site for tlohe regulation of alpha-tocopherol levels in the body. Many studies have also confirmed that the main site of VE metabolism is the liver, which is considered to be a VE storage tissue in animals (Brigelius-Flohé, 2009). The mechanism of VE metabolism in liver tissue is as follows: free-form alpha-tocopherol is sent to the liver and bound to LDL and HDL, which are captured by low-density lipoprotein receptors (LDLRs) and scavenger receptors (SR-BIs), respectively, then sent to lysosomes by cytokinesis, where it is released and becomes free again. The free alpha-tocopherol receptor protein is transported outside the lysosome via other routes to the cell membrane, where it binds to the ABCA1 transporter protein and is transported outside of the cell. Some of the free alpha-tocopherol conjugates enter the circulatory system and are broken down into LDL and HDL, which is the main pathway for the hepatic metabolism of VE in fish. High-fat diets can induce oxidative stress in the body, leading to abnormal liver function. The radical cause of fatty liver is also oxidative stress, as the liver is the center of lipid metabolism. Combined with Figure 1, it can be seen that the numbers of lipid droplets in the livers of fish in groups V1, V2, and V3 were greater than in groups V4, V5, and V6. This is due to increased deposition of VE in the liver and its function of promotion to lipid antioxidant and lipid metabolism (Williams et al., 1992).
Lipids are essential nutrients for fish growth, providing energy and fatty acids. The main pathways of lipid metabolism usually include digestion and absorption, lipid transport, lipid production, and β-oxidation (NRC, 2011). It is necessary to investigate serum lipid metabolism indicators in order to judge the relationship between VE supplementation and the lipid metabolism ability of fish fed a high-lipid diet. Serum TG, TC, HDL, LDL, and VLDL contents are important indicators of normal animal lipid metabolism. Changes in their levels can reflect the metabolic and physiological status of an animal (NRC, 2011; Liu et al., 2018). In the present study, serum TC levels were significantly reduced, which is consistent with previous studies showing that VE can lower serum cholesterol levels and slow the onset of atherosclerosis by regulating lipid metabolism (Yuan, 2009; Yang, 2012). It has been demonstrated that HDL and VLDL are related to the metabolic transport capacity of lipids (Ma et al., 2016). In our experiment, there was a positive relationship between the serum HDL content of groupers and dietary VE addition up to a certain level, while the opposite was true for LDL and VLDL. The reason for this may be that the stress of a high-lipid diet leads to lipid peroxidation and the production of peroxides, while the addition of dietary VE causes serum VE levels to rise; thus, free radicals are eliminated, lipid peroxidation is inhibited, and serum lipid levels are improved. This indicates that VE addition is beneficial for lipid metabolism in the serum of groupers. Similar results have been reported in other aquatic animals; for example, the addition of 124–243 mg/kg VE to a high-lipid diet improved the lipid metabolism of turbot (Niu et al., 2014b).
VE not only affects the serum biochemical parameters of groupers but also significantly affects liver lipase activity. The liver is the main organ of lipid metabolism (Li et al., 2016). Lipases mainly include LPL and HL, collectively known as total lipases. LPL catalyses VLDL and TG carried by celiac particles and is hydrolyzed to glycerol and FAs for tissue storage and utilization (Huang et al., 2018). HL has multiple lipase activities, acts as a ligand to facilitate the entry of LDL and celiac particles into hepatocytes, and is directly involved in the reversal and breakdown of HDL cholesterol (Cohen et al., 1999). It is also one of the enzymes associated with endogenous TG metabolism in the circulation and has a similar function to LPL. HL activity affects HDL cholesterol and produces small, dense LDL cholesterol, which implies a role in the formation of atherosclerosis (Schiekofer et al., 2017). HSL is the key neutral lipase, mainly responsible for the hydrolysis of triacylglycerol (TAG), diglycerides, and monoacylglycerols to free fatty acids (Huang et al., 2018). FAS, consisting of two identical subunits (260–270 kDa), is a key lipid synthase involved in the ab initio synthesis of long-chain fatty acids in the cytoplasm and catalyzes the condensation of acetyl coenzyme, malonyl coenzyme, and NADPH (Liu et al., 2010). It can also influence the rate of fatty acid synthesis and affect lipid deposition (Yue et al., 2012). The results of this study show that the addition of VE to the diet can increase the activity of LPL, HSL, and HL and decrease the activity of FAS. VE accelerates lipid metabolism and maintains lipid homeostasis by increasing the activity of lipolytic enzymes and decreasing the activity of FAS lipid synthesis enzymes. In other words, VE maintains lipid metabolism by influencing the rate of lipolysis and synthesis.
Peroxisome-proliferator-activated receptors (PPARs) are a new class of ligand-activated intranuclear receptor transcription factors that are members of the type-II nuclear hormone receptor superfamily (Liu et al., 2013; Fang et al., 2016). There are three isoforms of PPARs, namely, PPAR-α, PPAR-β, and PPAR-γ, each encoded by a different gene and performing a specific function (Abbott, 2009) . PPAR-α is related to lipid metabolism and regulates the β-oxidation of peroxisomes (Fang et al., 2015); PPAR-β is less reported and is known to be widely distributed; and PPAR-γ is mainly related to adipocyte differentiation and lipid metabolism. FABPs are members of a multigene family of intracellular lipid-binding proteins. All members of the FABP family share common features in the three-dimensional structure of proteins, namely, two short α-helices near the N-terminus of the protein molecule, immediately followed by 10 inversely parallel β-chains connected by hydrogen bonds, which then assemble into two almost orthogonal β-folds (Zhang et al., 2011; Yang et al., 2021). Studies have shown that FABPs play an important role in the intracellular uptake, transport, and metabolic regulation of long-chain fatty acids, allocating them to different metabolic pathways and preventing intracellular lipid accumulation (Ding et al., 2017; Li et al., 2020). However, there are few studies on the effects of VE on lipid metabolism and PPAR or FABP family genes in fish. As VE is a fat-soluble vitamin with antioxidant properties and the ability to inhibit lipid peroxidation, greater attention has been paid to the FABP and PPAR gene families related to lipid metabolism.
The PPAR family contains important transcription factors that regulate metabolic homeostasis and lipid and energy metabolism (Gross et al., 2017). The H-FABP gene is closely related to the development and function of adipose tissue and is considered to be a candidate gene for lipid metabolism, which can affect lipid deposition (Tyra et al., 2011; Liu et al., 2018). The expression of L-FABP receives many factors and mainly promotes FA uptake, diffusion, and metabolism (Her et al., 2003). In the present study, the expressions of PPARs in different groups were affected; similarly, the expressions of FABPs were also different. It is worth mentioning that the expressions of the PPARα and PPARβ genes were highest in group V4; also, the L-FABP and H-FABP genes achieved the highest expression in group V4. This is consistent with Li (2008) study, where the expressions of PPAR-β and H-FABP genes in broiler breast muscle were significantly increased at week 17 in a feeding experiment with different levels of dietary VE. Several previous studies have also confirmed that high levels of VE can significantly increase H-FABP and L-FABP gene expressions. The results of this experiment are generally consistent with these findings. A possible explanation is that VE does not directly regulate lipid metabolism and acts on lipid metabolic processes by influencing the PPAR-FABP signaling pathway and stimulating upregulation of expression. Another explanation is that VE can directly influence the expression of FABP-gene mRNA and, thus, affect body lipid metabolism. This has been confirmed by studies that found a positive correlation between FABP-gene mRNA expression and certain levels of dietary VE (Zhang, 2011; Ma et al., 2012; Liu et al., 2018).
In conclusion, adding an appropriate amount of VE to a high-lipid diet promotes lipid metabolism, inhibits lipid peroxidation, and reduces lipid deposition in groupers. High doses of VE also increase the deposition of α-tocopherol in the muscle and liver, with the liver being the main site of α-tocopherol metabolism. VE also affects the expression levels of PPARs and FABPs. We hypothesized that VE may affect lipid metabolism in groupers by activating the PPAR-FABP signaling pathway or by directly affecting FABP expression. Based on the results of the current study, we recommend adding an additional dose of 163–206 mg/kg VE under high-lipid conditions. This research provides a reference for improving the utilization efficiency of high-lipid diets by groupers, thereby improving the efficiency of aquaculture.
The original contributions presented in the study are included in the article/supplementary material. Further inquiries can be directed to the corresponding author.
The animal study was reviewed and approved by the Animal Ethics Committee of Guangdong Ocean University.
Designed study: XY, XD, and BT. Experimental operation: XY, WH, HL, XS, SP, TL, and YY. Sampling and data analysis: WH, XY, SP, TL, XS, and YY. Drafted paper: WH. Paper revision: XD and XY. All authors contributed to the article and approved the submitted version.
This study was supported financially by the Research and Demonstration of Precision Functional Compound Feed Technology of Major Cultured Fishes and Shrimps in South China (2021B0202050002), the General Program of Natural Science Foundation of Guangdong Province (2021A1515011165), the Department of Education of Guangdong Province (2021ZDZX4005), the National Natural Science Foundation of China (31972808), the Postgraduate Education Innovation Project of Guangdong Ocean University (202139), the Science and Technology Bureau of Zhanjiang (2020A05003), and the China Agriculture Research System of MOF and MARA (CARS-47).
The authors declare that the research was conducted in the absence of any commercial or financial relationships that could be construed as a potential conflict of interest.
All claims expressed in this article are solely those of the authors and do not necessarily represent those of their affiliated organizations, or those of the publisher, the editors and the reviewers. Any product that may be evaluated in this article, or claim that may be made by its manufacturer, is not guaranteed or endorsed by the publisher.
Abbott B. D. (2009). Review of the expression of peroxisome proliferator-activated receptors alpha (PPARα), beta (PPARβ), and gamma (PPARγ) in rodent and human development. Reprod. Toxicol. 27, 246–257. doi: 10.1016/j.reprotox.2008.10.001
Brigelius-Flohé R. (2009). Vitamin E: The shrew waiting to be tamed. Free Radical Biol. Med. 46, 543–554. doi: 10.1016/j.freeradbiomed.2008.12.007
Cohen J. C., Vega G. L., Grundy S. M. (1999). Hepatic lipase: New insights from genetic and metabolic studies. Curr. Opin. Lipidol 10, 259–267. doi: 10.1097/00041433-199906000-00008
Dan F., Liu B., Sun C., Zhou Q., Song C., Zhang H., et al. (2018). Effects of dietary vitamin e content on growth performance, serum biochemical indices and muscle quality of giant freshwater prawn (Macrobrachium rosenbergii). Chin. J. Anim. Nutr. 31, 1–12. doi: 10.3969/j.issn.1006-267x.2019.01.027
Ding L., Jin M., Sun P., Lu Y., Ma H., Yuan Y., et al. (2017). Cloning, tissue expression of the fatty acid-binding protein (Pt-FABP1) gene, and effects of dietary phospholipid levels on fabp and vitellogenin gene expression in the female swimming crab Portunus trituberculatus. Aquaculture 474, 57–65. doi: 10.1016/j.aquaculture.2017.03.029
Dong X., Yang J., Tan B., Yang Q., Chi S., Liu H., et al. (2015). Dietary lipid requirements of orange-spotted grouper (Epinephelus coioides) in juvenile and grow out stages. Chin. J. Anim. Nutr. 27, 133–146. doi: 10.3969/j.issn.1006-267x
Du Z., Liu Y., Tian L., Wang J., Wang Y., Liang G. (2005). Effect of dietary lipid level on growth, feed utilization and body composition by juvenile grass carp (Ctenopharyngodon idella). Aquacult Nutr. 11, 139–146. doi: 10.1111/j.1365-2095.2004.00333.x
Fang L. L., Chen G., Wang Z. L., Tang B. G., Zhang J. D., Huang J. S., et al. (2015). Molecular cloning and expression distribution and bioinformatics analysis of peroxisome proliferator activated receptors-α in Trachinotus ovatus. J. Guangdong Ocean Univ. 35, 1–9. doi: 10.3969/j.issn.1673-9159.2015.04.001
Fang L. L., Chen G., Wang Z. L., Tang B. G., Zhang J. D., Huang J. S., et al. (2016). Effects of fenofibrate on relatively expression of gene pparα and cpti. J. Guangdong Ocean Univ. 36, 13–18. doi: 10.3969/j.issn.1673-9159.2016.01.003
Gao J., Koshio S., Ishikawa M., Yokoyama S., Mamauag R., Han Y. (2012). Effects of dietary oxidized fish oil with vitamin e supplementation on growth performance and reduction of lipid peroxidation in tissues and blood of red sea bream Pagrus major. Aquaculture 356, 73–79. doi: 10.1016/j.aquaculture.2012.05.034
Gross B., Pawlak M., Lefebvre P., Staels B. (2017). PPARs in obesity-induced t2dm, dyslipidaemia and nafld. Nat. Rev. Endocrinol. 13, 36–49. doi: 10.1038/nrendo.2016.135
Hamre K., Oyvind L. (1995). α-tocopherol levels in different organs of atlantic salmon (Salmo salar l.)–effect of smoltification, dietary levels of n-3 polyunsaturated fatty acids and vitamin e. Comp. Biochem. Physiol. 111, 547–554. doi: 10.1016/0300-9629(95)00065-f
Her G. M., Chiang C. C., Chen W. Y., Wu J. L. (2003). In vivo studies of liver-type fatty acid binding protein (l-fabp) gene expression in liver of transgenic zebrafish (Danio rerio). FEBS Lett. 538, 125–133. doi: 10.1016/S0014-5793(03)00157-1
He Z., Wang J., Wu Y., Li C., Li S., Liu W. (2016). Effect of dietary lipid levels on serum biochemical indices, immune responses and antioxidant capability of juvenile furong crucian carp (Furong carp ♀ × Red crucian carp ♂). Acta Hydrobiologica Sin. 40, 655–662. doi: 10.7541/2016.88
Hirwitz W., Latimer G. (1995). Official methods of analysis of aoac international (16th edn). Trends Food Sci. Technol. 6, 382. doi: 10.1016/0924-2244(95)90022-5
Huang H., Zhang Y., Cao M., Xue L., Shen W. (2018). Effects of fasting on the activities and mrna expression levels of lipoprotein lipase (lpl), hormone se nsitive lipase (hsl) and fatty acid synthetase (fas) in spotted seabass (Lateolabrax maculatus). Fish Physiol. Biochem. 44, 387–400. doi: 10.1007/s10695-017-0442-4
Jia Y., Jing Q., Niu H., Huang B. (2017). Ameliorative effect of vitamin e on hepatic oxidative stress and hypoimmunity induced by high-fat diet in turbot (Scophthalmus maximus). Fish Shellfish Immunol. 67, 634–642. doi: 10.1016/j.fsi.2017.06.056
Lall S. P., Olivier G., Hines J. A., Ferguson H. W. (1988). The role of vitamin e in nutrition and immune response of Atlantic salmon (Salmo salar). Aquacult Assoc. Can. Bull. 88, 76–78.
Lee S. M., Jeon I. G., Lee J. Y. (2002). Effects of digestible protein and lipid levels in practical diets on growth, protein utilization and body composition of juvenile rockfish (Sebastes schlegeli). Aquaculture 211, 227–239. doi: 10.1016/S0044-8486(01)00880-8
Lee M. H., Shiau S. Y. (2004). Vitamin e requirements of juvenile grass shrimp, Penaeus monodon, and effects on non-specific immune responses. Fish Shellfish Immunol. 16 (4), 475–485. doi: 10.1016/j.fsi.2003.08.005
Li W. J. (2008). Filtration of functional genes related to lipid metabolism of meat quality and nutritional regulation in chickens (Beijing: Chinese Academy of Agricultural sciences).
Liang D., Yang Q., Tan B., Dong X., Chi S., Liu H., et al. (2020). Dietary vitamin a deficiency reduces growth performance, immune function of intestine, and alters tight junction proteins of intestine for juvenile hybrid grouper (Epinephelus fuscoguttatus ♀ × E. lanceolatus ♂). Fish Shellfish Immunol. 107, 346–356. doi: 10.1016/j.fsi.2020.10.016
Li B., Hao J., Zeng J., Sauter E. R. (2020). Snapshot: fabp functions. Cell 182, 1066. doi: 10.1016/j.cell.2020.07.027
Li X., Jiang Y., Liu W., Ge X. (2012). Protein-sparing effect of dietary lipid in practical diets for blunt snout bream (Megalobrama amblycephala) fingerlings: Effects on digestive and metabolic responses. Fish Physiol. Biochem. 38, 529–541. doi: 10.1007/s10695-011-9533-9
Li G., Li Y., Wang Q., Zhang X., Lin B., Chen L., et al. (2021). Effects of digestible crude protein and lipid levels on gowth prformance, mtabolism of eergy sbstances and atioxidant cpacity of jvenile gifi tilapia (Ohromis niloticus). Chin. Agric. Sci. Bull. 37, 126–135. doi: casb2020-0335
Lin Y. H., Shiau S. Y. (2003). Dietary lipid requirement of grouper (Epinephelus malabaricus), and effects on immune responses. Aquaculture 225, 243–250. doi: 10.1016/S0044-8486(03)00293-X
Lin Y. H., Shiau S. Y. (2005). Dietary selenium requirements of juvenile grouper, Epinephelus malabaricus. Aquaculture 250, 356–363. doi: 10.1016/j.aquaculture.2005.03.022
Liu M. Y., Bu Z., Liu W. T., Gan S. H., Mu S. L., Su L. H., et al. (2018). Effects of vitamin e on immune function, α-tocopherol deposition and gene expressions of lipoprotein lipase and fatty acid-binding proteins in tissues of sanhuang chicken. Chinses J. Anim. Nutr. 30, 368–374. doi: 10.3176/chem.geol.1975.2.10
Liu H., Dong X., Tan B., Du T., Zhang S., Chi S., et al. (2020). Effects of fish meal replacement by low-gossypol cottonseed meal on growth performance, digestive enzyme activity, intestine histology and inflammatory gene expression of silver sillago (Sillago sihama forsskál) (1775). Aquacult Nutr. 26, 1724–1735. doi: 10.1111/anu.13123
Liu H., Li L., Stephen A., Ze T., Wei F., Tan B., et al. (2021). Effects of dietary yeast culture supplementation on growth, intestinal morphology, immunity, and disease resistance in Epinephelus fuscoguttatus ♀ × Epinephelus lanceolatu ♂. J. Guangdong Ocean Univ. 41, 1–11. doi: 10.3969/j.issn.1673-9159.2021.03.001
Liu Z., Li Y., Yan S., Shi B., Zhao Q., Zhang P. (2018). Effects of chitosan on serum lipid and adipocytokine contents of laying breeders. Chin. J. Anim. Nutr. 30, 2310–2317. doi: 10.3969/j.issn.1006-267x.2018.06.035
Liu H., Su L., Zhao Y., Wang W. (2013). Advance in the clone and expression of pparα and pparγ. Chin. Agric. Sci. Bull. 29, 31–36. doi: 10.3969/j.issn.1000-6850.2013.14.007
Liu Y., Tian W. X., Ma X. F., Ding W. J. (2010). Evaluation of inhibition of fatty acid synthase by ursolic acid: Positive cooperation mechanism. Biochem. Biophys. Res. Commun. 392, 386–390. doi: 10.1016/j.bbrc.2010.01.031
Li A., Yuan X., Liang X., Liu L., Li J., Li B., et al (2016). Adaptations of lipid metabolism and food intake in response to low and high fat diets in juvenile grass carp (Ctenopharyngodon idellus). Aquaculture 457, 43–49. doi: 10.1016/j.aquaculture.2016.01.014
Long S., Dong X., Tan B., Zhang S., Chi S., Liu H., et al. (2022). The antioxidant ability, histology, proximate, amino acid and fatty acid compositions, and transcriptome analysis of muscle in juvenile hybrid grouper (♀ Epinephelus fuscoguttatus × ♂ Epinephelus lanceolatus) fed with oxidized fish oil. Aquaculture 545, 737510. doi: 10.1016/j.aquaculture.2021.737510
Long S., Dong X., Tan B., Zhang S., Xie S., Liu H., et al. (2021). Growth performance, antioxidant ability, biochemical index in serum, liver histology and hepatic metabolomics analysis of juvenile hybrid grouper (♀ epinephelus fuscoguttatus × ♂ epinephelus lanceolatus) fed with oxidized fish oil. Aquaculture 545, 737261. doi: 10.1016/j.aquaculture.2021.737261
Lu Z., He H., Huang X., Huang W. (2022). Effects of hypoxic stress on antioxidant and energy metabolism of hybrid grouper (♀ Epinephelus fuscoguttatus × ♂ Epinephelus lanceolatus). J. Guangdong Ocean Univ. 42, 13–19. doi: 10.3969/j.issn.1673-9159.2022.01.003
Ma Q., Zhao Y., Bai M., Zhang X., Guo L., Zhang F., et al (2012). Influence of vitamin E supplement with different levels on liver L-FABP gene expression in mule ducks. Feed industry 21, 4. doi: CNKI:SUN:FEED.0.2012-21-010
Ma F., Li X., Li B., Leng X. (2016). Effects of extruded and pelleted diets with differing lipid levels on growth, nutrient retention and serum biochemical indices of tilapia (Oreochromis aureus × Tilapia nilotica). Aquacult Nutr. 22, 61–71. doi: 10.1111/anu.12229
Montero D., Tort L., Robaina L., Vergara J. M., Izquierdo M. S. (2001). Low vitamin e in diet reduces stress resistance of gilthead seabream (Sparus aurata) juveniles. Fish Shellfish Immunol. 11, 473–490. doi: 10.1006/fsim.2000.0324
Mosca A., Crudele A., Smeriglio A., Braghini M. R., Alisi A. (2020). Antioxidant activity of hydroxytyrosol and vitamin e reduces systemic inflammation in children with paediatric nafld. Digestive Liver Dis. 53, 1154–1158. doi: 10.1016/j.dld.2020.09.021
Ni P. J., Jiang W. D., Wu P., Liu Y., Kuang S. Y., Tang L., et al. (2016). Dietary low or excess levels of lipids reduced growth performance, and impaired immune function and structure of head kidney, spleen and skin in young grass carp (Ctenopharyngodon idella) under the infection of aeromonas hydrophila. Fish Shellfish Immunol. 55, 28–47. doi: 10.1016/j.fsi.2016.03.163
Niu H., Jia Y., Hu P., Meng Z., Lei J. (2014a). Effect of dietary vitamin e on the growth performance and nonspecific immunity in sub-adult turbot (Scophthalmus maximus). Fish shellfish Immunol. 41 (2), 501–506. doi: 10.1016/j.fsi.2014.09.033
Niu H., Lei J., Chang J., Zhou X., Jia Y., Gao C., et al. (2014b). Effect of a vitamin e supplemented high-fat diet on the growth, lipid metabolism and antioxidant function of turbot (Scophthalmus maximus). J. Fishery Sci. China 21, 291–299. doi: 10.3724/SP.J.1118.2014.00291
NRC (2011). Nutrient requirements of fish and shrimp (Washington, DC, USA: The National Academy Press).
Pan S., Yan X., Dong X., Li T., Suo X., Liu H., et al. (2022). Influence of dietary inositol supplementation on growth, liver histology, lipid metabolism, and related genes expression on juvenile hybrid grouper (♀ epinephelus fuscoguttatus × ♂ e. lanceolatu) fed high-lipid diets. Aquacult Nutr. 2022, 1–13. doi: 10.1155/2022/6743690
Peng L. I., Gatlin I. D. M. (2009). Dietary vitamin e requirement of the red drum. Sciaenops ocellatus Aquacult Nutr. 15 (3), 313–319. doi: 10.1111/j.1365-2095.2008.00596.x
Qian M., Zhao Y., Bo M., Zhang X., Guo L., Zhang F., et al. (2012). Influence of vitamin esupplementation with different levels on liverl-fabp gene expression in mule ducks. Feed Industry 33, 30–33. doi: 10.01-99lX(2012)2l-0030-04
Schiekofer S., Kleber M. E., Maerz W., Rasche F. M., Schneider J. G. (2017). The proline 7 substitution in the preproneuropeptide y is associated with higher hepatic lipase activity in vivo. Int. J. Endocrinol. 2017, 1–7. doi: 10.1155/2017/2869090
Shapawi R., Abdullah F. C., Senoo S., Mustafa S. (2019). Nutrition, growth and resilience of tiger grouper (Epinephelus fuscoguttatus) × giant grouper (Epinephelus lanceolatus) hybrid-a review. Rev. Aquacult 11, 1–12. doi: 10.1111/rap.12292
Stephan G., Guillaume J., Lamour F. (1995). Lipid peroxidation in turbot (Scophthalmus maximus) tissue: effect of dietary vitamin e and dietary n-6 or n-3 polyunsaturated fatty acids. Aquaculture 130, 251–568. doi: 10.1016/0044-8486(94)00322-F
Tang H., Zhang J., Chen G., Huang J., Wang Z., Tang B., et al. (2018). Combined effects of breeding density, feeding frequency and feeding level on specific growth rate, feed conversion rate and pepsin activity of juvenile hybrid groupers (Epinephelus fuscoguttatus ♀ × e. lanceolatus ♂). J. Guangdong Ocean Univ. 38, 22–31. doi: 10.3969/j.issn.1673-9159.2018.01.004
Tocher D. R., Mourente G., Eecken A. V.D., Evjemo J. O., Olsen Y. (2002). Effects of dietary vitamin E on antioxidant defence mechanisms of juvenile turbot (scophthalmus maximus l.), halibut (hippoglossus hippoglossus l.) and sea bream (sparus aurata l.). Aquaculture Nutrition. 8 (3), 195–207. doi: 10.1046/j.1365-2095.2002.00205.x
Tuan L. A., Williams K. C. (2007). Optimum dietary protein and lipid specifications for juvenile malabar grouper (Epinephelus malabaricus). Aquaculture 267, 129–138. doi: 10.1016/j.aquaculture.2007.03.007
Tyra M., Ropka-Molik K., Eckert R., Piórkowska K., Oczkowicz M. (2011). H-fabp and lepr gene expression profile in skeletal muscles and liver during ontogenesis in various breeds of pigs. Domest Anim. Endocrinol. 40, 147–154. doi: 10.1016/j.domaniend.2010.10.001
Wang J. T., Liu Y. J., Tian L. X., Mai K. S., Du Z. Y., Wang Y., et al. (2005). Effect of dietary lipid level on growth performance, lipid deposition, hepatic lipogenesis in juvenile cobia (rachycentron canadum). Aquaculture 249 (1-4), 439–447. doi: 10.1016/j.aquaculture.2005.04.038
Wang L., Ma B., Chen D., Lou B., Zhan W., Chen R., et al. (2019). Effect of dietary level of vitamin e on growth performance, antioxidant ability, and resistance to vibrio alginolyticus challenge in yellow drum Nibea albiflora. Aquaculture 507, 119–125. doi: 10.1016/j.aquaculture.2019.04.003
Watanabe T. (1982). Lipid nutrition in fish. Comp. Biochem. Physiol. Part B Comp. Biochem. 73, 3–15. doi: 10.1016/0305-0491(82)90196-1
Wei H., Li R., Yang Q., Tan B., Ray A., Dong X., et al. (2021). Effects of zn on growth performance, immune enzyme activities, resistance to disease and intestinal flora for juvenile pearl gentian grouper (Epinephelus lanceloatu ♂ × Epinephelus fuscoguttatus ♀) under low fishmeal diet. Aquacult Rep. 21, 100880. doi: 10.1016/j.aqrep.2021.100880
Wei Y., Wang X., Mai K., Ai Q. (2011). Effect of dietary vitamin e on growth, tissue lipid peroxidation,and tissue antioxidant capacity of juvenile turbot (Scophthalmus maximus). Periodical Ocean Univ. China 41, 45–50. doi: 10.16441/j.cnki.hdxb.2011.06.008
Williams D. E., Carpenter H. M., Buhler D. R., Kelly J. D., Dutchuk M. (1992). Alterations in lipid peroxidation, antioxidant enzymes, and carcinogen metabolism in liver microsomes of vitamin e-deficient trout and rat. Toxicol. Appl. Pharmacol. 116, 78–84. doi: 10.1016/0041-008X(92)90147-K
Xiao X., Zhou X., Jian C., Wang W., Zhou X. (2013). Effect of dietary lipid level on body index, lipid deposition and lipid metabolic enzyme activities of juvenile onychostoma sima. J. Fisheries China 37 (9), 1349. doi: 10.3724/SP.J.1231.2013.38644
Yan X., Dong X., Tan B., Zhang S., Chi S., Yang Q., et al. (2020). Effects of alternative dietary oils on lipid metabolism and related gene expression in hybrid grouper (♀ Epinephelus fuscoguttatus × ♂ E. lanceolatu). Aquacult Nutr. 27, 1–11. doi: 10.1111/anu.13168
Yang Q. (2012). Effect of vitamin e on performance, antioxidant, lipid metabolism and obr gene expression of breeding-hens (Hebei: Agricultural university of Hebei).
Yang X., Ma W., Yang J., Gou P., Bao Y., Wang X., et al. (2021). SNPs screening of B-FABP gene and their association with growth traits in (Takifugu rubripes). J. Guangdong Ocean Univ. 41, 28–34. doi: 10.3969/j.issn.1673-9159.2021.05.004
Yan X., Pan S., Dong X., Tan B., Huang W., Li T., et al. (2022). Vitamin e amelioration of oxidative stress and low immunity induced by high-lipid diets in hybrid grouper (♀ epinephelus fuscoguttatus× ♂ e. lanceolatu). Fish Shellfish Immunol. 124, 156–163. doi: 10.1016/j.fsi.2022.03.038
Yin B., Liu H., Tan B., Dong X., Chi S., Yang Q., et al. (2018). Cottonseed protein concentrate (CPC) suppresses immune function in different intestinal segments of hybrid grouper ♀ Epinephelus. fuscoguttatus × ♂ Epinephelus. lanceolatu via tlr-2/myd88 signaling pathways. Fish Shellfish Immunol. 81, 318–328. doi: 10.1016/j
Yi X., Shen H., Li J., Wei Z., Shentu J., Zhang W., et al. (2018). Effects of dietary vitamin e and astaxanthin on growth, skin colour and antioxidative capacity of large yellow croaker (Larimichthys crocea). Aquacult Nutr. 24, 472–480. doi: 10.1111/anu.12580
Yuan N. (2009). Effect of marigold extract and vitamin e on egg quality, antioxidant ability and immune response of laying hens (Hebei: Agricultural university of Hebei).
Yue Y., Liu G., Zheng A., Zhang H., Wang X., Li T., et al. (2012). Modulating the expression of genes regulating key enzymes for lipid metabolism in growing animals. Chin. J. Anim. Nutr. 24, 232–238. doi: 10.3969/j.issn.1006-267x.2012.02.007
Zhang X. (2011). Effects of different vitamin e supplements on fatty liver quality and expression of l-FABP gene in mule ducks (Jilin: Jilin University).
hang H., Huang R., Guo X., Yang I., Chen H. (2012). Effects of vitamin e on performance, egg quality, yolk and tissue α-tocopherol contents of breeding hens under cold stress. Chin. J. Anim. Nutr. 24, 2243–2248. doi: 10.3969/j.issn.1006-267x.2012.11.024
Zhang C., Huang F., Li J., Wang L., Song K., Mai K., et al. (2016). Interactive effects of dietary magnesium and vitamin e on growth performance, body composition, blood parameters and antioxidant status in japanese seabass (Lateolabrax japonicus) fed oxidized oil. Aquacult Nutr. 22, 708–722. doi: 10.1111/anu.12393
Zhang L., Zhao Z., Du B., Jiang S., Wu W. (2011). Genetic divergence analysis on ex-fabp gene and its associations with intamuscularfat in chicken. J. Guangdong Ocean Univ. 31, 75–79. doi: 10.3969/j.issn.1673-9159.2011.03.013
Zhong Y., Lall S. P., Shahidi F. (2008). Effects of dietary oxidized oil and vitamin e on the growth, blood parameters and body composition of juvenile atlantic cod gadus morhua (Linnaeus 1758). Aquacult Res. 39, 1647–1657. doi: 10.1111/j.1365-2109.2008.02038.x
Keywords: ♀ Epinephelus fuscoguttatus × ♂ E. lanceolatus, high-lipid diet, vitamin E, lipid metabolism, lipid deposition
Citation: Huang W, Yan X, Liu H, Tan B, Suo X, Pan S, Li T, Yang Y and Dong X (2022) Effects of vitamin E supplementation of a high-lipid diet on the growth and biochemical parameters of hybrid groupers (♀ Epinephelus fuscoguttatus × ♂ E. lanceolatus). Front. Mar. Sci. 9:924018. doi: 10.3389/fmars.2022.924018
Received: 20 April 2022; Accepted: 13 July 2022;
Published: 04 August 2022.
Edited by:
Jun Qiang, Freshwater Fisheries Research Center (CAFS), ChinaReviewed by:
Chang’an Wang, Heilongjiang River Fisheries Research Institute (CAFS), ChinaCopyright © 2022 Huang, Yan, Liu, Tan, Suo, Pan, Li, Yang and Dong. This is an open-access article distributed under the terms of the Creative Commons Attribution License (CC BY). The use, distribution or reproduction in other forums is permitted, provided the original author(s) and the copyright owner(s) are credited and that the original publication in this journal is cited, in accordance with accepted academic practice. No use, distribution or reproduction is permitted which does not comply with these terms.
*Correspondence: Xiaohui Dong, ZG9uZ3hpYW9odWkyMDAzQDE2My5jb20=
†These authors have contributed equally to this work and share first authorship
Disclaimer: All claims expressed in this article are solely those of the authors and do not necessarily represent those of their affiliated organizations, or those of the publisher, the editors and the reviewers. Any product that may be evaluated in this article or claim that may be made by its manufacturer is not guaranteed or endorsed by the publisher.
Research integrity at Frontiers
Learn more about the work of our research integrity team to safeguard the quality of each article we publish.