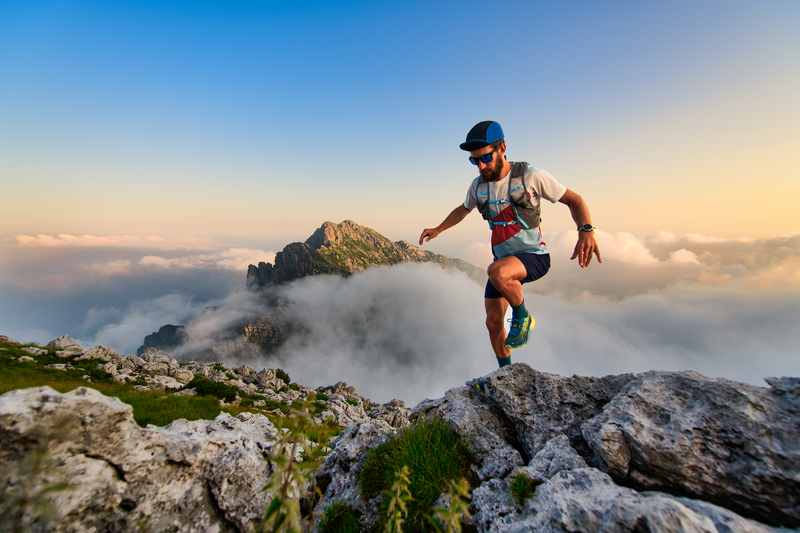
95% of researchers rate our articles as excellent or good
Learn more about the work of our research integrity team to safeguard the quality of each article we publish.
Find out more
ORIGINAL RESEARCH article
Front. Mar. Sci. , 24 June 2022
Sec. Marine Ecosystem Ecology
Volume 9 - 2022 | https://doi.org/10.3389/fmars.2022.921639
This article is part of the Research Topic Coralline Algae: Past, Present, and Future Perspectives View all 10 articles
Coralline algae are important components in a large variety of ecosystems. Among them, rhodoliths are a group of free-living coralline red algae that cover extensive coastal areas, from tropical to polar regions. In contrast to other ecosystem engineers, limited research efforts preclude our understanding of their physiology, underlying mechanisms, drivers and potential differences related to species under varying environments. In this study, we investigated the photosynthetic and calcification mechanisms of six Atlantic rhodolith species from different latitudes, as well as intra-specific differences in one species from four locations. Laboratory incubations under varying light levels provided simultaneous photosynthesis- and calcification-irradiance curves, allowing the assessment of inter- and intra-specific differences on the coupling between these two processes. Stable isotope analysis and specific inhibitor experiments were performed to characterize and compare carbon-concentrating mechanisms (CCMs), as well as the involvement of specific ion-transporters for calcification. Our findings showed significant differences in rhodolith physiological mechanisms that were partially driven by local environmental conditions (light, temperature). High variability was found in the coupling between photosynthesis and calcification, in CCM-strategies, and in the importance of specific ion transporters and enzymes involved in calcification. While calcification was strongly correlated with photosynthesis in all species, the strength of this link was species-specific. Calcification was also found to be reliant on photosynthesis- and light-independent processes. The latter showed a high plasticity in their expression among species, also influenced by the local environment. Overall, our findings demonstrate that (1) rhodolith calcification is a biologically-controlled process and (2) the mechanisms associated with photosynthesis and calcification display a large variability among species, suggesting potential differences not only in their individual, but also community responses to environmental changes, such as climate change.
Free-living coralline algae are known to form major marine carbonate-producing ecosystems, so-called rhodolith beds that provide habitat for a high diversity of fauna and flora (Riosmena-Rodriguez et al., 2017). These ecosystems occupy extensive areas around the world, from polar to tropical regions (Foster, 2001; Riosmena-Rodriguez et al., 2017), and the increasing number of recent discoveries (e.g., Harvey et al., 2017; Rebelo et al., 2018; Sreeraj et al., 2018; Bracchi et al., 2019; Jeong et al., 2019; Adams et al., 2020; Otero-Ferrer et al., 2020; Ribeiro and Neves, 2020; Neves et al., 2021; Ward et al., 2021), and global distributional models (Fragkopoulou et al., 2021; Rebelo et al., 2021), suggest that rhodolith beds may have an even wider distribution than anticipated, mainly shaped by temperature, light, pH, nutrients and water current velocity (Carvalho et al., 2020; Fragkopoulou et al., 2021; Sissini et al., 2021).
Despite their worldwide distribution and the acknowledged ecological services that rhodolith beds provide in coastal regions (Riosmena-Rodriguez et al., 2017), knowledge regarding organism- and community-level physiological performance and productivity is still scarce and regionally focused, e.g. in Europe (Figure 1A). At the community level, a few studies have been performed on temperate European rhodolith beds, to determine rhodolith-bed productivity or in situ effects of ocean acidification (Martin et al., 2005; Martin et al., 2007a; Attard et al., 2015; Burdett et al., 2018). Similarly, at the organismal level, our understanding of rhodolith physiological mechanisms and species-specific differences is still majorly lacking. A recent analysis identified a total of 106 rhodolith-forming species worldwide (Rebelo et al., 2021), though at a global scale, scattered physiological information is currently available for 15 species only (Figure 1A).
Figure 1 (A) Overview of rhodolith species and locations for which primary production and/or calcification rates have been reported. Sampling locations of rhodolith species studied in this work are indicated by red triangles (1- Galway, Ireland; 2- Armação de Pêra, South Portugal; 3- Bicudas, Porto Santo Island, Portugal; 4- Caniçal, Madeira Island, Portugal; 5- Arguineguín, Gran Canaria, Spain; 6- Gando, Gran Canaria, Spain; 7- Arvoredo, South Brazil). (B) Studied rhodolith species and their respective sampling location and depth (in parenthesis). Map was downloaded from Mapswire (https://mapswire.com/). (1Martin et al., 2005; 2Martin et al., 2007a; Martin et al., 2007b; 3Attard et al., 2015; 4Burdett et al., 2018; 5Littler et al., 1991; 6Büdenbender et al., 2011; 7Kamenos et al., 2013; 8Schoenrock et al., 2018; 9Legrand et al., 2021; 10Martin et al., 2006; 11Noisette et al., 2013a; 12Noisette et al., 2013b; 13Legrand et al., 2017; 14Legrand et al., 2019; 15Qui-Minet et al., 2019; 16Qui-Minet et al., 2021; 17Kim et al., 2020; 18Schubert et al., 2019; 19Carvalho et al., 2020; 20Schubert et al., 2021a; 21Reynier et al., 2015; 22Figueiredo et al., 2012; 23Steller et al., 2007; 24King and Schramm, 1982; 25Sordo et al., 2016; 26Sordo et al., 2018; 27Sordo et al., 2019; 28Sordo et al., 2020; 29Schubert et al., 2021b; 30Zweng et al., 2018; 31Vásquez-Elizondo and Enríquez, 2016; 32Cornwall et al., 2017; 33Semesi et al., 2009; 34Payri, 1997; 35Cornwall et al., 2018; 36Comeau et al., 2018; 37Comeau et al., 2019a; 38Comeau et al., 2019b; 39Narvarte et al., 2020).
Overall, coralline algal species have been shown to express a high variability regarding their physiological performance under similar environmental conditions (e.g., Chisholm, 2000; Chisholm, 2003; Vásquez-Elizondo and Enríquez, 2016; Qui-Minet et al., 2021; Schubert et al., 2021a), which suggest differences in physiological mechanisms and/or the extent to which they are expressed. This assumption is supported by recent studies that showed a high plasticity in inorganic carbon uptake strategies for photosynthesis (Hofmann and Heesch, 2018; Zweng et al., 2018; Bergstrom et al., 2020). Also, comparisons among different species, from the same habitat, suggest a variability in the strength of the coupling between photosynthesis and calcification (Vásquez-Elizondo and Enríquez, 2016; Qui-Minet et al., 2021). The direct relationship between these processes was demonstrated early on (Digby, 1977; Pentecost, 1978; Borowitzka, 1981; Borowitzka, 1987) and is supported by recent studies (e.g., Martin et al., 2013; Vásquez-Elizondo and Enríquez, 2016; Sordo et al., 2019), which showed a decline in calcification upon inhibition of photosynthesis (Hofmann et al., 2016; McNicholl et al., 2019). This link has been related to an elevation of the pH at the calcification site due to photosynthetic activity. Considering differences in species’ metabolic rates (Chisholm, 2000; Chisholm, 2003; Vásquez-Elizondo and Enríquez, 2016; Qui-Minet et al., 2021) and morphological traits, which affect pH conditions in the diffusive boundary layer (DBL; Cornwall et al., 2017; Schubert et al., 2021a) that are positively correlated with the pH of the calcifying fluid (pHcf; Comeau et al., 2019a), it can be assumed that its strength varies among species. Furthermore, coralline CaCO3 precipitation in the dark, with lower and highly variable rates among species (e.g., Chisholm, 2000; Vásquez-Elizondo and Enríquez, 2016; Qui-Minet et al., 2021; Schubert et al., 2021a), and when photosynthesis is inhibited (Hofmann et al., 2016; Hofmann et al., 2018) suggests that calcification is partially independent from photosynthesis. Hence, the relationship between the two processes might be species-specific.
Coralline algal calcification occurs within the cell walls, up to three cell layers deep (primary calcification), while secondary calcification can occur as inferfilament precipitation between cells and conceptacle in-filling (Borowitzka, 1987; Adey et al., 2013). The physiological process of calcification in coralline algae is still poorly understood, though over the last decade several conceptual models have been proposed. It has been suggested that calcification is a biologically induced, rather than a controlled process, associated with cell wall organic-matrix-induced mineralization (Nash et al., 2019). However, mechanistic studies and derived models suggest that coralline algae exert some control over the calcification process, by promoting favorable conditions for CaCO3 precipitation through photosynthesis and active dissolved inorganic carbon (DIC) and ion transport pathways (Comeau et al., 2013; Koch et al., 2013; Hofmann et al., 2016; Cornwall et al., 2017; McNicholl et al., 2019). Recent studies showed that the extent of photosynthesis-promoted increase of pH in the DBL (pHDBL) is species-specific and influenced by external seawater pH, but also by species’ morphology, due to its strong influence on DBL thickness (Cornwall et al., 2017; Hofmann et al., 2018; Schubert et al., 2021a). Further evidence suggests that it is coupled to the activity of the external carbonic anhydrase (CAext) and active influx and efflux of protons (Hofmann et al., 2016). In fact, it is generally assumed that an active proton transport exists in the form of an antiport, symport and/or a proton pump, likely involving HCO3-/H+ and/or OH-/H+ symport and/or a Ca2+-driven ATPase (Okazaki, 1977; Borowitzka, 1987; McConnaughey and Falk, 1991; Mori et al., 1996; Comeau et al., 2013; Koch et al., 2013; Hofmann et al., 2016; Cornwall et al., 2017; Hofmann et al., 2018; McNicholl et al., 2019). In addition, microsensor studies demonstrated (a) the presence of a light-induced, but photosynthesis-independent mechanism that contributes to the pH dynamics at the algal thallus surface (Hofmann et al., 2016; Hofmann et al., 2018; McNicholl et al., 2019), and (b) that an elevated thallus surface pH in darkness in an Artic rhodolith may sustain calcification under these conditions (Hofmann et al., 2018). Finally, Comeau et al. (2019a) demonstrated a linear relationship between the pH gradient in the DBL and the pHcf, which suggests that the elevation of the pHDBL favors the export of protons from the calcification site by reducing the proton gradient between the calcifying fluid and the bulk seawater.
Reviewing available information for rhodoliths, it becomes evident that only a few studies have focused on a general physiological characterization, including information on specific rates of photosynthesis and/or calcification, productivity estimates, seasonal variation in physiological rates and/or a physiological comparison among species (Littler et al., 1991; Payri, 1997; Martin et al., 2006; Martin et al., 2007a; Martin et al., 2007b; Sordo et al., 2020; Qui-Minet et al., 2021; Schubert et al., 2021a). Even less information is available regarding the mechanisms driving rhodolith photosynthesis and calcification (Cornwall et al., 2017; Hofmann and Heesch, 2018; Hofmann et al., 2018; Zweng et al., 2018). Essentially, most physiological data derive from studies that assessed the effects of climate change-related and other environmental factors on rhodolith performance (King and Schramm, 1982; Steller et al., 2007; Semesi et al., 2009; Büdenbender et al., 2011; Kamenos et al., 2013; Noisette et al., 2013a; Noisette et al., 2013b; Sordo et al., 2016; Vásquez-Elizondo and Enríquez, 2016; Cornwall et al., 2017; Legrand et al., 2017; Schoenrock et al., 2018; Sordo et al., 2018; Zweng et al., 2018; Legrand et al., 2019; Sordo et al., 2019; Comeau et al., 2019a; Comeau et al., 2019b; Qui-Minet et al., 2019; Schubert et al., 2019; Carvalho et al., 2020; Kim et al., 2020; Legrand et al., 2021; Schubert et al., 2021b). These studies show that rhodoliths express a wide array of responses to environmental changes (see also Martin and Hall-Spencer, 2017), suggesting a high variability in the mechanisms related to photosynthesis and calcification. Thus, the goal of this study was to advance our knowledge regarding these mechanisms and to identify potential species-specific differences, which could explain their variable responses and would allow identifying potential patterns. For this purpose, a general physiological characterization and comparison of rhodolith-bed forming species was performed, at the inter- and intra-specific level, specifically focusing on the mechanistic coupling between photosynthesis and calcification and potential species-specific and environmentally driven variability. Additionally, inorganic carbon uptake pathways for photosynthesis and calcification, as well as the importance of ion-transporters for these processes were compared among species.
Individuals of the predominant rhodolith species from rhodolith beds in Europe, off the coast of northwestern Africa and Brazil were collected by SCUBA diving (Figure 1) and kept under laboratory conditions during the time required for the measurements (for details see Supplementary Material). Samplings were undertaken during the respective warm seasons (early to late summer, 2017-2021), at depths between 5 and 33 m (Table 1). Also, light attenuation profiles in the water column were obtained (n=5-6 per location), using an underwater quantum sensor (LI-192, LI-COR Environmental, USA), attached to a LI-250A Light Meter (LI-COR Environmental, USA), to calculate light attenuation coefficients (Kd) for the different locations.
Table 1 Overview of the studied species, the locations they were collected and the respective depths and environmental conditions at the time of collection.
Species were identified based on previous molecular identification of specimens collected at the sampling locations and diagnostic morphological characters, including: Lithothamnion crispatum Hauck (Farias et al., 2010), Melyvonnea erubescens (Foslie) Athanasiadis & D.L.Ballantine (Sissini et al., 2014), Lithophyllum atlanticum Vieira-Pinto, M.C.Oliveira & P.A.Horta (Vieira-Pinto et al., 2014), Phymatolithon sp. (Madeira and Porto Santo Islands; Neves et al., 2021), and Phymatolithon lusitanicum V.Peña (Carro et al., 2014; Pardo et al., 2014; Peña et al., 2015a).
Specimens collected in Gran Canaria (the Canary Islands) were morphologically similar to the rhodolith species Phymatolithon sp., collected in nearby Madeira and Porto Santo Islands (Neves et al., 2021). To confirm their conspecificity, their identification was done using molecular tools. DNA was extracted from a selection of eight specimens from two locations (six from Arguineguín, and two from Gando), using a E.Z.N.A.® Tissue DNA Kit (Omega Bio-tek, United States) and following the manufacturer protocol. The psbA locus was amplified using primer pairs: psbA-F1/psbA-R2 (Yoon et al., 2002) and the thermal profile for the PCR reaction followed Peña et al. (2015b). The PCR product was purified and sequenced by the SAI-UBM department of the University of Coruña, Spain. Sequences were assembled with the assistance of CodonCode Aligner® (CodonCode Corporation, USA), adjusted by eye using SeaView version 4 (Gouy et al., 2010), and submitted to the Barcode of Life Data Systems (BOLD, Ratnasingham and Hebert, 2007) and GenBank. Estimates of genetic distance (uncorrected p-distances) between the eight psbA sequences generated in the present study and 29 publicly available sequences of the rhodolith species Phymatolithon sp. from the Madeira archipelago were calculated in MEGA v. 6 (Tamura et al., 2013). The low pairwise sequence divergence found between both regions (<0.6%, 0–5 bp difference) supported conspecificity of rhodolith collections and therefore, the identification of the Canary Islands rhodoliths as Phymatolithon sp.
Similarly, the identification of Phymatolithon calcareum (Pallas) Adey & McKibbin from Ireland was confirmed using molecular tools. The DNA of five specimens, collected in the Irish locality of Ardeast (Table 1), was extracted using a NucleoSpin® 96 Tissue kit (Macherey-Nagel, GmbH and Co. KG, Germany). The mitochondrial COI-5P fragment was PCR-amplified using the primer pair GazF1 and GazR1 (Saunders, 2005). The thermal profile for amplification and PCR reaction was as described above and the PCR products were purified and sequenced at the Muséum National d’Histoire Naturelle y Eurofins (Eurofins Scientific, Nantes, France). Sequences were assembled and analyzed as described above. The estimates of genetic distance (uncorrected p-distances) between the four COI-5P sequences, obtained from our collection and 96 publicly available sequences of P. calcareum, mainly from Atlantic European coasts (Pardo et al., 2014), were calculated in MEGA v. 6 (Tamura et al., 2013). The low pairwise sequence divergence found (<0.7%, 0–5 bp difference) supported the identification of P. calcareum.
Rhodolith individuals from each site (n=5 per species and location) were incubated at their respective temperature, recorded during sample collection (Table 1), with filtered seawater (0.45 µm) in sealed custom-made plexiglass chambers (V=150 mL) with internal circulation provided by a magnetic stirrer. After an initial incubation in darkness to determine dark respiration rates (RD), rhodoliths were exposed to a series of increasing light intensities and, subsequently, they were again incubated in darkness to determine post-illumination or light respiration rates (RL).
The incubation time at each light intensity varied between 0.5 h, for the subtropical Brazilian species, to 1 h for the other species; this was based on previous incubations, testing different incubation times, to assure a high enough signal-to-noise ratio for measuring oxygen concentration and alkalinity. At the beginning and end of each incubation, the oxygen concentration was measured with an oxygen meter (Fibox 4, PreSens, Germany) and water samples were taken, poisoned with HgCl2 and stored in borosilicate tubes (two tubes per incubation chamber, V=25 mL each) for later estimation of calcification rates. Afterwards, the rhodoliths were dried (48 h at 60°C) and their surface area was determined by the wax-dipping method (Schubert et al., 2021a), to normalize metabolic and calcification rates and calculate surface area to dry weight ratios (SA/DW).
Gross photosynthetic rates (GP) were calculated by adding respiration rates (average of RD and RL) to the measured net photosynthetic rates (NP). The photosynthetic quantum efficiency (α) was estimated from the initial slope of the light response curve, by linear least-squares regression. Irradiance of compensation (Ec) was calculated from the ratio RD/α, and the saturation irradiance (Ek) from the ratio Pmax/α. The maximum photosynthetic rates (NPmax and GPmax) were obtained from the average of the maximum values above saturating irradiance. Calcification rates of the rhodolith species were determined from alkalinity measurements of seawater samples before and after each incubation, using the alkalinity anomaly principle, based on the ratio of two equivalents of total alkalinity per each mole of CaCO3 precipitation (Smith and Kinsey, 1978). For alkalinity (TA) measurements, duplicate analyses of each sample were performed, using the Gran titration method (Hansson and Jagner, 1973; Bradshaw et al., 1981). The samples were titrated with HCl 0.1 M, using an automated titration system (Titroline 7000, SI Analytics, Mainz, Germany), coupled to an autosampler (TW alpha plus, SI Analytics, Mainz, Germany). Data were captured and processed with a computer, using Titrisoft 3.2 software (SI Analytics, Mainz, Germany). For quality control, a certified reference material of known total alkalinity was used to calibrate the method (CRMs, Batch No. 160; supplied by the Marine Physical Laboratory, Scripps Institution of Oceanography, USA), yielding TA values within 5 μmol kg-1 of the certified value. Similar to photosynthesis, the maximum light calcification rates (max. GLight) and the initial slope of the Calcification-Irradiance curve (αG) were determined. Here, the saturation irradiance (Ek) was estimated as the ratio of max. GLight/αG. In a posterior analysis, calcification was fitted against the respective gross photosynthesis for the different light levels and the initial slope of the curves was estimated using linear least-squares regression. Furthermore, mean ratios of max. GLight/GPmax (mol CaCO3 precipitated per mol O2 evolved) were obtained by averaging the ratios of the light levels above saturation.
These experiments assessed the absence/presence of carbon-concentrating mechanisms (CCMs) in the studied rhodolith species. For this, rhodolith individuals (n=5 per species and location) were incubated at their respective temperature and saturating light conditions (~2-3Ek, derived from P-E curves) for 9-10.5 h and the pH of the seawater was measured with a pH meter (Metrohm, Switzerland), at the beginning and the end of the incubation. These experiments are based on the hypothesis that, if the seawater pH in the sealed incubation becomes greater than 9.0, no more CO2 is present, and it can be assumed that the species is able to uptake HCO3- during photosynthesis (Maberly, 1990). After the incubations, the chambers (without samples) were left open to the ambient air for re-calibration, making sure that the change in pH was due to the metabolism of the algae.
To identify potential differences among species regarding a preferential use of CO2 or bicarbonate (or both) for photosynthetic carbon assimilation (Maberly et al., 1992), the δ13C content of the algal tissue was analyzed. For this, rhodoliths (n=5 per species and location) were dried (48 h at 60°C) and afterwards placed in 1N HCl until the calcareous tissue was removed. The remaining tissue was rinsed with distilled water, dried again (48 h at 60°C) and grounded into a fine powder, using a porcelain mortar and pestle. The samples were then weighed into pressed tin capsules and sent to the Stable Isotope Facility at University of California, Davis for analysis, using an Elementar vario MICRO cube elemental analyzer (Elementar Analysensysteme GmbH, Langenselbold, Germany) interfaced to a Sercon Europa 20-20 isotope ratio mass spectrometer (Sercon Ltd., Cheshire, UK).
To gain insight into rhodolith carbon-concentrating and calcification mechanisms, and potential species-specific differences, their physiological responses to different inhibitors were measured. The specific inhibitor of PSII, 3-(3,4-Dichlorphenyl)-1,1-dimethylurea (DCMU, Sigma Aldrich) was dissolved in ethanol and added to the seawater to a final concentration of 10 µM. Acetazolamide (AZ, Sigma Aldrich), a specific inhibitor of the external carbonic anhydrase, which mediates hydrolysis of HCO3-, was dissolved in 10 mM NaOH and used at a final concentration of 0.125 mM (Hofmann et al., 2016). The anion exchange inhibitor 4,4’-Diisothiocyano-2,2’-stilbenedisulfonic acid (DIDS, Sigma Aldrich) that blocks direct HCO3- uptake (Drechsler and Beer, 1991) was dissolved in dimethylsulfoxide (DMSO, Sigma Aldrich) and added at a final concentration of 0.6 mM. Ruthenium red (RR, Sigma Aldrich), a specific inhibitor of Ca-ATPase (Watson et al., 1971; Ip et al., 1991), and the Ca-channel inhibitor verapamil (VP, Sigma Aldrich) were both dissolved in DMSO and used at a final concentration of 100 µM (de Beer and Larkum, 2001; Marshall and Clode, 2003). The final concentrations of DMSO and ethanol in the inhibitor treatments were 0.1%.
Rhodolith photosynthetic, respiratory and calcification rates were determined before and after inhibitor treatment by incubating individuals (n=5 per species, location and inhibitor) at their respective temperatures for 0.5-1 h, at a saturating light intensity (~2-3Ek, derived from P-E curves), and subsequently for 0.5 h in darkness, to determine maximum photosynthesis and respiration rates, respectively. Also, water samples were taken before and after each incubation for later estimation of calcification rates, as described above. Afterwards, with exceptions of the treatments with DCMU and AZ, the same samples were incubated with the inhibitors for 1 h in darkness, to ensure complete penetration into the cells, and then incubated again in light as described above. In the case of DCMU, before incubating the samples with the inhibitor, they were kept in darkness for 1-1.5 h to ensure complete oxidation of the electron-transport chain after the previous light incubation, as this inhibitor attaches to the QB-site within PSII. On the other hand, AZ was added directly after finishing the control measurements of the samples, without the inhibitor, and the incubations were repeated, as the effect of this inhibitor is immediate (membrane impermeable inhibitor).
Data were tested for normality and heteroscedasticity, using the Shapiro-Wilk and Levene’s tests, respectively, and when required, log-transformed to normalize the data (RD, GPmax/RD, α, Ek, Ec, GLight, αG). Physiological parameters were analyzed using one-way ANOVAs, with species as the between-subjects factor. In cases where significant differences were found, a Tukey’s HSD post hoc test was conducted to determine significant groupings. Paired sample t-tests were used to compare the data from the inhibitor studies (before and after inhibitor treatment). To visualize differential inhibitor response profiles among rhodolith species, heat maps were generated using Python 3.10.2, along with the Pandas 1.3.5 (McKinney, 2011), Matplotlib 3.5.1 (Hunter, 2007) and Seaborn 0.11.2 (Waskom et al., 2020) libraries.
To visualize multivariate similarities in physiological characteristics across the range of species and locations under varying environmental contexts, RDA (Redundancy Analysis) ordinations, with the ‘vegan’ R package (Oksanen et al., 2020) and further customization via the ‘ggord’ R package ((Beck 2017), were carried out as a constrained ordination technique. Separate RDAs were implemented for both photosynthesis and calcification responses. Bi-plots were then constructed, displaying each rhodolith species, and for intra-specific comparison of Phymatolithon sp. from the different locations, including predictive variables (light, temperature) as vectors of varying length and direction. The significance of these multivariate configurations was tested by the ‘anova.cca’ function, which also assessed the significance of each axis (component) to explain a significant amount of variation of the multivariate dataset. Variance inflation factors (VIFs) were always <5, indicating low collinearity among predictor variables for the RDA configuration in the bi-dimensional space.
Both photosynthesis and calcification increased when rhodolith species were exposed to increasing light intensities, until reaching a saturation point after which their rates stabilized around a maximum value (Supplementary Figure S1). However, the rate of these increases and the maximum values differed between the two processes and among the rhodolith species. When plotted against each other, this resulted in significant species-specific differences in the relationship between photosynthesis and calcification, specifically in the initial slopes of the photosynthesis-calcification curves at sub-saturating irradiances (<Ek) and the maximum values reached (>Ek) (Figure 2; Table 2). Calcification increased with photosynthesis at rates of 0.11 to 2.26 mol O2 mol-1 CaCO3, while maximum ratios of GLight/GPmax ranged from 0.11 to 0.51 mol CaCO3 mol-1 O2 (Table 2). Among species, the cold-temperature Phymatolithon calcareum expressed the slowest increase of calcification with photosynthesis and the lowest ratio of max. GLight/GPmax, while the subtropical Brazilian species showed the fastest increases of CaCO3 precipitation with photosynthesis and the highest GLight/GPmax ratios (Figure 2; Table 2).
Figure 2 Photosynthesis-calcification relationships for Atlantic rhodolith species (location, depth), obtained from light curves (dotted line indicates Ek). Data points represent mean ± SE (n=5).
Table 2 Comparison of parameters derived from photosynthesis-calcification relationships in different Atlantic rhodolith species (Figure 2).
Differences among species were also reflected in their maximum photosynthetic performance (GPmax), which was lowest in Phymatolithon sp. from habitats with low light availability (20-33 m), also associated with the lowest Ek and Ec values (Figure 3A; Supplementary Table S1). On the other hand, the highest GPmax values were found in species from contrasting latitudes, such as the cold-temperate P. calcareum and the subtropical Phymatolithon sp. from Arguineguín (14 m) and Lithothamnion crispatum from South Brazil (8 m). While there was no clear pattern in GPmax, light calcification (GLight) was highest in the subtropical species, which inhabit depths under high light availability (20-21% of incident light), comprised of Phymatolithon sp. from Arguineguín (14 m) and the Brazilian species Lithophyllum atlanticum, Melyvonnea erubescens, and L. crispatum (Figure 3B; Supplementary Table S2). In contrast, dark calcification rates (GDark) varied among species and seemed to be rather species-specific. For example, the three southern Brazilian species from the same location and depth showed large differences in GDark, varying between positive and negative values (indicating night-time dissolution) (Figure 3B). The large variability was also reflected in the species’ GDark/GLight ratios, which ranged between 0.09-0.33, with the highest ratios expressed by Phymatolithon sp. from Gran Canaria (Supplementary Table S2).
Figure 3 Rhodolith physiological performance. (A) Maximum gross photosynthetic rates (GPmax) and dark respiration (RD, striped bars), and (B) maximum light calcification (GLight) and dark calcification (GDark, striped bars) of Atlantic rhodolith species (mean ± SE, n=5). Significant differences among species are indicated by different lowercase (GPmax, GLight) and uppercase (RD, GDark) letters (ANOVA, Tukey’s HSD posthoc, p<0.05). Data represent mean ± SE (n=5).
Multivariate analysis of the overall photosynthetic performance identified clear differences between the cold-temperate P. calcareum and the other species (except for L. crispatum), while the other two Phymatolithon species showed a high similarity (Figure 4A). The redundancy analysis revealed that light availability and temperature in the species’ natural habitat collectively explained 36% of the total variance, with light exerting a larger influence, strongly correlating with Ek, Ec and RD (Figure 4A; Supplementary Table S3). Regarding calcification performance, the different Phymatolithon species showed similarities, but differed greatly from M. erubescens (Figure 4B). Here, light and temperature explained 18% of the variance, specifically exerting an influence on GLight and αG (Figure 4A; Supplementary Table S3).
Figure 4 Influence of environmental factors on rhodolith physiological performance. Redundancy analysis (RDA) biplots show the contribution of local environmental conditions (light availability, temperature) to the variation in photosynthetic (A, C) and calcification parameters (B, D) of all studied Atlantic rhodolith species (A, B) and of Phymatolithon sp. sampled in different locations (C, D). Arrows represent correlations between environmental variables and the RDA axes, with arrow length indicating the strength of the correlation. Response variables are indicated by circles (GPmax, NPmax- max. gross and net photosynthesis; RD- dark respiration; α, alphaG- photosynthetic and calcification efficiency under subsaturating light intensities; Ek, Ec- saturating and compensatory light intensity; GLight, Gdark- max. light and dark calcification).
Intra-specific comparison of Phymatolithon sp. from four locations and depths showed no significant differences in the photosynthesis-calcification relationship, as rhodoliths from the different populations expressed similar initial slopes and max. ratios of GLight/GPmax, also similar to the South Portuguese species P. lusitanicum (Figure 2; Table 2). On the other hand, an increasing trend in GPmax, RD, GLight and GDark was found with increasing light availability and temperature (Figure 3; Supplementary Tables S1, S2). Redundancy analysis showed that rhodoliths from Arguineguín (Gran Canaria) differed greatly in their general physiological performance, relative to those from other populations (Figures 4C, D). Here, site-specific light and temperature conditions explained 51% and 57% of the intra-specific variance in photosynthetic and calcification performance, respectively. In the case of photosynthesis, light and temperature exerted a similar influence on the photosynthetic variability among locations, correlating with NPmax, GPmax and RD. The variability in calcification parameters was mostly correlated to differences in temperature among the locations, with weaker influence of light availability (Figures 4C, D; Supplementary Table S3).
The δ13C values differed significantly among the rhodolith species (ANOVA, p<0.0001), ranging between -19.9‰ and -14.1‰ (Figure 5A). The highest values were found in the subtropical Brazilian species (-16.9 to -14.1‰), while the lowest value were expressed by P. calcareum, P. lusitanicum and Phymatolithon sp. (Bicudas, Porto Santo Island). These values were strongly and linearly related with the pH compensation points determined in six of the species (R2 = 0.90, p=0.0027), with all of them showing the capacity to reach pH≥ 9.0 (Supplementary Figure S2), suggesting the presence of CCMs.
Figure 5 DIC-uptake strategies in Atlantic rhodolith species. (A) δ13C values, with significant differences among species (ANOVA, Tukey’s HSD posthoc, p<0.05) indicated by different letters (dotted line indicates the threshold, with values above indicating HCO3–only users), and (B) importance of active bicarbonate transport and external carbonic anhydrase activity for photosynthesis (expressed as significant changes before and after treatment with the respective inhibitors, paired sample t-test, p<0.05, Supplementary Table S3) (ns - not significant, nd - not determined). Data represent mean ± SE (n=5).
Inhibitor studies with DIDS and AZ, which inhibit active bicarbonate transport and CAext, respectively, indicated that the species expressed differences in their CCMs. While photosynthesis in the subtropical Brazilian species showed signs of active HCO3–transport (10-22% inhibition by DIDS), the other rhodolith species did not express any significant changes in photosynthetic activity under this inhibitor treatment (Figure 5B; Supplementary Table S4). In contrast, the AZ-treatment indicated that photosynthesis in all species relied on the activity of the CAext, as its inhibition significantly decreased GPmax (Figure 5B). The data showed that photosynthesis was generally 50-60% lower in absence of CAext activity, though Phymatolithon sp. from Caniçal (Madeira Island) showed a lesser effect (33% lower GPmax) (Figure 5B; Supplementary Table S4).
The use of different inhibitors revealed a highly dynamic pattern regarding the importance of specific processes for rhodolith calcification. All species showed a strong correlation of light calcification and photosynthesis, as the former experienced a significant decrease (68-91% lower GLight) upon inhibition of the latter, which was also directly related to the species’ decline in calcification in darkness (GDark; Figure 6). In fact, in most species responded with a decline of GLight under photosynthesis inhibition and in darkness, except for M. erubescens and L. atlanticum that expressed negative dark calcification rates. Similarly to photosynthesis, all species showed a significant decrease in GLight under CAext inhibition (by 51-125%, Figure 7; Supplementary Table S4, S5). To distinguish between direct effects of CAext inhibition on calcification and indirect effects due to a decline in photosynthesis, changes in GPmax/GLight ratios were analyzed, where non-significant changes in the ratios indicated only indirect effects on calcification due to photosynthesis decline. The results indicated that for the Brazilian species the recorded decline in calcification upon CAext inhibition was the sole result of an indirect effect due to the decline in GPmax. On the other hand, the other rhodolith species exhibited a larger decrease in GLight, compared to GPmax, which suggested a direct role of CAext for calcification (Figure 7; Supplementary Tables S4-S6).
Figure 6 Relationship between rhodolith calcification in darkness and after photosynthesis inhibition with DCMU in light, expressed as changes in relation to light calcification and to control values before inhibitor treatment, respectively. Data points represent mean ± SE (n=5).
Figure 7 Importance of external carbonic anhydrase and specific ion transporters for rhodolith physiological processes. Heat maps indicate the effects of inhibition of specific processes on (A) maximum gross photosynthesis and (B) light calcification in different rhodolith species, expressed as significant changes before and after the inhibitor treatment (paired sample t-test, p<0.05, Supplementary Tables S3, S4).
Likewise, the inhibition of active bicarbonate transport caused different responses among the species. While the absence of HCO3–transport did not cause significant effects in calcification in Phymatolithon sp. from Arguineguín (Gran Canaria), it induced a significant decrease in GLight in the same species from Caniçal (Madeira Island), in P. lusitanicum, and in the three Brazilian species, though the magnitude of the effect varied greatly (from 15 to 73% decrease). Comparing these findings to the inhibitor effect on species’ photosynthesis, it suggests the involvement of active bicarbonate transport in calcification, but not in photosynthesis, in the species from Europe and the coast of northwestern Africa (Figure 7; Supplementary Tables S4, S5). In the case of the Brazilian species, where significant responses in both photosynthesis and calcification under inhibitor treatment were found, the comparison of GPmax/GLight ratios indicated that active HCO3–transport was directly involved in both processes in L. atlanticum and L. crispatum, but not in M. erubescens, in which the inhibitor effect on calcification seemed to be caused exclusively by the decline in photosynthesis (Supplementary Table S6). Surprisingly, P. calcareum showed an opposite response to DIDS, with a 97% increase in calcification (Figure 7B; Supplementary Table S5). The cold-temperate P. calcareum also differed from the others regarding the importance of Ca-channel and –ATPase for calcification, as it did not exhibit any significant effects upon their inhibition (Figure 7). Among the other species, the importance of these two transporters varied greatly. Some species exhibited a significant decline in calcification under Ca-channel and/or Ca-ATPase inhibition, while others did not. For example, little to no significant effect of Ca-channel inhibition was found in two of the subtropical Brazilian species (L. crispatum and L. atlanticum, respectively), while the inhibition of the Ca-ATPase did not result in significant effects in Phymatolithon sp. from Arguineguín (Gran Canaria) and M. erubescens from Brazil (Figure 7).
When comparing the effects of the different inhibitors, at an intra-specific level in Phymatolithon sp. from two locations, the results show that the calcification responses varied greatly, especially regarding the importance of DIDS-sensitive bicarbonate transport and Ca-ATPase (Figure 7; Supplementary Table S5). Also, CAext was seemingly more important for calcification in the rhodoliths from Arguineguín (Gran Canaria), compared to those from Caniçal (Madeira Island) (Figure 7B; Supplementary Table S5), also shown by its larger changes in GPmax/GLight ratios (Supplementary Table S6).
This study demonstrated that rhodoliths express high inter-specific variability in their physiological performance and underlying mechanisms. Specifically, the different species show a high plasticity in the strength of the coupling of photosynthesis and calcification, in the CCM-strategies and in the ion transporters and enzymes involved in calcification. Furthermore, intra-specific comparisons suggest that this variability is at least partially modulated by local environmental conditions.
Overall, our findings regarding the importance of temperature and light for rhodolith performance agrees with recently published distributional models, identifying these factors as two of the main drivers of rhodolith distribution (Carvalho et al., 2020; Simon-Nutbrown et al., 2020; Fragkopoulou et al., 2021; Sissini et al., 2021). At the inter-specific level, rhodolith photosynthetic performance was mainly driven by light, but very little by temperature and hence, it did not show a latitudinal pattern. On the other hand, temperature and light were identified as strong environmental drivers for rhodolith light calcification, which was reflected in increased GLight towards lower latitudes. This is consistent with higher summer calcification rates reported for cold- and warm-temperate rhodolith species (Sordo et al., 2020; Qui-Minet et al., 2021). Dark calcification rates, on the other hand, seemed to be related to species identity rather than environmental conditions, which is in accordance with other comparative studies for crustose coralline algae (Chisholm, 2000) and rhodoliths (Qui-Minet et al., 2021; Schubert et al., 2021a). In their comparative study of three rhodolith species, Qui-Minet et al. (2021) also showed that GDark can vary seasonally, from negative rates in winter to positive rates in summer, though the relative differences between species were maintained. This agrees with our findings of increased GDark with temperature at the intra-specific level in Phymatolithon sp. (Figure 3B). In fact, temperature was the main driver for the calcification differences in this species from different locations. The variability in the photosynthetic performance was induced by temperature and light availability, resulting in higher physiological rates of the specimens collected at the shallowest subtropical rhodolith bed (Arguineguín).
Species identity has a strong influence on the relationship between photosynthesis and calcification, as evidenced by significant differences among species and, specifically, those found among species from the same location (see L. atlanticum, M. erubescens, L. crispatum). This agrees with a recent comparison among the temperate rhodolith species, Lithothamnion corallioides, Lithophyllum incrustans and Phymatolithon calcareum, from the same habitat, which also exhibited significant differences in their GLight/GPmax ratios (Qui-Minet et al., 2021). This species-specific dependence of calcification on photosynthesis was also supported by differences in the decline of the former in the absence of the latter, either by using inhibitors, or under dark conditions (discussed in more detail below). Additionally, comparison at the intra-specific level suggests that local environmental conditions induced some variability in the photosynthesis-calcification relationship, as evidenced by differences found among Phymatolithon sp. from different locations. The likely cause for the variability was the differential influence of environmental factors on the two processes, as differences in calcification among locations were mostly driven by temperature, while variability in photosynthetic performance was induced, to a similar extent, by both light and temperature. Similar findings have also been reported for the temperate rhodolith Lithothamnion corallioides, which not only showed variable GLight/GPmax ratios among different locations, but also between seasons (0.40-0.53 in winter, 0.26-0.32 in summer) (Qui-Minet et al., 2021).
Marine macroalgae can express three mechanisms of DIC assimilation: (1) diffusive CO2 uptake, (2) CO2 uptake dependent on CAext catalyzed dehydration of HCO3-, and (3) direct transport of HCO3- (Johnston, 1991). The degree to which these mechanisms are used by an alga determines the extent to which the alga can use bicarbonate as inorganic carbon source, to maintain maximum, or near maximum, photosynthetic performance during times when DIC becomes limited due to high photosynthesis and/or the presence of a thick DBL.
Our findings, as well as previous studies, demonstrate that rhodoliths express active CCMs, but also a variability in δ13C values associated to the species and/or environmental conditions (Hofmann and Heesch, 2018; Hofmann et al., 2018). All the rhodolith species studied here expressed δ13C values that identified them as both CO2 and bicarbonate users (δ13C= -30 to -10‰, Maberly et al., 1992). Together with the pH-drift experiments, this demonstrated that the species were able to raise the pH>9.0, at which DIC in form of CO2 is functionally 0, suggesting the presence of CCMs. This agrees with reports of δ13C in other rhodoliths and crustose coralline algae (e.g., Wang and Yeh, 2003; Cornwall et al., 2015; Diaz-Pulido et al., 2016; Hofmann and Heesch, 2018; Zweng et al., 2018; Bergstrom et al., 2020).
The non-diffusive and diffusive DIC uptake in algae vary and can involve both the active transport of HCO3- and the catalytic conversion of HCO3- to CO2 by CA, with subsequent passive diffusion of CO2, respectively. Here, the use of specific inhibitors demonstrated that all studied rhodolith species relied on the activity of CAext for photosynthesis, though its importance varied among species. In addition, the subtropical Brazilian species expressed active HCO3- transport as CCM, as demonstrated by the significant decline in photosynthesis under DIDS-treatment. This evidently increased the carbon-concentrating efficiency in these species, as shown by their higher pH compensation point (see Supplementary Figure S2). Overall, it agrees with the seemingly prevalent importance of CAext for inorganic carbon assimilation in coralline algae (Hofmann et al., 2016; Hofmann et al., 2018; Hofmann and Heesch, 2018; Zweng et al., 2018). On the other hand, the presence/absence of a DIDS-sensitive active HCO3–transport appears to be more variable among species (this study; Hofmann et al., 2016; Hofmann et al., 2018; Zweng et al., 2018), though the here demonstrated importance of both CAext and active DIDS-sensitive bicarbonate transport in the subtropical Brazilian species agree with those in other tropical coralline algae (Hofmann et al., 2016; McNicholl et al., 2019). These findings were consistent with the higher (less negative) δ13C values of the subtropical Brazilian species, while the lower (more negative) values of the other species may indicate a higher reliance on CAext activity (Mercado et al., 2009). Nevertheless, the δ13C values identified all species as both bicarbonate and CO2 users, suggesting the presence of other bicarbonate uptake mechanisms, insensitive to DIDS.
CCM strategies in algae, and the degree to which they are expressed, are modulated by a variety of environmental conditions (e.g., light, temperature, pH, salinity, nutrients; Beardall et al., 1998; Beardall and Giordano, 2002; Giordano et al., 2005). As for the influence of pH conditions, these can also be modified by the organisms themselves, as photosynthetic activity increases seawater pH and simultaneously decreases CO2 concentration and it has been shown previously that this combination increased markedly the expression of CAext activity (Berman-Frank et al., 1995; Berman-Frank et al., 1998). Thus, when considering the demonstrated effect of algal morphology on DBL thickness and related pH dynamics (Hurd et al., 2011; Cornwall et al., 2013; Cornwall et al., 2014; Schubert et al., 2021a), morphology may be one of the factors driving species’ differences in CCM strategies.
A recent study on the Brazilian rhodolith species showed the effect of their morphology on DBL thickness and associated thallus surface pH dynamics (Schubert et al., 2021a). The species studied here differ in their general morphology (round, thin-branched, flattened), but also in the rugosity of the thallus surface, as indicated by their surface area to dry weight ratios. Brazilian rhodoliths have higher ratios, related to a higher number and length of protuberances, than the other studied species with similar round morphologies (not considering P. calcareum and P. lusitanicum, which have thin-branched and flattened morphologies, respectively) (Supplementary Table S7). These differences might explain the greater diversity and importance of CCMs, such as the presence of a DIDS-sensitive direct entry of HCO3- in the Brazilian species, which was absent in the others. The morphology-induced built-up of thicker DBLs results in an increase in the lengths of CO2 diffusive pathway, which together with a high photosynthetic activity will lead to carbon limitation. Thus, the lower morphological complexity of species from Europe and the coast of northwestern Africa with similar round morphologies, suggests thinner DBLs and consequently shorter CO2 diffusion pathways. This agrees with the importance of CAext and a higher reliance on diffusive CO2 uptake. In contrast, in the three Brazilian species (with thicker DBLs) CCMs are required to maintain photosynthetic activity. Indeed, the increase in the pHDBL between the rhodolith protuberances under light conditions found previously in the Brazilian species (mean=0.33-0.55 units higher than bulk seawater pH; Schubert et al., 2021a) might explain the found expression of an active HCO3- transport, as it is a carbon uptake mechanism that can be induced by high external pH values (Axelsson et al., 1995). Hence, the higher δ13C of the Brazilian species could be explained by their higher morphological complexity that drives the formation of thicker DBLs and results in an increase in the length of CO2 diffusion pathways and the need for CCMs (CAext and active HCO3- uptake). Based on this premise, and the assumption that coralline algae with lower CCM capacity have a higher diffusive CO2 uptake (Bergstrom et al., 2020), it could be hypothesized that among the studied species, morphologically less complex species, such as Phymatolithon sp. from Caniçal (Madeira Island), rely to a higher degree on diffusive CO2 entry as DIC uptake strategy, as evidenced by its lower pH compensation point and lesser importance of CAext for photosynthesis. This may also be the case in P. lusitanicum, which expresses a flattened morphology that is not inducive to thick DBLs and hence, diffusive CO2 uptake may also be more important in this species, as supported by its lower δ13C values.
Overall, inhibitor studies in different groups of calcifiers have shown that CAext, bicarbonate transporters, Ca-channels and Ca-ATPase are involved in carbonate precipitation (e.g., Dubois and Chen, 1989; McConnaughey, 1989; McConnaughey and Falk, 1991; Allemand and Grillo, 1992; Tambutté et al., 1996; Comeau et al., 2013; Hofmann et al., 2016; Hofmann et al., 2018). The present study supports this, though it also clearly shows that rhodoliths express inter-specific differences, not only in the dependence of calcification on photosynthesis, but also the importance of CAext and specific ion-transporters for calcification.
Firstly, our results showed a high variability in the decline of calcification upon photosynthesis inhibition, suggesting species-specific differences in the strength of the link between these two processes. Additionally, environmental conditions seem to exert an influence on this relationship, as shown in Phymatolithon sp., with rhodoliths from Arguineguín (Gran Canaria) showing a lower dependence of calcification on photosynthesis, compared to the same species from Caniçal (Madeira Island). The similar decrease in calcification rates in darkness (when compared to GLight), and upon photosynthesis inhibition in light in most of the studied species, points towards not only a photosynthesis- but also light-independent mechanism that enables rhodoliths to maintain positive calcification rates in the absence of photosynthesis in light, as well as in darkness. In this context, evidence for a HCO3-/H+ symport that moves protons away from the calcification site under both light and dark conditions, resulting in an increase in surface pH, has been found in the Arctic rhodolith Lithothamnion glaciale (Hofmann et al., 2018). The exceptions among the studied species were the two Brazilian species L. atlanticum and M. erubescens, in which this potential light-independent mechanism was either less effective (operating at a slower rate) or absent, resulting in carbonate dissolution in darkness and an almost complete cease of light calcification upon photosynthesis inhibition. As a recent comparative microsensor study showed, this feature is related to a pH decline at the thallus surface in darkness in these two species that was not found in L. crispatum from the same location (Schubert et al., 2021a). The magnitude of night-time decalcification was shown to be directly related to the pH decline in darkness, which was larger in L. atlanticum, resulting in higher carbonate dissolution, compared to M. erubescens. In previous studies, Hofmann et al. (2016; Hofmann et al., 2018) provided evidence that some coralline algae present a light-mediated, but photosynthesis-independent proton pump. The presence of such a mechanism might decrease the dependence of calcification on photosynthesis and could explain the significantly faster increase of calcification upon light exposure (αG) in the two aforementioned species, compared to the other species.
Secondly, differences among species were also found regarding the importance of bicarbonate-transporters and CAext for calcification. Our results show that they not only supported photosynthesis, but also calcification in some rhodolith species, while in others they were important only for one of those processes (Figure 8). Noteworthy here was the finding of the direct involvement of CAext in photosynthesis and calcification in the temperate and subtropical Phymatolithon species, while in the subtropical Brazilian species the activity was seemingly important only for photosynthesis and hence, only indirectly for calcification. Generally, it is assumed that CAext is involved directly in coralline algal calcification, by elevating the pH due to removal of H+ through HCO3- dehydration (HCO3-+H+↔CO2+H2O), with the resulting CO2 diffusing into the cells to be used for photosynthesis (Borowitzka, 1987; Koch et al., 2013; Hofmann et al., 2018; McNicholl et al., 2019). Though, in the proposed models, no clear distinction is made between its direct and indirect importance (activity related to photosynthetic DIC uptake) and more studies are needed to differentiate the functional role of CAext in coralline algal calcification. Another surprising difference found among the studied species was related to the importance of DIDS-sensitive active HCO3–transport. Even though this transporter did not play a role for photosynthesis in the species from Europe and those off the coast of northwestern Africa, in some of them it seemed to play a role in calcification (Figure 8). Yet, the extent to which this transporter was involved in calcification differed greatly among species. Furthermore, in the cold-temperate P. calcareum and the subtropical Phymatolithon sp. (Arguineguín), the DIDS-sensitive bicarbonate transporter did not play a role in neither photosynthesis nor calcification. In the case of the latter species, this also suggests an intra-specific variability in the degree to which calcification depends on this bicarbonate transporter. Phymatolithon sp. from Arguineguín (Gran Canaria) did not express active DIDS-sensitive bicarbonate transport, but its calcification depended highly on the activity of CAext, while in the same species from Caniçal (Madeira Island) both mechanisms were important to a certain degree (Figure 8).
Figure 8 Summarized overview of the importance of CAext and specific ion-transporters for rhodolith photosynthesis and/or calcification, based on inhibitor effects and accounting for declines in calcification caused indirectly by inhibitor effects on photosynthesis (“Both”- inhibitor effect on both processes, but larger in calcification, indicating direct effect on the latter; Supplementary Tables S3–S5).
Thirdly, a large variability among rhodolith species regarding calcium transporters was found. Generally, it is assumed that Ca-influx to cells occurs through voltage sensitive Ca-channels, while Ca-efflux is associated to a Ca-ATPase (McConnaughey, 1989). The latter has been shown to be associated with calcification in corals and algae (e.g., Okazaki, 1977; Okazaki et al., 1984; Kingsley and Watabe, 1985). The “trans” calcification mechanism model proposed for these calcifiers suggests that Ca-ATPase supplies Ca2+ to and removes H+ from the site of calcification, resulting in an increase in pH and [Ca2+] that favors CaCO3 precipitation (McConnaughey and Falk, 1991; McConnaughey and Whelan, 1997). Among the different groups of calcareous algae, Ca-ATPase has been shown to be specifically relevant in coralline algae (Okazaki, 1977). Evidence for its involvement in calcification has been provided by a recent microsensor study in a crustose coralline alga (Hofmann et al., 2016). Here, Ca2+ and pH dynamics recorded at the algal thallus surface under different light conditions, clearly demonstrated the presence of an active Ca-efflux and H+-influx into the cells in light, resulting in an increase of surface pH, and the inverse pattern in darkness. It also agrees with our findings of a significant decline in calcification upon ATPase-inhibition. Still, this effect was found only in four of the rhodolith species (Figure 8), indicating that its expression might not be universal in all species of this group and/or driven by the environment (see Phymatolithon sp. from Caniçal and Arguineguín). For example, the lack of any effects of Ca-ATPase inhibition in M. erubescens suggests that this species may express a different Ca2+/H+ exchanger to regulate pH at the calcification site.
The rhodolith species also exhibited differences regarding the importance of Ca-channels, as found through the treatment with verapamil. This inhibitor is specific for L-type (voltage-dependent, with long-lasting activation) Ca-channels (Triggle, 2006) and the lack of effects in L. atlanticum in either photosynthesis, or calcification, may suggest a different type of Ca-channel in this species, insensitive to verapamil. Differences in Ca-channels have been reported also for other calcareous macrophytes, such as Chara sp. and Halimeda discoidea Decaisne. The calcium flux in Chara spp. has been found to be insensitive to verapamil, but showed sensitivity to nifedipine, an inhibitor of N-type Ca-channels (Reid and Smith, 1992; Stento et al., 2000). On the other hand, neither the inhibition of L- nor N-type Ca-channels had an effect on Ca2+ dynamics in H. discoidea (de Beer and Larkum, 2001). Altogether, our results show a highly diverse and dynamic pattern regarding the expression of calcium transporters (Ca-channel, Ca-ATPase), which seems to be related to the species, but based on the intra-specific comparison of Phymatolithon sp., can also be modulated by the environment (Figure 8). It agrees with the reported high variability in the presence/absence of gene sequences associated to calcium transporters, such as Ca-ATPase and Ca2+/H+ exchangers in Rhodophyta (Schönknecht, 2013; Tong et al., 2021).
Our study shows that rhodolith species, and most likely coralline algae in general, display a large variability in (a) their general physiology and associated mechanisms, and (b) their maximum physiological performance. The former has implications for potential differences in the response to climate-change associated factors, such as ocean acidification. Large variability in the photosynthesis-calcification relationships and calcification mechanism suggests species’ differences in their capacity to maintain a high pH-environment at the calcification site and, consequently, in their susceptibility to OA. Our findings did not identify a general pattern for all species, but rather a species-specific interplay of mechanisms, driven by species physiological and morphological traits, but also by intra-specific modulation of acclimation/adaptation to environmental conditions. Furthermore, the large variability in maximum physiological performance among rhodolith species, which is modulated by light and/or temperature, suggests differences in net productivity not only at the individual, but also scaled up rhodolith-bed community level at different latitudes.
The original contributions presented in the study are included in the article/Supplementary Material. Further inquiries can be directed to the corresponding author.
NS and JS developed the concept, including the method and approach to be used; NS, VS, PH, PN, CR, FO-F, FT, FE, KS, and JS conducted the sampling collections; NS, VS, and JS conducted the experiments, NS performed sample analysis, NS, FT, and VS performed the data analysis; VP and LG performed the molecular identification of the rhodolith species, NS wrote the original draft of the article, supported by all authors who contributed to the writing, reviewing, and editing of the paper. All authors have read and agreed to the published version of the manuscript.
This research was funded by the European Union’s Horizon 2020 research and innovation programs under the Marie Sklodowska-Curie Grant agreement No. 844703, the European Union’s Horizon 2020 research and innovation program under grant agreement No. 730984, ASSEMBLE Plus project (Transnational Access #11154) and the FCT - Foundation for Science and Technology through Stimulus of Scientific Employment, Individual Support (CEECIND; 3rd Edition 2020.01282.CEECIND). This study was also supported by the Portuguese national funds from FCT - Foundation for Science and Technology through projects UIDB/04326/2020, UIDP/04326/2020 and LA/P/0101/2020, and from the operational programs CRESC Algarve 2020 and COMPETE 2020 through project EMBRC.PT ALG-01-0145-FEDER-022121. PH was supported by grants from FINEP/Rede CLIMA (01.13.0353-00) and CNPq-Universal (426215/2016-8). VS was supported by the scholarship CNPq PIBIC 135940/2016-8. CR and PN were financially supported by the Oceanic Observatory of Madeira Project (M1420- 01-0145-FEDER-000001 - Observatório Oceânico da Madeira-OOM).
The authors declare that the research was conducted in the absence of any commercial or financial relationships that could be construed as a potential conflict of interest.
All claims expressed in this article are solely those of the authors and do not necessarily represent those of their affiliated organizations, or those of the publisher, the editors and the reviewers. Any product that may be evaluated in this article, or claim that may be made by its manufacturer, is not guaranteed or endorsed by the publisher.
NS thanks intern Marie Brock for her help with the alkalinity sample analysis, Maeve Edwards (National University of Galweay) for her support with the experiments in Ireland, and Miguel Rodrigues (DiveSpot) and the AguaViva team for their support with the fieldwork at Armação de Pêra (Portugal) and in Arvoredo (Brazil), respectively. The authors thank the Museu da Baleia da Madeira for the logistical support during the sampling efforts in Caniçal, the Instituto das Florestas e Conservação da Natureza for house assistance in Porto Santo Island, and Estação de Biologia Marinha do Funchal for all the logistical support during the Madeira Island field campaigns. In Canary Islands, rhodolith samples were collected under license n° SGPM/BDM/AUTSPP/5212019, provided by the General Sub-Directorate General for the Protection of the Sea (Ministery for the Ecological Transition and the Demographic Challenge- Spain). VP thanks Marie Marbaix (Museum national d´Histoire Naturelle) for their help with molecular work. Acquisition of part of the molecular data was carried out at the ‘Service de Systématique Moléculaire’, UMS 2700 2AD, MNHN, CNR. The authors are also thankful for the thorough revision and helpful comments by reviewers, which greatly improved the quality of the manuscript.
The Supplementary Material for this article can be found online at: https://www.frontiersin.org/articles/10.3389/fmars.2022.921639/full#supplementary-material
Adams L. A., Maneveldt G. W., Green A., Karenyi N., Parker D., Samaai T., et al. (2020). Rhodolith Bed Discovered Off the South African Coast. Diversity 12, 125. doi: 10.3390/d12040125
Adey W. H., Halfar J., Williams B. (2013). The Coralline Genus Clathromorphum Foslie Emend. Adey: Biological, Physiological, and Ecological Factors Controlling Carbonate Production in an Arctic-Subarctic Climate Archive. Smithsonian Contr. Mar.Sci. 40, 1–41.
Allemand D., Grillo M. C. (1992). Biocalcification Mechanism in Gorgonians: 45Ca Uptake and Deposition by the Mediterranean Red Coral Corallium Rubrum. J. Exp. Zool. 262 (3), 237–246. doi: 10.1002/jez.1402620302
Attard K. M., Stahl H., Kamenos N. A., Turner G., Burdett H. L., Glud R. N. (2015). Benthic Oxygen Exchange in a Live Coralline Algal Bed and an Adjacent Sandy Habitat: An Eddy Covariance Study. Mar. Ecol. Prog. Ser. 535, 99–115. doi: 10.3354/meps11413
Axelsson L., Ryberg H., Beer S. (1995). Two Modes of Bicarbonate Utilization in the Marine Green Macroalga Ulva Lactuca. Plant Cell Environ. 18, 439–445. doi: 10.1111/j.1365-3040.1995.tb00378.x
Beardall J., Giordani M. (2002). Ecological Implications of Microalgal and Cyanobacterial CO2 Concentrating Mechanisms, and Their Regulation. Funct. Plant Biol. 29, 335–347. doi: 10.1071/PP01195
Beardall J., Johnston A., Raven J. (1998). Environmental Regulation of CO2-Concentrating Mechanisms in Microalgae. Can. J. Bot. 76, 1010–1017. doi: 10.1139/b98-079
Bergstrom E., Ordoñez A., Ho M., Hurd C. L., Fry B., Diaz-Pulido G. (2020). Inorganic Carbon Uptake Strategies in Coralline Algae: Plasticity Across Evolutionary Lineages Under Ocean Acidification and Warming. Mar. Environ. Res. 161, 105107. doi: 10.1016/j.marenvres.2020.105107
Berman-Frank I., Erez J., Kaplan A. (1998). Changes in Inorganic Carbon Uptake During the Progression of a Dinoflagellate Bloom in a Lake Ecosystem. Can. J. Bot. 76 (6), 1043–1051. doi: 10.1139/b98-075
Berman-Frank I., Kaplan A., Zohary T., Dubinsky Z. (1995). Carbonic Anhydrase Activity in the Bloom-Forming Dinoflagellate Peridinium Gatunense. J. Phycol. 31 (6), 906–913. doi: 10.1111/j.0022-3646.1995.00906.x
Borowitzka M. A. (1981). Photosynthesis and Calcification in the Articulated Coralline Red Algae Amphiroa Anceps and A. Foliacea. Mar. Biol. 62, 17–23. doi: 10.1007/BF00396947
Borowitzka M. A. (1987). Calcification in Algae: Mechanisms and the Role of Metabolism. CRC Crit. Rev. Plant Sci. 6, 1–45. doi: 10.1080/07352688709382246
Bracchi V. A., Angeletti L., Marchese F., Taviani M., Cardone F., Hajdas I., et al. (2019). A Resilient Deep-Water Rhodolith Bed Off the Egadi Archipelago (Mediterranean Sea) and its Actuopaleontological Significance. Alp. Medit. Quat. 32 (2), 131–150. doi: 10.26382/AMQ.2019.09
Bradshaw A. L., Brewer P. G., Sharer D. K., Williams R. T. (1981). Measurements of Total Carbon Dioxide and Alkalinity by Potentiometric Titration in the GEOSECS Program. Earth Planet. Sci. Lett. 55, 99–115. doi: 10.1016/0012-821X(81)90090-X
Büdenbender J., Riebesell U., Form A. (2011). Calcification of the Arctic Coralline Red Algae Lithothamnion Glaciale in Response to Elevated CO2. Mar. Ecol. Prog. Ser. 441, 79–87. doi: 10.3354/meps09405
Burdett H. L., Perna G., McKay L., Broomhead G., Kamenos N. A. (2018). Community-Level Sensitivity of a Calcifying Ecosystem to Acute in Situ CO2 Enrichment. Mar. Ecol. Prog. Ser. 587, 73–80. doi: 10.3354/meps12421
Carro B., Lopez L., Peña V., Bárbara I., Barreiro R. (2014). DNA Barcoding Allows the Accurate Assessment of European Maerl Diversity: A Proof-Of-Concept Study. Phytotaxa 190 (1), 176–189. doi: 10.11646/phytotaxa.190.1.12
Carvalho V. F., Assis J., Serrão E. A., Nunes J. M., Batista A. A., Batista M. B., et al. (2020). Environmental Drivers of Rhodolith Beds and Epiphytes Community Along the South Western Atlantic Coast. Mar. Environ. Res. 154, 104827. doi: 10.1016/j.marenvres.2019.104827
Chisholm J. R. W. (2000). Calcification by Crustose Algae on the Northern Great Barrier Reef, Australia. Limnol. Oceanogr. 45, 1476–1484. doi: 10.4319/lo.2000.45.7.1476
Chisholm J. R. W. (2003). Primary Productivity of Reef-Building Crustose Coralline Algae. Limnol. Oceanogr. 48, 1376–1387. doi: 10.4319/lo.2003.48.4.1376
Comeau S., Carpenter R. C., Edmunds P. J. (2013). Coral Reef Calcifiers Buffer Their Response to Ocean Acidification Using Both Bicarbonate and Carbonate. Proc. R. Soc London Ser. B 280 (1753), 20122374. doi: 10.1098/rspb.2012.2374
Comeau S., Cornwall C. E., DeCarlo T. M., Doo S. S., Carpenter R. C., McCulloch M. T. (2019b). Resistance to Ocean Acidification in Coral Reef Taxa is Not Gained by Acclimatization. Nat. Climate Change 9, 477–483. doi: 10.1038/s41558-019-0486-9
Comeau S., Cornwall C. E., De Carlo T. M., Krieger E., McCulloch M. (2018). Similar Controls on Calcification Under Ocean Acidification Across Unrelated Coral Reef Taxa. Global Change Biol. 24, 4857–4868. doi: 10.1111/gcb.14379
Comeau S., Cornwall C. E., Pupier C. A., DeCarlo T. M., Alessi C., Trehern R., et al. (2019a). Flow-Driven Micro-Scale pH Variability Affects the Physiology of Corals and Coralline Algae Under Ocean Acidification. Sci. Rep. 9, 12829. doi: 10.1038/s41598-019-49044-w
Cornwall C. E., Boyd P. W., McGraw C. M., Hepburn C. D., Pilditch C. A., Morris J. N., et al. (2014). Diffusion Boundary Layers Ameliorate the Negative Effects of Ocean Acidification on the Temperate Coralline Macroalga Arthrocardia Corymbosa. PLoS One 9, e97235. doi: 10.1371/journal.pone.0097235
Cornwall C. E., Comeau S., DeCarlo T. M., Moore B., D'alexis Q., McCulloch M. T. (2018). Resistance of Corals and Coralline Algae to Ocean Acidification: Physiological Control of Calcification Under Natural pH Variability. Proc. R. Soc B 285 (1884), 20181168. doi: 10.1098/rspb.2018.1168
Cornwall C. E., Comeau S., McCulloch M. T. (2017). Coralline Algae Elevate pH at the Site of Calcification Under Ocean Acidification. Global Change Biol. 23, 4245–4256.
Cornwall C. E., Hepburn C. D., Pilditch C. A., Hurd C. L. (2013). Concentration Boundary Layers Around Complex Assemblages of Macroalgae: Implications for the Effects of Ocean Acidification on Understory Coralline Algae. Limnol. Oceanogr. 58, 121–130. doi: 10.4319/lo.2013.58.1.0121
Cornwall C. E., Revill A. T., Hurd C. L. (2015). High Prevalence of Diffusive Uptake of CO2 by Macroalgae in a Temperate Subtidal Ecosystem. Photosynth. Res. 124 (2), 181–190. doi: 10.1007/s11120-015-0114-0
de Beer D., Larkum A. (2001). Photosynthesis and Calcification in the Calcifying Algae Halimeda Discoidea Studied With Microsensors. Plant Cell Environ. 24, 1209–1217. doi: 10.1046/J.1365-3040.2001.00772.X
Diaz-Pulido G., Cornwall C. E., Gartrell P., Hurd C. L., Tran D. V. (2016). Strategies of Dissolved Inorganic Carbon Use in Macroalgae Across a Gradient of Terrestrial Influence: Implications for the Great Barrier Reef in the Context of Ocean Acidification. Coral Reefs 35, 1327–1341. doi: 10.1007/s00338-016-1481-5
Digby P. S. B. (1977). Photosynthesis and Respiration in the Coralline Algae, Clathromorphum Circumscriptum and Corallina Officinalis and the Metabolic Basis of Calcification. J. Mar. Biol. Ass. U.K. 57, 1111–1124. doi: 10.1017/S0025315400026163
Drechsler Z., Beer S. (1991). Utilization of Inorganic Carbon by Ulva Lactuca. Plant Physiol. 97, 1439–1444. doi: 10.1104/pp.97.4.1439
Farias J. N., Riosmena-Rodríguez R., Bouzon Z., Oliveira E. C., Horta P. A. (2010). Lithothamnion Superpositum (Corallinales; Rhodophyta): First Description for the Western Atlantic or Rediscovery of a Species? Phycol. Res. 58, 210–216. doi: 10.1111/j.1440-1835.2010.00581.x
Figueiredo M. A. O., Coutinho R., Villas-Boas A. B., Tâmega F. T. S., Mariath R. (2012). Deep-Water Rhodolith Productivity and Growth in the Southeastern Atlantic. J. Appl. Ecol. 24, 487–493. doi: 10.1007/s10811-012-9802-8
Foster M. S. (2001). Rhodoliths: Between Rocks and Soft Places. J. Phycol. 37 (5), 659–667. doi: 10.1046/j.1529-8817.2001.00195.x
Fragkopoulou E., Serrão E. A., Horta P. A., Koerich G., Assis J. (2021). Bottom Trawling Threatens Future Climate Refugia of Rhodoliths Globally. Front. Mar. Sci. 7. doi: 10.3389/fmars.2020.594537
Giordano M., Beardall J., Raven J. A. (2005). CO2 concentrating mechanisms in algae: mechanisms, environmental modulation, and evolution. Ann. Rev. Plant Biol. 56(1), 99–131. doi: 10.1146/annurev.arplant.56.032604.144052
Gouy M., Guindon S., Gascuel O. (2010). SeaView Version 4: A Multiplatform Graphical User Interface for Sequence Alignment and Phylogenetic Tree Building. Mol. Biol. Evol. 27, 221–224. doi: 10.1093/molbev/msp259
Hansson I., Jagner D. (1973). Evaluation of the Accuracy of Gran Plots by Means of Computer Calculations: Application to the Potentiometric Titration of the Total Alkalinity and Carbonate Content in Sea Water. Anal. Chim. Acta 75, 363–373. doi: 10.1016/S0003-2670(01)82503-4
Harvey A. S., Harvey R. M., Merton E. (2017). The Distribution, Significance and Vulnerability of Australian Rhodolith Beds: A Review. Mar. Freshw. Res. 68, 411–428. doi: 10.1071/MF15434
Hofmann L. C., Heesch S. (2018). Latitudinal Trends in Stable Isotope Signatures and Carbon-Concentrating Mechanisms of Northeast Atlantic Rhodoliths. Biogeosciences 15, 6139–6149. doi: 10.5194/bg-15-6139-2018
Hofmann L. C., Koch M., de Beer D. (2016). Biotic Control of Surface pH and Evidence of Light-Induced H+ Pumping and Ca2+-H+ Exchange in a Tropical Crustose Coralline Alga. PLoS One 11, e0159057. doi: 10.1371/journal.pone.0159057
Hofmann L. C., Schoenrock K. M., de Beer D. (2018). Arctic Coralline Algae Elevate Surface pH Annd Carbonate in the Dark. Front. Plant Sci. 9. doi: 10.3389/fpls.2018.01416
Hurd C. L., Cornwall C. E., Currie K. I., Hepburn C. D., McGraw C. M., Hunter K. A., et al. (2011). Metabolically Induced pH Fluctuations by Some Coastal Calcifiers Exceed Projected 22nd Century Ocean Acidification: A Mechanism for Differential Susceptibility. Global Change Biol. 17, 3254–3262. doi: 10.1111/j.1365-2486.2011.02473.x
Ip Y. K., Lim A. L. L., Lim R. W. L. (1991). Some Properties of Calcium-Activated Adenosine Triphosphatase From the Hermatypic Coral Galaxea Fascicularis. Mar. Biol. 111 (2), 191–197. doi: 10.1007/BF01319700
Jeong J. B., Kim S. Y., Seo Y. K., Kim J.-K., Shin J., Woo K. S. (2019). Influence of Submarine Topography and Associated Sedimentary Processes on the Distribution of Live and Dead Rhodoliths Near Udo Island, Korea. Geo-Marine Lett. 40 (1), 35–51. doi: 10.1007/s00367-019-00623-w
Johnston A. M. (1991). The Acquisition of Inorganic Carbon by Marine Macroalgae. Can. J. Bot. 69, 1123–1132. doi: 10.1139/b91-144
Kamenos N. A., Burdett H. L., Aloisio E., Findlay H. S., Martin S., Longbone C., et al. (2013). Coralline Algal Structure is More Sensitive to Rate, Rather Than the Magnitude, of Ocean Acidification. Global Change Biol. 19, 3621–3628. doi: 10.1111/gcb.12351
Kim J.-H., Steller D. L., Edwards M. S. (2020). Variation in Photosynthetic Performance Relative to Thallus Microhabitat Heterogeneity in Lithothamnion Australe (Rhodophyta, Corallinales) Rhodoliths. J. Phycol. 57 (1), 234.244. doi: 10.1111/jpy.13080
King R. J., Schramm W. (1982). Calcification in the Maerl Coralline Alga Phymatolithon Calcareum: Effects of Salinity and Temperature. Mar. Biol. 70, 197–204.
Kingsley R. J., Norimitsu W. (1985). Ca-ATPase Localization and Inhibition in the Gorgonian Leptogorgia Virgulata (Lamarck)(Coelenterata: Gorgonacea). J. Exp. Mar. Biol. Ecol. 93 (1-2), 157–167. doi: 10.1016/0022-0981(85)90156-X
Koch M., Bowes G., Ross C., Zhang X.-H. (2013). Climate Change and Ocean Acidification Effects on Seagrasses and Marine Macroalgae. Global Change Biol. 19, 103–132. doi: 10.1111/j.1365-2486.2012.02791.x
Legrand E., Kutti T., Casal E. V. G., Rastrick S. P., Andersen S., Husa V. (2021). Reduced Physiological Performance in a Free-Living Coralline Alga Induced by Salmon Faeces Deposition. Aquacult. Environ. Interact. 13, 225–236. doi: 10.3354/aei00403
Legrand E., Riera P., Lutier M., Coudret J., Grall J., Martin S. (2017). Species Interactions can Shift the Response of a Maerl Bed Community to Ocean Acidification and Warming. Biogeosciences 14, 5359–5376. doi: 10.5194/bg-14-5359-2017
Legrand E., Riera P., Lutier M., Coudret J., Grall J., Martin S. (2019). Grazers Increase the Sensitivity of Coralline Algae to Ocean Acidification and Warming. J. Sea Res. 148-149, 1–7. doi: 10.1016/j.seares.2019.03.001
Littler M. M., Littler D. S., Hanisack M. D. (1991). Deep-Water Rhodolith Distribution, Productivity, and Growth History at Sites of Formation and Subsequent Degradation. J. Exp. Mar. Biol. Ecol. 150, 163–182. doi: 10.1016/0022-0981(91)90066-6
MAArE (2017). Projeto De Monitoramento Ambiental Da Reserva Biológica Marinha do Arvoredo E Entorno (Florianópolis, Brazil: ICMBio/UFSC).
Maberly S. C. (1990). Exogenous Sources for Inorganic Carbon for Photosynthesis by Marine Macroalgae. J. Phycol. 26, 439–449. doi: 10.1111/j.0022-3646.1990.00439.x
Maberly S. C., Raven J. A., Johnston A. M. (1992). Discrimination Between 12C and 13C by Marine Plants. Oecologia 91, 481–492. doi: 10.1007/BF00650320
Marshall A. T., Clode P. I. (2003). Light-Regulated Ca2+ Uptake and O2 Secretion at the Surface of a Scleractinian Coral Galaxea Fascicularis. Comp. Biochem. Physiol. Part A 136, 417–426. doi: 10.1016/s1095-6433(03)00201-0
Martin S., Castets M. D., Clavier J. (2006). Primary Production, Respiration and Calcification of the Temperate Free-Living Coralline Alga Lithothamnion Corallioides. Aquat. Bot. 85 (2), 121–128. doi: 10.1016/j.aquabot.2006.02.005
Martin S., Charnoz A., Gattuso J.-P. (2013). Photosynthesis, Respiration and Calcification in the Mediterranean Crustose Coralline Alga Lithophyllum Cabiochae (Corallinales, Rhodophyta). Eur. J. Phycol. 48, 163–172. doi: 10.1080/09670262.2013.786790
Martin S., Clavier J., Chauvaud L., Thouzean G. (2007b). Community Metabolism in Temperate Maerl Beds. II. Nutrient Fluxes. Mar. Ecol. Prog. Ser. 335, 31–41. doi: 10.3354/meps335019
Martin S., Clavier J., Chauvaud L., Thouzeau G. (2007a). Community Metabolism in Temperate Maerl Beds. I. Carbon and Carbonate Fluxes. Mar. Ecol. Prog. Ser. 335, 19–29. doi: 10.3354/meps335019
Martin S., Clavier J., Guarini J.-M., Chauvaud L., Hily C., Grall J., et al. (2005). Comparison of Zostera Marina and Maerl Community Metabolism. Aquat. Bot. 83, 161–174. doi: 10.1016/j.aquabot.2005.06.002
Martin S., Hall-Spencer J. M. (2017). “Effects of Ocean Warming and Acidification on Rhodolith/Maërl Beds,” in Rhodolith/maërl Beds: A Global Perspective. Eds. Riosmena-Rodríguez R., Nelson W., Aguirre J. (Berlin:Springer), 55–85. doi: 10.1007/978-3-319-29315-8_3
McConnaughey T. (1989). “Biomineralization Mechanisms,” in Origin, Evolution, and Modern Aspects of Biomineralization in Plants and Animals. Ed. Crick R. E. (New York:Springer), 57–73. doi: 10.1007/978-1-4757-6114-6_5
McConnaughey T. A., Falk R. H. (1991). Calcium-Proton Exchange During Algal Calcification. Biol. Bull. 180, 185–195. doi: 10.2307/1542440
McConnaughey T. A., Whelan J. F. (1997). Calcification Generates Protons for Nutrient and Bicarbonate Uptake. Earth-Science Rev. 42 (1-2), 95–117. doi: 10.1016/S0012-8252(96)00036-0
McKinney W. (2011). Pandas: A Foundational Python Library for Data Analysis and Statistics. Python High Perform. Sci. computing 14 (9), 1–9.
McNicholl C., Koch M. S., Hofmann L. C. (2019). Photosynthesis and Light-Dependent Proton Pumps Increase Boundary Layer pH in Tropical Macroalgae: A Proposed Mechanism to Sustain Calcification Under Ocean Acidification. J. Exp. Mar. Biol. Ecol. 521, 151208. doi: 10.1016/j.jembe.2019.151208
Mercado J. M., Carmen B., Pérez-Lloréns J. L., Vergara J. J. (2009). Carbon Isotopic Fractionation in Macroalgae From Cádiz Bay (Southern Spain): Comparison With Other Bio-Geographic Regions. Est. Coast. Shelf Sci. 85 (3), 449–458. doi: 10.1016/j.ecss.2009.09.005
Mori I. C., Sato G., Okazaki M. (1996). Ca2+-Dependent ATPase Associated With Plasma Membrane From a Calcareous Alga, Serraticardia Maxima (Corallinaceae, Rhodophyta). Phycol. Res. 44, 193–202. doi: 10.1111/j.1440-1835.1996.tb00049.x
Narvarte B. C. V., Nelson W. A., Roleda M. Y. (2020). Inorganic Carbon Utilization of Tropical Calcifying Macroalgae and the Impacts of Intensive Mariculture-Derived Coastal Acidification on the Physiological Performance of the Rhodolith. Sporolithon sp. Environ. Poll. 266, 115344. doi: 10.1016/j.envpol.2020.115344
Nash M. C., Diaz-Pulido G., Harvey A. S., Adey W. (2019). Coralline Algal Calcification: A Morphological and Process-Based Understanding. PLoS One 14 (9), e0221396. doi: 10.1371/journal.pone.0221396
Neves P., Silva J., Peña V., Ribeiro C. (2021). “Pink Round Stones”—Rhodolith Beds: An Overlooked Habitat in Madeira Archipelago. Biodiv. Conserv. 30 (12), 3359–3383. doi: 10.1007/s10531-021-02251-2
Noisette F., Duong G., Six C., Davoult D., Martin S. (2013a). Effects of Elevated Pco2 on the Metabolism of a Temperate Rhodolith Lithothamnion Corallioides Grown Under Different Temperatures. J. Phycol. 49, 746–757. doi: 10.1016/j.jembe.2013.07.006
Noisette F., Egilsdottir H., Davoult D., Martin S. (2013b). Physiological Responses of Three Temperate Coralline Algae From Contrasting Habitats to Near-Future Ocean Acidification. J. Exp. Mar. Biol. Ecol. 448, 179–187.
Okazaki M. (1977). Some Enzymatic Properties of Ca2+-Dependent Adenosine Triphosphatase From a Calcareous Red Alga, Serraticardia Maxima and its Distribution in Marine Algae. Bot. Mar. 20, 347–354. doi: 10.1515/botm.1977.20.6.347
Okazaki M., Fujii M., Usuda Y., Furuya K. (1984). Soluble Ca2+-Activated ATPase and its Possible Role in Calcification of the Coccolithophorid Cricosphaera Roscoffensis Var. Haptonemofera (Haptophyta) (Studies on the Calcium Carbonate Deposition of Algae V). Bot. Mar. 27, 363–369. doi: 10.1515/botm.1984.27.8.363
Otero-Ferrer F., Cosme M., Tuya F., Espino F., Haroun R. (2020). Effect of Depth and Seasonality on the Functioning of Rhodolith Seabeds. Est. Coast. Shelf Sci. 235, 106579. doi: 10.1016/j.ecss.2019.106579
Pardo C., Lopez L., Peña V., Hernández-Kantún J., Le Gall L., Bárbara I., et al. (2014). A Multilocus Species Delimitation Reveals a Striking Number of Species of Coralline Algae Forming Maerl in the OSPAR Maritime Area. PLoS One 9 (8), e104073. doi: 10.1371/journal.pone.0104073
Payri C. E. (1997). “Hydrolithon Reinboldii Rhodolith Distribution, Growth and Carbon Production of a French Polynesian Reef,” in Proc. 8th Int. Coral Reef Symp, Vol. 1. 755–760. (Balboa, Panama:Smithsonian Tropical Research.
Peña V., De Clerck O., Afonso-Carrillo J., Ballesteros E., Bárbara I., Barreiro R., et al. (2015b). An Integrative Systematic Approach to Species Diversity and Distribution in the Genus Mesophyllum (Corallinales, Rhodophyta) in Atlantic and Mediterranean Europe. Eur. J. Phycol. 50, 20–36. doi: 10.1080/09670262.2014.981294
Peña V., Pardo C., López L., Carro B., Hernandez-Kantun J., Adey W. H., et al. (2015a). Phymatolithon Lusitanicum Sp. Nov. (Hapalidiales, Rhodophyta): The Third Most Abundant Maerl-Forming Species in the Atlantic Iberian Peninsula. Cryptogamie Algol. 36 (4), 429–459. doi: 10.7872/crya/v36.iss4.2015.429
Pentecost A. (1978). Calcification and Photosynthesis in Corallina Officinalis L. Using the 14CO2 Method. Br. Phycol. J. 13, 383–390. doi: 10.1080/00071617800650431
Qui-Minet Z. M., Coudret J., Davoult D., Grall J., Mendez-Sandin M., Cariou T., et al. (2019). Combined Effects of Global Climate Change and Nutrient Enrichment on the Physiology of Three Temperate Maerl Species. Ecol. Evol. 9, 13787–13807. doi: 10.1002/ece3.5802
Qui-Minet Z. N., Davoult D., Grall J., Delaunay C., Six C., Cariou T., et al. (2021). Physiology of Maerl Algae: Comparison of Inter- and Intraspecies Variations. J. Phycol. 57 (3), 831–848. doi: 10.1111/jpy.13119
Ratnasingham S., Hebert P. D. N. (2007). The Barcode of Life Data System (Www.Barcodinglife.Org). Mol. Ecol. Notes 7, 355–364. doi: 10.1111/j.1471-8286.2007.01678
Rebelo A. C., Johnson M. E., Quartau R., Rasser M. W., Melo C. S., Neto A. I., et al. (2018). Modern Rhodoliths From the Insular Shelf of Pico in the Azores (Northeast Atlantic Ocean). Est. Coast. Shelf Sci. 210, 7–17. doi: 10.1016/j.ecss.2018.05.029
Rebelo A. C., Johnson M. E., Rasser M. W., Silva L., Melo C. S., Ávila S. P. (2021). Global Biodiversity and Biogeography of Rhodolith-Forming Species. Front. Biogeography 13 (1), e50646. doi: 10.21425/F5FBG50646
Reid R. J., Smith F. A. (1992). Regulation of Calcium Influx in Chara: Effects of K+, Ph, Metabolic Inhibition, and Calcium Channel Blockers. Plant Physiol. 100 (2), 637–643. doi: 10.1104/pp.100.2.637
Reynier M. V., Tâmega F. T. S., Daflon S. D. A., Santos M. A. B., Coutinho R., Figueiredo M. A. O. (2015). Long- and Short-Term Effects of Smothering and Burial by Drill Cuttings on Calcareous Algae in a Static-Renewal Test. Environ. Toxicol. Chem. 34, 1572–1577. doi: 10.1002/etc.2938
Ribeiro C., Neves P. (2020). Habitat Mapping of Cabo Girão Marine Park (Madeira Island): A Tool for Conservation and Management. J. Coast. Conserv. 24, 22. doi: 10.1007/s11852-019-00724-9
Riosmena-Rodríguez R., Nelson W., Aguirre J. (2017). Rhodolith/maërl Beds: A Global Perspective (Switzerland: Springer International Publishing). doi: 10.1007/978-3-319-29315-8
Saunders G. W. (2005). Applying DNA Barcoding to Red Macroalgae: A Preliminary Appraisal Holds Promise for Future Applications. Phil. Trans. R. Soc B 360, 1879–1888. doi: 10.1098/rstb.2005.1719
Schoenrock K. M., Bacquet M., Pearce D., Rea B. R., Schofield J. E., Lea J., et al. (2018). Influences of Salinity on the Physiology and Distribution of the Arctic Coralline Algae, Lithothamnion Glaciale (Corallinales, Rhodophyta). J. Phycol. 54, 690–702. doi: 10.1111/jpy.12774
Schönknecht G. (2013). Calcium Signals From the Vacuole. Plants 2 (4), 589–614. doi: 10.3390/plants2040589
Schubert N., Hofmann L. C., Almeida Saá A. C., Moreira A. C., Arenhart R. G., Fernandes C. P., et al. (2021a). Calcification in Free-Living Coralline Algae is Strongly Influenced by Morphology: Implications for Susceptibility to Ocean Acidification. Sci. Rep. 11, 11232. doi: 10.1038/s41598-021-90632-6
Schubert N., Salazar V. W., Rich W. A., Vivanco Bercovich M., Almeida Saá A. C., Fadigas S. D., et al. (2019). Rhodolith Primary and Carbonate Production in a Changing Ocean: The Interplay of Warming and Nutrients. Sci. Total Environ. 676, 455–468. doi: 10.1016/j.scitotenv.2019.04.280
Schubert N., Santos R., Silva J. (2021b). Living in a Fluctuating Environment Increases Tolerance to Marine Heatwaves in the Free-Living Coralline Alga Phymatolithon Lusitanicum. Front. Mar. Sci. 8. doi: 10.3389/fmars.2021.791422
Semesi I. S., Beer S., Björk M. (2009). Seagrass Photosynthesis Controls Rates of Calcification and Photosynthesis of Calcareous Macroalgae in a Tropical Seagrass Meadow. Mar. Ecol. Prog. Ser. 382, 41–47. doi: 10.3354/MEPS07973
Simon-Nutbrown C., Hollingsworth P. M., Fernandes T. F., Kamphausen L., Baxter J. M., Burdett H. L. (2020). Species Distribution Modeling Predicts Significant Declines in Coralline Algae Populations Under Projected Climate Change With Implications for Conservation Policy. Front. Mar. Sci. 7. doi: 10.3389/fmars.2020.575825
Sissini M. N., Koerich G., de Barros-Barreto M. B., Coutinho L. M., Gomes F. P., Oliveira W, et al. (2021). Diversity, Distribution, and Environmental Drivers of Coralline Red Algae: The Major Reef Builders in the Southwestern Atlantic. Coral Reefs. doi: 10.1007/s00338-021-02171-1
Sissini M. N., Oliveira M. C., Gabrielson P. W., Robinson N. M., Okolodkov Y. B., Riosmena-Rodríguez R., et al. (2014). Mesophyllum Erubescens (Corallinales, Rhodophyta)- So Many Species in One Epithet. Phytotaxa 190, 299–319. doi: 10.11646/phytotaxa.190.1.18
Sordo L., Santos R., Barrote I., Freitas C., Silva J. (2020). Seasonal Photosynthesis, Respiration and Calcification of a Temperate Maërl Bed in Southern Portugal. Front. Mar. Sci. 7. doi: 10.3389/fmars.2020.00136
Sordo L., Santos R., Barrote I., Silva J. (2018). High CO2 Decreases the Long-Term Resilience of the Free-Living Coralline Algae Phymatolithon Lusitanicum. Ecol. Evol. 8, 4781–4792. doi: 10.1002/ece3.4020
Sordo L., Santos R., Barrote I., Silva J. (2019). Temperature Amplifies the Effect of High CO2 on the Photosynthesis, Respiration, and Calcification of the Coralline Algae Phymatolithon Lusitanicum. Ecol. Evol. 9 (19), 11000–11009. doi: 10.1002/ece3.5560
Sordo L., Santos R., Reis J., Shulika A., Silva J. (2016). A Direct CO2 Control System for Ocean Acidification Experiments: Testing Effects on the Coralline Red Algae Phymatolithon Lusitanicum. Peer J. 4, e2503. doi: 10.7717/peerj.2503
Sreeraj C. R., Abhilash K. R., Deepak Samuel V., Krishnan P., Purvaja R., Ramesh R. (2018). Occurrence of Live Rhodolith Bed of Lithophyllum Kotschyanum Unger (Corallinaceae: Lithophylloidae) in Palk Bay: First Record From India. Curr. Sci. 114, 1–3. doi: 10.18520/cs%2Fv114%2Fi03%2F445-446
Steller D. L., Hernández-Ayón J. M., Riosmena-Rodríguez R., Cabello-Pasini A. (2007). Effect of Temperature on Photosynthesis, Growth and Calcification Rates of the Free-Living Coralline Alga Lithophyllum Margaritae. Cienc. Mar. 33 (4), 441–456. doi: 10.7773/cm.v33i4.1255
Stento N. A., Ryba N. G., Kiegle E. A., Bisson M. A. (2000). Turgor Regulation in the Salt-Tolerant Alga Chara Longifolia. Plant Cell Environ. 23 (6), 629–637. doi: 10.1046/j.1365-3040.2000.00571.x
Smith S. V., Kinsey D. W (1996). "Calcification and organic carbon metabolism as indicated by carbon dioxide". In Coral Reefs: Research Methods, Monographs on Oceanographic Methodology Vol. 5 edsStoddart D. R., Johannes R. E. (Paris:UNESCO), 469–84
Tambutté É., Allemand D., Mueller E., Jaubert J. (1996). A Compartmental Approach to the Mechanism of Calcification in Hermatypic Corals. J. Exp. Biol. 199 (5), 1029–1041. doi: 10.1242/jeb.199.5.1029
Tamura K., Stecher G., Peterson D., Filipski A., Kumar S. (2013). MEGA6: Molecular Evolutionary Genetics Analysis Version 6.0. Mol. Biol. Evol. 30, 2725–2729. doi: 10.1093/molbev/mst197
Tong T., Li Q., Jiang W., Chen G., Xue D., Deng F., et al. (2021). Molecular Evolution of Calcium Signaling and Transport in Plant Adaptation to Abiotic Stress. Int. J. Mol. Sci. 22 (22), 12308. doi: 10.3390/ijms222212308
Triggle D. J. (2006). L-Type Calcium Channels. Curr. Pharm. Des. 12 (4), 443–457. doi: 10.2174/138161206775474503
Vásquez-Elizondo R. M., Enríquez S. (2016). Coralline Algal Physiology is More Adversely Affected by Elevated Temperature Than Reduced pH. Sci. Rep. 6 (1), 19030. doi: 10.1038/srep19030
Vieira-Pinto T., Oliveira M.C., Bouzon J., Sissini M., Richards J.L., Riosmena-Rodríguez R, et al (2014). Lithophyllum species from Brazilian coast: range extension of Lithophyllum margaritae and description of Lithophyllum atlanticum sp. nov. Corallinales, Corallinophycidae, Rhodophyta.Phytotaxa 190 (1), 355–69. doi: 10.11646/phytotaxa.190.1.21
Wang W.-L., Yeh H.-W. (2003). δ13C Values of Marine Macroalgae From Taiwan. Bot. Bull. Acad. Sin. 44, 107–112.
Ward D. H., Amundson C. L., Fitzmorris P. J., Menning D. M., Markis J. A., Sowl K. M., et al. (2021). Abundance of a Recently Discovered Alaskan Rhodolith Bed in a Shallow, Seagrass-Dominated Lagoon. Bot. Mar. 64 (2), 119–127. doi: 10.1515/bot-2020-0072
Waskom M., Botvinnik O., Ostblom J., Gelbart M., Lukauskas S., Hobson P., et al. (2020). Mwaskom/Seaborn: V0. 10.1. doi: 10.5281/zenodo.3767070
Watson E. L., Vincenz F. F., Davis P. W. (1971). Ca2+-Activated Membrane ATPase: Selective Inhibition by Ruthenium Red. Biochim. Biophys. Acta 249, 606–610. doi: 10.1016/0005-2736(71)90140-4
Yoon H. S., Hackett J. D., Bhattacharya D. (2002). A Single Origin of the Peridinin- and Fucoxanthin-Containing Plastids in Dinoflagellates Through Tertiary Endosymbiosis. Proc. Nat. Acad. Sci. U.S.A. 99, 11724–11729. doi: 10.1073/pnas.172234799
Keywords: calcification mechanism, carbon-concentrating mechanism (CCM), coralline algae (maërl), photosynthesis-calcification relationship, rhodolith beds
Citation: Schubert N, Peña V, Salazar VW, Horta PA, Neves P, Ribeiro C, Otero-Ferrer F, Tuya F, Espino F, Schoenrock K, Hofmann LC, Le Gall L, Santos R and Silva J (2022) Rhodolith Physiology Across the Atlantic: Towards a Better Mechanistic Understanding of Intra- and Interspecific Differences. Front. Mar. Sci. 9:921639. doi: 10.3389/fmars.2022.921639
Received: 16 April 2022; Accepted: 31 May 2022;
Published: 24 June 2022.
Edited by:
Bernardo Antonio Perez Da Gama, Fluminense Federal University, BrazilReviewed by:
Christopher Edward Cornwall, Victoria University of Wellington, New ZealandCopyright © 2022 Schubert, Peña, Salazar, Horta, Neves, Ribeiro, Otero-Ferrer, Tuya, Espino, Schoenrock, Hofmann, Le Gall, Santos and Silva. This is an open-access article distributed under the terms of the Creative Commons Attribution License (CC BY). The use, distribution or reproduction in other forums is permitted, provided the original author(s) and the copyright owner(s) are credited and that the original publication in this journal is cited, in accordance with accepted academic practice. No use, distribution or reproduction is permitted which does not comply with these terms.
*Correspondence: Nadine Schubert, bmFkaW5lX3NjaHViZXJ0QGhvdG1haWwuY29t
†Present Address: Vinícius W. Salazar, Melbourne Integrative Genomics, The University of Melbourne, Parkville, VIC, Australia
‡These authors have contributed equally to this work
Disclaimer: All claims expressed in this article are solely those of the authors and do not necessarily represent those of their affiliated organizations, or those of the publisher, the editors and the reviewers. Any product that may be evaluated in this article or claim that may be made by its manufacturer is not guaranteed or endorsed by the publisher.
Research integrity at Frontiers
Learn more about the work of our research integrity team to safeguard the quality of each article we publish.